- 1Critical Care Research Group, The Prince Charles Hospital, Chermside, QLD, Australia
- 2Faculty of Medicine, University of Queensland, St Lucia, QLD, Australia
- 3Science and Engineering Faculty, Queensland University of Technology, Brisbane, QLD, Australia
- 4Division of Cardiac Surgery, The Ohio State University Wexner Medical Center, Columbus, OH, United States
- 5Department of Global Health and Infection, Brighton and Sussex Medical School, Brighton, United Kingdom
- 6Oxford University Clinical Research Unit, Wellcome Trust Africa Asia Programme, Ho Chi Minh City, Vietnam
- 7Centre for Tropical Medicine and Global Health, University of Oxford, Oxford, United Kingdom
- 8Division of Infectious Diseases and Vaccinology, School of Public Health, University of California, Berkeley, Berkeley, CA, United States
All human cells are coated by a surface layer of proteoglycans, glycosaminoglycans (GAGs) and plasma proteins, called the glycocalyx. The glycocalyx transmits shear stress to the cytoskeleton of endothelial cells, maintains a selective permeability barrier, and modulates adhesion of blood leukocytes and platelets. Major components of the glycocalyx, including syndecans, heparan sulfate, and hyaluronan, are shed from the endothelial surface layer during conditions including ischaemia and hypoxia, sepsis, atherosclerosis, diabetes, renal disease, and some viral infections. Studying mechanisms of glycocalyx damage in vivo can be challenging due to the complexity of immuno-inflammatory responses which are inextricably involved. Previously, both static as well as perfused in vitro models have studied the glycocalyx, and have reported either imaging data, assessment of barrier function, or interactions of blood components with the endothelial monolayer. To date, no model has simultaneously incorporated all these features at once, however such a model would arguably enhance the study of vasculopathic processes. This review compiles a series of current in vitro models described in the literature that have targeted the glycocalyx layer, their limitations, and potential opportunities for further developments in this field.
Introduction
All cells in humans are coated by a surface layer of glycans called the glycocalyx (1, 2). This coating is a matrix consisting of various proteoglycans, glycosaminoglycans (GAGs), and plasma proteins, and it provides endothelial cellular mechano-sensation and transduction (3). Its principal GAGs include heparan sulfate (HS) and hyaluronic acid (HA), and core proteins primarily include syndecans and glypicans (1). The composition of the glycocalyx is in a state of constant flux, as it continuously replenishes components that are removed by flowing plasma (4). The glycocalyx offers a scaffold to which plasma proteins and GAGs may bind (4, 5), and remains an inactive structure until plasma constituents are bound, at which point it becomes the physiologically active endothelial surface layer (6).
In health, the glycocalyx performs a number of physiological functions, including the exertion of profound influence of shear stress on the vascular wall, maintenance of a selective permeability barrier and a low hydraulic conductivity, and modulation of adhesion of blood leukocytes and platelets (7). These responses are vital for the regulation of blood pressure, maintenance of tissue perfusion, and control of leukocyte recruitment during innate immune responses and inflammation (8). In particular, its constituent GAGs play a key role in cell-cell signaling and tissue injury. Innate immune cells, such as fibroblasts, mast cells, dendritic cells, monocytes and neutrophils recognize pathogenic invasion using pattern recognition receptors. When activated, these receptors drive signaling cascades which ultimately recruit leucocytes to the site of tissue injury (9). Furthermore, cytokines such as tumor necrosis factor (TNF) and interleukin 1 (IL1) promote leukocyte extravasation by increasing the levels of leukocyte adhesion molecules on endothelial cells (9). In a murine model by Wang et al. reducing sulphation by 60% with an endothelium-specific knockout of N-acetyl glucosamine N-deacetylase-N-sulfotransferase-1, which is required for the addition of sulfate to heparan sulfate chains, decreased neutrophil infiltration (10). This emphasizes the extent to which glycocalyx integrity modulates feedback between innate immune responses and inflammation.
The glycocalyx is also critically involved in angiogenesis. A study by Park-Windol et al. reported that in vitro knockout of endomucin-1, an integral membrane glycocalyx glycoprotein, reduced migration, inhibited cell growth without compromising cell survival, and suppressed tube morphogenesis of endothelial cells, whereas over-expression of endomucin-1 led to increased migration, proliferation and tube formation (11).
During homeostatic conditions, the glycocalyx lies in dynamic equilibrium between shedding of its components following the shear stress of blood flow, and de novo synthesis of its components, such as hyaluronic acid, by endothelial cells (12). The glycocalyx, being a dynamic structure, is also readily susceptible to injury. Destruction of the endothelial glycocalyx, which ranges from 200 to 2,000 nm in thickness, decreases vascular barrier function and leads to protein extravasation and tissue oedema, loss of substrate supply to tissues, and an increase in platelet and leucocyte adhesion (13). Major components of the glycocalyx, including syndecans, heparan sulfate, and hyaluronan, are shed from the endothelial surface layer under various acute and chronic clinical conditions, with the best characterized examples including ischaemia and hypoxia, sepsis, atherosclerosis, diabetes, renal disease, and haemorrhagic viral infections (7). Critically, heparan sulfate and syndecan-1 are released into the circulation during periods of ischaemia-reperfusion injury, and these levels reduce accordingly during therapeutic intervention (14). These may therefore be used as surrogate biomarkers of endothelial injury (14). Furthermore, syndecans have been shown to act as soluble messengers. Jannaway et al. demonstrated that thrombin can cleave syndecan 3/4 ectodomain into fragments which interact with endothelial cells, causing paracellular hyperpermeability (15). Through contact independent signaling, this vascular leakage may be amplified by perivascular cells, such as in the case of Dengue viral protein NS1 (16).
There has been a significant increase in academic interest in the role of the glycocalyx in cardiovascular pathophysiology. Previously, both static as well as perfused in vitro models have reported imaging data of glycocalyx components under flow, assessment of barrier function, or the interplay between blood components (e.g., leucocytes) and the glycocalyx. No model to date has combined all of these parameters, however this would be highly advantageous in the study of a vast array of pathophysiological states (e.g., infective vasculopathies, ischaemia-reperfusion injury, shock, sepsis, responses to foreign antigens). This paper aims to review current in vitro models which study glycocalyx pathophysiology, with an aim to identify areas of potential further development in this field.
