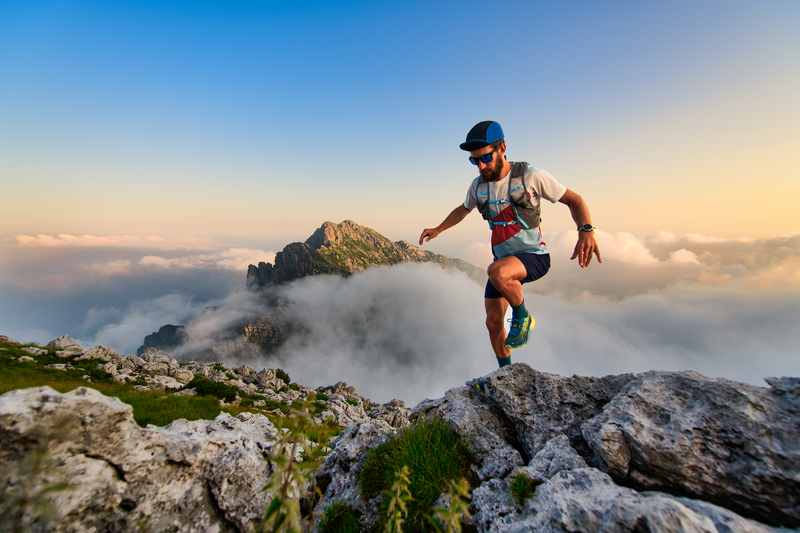
95% of researchers rate our articles as excellent or good
Learn more about the work of our research integrity team to safeguard the quality of each article we publish.
Find out more
METHODS article
Front. Cardiovasc. Med. , 04 June 2021
Sec. Pediatric Cardiology
Volume 8 - 2021 | https://doi.org/10.3389/fcvm.2021.642011
This article is part of the Research Topic Additive Manufacturing Applications in Cardiovascular Research View all 5 articles
This is a 7-years single institution study on low-cost cardiac three-dimensional (3D) printing based on the use of free open-source programs and affordable printers and materials. The process of 3D printing is based on several steps (image acquisition, segmentation, mesh optimization, slicing, and three-dimensional printing). The necessary technology and the processes to set up an affordable three-dimensional printing laboratory are hereby described in detail. Their impact on surgical and interventional planning, medical training, communication with patients and relatives, patients' perception on care, and new cardiac device development was analyzed. A total of 138 low-cost heart models were designed and printed from 2013 to 2020. All of them were from different congenital heart disease patients. The average time for segmentation and design of the hearts was 136 min; the average time for printing and cleaning the models was 13.5 h. The average production cost of the models was €85.7 per model. This is the most extensive series of 3D printed cardiac models published to date. In this study, the possibility of manufacturing three-dimensional printed heart models in a low-cost facility fulfilling the highest requirements from a technical and clinical point of view is demonstrated.
Personalized three-dimensional (3D) printed models have become a powerful tool for clinicians and surgeons in recent years. 3D printed models provide tactile feedback and enable users to simulate realistic anatomical navigation. All these operations are difficult to reproduce in computer software. 3D replicas offer an enhanced appreciation of the visuospatial relationship between anatomical structures (1). This can be translated into shorter operative time, reduced exposure to general anesthesia, shorter wound exposure time, and reduced intraoperative blood loss (2).
In recent years, researchers and clinicians have demonstrated that patient-specific heart models of different pathologies are useful to plan surgical and interventional procedures and also to teach students and communicate with patients and their families (1, 3–10). Different clinical areas have incorporated this technology in their current clinical practice including plastic (11), maxillofacial (12), orthopedic (13), or cardiac surgery (6).
Although many publications describe how useful 3D models are in different medical applications (14), one of the main issues of this technology is the high cost inherent to the equipment and software needed to start a 3D printing facility (15). More efforts are necessary to maximize the benefits of this solution while reducing the costs associated (16–18).
