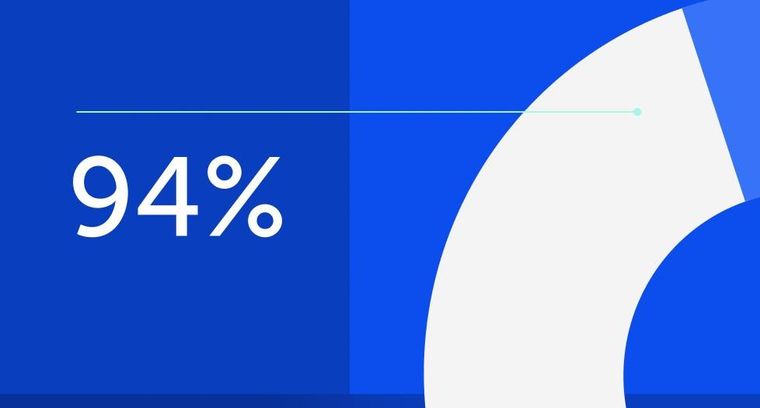
94% of researchers rate our articles as excellent or good
Learn more about the work of our research integrity team to safeguard the quality of each article we publish.
Find out more
REVIEW article
Front. Cardiovasc. Med., 13 April 2021
Sec. Cardiovascular Metabolism
Volume 8 - 2021 | https://doi.org/10.3389/fcvm.2021.649785
This article is part of the Research TopicOxidative Stress in Cardiovascular Diseases and Pulmonary HypertensionView all 8 articles
Reactive oxygen species (ROS) plays a role in intracellular signal transduction under physiological conditions while also playing an essential role in diseases such as hypertension, ischemic heart disease, and diabetes, as well as in the process of aging. The influence of ROS has some influence on the frequent occurrence of cardiovascular diseases (CVD) in diabetic patients. In this review, we considered the pathophysiological relationship between diabetes and CVD from the perspective of ROS. In addition, considering organ damage due to ROS elevation during ischemia–reperfusion, we discussed heart and lung injuries. Furthermore, we have focused on the transient receptor potential (TRP) channels and L-type calcium channels as molecular targets for ROS in ROS-induced tissue damages and have discussed about the pathophysiological mechanism of the injury.
At first glance, diabetes, which causes abnormal blood glucose control, and ischemia–reperfusion injury (IRI) of the heart, which causes myocardial infarction, seem to have nothing in common. However, both these diseases are consistent in that they cause inflammation with the release of cytokines and the responses of immune cells. These reactions are triggered by the oxidative stress (OS) that occurs in the body. Oxidative stress is defined as an imbalance between oxidants and anti-oxidants in favor of the oxidants (1). Reactive oxygen species (ROS) including hydrogen peroxide (H2O2) and superoxide (.) that are generated in the cells cause OS when they become excessive. Oxidative stress causes diseases such as diabetes (2), IRI (3), cancer (4), and Alzheimer's disease (5), and, notably, this condition is affected by diet and obesity (6).
While the organ heart has drawn much attention in the context of ischemic heart diseases, which is the leading cause of death among humans (7), IRI also occurs in several other organs such as the lung (8). In addition, transplantation of organs, such as lungs and kidneys, can result in IRI due to blood reperfusion in ischemic-isolated organs (9). While having their own specific mechanisms for the development of diseases, the pathological conditions of diabetes and IRI also share a common molecular basis in a series of intracellular signal transduction mechanisms originating from OS, as discussed in the present review. In addition to diabetes, extending the pathophysiology of IRI from the perspective of OS is meaningful to understand the diseases and development of preventive measures and treatments involved.
As the life-expectancy of diabetic patients has increased significantly, the cardiovascular complications of diabetes have become prominent. When compared with people without diabetes, people with type 2 diabetes (T2DM) are at an increased risk of cardiovascular diseases (CVD) (10). The increased production of ROS in the diabetic heart is an important factor in the occurrence and development of diabetic cardiomyopathy (11). Reactive oxygen species can induce the inactivation of the signaling mechanism between the insulin receptor and the glucose transport system, which can lead to insulin resistance (12). Meanwhile, diabetes is a producer of OS, which can lead to atherosclerosis (13, 14). We have explored the mechanisms by which T2DM triggers OS and increases the risk of CVD from the prospect of obesity, hyperglycemia, and intracellular calcium.
A recent study reported presence of differences in the factors causing OS in the hearts of obese and non-obese diabetic mice. In addition, the decreased expression of antioxidant molecules in the hearts of non-obese diabetic mice was reported to act as an important factor that leads to the development of heart diseases (15). In this study, Li et al. created two groups of T2DM mouse models: obese and non-obese groups. They found that obese T2DM mice demonstrated more severe heart remodeling and earlier contractile dysfunction than non-obese T2DM mice. In addition, obese T2DM mice revealed severe and persistent myocardial lipotoxicity, which was manifested by increased free fatty acids (FFA) uptake. Excessive FFA uptake activates the peroxisome proliferator-activated receptor alpha (PPARα) pathway and phosphorylate glycogen synthase kinase 3 beta (GSK-3β), while inhibiting glucose transporter 4 (GLUT4) and fatty triglyceride lipase (ATGL). Among the tissue damage caused by lipotoxicity, OS is the main factor (16). Under the effect of lipotoxicity, the tissues absorb a large amount of FFA, leading to excessive oxidation of FFA, a sharp increase in the amount of oxygen consumption, and excessive ROS production (17–20). In addition, excessive FFA and resultant oxidation lead to ceramide synthesis, which in turn leads to increased cardiomyocyte apoptosis through the mitochondrial pathway (20).
Another interesting mechanism by which obesity affects the development of atherosclerosis through OS is Na/K-ATPase. According to Krithika Srikanthan et al., activation of the Na/K-ATPase signal cascade exacerbates obesity, diabetes, dyslipidemia, and atherosclerosis, and these conditions are all related to the imbalance of OS (21). Na/K-ATPase is a scaffold and signaling protein, and is also involved in many clinical conditions, including CVD and chronic kidney disease (22, 23). Fat accumulation in humans and mice is related to systemic OS (24). The white adipose tissue of obese mice has a trend of increased expression of NADPH oxidase (NOX) and decreased expression of antioxidant enzymes (25, 26). In cultured adipocytes, the production of ROS was significantly increased during the differentiation of 3T3-L1 cells into adipocytes, indicating that the production of ROS increased simultaneously with the accumulation of fat in adipocytes (27). Besides, the increase in free fatty acid levels can induce ROS production through the activation of NOX (28). Furthermore, diet-induced OS can activate the Na/K-ATPase/Src/ROS amplification loop, leading to the occurrence and development of dyslipidemia and atherosclerosis (21).
The nuclear factor erythroid 2-related factor 2 (NRF2) pathway is closely related to antioxidant effects and is activated at the onset of OS (29). Li et al. reported that the expression level of NRF2 and its target genes heme oxygenase 1 (HO-1) and NAD(P)H quinone dehydrogenase 1 (NQO1) increased significantly in the heart of obese T2DM mice, but they decreased in the hearts of non-obese T2DM mice (15). This result implies that myocardial lipotoxicity and antioxidant pathway activation occur in obese T2DM patients. This finding may provide a new guidance for the prevention and clinical treatment of diabetic heart diseases.
Hyperglycemia (high levels of blood glucose) leads to increased production of ROS, which ultimately leads to vascular dysfunction (30). Meanwhile, OS from hyperglycemia leads to insufficient glucose uptake by muscles and fat cells. Furthermore, OS from hyperglycemia may promote β-cell dysfunction and reduce insulin secretion by β cells (13, 31). This event also leads to further aggravation of hyperglycemia. As a result, hyperglycemia and OS interact. It is therefore important to understand how to reduce OS so as to reduce hyperglycemia.
Another question that needs resolution is how does high blood sugar level trigger OS and lead to cardiovascular dysfunction. Under a hyperglycemic condition, ROS accumulates, damages DNA and proteins, and injures cardiomyocytes. The increase in ROS production caused by hyperglycemia occurs through the following ways: activation of the protein kinase C (PKC) pathway via diacylglycerol (DAG), increased hexosamine pathway flux, increased production of advanced glycation-end product, and increased flux in the polyol pathway (32, 33). During the ROS production in the polyol pathway, when aldose reductase reduces glucose to sorbitol, excess glucose enters the polyol pathway (Figure 1) (34). This reaction oxidizes NADPH to NADP+, consuming NADPH (34). As NADPH is essential for antioxidant regeneration, the decrease in the amount of NAPDH leads to the facilitation of OS.
Figure 1. Development of atherosclerosis via ROS production in the polyol pathway in the condition of hyperglycemia. In the process of the reduction of glucose to sorbitol by aldose reductase, NADPH is oxidized to NADP+, consuming NADPH. As NADPH is essential for regeneration of antioxidant glutathione (GSH), the reaction of reducing H2O2 to H2O is suppressed. The accumulation of H2O2 causes inflammation, resulting in the development of atherosclerosis. GSSG, glutathione disulfide.
Simultaneously, the accumulation of ROS caused by hyperglycemia triggers insulin resistance (13, 35, 36). Insulin resistance occurs when the cells in the muscles, fat, and liver do not respond appropriately to insulin and cannot uptake glucose from the blood for deriving energy (37). In response, the pancreas produce more insulin (37). Interestingly, insulin resistance is a component of T2DM, high blood pressure, and dyslipidemia; these characteristics together constitute a major risk of CVD (38).
Past studies have reported that mitochondrial OS is related to insulin resistance (39). Therefore, under high blood sugar level conditions, the mitochondria are active and produce more ROS (40). Elevated ROS levels can induce mitochondrial division, which in turn affects the insulin-PI3K-AKT pathway and GLUT4 (12). Glucose transporter 4 is the main glucose transporter (41) in the skeletal muscles and adipose tissue. The cells respond to insulin by increasing the expression of GLUT4 in the plasma membrane, thereby increasing the cellular uptake of blood glucose. When the glucose level is high, the body produces insulin, which then activates the PI3K/AKT pathway (42). Mitochondrial fission is directly related to insulin resistance of the skeletal muscles (43). Past studies have also demonstrated that restricting mitochondrial overactivation can prevent insulin resistance (44). In addition, insulin resistance caused by mitochondrial dysfunction may lead to metabolic and cardiovascular abnormalities, thereby increasing the incidence of CVD (38, 45). In summary, OS caused by hyperglycemia plays an important role in cardiovascular dysfunction and both the conditions interact with and influence each other.
Redox regulation of calcium-handling proteins directly affects cardiac contraction by changing intracellular calcium concentration (46). As discussed earlier, hyperglycemia in the cells can lead to excessive ROS production. The increase in the ROS level can inhibit autonomic ganglion synaptic transmission by oxidizing the α3 subunit of nicotinic acetylcholine receptor, which may in turn result in fatal arrhythmia (47). At the same time, ROS leads to sudden death of a diabetic patient after myocardial infarction by increasing post-translational protein modification, which leads to the downregulation of Ca2+-ATPase transcription in the sarcoplasmic reticulum.
Ventricular contraction and relaxation are mainly controlled by the release and uptake of Ca2+ by the sarcoplasmic reticulum Ca2+-ATPase 2 (SERCA2) pump (48, 49). In hypertrophic and failing myocardium, the level of SERCA2 protein and its ability to absorb Ca2+ are inhibited. Reactive oxygen species can oxidize and directly enhance CaMKII activity, which in turn phosphorylates and activates several Ca2+-handling proteins such as the cardiac ryanodine receptor RyR2 or cardiac SERCA (50).
Protein O-linked-N-acetylglucosaminylation (O-GlcNAcylation) plays important roles in calcium handling under diabetic conditions (Figure 2). For example, hyperglycemia increases the O-GlcNAc modification of calcium/calmodulin-dependent protein kinase IIδ (CaMKIIδ), which in turn leads to the autonomous activation of CaMKII (51, 52). Furthermore, the hyperglycemia-induced O-GlcNAcylation of CaMKII causes ROS production by NOX2 (53). Autonomous activation of CaMKII can lead to decreased cardiac contractility and potential fatal arrhythmias, such as ventricular premature beats and delayed depolarization. In fact, delayed depolarization is related to long QT interval arrhythmia (54). On the other hand, in the chronic hyperglycemia condition in diabetes, O-GlcNAc transferase reduces the transcription of SERCA2, which results in decreased calcium reuptake and impaired relaxation (55). The overexpression of GlcNAcase or the inhibition of GlcNAc modification increases the expression of SERCA2a, the ablated sarcoplasmic reticulum Ca2+ leakage, improved cardiac contractility, and reduced arrhythmia events (56).