Studying the Endothelial Glycocalyx: Models and Imaging Techniques
Given the greatly increased level of interest in the endothelial glycocalyx in recent years, a wide range of in vitro models to study the glycocalyx layer have been developed, integrating a variety of imaging modalities. These models have been used to study numerous pathophysiological states, including hyperglycaemia, endotheliopathy of shock, and ischaemic preconditioning (17–19). A summary of selected in vitro models in the literature is given in Table 1.
With reported thicknesses of the glycocalyx being in the order of nanometres, imaging modalities form a critical component of any model making an assessment of the glycocalyx in vivo, as well as the glycocalyx layer in vitro, and this field has evolved considerably. The first imaging of the endothelial glycocalyx by electron microscopy (EM) used the cationic dye ruthenium red (31), with subsequent studies using gold colloids and immunoperoxidase labeling (32). A major disadvantage of these methods was the degradation of the glycocalyx, which occurred with dehydration associated with fixative agents. Many of the methods incorporating EM yielded endothelial glycocalyx thicknesses <100 nm in size (3).
Newer imaging methods to visualize the glycocalyx have since evolved. These include laser scanning confocal microscopy and multi-photon microscopy, with fluorescently labeled antibodies to heparan sulfate or hyaluronic acid binding protein, or wheat germ agglutinin (which binds to sialic acid) to bind to components of the endothelial glycocalyx. In comparison to EM, application of these methods has demonstrated endothelial glycocalyx thicknesses in large blood vessels of 4,300–4,500 nm in the mouse common carotid artery, 2,200 nm in the mouse internal carotid artery, and 2,500 nm in the external carotid artery (33–35). Novel cryogenic protocols combined with electron microscopy have yielded promising results, with one study by Ebong et al. demonstrating that a rapid freezing/freeze substitution method combined with transmission electron microscopy revealed substantial glycocalyx layers on cultured endothelial cells (26). However, more recently, chemical and cryogenic fixation have been shown to lead to substantial collapse of the glycocalyx (36).
Sidestream dark field imaging (SDF) is a non-invasive technique and has been used to analyse the glycocalyx in vivo. In a bedside evaluation of the sublingual microvascular glycocalyx in sepsis, acutely ill patients demonstrated damage to the glycocalyx compared to healthy control subjects, with more severe alterations during sepsis (37). One observational study based in Vietnam demonstrated that in patients with Dengue, SDF imaging demonstrated disruption to the glycocalyx, with worse observed glycocalyx injury and higher plasma concentrations of degradation products being associated with worse plasma leakage. The authors reported that in patients with dengue, the perfused boundary region (PBR hf), a surrogate for glycocalyx degradation, was higher in patients with Grade 2 vs. Grade 0 plasma leakage during the critical phase (PBR hf 1.96 vs. 1.36 μm for Grade 2 vs. Grade 0 plasma leakage on days 4–6, respectively, p < 0.001) (38). SDF, unfortunately, also has associated drawbacks including that it is prone to impaired visualization of capillaries, as well as interference of moving blood components, such as erythrocytes, with the quality of the image obtained (39).
Importantly, techniques such as confocal microscopy and SDF are applicable both in vivo and in vitro, whilst the need to fix samples for scanning electron microscopy precludes its use in vivo. The pursuit of the optimal imaging modality for studying the glycocalyx remains a field of ongoing research.
Limitations of Existing in vitro Models
Whilst a number of studies have successfully demonstrated the structure, shedding and function of the endothelial glycocalyx layer across a range of different pathophysiological conditions, there are inherent limitations remaining with current in vitro models. These may be approached systematically using categories of (1) flow conditions, (2) materials employed, (3) assessment of endothelial barrier function, and (4) usage of blood products (Figure 1).
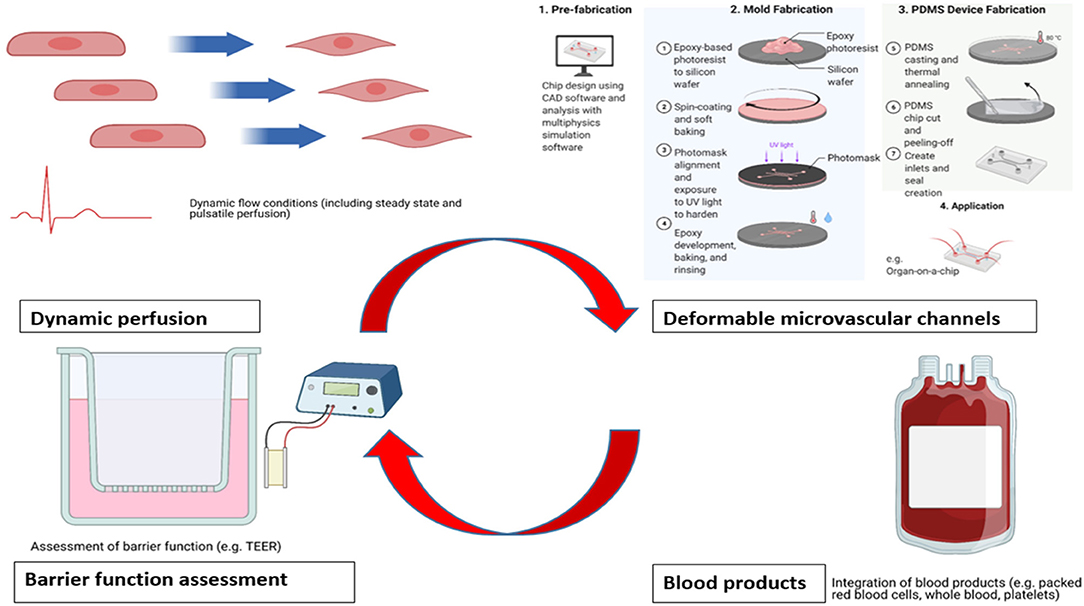
Figure 1. Areas for potential enhancement for existing in vitro benchtop models studying glycocalyx pathophysiology. Acknowledgment: Biorender.com.