3D printing is a process that requires several complex steps, and it is necessary to study and analyze the investment needed for each one. The price of the software utilized for 3D printing varies from 0 to more than €10,000 per license and year. 3D printers can be purchased following a wide range of prices, ranging from a few hundred euros to more than €150,000. Materials also vary from €15/kg to more than €300/kg. All these expenses associated with the design and fabrication of the models are key factors that can complicate the translation of this technology from research to normal clinical practice.
The design and fabrication of a patient-specific 3D printed heart model has been previously described in the literature (3, 5, 19–21). In this article, we present our institutional experience using affordable technology over the last 7 years in the field of cardiac 3D printing, analyzing and describing each technical and economic factor involved in the design and manufacturing process. The aim of this article is to demonstrate the feasibility from a technical and economic point of view. The clinical utility of the models is briefly described in this article to contextualize the application of the technology.
Medical images from CT (computed tomography), CMR (cardiac magnetic resonance), or echocardiography are stored in the digital imaging and communication in medicine (DICOM) format and loaded into segmentation software.
There are several tools for segmentation; some of them are free and open source, and others are licensed. Mimics (Materialize NV, Leuven, Belgium) is a payment license program included in the Mimics Innovation Suite (~€10,000/license and years) and one of the most cited programs in scientific literature (22). Osirix (Pixmeo, Geneva, Switzerland) only runs on Mac; it has a free version and an FDA- and CE-approved version that costs €756 per year and license.
There are other popular free open-source alternatives in medical 3D printing such as MITK (German Cancer Research Center, Heidelberg, Germany), ITK Snap, and 3D Slicer.
All of them have similar reliability in the volume segmentation of the anatomy and similar accuracies (23). Image resolution under 1 mm is recommended to perform high-quality reconstructions (24). Some programs are able to perform a semi-automated segmentation, but it is mainly reliable on normal heart anatomies. For complex congenital heart disease, a manual segmentation is recommended. However, it can be a laborious task and user-dependent as it relies on the expertise on heart morphology and image processing of the person performing the segmentation (22).
Once the segmentation is finished, the mesh is exported as an STL file (standard tessellation language). The meshes directly obtained from the segmentation are not valid for 3D printing, and it is necessary to perform some modifications to the geometries. There is often noise derived from image artifacts or related to the lack of contrast in venous areas. When the image study has low spatial resolution, the meshes have irregular surfaces that do not accurately represent the true anatomy of the patient. All this inaccuracies have to be corrected in order to obtain a reliable replica of the anatomy.
Some of the most popular computer-aided design (CAD) programs have a yearly license fee. However, there is an increasing amount of options that are available for free. Some examples of free open-source software are as follows: Meshmixer (Autodesk Inc., San Rafael, CA, USA) and Blender (Blender Foundation, Amsterdam, Netherlands). Some licensed software packages are SketchUp (Trimble Inc, Sunnyvale, CA, USA) with research and professional versions ($49 and $695), SolidWorks (Solidworks, Dassault Systèmes, Waltham, MA, USA) from $150 to $8,000, ZBrush 4R8 (Pixologic, Los Angeles, CA, USA) $795, Rhinoceros (Mac Neel Europe, Barcelona, Spain) costing €195 to €1,695, and 3Matic (Materialize NV, Leuven, Belgium) included in the Mimics Innovation Suite (€10,000/license and year).
The last step of the process is the lamination or slicing. Some printers have its own programs like Objet Studio (Stratasys Ltd., Eden Prairie, MN, USA) for Stratasys printers, Z-Suite (Zortrax, Olsztyn, Poland) for Zortrax printers, PreForm (Formlabs, Somerville, MA, USA), and MakerBot Print (MakerBot Industries, LLC, Brooklyn, NY, USA) for MakerBot. Others can work with open-source software such as Cura (Ultimaker BV, Geldermalsen, Netherlands), Repetier (Hot-World, Willich, Germany), Slic3r, or KiSSlicer.
