- 1Centre for Tissue Engineering and Regenerative Medicine, Faculty of Medicine, Universiti Kebangsaan Malaysia, Kuala Lumpur, Malaysia
- 2Department of Surgery, Hospital Canselor Tuanku Muhriz, Universiti Kebangsaan Malaysia, Kuala Lumpur, Malaysia
Developments in tissue engineering techniques have allowed for the creation of biocompatible, non-immunogenic alternative vascular grafts through the decellularization of existing tissues. With an ever-growing number of patients requiring life-saving vascular bypass grafting surgeries, the production of functional small diameter decellularized vascular scaffolds has never been more important. However, current implementations of small diameter decellularized vascular grafts face numerous clinical challenges attributed to premature graft failure as a consequence of common failure mechanisms such as acute thrombogenesis and intimal hyperplasia resulting from insufficient endothelial coverage on the graft lumen. This review summarizes some of the surface modifying coating agents currently used to improve the re-endothelialization efficiency and endothelial cell persistence in decellularized vascular scaffolds that could be applied in producing a better patency small diameter vascular graft. A comprehensive search yielding 192 publications was conducted in the PubMed, Scopus, Web of Science, and Ovid electronic databases. Careful screening and removal of unrelated publications and duplicate entries resulted in a total of 16 publications, which were discussed in this review. Selected publications demonstrate that the utilization of surface coating agents can induce endothelial cell adhesion, migration, and proliferation therefore leads to increased re-endothelialization efficiency. Unfortunately, the large variance in methodologies complicates comparison of coating effects between studies. Thus far, coating decellularized tissue gave encouraging results. These developments in re-endothelialization could be incorporated in the fabrication of functional, off-the-shelf alternative small diameter vascular scaffolds.
Introduction
Cardiovascular diseases (CVD) describe a variety of linked pathologies affecting the heart and blood vessels and are often associated with ischemic tissue damage predominantly as a consequence of severe arterial occlusion resulting from common underlying conditions such as atherosclerosis (1). Accountable for approximately 17.9 million deaths every year with projected annual mortalities rising to a staggering 23.3 million by 2030, CVDs represent a significant public health concern and is currently the leading cause of mortality and morbidity around the globe (2, 3). Despite these fatality rates, rapid identification and rectification of modifiable atherosclerotic risk factors through the employment of noninvasive treatment options such as dietary and lifestyle modifications (e.g., lipid control, smoking cessation) and pharmaceutical therapies (e.g., statins) can retard the occlusive process which greatly reduces the risk of premature CVD (4, 5). Patients experiencing severe arterial occlusion, as defined by the presence of >50% stenosis in the left coronary artery or >70% stenosis in a major coronary vessel (6), may opt for invasive vascular procedures such as percutaneous coronary intervention (PCI) or coronary artery bypass grafting (CABG) to achieve revascularization in affected arteries over extended periods of time (7). CABG has been associated with improved survival rates and reduced recurrence of major cardiovascular events and compared to PCI, particularly in patients with multivessel occlusions, however, making it the preferable treatment option over PCI (8–10).
The saphenous vein represents the current bypass conduit of choice for CABG procedures despite exhibiting poor long-term patency and high graft failure rates (~50% failure after 10 years) compared to autologous arterial grafts such as the internal mammary artery due to its ease of harvest with minimal complications (11, 12). Nevertheless, the use of autologous vessels for vascular bypass grafting comes with its own set of issues—vessel removal could cause damage at the extraction site and the extracted vessel may be of poor quality, thus preventing it from being used as a bypass conduit (13). Developments in tissue engineering techniques have allowed for the circumvention of these limitations, however, as the decellularization technique can be utilized to fabricate alternative biocompatible vascular scaffolds derived either from allogeneic or xenogeneic sources (14). These decellularized scaffolds can be used as conduits for vascular bypass, eliminating the need for autograft surgery.
The decellularization process involves striking a fine balance between the targeted removal of cellular and nuclear material contained in existing vascular tissues whilst minimizing damage to the extracellular matrix (ECM) constituents (14). This can be achieved via treatment of vascular tissues with chemical agents such as detergents and alcohols, biological agents such as enzymes and chelating agents, and physical methods including freeze-thawing, hydrostatic pressure, and non-thermal irreversible electroporation (15). Successful decellularization allows vascular scaffolds to retain their biomechanical properties whilst reducing their immunogenicity (13, 14, 16). Inadequate cellular depopulation could lead to immune-mediated damage which could result in graft failure. Overly aggressive decellularization is also unfavorable as it potentially results in the elimination or disruption of critical ECM components, which would adversely affect the structural integrity and mechanical properties of the decellularized tissue (17–19). Decellularized vascular scaffolds have exhibited their capability of supporting the adhesion and development of endothelial cells (EC) and smooth muscle cells (SMC) in multiple studies (20–22), making the prospect of producing a non-immunogenic tissue-engineered alternative small diameter vascular graft very promising.
A number of commercially available decellularized vascular grafts derived from bovine blood vessels and bovine ureters have been utilized in bypass surgeries in the past, but wide-scale implementation and utilization of these grafts as bypass conduits have not occurred to date due to their poor clinical outcomes post-implantation in regard to low patency and premature graft failure as a consequence acute thrombogenicity and intimal hyperplasia (13, 23). Multiple studies have implied that the absence of a luminal EC layer in decellularized vascular grafts is responsible for the development of thrombosis and ultimately premature graft failure upon in vivo implantation (13, 24–29). This occurs as the presence of a viable luminal endothelium aids to prevent exposed collagen from triggering the extrinsic blood coagulation pathway when coming into contact with peripheral blood, therefore an absence of ECs would result in thrombosis (29, 30). Intimal hyperplasia also represents a significant factor adversely affecting the long-term patency of decellularized scaffolds (27, 31, 32), in which a thickening of the neovascular neointima is observed. Although the exact mechanism responsible for the development of intimal hyperplasia is not fully understood, excessive vascular SMC migration and proliferation from the vessel media to the intima along with excessive ECM protein deposition have previously been suggested to be the cause (33). The presence of a compliance mismatch between the vascular graft and its adjacent native vessel, and the absence of a functional endothelium are some additional causes of intimal hyperplasia that have been identified (34–36). Nevertheless, the risks of the aforementioned conditions can be mitigated through careful donor species, site, and tissue processing and antigen removal methodology selection alongside timely re-endothelialization with functional ECs (37, 38).
All tissue-engineered vascular grafts (TEVGs), including decellularized scaffolds, will encounter a certain degree of re-endothelialization post-transplantation. Current identified mechanisms reveal that a majority of the re-endothelialization processes required for the generation of an effective and persistent endothelium occurs spontaneously during in situ/in vivo regeneration, but in vitro re-endothelialization involving luminal cell seeding and maturation prior to graft implantation remains the most commonly used technique to achieve re-endothelialization in TEVGs to date (39). The aforementioned in situ re-endothelialization processes include host intima ingrowth from the anastomotic regions predominantly occurring in TEVGs less than 2 cm in length (trans-anastomotic ingrowth) (40, 41), migration of capillary endothelium from adventitial granulation tissue onto the intimal surface (trans-mural capillary ingrowth) (42, 43), and deposition of circulating endothelial progenitor cells (EPCs), a small subset of CD34+ mononuclear cells capable of differentiating into an EC-like phenotype whilst expressing EC-specific markers, through recognition of proteins adsorbed on the surface of graft (fallout endothelialization) (39, 44, 45). Upon successful re-endothelialization and cellular repopulation, Henry et al. observed graft remodeling with an influx of MHC+ SMCs localized within the graft wall, and extensive matrix deposition of the ECM components collagen and elastin with a high percentage of cellular infiltration into graft (46). An increase in collagen and elastin deposition would be beneficial for the mechanical properties of a TEVG as collagen density has been shown to directly correlate with strength and stiffness whilst elastin imparts extensibility (47, 48).
Nevertheless, achieving and maintaining a healthy and functional endothelial lining has proven to be tricky. Depending on the length and diameter of the scaffold in question, in situ re-endothelialization mechanisms may or may not suffice for the generation of fully patent vascular grafts. Vascular grafts with an inner diameter size larger than 6 mm have demonstrated excellent patency (93%) after a period of 5 years post-implantation without the need for active in vitro re-endothelialization (49, 50), but these results cannot be extrapolated to shorter grafts with smaller inner diameters (<4 mm diameter, <5 cm length) as these grafts are extremely prone to acute thrombotic obstruction post-implantation; a study revealed that 5 of 6 rats implanted with non-endothelialized decellularized rat abdominal aortas succumbing to endothelial damage and acute thrombosis after 3 days with a final graft patency of 50% after 14 days, while 4 out of 6 rats remained alive after 14 days of implanting the reendothelialized graft, retaining a graft patency of 63% (51). Despite achieving confluent in vitro endothelialization prior to implantation of the reendothelialized grafts, graft patency and rat survival rates were significantly lower than that of the control (undecellularized rat abdominal aorta; 100% patency and survival). This occurrence likely resulted from exposure to high shear stresses from the pulsatile blood flow present in the circulatory system; previous studies have reported a maximal cell loss of 70% in EC-seeded grafts within minutes of exposure to flow (52, 53).