Static vs. Dynamic Flow Conditions
A critical component in the investigation of the glycocalyx layer is the exposure of the cells to dynamic, rather than static, flow conditions that more closely model physiological conditions. The use of static endothelial cell models, usually Transwell systems, readily enables the assessment of endothelial barrier function and its response to chemical or biological stimuli (20). However, the morphology of cultured endothelial cells differs considerably under static compared to perfused conditions, and the thickness of the glycocalyx has been shown to be limited under static conditions, which diminishes its physiological relevance (40). Ueda et al. reported a glycocalyx thickness under static conditions of ~22 nm under static conditions (41), in comparison to Tsvirkun et al. who reported a glycocalyx thickness of ~600 nm when cultured under flow conditions (42). This was further corroborated by Gouverneur et al. who demonstrated that the application of fluid shear stress to cultured HUVECs resulted in a 3-fold higher level of hyaluronan binding protein compared to cells under static conditions. This increased incorporation of hyaluronan may well contribute vasculoprotective properties against inflammation and atherosclerosis to the glycocalyx (4).
Flow-induced shear stress is not only important for the development of the glycocalyx, but it also plays a critical role in maintaining endothelial cell integrity and mechanotransduction (43), particularly in specific cell signaling processes (44). Thi et al. showed that a stable glycocalyx layer is required for the endothelial cell cytoskeleton to respond to shear stress (45), which activates endothelial nitric oxide synthase (eNOS) for the production of nitric oxide (46). This enzyme is critical for modulating the control of vascular tone, leucocyte immigration, and blood clotting (47). Furthermore, the majority of studies conducted under flow conditions have integrated steady-state flow, which provides a constant shear stress on the endothelial cells. However, physiologic pulsatile flow delivers fluctuating shear stress. It has been shown previously that pulsatile flow will vary endothelial cell morphology, enzymatic activity and gene expression, compared to continuous flow (48–51); Uzarski et al. demonstrated that variable shear stress, with temporal changes in pulsatile flow, leads to anti-inflammatory and anti-thrombotic phenotype expression (50), in other words, consistent with the homeostatic vascular endothelium. Furthermore, previous studies have demonstrated that endothelial phenotypes have changed based on pulse frequency (52), pulse amplitude (53), and magnitude of shear stress (54). However, comparative studies of steady-state vs. pulsatile flow specifically on glycocalyx integrity are few. For greater translatability, future studies investigating endothelial and glycocalyx responses to inflammation, coagulation or arterial wall damage should integrate dynamic flow conditions as a priority, such as a comparison between steady state laminar fluid shear stress and pulsatile shear stresses, increased incrementally.
To cite one relevant clinical example, the effect of pulsatile flow on the microcirculation remains a novel field of significant academic inquiry. Koning et al. reported outcomes of pulsatile vs. non-pulsatile flow on microcirculatory perfusion in a clinical study of patients undergoing coronary artery bypass graft surgery. Sublingual mucosal microvascular perfusion was measured using sidestream dark field imaging. The observed reduction in perfused vessel density during aorta cross-clamping was only restored in the pulsatile flow group and increased from 15.5 ± 2.4 to 20.3 ± 3.7 mm/mm2 upon intensive care admission (P < 0.01). The median post-operative microvascular flow index was higher in the pulsatile group [2.6 (2.5–2.9)] than in the non-pulsatile group [2.1 (1.7–2.5); P = 0.001] (55).
Material Selection and Channel Dimensions
The materials used in perfused microchannels can have a large influence on cell morphology and the thickness of the glycocalyx developed, as the materials will have different mechanical properties than the native ECM, thereby changing the stiffness and associated mechanical environment stimulation of the endothelial cells. Microfluidics is the technology of fluid manipulation in channels with dimensions of tens of micrometers (56). Selecting a material for a microfluidics application can be a sophisticated process. Flexibility, air permeability, electrical conductivity, non-specific adsorption, cellular compatibility, solvent compatibility and optical transparency are all physical characteristics nominated by Nge et al. as being potentially significant factors affecting choice of material used in microfluidics applications (57). For the purposes of microfluidic models to study the endothelium, biocompatible channel materials that will promote cell adhesion, growth and differentiation with minimal inflammatory response are highly favored (58). Silicon and glass were the original materials used for this purpose. Collagen scaffolds were also used for early in vitro models that successfully demonstrated confluent monolayers of endothelial cells. Subsequent studies built on this concept, extending bioremodelable hydrogels (type I collagen) to develop 3D microvascular networks, with user-defined geometries (59). This was followed by a shift to polymer substrates, and in particular, polydimethylsiloxane (PDMS) (57), which is able to be manufactured effectively into small-diameter channels (<1,000 μm) if required and provides sufficient mechanical stiffness to support physiologically relevant flows at this size.
PDMS has also been shown to support more sophisticated, fully endothelialized microvascular networks. Tsvirkun et al. first successfully demonstrated that endothelial cells confined within microchannels and exposed to a physiologically relevant level of fluid shear stress exhibit a glycocalyx that lines the entire lumen of the channels (42). Importantly, PDMS has also demonstrated pressure-induced deformation under flow, which is important for simulating vessel compliance (60). One significant drawback of PDMS is its ability to absorb very small molecules, which may not be desirable for certain applications (e.g., drugs) (61).
Flow channel dimensions, whilst influential on platelet-surface and platelet-platelet interactions, are not widely reported in existing models. The shift from platelet-surface interactions or platelet-platelet interactions occurs as a function of channel size and aspect ratio (height/width) with values of <0.2 are being recommended to achieve constant shear stress, and hence laminar flow (62).
Assessment of Endothelial Barrier Function
The demonstration of endothelial barrier function is another important consideration and limitation in current models, in order to confirm that the presence of observed structural changes in glycocalyx components are occurring within a functioning endothelium. A critical characteristic of the endothelium is its barrier function; therefore, a model that stipulates that it has replicated the glycocalyx in vitro should also demonstrate that its barrier function is maintained. Commonly used examples include direct methods, such as transendothelial electrical resistance (TEER), or indirect methods using a permeability coefficient from transendothelial filtration of tracer molecules (e.g., FITC-Dextran).
TEER measurements have become the gold standard for assessing endothelial monolayer integrity, which typically consist of a volt-ohm resistance meters fitted with “chopstick” or chamber electrodes (63). When serial resistance measurements are taken over time, increasing confluence of the endothelial monolayer is then reflected by stabilization of resistance. This may also be corroborated with an observed increase in confluence, as demonstrated by Ferrell et al. in a microfluidic bioreactor with integrated TEER capability (64). Advantages of this method include its relative simplicity and reproducibility (65); however, it does not demonstrate whether the transition of a molecule of interest across the endothelial barrier, such as a protein, occurs or not (66).