Prior to slicing, it is necessary to define the parameters of the printing process. Once finished, the 3D mesh is laminated in different layers and transferred to the 3D printer.
All 3D printing technologies are based on the same process, deposition of successive layers of material to create an object. The most relevant technologies used in medical field are Stereolithography, Polyjet, and Fused Deposition Modeling. The most important characteristics of each of these methods are summarized in Table 1.
Stereolithography (SLA) is based on the photopolimerization of epoxy resin layers with an ultraviolet (UV) light. Models need support structures to be printed; these supports are removed manually.
• Advantages: The precision of the pieces manufactured can reach 0.025 mm, and it is possible to make internal cavities and complex structures.
• Disadvantages: It is more expensive than other technologies because of the high cost of the printing materials and the maintenance required. Low-cost printers have a limited printing size. Max dimensions in Form2 (FormLabs Inc, Somerville, MA, USA) are 145 × 145 × 175 mm. The printer is affordable (€3,800), but flexible materials are more expensive (€190/L).
Polyjet (Stratasys Ltd., Eden Prairie, MN, USA) photopolymers are deposited in a wide area, and it is possible to print in different materials at the same time.
• Advantages: The support is dissolvable and washable in a subsequent step. The quality of the printing is similar to that of SLA. Flexible materials (Agilus and Tango) are soft and emulate the stiffness of cardiac tissues.
• Disadvantages: The maintenance required is considerable. Machines and materials are also expensive, and there are no low-cost options in the market (their price ranges from €30,000 to more than €150,000). A significant amount of support material is needed for each model, increasing the total cost (25). Flexible materials in Polyjet technology can break without much difficulty as they get easily torn. The cost is over €300/L.
Fused deposition modeling (FDM) is based on a continuous extrusion of a melted filament from a temperature-controlled nozzle. FDM is the most affordable and most commonly used technology for medical 3D printing.
The model is made by solidification and adhesion of fine lines of thermoplastic material to form the layers.
The most important FDM 3D printer machines are the following: Prusa i3 MK3S (Prusa Research, Prague, Czechia), LulzBot TAZ 6 (Aleph Objects, Inc., Loveland, CO, USA), and Ultimaker 3 (Ultimaker BV, Geldermasen, Netherlands).
• Advantages: There are lots of printer models under €4,000, and they are able to print with Z-axis precision under 0.05 mm. The majority of FDM 3D printers accept several rigid and flexible materials.
• Disadvantages: FDM low-cost printers have lower resolution, speed, and reliability than the other technologies. The flexibility of the models printed with flexible filaments in FDM printers is inferior to models printed in Polyjet printers.
Patients with congenital heart disease were recruited from several hospitals in Spain, United Kingdom, Netherlands, Italy, Germany, Lebanon, and Canada. Imaging datasets were transferred to our secure server. All 3D printing models were segmented and printed in our unit.
3D models were created from computed tomography (CT) or cardiac magnetic resonance (CMR).
The preference between one imaging technique or another was based on an institutional protocol, the main anatomical structure of interest to be visualized, and the age of the patient, with a tendency to use CMR in younger population to avoid radiation (26). 3D echocardiography was also utilized in combination with CT or CMR when cardiac valves were essential for the segmentation (27, 28).
Free open software ITK-SNAP was the program selected for segmentation (Figure 1). Different algorithms were used, such as thresholding, classification preset, clustering, and edge attraction. After the upper and lower thresholding levels were set, a number of seeds were applied. Seeds were grown using reiterative steps. When the growing seeds collided, the affected anatomical structures were manually separated.
Figure 1. ITK Snap Segmentation. ITK toolbox on the left side of the screen. Axial (up left), sagittal (up right), 3D reconstruction (down left), and coronal (down right) views in ITK-Snap. Thresholding and manual segmentation were applied to select blood pool (red) and coronary arteries (yellow).