As such, enhancing the efficiency of the re-endothelialization process and improving EC retention post-implantation proves to be critical for the development of functional small diameter decellularized vascular scaffolds. One approach that can be utilized to achieve these goals is through the functionalization of the surface of decellularized scaffolds through the application and integration of specific proteins, such as growth factors, as bioactive surface coatings or modifications. This approach has previously been shown to result in grafts with improved patency with lower risks of thrombosis and intimal hyperplasia (32, 54–56). In the case of the previously explained study conducted by Hsia et al., sphingosine-1-phosphate (S1P), which has been shown to possess antithrombotic and pro-angiogenic properties (57), was utilized as the bioactive coating of choice (51). The results of their study demonstrated that rats implanted with re-endothelialized rat abdominal aortas coated with S1P exhibited 100% survival and patency rates as a result of increased EC migration and adhesion strength (51). This rise in EC migration could possibly be attributed to the pro-angiogenic properties of the S1P coating, as endothelial cells have a propensity to proliferate toward angiogenic stimuli (39). In principle, a combination of in vitro cell seeding in the presence of a bioactive coating appears to be a promising strategy to enhance in situ endothelial regeneration, which is ideal to achieve a persistent and functional endothelium. Thus, this article aims to systematically review existing literature on various examples of surface modifications used improve the efficiency and efficacy of the re-endothelialization process for the production of functional decellularized vascular scaffolds.
Methods
This review was conducted in accordance with the guidelines stated in the Preferred Reporting Items for Systematic Reviews and Meta-Analyses (PRISMA) statement (58) to systematically assess publications in regard to the application and effects of coatings on the re-endothelialization of decellularized vascular scaffolds.
Electronic databases including PubMed, Scopus, Web of Science (WOS), and Ovid were utilized to acquire relevant primary studies, with results shown up to January 2021. The specific string used for all database searches was “((Decellularis* OR Decellulariz*) AND (Arter* OR Vein OR Vessel)) AND (coat*) AND (endotheliali* OR populat*) NOT (review)” to ensure the inclusion of studies involving the coating of decellularized vascular scaffolds and cellular repopulation or re-endothelialization whilst excluding review articles. Search results were screened based on title and only publications regarding relevant primary studies written in the English language were included. Publications qualifying through the preliminary title screening were subjected to abstract screening, and only articles with abstracts that illustrated the use of decellularized tissues or organs coated with bioactive molecules that have been shown to positively affect endothelial cell or endothelial progenitor cell recruitment/adherence/attachment/coverage or the re-endothelialization/cellular repopulation process were shortlisted for inclusion. Publications utilizing modifications that aim to improve the feasibility of decellularized grafts as potential cardiovascular therapeutics but do not affect the re-endothelialization process such as those enhancing graft mechanical properties or reducing intimal hyperplasia/thrombosis without enhancing endothelial coverage were deemed ineligible as the scope of this review is to enhance the re-endothelialization process. All eligibility assessments were conducted by two reviewers to reduce author/selection bias in the article selection process, and disagreements regarding the eligibility of included publications and/or data were resolved through discussion between reviewers. Publications meeting any of the following exclusion criteria were also eliminated from further review: non-English language articles, conference abstracts, news articles, letters, editorials, case studies, and review articles.
Duplicate entries were removed from the inclusion group, and a data extraction table (Table 1) was generated to summarize the following information from included publications: author, tissue decellularized, coating used, and findings.
Results
Search Results
Primary database search yielded a total of 192 results, of which 19 articles were derived from PubMed, 24 articles from Scopus, 28 articles from WoS, and 121 articles from Ovid. Independent screening of the initial results was conducted by two reviewers in accordance with the inclusion and exclusion criteria to reduce bias in the selection process, followed by a joint discussion which resulted in the unanimous decision to eliminate 135 unrelated articles and include 57 articles: 14 from PubMed, 14 from Scopus, 20 from WoS, and 9 from Ovid. Abstracts were carefully screened, and 20 articles were removed as they did not match the inclusion criteria, leaving a total of 37 articles in the inclusion group. Duplicate entries were screened for, and 21 articles were removed, resulting in a final tally of 16 publications, of which 11 were from PubMed, one from Scopus, one from WoS, and three from Ovid. A flow chart depicting the selection process is shown in Figure 1.
Study Characteristics
Decellularized biological scaffolds were utilized in all selected studies. However, the type of tissue decellularized and its origin differed. Decellularized scaffolds were modified through the addition of a coating agent prior to re-endothelialization in all included publications, but the coating agent utilized varied between each study. A summary of included publications is provided in Table 1.
Discussion
Sixteen publications were selected from a pool of 192 results as they utilized different decellularized vascular scaffolds coated with various substances to improve re-endothelialization. Information regarding the types of decellularized vascular scaffolds and coatings utilized in the studies are summarized in Table 1. Effects of the coating agents on the re-endothelialization of decellularized vascular grafts are further discussed.
Heparin
The sulphated polysaccharide heparin has been well-documented as a potent, endothelial cell-binding anticoagulant agent. Heparin impedes the development of thrombosis via inhibition of specific serine proteinases involved in the blood coagulation cascade through the potentiation of antithrombin III (75). Hussein et al. (59) established that the coating of the decellularized porcine liver right lobe with heparin-gelatin, significantly increases attachment of EA.hy926 endothelial cells on vascular surfaces whilst reducing endothelial cell migration into the parenchyma. 3-(4,5-dimethylthiazol-2-yl)-2,5-diphenyl tetrazolium bromide (MTT) assay results showed a 3.8-fold increase in cell attachment on heparin-gelatin coated samples compared to uncoated control samples. Heparin and gelatin coated samples yielded a smaller 2.1-fold and 2.5-fold increase, respectively. Increased EC attachment was observed in heparin-gelatin coated samples likely due to an increase presence of VEGF and bFGF, as these growth factors exhibit high affinity for heparin, and ECs have been shown to express receptors for these growth factors (59, 76–78).
Musilkova et al. (67) used a different approach for their study, where decellularized human pericardium (DP) was strongly or weakly crosslinked with glutaraldehyde and/or genipin prior to coating with a fibrin mesh modified with heparin, fibronectin, heparin and fibronectin, or unmodified. Genipin acts as a natural, low-toxic crosslinking agent with prior experiments demonstrating its ability to stabilize decellularized scaffolds with minimal cytotoxicity and immunogenicity as compared to glutaraldehyde-crosslinked decellularized scaffolds (79–82). Results from Musilkova et al.'s study indicated that glutaraldehyde crosslinking, irrespective of crosslinking strength, resulted in a reduction of seeded human umbilical vein endothelial cells (HUVEC) metabolic activity (MTA), while genipin crosslinking increased MTA across all DP samples. Highest MTA was produced in HUVECs seeded onto DP weakly crosslinked with genipin and coated with fibrin and fibronectin. MTA in samples coated with heparin-modified fibrin mesh and heparin and fibronectin-modified fibrin mesh were lower as compared to other treatments. Despite exhibiting lower MTA values, the anticoagulatory and anti-inflammatory effects of heparin-fibrin may be beneficial in inhibiting thrombosis prior to re-endothelialization (67, 83).
Heparin-treated decellularized porcine carotid arteries were coated with bFGF in Conklin et al.'s study (71) to analyze the impact of bFGF on the proliferation of human microvascular endothelial cells (HMEC) and canine endothelial progenitor cells (CEPC). The decellularized carotid arteries were pre-treated with heparin to enhance adhesion of bFGF as it is a heparin-binding growth factor that has been previously shown to enhance EC migration and proliferation, angiogenesis, and re-endothelialization (84–87). Experimental results shown that the coating improves in vitro proliferation rate of both HMECs and CEPCs, with bFGF-coated samples seeing a 2.4-fold increase in HMEC and 2.3-fold rise in CEPC cell quantities compared to uncoated samples after 4 days and 2 days, respectively. HMECs seeded on coated scaffolds were also found to be more resistant than uncoated samples when cultured under shear stresses (71).
VEGF
VEGF was also utilized in a number of studies as it functions as a powerful angiogenic and mitogenic agent that is capable of inducing EC migration and proliferation to accelerate the vascularization process when bound to its specific VEGF receptors (88–91). A study conducted using decellularized murine descending aorta coated with VEGF established that incorporation of the growth factor facilitated the re-endothelialization process and led to decreased development of neointimal lesions in the murine aortic graft (66). This occurrence is presumably a result of VEGF's capacity to trigger differentiation of lesional progenitor cells toward an endothelial lineage (66), as evidenced by the development of endothelial-specific markers (eNOS, vWF, CD31, CD144, and VEGFR 1/2) on lesional progenitor cells cultured in vitro in the presence of VEGF (66). The authors also demonstrated that these in vitro results were extrapolatable to in vivo subjects, as local application of VEGF onto the implanted vascular graft decreased neointimal development and promoted EC localization to surface of the graft (66).
Iijima et al. (68) explored the impacts of coating decellularized murine aorta with VEGF conjugated to a temperature-sensitive aliphatic polyester hydrogel (HG-VEGF) which allows for a steady and sustained release of the growth factor (92). They concluded that the sustained VEGF exposure enhanced EC adhesion to the basement membrane and promoted the formation of a functional endothelial layer, with HG-VEGF coated samples presenting 64.8 ± 7.6% EC coverage on their luminal surface 4-weeks post-treatment, while uncoated samples presented 40.4 ± 8.3% EC coverage. Medial repopulation was also increased in coated vessels, with the absolute cell count of coated samples at 4 weeks and 8 weeks being 7.3 ± 5.9 cells, and 22.1 ± 13.0 cells, respectively. Uncoated vessels had 0.80 ± 1.2 cells at 4 weeks and 3.2 ± 3.6 cells at 8 weeks. Despite the promising increase in re-endothelialization, use of the coating eventually resulted in neointimal hyperplasia, which led to a significantly increased intima-to-media ratio (68).