Figure 2. Calcium handling in cardiomyocytes in the condition of hyperglycemia. Hyperglycemia causes modification of CaMKII by O-linked N-acetylgulcosamine (O-GlcNAcylation). This modification facilitates ROS production via NOX2. ROS enhances CaMKII activity by oxidation. CaMKII phosphorylates RyR2 and SERCA. On the other hand, hyperglycemia induces GlcNAcylation of the transcription factor Sp1, reducing the transcription of SERCA2. CaMKII, Ca2+/calmodulin-dependent protein kinase II; ROS, reactive oxygen species; NOX2, NADPH oxidase 2; RyR2, ryanodine receptor 2; SERCA, sarcoplasmic reticulum Ca2+-ATPase 2.
In summary, calcium plays an important role in cardiac dysfunction caused by ROS derived under the condition of hyperglycemia.
ischemia–reperfusion injury is a type of tissue damage that occurs when the blood flows back to the tissue after a period of ischemia or under the lack of oxygen. IRI is often detected in cases of organ transplants, major organ resections, and shock. The main organs in which IRI occurs are the heart, lung, brain, liver, kidney, and intestine (57–62). This finding contributes to morbidity and mortality occurring in a variety of pathologies, such as myocardial infarction and stroke caused by coronary atherosclerosis (63).
ischemia–reperfusion is often associated with microvascular injury, especially due to increased permeability of the capillaries and arterioles, which lead to increased interstitial diffusion and fluid filtration across the tissues. After ischemia, the re-entry of blood into the tissue induces the release of large amounts of oxygen free radicals. These free radicals trigger enzymatic reactions, leading to oxidative damage to the cell membranes as well as the production of toxic metabolites and cell injury involving DNA, proteins, and lipids (63, 64).
Interestingly, the common factor between diabetes, as discussed in the previous section, and IRI is that OS affects the deterioration of the pathological processes, including inflammation. During IRI, the damaged tissues produce excessive amounts of ROS, causing the release of proinflammatory cytokines and apoptosis (64–66). After myocardial ischemia, cardiac surgery, cardiogenic shock, or circulatory arrest, myocardial IRI can lead to adverse cardiac events. Although it is necessary to restore the blood flow to nourish the cells, reperfusion is known for its harmful effects because of OS and the subsequent development of intense inflammation and immune responses (67–75). The following subsections discuss the role of the three molecules involved in the development of IRI.
Innate immune response to invading pathogens, which is derived from the toll receptors, is shared extensively among insects and vertebrates (76). Toll-like receptor 4 (TLR4) binds to various types of ligands such as lipopolysaccharides (LPS), low-density lipoproteins, and heat-shock proteins (77, 78). Among the toll-like receptors (TLRs) consisting of 11 subtypes in humans, TLR2 and TLR4, predominantly TLR4, are involved in the development of IRI (79). The TLR4-signaling pathway is an important inflammatory cascade in IRI with essential functions in the adaptive immune system (80, 81). Toll-like receptor 4 responds to endogenous molecules during the sterile inflammatory processes such as IRI (82) and is considered as the key regulator in several ischemia–reperfusion models.
As discussed earlier, OS is critically involved in the pathogenesis of IRI. In fact, ROS facilitates TLR4 trafficking to the plasma membrane, thereby promoting the TLR4 activity (83, 84). This event implies that the pathogenesis of IRI is at least partly attributable to the effect of ROS on the TLR4 activation. Furthermore, Pahwa et al. postulate that ROS act as a potential activator of TLRs and that hyperglycemia-induced OS activates TLRs, subsequently inducing inflammatory responses in diabetes (85).
The activations of TLR2, TLR3, and TLR4 increases oxidation levels of lipids and proteins (86). In addition to the TLR4 activation by ROS mentioned earlier, the relationship between ROS and TLR4 includes ROS production through the TLR4 activation. For example, TLR4 activation induced by LPS facilitate intracellular ROS production via NOX-4 (87). In TLR4-deficient mice, the ROS generation is reduced (88).
NF-κB initiates and disseminates innate immune responses by regulating the gene pools that encode proinflammatory/inflammatory cytokines (i.e., TNF-α, IL-1β, IL-6, and granulocyte/macrophage-colony stimulating factor), adhesion molecules (i.e., vascular cell adhesion molecule-1, intercellular adhesion molecule-1, and E-selectin), and chemokines (e.g., IL-8, regulated by the activation of normal T-cells expressed and secreted, MIP-1α, and MCP-1) (89, 90). The activation of TLR4, which forms a complex with several proteins such as CD14, myeloid differentiation primary response 88 (MyD88), and tumor necrosis factor receptor-associated factor 6 (TRAF6), leads to NF-κB activation (91–93). Reactive oxygen species acts on this TLR4/NF-κB pathway and further facilitates the NF-κB activation (94). Ischemia–reperfusion also leads to NF-κB activation (95).
The TLR4/NF-κB pathway is involved in the development of myocardial IRI. TLR4, initially detected in monocytes, is also expressed in other tissues, including the heart (76). Moreover, TLR4 is strongly expressed in injured myocardium (96). MAPKs, such as p38 and c-Jun NH2-terminal kinase (JNK), are activated during myocardial IRI (97), which in turn induces an acute inflammatory reaction. According to Lee et al., ROS produced by NOX-2/4 causes MAPK activation (98). TLR4-deficient mice have significantly less myocardial injury, as characterized by the reduction in the myocardial infarction area, decrease in the JNK and NF-κB activation, as well as reduction in the mRNA expression of inflammatory cytokines, such as IL-1β, IL-6, and MCP-1 (99).
The TLR4/NF-κB pathway is also involved in the development of IRI in other organs. The deletion of TLR4 or pharmacological antagonists reduces the severity of IRI in cardiac, hepatic, renal, and pulmonary models (99–108). In case of the lung IRI, the levels of phosphorylated JNK and NF-κB are diminished in TLR4-deficient mice (106, 108). Two pathways that possibly get activated during the lung IRI are apoptosis, induced by the activation of a transcriptional program controlled by NF-κB and acute inflammation promoted by the activation of resident alveolar macrophages and the expression of several proinflammatory cytokines and chemokines, such as TNF-α, IL-1β, IL-8, and macrophage inflammatory protein 2 (MIP-2) (109). The markers of lung injury, including permeability index, myeloperoxidase content, and bronchoalveolar lavage inflammatory cell counts were all decreased with TLR4 knockdown. The TLR4 knockdown in alveolar macrophages resulted in almost complete weakening of the lung IRI. The protective effect of TLR4 knockdown appears to be partly mediated by the significant reduction in pre-transcriptional signaling through MAPKs phosphorylation and possibly due to the nuclear translocation of transcription factors, such as NF-κB and activator protein-1 (107, 110).
Dipeptidyl peptidase-4 (DPP4), also known as CD26, is a cell-surface protease offers a wide range of biological functions. As a serine-type protease, DPP4 cleaves dipeptides from the N-terminus, with proline residues in the penultimate position (111, 112). Clinical and experimental study over the past 30 years has clearly demonstrated that the DPP4/CD26 pathway is involved in a variety of physiological processes and immune system diseases (113). In addition, DPP4/CD26 transmembrane glycoproteins are expressed not only by various cells of the immune system but also by the epithelial and systemic vascular endothelial cells, by the endothelial cells of venules and capillaries, by the cells of the heart, kidney, lung, pancreas, spleen, and small intestine, by the vascular smooth muscle cells, and by monocytes and hepatocytes; moreover, it is soluble in the plasma (111, 114, 115).
DPP4 lyses multiple peptide substrates, including the incretin hormone glucagon-like peptide-1 (GLP-1) (116). Glucagon-like peptide-1 inhibits OS generation and the subsequent inflammation (117–119). For example, GLP-1 exerts antioxidant effects via cyclic adenosine monophosphate (cAMP), phosphoinositide 3-kinase (PI3K), and protein kinase C-delta (PKCδ) pathways in diabetes (120). Dipeptidyl peptidase-4 inhibitors prolong the bioavailability of the endogenously secreted GLP-1, thereby exerting a beneficial therapeutic effect on diabetes (116, 121).
In addition to its involvement in the development of diabetes, accumulating evidence indicates the role of DPP4 in IRI (122). Dipeptidyl peptidase-4 deficiency preserves cardiac functions via GLP-1 signaling in myocardial IRI (123). In this regard, cardiomyocytes deficient in DPP4 are resistant to H2O2-induced cell death by activating the AKT signaling (124). Dipeptidyl peptidase-4 inhibitors reduce myocardial infarct size, improve the cardiac function, and promote the myocardial regeneration (125). The involvement of GLP-1 signaling in the preservation of cardiac functions has been confirmed in various animal model experiments, such as heart failure and myocardial infarction (123, 126–129). Glucagon-like peptide-1 inhibits apoptosis or necrosis of endothelial cells (118) and cardiomyocytes (130). Glucagon-like peptide-1-based therapies play an important role in the protection from myocardial IRI (127, 131–133).
The lung is the second-highest expressed organ of DDP4 in rats (134). Dipeptidyl peptidase-4 can directly affect the dynamics of lung inflammation and may itself act as a proinflammatory signaling molecule (135, 136). In the lung, the capillaries may act as the main source of DPP4 activity, while the submucosal serous gland and alveolar cells also express DPP4 (111). Similar to the case of myocardial IRI, GLP-1 is believed to exert a protective effect also in the lung IRI by suppressing the production of OS (137).
The presence of excessive free heme facilitates ROS formation, thereby leading to abnormal endothelial cell function, as observed in systemic hypertension, diabetes, and IRI (19384082). HO is important to reduce the production of ROS (138). Specifically, HO possesses the ability to degrade heme and produce carbon monoxide (CO), a heme ligand, and biliverdin, an antioxidant (139). Human HO exists in three isoforms, HO-1, HO-2, and HO-3. Among these, HO-1 is involved in exerting protective effect against IRI.
The expression of HO-1 is modulated by the transcription factor NRF2, as discussed in Section Obesity plays an important role in heart disease of diabetic patients. NRF2, which translocated to the nucleus under OS, activates antioxidant response element and increases the transcription of antioxidant genes, including HO-1 (140). The HO-1 system includes four main functions: (1) antioxidant function; (2) maintenance of microcirculation; (3) regulation of cell cycle; and (4) anti-inflammatory function (141). Overexpression of HO-1 exerts a potent cellular protective effect in rat heart ischemia–reperfusion models. HO-1 can reduce IRI due to the enhanced antioxidant and anti-apoptotic activities (142, 143).
Moreover, HO-1 possesses antiapoptotic outcomes. These effects get mediated through the p38 MAPK-signaling transduction pathway activated by CO (144). In addition, CO-exposed animals, at least partially, demonstrate a significant reduction in hyperoxia-induced lung apoptosis through the anti-inflammatory MKK3/P38 MAPK pathway (144). Three major MAPKs in cardiomyocytes are affected by the ischemia–reperfusion, and the ERK pathway may be critical for cell survival by protecting the cells from programmed cell death caused by stress-induced activation of p38 and JNK (145).
The transient receptor potential (TRP) melastatin (TRPM) subfamily belongs to the TRP cation channel superfamily, and most of its members either have calcium ion permeability or are calcium ion activating proteins (146, 147). Changes in the concentration of Ca2+/Mg2+ in cells or changes in the cell membrane potential and electrical activity can affect various biological processes, including the cellular OS level (148), endothelial cell permeability (149), and cell death (150). Therefore, in the past 10 years, the members of this family have attracted more and more interest and attention to CVD (151, 152), T2DM (153), and inflammation (154). The activity of some members of the TRPM subfamily is regulated by OS (155). Therefore, the emergence of OS-regulated ion channels in an oxidative environment creates favorable conditions for disease development.
TRPM4 is widely expressed in various tissues (156–159), including the atria and ventricles in both rodents (160, 161) and human (162, 163).