We processed STL files in Meshmixer (Autodesk Inc., San Rafael, CA, USA). The meshes were analyzed with an automatic inspector tool to detect and repair errors (holes, isolated parts, and non-manifold surfaces). A 0.8-mm offset was applied to the meshes in order to keep the blood pool unmodified. Cantilever parts and slender supports were reduced or eliminated. The geometry was analyzed in every case to find the optimal orientation in the space prior to printing (Figure 2).
Figure 2. Tools and operations in Meshmixer. (A–C) Optimal printing positions of the heart model calculated by the orientation tool in Meshmixer. (D) Different anatomical structures segmented in ITK Snap. Blood pool in gray, pulmonary veins in red, and coronary in blue. (E) Smooth brush applied on the aorta surface.
When the model was composed of several parts (i.e., aorta, pulmonary veins, inferior and superior cava veins, atriums, ventricles, etc.), all the meshes were merged using the combine tool in Meshmixer (Figure 2).
We used the sculpting module in Meshmixer to shape meshes with different brushes and parameters (Figure 2).
Once the 3D modeling operations were done, the accuracy of the model was checked. To fulfill this task, several key lengths in the model were compared with the information given by the cardiologist/ radiologist (6).
The open-source software Cura was used to generate the support structure for the model and to define all the parameters needed for the FDM printing. These parameters were as follows: type of material, Filaflex (Recreus, Elda, Spain); temperature of the nozzle, 230°C; size of the nozzle, 0.4 mm; speed of the printing head, 20 mm/s; layer thickness, 0.15 mm; wall thickness, 0.8 mm; and infill pattern, none.
Before printing, the process was checked in a virtual simulation tool that showed all the layers and paths of the nozzle (Figure 3).
Figure 3. Printing parameters in Cura. Heart model visualization in Cura and virtual simulation of the printing process. Red: external layer; yellow: medium layer; and green: internal layer.
The BQ Witbox Fused Deposition Modeling (FDM) 3D printer was used to print all the heart models. This is a low-cost and open-source firmware and hardware printer capable to work with flexible filaments.
G-Code files were generated in Cura (version 3.6.0). They were transferred to the printer to start the printing process. The maximum printing volume of the machine is 297 × 210 × 200 mm, enough to print an adult heart (Figure 4). The maximum resolution in the z-axis is 0.02 mm. The heart models were printed with 0.15-mm Z resolution.
Figure 4. 3D printing process and manual finishing operations. Heart model in BQ Witbox 2 printing plate (up left). Left heart model with supports (up right). Tools used for removing supports and repairing the model (scissors, tweezers, scalpel, gas blowtorch, and bone forceps).
Flexible filaments are highly adhesive on the printing plate, and there was no need for extra adhesion operations or devices such as lack spray, blue tape, or heated bed (Figure 4).
Models and supports were printed with the same material. Once printed, the support structures adhered to the piece were removed using tools such as scissors, tweezers, scalpel, gas blowtorch, and bone forceps (Figure 4).
The material used for printing was a thermoplastic polyether-polyurethane, low-temperature flexibility (Filaflex; Recreus Industries S.L., Elda, Spain). The most important features of Filaflex are as follows: printing temperature: 210–240°C; hardness, 82 Shore A; density, 1.12 g/cm3; tensile strength, 45 MPa; elongation at break: 650 %; and odorless and not soluble in water.
For each model, segmentation time (doctor), computer-aided design time (design expert), printing time, removing supports time (technician), overall amount of material needed, printing success, and cost were recorded. Printing success was defined as hearts correctly printed at first attempt.
Quantitative variables are presented as mean ± standard deviation.
The cost of the models is divided in different concepts: segmentation, computer-aided design, cleaning, printer amortization, and material.
The cost of the personnel has to be adapted to each country and legislation. In this study, the cost was estimated to be €35.59/h for doctors, €26.63/h for design experts, and €13.44/h for technicians.
The amortization of the printer has been calculated with an estimated duration of 4 years. Costs associated with the printer were as follows: 3D printer (€1,449.90, VAT included), 4 years maintenance plan (€200/years, VAT included). We have estimated 2,000 h a year of operating time. Filament cost was €52.03/kg.