Marinval et al. (63) proposed the modification of decellularized porcine heart valve scaffolds via a multi-layer application of the brown algae-derived sulphated polysaccharide, fucoidan, and VEGF (63, 93). Multiple studies have shown that fucoidan promotes the adhesion, migration, and proliferation of endothelial cells, and has antithrombotic properties similar to that of heparin but carries a lower hemorrhagic risk than the former (93–96). The authors disclosed that the coating led to an improvement in HUVEC adhesion accompanied by enhanced cell density and viability on the decellularized valvular scaffold in both static and dynamic cultures. Visual examination of HUVEC re-endothelialization in static culture conditions showed endothelial cells presenting as a highly connective homogenous monolayer, with a larger number of adherent, living cells present 6-h post-treatment with fucoidan/VEGF compared to untreated samples. Similar evaluations conducted on HUVECs cultured under perfusion revealed ECs aligning toward the direction of perfusion with greater endothelial cell adhesion in coated samples. Cell viability under perfusion was also significantly enhanced, with coated samples showing 4,549 ± 325 viable cells per field, while uncoated samples showed 3,343 ± 292 cells per field. Fucoidan/VEGF coating can be used to enhance re-endothelialization whilst reducing the risk of thrombosis (63).
Human and rat kidneys were decellularized by Leuning and co-workers (74), and the effects of VEGF and Ang-1 coating on decellularized kidney scaffolds were investigated. Growth factor loading was determined to be an essential measure for maximal endothelial cell adherence, survival, and coverage, as samples coated with VEGF and Ang-1 exhibited increased EC adherence and viability. Human induced pluripotent stem cell-derived endothelial cells (hIPS-ECs) were also seeded onto the coated samples, and similar results were produced; VEGF and Ang-1 coating resulted in enhanced EC adherence and viability. hIPS-ECs were also seeded on decellularized human kidney, and the authors reported enhanced hIPS-EC proliferation in VEGF + Ang-1 coated samples with the potential to scale the re-endothelialization process throughout the entire kidney. Minimal vascular obstructions were also observed in coated samples, highlighting the importance of growth factor reconstitution for the re-endothelialization of decellularized kidney scaffolds (74).
Cellular Communication Network Factor 1 (CCN1)
CCN1 is a secreted surface-associated pro-angiogenic matricellular protein that is recognized to mediate cell adhesion and migration, and cell proliferation through integrin interaction and induction of growth factor-associated DNA synthesis respectively (97–100). CCN1 has also recently been implicated to be capable of inducing the recruitment and localization of circulating CD34+ progenitor cells to the endothelial layer, contributing to the regeneration of the endothelium due to their ability to differentiate into mature ECs (101–103). The recruited EPCs may also positively influence angiogenesis and neovascularization through the paracrine secretion of pro-angiogenic cytokines (104, 105).
The study conducted by Bär et al. (61) utilized human cord blood-derived endothelial cells (hCBEC) to repopulate decellularized porcine small intestines with preserved pedicles (BioVaM) coated with CCN1. hCBEC attachment experiments were conducted on plates coated with gelatin or CCN1-enriched gelatin. Results showed significantly improved hCBEC adhesion and retention when seeded on CCN1-enriched gelatin-coated plates compared to hCBEC seeded on plates coated with only gelatin. When hCBECs were seeded on the decellularized scaffolds, enhanced re-endothelialization efficiency was observed in CCN1-coated BioVaMs compared to their uncoated counterparts (84 ± 9% cell retention vs. 47 ± 4% cell retention). DNA content analyses conducted 12 h post-re-endothelialization revealed significantly higher DNA content in coated samples (37 ± 2 μg/g) compared to uncoated samples (11 ± 3 μg/g), clearly showcasing the effects of CCN1 coating on decellularized porcine small intestine scaffolds (61).
A similar study (65) conducted using decellularized equine carotid arteries coated with CCN1 observed that the CCN1 coating not only facilitates circulating endothelial cell attachment, but also induces endothelial and smooth muscle cell proliferation, neomedia formation, organized neovascularization, reduced local inflammatory reactions, and induced immunological tolerance which collectively enhances biocompatibility of decellularized vascular grafts significantly (65).
Fibronectin
Fibronectin (FN) represents a major ECM constituent that has the capacity to induce endothelial cell adhesion, migration, and differentiation through conjugation or adhesion with biomaterial surfaces (106). Therefore, Assmann et al. (70) and Flameng et al. (72) conducted studies utilizing FN as a coating for their decellularized vascular scaffolds to mimic what has been done in vitro to assess FN applicability in 3D.
Assmann et al. (70) investigated the effects of FN on the autologous in vivo re-endothelialization of decellularized murine aortic conduits and concluded that FN greatly enhanced EC adhesion capacity and decellularized graft biocompatibility, resulting in accelerated re-endothelialization. Examination of the luminal surface 8-weeks post-treatment showed 89.9 ± 5.45% repopulation on the luminal surface of the coated samples, while uncoated samples displayed 73.6 ± 13.14% re-endothelialization. Immunofluorescence also revealed that the cells present in the luminal zone stained positive for vWF, confirming the presence of endothelial cells. Unfortunately, additional findings also suggest that FN exacerbated the development of hyperplastic neointima. Thus, FN may not represent the optimal coating agent for decellularized vascular grafts (70).
Flameng et al. (72) coated decellularized ovine aortic valves with fibronectin and stromal cell-derived factor 1α (FN/SDF-1α) and noticed that the coating substantially improved re-endothelialization performance on coated decellularized samples, displaying values comparable to that of native cryopreserved aortic grafts, which exhibited 39 ± 8% re-endothelialization at the leaflet region and 37 ± 5% at the wall region of the aortic graft 5 months post-implantation in Lovenaar sheep. In contrast, uncoated decellularized samples only demonstrated 10–15% re-endothelialization within the same timeframe (72).
Biomimetic Peptides
RGD (arginine-glycine-aspartic acid) represents an extensively studied biomimetic peptide that has been widely utilized as a surface modifier for biomaterials. RGD has been shown to be capable of stimulating cell adhesion, cell migration, and cell proliferation through specific recognition and interaction with integrins (107, 108). However, RGD peptides exhibit low biological activity innately, but this issue is easily counteracted via the introduction of chemical modifications to RGD to form biologically active peptides GRGDS and GRGDSPC (109). Research conducted by Wan et al. (73) modified decellularized murine pancreas with the GRGDSPC peptide to stabilize HUVECs on the scaffold. Immunofluorescent staining revealed that both GRGDSPC-conjugated samples and uncoated samples expressed Ki67 and CD31, suggesting that HUVECs effectively adhered and proliferated in groups, however, the levels of these markers were substantially higher in GRGDSPC-conjugated samples, indicating greater biocompatibility for growth and proliferation of HUVECs. The study concludes that the GRGDSPC peptide successfully binds to the pancreatic scaffold facilitating HUVEC proliferation and functional endothelialization, presumably through enhanced expression of integrins αvβ3, α5β1, and αIIβ3 (73).
In their study, Lee et al. (69) incorporated a mussel-inspired polydopamine (pDA) coating for use on a decellularized canine vein matrix consisting of the inferior vena cava and jugular vein (DVM). pDA-coated decellularized vein matrices (pDA-DVMs) were then conjugated with the RGD and YIGSR peptides to produce (CGGRGD)-pDA-DVMs and (CGGYIGSR)-pDA-DVMs, respectively. Human cord blood-derived endothelial precursor cells (hCB-EPCs) and human embryonic stem cell-derived endothelial precursor cells (hESC-EPCs) were seeded onto pDA-coated DVMs (pDA-DVM) to analyze the effects of conjugated and unconjugated pDA coatings on the efficiency of re-endothelialization. Increased metabolic activity in hCB-EPCs seeded onto (CGGYIGSR)-pDA-DVMs were observed in comparison to uncoated DVMs and pDA-DVMs. qRT-PCR suggested enhanced precursor cell differentiation into endothelial cells on the peptide-modified DVMs, as indicated by an increased expression of endothelial specific markers, with the largest increase seen in cells seeded on (CGGRGD)-pDA-DVMs. Adhesion of hEPCs on peptide modified DVMs was also improved compared to uncoated control samples. With these results, Lee et al. confirmed that the modified PDA coating positively impacted EC adhesion and metabolic activity whilst inducing the differentiation of hEPCs into an endothelial lineage. All these factors contribute to increased re-endothelialization efficiency in decellularized vascular scaffolds (69).