With the increase of OS, the TRPM4 channel functions abnormally, which promotes the onset and development of the disease. To verify this point, it became necessary to create an ischemic and hypoxic cellular environment. Presently, cobalt chloride (CoCl2) (164) and H2O2 (165, 166), in a laboratory setting, are widely used to establish OS models and fully characterized chemical agents. CoCl2 can be used to establish a simple in vitro model of hypoxic/ischemic disease in the laboratory, but up to now, there are few studies on TRPM4 channel induced by CoCl2. The possible reason is that CoCl2 can induce the production of ROSs, but also affect the expression of some genes, such as HIF-1α, p53, p21, and PCNA (167–169). CoCl2 may also affect the remodeling of CMs in hypoxic/ischemic area by activating PI3K/Akt and MAPK pathways (170), and CoCl2-induced apoptosis may be related to mitochondria-mediated apoptosis pathway (171). Hydrogen peroxide increases the activity of TRPM4 (172), while ATP and ADP inhibit its activity (173). When ATP production in hypoxia is insufficient, cardiomyocytes activates the KATP channels (174) and cause cell hyperpolarization, thereby preventing arrhythmia. However, this process may be affected by electrical disturbances induced by TRPM4 protein, because the channel is sensitive to Ca2+ and ATP (175, 176). Meanwhile, our previous research results (166) demonstrated that TRPM4 is involved in the death of cardiomyocytes mediated by H2O2. At higher concentrations, H2O2 increases cell death in a concentration-dependent manner, while 9-phenanthrol (9-Phe) can partially reverse H2O2-induced cell death. The reversal effect is probably the result of 9-Phe's direct effect on the TRPM4 channel (166, 177, 178).
Unlike TRPM4, TRPM2 is a cation channel permeable to Ca2+ (179). TRPM2 also plays an important role in cell proliferation and survival (180). It is widely distributed and sensitive to OS (181). However, at present, there is little information available on the physiological and pathophysiological functions of TRPM2 in the heart. Early studies of the TRPM2 channel function support the observation that TRPM2 activation induces cell death by continuously increasing the [Ca2+]i (182– 184).
Mitochondrial integrity is critical to the survival and function of cardiomyocytes and is essential for maintaining the high-energy requirements of cardiomyocytes. Ca2+ overload can lead to mitochondrial permeability transition (MPT), but Ca2+ overload is the result of bioenergy failure after MPT occurs following myocardial ischemia–reperfusion (185). This result can be corroborated from the study of Davidson et al. (186). In Langendorff-perfused mouse hearts, MitoQ, a mitochondrial-targeted scavenger of ROS, could significantly reduce the Ca2+ wave-related mPTP opening. The mitochondria can thus benefit from the calcium influx mediated by TRPM2 to reduce the mitochondrial ROS production (179).
The heart consumes an equivalent of 6 kg of ATP per day, most of which is produced through mitochondrial oxidative phosphorylation (187). Myocardial ischemia consumes a large amount of ATP and produces a large amount of ROS; this process reduces mitochondrial biogenesis and mitochondrial dysfunction, ultimately leading to cell death (39, 188). However, the results of a study showed (189) that TRPM2 can rescue the ATP levels in the cells. During OS, TRPM2 maintains cell survival after OS by regulating the antioxidant pathway and cofactors that are regulated by NRF2.
Moreover, the TRPM2 channels can protect cardiomyocytes from IRI (181), which may be due to the Ca2+ flux mediated by TRPM2 that enhances the activity of calcineurin and the stability of hypoxia-inducible factor (HIF) (190). In immune cells, the NOX activity depends on membrane depolarization (191) when the TRPM2 channel is activated and it inhibits the production of ROS. TRPM2-mediated calcium influx can reduce the production of ROS through the depolarization of the plasma membrane of immune cells and the negative feedback regulation of ROS production (192). This event contributes to cell functions such as cytokine production, insulin release, cell motility, and cell death (193).
Pulmonary circulation is characterized by low resistance and low pressure, and the mean pulmonary arterial pressure (mPAP) is <20 mmHg (194). Hypoxic pulmonary vasoconstriction (HPV) is a physiological response of the arterioles. However, there is usually no obvious effect on the pulmonary arterial pressure during HPV on limiting the hypoxia area (195). Persistent hypoxia induces pulmonary vasoconstriction and vascular remodeling mediated by the contraction and proliferation of pulmonary artery smooth muscle cells (PASMC), which eventually led to pulmonary hypertension (PH) (196). Pulmonary hypertension associated with hypoxia belongs to the third group in the classification of PH (194). Although there is no unified view yet on this association, hypoxia could increase the level of ROS in PASMC (197–205).
Excessive ROS is considered to be the main factor of arterial remodeling in PH induced by chronic hypoxia (CH) (206, 207). The specific mechanism of ROS promoting PH has not been clarified yet, but it is evident that ROS plays an important role in CH-induced PH vasoconstriction. Abnormal voltage-dependent Ca2+ influx is considered to be related to the pathogenesis of hypoxic PH (HPH) (208). In PASMC, cytosolic Ca2+ concentration ([Ca2+]cyt) is regulated by two pathways: voltage-dependent Ca2+ influx and voltage-independent Ca2+ influx. The influx of Ca2+ through L-type voltage-gated calcium channels (VGCC) is an important [Ca2+]cyt regulatory pathway in HPH. Nifedipine and verapamil, which are L-type VGCC antagonists, can prevent HPV, inhibit PASMC proliferation, and alleviate HPH (208–211). L-type VGCC belongs to one of the calcium ion channels, which is a polymer transmembrane protein complex composed of five subunits of α1, α2, δ, β, and γ. Here α1 is the main functional subunit, while the others are auxiliary subunits. There are four subtypes of α1: α1S (Cav1.1), α1C (Cav1.2), α1D (Cav1.3), and α1F (Cav1.4) (212). Cav1.2 was upregulated, while L-type VGCC could functionally enhance pulmonary vasoconstriction associated with Ca2+ influx in PASMCs after CH exposure (213).
The existing pharmacological data indicates that L-type VGCC plays an important role in the increase of [Ca2+]i in PASMC induced by acute O2 tension (214–218). Experiments are hence necessary to investigate the effects of specific inhibitors (such as mibefradil) of T-type VGCC to determine their role in maintaining [Ca2+]i during hypoxia, although mounting evidence have demonstrated that the application of H2O2 (219– 221) and oxidized glutathione (GSSG) (222, 223) resulted in Ca2+ influx through L-type VGCC. In addition, the possibility of channel opening and inward Ca2+ currents are increased by Cav1.2 subunit of L-type VGCC, which was glutathionylated by H2O2 and GSSG in subsequent studies (222, 223). Moreover, Ca2+ signaling contributed to the contraction of PA (224). Furthermore, L-type VGCC has been reported to be sensitive to plasma membrane depolarization (225). Interestingly, vasoconstrictor endothelin-1 (ET-1) can stimulate L-type VGCC-mediated increase of Ca2+ in PASMCs of CH Wistar rats through the PKC and Rho kinase-dependent ways (226, 227). This situation is not difficult to understand, because both PKC (228) and Rho kinase (229) can be activated by oxidation to regulate this process. An indirect evidence of this finding is that ET-1 could increase the production of ROS in PASMCs (230–232). This hypothesis has not been tested in pulmonary circulation, but the activation of L-type VGCC induced by ET-1 in isolated cardiomyocytes is now known to be mediated by. (233).
Oxidative stress is based on the balance between oxidant and antioxidant activities derived from numerous molecules and pathways. In this review, we discussed ROS production in hyperglycemia under diabetic conditions, and, interestingly, the effect of obesity on it. Moreover, OS affects calcium handling via SERCA2 and CaMKII, thereby exacerbating cardiac functions in diabetes. In this way, OS is involved in the effects of diabetes on CVD. Moreover, a common mechanism is involved in the pathology of diabetes and IRI. For example, the OS-induced inflammation basically shares the common mechanism of TLR4/NF-κB and TLR4/MAPK pathways in diabetes and IRI. In addition, the DPP4/GLP-1 and NRF2/HO-1 systems are involved in ROS scavenging in diabetes and IRI. We also discussed the effect of OS on the activities of ion channels, such as TRPM2, TRPM4, and L-type VGCC, and their implications with diseases, including IRI. Further understanding of these mechanisms is expected to promote the development of new strategies for the prevention and cure of these formidable diseases.
All authors listed have made a substantial, direct and intellectual contribution to the work, and approved it for publication.
This research was funded by JSPS KAKENHI, Fund for the Promotion of Joint International Research (Fostering Joint International Research), 17KK0168 and JSPS KAKENHI Grant-In-Aid for Scientific Research (B), 20H04518.
The authors declare that the research was conducted in the absence of any commercial or financial relationships that could be construed as a potential conflict of interest.
1. Sies H. Oxidative stress: a concept in redox biology and medicine. Redox Biol. (2015) 4:180–3. doi: 10.1016/j.redox.2015.01.002
2. Newsholme P, Cruzat VF, Keane KN, Carlessi R, de Bittencourt PI, Jr. Molecular mechanisms of ROS production and oxidative stress in diabetes. Biochem J. (2016) 473:4527–50. doi: 10.1042/BCJ20160503C
3. Cadenas S. ROS and redox signaling in myocardial ischemia-reperfusion injury and cardioprotection. Free Radic Biol Med. (2018) 117:76–89. doi: 10.1016/j.freeradbiomed.2018.01.024
4. Reuter S, Gupta SC, Chaturvedi MM, Aggarwal BB. Oxidative stress, inflammation, and cancer: how are they linked? Free Radic Biol Med. (2010) 49:1603–16. doi: 10.1016/j.freeradbiomed.2010.09.006
5. Tonnies E, Trushina E. Oxidative stress, synaptic dysfunction, and Alzheimer's disease. J Alzheimers Dis. (2017) 57:1105–21. doi: 10.3233/JAD-161088
6. Bjorklund G, Chirumbolo S. Role of oxidative stress and antioxidants in daily nutrition and human health. Nutrition. (2017) 33:311–21. doi: 10.1016/j.nut.2016.07.018
7. Nowbar AN, Gitto M, Howard JP, Francis DP, Al-Lamee R. Mortality from ischemic heart disease. Circ Cardiovasc Qual Outcomes. (2019) 12:e005375. doi: 10.1161/CIRCOUTCOMES.118.005375
8. Laubach VE, Sharma AK. Mechanisms of lung ischemia-reperfusion injury. Curr Opin Organ Transplant. (2016) 21:246–52. doi: 10.1097/MOT.0000000000000304
9. Nieuwenhuijs-Moeke GJ, Pischke SE, Berger SP, Sanders JSF, Pol RA, Struys M, et al. Ischemia and reperfusion injury in kidney transplantation: relevant mechanisms in injury and repair. J Clin Med. (2020) 9;253. doi: 10.3390/jcm9010253
10. Martin-Timon I, Sevillano-Collantes C, Segura-Galindo A, Del Canizo-Gomez FJ. Type 2 diabetes and cardiovascular disease: have all risk factors the same strength? World J Diabetes. (2014) 5:444–70. doi: 10.4239/wjd.v5.i4.444
11. Kaludercic N, Di Lisa F. Mitochondrial ROS formation in the pathogenesis of diabetic cardiomyopathy. Front Cardiovasc Med. (2020) 7:12. doi: 10.3389/fcvm.2020.00012
12. Boucher J, Kleinridders A, Kahn CR. Insulin receptor signaling in normal and insulin-resistant states. Cold Spring Harb Perspect Biol. (2014) 6:a009191. doi: 10.1101/cshperspect.a009191