The total number of heart models printed was 138: surgical planning 83 cases, interventional planning 48 cases, and interventional simulator seven cases. Images of the heart models numbers 1 to 42 are presented as Supplementary Figure 1a, heart models from 43 to 91 as Supplementary Figure 1b, and heart models from 92 to 138 as Supplementary Figure 1c.
The segmentation time was 63.82 ± 17.15 min, and the CAD time was 73.43 ± 18.9 min. The printing time was 13.35 ± 7.85 h, and it was directly related to the patient's heart size, which ranges from 2.08 to 24 h for children hearts and from 24 to 42.65 h for adult-size hearts. The cleaning time was 37.24 ± 8.42 min (Table 2).
All the data collected in this study are presented as Supplementary Table 1.
It was necessary to launch the printing process 251 times to have 138 successful 3D printed hearts.
The most important reasons for failure were clogging of the nozzle, support failure, and printing failure of overhanging structures.
The most important costs associated with the design and fabrication of the heart models were the segmentation process and CAD (Table 3). The rest of the factors associated with manufacturing barely had an impact on the final cost because throughout the process, low-cost materials and technologies were used.
A total of 83 out of 138 3D printed models were designed and manufactured for surgical planning. The congenital heart diseases treated were as follows: double outlet right ventricle (Figure 5), complex transposition of the great arteries, univentricular heart physiology, large ventricular septal defects, crisscross heart, left ventricle outflow tract obstruction, and heterotaxy syndrome, among others (2, 6, 29). As we demonstrated in a previous study (6), the surgical planning of these patients was modified in 47.5% of the cases compared to traditional surgical planning using only clinical information and medical imaging.
Figure 5. Examples of heart models for surgical and interventional planning. Double outlet right ventricle (Case 135), internal vision from the left ventricle (upper left). Double outlet right ventricle (Case 133), external view (upper right). Testing of the personalized catheterization simulator in real conditions. Two fluid inlets (superior and inferior cava vein) and two fluid outlets (aortic arch and descending aorta) (down left). Percutaneous pulmonary valve implantation in a patient with severe right ventricle dilatation. Interventional planning with 3D printed model and real intervention in the patient (down right).
Forty-eight heart models were used to plan catheterization procedures. There are several pathologies that can be planned or simulated using this technology. We have designed and manufactured models for aortic pathology, atrial septal defect closure, stent angioplasty of pulmonary venous baffle obstruction, percutaneous mitral annuloplasty, pulmonary valve implantation (Figure 6), and transcatheter aortic valve implantation, among others (2, 3, 30).
Figure 6. Catheterization applications. Testing of the personalized catheterization simulator in real conditions. Two fluid inlets (superior and inferior cava vein) and two fluid outlets (aortic arch and descending aorta) (left). Percutaneous pulmonary valve implantation in a patient with severe right ventricle dilatation. Interventional planning with 3D printed model (up right) and real intervention in the patient (down right).
The mean segmentation cost was €37.86, the mean CAD cost was €32.59, the mean cleaning model cost was €8.34 €, the printer cost associated with each model was €3.67, and the mean 3D printing material cost was €3.2€. The total mean cost of the 3D printed hearts was €85.66 (Table 3). The amount of material needed for the hearts was 61.55 ± 36.03 g.
Models were used to teach registrars and young clinicians and surgeons. Our team is currently working on a catheterization simulator where we expect to be able to perform different interventional procedures (Figure 6). The simulator is based on a pulsatile fluid pump and a network of plastic pipes emulating vascular structures (31).
The models were used in most cases for informed consent. Thanks to the use of the models, it was possible to improve the understanding of the pathology, as well as the surgical intervention to be performed and the possible risks involved.
A total of 13 cardiac surgeons and 30 pediatric cardiologists (from different hospitals and countries) were asked to give feedback on the models. The satisfaction rate on the use of the 3D models was quantified as 9.3 (surgeons) and 9.0 (pediatric cardiologists) out of 10, respectively; 67% of them agreed or strongly agreed that the model was useful to communicate with parents and patients (6).