Other Coating Agents
López-Ruiz et al. (60) demonstrated improved re-endothelialization with decreased thrombosis risk in decellularized porcine carotid arteries coated with the polymer poly(ethylmethacrylate-co-diethylaminoethylacrylate) (8g7). 8g7 has been previously described as a novel biocompatible polymer that carries the ability to facilitate and improve EC adhesion and viability (110). DAPI staining revealed cellular repopulation occurring in a similar manner in both 8g7-coated and uncoated samples, but with a significant higher number of visible cells attached to the coated sample. Platelet adhesion testing on 8g7-coated coverslips revealed reduced platelet adhesion under increasing shear stresses in a flow system. Additionally, ECs grown on coated coverslips exhibited increased angiogenic properties as evidenced by the formation of capillary-like tubes after 4 h. Biomechanical testing of the samples showed a substantial difference in the vessel burst pressure between native, non-decellularized arteries and 8g7-coated decellularized arteries (1,330 ± 135 mbar vs. 1,153 ± 138 mbar). Tensile strength of coated samples, however, was comparable to native arteries, while uncoated decellularized arteries showed a much lower maximum load (60).
Fibrin glue (FG) is an essential biological adhesive generated from the activation of fibrinogen by thrombin and is essential in the blood coagulation cascade (111). This material was previously observed by Almelkar and colleagues to support angiogenesis, endothelial cell adhesion, migration, and proliferation (112). In their succeeding study, decellularized bovine aorta was coated with FG composed of a 1:1 ratio of fibrinogen and thrombin (62). Sheep external jugular vein endothelial cells (SEJVEC) cultured on coated and uncoated samples revealed that SEJVECs seeded onto FG-coated samples achieved full confluency in 5 days, with visual examinations showing that the seeded cells assumed a flat morphology with an expansion of filopodia, comparable to endothelial cells found in physiological conditions. Cell viability was also unaffected by the coating. In contrast, SEJVECs seeded onto uncoated samples only achieved 70% confluence after 10 days while adopting a cobblestone morphology. Immunocytochemistry revealed presence of vWF and lectin on FG-coated samples which was absent on uncoated samples. These results indicate that fibrin glue is a non-toxic coating that can be used to greatly enhance the re-endothelialization of large diameter decellularized vascular scaffolds (62).
Kim et al. (64) proposed using an anti-CD31 aptamer coating to promote re-endothelialization in decellularized murine liver scaffolds. Nucleic acid aptamers are short sequences of synthesized single-stranded oligonucleotides that have low immunogenicity and high binding affinity for specific proteins, and thus, are often considered alternatives to antibodies. Compared to antibodies, however, aptamers are often easily adjusted and producible in massive amounts at a relatively low cost with limited risk of variability and contamination (113–115). Kim et al. determined that anti-CD31 aptamer coating of decellularized liver scaffolds facilitates re-endothelialization. These contribute to the development of vascular networks that can support perfusion with increased functionality and viability through the potentiation of integrin-Akt signaling cascades (64). ECs seeded onto anti-CD31 aptamer coated grafts exhibited significantly higher cell attachment compared to uncoated grafts or anti-CD31 antibody coated grafts. Attached ECs were also more resistant to shear stresses and were less likely to detach, with aptamer-coated grafts retaining 57.84 ± 2.9% cell adhesion under shear stress and uncoated grafts retaining 21.87 ± 1.2%. HUVEC endothelial coverage on aptamer-coated grafts were also significantly higher after 7 days in culture, at 76.10 ± 3.54% coverage compared to HUVECs on uncoated grafts which had 35.22 ± 7.74% endothelial coverage (64).
Although the re-endothelialization of decellularized small dimeter vascular grafts in the context of cardiovascular diseases remain the priority for this review, the studies included utilized both decellularized vascular grafts and decellularized tissues due to the limited number of studies conducted involving bioactive coatings or surface modifications and decellularized small diameter vascular grafts. Nevertheless, the results obtained from these studies remain valuable as the core goal is to improve reendothelialization efficiency and endothelial cell retention in decellularized scaffolds whilst contending against shear stresses post-implantation. As explained previously, exposure of decellularized grafts lacking a functional endothelium to the blood stream increases the risk of acute thrombogenesis and premature graft failure, and as such, improving the efficiency the reendothelialization process is critical to minimize contact between these surfaces to reduce the chances of the aforementioned risks. The coatings discussed in the included studies generally function to enhance in situ fallout endothelialization and transmural capillary ingrowth by increasing adhesion, migration, and proliferation while promoting angiogenesis in endothelial and/or endothelial progenitor cells. VEGF, Fibronectin, and CCN1/RGD peptides represent the most utilized coatings. A similarity between these molecules is that they share is their ability to interact with various subtypes of the cell surface receptor integrin present on endothelial cells and endothelial progenitor cells, forming the basis for their effects on promoting endothelialization (65, 116, 117).
To elaborate using VEGF as an example, the interaction between VEGF-A and its specific tyrosine kinase receptor VEGFR2 results in the autophosphorylation of the receptor and thus, the activation of the receptor. The association between VEGFR2 and integrin αvβ3 expressed on circulating ECs and EPCs leads to the phosphorylation of the β3 subunit of the integrin, resulting in its activation which in turn upregulates VEGF expression, induces cell migration and activates the mitogen-activated protein kinase (MAPK) pathway, which has been shown to be involved in progenitor cell proliferation, differentiation, and survival (117–120), among many other crucial downstream processes (121–123). Fibronectin and CCN1 are both also similarly able to interact with different integrin subtypes, i.e., FN and CCN1 with integrin αvβ3, FN with α5β1, and CCN1 with α6β1 (124, 125) to carry out the aforementioned functions which helps improve in situ re-endothelialization mechanisms for the production of functional decellularized vascular grafts. Some studies described in this review resorted to coating with a combination of bioactive materials or pre-treating their decellularized grafts with different proteins prior to application of the main coating agent, such as can be seen in Hussein et al.'s (59) study combining heparin with gelatin, and Marinval et al.'s (63) study incorporated fucoidan with VEGF. This is a promising approach that could enhance the effectiveness of existing coating materials, as the benefits the secondary material, such as the antithrombogenic and anticoagulant properties of fucoidan and the myriad of integrin binding sites present on gelatin, could be garnered to produce a more effective coating. The potential complications such as cell toxicity that could arise from combining these coatings has to be taken into consideration, however. Nevertheless, this could be an avenue for future studies aiming to improve the quality of re-endothelialization in decellularized vascular scaffolds.
Conclusion
Multiple different coating agents and their effects on re-endothelialization have been discussed in this review, and the use of coating agents are capable of inducing EC adhesion, migration, and proliferation resulting in enhanced re-endothelialization efficiency. A schematic representation of the various positive effect of these coatings on decellularized vascular grafts are illustrated in Figure 2. However, the variation in testing methodologies and types of decellularized tissues complicates the comparison of coating effects across the studies. This is illustrated when comparing the studies conducted by Iijima et al. (68) and Assmann et al. (70) with other studies employing the same coating agents, as both studies reported significant neointimal hyperplasia while the same did not occur in other studies. Thus, a more standardized analysis or criteria should be established to enable more robust comparison across studies. Furthermore, future studies could compare the performance of different coating agents to ascertain the optimal reagent required to produce the highest re-endothelialization efficiency. In addition, the feasibility of large-scale implementation of these coating methodologies as well as the long-term potential of coated scaffolds is also yet to be determined. Nevertheless, these findings are promising and suggest that the creation of fully functional non-immunogenic off-the-shelf tissue engineered vascular graft alternatives could be feasible sooner than later with additional research.
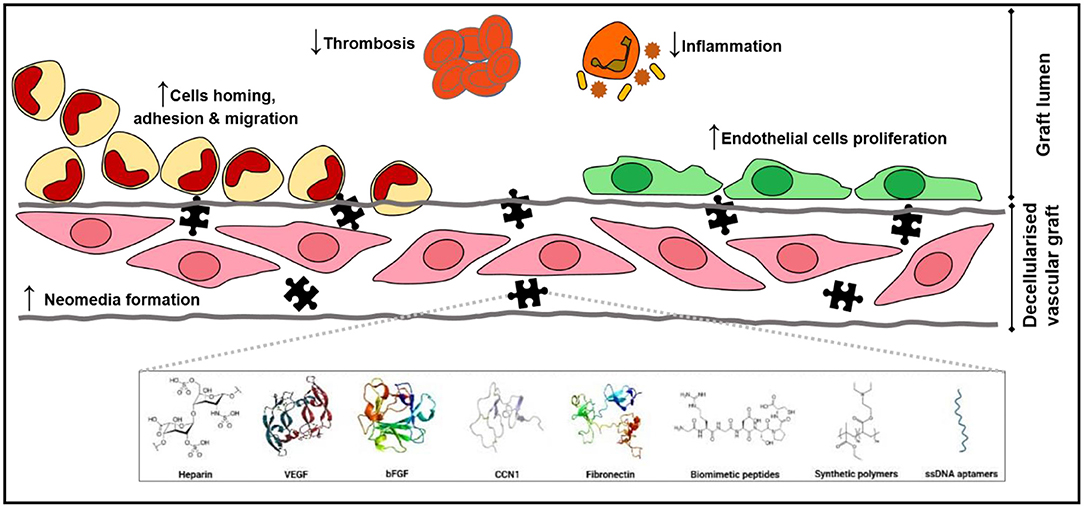
Figure 2. The missing puzzle piece. Coatings of decellularized vascular graft present a myriad of positive effects that promote cell adhesion, migration, and proliferation both on the luminal surface, enhancing reendothelialization, and in the decellularized vascular graft, establishing a neomedia that consequently strengthens mechanical properties of the graft. Coatings also halt thrombosis and reduce inflammatory reactions.
Data Availability Statement
The original contributions generated for this study are included in the article/supplementary material, further inquiries can be directed to the corresponding author/s.