In contrast, assessing endothelial barrier function using permeability studies involve the measured diffusion of a known concentration of analyte from the apical to basolateral side of the membrane over a predetermined period of time, also known as solute flux assays. Commonly used substances to perform this include fluorescein isothiocyanate (FITC)–dextran, which is a 3–5 kDa marker used to measure tight junction permeability (67, 68). A disadvantage of this technique is that it does not accurately represent the microvascular environment, due to static conditions on the apical side of the membrane (63). This is a particularly important drawback to note in the development of models of vasculopathic processes, as it may hinder the capacity of such models to study the impact of pathological stimuli and therapeutic interventions. It should also be noted that maintained barrier function alone is not necessarily an absolute indication of a fully functionally intact glycocalyx. Therefore, in order to address this concern, other surrogates for glycocalyx integrity must be considered. For example, an intact glycocalyx is also a critical regulator of cell membrane expression of gap junction proteins (69). On the other hand, degraded HS from the glycocalyx during injury will cause the dislocation of gap junction alpha-1 protein (Cx43) and malfunction of gap junction channels (69). Assessing immunohistochemical surrogates for glycocalyx integrity, such as Cx-containing gap junction activity by measuring interendothelial spread of gap junction permeable Lucifer Yellow dye (69), is one potential method to circumvent this issue.
Use of Blood Products
Finally, the glycocalyx modulates adhesion of leukocytes and platelets to the endothelium. Incorporating blood products (such as packed red blood cells, leucocytes, or platelets), allows the study of the interplay between endothelial damage and immuno-inflammatory responses, which is not possible if the perfusate is only cell media. Therefore, an important component in modeling glycocalyx layer structure and function in vitro is its exposure to whole blood, blood products, or combinations of the two (70). Whilst there are inherent difficulties in perfusion culture of endothelial cells with whole blood, the consideration of blood-endothelial interactions is nonetheless important. However, the majority of studies investigating the glycocalyx layer in vitro have used cell culture media as a perfusate, rather than blood (Table 1). Urner et al. demonstrated that incubation with blood products was associated with increased inflammatory mediator release from endothelial cells (71), whilst McDonald et al. demonstrated that enzymatic degradation of the endothelial glycocalyx in cultured endothelial cells under flow resulted in the development of a proinflammatory phenotype and an increase in leukocyte adhesion (27). Thus, studies using microfluidic devices incorporating RBCs or whole blood are warranted. Anticoagulation is a necessary addition for whole-blood perfusion studies to minimize thrombus formation; however, this limits endothelial-blood studies investigating platelet activation or aggregation (72). Whole blood is also opaque, which makes real-time imaging of blood-endothelial interactions difficult (73); however, diluted blood may still allow for microscopic analysis and therefore retain many advantages of using blood.
The selection of endothelial cells is a significant, influential variable. Dong et al. quantitatively examined different sources of endothelial cells in flow studies. They reported that the number of ultralong Von Willebrand Factor (VWF) strings is endothelial source dependent, with fewer ultralarge VWF strings forming on human coronary artery endothelial cells and human lung microvascular endothelial cells, both used after 4–5 passages, than on primary HUVECs and human umbilical artery endothelial cells (74).
Finally, it has been demonstrated by Lanotte et al. that flow resistance in a microcapillary model could more closely resemble in vivo scenarios, with a reduction in the velocity of red blood cells when microcapillaries were coated with a polymer brush to mimic the glycocalyx (75). This has potential implications for red blood cell deformation and mechanotransduction of the endothelial cells and further reinforces the utility of integrating blood products.
Discussion
There is a significant unmet global need in targeted therapeutics for endothelial dysfunction. To cite one notorious example, Dengue is regarded as the most prevalent and rapidly spreading mosquito-borne viral disease of human beings (76). Whilst the course of this disease is most frequently self-limiting, a small proportion will progress to a syndrome characterized by increased capillary permeability, extravasation of plasma and in some cases, irreversible shock and death. Currently, there are no targeted treatment options available, with supportive care being the cornerstone of management.
Therefore, there is high demand for authentic benchtop models which can examine vascular pathophysiology, as well as opportunities for targeted therapeutic strategies, which includes the glycocalyx. The concept of an “ideal” in vitro model is attractive, namely because it carries the benefit of tighter control of experimental variables. In order to advance this field, the four areas identified by the authors to enhance current in vitro model design have therefore included the use of dynamic flow conditions, material selection, assessment of barrier function and usage of blood products, as described in section Limitations of Existing in vitro Models. Their justification, as well as particular controversies which remain in the study of the glycocalyx in vitro vs. in vivo, are now discussed as follows.
Firstly, in vitro models carry the distinct advantage that the glycocalyx layer and its responses to individual variables may be studied in a highly controlled environment, and therefore they support mechanistic lines of scientific inquiry. In contrast, in vivo models offer the distinct advantage of being able to capture critical biological feedback mechanisms, such as those between the glycocalyx and acute inflammatory mediators, which is extremely difficult to replicate in vitro. Furthermore, whilst the dimensions of the glycocalyx are highly dynamic and will fluctuate with homeostatic mechanisms, there remains considerable variation in the literature between dimensions described from in vivo and in vitro models. Variations in measured thicknesses of the glycocalyx are even observed from within the same type of human cell. Chappell et al. reported an average thickness of 878 +/– 612 nm in six ex vivo umbilical cords, compared to cultured HUVECs with an average thickness of 29.4 ± 5.8 nm for the dense zone and of 117.9 ± 39.1 nm for the outer zone (40). Previous studies have even declared that the glycocalyx observed in vivo did not exist in vitro at all, although the conditions under which cells have been cultured should be carefully noted. One study by Potter and Damiano described that the glycocalyx observed in microvessels in vivo was ~520 nm in thickness, but only ~30 nm in thickness in vitro in human umbilical vein endothelial cells under standard cell culture conditions (77). This variation of glycocalyx dimensions in the literature is important, because it effectively forms the “confidence interval” within which the efficacy of targeted endothelial protection strategies on glycocalyx preservation may be determined.