In this article, we present the most extensive series of 3D printed cardiac models published to date. We provide detailed information on how to implement a low-cost 3D printing laboratory for heart models.
In our study, the most important costs were those associated with the segmentation and computer design of the heart models (Table 3). The rest of the factors had little impact on the final cost because low-cost materials and technologies were used throughout the process.
The use of commercial software packages such as Mimics would have had an approximate economic impact of €10,000 per year.
Printing the heart models on a Stratasys Polyjet machine would have involved an equipment acquisition cost of over €100,000 and an average cost per heart of €196.19 in printing materials vs. € 3.2 with flexible filament in an FDM 3D printer (Supplementary Table 2).
All the hearts that we have fabricated in the last 7 years have been used in clinical patients, and we have demonstrated a positive impact on congenital heart disease surgical decision process. Planning complex surgeries with a 3D printed heart can help medical teams to decide on the surgical approach. In our previous study, in 40% of the cases, the initial surgical plan was changed after reviewing the model (6).
We have studied and published the dimensional accuracy of the models, and we have found an excellent agreement between the dimensions of the 3D models and the medical images—CMR: mean difference −0.30 ± 0.67 mm, t-test P = 0.66; and CT images: mean difference −0.16 ± 0.85 mm, t-test P = 0.85 (6). The accuracy of the models allowed us to plan in detail specific interventions, and they have been essential in the development of new strategies such us percutaneous pulmonary valve implantation in large and dilated right ventricular outflow tracts (2).
Several authors have demonstrated the utility of 3D printing models to improve medical training (32). It is the scope of our future research to test how different 3D printed pathologic hearts can be used in the simulator in order to train clinicians to reproduce a wide range of catheterization procedures.
We believe that 3D printed models of the heart may become an essential tool for cardiologists and surgeons in interventional and surgical planning of complex cases. We hope that this publication facilitates the adoption of the technology in order to improve the quality of care of these patients.
New materials and 3D printing technologies are emerging every day. Automatic segmentation of the anatomy, 3D printing time reduction, human tissue-like materials, and biocompatible materials are the most important goals in which researchers are focusing nowadays. We are still far from being able to fabricate a complete and functional heart, but we are close to being able to produce patches and parts of the heart through tissue engineering.
We have demonstrated that it is possible to print high-quality and affordable 3D printed cardiac models. These models have been useful to improve the quality of care of the patients who participated in the study.
The original contributions generated for the study are included in the article/Supplementary Material, further inquiries can be directed to the corresponding author/s.
The studies involving human participants were reviewed and approved by Comité de Ética de Investigación Costa del Sol Luís Baro Rodríguez Alejandro Pérez Cabeza Francisco Rivas Ruiz Others. Written informed consent to participate in this study was provided by the participants' legal guardian/next of kin.
GG-C: funding acquisition, investigation, writing—original draft, and writing—review and editing. TG-C: funding acquisition, supervision, and writing—review and editing. JR-G and MV: research strategy and writing—review and editing. IV: funding acquisition, supervision, investigation, and writing—review and editing. All authors contributed to the article and approved the submitted version.
This work has been partially funded by Instituto de Salud Carlos III, Spanish Ministry of Health, PI14/00180 3D printed cardiovascular models for congenital heart disease surgical planning, and by Andalusian Ministry of Health PIN-213-2018 3D printing laboratory for the Andalusian Health Service.
The authors declare that the research was conducted in the absence of any commercial or financial relationships that could be construed as a potential conflict of interest.