Author Contributions
NS, MY, and MA laid out the broad initial research question for this systematic review. JH, NS, and MY designed the search and refine the research question. JH performed article selection and screening, data collection and extraction, manuscript writing, and data analysis. NS oversees, review and verify articles selection, data collection, and analysis. MY and MA performed final proofreading of the manuscript. All authors contributed to the article and approved the submitted version.
Funding
This study was made possible by an International Matching Fund (MyPAIR)-UK-MY Joint Partnership on Non-Communicable Diseases (NCD): NEWTON-Medical Research Council 2020-1 (Grant code: NEWTON-MRC/2020/001) from the Ministry of Education Malaysia. The funder does not have any contribution and decision on publishing or preparation of the manuscript.
Conflict of Interest
The authors declare that the research was conducted in the absence of any commercial or financial relationships that could be construed as a potential conflict of interest.
Publisher's Note
All claims expressed in this article are solely those of the authors and do not necessarily represent those of their affiliated organizations, or those of the publisher, the editors and the reviewers. Any product that may be evaluated in this article, or claim that may be made by its manufacturer, is not guaranteed or endorsed by the publisher.
Acknowledgments
All authors would like to thank the Faculty of Medicine, UKM, for the resources used to complete this review.
References
1. Flora GD, Nayak MK. A brief review of cardiovascular diseases, associated risk factors and current treatment regimes. Curr Pharm Des. (2019) 25:4063–84. doi: 10.2174/1381612825666190925163827
2. World Health Organization. Cardiovascular diseases (CVDs). (2017). Available online at: https://www.who.int/en/news-room/fact-sheets/detail/cardiovascular-diseases-(cvds) (accessed January 19, 2021).
3. Mathers CD, Loncar D. Projections of global mortality and burden of disease from 2002 to (2030). PLoS Med. (2006) 3:e442. doi: 10.1371/journal.pmed.0030442
4. El Fakiri F, Bruijnzeels MA, Hoes AW. Prevention of cardiovascular diseases: focus on modifiable cardiovascular risk. Heart. (2006) 92:741. doi: 10.1136/hrt.2005.068114
5. Rafieian-Kopaei M, Setorki M, Doudi M, Baradaran A, Nasri H. Atherosclerosis: process, indicators, risk factors and new hopes. Int J Prev Med. (2014) 5:927–46.
6. Neglia D, Rovai D, Caselli C, Pietila M, Teresinska A, Aguadé-Bruix S, et al. Detection of significant coronary artery disease by noninvasive anatomical and functional imaging. Circ Cardiovasc Imaging. (2015) 8:e002179. doi: 10.1161/CIRCIMAGING.114.002179
7. Abdulhannan P, Russell DA, Homer-Vanniasinkam S. Peripheral arterial disease: a literature review. Br Med Bull. (2012) 104:21–39. doi: 10.1093/bmb/lds027
8. Mohr FW, Morice MC, Kappetein AP, Feldman TE, Ståhle E, Colombo A, et al. Coronary artery bypass graft surgery versus percutaneous coronary intervention in patients with three-vessel disease and left main coronary disease: 5-year follow-up of the randomised, clinical SYNTAX trial. Lancet. (2013) 381:629–38. doi: 10.1016/S0140-6736(13)60141-5
9. Weintraub WS, Grau-Sepulveda MV, Weiss JM, O'Brien SM, Peterson ED, Kolm P, et al. Comparative effectiveness of revascularization strategies. N Engl J Med. (2012) 366:1467–76. doi: 10.1056/NEJMoa1110717
10. Spadaccio C, Benedetto U. Coronary artery bypass grafting (CABG) vs. percutaneous coronary intervention (PCI) in the treatment of multivessel coronary disease: quo vadis? -a review of the evidences on coronary artery disease. Ann Cardiothorac Surg. (2018) 7:506–15. doi: 10.21037/acs.2018.05.17
11. Harskamp RE, Lopes RD, Baisden CE, de Winter RJ, Alexander JH. Saphenous vein graft failure after coronary artery bypass surgery: pathophysiology, management, and future directions. Ann Surg. (2013) 257:824–33. doi: 10.1097/SLA.0b013e318288c38d
12. Altshuler P, Nahirniak P, Welle NJ. Saphenous Vein Grafts. StatPearls. Treasure Island (FL): StatPearls Publishing (2020).
13. Pashneh-Tala S, MacNeil S, Claeyssens F. The tissue-engineered vascular graft-past, present, and future. Tissue Eng Part B Rev. (2016) 22:68–100. doi: 10.1089/ten.teb.2015.0100
14. Gilbert TW, Sellaro TL, Badylak SF. Decellularization of tissues and organs. Biomaterials. (2006) 27:3675–83. doi: 10.1016/j.biomaterials.2006.02.014
15. Crapo PM, Gilbert TW, Badylak SF. An overview of tissue and whole organ decellularization processes. Biomaterials. (2011) 32:3233–43. doi: 10.1016/j.biomaterials.2011.01.057
16. Badylak SF, Taylor D, Uygun K. Whole-organ tissue engineering: decellularization and recellularization of three-dimensional matrix scaffolds. Annu Rev Biomed Eng. (2011) 13:27–53. doi: 10.1146/annurev-bioeng-071910-124743
17. Keane TJ, Londono R, Turner NJ, Badylak SF. Consequences of ineffective decellularization of biologic scaffolds on the host response. Biomaterials. (2012) 33:1771–81. doi: 10.1016/j.biomaterials.2011.10.054
18. Roy S, Silacci P, Stergiopulos N. Biomechanical proprieties of decellularized porcine common carotid arteries. Am J Physiol Heart Circ Physiol. (2005) 289:H1567–76. doi: 10.1152/ajpheart.00564.2004
19. Williams C, Liao J, Joyce EM, Wang B, Leach JB, Sacks MS, et al. Altered structural and mechanical properties in decellularized rabbit carotid arteries. Acta Biomater. (2009) 5:993–1005. doi: 10.1016/j.actbio.2008.11.028
20. Dahl SL, Kypson AP, Lawson JH, Blum JL, Strader JT, Li Y, et al. Readily available tissue-engineered vascular grafts. Sci Transl Med. (2011) 3:68ra9. doi: 10.1126/scitranslmed.3001426
21. Gui L, Muto A, Chan SA, Breuer CK, Niklason LE. Development of decellularized human umbilical arteries as small-diameter vascular grafts. Tissue Eng Part A. (2009) 15:2665–76. doi: 10.1089/ten.tea.2008.0526
22. Fitriatul N, Sha'ban M, Azhim A. Evaluation of recellularization on decellularized aorta scaffolds engineered by ultrasonication treatment. Annu Int Conf IEEE Eng Med Biol Soc. (2017) 2017:2072–5. doi: 10.1109/EMBC.2017.8037261
23. Lin C-H, Hsia K, Ma H, Lee H, Lu J-H. In vivo performance of decellularized vascular grafts: a review article. Int J Mol Sci. (2018) 19:2101. doi: 10.3390/ijms19072101
24. Chemla ES, Morsy M. Randomized clinical trial comparing decellularized bovine ureter with expanded polytetrafluoroethylene for vascular access. BJS. (2009) 96:34–9. doi: 10.1002/bjs.6434
25. Sabanayagam P, Schwartz AB, Soricelli RR, Lyons P, Chinitz J. A comparative study of 402 bovine heterografts and 225 reinforced expanded PTFE grafts as AVF in the ESRD patient. Trans Am Soc Artif Intern Organs. (1980) 26:88–92.