Secondly, another area of controversy is that whilst glycocalyx components may be demonstrated through imaging, this does not necessarily correspond to a functioning endothelium. A critical characteristic of the endothelium is its barrier function; therefore, a model that stipulates that it has replicated the glycocalyx in vitro should also demonstrate that its barrier function is maintained. Whilst several methods, including TEER and permeability assays, have been well-documented to demonstrate endothelial barrier function, they are not always included alongside imaging or quantitative studies of the glycocalyx. Their usage should arguably now be the minimum standard in any in vitro model, and not an optional inclusion.
Thirdly, and as a corollary from the preceding two points, many models described in the literature have so far concentrated on providing imaging of the glycocalyx, demonstrating alterations in its level of permeability, or studying specific interactions with blood components or proteins of interest. The majority of these have been demonstrated in a static environment. Very few, if any, models have been able to integrate all of these aspects into one model; in other words, demonstrate the glycocalyx visually, along with assessment of its barrier function, in a dynamic flow environment. The ideal model should be able to perform all of these functions simultaneously.
The addition of blood products to in vitro microfluidics models represents an attractive frontier to replicate vascular pathophysiological processes, such as thrombosis, more faithfully. However, the study of thrombosis specifically applied to microfluidics devices is notoriously difficult. By definition, microfluidic models are built around the precise control of flow rates and volumes in the order of microlitres, or picolitres. As a result of these minute volumes, it therefore becomes difficult to obtain Reynolds numbers of sufficient magnitude to obtain boundary layer separation and reattachment representative of downstream turbulent flow from a stenotic lesion (78).
The study of coagulation within microfluidics devices is still in its relative infancy. The performance of conventional anticoagulants, such as heparin, which is readily used clinically and in vivo, can vary significantly in the setting of microfluidics. Two papers are worthy of note in this regard. Firstly, Harris et al. reported that in a model of human blood being perfused over porcine endothelial cells, there was a strong reduction in platelet surface area coverage and thrombus volume upon treatment of heparinized whole blood with the thrombin inhibitor bivalirudin. This implied that anticoagulation with heparin was insufficient to block thrombin generation in this model (79). Secondly, Ciciliano et al. recalcified citrated blood to allow coagulation to occur, and reported a reduction in channel occlusion with hirudin and heparin in this setup (80).
Conclusion
The glycocalyx is an essential homeostatic organ and is widely implicated in medical and surgical pathophysiology. It is also an elusive, dynamic structure to investigate. Whilst true dimensions of the glycocalyx may indeed be variable, what remains an essential priority is that in vitro model designs advance to the level whereby the endothelium is simultaneously able to be cultured and studied under perfusion, its surface layer is able to be consistently imaged, and its barrier function able to be assessed dynamically. A model of this kind would be of tremendous value in developing targeted therapeutic interventions for vasculopathic conditions.
Author Contributions
AH, NB, and EW prepared the manuscript. MV, AM, SY, SB, EH, JS, and JF provided expert opinion, critical review, and feedback on the manuscript. All authors contributed to the article and approved the submitted version.
Conflict of Interest
The authors declare that the research was conducted in the absence of any commercial or financial relationships that could be construed as a potential conflict of interest.
References
1. Tarbell JM, Cancel LM. The glycocalyx and its significance in human medicine. J Intern Med. (2016) 280:97–113. doi: 10.1111/joim.12465
2. Möckl L. The emerging role of the mammalian glycocalyx in functional membrane organization and immune system regulation. Front Cell Dev Biol. (2020) 8:253. doi: 10.3389/fcell.2020.00253
3. Fu BM, Tarbell JM. Mechano-sensing and transduction by endothelial surface glycocalyx: composition, structure, and function. Wiley Interdiscip Rev Syst Biol Med. (2013) 5:381–90. doi: 10.1002/wsbm.1211
4. Gouverneur M, Spaan JAE, Pannekoek H, Fontijn RD, Vink H. Fluid shear stress stimulates incorporation of hyaluronan into endothelial cell glycocalyx. Am J Physiol Heart Circu Physiol. (2006) 290:H458–2. doi: 10.1152/ajpheart.00592.2005
5. van den Berg BM, Nieuwdorp M, Stroes ES, Vink H. Glycocalyx and endothelial (dys) function: from mice to men. Pharmacol Rep. (2006) 58:75–80.
6. Jacob M, Bruegger D, Rehm M, Stoeckelhuber M, Welsch U, Conzen P, et al. The endothelial glycocalyx affords compatibility of Starling's principle and high cardiac interstitial albumin levels. Cardiovasc Res. (2007) 73:575–86. doi: 10.1016/j.cardiores.2006.11.021