13. Tangvarasittichai S. Oxidative stress, insulin resistance, dyslipidemia and type 2 diabetes mellitus. World J Diabetes. (2015) 6:456–80. doi: 10.4239/wjd.v6.i3.456
14. Yuan T, Yang T, Chen H, Fu D, Hu Y, Wang J, et al. New insights into oxidative stress and inflammation during diabetes mellitus-accelerated atherosclerosis. Redox Biol. (2019) 20:247–60. doi: 10.1016/j.redox.2018.09.025
15. Li X, Wu Y, Zhao J, Wang H, Tan J, Yang M, et al. Distinct cardiac energy metabolism and oxidative stress adaptations between obese and non-obese type 2 diabetes mellitus. Theranostics. (2020) 10:2675–95. doi: 10.7150/thno.40735
16. Hauck AK, Bernlohr DA. Oxidative stress and lipotoxicity. J Lipid Res. (2016) 57:1976–86. doi: 10.1194/jlr.R066597
17. A ISS, C AB, A JS. Changes in plasma free fatty acids associated with type-2 diabetes. Nutrients. (2019) 11:2022. doi: 10.3390/nu11092022
18. Lytrivi M, Castell AL, Poitout V, Cnop M. Recent insights into mechanisms of beta-cell lipo- and glucolipotoxicity in type 2 diabetes. J Mol Biol. (2020) 432:1514–34. doi: 10.1016/j.jmb.2019.09.016
19. Senoner T, Dichtl W. Oxidative stress in cardiovascular diseases: still a therapeutic target? Nutrients. (2019) 11:2090. doi: 10.3390/nu11092090
20. Moris D, Spartalis M, Spartalis E, Karachaliou GS, Karaolanis GI, Tsourouflis G, et al. The role of reactive oxygen species in the pathophysiology of cardiovascular diseases and the clinical significance of myocardial redox. Ann Transl Med. (2017) 5:326. doi: 10.21037/atm.2017.06.27
21. Srikanthan K, Shapiro JI, Sodhi K. The role of Na/K-ATPase signaling in oxidative stress related to obesity and cardiovascular disease. Molecules. (2016) 21:1772. doi: 10.3390/molecules21091172
22. Wang X, Liu J, Drummond CA, Shapiro JI. Sodium potassium adenosine triphosphatase (Na/K-ATPase) as a therapeutic target for uremic cardiomyopathy. Expert Opin Ther Targets. (2017) 21:531–41. doi: 10.1080/14728222.2017.1311864
23. Yan Y, Shapiro JI. The physiological and clinical importance of sodium potassium ATPase in cardiovascular diseases. Curr Opin Pharmacol. (2016) 27:43–9. doi: 10.1016/j.coph.2016.01.009
24. Furukawa S, Fujita T, Shimabukuro M, Iwaki M, Yamada Y, Nakajima Y, et al. Increased oxidative stress in obesity and its impact on metabolic syndrome. J Clin Invest. (2004) 114:1752–61. doi: 10.1172/JCI21625
25. Sakurai T, Ogasawara J, Shirato K, Izawa T, Oh-Ishi S, Ishibashi Y, et al. Exercise training attenuates the dysregulated expression of adipokines and oxidative stress in white adipose tissue. Oxid Med Cell Longev. (2017) 2017:9410954. doi: 10.1155/2017/9410954
26. Hafstad AD, Hansen SS, Lund J, Santos CXC, Boardman NT, Shah AM, et al. NADPH oxidase 2 mediates myocardial oxygen wasting in obesity. Antioxidants (Basel). (2020) 9:171. doi: 10.3390/antiox9020171
27. Lee O-H, Kwon Y-I, Hong H-D, Park C-S, Lee B-Y, Kim Y-C. Production of reactive oxygen species and changes in antioxidant enzyme activities during differentiation of 3T3-L1 adipocyte. J. Korean Soc. Appl. Biol. Chem. (2009) 52:70–5. doi: 10.3839/jksabc.2009.012
28. Inoguchi T, Li P, Umeda F, Yu HY, Kakimoto M, Imamura M, et al. High glucose level and free fatty acid stimulate reactive oxygen species production through protein kinase C–dependent activation of NAD(P)H oxidase in cultured vascular cells. Diabetes. (2000) 49:1939–45. doi: 10.2337/diabetes.49.11.1939
29. Ma Q. Role of nrf2 in oxidative stress and toxicity. Annu Rev Pharmacol Toxicol. (2013) 53:401–26. doi: 10.1146/annurev-pharmtox-011112-140320
30. Volpe CMO, Villar-Delfino PH, Dos Anjos PMF, Nogueira-Machado JA. Cellular death, reactive oxygen species (ROS) and diabetic complications. Cell Death Dis. (2018) 9:119. doi: 10.1038/s41419-017-0135-z
31. Newsholme P, Keane KN, Carlessi R, Cruzat V. Oxidative stress pathways in pancreatic beta-cells and insulin-sensitive cells and tissues: importance to cell metabolism, function, and dysfunction. Am J Physiol Cell Physiol. (2019) 317:C420–C33. doi: 10.1152/ajpcell.00141.2019
32. Giacco F, Brownlee M. Oxidative stress and diabetic complications. Circ Res. (2010) 107:1058–70. doi: 10.1161/CIRCRESAHA.110.223545
33. Safi SZ, Qvist R, Kumar S, Batumalaie K, Ismail IS. Molecular mechanisms of diabetic retinopathy, general preventive strategies, and novel therapeutic targets. Biomed Res Int. (2014) 2014:801269. doi: 10.1155/2014/801269
34. Brownlee M. The pathobiology of diabetic complications: a unifying mechanism. Diabetes. (2005) 54:1615–25. doi: 10.2337/diabetes.54.6.1615
35. Houstis N, Rosen ED, Lander ES. Reactive oxygen species have a causal role in multiple forms of insulin resistance. Nature. (2006) 440:944–8. doi: 10.1038/nature04634
36. Pitocco D, Tesauro M, Alessandro R, Ghirlanda G, Cardillo C. Oxidative stress in diabetes: implications for vascular and other complications. Int J Mol Sci. (2013) 14:21525–50. doi: 10.3390/ijms141121525
37. Lebovitz HE. Insulin resistance: definition and consequences. Exp Clin Endocrinol Diabetes. (2001) 109(Suppl 2):S135–48. doi: 10.1055/s-2001-18576
38. Ormazabal V, Nair S, Elfeky O, Aguayo C, Salomon C, Zuniga FA. Association between insulin resistance and the development of cardiovascular disease. Cardiovasc Diabetol. (2018) 17:122. doi: 10.1186/s12933-018-0762-4
39. Kim JA, Wei Y, Sowers JR. Role of mitochondrial dysfunction in insulin resistance. Circ Res. (2008) 102:401–14. doi: 10.1161/CIRCRESAHA.107.165472
40. Henriksen EJ, Diamond-Stanic MK, Marchionne EM. Oxidative stress and the etiology of insulin resistance and type 2 diabetes. Free Radic Biol Med. (2011) 51:993–9. doi: 10.1016/j.freeradbiomed.2010.12.005
41. Blanco CL, McGill-Vargas LL, Gastaldelli A, Seidner SR, McCurnin DC, Leland MM, et al. Peripheral insulin resistance and impaired insulin signaling contribute to abnormal glucose metabolism in preterm baboons. Endocrinology. (2015) 156:813–23. doi: 10.1210/en.2014-1757
42. Huang X, Liu G, Guo J, Su Z. The PI3K/AKT pathway in obesity and type 2 diabetes. Int J Biol Sci. (2018) 14:1483–96. doi: 10.7150/ijbs.27173
43. Jheng HF, Tsai PJ, Guo SM, Kuo LH, Chang CS, Su IJ, et al. Mitochondrial fission contributes to mitochondrial dysfunction and insulin resistance in skeletal muscle. Mol Cell Biol. (2012) 32:309–19. doi: 10.1128/MCB.05603-11
44. Matsuda M, Shimomura I. Increased oxidative stress in obesity: implications for metabolic syndrome, diabetes, hypertension, dyslipidemia, atherosclerosis, and cancer. Obes Res Clin Pract. (2013) 7:e330–41. doi: 10.1016/j.orcp.2013.05.004
45. Burgos-Moron E, Abad-Jimenez Z, Maranon AM, Iannantuoni F, Escribano-Lopez I, Lopez-Domenech S, et al. Relationship between oxidative stress, er stress, and inflammation in type 2 diabetes: the battle continues. J Clin Med. (2019) 8:1385. doi: 10.3390/jcm8091385
46. Steinberg SF. Oxidative stress and sarcomeric proteins. Circ Res. (2013) 112:393–405. doi: 10.1161/CIRCRESAHA.111.300496
47. Shah MS, Brownlee M. Molecular and cellular mechanisms of cardiovascular disorders in diabetes. Circ Res. (2016) 118:1808–29. doi: 10.1161/CIRCRESAHA.116.306923
48. Nelson BR, Makarewich CA, Anderson DM, Winders BR, Troupes CD, Wu F, et al. A peptide encoded by a transcript annotated as long noncoding RNA enhances SERCA activity in muscle. Science. (2016) 351:271–5. doi: 10.1126/science.aad4076
49. Venetucci LA, Trafford AW, O'Neill SC, Eisner DA. The sarcoplasmic reticulum and arrhythmogenic calcium release. Cardiovasc Res. (2008) 77:285–92. doi: 10.1093/cvr/cvm009
50. Erickson JR, Joiner ML, Guan X, Kutschke W, Yang J, Oddis CV, et al. A dynamic pathway for calcium-independent activation of CaMKII by methionine oxidation. Cell. (2008) 133:462–74. doi: 10.1016/j.cell.2008.02.048
51. Nishio S, Teshima Y, Takahashi N, Thuc LC, Saito S, Fukui A, et al. Activation of CaMKII as a key regulator of reactive oxygen species production in diabetic rat heart. J Mol Cell Cardiol. (2012) 52:1103–11. doi: 10.1016/j.yjmcc.2012.02.006
52. Singer HA. Ca2+/calmodulin-dependent protein kinase II function in vascular remodelling. J Physiol. (2012) 590:1349–56. doi: 10.1113/jphysiol.2011.222232
53. Lu S, Liao Z, Lu X, Katschinski DM, Mercola M, Chen J, et al. Hyperglycemia acutely increases cytosolic reactive oxygen species via O-linked GlcNAcylation and CaMKII activation in mouse ventricular myocytes. Circ Res. (2020) 126:e80–96. doi: 10.1161/CIRCRESAHA.119.316288
54. Landstrom AP, Dobrev D, Wehrens XHT. Calcium signaling and cardiac arrhythmias. Circ Res. (2017) 120:1969–93. doi: 10.1161/CIRCRESAHA.117.310083
55. Marsh SA, Collins HE, Chatham JC. Protein O-GlcNAcylation and cardiovascular (patho)physiology. J Biol Chem. (2014) 289:34449–56. doi: 10.1074/jbc.R114.585984
56. Hamilton S, Terentyev D. Proarrhythmic remodeling of calcium homeostasis in cardiac disease; implications for diabetes and obesity. Front Physiol. (2018) 9:1517. doi: 10.3389/fphys.2018.01517
57. Jennings RB. Historical perspective on the pathology of myocardial ischemia/reperfusion injury. Circ Res. (2013) 113:428–38. doi: 10.1161/CIRCRESAHA.113.300987
58. den Hengst WA, Gielis JF, Lin JY, Van Schil PE, De Windt LJ, Moens AL. Lung ischemia-reperfusion injury: a molecular and clinical view on a complex pathophysiological process. Am J Physiol Heart Circ Physiol. (2010) 299:H1283–99. doi: 10.1152/ajpheart.00251.2010
59. Jin R, Yang G, Li G. Inflammatory mechanisms in ischemic stroke: role of inflammatory cells. J Leukoc Biol. (2010) 87:779–89. doi: 10.1189/jlb.1109766
60. Konishi T, Lentsch AB. Hepatic ischemia/reperfusion: mechanisms of tissue injury, repair, and regeneration. Gene Expr. (2017) 17:277–87. doi: 10.3727/105221617X15042750874156
61. Ponticelli C. Ischaemia-reperfusion injury: a major protagonist in kidney transplantation. Nephrol Dial Transplant. (2014) 29:1134–40. doi: 10.1093/ndt/gft488
62. Mallick IH, Yang W, Winslet MC, Seifalian AM. Ischemia-reperfusion injury of the intestine and protective strategies against injury. Dig Dis Sci. (2004) 49:1359–77. doi: 10.1023/b:ddas.0000042232.98927.91
63. Kalogeris T, Baines CP, Krenz M, Korthuis RJ. Ischemia/Reperfusion. Compr Physiol. (2016) 7:113–70. doi: 10.1002/cphy.c160006
64. Carden DL, Granger DN. Pathophysiology of ischaemia-reperfusion injury. J Pathol. (2000) 190:255–66. doi: 10.1002/(SICI)1096-9896(200002)190:3<255::AID-PATH526>3.0.CO;2-6
65. Malek M, Nematbakhsh M. Renal ischemia/reperfusion injury; from pathophysiology to treatment. J Renal Inj Prev. (2015) 4:20–7. doi: 10.12861/jrip.2015.06
66. Pantazi E, Bejaoui M, Folch-Puy E, Adam R, Rosello-Catafau J. Advances in treatment strategies for ischemia reperfusion injury. Expert Opin Pharmacother. (2016) 17:169–79. doi: 10.1517/14656566.2016.1115015
67. Chen YS, Chao A, Yu HY, Ko WJ, Wu IH, Chen RJ, et al. Analysis and results of prolonged resuscitation in cardiac arrest patients rescued by extracorporeal membrane oxygenation. J Am Coll Cardiol. (2003) 41:197–203. doi: 10.1016/s0735-1097(02)02716-x
68. Ito H, Maruyama A, Iwakura K, Takiuchi S, Masuyama T, Hori M, et al. Clinical implications of the 'no reflow' phenomenon. A predictor of complications and left ventricular remodeling in reperfused anterior wall myocardial infarction. Circulation. (1996) 93:223–8. doi: 10.1161/01.cir.93.2.223
69. Kloner RA, Ellis SG, Lange R, Braunwald E. Studies of experimental coronary artery reperfusion. Effects on infarct size, myocardial function, biochemistry, ultrastructure and microvascular damage. Circulation. (1983) 68(2 Pt 2):8–15.