26. Cho S-W, Lim SH, Kim I-K, Hong YS, Kim S-S, Yoo KJ, et al. Small-diameter blood vessels engineered with bone marrow-derived cells. Ann Surg. (2005) 241:506–15. doi: 10.1097/01.sla.0000154268.12239.ed
27. Quint C, Kondo Y, Manson RJ, Lawson JH, Dardik A, Niklason LE. Decellularized tissue-engineered blood vessel as an arterial conduit. Proc Natl Acad Sci U S A. (2011) 108:9214–9. doi: 10.1073/pnas.1019506108
28. Kaushal S, Amiel GE, Guleserian KJ, Shapira OM, Perry T, Sutherland FW, et al. Functional small-diameter neovessels created using endothelial progenitor cells expanded ex vivo. Nat Med. (2001) 7:1035–40. doi: 10.1038/nm0901-1035
29. McGuigan AP, Sefton MV. The influence of biomaterials on endothelial cell thrombogenicity. Biomaterials. (2007) 28:2547–71. doi: 10.1016/j.biomaterials.2007.01.039
30. Braghirolli DI, Helfer VE, Chagastelles PC, Dalberto TP, Gamba D, Pranke P. Electrospun scaffolds functionalized with heparin and vascular endothelial growth factor increase the proliferation of endothelial progenitor cells. Biomed Mater. (2017) 12:025003. doi: 10.1088/1748-605X/aa5bbc
31. Martin ND, Schaner PJ, Tulenko TN, Shapiro IM, Dimatteo CA, Williams TK, et al. In vivo behavior of decellularized vein allograft. J Surg Res. (2005) 129:17–23. doi: 10.1016/j.jss.2005.06.037
32. Zhou M, Liu Z, Wei Z, Liu C, Qiao T, Ran F, et al. Development and validation of small-diameter vascular tissue from a decellularized scaffold coated with heparin and vascular endothelial growth factor. Artif Organs. (2009) 33:230–9. doi: 10.1111/j.1525-1594.2009.00713.x
33. Wu MH, Shi Q, Sauvage LR, Kaplan S, Hayashida N, Patel MD, et al. The direct effect of graft compliance mismatch per se on development of host arterial intimal hyperplasia at the anastomotic interface. Ann Vasc Surg. (1993) 7:156–68. doi: 10.1007/BF02001010
34. Sarkar S, Salacinski HJ, Hamilton G, Seifalian AM. The mechanical properties of infrainguinal vascular bypass grafts: their role in influencing patency. Eur J Vasc Endovasc Surg. (2006) 31:627–36. doi: 10.1016/j.ejvs.2006.01.006
35. Tiwari A, Cheng KS, Salacinski H, Hamilton G, Seifalian AM. Improving the patency of vascular bypass grafts: the role of suture materials and surgical techniques on reducing anastomotic compliance mismatch. Eur J Vasc Endovasc Surg. (2003) 25:287–95. doi: 10.1053/ejvs.2002.1810
36. Lemson MS, Tordoir JH, Daemen MJ, Kitslaar PJ. Intimal hyperplasia in vascular grafts. Eur J Vasc Endovasc Surg. (2000) 19:336–50. doi: 10.1053/ejvs.1999.1040
37. Lopera Higuita M, Griffiths LG. Small diameter xenogeneic extracellular matrix scaffolds for vascular applications. Tissue Eng B Rev. (2019) 26:26–45. doi: 10.1089/ten.teb.2019.0229
38. Cissell DD, Hu JC, Griffiths LG, Athanasiou KA. Antigen removal for the production of biomechanically functional, xenogeneic tissue grafts. J Biomech. (2014) 47:1987–96. doi: 10.1016/j.jbiomech.2013.10.041
39. Sánchez PF, Brey EM, Briceño JC. Endothelialization mechanisms in vascular grafts. J Tissue Eng Regen Med. (2018) 12:2164–78. doi: 10.1002/term.2747
40. Berger K, Sauvage LR, Rao AM, Wood SJ. Healing of arterial prostheses in man: its incompleteness. Ann Surg. (1972) 175:118–27. doi: 10.1097/00000658-197201000-00018
41. Pennel T, Zilla P, Bezuidenhout D. Differentiating transmural from transanastomotic prosthetic graft endothelialization through an isolation loop-graft model. J Vasc Surg. (2013) 58:1053–61. doi: 10.1016/j.jvs.2012.11.093
42. Clowes AW, Kirkman TR, Reidy MA. Mechanisms of arterial graft healing. Rapid transmural capillary ingrowth provides a source of intimal endothelium and smooth muscle in porous PTFE prostheses. Am J Pathol. (1986) 123:220–30.
43. Zhang Z, Briana S, Douville Y, Zhao H, Gilbert N. Transmural communication at a subcellular level may play a critical role in the fallout based-endothelialization of dacron vascular prostheses in canine. J Biomed Mater Res A. (2007) 81A:877–87. doi: 10.1002/jbm.a.31124
44. Ren X, Feng Y, Guo J, Wang H, Li Q, Yang J, et al. Surface modification and endothelialization of biomaterials as potential scaffolds for vascular tissue engineering applications. Chem Soc Rev. (2015) 44:5680–742. doi: 10.1039/C4CS00483C
45. Balistreri CR, Buffa S, Pisano C, Lio D, Ruvolo G, Mazzesi G. Are endothelial progenitor cells the real solution for cardiovascular diseases? Focus on Controversies and Perspectives. BioMed Res Int. (2015) 2015:835934. doi: 10.1155/2015/835934
46. Henry JJD, Yu J, Wang A, Lee R, Fang J, Li S. Engineering the mechanical and biological properties of nanofibrous vascular grafts for in situ vascular tissue engineering. Biofabrication. (2017) 9:035007. doi: 10.1088/1758-5090/aa834b
47. Grassl ED, Oegema TR, Tranquillo RT. A fibrin-based arterial media equivalent. J Biomed Mater Res A. (2003) 66A:550–61. doi: 10.1002/jbm.a.10589
48. Wang R, de Kort BJ, Smits AIPM, Weiss AS. Elastin in vascular grafts. In: Walpoth B, Bergmeister H, Bowlin G, Kong D, Rotmans J, Zilla P, editors. Tissue-Engineered Vascular Grafts. Cham: Springer International Publishing (2019). p. 1–32. doi: 10.1007/978-3-319-71530-8_13-1
49. Abbott WM, Callow A, Moore W, Rutherford R, Veith F, Weinberg S. Evaluation and performance standards for arterial prostheses. J Vasc Surg. (1993) 17:746–56. doi: 10.1016/0741-5214(93)90120-B
50. Bennion R, Williams R, Stabile B, Fox M, Owens M, Wilson S. Patency of autogenous saphenous vein versus polytetrafluoroethylene grafts in femoropopliteal bypass for advanced ischemia of the extremity. Surg Gynecol Obstet. (1985) 160:239–42.
51. Hsia K, Lin CH, Lee HY, Chen WM, Yao CL, Chen CC, et al. Sphingosine-1-phosphate in endothelial cell recellularization improves patency and endothelialization of decellularized vascular grafts in vivo. Int J Mol Sci. (2019) 20:1641. doi: 10.3390/ijms20071641
52. Menu P, Stoltz JF, Kerdjoudj H. Progress in vascular graft substitute. Clin Hemorheol Microcirc. (2013) 53:117–29. doi: 10.3233/CH-2012-1580
53. Kurobe H, Maxfield MW, Breuer CK, Shinoka T. Concise review: tissue-engineered vascular grafts for cardiac surgery: past, present, and future. Stem Cells Transl Med. (2012) 1:566–71. doi: 10.5966/sctm.2012-0044
54. Zhou M, Liu Z, Li K, Qiao W, Jiang X, Ran F, et al. Beneficial effects of granulocyte-colony stimulating factor on small-diameter heparin immobilized decellularized vascular graft. J Biomed Mater Res A. (2010) 95:600–10. doi: 10.1002/jbm.a.32864
55. Li J, Cai Z, Cheng J, Wang C, Fang Z, Xiao Y, et al. Characterization of a heparinized decellularized scaffold and its effects on mechanical and structural properties. J Biomater Sci Polym Ed. (2020) 31:999–1023. doi: 10.1080/09205063.2020.1736741
56. Dimitrievska S, Cai C, Weyers A, Balestrini JL, Lin T, Sundaram S, et al. Click-coated, heparinized, decellularized vascular grafts. Acta Biomater. (2015) 13:177–87. doi: 10.1016/j.actbio.2014.11.015
57. Hsia K, Yang MJ, Chen WM, Yao CL, Lin CH, Loong CC, et al. Sphingosine-1-phosphate improves endothelialization with reduction of thrombosis in recellularized human umbilical vein graft by inhibiting syndecan-1 shedding in vitro. Acta Biomater. (2017) 51:341–50. doi: 10.1016/j.actbio.2017.01.050
58. Hutton B, Salanti G, Caldwell DM, Chaimani A, Schmid CH, Cameron C, et al. The PRISMA extension statement for reporting of systematic reviews incorporating network meta-analyses of health care interventions: checklist and explanations. Ann Intern Med. (2015) 162:777–84. doi: 10.7326/M14-2385
59. Hussein KH, Park KM, Kang KS, Woo HM. Heparin-gelatin mixture improves vascular reconstruction efficiency and hepatic function in bioengineered livers. Acta Biomater. (2016) 38:82–93. doi: 10.1016/j.actbio.2016.04.042
60. López-Ruiz E, Venkateswaran S, Perán M, Jiménez G, Pernagallo S, Díaz-Mochón JJ, et al. Poly(ethylmethacrylate-co-diethylaminoethyl acrylate) coating improves endothelial re-population, bio-mechanical and anti-thrombogenic properties of decellularized carotid arteries for blood vessel replacement. Sci Rep. (2017) 7:407. doi: 10.1038/s41598-017-00294-6