7. Becker BF, Jacob M, Leipert S, Salmon AHJ, Chappell D. Degradation of the endothelial glycocalyx in clinical settings: searching for the sheddases. Br J Clin Pharmacol. (2015) 80:389–402. doi: 10.1111/bcp.12629
8. Zeng Y, Tarbell JM. The adaptive remodeling of endothelial glycocalyx in response to fluid shear stress. PLoS ONE. (2014) 9:e86249. doi: 10.1371/journal.pone.0086249
9. Newton K, Dixit VM. Signaling in innate immunity and inflammation. Cold Spring Harb Perspect Biol. (2012) 4:a006049. doi: 10.1101/cshperspect.a006049
10. Wang L, Fuster M, Sriramarao P, Esko JD. Endothelial heparan sulfate deficiency impairs L-selectin- and chemokine-mediated neutrophil trafficking during inflammatory responses. Nat Immunol. (2005) 6:902–10. doi: 10.1038/ni1233
11. Park-Windhol C, Ng YS, Yang J, Primo V, Saint-Geniez M, D'Amore PA. Endomucin inhibits VEGF-induced endothelial cell migration, growth, and morphogenesis by modulating VEGFR2 signaling. Sci Rep. (2017) 7:17138. doi: 10.1038/s41598-017-16852-x
12. Dogné S, Flamion B, Caron N. Endothelial glycocalyx as a shield against diabetic vascular complications. Arteriosc Thromb Vasc Biol. (2018) 38:1427–39. doi: 10.1161/ATVBAHA.118.310839
13. Becker BF, Chappell D, Bruegger D, Annecke T, Jacob M. Therapeutic strategies targeting the endothelial glycocalyx: acute deficits, but great potential. Cardiovasc Res. (2010) 87:300–10. doi: 10.1093/cvr/cvq137
14. Abassi Z, Armaly Z, Heyman SN. Glycocalyx degradation in ischemia-reperfusion injury. Am J Pathol. (2020) 190:752–67. doi: 10.1016/j.ajpath.2019.08.019
15. Jannaway M, Yang X, Meegan JE, Coleman DC, Yuan SY. Thrombin-cleaved syndecan-3/-4 ectodomain fragments mediate endothelial barrier dysfunction. PLoS ONE. (2019) 14:e0214737. doi: 10.1371/journal.pone.0214737
16. Cheung YP, Mastrullo V, Maselli D, Butsabong T, Madeddu P, Maringer K, et al. A critical role for perivascular cells in amplifying vascular leakage induced by dengue virus nonstructural protein 1. mSphere. (2020) 5:e00258–20. doi: 10.1128/mSphere.00258-20
17. Diebel LN, Diebel ME, Martin JV, Liberati DM. Acute hyperglycemia exacerbates trauma-induced endothelial and glycocalyx injury: an in vitro model. J Trauma Acute Care Surg. (2018) 85:960–7. doi: 10.1097/TA.0000000000001993
18. Diebel LN, Martin JV, Liberati DM. Early tranexamic acid administration ameliorates the endotheliopathy of trauma and shock in an in vitro model. J Trauma Acute Care Surg. (2017) 82:1080–6. doi: 10.1097/TA.0000000000001445
19. Beresewicz A, Czarnowska E, Maczewski M. Ischemic preconditioning and superoxide dismutase protect against endothelial dysfunction and endothelium glycocalyx disruption in the postischemic guinea-pig hearts. Mol Cell Biochem. (1998) 186:87–97. doi: 10.1007/978-1-4615-4979-6_11
20. Martin JV, Liberati DM, Diebel LN. Disparate effects of catecholamines under stress conditions on endothelial glycocalyx injury: an in vitro model. Am J Surg. (2017) 214:1166–72. doi: 10.1016/j.amjsurg.2017.09.018
21. Puerta-Guardo H, Glasner DR, Harris E. Dengue virus NS1 disrupts the endothelial glycocalyx, leading to hyperpermeability. PLOS Pathog. (2016) 12:e1005738. doi: 10.1371/journal.ppat.1005738
22. Puerta-Guardo H, Glasner DR, Espinosa DA, Biering SB, Patana M, Ratnasiri K, et al. Flavivirus NS1 triggers tissue-specific vascular endothelial dysfunction reflecting disease tropism. Cell Rep. (2019) 26:1598–613.e8. doi: 10.1016/j.celrep.2019.01.036
23. Wang GH, Ma KL, Zhang Y, Hu ZB, Liu L, Lu J, et al. Platelet microparticles contribute to aortic vascular endothelial injury in diabetes via the mTORC1 pathway. Acta Pharmacol Sin. (2019) 40:468–76. doi: 10.1038/s41401-018-0186-4
24. Butler MJ, Ramnath R, Kadoya H, Desposito D, Riquier-Brison A, Ferguson JK, et al. Aldosterone induces albuminuria via matrix metalloproteinase-dependent damage of the endothelial glycocalyx. Kidney Int. (2019) 95:94–107. doi: 10.1016/j.kint.2018.08.024
25. Glasner DR, Ratnasiri K, Puerta-Guardo H, Espinosa DA, Beatty PR, Harris E. Dengue virus NS1 cytokine-independent vascular leak is dependent on endothelial glycocalyx components. PLoS Pathog. (2017) 13:e1006673. doi: 10.1371/journal.ppat.1006673
26. Ebong EE, Macaluso FP, Spray DC, Tarbell JM. Imaging the endothelial glycocalyx in vitro by rapid freezing/freeze substitution transmission electron microscopy. Arterioscler Thromb Vasc Biol. (2011) 31:1908–15. doi: 10.1161/ATVBAHA.111.225268
27. McDonald KK, Cooper S, Danielzak L, Leask RL Glycocalyx degradation induces a proinflammatory phenotype and increased leukocyte adhesion in cultured endothelial cells under flow. PLoS ONE. (2016) 11:e0167576. doi: 10.1371/journal.pone.0167576
28. Potter DR, Jiang J, Damiano ER. The recovery time course of the endothelial cell glycocalyx in vivo and its implications in vitro. Circu Res. (2009) 104:1318–25. doi: 10.1161/CIRCRESAHA.108.191585
29. Yao Y, Rabodzey A, Dewey CF Jr. Glycocalyx modulates the motility and proliferative response of vascular endothelium to fluid shear stress. Am J Physiol Heart Circ Physiol. (2007) 293:H1023–30. doi: 10.1152/ajpheart.00162.2007
30. Bai K, Wang W. Shear stress-induced redistribution of the glycocalyx on endothelial cells in vitro. Biomech Model Mechanobiol. (2014) 13:303–11. doi: 10.1007/s10237-013-0502-3
31. Luft JH. Fine structures of capillary and endocapillary layer as revealed by ruthenium red. Fed Proc. (1966) 25:1773–83.