70. Rokos IC, French WJ, Koenig WJ, Stratton SJ, Nighswonger B, Strunk B, et al. Integration of pre-hospital electrocardiograms and ST-elevation myocardial infarction receiving center (SRC) networks: impact on Door-to-Balloon times across 10 independent regions. JACC Cardiovasc Interv. (2009) 2:339–46. doi: 10.1016/j.jcin.2008.11.013
71. De Scheerder I, Vandekerckhove J, Robbrecht J, Algoed L, De Buyzere M, De Langhe J, et al. Post-cardiac injury syndrome and an increased humoral immune response against the major contractile proteins (actin and myosin). Am J Cardiol. (1985) 56:631–3. doi: 10.1016/0002-9149(85)91024-0
72. Frangogiannis NG. The immune system and cardiac repair. Pharmacol Res. (2008) 58:88–111. doi: 10.1016/j.phrs.2008.06.007
73. Frangogiannis NG, Smith CW, Entman ML. The inflammatory response in myocardial infarction. Cardiovasc Res. (2002) 53:31–47. doi: 10.1016/s0008-6363(01)00434-5
74. Lambert JM, Lopez EF, Lindsey ML. Macrophage roles following myocardial infarction. Int J Cardiol. (2008) 130:147–58. doi: 10.1016/j.ijcard.2008.04.059
75. Lange LG, Schreiner GF. Immune mechanisms of cardiac disease. N Engl J Med. (1994) 330:1129–35. doi: 10.1056/NEJM199404213301607
76. Schuster JM, Nelson PS. Toll receptors: an expanding role in our understanding of human disease. J Leukoc Biol. (2000) 67:767–73. doi: 10.1002/jlb.67.6.767
77. Chow JC, Young DW, Golenbock DT, Christ WJ, Gusovsky F. Toll-like receptor-4 mediates lipopolysaccharide-induced signal transduction. J Biol Chem. (1999) 274:10689–92. doi: 10.1074/jbc.274.16.10689
78. Brubaker SW, Bonham KS, Zanoni I, Kagan JC. Innate immune pattern recognition: a cell biological perspective. Annu Rev Immunol. (2015) 33:257–90. doi: 10.1146/annurev-immunol-032414-112240
79. Arumugam TV, Okun E, Tang SC, Thundyil J, Taylor SM, Woodruff TM. Toll-like receptors in ischemia-reperfusion injury. Shock. (2009) 32:4–16. doi: 10.1097/SHK.0b013e318193e333
80. Zhao H, Perez JS, Lu K, George AJ, Ma D. Role of Toll-like receptor-4 in renal graft ischemia-reperfusion injury. Am J Physiol Renal Physiol. (2014) 306:F801–11. doi: 10.1152/ajprenal.00469.2013
81. Zhang J, Xia J, Zhang Y, Xiao F, Wang J, Gao H, et al. HMGB1-TLR4 signaling participates in renal ischemia reperfusion injury and could be attenuated by dexamethasone-mediated inhibition of the ERK/NF-kappaB pathway. Am J Transl Res. (2016) 8:4054–67.
82. Chen GY, Nunez G. Sterile inflammation: sensing and reacting to damage. Nat Rev Immunol. (2010) 10:826–37. doi: 10.1038/nri2873
83. Nakahira K, Kim HP, Geng XH, Nakao A, Wang X, Murase N, et al. Carbon monoxide differentially inhibits TLR signaling pathways by regulating ROS-induced trafficking of TLRs to lipid rafts. J Exp Med. (2006) 203:2377–89. doi: 10.1084/jem.20060845
84. Powers KA, Szaszi K, Khadaroo RG, Tawadros PS, Marshall JC, Kapus A, et al. Oxidative stress generated by hemorrhagic shock recruits Toll-like receptor 4 to the plasma membrane in macrophages. J Exp Med. (2006) 203:1951–61. doi: 10.1084/jem.20060943
85. Pahwa R, Jialal I. Hyperglycemia induces toll-like receptor activity through increased oxidative stress. Metab Syndr Relat Disord. (2016) 14:239–41. doi: 10.1089/met.2016.29006.pah
86. Latorre E, Mendoza C, Layunta E, Alcalde AI, Mesonero JE. TLR2, TLR3, and TLR4 activation specifically alters the oxidative status of intestinal epithelial cells. Cell Stress Chaperones. (2014) 19:289–93. doi: 10.1007/s12192-013-0461-8
87. Zhao H, Zhang M, Zhou F, Cao W, Bi L, Xie Y, et al. Cinnamaldehyde ameliorates LPS-induced cardiac dysfunction via TLR4-NOX4 pathway: The regulation of autophagy and ROS production. J Mol Cell Cardiol. (2016) 101:11–24. doi: 10.1016/j.yjmcc.2016.10.017
88. Pushpakumar S, Ren L, Kundu S, Gamon A, Tyagi SC, Sen U. Toll-like receptor 4 deficiency reduces oxidative stress and macrophage mediated inflammation in hypertensive kidney. Sci Rep. (2017) 7:6349. doi: 10.1038/s41598-017-06484-6
89. Bonizzi G, Karin M. The two NF-kappaB activation pathways and their role in innate and adaptive immunity. Trends Immunol. (2004) 25:280–8. doi: 10.1016/j.it.2004.03.008
90. Baeuerle PA, Henkel T. Function and activation of NF-kappa B in the immune system. Annu Rev Immunol. (1994) 12:141–79. doi: 10.1146/annurev.iy.12.040194.001041
91. Verstrepen L, Bekaert T, Chau TL, Tavernier J, Chariot A, Beyaert R. TLR-4, IL-1R and TNF-R signaling to NF-kappaB: variations on a common theme. Cell Mol Life Sci. (2008) 65:2964–78. doi: 10.1007/s00018-008-8064-8
92. Moynagh PN. The NF-kappaB pathway. J Cell Sci. (2005) 118(Pt 20):4589–92. doi: 10.1242/jcs.02579
93. Moynagh PN. TLR signalling and activation of IRFs: revisiting old friends from the NF-kappaB pathway. Trends Immunol. (2005) 26:469–76. doi: 10.1016/j.it.2005.06.009
94. Morgan MJ, Liu ZG. Crosstalk of reactive oxygen species and NF-kappaB signaling. Cell Res. (2011) 21:103–15. doi: 10.1038/cr.2010.178
95. Li C, Ha T, Kelley J, Gao X, Qiu Y, Kao RL, et al. Modulating Toll-like receptor mediated signaling by (1–>3)-beta-D-glucan rapidly induces cardioprotection. Cardiovasc Res. (2004) 61:538–47. doi: 10.1016/j.cardiores.2003.09.007
96. Frantz S, Kobzik L, Kim YD, Fukazawa R, Medzhitov R, Lee RT, et al. Toll4 (TLR4) expression in cardiac myocytes in normal and failing myocardium. J Clin Invest. (1999) 104:271–80. doi: 10.1172/JCI6709
97. Ravingerova T, Barancik M, Strniskova M. Mitogen-activated protein kinases: a new therapeutic target in cardiac pathology. Mol Cell Biochem. (2003) 247:127–38. doi: 10.1023/a:1024119224033
98. Lee IT, Shih RH, Lin CC, Chen JT, Yang CM. Role of TLR4/NADPH oxidase/ROS-activated p38 MAPK in VCAM-1 expression induced by lipopolysaccharide in human renal mesangial cells. Cell Commun Signal. (2012) 10:33. doi: 10.1186/1478-811X-10-33
99. Chong AJ, Shimamoto A, Hampton CR, Takayama H, Spring DJ, Rothnie CL, et al. Toll-like receptor 4 mediates ischemia/reperfusion injury of the heart. J Thorac Cardiovasc Surg. (2004) 128:170–9. doi: 10.1016/j.jtcvs.2003.11.036
100. Stapel H, Kim SC, Osterkamp S, Knuefermann P, Hoeft A, Meyer R, et al. Toll-like receptor 4 modulates myocardial ischaemia-reperfusion injury: Role of matrix metalloproteinases. Eur J Heart Fail. (2006) 8:665–72. doi: 10.1016/j.ejheart.2006.03.005
101. Shimamoto A, Chong AJ, Yada M, Shomura S, Takayama H, Fleisig AJ, et al. Inhibition of Toll-like receptor 4 with eritoran attenuates myocardial ischemia-reperfusion injury. Circulation. (2006) 114(1 Suppl):I270–4. doi: 10.1161/CIRCULATIONAHA.105.000901
102. Oyama J, Blais C, Jr., Liu X, Pu M, Kobzik L, Kelly RA, et al. Reduced myocardial ischemia-reperfusion injury in toll-like receptor 4-deficient mice. Circulation. (2004) 109:784–9. doi: 10.1161/01.CIR.0000112575.66565.84
103. Wu HS, Zhang JX, Wang L, Tian Y, Wang H, Rotstein O. Toll-like receptor 4 involvement in hepatic ischemia/reperfusion injury in mice. Hepatobiliary Pancreat Dis Int. (2004) 3:250–3.