61. Bär A, Dorfman SE, Fischer P, Hilfiker-Kleiner D, Cebotari S, Tudorache I, et al. The pro-angiogenic factor CCN1 enhances the re-endothelialization of biological vascularized matrices in vitro. Cardiovasc Res. (2010) 85:806–13. doi: 10.1093/cvr/cvp370
62. Walawalkar S, Almelkar S. Fabrication of aortic bioprosthesis by decellularization, fibrin glue coating and re-endothelization: a cell scaffold approach. Prog Biomater. (2019) 8:197–210. doi: 10.1007/s40204-019-00122-2
63. Marinval N, Morenc M, Labour MN, Samotus A, Mzyk A, Ollivier V, et al. Fucoidan/VEGF-based surface modification of decellularized pulmonary heart valve improves the antithrombotic and re-endothelialization potential of bioprostheses. Biomaterials. (2018) 172:14–29. doi: 10.1016/j.biomaterials.2018.01.054
64. Kim D-H, Ahn J, Kang HK, Kim M-S, Kim N-G, Kook MG, et al. Development of highly functional bioengineered human liver with perfusable vasculature. Biomaterials. (2021) 265:120417. doi: 10.1016/j.biomaterials.2020.120417
65. Böer U, Spengler C, Jonigk D, Klingenberg M, Schrimpf C, Lützner S, et al. Coating decellularized equine carotid arteries with CCN1 improves cellular repopulation, local biocompatibility, and immune response in sheep. Tissue Eng A. (2013) 19:1829–42. doi: 10.1089/ten.tea.2012.0558
66. Tsai T-N, Kirton JP, Campagnolo P, Zhang L, Xiao Q, Zhang Z, et al. Contribution of stem cells to neointimal formation of decellularized vessel grafts in a novel mouse model. Am J Pathol. (2012) 181:362–73. doi: 10.1016/j.ajpath.2012.03.021
67. Musilkova J, Filova E, Pala J, Matejka R, Hadraba D, Vondrasek D, et al. Human decellularized and crosslinked pericardium coated with bioactive molecular assemblies. Biomed Mater. (2019) 15:015008. doi: 10.1088/1748-605X/ab52db
68. Iijima M, Aubin H, Steinbrink M, Schiffer F, Assmann A, Weisel RD, et al. Bioactive coating of decellularized vascular grafts with a temperature-sensitive VEGF-conjugated hydrogel accelerates autologous endothelialization in vivo. J Tissue Eng Regen Med. (2018) 12:e513–22. doi: 10.1002/term.2321
69. Lee JS, Lee K, Moon SH, Chung HM, Lee JH, Um SH, et al. Mussel-inspired cell-adhesion peptide modification for enhanced endothelialization of decellularized blood vessels. Macromol Biosci. (2014) 14:1181–9. doi: 10.1002/mabi.201400052
70. Assmann A, Delfs C, Munakata H, Schiffer F, Horstkötter K, Huynh K, et al. Acceleration of autologous in vivo recellularization of decellularized aortic conduits by fibronectin surface coating. Biomaterials. (2013) 34:6015–26. doi: 10.1016/j.biomaterials.2013.04.037
71. Conklin BS, Wu H, Lin PH, Lumsden AB, Chen C. Basic fibroblast growth factor coating and endothelial cell seeding of a decellularized heparin-coated vascular graft. Artif Organs. (2004) 28:668–75. doi: 10.1111/j.1525-1594.2004.00062.x
72. Flameng W, De Visscher G, Mesure L, Hermans H, Jashari R, Meuris B. Coating with fibronectin and stromal cell–derived factor-1α of decellularized homografts used for right ventricular outflow tract reconstruction eliminates immune response–related degeneration. J Thorac Cardiovasc Surg. (2014) 147:1398–404.e2. doi: 10.1016/j.jtcvs.2013.06.022
73. Wan J, Wang L, Huang Y, Fan H, Chen C, Yuan X, et al. Using GRGDSPC peptides to improve re-endothelialization of decellularized pancreatic scaffolds. Artif Organs. (2020) 44:E172–e80. doi: 10.1111/aor.13602
74. Leuning DG, Witjas FMR, Maanaoui M, de Graaf AMA, Lievers E, Geuens T, et al. Vascular bioengineering of scaffolds derived from human discarded transplant kidneys using human pluripotent stem cell-derived endothelium. Am J Transplant. (2019) 19:1328–43. doi: 10.1111/ajt.15200
75. Bârzu T, Van Rijn JLML, Petitou M, Molho P, Tobelem G, Caen JP. Endothelial binding sites for heparin. Specificity and role in heparin neutralization. Biochem J. (1986) 238:847–54. doi: 10.1042/bj2380847
76. Murga M, Yao L, Tosato G. Derivation of endothelial cells from CD34- umbilical cord blood. Stem Cells. (2004) 22:385–95. doi: 10.1634/stemcells.22-3-385
77. Zhang W, Swanson R, Xiong Y, Richard B, Olson ST. Antiangiogenic antithrombin blocks the heparan sulfate-dependent binding of proangiogenic growth factors to their endothelial cell receptors: EVIDENCE FOR DIFFERENTIAL BINDING OF ANTIANGIOGENIC AND ANTICOAGULANT FORMS OF ANTITHROMBIN TO PROANGIOGENIC HEPARAN SULFATE DOMAINS*. J Biol Chem. (2006) 281:37302–10. doi: 10.1074/jbc.M604905200
78. Zieris A, Prokoph S, Levental KR, Welzel PB, Grimmer M, Freudenberg U, et al. FGF-2 and VEGF functionalization of starPEG–heparin hydrogels to modulate biomolecular and physical cues of angiogenesis. Biomaterials. (2010) 31:7985–94. doi: 10.1016/j.biomaterials.2010.07.021
79. Sung H-W, Liang IL, Chen C-N, Huang R-N, Liang H-F. Stability of a biological tissue fixed with a naturally occurring crosslinking agent (genipin). J Biomed Mater Res. (2001) 55:538–46. doi: 10.1002/1097-4636(20010615)55:4<538::aid-jbm1047>3.0.co;2-2
80. Wassenaar JW, Braden RL, Osborn KG, Christman KL. Modulating in vivo degradation rate of injectable extracellular matrix hydrogels. J Mater Chem B. (2016) 4:2794–802. doi: 10.1039/C5TB02564H
81. Sung H-W, Huang R-N, Huang LLH, Tsai C-C, Chiu C-T. Feasibility study of a natural crosslinking reagent for biological tissue fixation. J Biomed Mater Res. (1998) 42:560–7. doi: 10.1002/(sici)1097-4636(19981215)42:4<560::aid-jbm12>3.0.co;2-i
82. Sung H-W, Chang W-H, Ma C-Y, Lee M-H. Crosslinking of biological tissues using genipin and/or carbodiimide. J Biomed Mater Res A. (2003) 64A:427–38. doi: 10.1002/jbm.a.10346
83. Kaplan O, Hierlemann T, Krajewski S, Kurz J, Nevoralová M, Houska M, et al. Low-thrombogenic fibrin-heparin coating promotes in vitro endothelialization. J Biomed Mater Res A. (2017) 105:2995–3005. doi: 10.1002/jbm.a.36152
84. Roghani M, Mansukhani A, Dell'Era P, Bellosta P, Basilico C, Rifkin DB, et al. Heparin increases the affinity of basic fibroblast growth factor for its receptor but is not required for binding. J Biol Chem. (1994) 269:3976–84 doi: 10.1016/S0021-9258(17)41730-3
85. Klagsbrun M. Mediators of angiogenesis: the biological significance of basic fibroblast growth factor (bFGF)-heparin and heparan sulfate interactions. Semin Cancer Biol. (1992) 3:81–7.
86. Meurice T, Bauters C, Vallet B, Corseaux D, Van Belle E, Hamon M, et al. Basic fibroblast growth factor restores endothelium-dependent responses of hypercholesterolemic rabbit thoracic aorta. Fundam Clin Pharmacol. (1997) 2:185. doi: 10.1152/ajpheart.1997.272.2.H613
87. Lindner V, Majack R, Reidy M. Basic fibroblast growth factor stimulates endothelial regrowth and proliferation in denuded arteries. J Clin Investig. (1990) 85:2004–8. doi: 10.1172/JCI114665
88. Ferrara N, Henzel WJ. Pituitary follicular cells secrete a novel heparin-binding growth factor specific for vascular endothelial cells. Biochem Biophys Res Commun. (1989) 161:851–8. doi: 10.1016/0006-291X(89)92678-8
89. Keck PJ, Hauser SD, Krivi G, Sanzo K, Warren T, Feder J, et al. Vascular permeability factor, an endothelial cell mitogen related to PDGF. Science. (1989) 246:1309–12. doi: 10.1126/science.2479987
90. Connolly DT, Heuvelman DM, Nelson R, Olander JV, Eppley BL, Delfino J, et al. Tumor vascular permeability factor stimulates endothelial cell growth and angiogenesis. J Clin Investig. (1989) 84:1470–8. doi: 10.1172/JCI114322
91. Leung DW, Cachianes G, Kuang W-J, Goeddel DV, Ferrara N. Vascular endothelial growth factor is a secreted angiogenic mitogen. Science. (1989) 246:1306–9. doi: 10.1126/science.2479986
92. Wu J, Zeng F, Huang XP, Chung JC, Konecny F, Weisel RD, et al. Infarct stabilization and cardiac repair with a VEGF-conjugated, injectable hydrogel. Biomaterials. (2011) 32:579–86. doi: 10.1016/j.biomaterials.2010.08.098
93. Cumashi A, Ushakova NA, Preobrazhenskaya ME, D'Incecco A, Piccoli A, Totani L, et al. A comparative study of the anti-inflammatory, anticoagulant, antiangiogenic, and antiadhesive activities of nine different fucoidans from brown seaweeds. Glycobiology. (2007) 17:541–52. doi: 10.1093/glycob/cwm014
94. Colliec-Jouault S, Millet J, Helley D, Sinquin C, Fischer AM. Effect of low-molecular-weight fucoidan on experimental arterial thrombosis in the rabbit and rat. J Thromb Haemost. (2003) 1:1114–5. doi: 10.1046/j.1538-7836.2003.t01-1-00215.x