32. Sarrazin S, Lamanna WC, Esko JD. Heparan sulfate proteoglycans. Cold Spring Harb Perspect Biol. (2011) 3:4952. doi: 10.1101/cshperspect.a004952
33. Megens RT, Reitsma S, Schiffers PH, Hilgers RH, De Mey JG, Slaaf DW, et al. Two-photon microscopy of vital murine elastic and muscular arteries. Combined structural and functional imaging with subcellular resolution. J Vasc Res. (2007) 44:87–98. doi: 10.1159/000098259
34. van den Berg BM, Spaan JA, Vink H. Impaired glycocalyx barrier properties contribute to enhanced intimal low-density lipoprotein accumulation at the carotid artery bifurcation in mice. Pflugers Arch. (2009) 457:1199–206. doi: 10.1007/s00424-008-0590-6
35. Reitsma S, oude Egbrink MG, Vink H, van den Berg BM, Passos VL, Engels W, et al. Endothelial glycocalyx structure in the intact carotid artery: a two-photon laser scanning microscopy study. J Vasc Res. (2011) 48:297–306. doi: 10.1159/000322176
36. Hempel C, Kapishnikov S. The need to freeze-Dehydration during specimen preparation for electron microscopy collapses the endothelial glycocalyx regardless of fixation method. Microcirculation. (2020) 27:e12643. doi: 10.1111/micc.12643
37. Donati A, Damiani E, Domizi R, Romano R, Adrario E, Pelaia P, et al. Alteration of the sublingual microvascular glycocalyx in critically ill patients. Microvasc Res. (2013) 90:86–9. doi: 10.1016/j.mvr.2013.08.007
38. Lam PK, McBride A D., Le HT, Huynh TT, Vink H, Wills B, et al. Visual and biochemical evidence of glycocalyx disruption in human dengue infection, and association with plasma leakage severity. Front Med. (2020) 7:545813. doi: 10.3389/fmed.2020.545813
39. Treu CM, Lupi O, Bottino DA, Bouskela E. Sidestream dark field imaging: the evolution of real-time visualization of cutaneous microcirculation and its potential application in dermatology. Arch Dermatol Res. (2011) 303:69–78. doi: 10.1007/s00403-010-1087-7
40. Chappell D, Jacob M, Paul O, Rehm M, Welsch U, Stoeckelhuber M, et al. The glycocalyx of the human umbilical vein endothelial cell: an impressive structure ex vivo but not in culture. Circ Res. (2009) 104:1313–7. doi: 10.1161/CIRCRESAHA.108.187831
41. Ueda A, Shimomura M, Ikeda M, Yamaguchi R, Tanishita K. Effect of glycocalyx on shear-dependent albumin uptake in endothelial cells. Am J Physiol Heart Circu Physiol. (2004) 287:H2287–94. doi: 10.1152/ajpheart.00808.2003
42. Tsvirkun D, Grichine A, Duperray A, Misbah C, Bureau L. Microvasculature on a chip: study of the endothelial surface layer and the flow structure of red blood cells. Sci Rep. (2017) 7:45036. doi: 10.1038/srep45036
43. Liu HQ, Li J, Xuan CL, Ma HC. A review on the physiological and pathophysiological role of endothelial glycocalyx. J Biochem Mol Toxicol. (2020) 34:e22571. doi: 10.1002/jbt.22571
44. Tarbell JM, Pahakis MY. Mechanotransduction and the glycocalyx. J Intern Med. (2006) 259:339–50. doi: 10.1111/j.1365-2796.2006.01620.x
45. Thi MM, Tarbell JM, Weinbaum S, Spray DC. The role of the glycocalyx in reorganization of the actin cytoskeleton under fluid shear stress: A “bumper-car” model. Proc Natl Acad Sci USA. (2004) 101:16483–8. doi: 10.1073/pnas.0407474101
46. Fisslthaler B, Dimmeler S, Hermann C, Busse R, Fleming I. Phosphorylation and activation of the endothelial nitric oxide synthase by fluid shear stress. Acta Physiol Scand. (2000) 168:81–8. doi: 10.1046/j.1365-201x.2000.00627.x
47. Heiss C, Rodriguez-Mateos A, Kelm M. Central role of eNOS in the maintenance of endothelial homeostasis. Antioxid Redox Signal. (2015) 22:1230–42. doi: 10.1089/ars.2014.6158
48. Blackman BR, García-Cardeña G, Gimbrone MA. A new in vitro model to evaluate differential responses of endothelial cells to simulated arterial shear stress waveforms. J Biomech Eng. (2002) 124:397–407. doi: 10.1115/1.1486468
49. Dekker RJ, van Thienen JV, Rohlena J, de Jager SC, Elderkamp YW, Seppen J, et al. Endothelial KLF2 links local arterial shear stress levels to the expression of vascular tone-regulating genes. Am J Pathol. (2005) 167:609–18. doi: 10.1016/S0002-9440(10)63002-7
50. Uzarski JS, Scott EW, McFetridge PS. Adaptation of endothelial cells to physiologically-modeled, variable shear stress. PLoS ONE. (2013) 8:e57004. doi: 10.1371/journal.pone.0057004
51. Yee A, Bosworth K, Conway D, Eskin S, McIntire L. Gene expression of endothelial cells under pulsatile non-reversing vs. steady shear stress; comparison of nitric oxide production. Ann Biomed Eng. (2008) 36:571–9. doi: 10.1007/s10439-008-9452-9
52. Himburg HA, Dowd SE, Friedman MH. Frequency-dependent response of the vascular endothelium to pulsatile shear stress. Am J Physiol Heart Circ Physiol. (2007) 293:H645. doi: 10.1152/ajpheart.01087.2006
53. Li M, Scott DE, Shandas R, Stenmark KR, Tan W. High pulsatility flow induces adhesion molecule and cytokine mRNA expression in distal pulmonary artery endothelial cells. Ann Biomed Eng. (2009) 37:1082–92. doi: 10.1007/s10439-009-9684-3
54. Rouleau L, Rossi J, Leask RL. The response of human aortic endothelial cells in a stenotic hemodynamic environment: effect of duration, magnitude, and spatial gradients in wall shear stress. J Biomech Eng. (2010) 132:071015. doi: 10.1115/1.4001217
55. Koning NJ, Vonk AB, van Barneveld LJ, Beishuizen A, Atasever B, van den Brom CE, et al. Pulsatile flow during cardiopulmonary bypass preserves postoperative microcirculatory perfusion irrespective of systemic hemodynamics. J Appl Physiol. (2012) 112:1727–34. doi: 10.1152/japplphysiol.01191.2011
56. Whitesides GM. The origins and the future of microfluidics. Nature. (2006) 442:368–73. doi: 10.1038/nature05058
57. Nge PN, Rogers CI, Woolley AT. Advances in microfluidic materials, functions, integration, and applications. Chem Rev. (2013) 113:2550–83. doi: 10.1021/cr300337x