104. Tsung A, Hoffman RA, Izuishi K, Critchlow ND, Nakao A, Chan MH, et al. Hepatic ischemia/reperfusion injury involves functional TLR4 signaling in nonparenchymal cells. J Immunol. (2005) 175:7661–8. doi: 10.4049/jimmunol.175.11.7661
105. Kim BS, Lim SW, Li C, Kim JS, Sun BK, Ahn KO, et al. Ischemia-reperfusion injury activates innate immunity in rat kidneys. Transplantation. (2005) 79:1370–7. doi: 10.1097/01.tp.0000158355.83327.62
106. Shimamoto A, Pohlman TH, Shomura S, Tarukawa T, Takao M, Shimpo H. Toll-like receptor 4 mediates lung ischemia-reperfusion injury. Ann Thorac Surg. (2006) 82:2017–23. doi: 10.1016/j.athoracsur.2006.06.079
107. Merry HE, Phelan P, Doak MR, Zhao M, Hwang B, Mulligan MS. Role of toll-like receptor-4 in lung ischemia-reperfusion injury. Ann Thorac Surg. (2015) 99:1193–9. doi: 10.1016/j.athoracsur.2014.12.062
108. Zanotti G, Casiraghi M, Abano JB, Tatreau JR, Sevala M, Berlin H, et al. Novel critical role of Toll-like receptor 4 in lung ischemia-reperfusion injury and edema. Am J Physiol Lung Cell Mol Physiol. (2009) 297:L52–63. doi: 10.1152/ajplung.90406.2008
109. Ishiyama T, Dharmarajan S, Hayama M, Moriya H, Grapperhaus K, Patterson GA. Inhibition of nuclear factor kappaB by IkappaB superrepressor gene transfer ameliorates ischemia-reperfusion injury after experimental lung transplantation. J Thorac Cardiovasc Surg. (2005) 130:194–201. doi: 10.1016/j.jtcvs.2005.02.040
110. Phelan P, Merry HE, Hwang B, Mulligan MS. Differential toll-like receptor activation in lung ischemia reperfusion injury. J Thorac Cardiovasc Surg. (2015) 149:1653–61. doi: 10.1016/j.jtcvs.2015.02.045
111. Lambeir AM, Durinx C, Scharpe S, De Meester I. Dipeptidyl-peptidase IV from bench to bedside: an update on structural properties, functions, and clinical aspects of the enzyme DPP IV. Crit Rev Clin Lab Sci. (2003) 40:209–94. doi: 10.1080/713609354
112. Rohrborn D, Wronkowitz N, Eckel J. DPP4 in diabetes. Front Immunol. (2015) 6:386. doi: 10.3389/fimmu.2015.00386
113. Waumans Y, Baerts L, Kehoe K, Lambeir AM, De Meester I. The dipeptidyl peptidase family, prolyl oligopeptidase, and prolyl carboxypeptidase in the immune system and inflammatory disease, including atherosclerosis. Front Immunol. (2015) 6:387. doi: 10.3389/fimmu.2015.00387
114. Lei Y, Hu L, Yang G, Piao L, Jin M, Cheng X. Dipeptidyl peptidase-IV inhibition for the treatment of cardiovascular disease- recent insights focusing on angiogenesis and neovascularization. Circ J. (2017) 81:770–6. doi: 10.1253/circj.CJ-16-1326
115. Durinx C, Lambeir AM, Bosmans E, Falmagne JB, Berghmans R, Haemers A, et al. Molecular characterization of dipeptidyl peptidase activity in serum: soluble CD26/dipeptidyl peptidase IV is responsible for the release of X-Pro dipeptides. Eur J Biochem. (2000) 267:5608–13. doi: 10.1046/j.1432-1327.2000.01634.x
116. Duez H, Cariou B, Staels B. DPP-4 inhibitors in the treatment of type 2 diabetes. Biochem Pharmacol. (2012) 83:823–32. doi: 10.1016/j.bcp.2011.11.028
117. Chen YT, Tsai TH, Yang CC, Sun CK, Chang LT, Chen HH, et al. Exendin-4 and sitagliptin protect kidney from ischemia-reperfusion injury through suppressing oxidative stress and inflammatory reaction. J Transl Med. (2013) 11:270. doi: 10.1186/1479-5876-11-270
118. Oeseburg H, de Boer RA, Buikema H, van der Harst P, van Gilst WH, Sillje HH. Glucagon-like peptide 1 prevents reactive oxygen species-induced endothelial cell senescence through the activation of protein kinase A. Arterioscler Thromb Vasc Biol. (2010) 30:1407–14. doi: 10.1161/ATVBAHA.110.206425
119. Shimoda M, Kanda Y, Hamamoto S, Tawaramoto K, Hashiramoto M, Matsuki M, et al. The human glucagon-like peptide-1 analogue liraglutide preserves pancreatic beta cells via regulation of cell kinetics and suppression of oxidative and endoplasmic reticulum stress in a mouse model of diabetes. Diabetologia. (2011) 54:1098–108. doi: 10.1007/s00125-011-2069-9
120. Oh YS, Jun HS. Effects of glucagon-like peptide-1 on oxidative stress and Nrf2 signaling. Int J Mol Sci. (2017) 19:26. doi: 10.3390/ijms19010026
121. Ye Y, Keyes KT, Zhang C, Perez-Polo JR, Lin Y, Birnbaum Y. The myocardial infarct size-limiting effect of sitagliptin is PKA-dependent, whereas the protective effect of pioglitazone is partially dependent on PKA. Am J Physiol Heart Circ Physiol. (2010) 298:H1454–65. doi: 10.1152/ajpheart.00867.2009
122. Matheeussen V, Jungraithmayr W, De Meester I. Dipeptidyl peptidase 4 as a therapeutic target in ischemia/reperfusion injury. Pharmacol Ther. (2012) 136:267–82. doi: 10.1016/j.pharmthera.2012.07.012
123. Ku HC, Chen WP, Su MJ. DPP4 deficiency preserves cardiac function via GLP-1 signaling in rats subjected to myocardial ischemia/reperfusion. Naunyn Schmiedebergs Arch Pharmacol. (2011) 384:197–207. doi: 10.1007/s00210-011-0665-3
124. Ku HC, Chen WP, Su MJ. DPP4 deficiency exerts protective effect against H2O2 induced oxidative stress in isolated cardiomyocytes. PLoS ONE. (2013) 8:e54518. doi: 10.1371/journal.pone.0054518
125. Wang XM, Yang YJ, Wu YJ. The emerging role of dipeptidyl peptidase-4 inhibitors in cardiovascular protection: current position and perspectives. Cardiovasc Drugs Ther. (2013) 27:297–307. doi: 10.1007/s10557-013-6459-8
126. Nikolaidis LA, Mankad S, Sokos GG, Miske G, Shah A, Elahi D, et al. Effects of glucagon-like peptide-1 in patients with acute myocardial infarction and left ventricular dysfunction after successful reperfusion. Circulation. (2004) 109:962–5. doi: 10.1161/01.CIR.0000120505.91348.58
127. Noyan-Ashraf MH, Momen MA, Ban K, Sadi AM, Zhou YQ, Riazi AM, et al. GLP-1R agonist liraglutide activates cytoprotective pathways and improves outcomes after experimental myocardial infarction in mice. Diabetes. (2009) 58:975–83. doi: 10.2337/db08-1193
128. Sokos GG, Nikolaidis LA, Mankad S, Elahi D, Shannon RP. Glucagon-like peptide-1 infusion improves left ventricular ejection fraction and functional status in patients with chronic heart failure. J Card Fail. (2006) 12:694–9. doi: 10.1016/j.cardfail.2006.08.211
129. Bao W, Aravindhan K, Alsaid H, Chendrimada T, Szapacs M, Citerone DR, et al. Albiglutide, a long lasting glucagon-like peptide-1 analog, protects the rat heart against ischemia/reperfusion injury: evidence for improving cardiac metabolic efficiency. PLoS ONE. (2011) 6:e23570. doi: 10.1371/journal.pone.0023570
130. Ravassa S, Zudaire A, Diez J. GLP-1 and cardioprotection: from bench to bedside. Cardiovasc Res. (2012) 94:316–23. doi: 10.1093/cvr/cvs123
131. Zhao T, Parikh P, Bhashyam S, Bolukoglu H, Poornima I, Shen YT, et al. Direct effects of glucagon-like peptide-1 on myocardial contractility and glucose uptake in normal and postischemic isolated rat hearts. J Pharmacol Exp Ther. (2006) 317:1106–13. doi: 10.1124/jpet.106.100982
132. Fields AV, Patterson B, Karnik AA, Shannon RP. Glucagon-like peptide-1 and myocardial protection: more than glycemic control. Clin Cardiol. (2009) 32:236–43. doi: 10.1002/clc.20456
133. Dokken BB, Hilwig WR, Teachey MK, Panchal RA, Hubner K, Allen D, et al. Glucagon-like peptide-1 (GLP-1) attenuates post-resuscitation myocardial microcirculatory dysfunction. Resuscitation. (2010) 81:755–60. doi: 10.1016/j.resuscitation.2010.01.031
134. Schade J, Stephan M, Schmiedl A, Wagner L, Niestroj AJ, Demuth HU, et al. Regulation of expression and function of dipeptidyl peptidase 4 (DP4), DP8/9, and DP10 in allergic responses of the lung in rats. J Histochem Cytochem. (2008) 56:147–55. doi: 10.1369/jhc.7A7319.2007
135. Meyerholz DK, Lambertz AM, McCray PB Jr. Dipeptidyl peptidase 4 distribution in the human respiratory tract: implications for the middle east respiratory syndrome. Am J Pathol. (2016) 186:78–86. doi: 10.1016/j.ajpath.2015.09.014
136. Wronkowitz N, Gorgens SW, Romacho T, Villalobos LA, Sanchez-Ferrer CF, Peiro C, et al. Soluble DPP4 induces inflammation and proliferation of human smooth muscle cells via protease-activated receptor 2. Biochim Biophys Acta. (2014) 1842:1613–21. doi: 10.1016/j.bbadis.2014.06.004
137. Beckers PAJ, Gielis JF, Van Schil PE, Adriaensen D. Lung ischemia reperfusion injury: the therapeutic role of dipeptidyl peptidase 4 inhibition. Ann Transl Med. (2017) 5:129. doi: 10.21037/atm.2017.01.41
138. Peterson SJ, Frishman WH, Abraham NG. Targeting heme oxygenase: therapeutic implications for diseases of the cardiovascular system. Cardiol Rev. (2009) 17:99–111. doi: 10.1097/CRD.0b013e31819d813a
139. Maines MD. The heme oxygenase system: a regulator of second messenger gases. Annu Rev Pharmacol Toxicol. (1997) 37:517–54. doi: 10.1146/annurev.pharmtox.37.1.517
140. Loboda A, Damulewicz M, Pyza E, Jozkowicz A, Dulak J. Role of Nrf2/HO-1 system in development, oxidative stress response and diseases: an evolutionarily conserved mechanism. Cell Mol Life Sci. (2016) 73:3221–47. doi: 10.1007/s00018-016-2223-0
141. Katori M, Busuttil RW, Kupiec-Weglinski JW. Heme oxygenase-1 system in organ transplantation. Transplantation. (2002) 74:905–12. doi: 10.1097/00007890-200210150-00001
142. Katori M, Tamaki T, Takahashi T, Tanaka M, Kawamura A, Kakita A. Prior induction of heat shock proteins by a nitric oxide donor attenuates cardiac ischemia/reperfusion injury in the rat. Transplantation. (2000) 69:2530–7. doi: 10.1097/00007890-200006270-00011
143. Katori M, Buelow R, Ke B, Ma J, Coito AJ, Iyer S, et al. Heme oxygenase-1 overexpression protects rat hearts from cold ischemia/reperfusion injury via an antiapoptotic pathway. Transplantation. (2002) 73:287–92. doi: 10.1097/00007890-200201270-00023
144. Brouard S, Otterbein LE, Anrather J, Tobiasch E, Bach FH, Choi AM, et al. Carbon monoxide generated by heme oxygenase 1 suppresses endothelial cell apoptosis. J Exp Med. (2000) 192:1015–26. doi: 10.1084/jem.192.7.1015
145. Yue TL, Wang C, Gu JL, Ma XL, Kumar S, Lee JC, et al. Inhibition of extracellular signal-regulated kinase enhances Ischemia/Reoxygenation-induced apoptosis in cultured cardiac myocytes and exaggerates reperfusion injury in isolated perfused heart. Circ Res. (2000) 86:692–9. doi: 10.1161/01.res.86.6.692
146. Fleig A, Penner R. The TRPM ion channel subfamily: molecular, biophysical and functional features. Trends Pharmacol Sci. (2004) 25:633–9. doi: 10.1016/j.tips.2004.10.004
147. Montell C. The TRP superfamily of cation channels. Sci STKE. (2005) 2005:re3. doi: 10.1126/stke.2722005re3
148. Simon F, Varela D, Cabello-Verrugio C. Oxidative stress-modulated TRPM ion channels in cell dysfunction and pathological conditions in humans. Cell Signal. (2013) 25:1614–24. doi: 10.1016/j.cellsig.2013.03.023