95. Durand E, Helley D, Al Haj Zen A, Dujols C, Bruneval P, Colliec-Jouault S, et al. Effect of low molecular weight fucoidan and low molecular weight heparin in a rabbit model of arterial thrombosis. J Vasc Res. (2008) 45:529–37. doi: 10.1159/000129687
96. Hlawaty H, Suffee N, Sutton A, Oudar O, Haddad O, Ollivier V, et al. Low molecular weight fucoidan prevents intimal hyperplasia in rat injured thoracic aorta through the modulation of matrix metalloproteinase-2 expression. Biochem Pharmacol. (2011) 81:233–43. doi: 10.1016/j.bcp.2010.09.021
97. Kireeva ML, Mo FE, Yang GP, Lau LF. Cyr61, a product of a growth factor-inducible immediate-early gene, promotes cell proliferation, migration, and adhesion. Mol Cell Biol. (1996) 16:1326–34. doi: 10.1128/MCB.16.4.1326
98. Kireeva ML, Lam SC, Lau LF. Adhesion of human umbilical vein endothelial cells to the immediate-early gene product Cyr61 is mediated through integrin alphavbeta3. J Biol Chem. (1998) 273:3090–6. doi: 10.1074/jbc.273.5.3090
99. Kireeva ML, Latinkić BV, Kolesnikova TV, Chen CC, Yang GP, Abler AS, et al. Cyr61 and Fisp12 are both ECM-associated signaling molecules: activities, metabolism, and localization during development. Exp Cell Res. (1997) 233:63–77. doi: 10.1006/excr.1997.3548
100. Kolesnikova TV, Lau LF. Human CYR61-mediated enhancement of bFGF-induced DNA synthesis in human umbilical vein endothelial cells. Oncogene. (1998) 16:747–54. doi: 10.1038/sj.onc.1201572
101. Grote K, Salguero G, Ballmaier M, Dangers M, Drexler H, Schieffer B. The angiogenic factor CCN1 promotes adhesion and migration of circulating CD34+ progenitor cells: potential role in angiogenesis and endothelial regeneration. Blood. (2007) 110:877–85. doi: 10.1182/blood-2006-07-036202
102. Jiang L, Sun X, Deng J, Hu Y, Xu Q. Different roles of stem/progenitor cells in vascular remodeling. Antioxid Redox Signal. (2021) 35:192–203. doi: 10.1089/ars.2020.8199
103. Asahara T, Murohara T, Sullivan A, Silver M, van der Zee R, Li T, et al. Isolation of putative progenitor endothelial cells for angiogenesis. Science. (1997) 275:964–6. doi: 10.1126/science.275.5302.964
104. Abe Y, Ozaki Y, Kasuya J, Yamamoto K, Ando J, Sudo R, et al. Endothelial progenitor cells promote directional three-dimensional endothelial network formation by secreting vascular endothelial growth factor. PLoS ONE. (2013) 8:e82085. doi: 10.1371/journal.pone.0082085
105. O'Neill TJ IV, Wamhoff BR, Owens GK, Skalak TC. Mobilization of bone marrow–derived cells enhances the angiogenic response to hypoxia without transdifferentiation into endothelial cells. Circ Res. (2005) 97:1027–35. doi: 10.1161/01.RES.0000189259.69645.25
106. Bedair TM, ElNaggar MA, Joung YK, Han DK. Recent advances to accelerate re-endothelialization for vascular stents. J Tissue Eng. (2017) 8:2041731417731546. doi: 10.1177/2041731417731546
107. Alipour M, Baneshi M, Hosseinkhani S, Mahmoudi R, Jabari Arabzadeh A, Akrami M, et al. Recent progress in biomedical applications of RGD-based ligand: from precise cancer theranostics to biomaterial engineering: a systematic review. J Biomed Mater Res A. (2020) 108:839–50. doi: 10.1002/jbm.a.36862
108. Hersel U, Dahmen C, Kessler H. RGD modified polymers: biomaterials for stimulated cell adhesion and beyond. Biomaterials. (2003) 24:4385–415. doi: 10.1016/S0142-9612(03)00343-0
109. Verrier S, Pallu S, Bareille R, Jonczyk A, Meyer J, Dard M, et al. Function of linear and cyclic RGD-containing peptides in osteoprogenitor cells adhesion process. Biomaterials. (2002) 23:585–96. doi: 10.1016/S0142-9612(01)00145-4
110. Pernagallo S, Tura O, Wu M, Samuel K, Diaz-Mochon JJ, Hansen A, et al. Novel biopolymers to enhance endothelialisation of intra-vascular devices. Adv Healthcare Mater. (2012) 1:646–56. doi: 10.1002/adhm.201200130
111. Buckley RC, Breazeale EE, Edmond JA, Brzezienski MA. A simple preparation of autologous fibrin glue for skin-graft fixation. Plast Reconstr Surg. (1999) 103:202–6. doi: 10.1097/00006534-199901000-00033
112. Almelkar SI, Patwardhan AM, Divate SA, Agrawal NB, Bhonde RR, Chaukar AP. Fibrin matrix supports endothelial cell adhesion and migration in culture. OA Biol. (2014) 2:5.
113. Song K-M, Lee S, Ban C. Aptamers and their biological applications. Sensors. (2012) 12:612–31. doi: 10.3390/s120100612
114. Zhou J, Rossi J. Aptamers as targeted therapeutics: current potential and challenges. Nat Rev Drug Discov. (2017) 16:181–202. doi: 10.1038/nrd.2016.199
115. Toh SY, Citartan M, Gopinath SCB, Tang T-H. Aptamers as a replacement for antibodies in enzyme-linked immunosorbent assay. Biosens Bioelectron. (2015) 64:392–403. doi: 10.1016/j.bios.2014.09.026
116. Chang H-K, Kim P-H, Kim DW, Cho H-M, Jeong MJ, Kim DH, et al. Coronary stents with inducible VEGF/HGF-secreting UCB-MSCs reduced restenosis and increased re-endothelialization in a swine model. Exp Mol Med. (2018) 50:1–14. doi: 10.1038/s12276-018-0143-9
117. Almalki SG, Agrawal DK. ERK signaling is required for VEGF-A/VEGFR2-induced differentiation of porcine adipose-derived mesenchymal stem cells into endothelial cells. Stem Cell Res Ther. (2017) 8:113. doi: 10.1186/s13287-017-0568-4
118. Sase H, Watabe T, Kawasaki K, Miyazono K, Miyazawa K. VEGFR2-PLCγ1 axis is essential for endothelial specification of VEGFR2+ vascular progenitor cells. J Cell Sci. (2009) 122:3303–11. doi: 10.1242/jcs.049908
119. Atay O, Skotheim JM. Spatial and temporal signal processing and decision making by MAPK pathways. J Cell Biol. (2017) 216:317–30. doi: 10.1083/jcb.201609124
120. Masson-Gadais B, Houle F, Laferrière J, Huot J. Integrin alphavbeta3, requirement for VEGFR2-mediated activation of SAPK2/p38 and for Hsp90-dependent phosphorylation of focal adhesion kinase in endothelial cells activated by VEGF. Cell Stress Chaperones. (2003) 8:37–52. doi: 10.1379/1466-1268(2003)8<37:ivrfva>2.0.co;2
121. Mahabeleshwar GH, Feng W, Reddy K, Plow EF, Byzova TV. Mechanisms of integrin-vascular endothelial growth factor receptor cross-activation in angiogenesis. Circ Res. (2007) 101:570–80. doi: 10.1161/CIRCRESAHA.107.155655
122. Lorger M, Krueger JS, O'Neal M, Staflin K, Felding-Habermann B. Activation of tumor cell integrin α <sub>v</sub>β <sub>3</sub> controls angiogenesis and metastatic growth in the brain. Proc Natl Acad Sci. (2009) 106:10666–71. doi: 10.1073/pnas.0903035106
123. Abhinand CS, Raju R, Soumya SJ, Arya PS, Sudhakaran PR. VEGF-A/VEGFR2 signaling network in endothelial cells relevant to angiogenesis. J Cell Commun Signal. (2016) 10:347–54. doi: 10.1007/s12079-016-0352-8
124. Charo IF, Nannizzi L, Smith JW, Cheresh DA. The vitronectin receptor alpha v beta 3 binds fibronectin and acts in concert with alpha 5 beta 1 in promoting cellular attachment and spreading on fibronectin. J Cell Biol. (1990) 111:2795–800. doi: 10.1083/jcb.111.6.2795
Keywords: decellularize, coating, endothelial, scaffold, treatment, small diameter, cardiovascular disease
Citation: Heng JW, Yazid MD, Abdul Rahman MR and Sulaiman N (2021) Coatings in Decellularized Vascular Scaffolds for the Establishment of a Functional Endothelium: A Scoping Review of Vascular Graft Refinement. Front. Cardiovasc. Med. 8:677588. doi: 10.3389/fcvm.2021.677588
Received: 08 March 2021; Accepted: 06 July 2021;
Published: 29 July 2021.
Edited by:
Jessica E. Wagenseil, Washington University in St. Louis, United StatesReviewed by:
Kamal Hany Hussein, Assiut University, EgyptWalter Murfee, University of Florida, United States
Copyright © 2021 Heng, Yazid, Abdul Rahman and Sulaiman. This is an open-access article distributed under the terms of the Creative Commons Attribution License (CC BY). The use, distribution or reproduction in other forums is permitted, provided the original author(s) and the copyright owner(s) are credited and that the original publication in this journal is cited, in accordance with accepted academic practice. No use, distribution or reproduction is permitted which does not comply with these terms.
*Correspondence: Nadiah Sulaiman, bmFkaWFoc3VsYWltYW5AdWttLmVkdS5teQ==