58. Tehranirokh M, Kouzani AZ, Francis PS, Kanwar JR. Microfluidic devices for cell cultivation and proliferation. Biomicrofluidics. (2013) 7:051502. doi: 10.1063/1.4826935
59. Morgan JP, Delnero PF, Zheng Y, Verbridge SS, Chen J, Craven M, et al. Formation of microvascular networks in vitro. Nat Protocls. (2013) 8:1820–36. doi: 10.1038/nprot.2013.110
60. Hunter L, Gala de Pablo, Stammers JAC, Thomson NH, Evans SD, Shim JU. On-chip pressure measurements and channel deformation after oil absorption. SN Appl Sci. (2020) 2:1501. doi: 10.1007/s42452-020-03288-8
61. van Meer BJ, de Vries H K., Firth SA, van Weerd J, Tertoolen LGJ, Karperien HBJ, et al. Small molecule absorption by PDMS in the context of drug response bioassays. Biochem Biophys Res Commun. (2017) 482:323–8. doi: 10.1016/j.bbrc.2016.11.062
62. Coenen DM, Mastenbroek TG. Platelet interaction with activated endothelium: mechanistic insights from microfluidics. Blood. (2017) 130:2819–28. doi: 10.1182/blood-2017-04-780825
63. Vogel PA, Halpin ST, Martin RS, Spence DM. Microfluidic transendothelial electrical resistance measurement device that enables blood flow and postgrowth experiments. Anal Chem. (2011) 83:4296–301. doi: 10.1021/ac2004746
64. Ferrell N, Desai RR, Fleischman AJ, Roy S, Humes HD, Fissell WH. A microfluidic bioreactor with integrated transepithelial electrical resistance (TEER) measurement electrodes for evaluation of renal epithelial cells. Biotechnol Bioeng. (2010) 107:707–16. doi: 10.1002/bit.22835
65. Li BR, Wu J, Li HS, Jiang ZH, Zhou XM, Xu CX, et al. In vitro and in vivo approaches to determine intestinal epithelial cell permeability. J Vis Exp. (2018) e57032. doi: 10.3791/57032
66. Pauty J, Usuba R, Takahashi H, Suehiro J, Fujisawa K, Yano K, et al. A vascular permeability assay using an in vitro human microvessel model mimicking the inflammatory condition. Nanotheranostics. (2017) 1:103–13. doi: 10.7150/ntno.18303
67. Yang J, Elbaz-Younes I, Primo C, Murungi D, Hirschi KD. Intestinal permeability, digestive stability and oral bioavailability of dietary small RNAs. Sci Rep. (2018) 8:10253. doi: 10.1038/s41598-018-28207-1
68. Baxter MFA, Merino-Guzman R, Latorre JD, Mahaffey BD, Yang Y, Teague KD, et al. Optimizing fluorescein isothiocyanate dextran measurement as a biomarker in a 24-h feed restriction model to induce gut permeability in broiler chickens. Front Vet Sci. (2017) 4:56. doi: 10.3389/fvets.2017.00056
69. Mensah SA, Cheng MJ, Homayoni H, Plouffe BD, Coury AJ, Ebong EE. Regeneration of glycocalyx by heparan sulfate and sphingosine 1-phosphate restores inter-endothelial communication. PLoS ONE. (2017) 12:e0186116. doi: 10.1371/journal.pone.0186116
70. Lipowsky HH. The endothelial glycocalyx as a barrier to leukocyte adhesion and its mediation by extracellular proteases. Ann Biomed Eng. (2012) 40:840–8. doi: 10.1007/s10439-011-0427-x
71. Urner M, Herrmann IK, Buddeberg F, Schuppli C, Roth Z'graggen B, Hasler M, et al. Effects of blood products on inflammatory response in endothelial cells in vitro. PLoS ONE. (2012) 7:e33403. doi: 10.1371/journal.pone.0033403
72. Hesh CA, Qiu Y, Lam WA. Vascularized microfluidics and the blood-endothelium interface. Micromachines. (2019) 11:18. doi: 10.3390/mi11010018
73. Monica SYN, Jacky YS, John-Paul T, John FF. Endothelialized flow models for blood transfusion research. Haematologica. (2019) 104:428–34. doi: 10.3324/haematol.2018.205203
74. Dong JF, Moake JL, Nolasco L, Bernardo A, Arceneaux W, Shrimpton CN, et al. ADAMTS-13 rapidly cleaves newly secreted ultralarge von Willebrand factor multimers on the endothelial surface under flowing conditions. Blood. (2002) 100:4033–9. doi: 10.1182/blood-2002-05-1401
75. Lanotte L, Tomaiuolo G, Misbah C, Bureau L, Guido S. Red blood cell dynamics in polymer brush-coated microcapillaries: a model of endothelial glycocalyx in vitro. Biomicrofluidics. (2014) 8:014104. doi: 10.1063/1.4863723
77. Potter DR, Damiano ER. The hydrodynamically relevant endothelial cell glycocalyx observed in vivo is absent in vitro. Circ Res. (2008) 102:770–6. doi: 10.1161/CIRCRESAHA.107.160226
78. Herbig BA, Yu X, Diamond SL. Using microfluidic devices to study thrombosis in pathological blood flows. Biomicrofluidics. (2018) 12:042201. doi: 10.1063/1.5021769
79. Harris DG, Benipal PK, Cheng X, Burdorf L, Azimzadeh AM, Pierson RN III. Four-dimensional characterization of thrombosis in a live-cell, shear-flow assay: development and application to xenotransplantation. PLoS ONE. (2015) 10:e0123015. doi: 10.1371/journal.pone.0123015
Keywords: endothelium, glycocalyx, vasculopathy, vascular, endothelial surface layer
Citation: Haymet AB, Bartnikowski N, Wood ES, Vallely MP, McBride A, Yacoub S, Biering SB, Harris E, Suen JY and Fraser JF (2021) Studying the Endothelial Glycocalyx in vitro: What Is Missing? Front. Cardiovasc. Med. 8:647086. doi: 10.3389/fcvm.2021.647086
Received: 26 January 2021; Accepted: 19 March 2021;
Published: 14 April 2021.
Edited by:
Hideshi Okada, Gifu University, JapanReviewed by:
Eno Ebong, Northeastern University, United StatesLawrence Diebel, Wayne State University, United States
Copyright © 2021 Haymet, Bartnikowski, Wood, Vallely, McBride, Yacoub, Biering, Harris, Suen and Fraser. This is an open-access article distributed under the terms of the Creative Commons Attribution License (CC BY). The use, distribution or reproduction in other forums is permitted, provided the original author(s) and the copyright owner(s) are credited and that the original publication in this journal is cited, in accordance with accepted academic practice. No use, distribution or reproduction is permitted which does not comply with these terms.
*Correspondence: Andrew B. Haymet, YS5oYXltZXRAZ21haWwuY29t