149. Zholos A, Johnson C, Burdyga T, Melanaphy D. TRPM channels in the vasculature. Adv Exp Med Biol. (2011) 704:707–29. doi: 10.1007/978-94-007-0265-3_37
150. McNulty S, Fonfria E. The role of TRPM channels in cell death. Pflugers Arch. (2005) 451:235–42. doi: 10.1007/s00424-005-1440-4
151. Abriel H, Syam N, Sottas V, Amarouch MY, Rougier JS. TRPM4 channels in the cardiovascular system: physiology, pathophysiology, and pharmacology. Biochem Pharmacol. (2012) 84:873–81. doi: 10.1016/j.bcp.2012.06.021
152. Wang C, Naruse K, Takahashi K. Role of the TRPM4 Channel in Cardiovascular Physiology and Pathophysiology. Cells. (2018) 7. doi: 10.3390/cells7060062
153. Vennekens R, Mesuere M, Philippaert K. TRPM5 in the battle against diabetes and obesity. Acta Physiol (Oxf). (2018) 222:e12949. doi: 10.1111/apha.12949
154. Zierler S, Hampe S, Nadolni W. TRPM channels as potential therapeutic targets against pro-inflammatory diseases. Cell Calcium. (2017) 67:105–15. doi: 10.1016/j.ceca.2017.05.002
155. Miller BA. The role of TRP channels in oxidative stress-induced cell death. J Membr Biol. (2006) 209:31–41. doi: 10.1007/s00232-005-0839-3
156. Launay P, Fleig A, Perraud AL, Scharenberg AM, Penner R, Kinet JP. TRPM4 is a Ca2+-activated nonselective cation channel mediating cell membrane depolarization. Cell. (2002) 109:397–407. doi: 10.1016/s0092-8674(02)00719-5
157. Murakami M, Xu F, Miyoshi I, Sato E, Ono K, Iijima T. Identification and characterization of the murine TRPM4 channel. Biochem Biophys Res Commun. (2003) 307:522–8. doi: 10.1016/s0006-291x(03)01186-0
158. Nilius B, Prenen J, Droogmans G, Voets T, Vennekens R, Freichel M, et al. Voltage dependence of the Ca2+-activated cation channel TRPM4. J Biol Chem. (2003) 278:30813–20. doi: 10.1074/jbc.M305127200
159. Jang Y, Lee Y, Kim SM, Yang YD, Jung J, Oh U. Quantitative analysis of TRP channel genes in mouse organs. Arch Pharm Res. (2012) 35:1823–30. doi: 10.1007/s12272-012-1016-8
160. Guinamard R, Demion M, Magaud C, Potreau D, Bois P. Functional expression of the TRPM4 cationic current in ventricular cardiomyocytes from spontaneously hypertensive rats. Hypertension. (2006) 48:587–94. doi: 10.1161/01.HYP.0000237864.65019.a5
161. Mathar I, Kecskes M, Van der Mieren G, Jacobs G, Camacho Londono JE, Uhl S, et al. Increased beta-adrenergic inotropy in ventricular myocardium from Trpm4−/− mice. Circ Res. (2014) 114:283–94. doi: 10.1161/CIRCRESAHA.114.302835
162. Guinamard R, Chatelier A, Demion M, Potreau D, Patri S, Rahmati M, et al. Functional characterization of a Ca(2+)-activated non-selective cation channel in human atrial cardiomyocytes. J Physiol. (2004) 558(Pt 1):75–83. doi: 10.1113/jphysiol.2004.063974
163. Liu H, El Zein L, Kruse M, Guinamard R, Beckmann A, Bozio A, et al. Gain-of-function mutations in TRPM4 cause autosomal dominant isolated cardiac conduction disease. Circ Cardiovasc Genet. (2010) 3:374–85. doi: 10.1161/CIRCGENETICS.109.930867
164. Guan D, Su Y, Li Y, Wu C, Meng Y, Peng X, et al. Tetramethylpyrazine inhibits CoCl2 -induced neurotoxicity through enhancement of Nrf2/GCLc/GSH and suppression of HIF1alpha/NOX2/ROS pathways. J Neurochem. (2015) 134:551–65. doi: 10.1111/jnc.13161
165. Nakayama N, Yamaguchi S, Sasaki Y, Chikuma T. Hydrogen peroxide-induced oxidative stress activates proteasomal trypsin-like activity in human U373 glioma cells. J Mol Neurosci. (2016) 58:297–305. doi: 10.1007/s12031-015-0680-9
166. Piao H, Takahashi K, Yamaguchi Y, Wang C, Liu K, Naruse K. Transient receptor potential melastatin-4 is involved in hypoxia-reoxygenation injury in the cardiomyocytes. PLoS ONE. (2015) 10:e0121703. doi: 10.1371/journal.pone.0121703
167. Zou W, Yan M, Xu W, Huo H, Sun L, Zheng Z, et al. Cobalt chloride induces PC12 cells apoptosis through reactive oxygen species and accompanied by AP-1 activation. J Neurosci Res. (2001) 64:646–53. doi: 10.1002/jnr.1118
168. Jung JY, Kim WJ. Involvement of mitochondrial- and Fas-mediated dual mechanism in CoCl2-induced apoptosis of rat PC12 cells. Neurosci Lett. (2004) 371:85–90. doi: 10.1016/j.neulet.2004.06.069
169. Zou W, Zeng J, Zhuo M, Xu W, Sun L, Wang J, et al. Involvement of caspase-3 and p38 mitogen-activated protein kinase in cobalt chloride-induced apoptosis in PC12 cells. J Neurosci Res. (2002) 67:837–43. doi: 10.1002/jnr.10168
170. Cheng CI, Lee YH, Chen PH, Lin YC, Chou MH, Kao YH. Cobalt chloride induces RhoA/ROCK activation and remodeling effect in H9c2 cardiomyoblasts: Involvement of PI3K/Akt and MAPK pathways. Cell Signal. (2017) 36:25–33. doi: 10.1016/j.cellsig.2017.04.013
171. Jung JY, Mo HC, Yang KH, Jeong YJ, Yoo HG, Choi NK, et al. Inhibition by epigallocatechin gallate of CoCl2-induced apoptosis in rat PC12 cells. Life Sci. (2007) 80:1355–63. doi: 10.1016/j.lfs.2006.11.033
172. Simon F, Leiva-Salcedo E, Armisen R, Riveros A, Cerda O, Varela D, et al. Hydrogen peroxide removes TRPM4 current desensitization conferring increased vulnerability to necrotic cell death. J Biol Chem. (2010) 285:37150–8. doi: 10.1074/jbc.M110.155390
173. Nilius B, Prenen J, Voets T, Droogmans G. Intracellular nucleotides and polyamines inhibit the Ca2+-activated cation channel TRPM4b. Pflugers Arch. (2004) 448:70–5. doi: 10.1007/s00424-003-1221-x
174. Benndorf K, Friedrich M, Hirche H. Reoxygenation-induced arrhythmogenic transient inward currents in isolated cells of the guinea-pig heart. Pflugers Arch. (1991) 418:248–60. doi: 10.1007/BF00370523
175. Carmeliet E. Cardiac ionic currents and acute ischemia: from channels to arrhythmias. Physiol Rev. (1999) 79:917–1017. doi: 10.1152/physrev.1999.79.3.917
176. Kalogeris T, Baines CP, Krenz M, Korthuis RJ. Cell biology of ischemia/reperfusion injury. Int Rev Cell Mol Biol. (2012) 298:229–317. doi: 10.1016/B978-0-12-394309-5.00006-7
177. Grand T, Demion M, Norez C, Mettey Y, Launay P, Becq F, et al. 9-phenanthrol inhibits human TRPM4 but not TRPM5 cationic channels. Br J Pharmacol. (2008) 153:1697–705. doi: 10.1038/bjp.2008.38
178. Wang C, Chen J, Wang M, Naruse K, Takahashi K. Role of the TRPM4 channel in mitochondrial function, calcium release, and ROS generation in oxidative stress. Biochem Biophys Res Commun. (2021). Available online at: https://authors.elsevier.com/tracking/article/details.do?aid=45815&jid=YBBRC&surname=Takahashi
179. Hoffman NE, Miller BA, Wang J, Elrod JW, Rajan S, Gao E, et al. Ca(2)(+) entry via Trpm2 is essential for cardiac myocyte bioenergetics maintenance. Am J Physiol Heart Circ Physiol. (2015) 308:H637–50. doi: 10.1152/ajpheart.00720.2014
180. Malko P, Jiang LH. TRPM2 channel-mediated cell death: An important mechanism linking oxidative stress-inducing pathological factors to associated pathological conditions. Redox Biol. (2020) 37:101755. doi: 10.1016/j.redox.2020.101755
181. Miller BA, Cheung JY. TRPM2 protects against tissue damage following oxidative stress and ischaemia-reperfusion. J Physiol. (2016) 594:4181–91. doi: 10.1113/JP270934
182. Hara Y, Wakamori M, Ishii M, Maeno E, Nishida M, Yoshida T, et al. LTRPC2 Ca2+-permeable channel activated by changes in redox status confers susceptibility to cell death. Mol Cell. (2002) 9:163–73. doi: 10.1016/s1097-2765(01)00438-5
183. Kaneko S, Kawakami S, Hara Y, Wakamori M, Itoh E, Minami T, et al. A critical role of TRPM2 in neuronal cell death by hydrogen peroxide. J Pharmacol Sci. (2006) 101:66–76. doi: 10.1254/jphs.fp0060128
184. Hecquet CM, Ahmmed GU, Vogel SM, Malik AB. Role of TRPM2 channel in mediating H2O2-induced Ca2+ entry and endothelial hyperpermeability. Circ Res. (2008) 102:347–55. doi: 10.1161/CIRCRESAHA.107.160176
185. Lemasters JJ, Theruvath TP, Zhong Z, Nieminen AL. Mitochondrial calcium and the permeability transition in cell death. Biochim Biophys Acta. (2009) 1787:1395–401. doi: 10.1016/j.bbabio.2009.06.009
186. Davidson SM, Yellon DM, Murphy MP, Duchen MR. Slow calcium waves and redox changes precede mitochondrial permeability transition pore opening in the intact heart during hypoxia and reoxygenation. Cardiovasc Res. (2012) 93:445–53. doi: 10.1093/cvr/cvr349
187. Ren J, Pulakat L, Whaley-Connell A, Sowers JR. Mitochondrial biogenesis in the metabolic syndrome and cardiovascular disease. J Mol Med (Berl). (2010) 88:993–1001. doi: 10.1007/s00109-010-0663-9
188. Shigenaga MK, Hagen TM, Ames BN. Oxidative damage and mitochondrial decay in aging. Proc Natl Acad Sci USA. (1994) 91:10771–8. doi: 10.1073/pnas.91.23.10771
189. Bao L, Festa F, Freet CS, Lee JP, Hirschler-Laszkiewicz IM, Chen SJ, et al. The human transient receptor potential melastatin 2 ion channel modulates ROS through Nrf2. Sci Rep. (2019) 9:14132. doi: 10.1038/s41598-019-50661-8
190. Liu YV, Hubbi ME, Pan F, McDonald KR, Mansharamani M, Cole RN, et al. Calcineurin promotes hypoxia-inducible factor 1alpha expression by dephosphorylating RACK1 and blocking RACK1 dimerization. J Biol Chem. (2007) 282:37064–73. doi: 10.1074/jbc.M705015200
191. Bankers-Fulbright JL, Gleich GJ, Kephart GM, Kita H, O'Grady SM. Regulation of eosinophil membrane depolarization during NADPH oxidase activation. J Cell Sci. (2003) 116(Pt 15):3221–6. doi: 10.1242/jcs.00627
192. Di A, Gao XP, Qian F, Kawamura T, Han J, Hecquet C, et al. The redox-sensitive cation channel TRPM2 modulates phagocyte ROS production and inflammation. Nat Immunol. (2011) 13:29–34. doi: 10.1038/ni.2171
193. Sumoza-Toledo A, Penner R. TRPM2: a multifunctional ion channel for calcium signalling. J Physiol. (2011) 589(Pt 7):1515–25. doi: 10.1113/jphysiol.2010.201855
194. Simonneau G, Montani D, Celermajer DS, Denton CP, Gatzoulis MA, Krowka M, et al. Haemodynamic definitions and updated clinical classification of pulmonary hypertension. Eur Respir J. (2019) 53. doi: 10.1183/13993003.01913-2018
195. Nakanishi K, Tajima F, Osada H, Nakamura A, Yagura S, Kawai T, et al. Pulmonary, vascular responses in rats exposed to chronic hypobaric hypoxia at two different altitude levels. Pathol Res Pract. (1996) 192:1057–67. doi: 10.1016/S0344-0338(96)80049-5
196. Kuhr FK, Smith KA, Song MY, Levitan I, Yuan JX. New mechanisms of pulmonary arterial hypertension: role of Ca(2)(+) signaling. Am J Physiol Heart Circ Physiol. (2012) 302:H1546–62. doi: 10.1152/ajpheart.00944.2011
197. Waypa GB, Chandel NS, Schumacker PT. Model for hypoxic pulmonary vasoconstriction involving mitochondrial oxygen sensing. Circ Res. (2001) 88:1259–66. doi: 10.1161/hh1201.091960
198. Waypa GB, Guzy R, Mungai PT, Mack MM, Marks JD, Roe MW, et al. Increases in mitochondrial reactive oxygen species trigger hypoxia-induced calcium responses in pulmonary artery smooth muscle cells. Circ Res. (2006) 99:970–8. doi: 10.1161/01.RES.0000247068.75808.3f
199. Jernigan NL, Resta TC, Walker BR. Contribution of oxygen radicals to altered NO-dependent pulmonary vasodilation in acute and chronic hypoxia. Am J Physiol Lung Cell Mol Physiol. (2004) 286:L947–55. doi: 10.1152/ajplung.00215.2003
200. Killilea DW, Hester R, Balczon R, Babal P, Gillespie MN. Free radical production in hypoxic pulmonary artery smooth muscle cells. Am J Physiol Lung Cell Mol Physiol. (2000) 279:L408–12. doi: 10.1152/ajplung.2000.279.2.L408
201. Leach RM, Hill HM, Snetkov VA, Robertson TP, Ward JP. Divergent roles of glycolysis and the mitochondrial electron transport chain in hypoxic pulmonary vasoconstriction of the rat: identity of the hypoxic sensor. J Physiol. (2001) 536(Pt 1):211–24. doi: 10.1111/j.1469-7793.2001.00211.x
202. Liu JQ, Sham JS, Shimoda LA, Kuppusamy P, Sylvester JT. Hypoxic constriction and reactive oxygen species in porcine distal pulmonary arteries. Am J Physiol Lung Cell Mol Physiol. (2003) 285:L322–33. doi: 10.1152/ajplung.00337.2002
203. Paddenberg R, Ishaq B, Goldenberg A, Faulhammer P, Rose F, Weissmann N, et al. Essential role of complex II of the respiratory chain in hypoxia-induced ROS generation in the pulmonary vasculature. Am J Physiol Lung Cell Mol Physiol. (2003) 284:L710–9. doi: 10.1152/ajplung.00149.2002
204. Rathore R, Zheng YM, Li XQ, Wang QS, Liu QH, Ginnan R, et al. Mitochondrial ROS-PKCepsilon signaling axis is uniquely involved in hypoxic increase in [Ca2+]i in pulmonary artery smooth muscle cells. Biochem Biophys Res Commun. (2006) 351:784–90. doi: 10.1016/j.bbrc.2006.10.116
205. Wang QS, Zheng YM, Dong L, Ho YS, Guo Z, Wang YX. Role of mitochondrial reactive oxygen species in hypoxia-dependent increase in intracellular calcium in pulmonary artery myocytes. Free Radic Biol Med. (2007) 42:642–53. doi: 10.1016/j.freeradbiomed.2006.12.008
206. Ismail S, Sturrock A, Wu P, Cahill B, Norman K, Huecksteadt T, et al. NOX4 mediates hypoxia-induced proliferation of human pulmonary artery smooth muscle cells: the role of autocrine production of transforming growth factor-{beta}1 and insulin-like growth factor binding protein-3. Am J Physiol Lung Cell Mol Physiol. (2009) 296:L489–99. doi: 10.1152/ajplung.90488.2008
207. Liu JQ, Zelko IN, Erbynn EM, Sham JS, Folz RJ. Hypoxic pulmonary hypertension: role of superoxide and NADPH oxidase (gp91phox). Am J Physiol Lung Cell Mol Physiol. (2006) 290:L2–10. doi: 10.1152/ajplung.00135.2005
208. Hirenallur SD, Haworth ST, Leming JT, Chang J, Hernandez G, Gordon JB, et al. Upregulation of vascular calcium channels in neonatal piglets with hypoxia-induced pulmonary hypertension. Am J Physiol Lung Cell Mol Physiol. (2008) 295:L915–24. doi: 10.1152/ajplung.90286.2008
209. Bonnet S, Hyvelin JM, Bonnet P, Marthan R, Savineau JP. Chronic hypoxia-induced spontaneous and rhythmic contractions in the rat main pulmonary artery. Am J Physiol Lung Cell Mol Physiol. (2001) 281:L183–92. doi: 10.1152/ajplung.2001.281.1.L183
210. Davidson A, Bossuyt A, Dab I. Acute effects of oxygen, nifedipine, and diltiazem in patients with cystic fibrosis and mild pulmonary hypertension. Pediatr Pulmonol. (1989) 6:53–9. doi: 10.1002/ppul.1950060113
211. Fike CD, Kaplowitz MR. Nifedipine inhibits pulmonary hypertension but does not prevent decreased lung eNOS in hypoxic newborn pigs. Am J Physiol. (1999) 277:L449–56. doi: 10.1152/ajplung.1999.277.3.L449
212. Barry EL. Expression of mRNAs for the alpha 1 subunit of voltage-gated calcium channels in human osteoblast-like cell lines and in normal human osteoblasts. Calcif Tissue Int. (2000) 66:145–50. doi: 10.1007/s002230010029
213. Wan J, Yamamura A, Zimnicka AM, Voiriot G, Smith KA, Tang H, et al. Chronic hypoxia selectively enhances L- and T-type voltage-dependent Ca2+ channel activity in pulmonary artery by upregulating Cav1.2 and Cav3.2. Am J Physiol Lung Cell Mol Physiol. (2013) 305:L154–64. doi: 10.1152/ajplung.00313.2012
214. Yuan XJ. Voltage-gated K+ currents regulate resting membrane potential and [Ca2+]i in pulmonary arterial myocytes. Circ Res. (1995) 77:370–8. doi: 10.1161/01.res.77.2.370
215. Bakhramov A, Evans AM, Kozlowski RZ. Differential effects of hypoxia on the intracellular Ca2+ concentration of myocytes isolated from different regions of the rat pulmonary arterial tree. Exp Physiol. (1998) 83:337–47. doi: 10.1113/expphysiol.1998.sp004117
216. Cornfield DN, Stevens T, McMurtry IF, Abman SH, Rodman DM. Acute hypoxia causes membrane depolarization and calcium influx in fetal pulmonary artery smooth muscle cells. Am J Physiol. (1994) 266(4 Pt 1):L469–75. doi: 10.1152/ajplung.1994.266.4.L469
217. Urena J, Franco-Obregon A, Lopez-Barneo J. Contrasting effects of hypoxia on cytosolic Ca2+ spikes in conduit and resistance myocytes of the rabbit pulmonary artery. J Physiol. (1996) 496 (Pt 1):103–9. doi: 10.1113/jphysiol.1996.sp021668
218. Wang J, Shimoda LA, Weigand L, Wang W, Sun D, Sylvester JT. Acute hypoxia increases intracellular [Ca2+] in pulmonary arterial smooth muscle by enhancing capacitative Ca2+ entry. Am J Physiol Lung Cell Mol Physiol. (2005) 288:L1059–69. doi: 10.1152/ajplung.00448.2004
219. Viola HM, Arthur PG, Hool LC. Transient exposure to hydrogen peroxide causes an increase in mitochondria-derived superoxide as a result of sustained alteration in L-type Ca2+ channel function in the absence of apoptosis in ventricular myocytes. Circ Res. (2007) 100:1036–44. doi: 10.1161/01.RES.0000263010.19273.48
220. Akaishi T, Nakazawa K, Sato K, Saito H, Ohno Y, Ito Y. Hydrogen peroxide modulates whole cell Ca2+ currents through L-type channels in cultured rat dentate granule cells. Neurosci Lett. (2004) 356:25–8. doi: 10.1016/j.neulet.2003.11.012
221. Yang L, Xu J, Minobe E, Yu L, Feng R, Kameyama A, et al. Mechanisms underlying the modulation of L-type Ca2+ channel by hydrogen peroxide in guinea pig ventricular myocytes. J Physiol Sci. (2013) 63:419–26. doi: 10.1007/s12576-013-0279-2
222. Tang H, Viola HM, Filipovska A, Hool LC. Ca(v)1.2 calcium channel is glutathionylated during oxidative stress in guinea pig and ischemic human heart. Free Radic Biol Med. (2011) 51:1501–11. doi: 10.1016/j.freeradbiomed.2011.07.005
223. Johnstone VP, Hool LC. Glutathionylation of the L-type Ca2+ channel in oxidative stress-induced pathology of the heart. Int J Mol Sci. (2014) 15:19203–25. doi: 10.3390/ijms151019203
224. Lai N, Lu W, Wang J. Ca(2+) and ion channels in hypoxia-mediated pulmonary hypertension. Int J Clin Exp Pathol. (2015) 8:1081–92.
225. Nelson MT, Patlak JB, Worley JF, Standen NB. Calcium channels, potassium channels, and voltage dependence of arterial smooth muscle tone. Am J Physiol. (1990) 259(1 Pt 1):C3–18. doi: 10.1152/ajpcell.1990.259.1.C3
226. Shimoda LA, Sham JS, Shimoda TH, Sylvester JT. L-type Ca(2+) channels, resting [Ca(2+)](i), and ET-1-induced responses in chronically hypoxic pulmonary myocytes. Am J Physiol Lung Cell Mol Physiol. (2000) 279:L884–94. doi: 10.1152/ajplung.2000.279.5.L884
227. Luke T, Maylor J, Undem C, Sylvester JT, Shimoda LA. Kinase-dependent activation of voltage-gated Ca2+ channels by ET-1 in pulmonary arterial myocytes during chronic hypoxia. Am J Physiol Lung Cell Mol Physiol. (2012) 302:L1128–39. doi: 10.1152/ajplung.00396.2011
228. Cosentino-Gomes D, Rocco-Machado N, Meyer-Fernandes JR. Cell signaling through protein kinase C oxidation and activation. Int J Mol Sci. (2012) 13:10697–721. doi: 10.3390/ijms130910697
229. MacKay CE, Shaifta Y, Snetkov VV, Francois AA, Ward JPT, Knock GA. ROS-dependent activation of RhoA/Rho-kinase in pulmonary artery: Role of Src-family kinases and ARHGEF1. Free Radic Biol Med. (2017) 110:316–31. doi: 10.1016/j.freeradbiomed.2017.06.022
230. Norton CE, Sheak JR, Yan S, Weise-Cross L, Jernigan NL, Walker BR, et al. Augmented pulmonary vasoconstrictor reactivity after chronic hypoxia requires src kinase and epidermal growth factor receptor signaling. Am J Respir Cell Mol Biol. (2020) 62:61–73. doi: 10.1165/rcmb.2018-0106OC
231. Wu W, Platoshyn O, Firth AL, Yuan JX. Hypoxia divergently regulates production of reactive oxygen species in human pulmonary and coronary artery smooth muscle cells. Am J Physiol Lung Cell Mol Physiol. (2007) 293:L952–9. doi: 10.1152/ajplung.00203.2007
232. Wedgwood S, Black SM. Endothelin-1 decreases endothelial NOS expression and activity through ETA receptor-mediated generation of hydrogen peroxide. Am J Physiol Lung Cell Mol Physiol. (2005) 288:L480–7. doi: 10.1152/ajplung.00283.2004
Keywords: oxidative stress, reactive oxygen species, inflammation, diabetes mellitus, ischemia–reperfusion injury, mitochondria, transient receptor potential channels
Citation: Wang M, Liu Y, Liang Y, Naruse K and Takahashi K (2021) Systematic Understanding of Pathophysiological Mechanisms of Oxidative Stress-Related Conditions—Diabetes Mellitus, Cardiovascular Diseases, and Ischemia–Reperfusion Injury. Front. Cardiovasc. Med. 8:649785. doi: 10.3389/fcvm.2021.649785
Received: 05 January 2021; Accepted: 22 March 2021;
Published: 13 April 2021.
Edited by:
Nirmal Parajuli, Henry Ford Health System, United StatesReviewed by:
Mahesh Ramalingam, Chonnam National University Medical School, South KoreaCopyright © 2021 Wang, Liu, Liang, Naruse and Takahashi. This is an open-access article distributed under the terms of the Creative Commons Attribution License (CC BY). The use, distribution or reproduction in other forums is permitted, provided the original author(s) and the copyright owner(s) are credited and that the original publication in this journal is cited, in accordance with accepted academic practice. No use, distribution or reproduction is permitted which does not comply with these terms.
*Correspondence: Ken Takahashi, dGFrYWgtazJAb2theWFtYS11LmFjLmpw
Disclaimer: All claims expressed in this article are solely those of the authors and do not necessarily represent those of their affiliated organizations, or those of the publisher, the editors and the reviewers. Any product that may be evaluated in this article or claim that may be made by its manufacturer is not guaranteed or endorsed by the publisher.
Research integrity at Frontiers
Learn more about the work of our research integrity team to safeguard the quality of each article we publish.