The Supplementary Material for this article can be found online at: https://www.frontiersin.org/articles/10.3389/fcvm.2021.642011/full#supplementary-material
1. Smith E, Prabhu SP, Flynn K, Weinstock P, Orbach DB. Optimizing cerebrovascular surgical and endovascular procedures in children via personalized 3D printing. J Neurosurg Pediatr. (2015) 16:584–9. doi: 10.3171/2015.3.PEDS14677
2. Valverde I, Sarnago F, Prieto R, Zunzunegui JL. Three-dimensional printing in vitro simulation of percutaneous pulmonary valve implantation in large right ventricular outflow tract. Europ Heart J. (2017) 38:1262–3. doi: 10.1093/eurheartj/ehw546
3. Gómez-Ciriza G, Hussain T, Gómez-Cía T, Valverde I. Potential of 3D-printed models in planning structural interventional procedures. Interv Cardiol. (2015) 7:343–50. doi: 10.2217/ica.15.25
4. Farooqi KM, Nielsen JC, Uppu SC, Srivastava S, Parness IA, Sanz J, et al. Use of 3-dimensional printing to demonstrate complex intracardiac relationships in double-outlet right ventricle for surgical planning. Circul Cardiovas Imaging. (2015) 38:43. doi: 10.1161/CIRCIMAGING.114.003043
5. Schmauss D, Haeberle S, Hagl C, Sodian R. Three-dimensional printing in cardiac surgery and interventional cardiology: a single-centre experience. Eur J Cardio-thoracic Surg. (2014) 47:1044–52. doi: 10.1093/ejcts/ezu310
6. Valverde I, Gomez-Ciriza G, Hussain T, Suarez-Mejias C, Velasco-Forte MN, Byrne N, et al. Three-dimensional printed models for surgical planning of complex congenital heart defects: an international multicentre study. Eur J Cardio-Thoracic Surg. (2017) 52:1139–48. doi: 10.1093/ejcts/ezx208
7. Kurenov SN, Ionita C, Sammons D, Demmy TL. Three-dimensional printing to facilitate anatomic study, device development, simulation, and planning in thoracic surgery. J Thorac Cardiovasc Surg. (2015) 149:973–9.e1. doi: 10.1016/j.jtcvs.2014.12.059
8. Giannopoulos AA, Mitsouras D, Yoo SJ, Liu PP, Chatzizisis YS, Rybicki FJ. Applications of 3D printing in cardiovascular diseases [Internet]. Nat Rev Cardiol. (2016) 13:701–18. doi: 10.1038/nrcardio.2016.170
9. Smith CF, Tollemache N, Covill D, Johnston M. Take away body parts! An investigation into the use of 3D-printed anatomical models in undergraduate anatomy education. Anat Sci Educ. (2018) 11:44–53. doi: 10.1002/ase.1718
10. Little SH, Vukicevic M, Avenatti E, Ramchandani M, Barker CM. 3D printed modeling for patient-specific mitral valve intervention. JACC Cardiovasc Interv. (2016) 9:973–5. doi: 10.1016/j.jcin.2016.02.027
11. Findlay MW, McMenamin PG, Hunter-Smith DJ, Chae MP, Rozen WM, Spychal RT. Emerging applications of bedside 3d printing in plastic surgery. Front Surg. (2015) 2:25. doi: 10.3389/fsurg.2015.00025
12. Cohen A, Laviv A, Berman P, Nashef R, Abu-Tair J. Mandibular reconstruction using stereolithographic 3-dimensional printing modeling technology. Oral Surg Oral Med Oral Pathol Oral Radiol Endodontology. (2009) 108:661–6. doi: 10.1016/j.tripleo.2009.05.023
13. Banse X, Cartiaux O, Docquier P-L, Francq BG, Paul L. Improved accuracy with 3d planning and patient-specific instruments during simulated pelvic bone tumor surgery. Ann Biomed Eng. (2013) 42:205–13. doi: 10.1007/s10439-013-0890-7
14. Ventola CL. Medical applications for 3D printing: current and projected uses. P T. (2014) 39:704–11.
15. Lau I, Sun Z. Three-dimensional printing in congenital heart disease: a systematic review [Internet]. J Med Radiat Sci. (2018) 65:226–36. doi: 10.1002/jmrs.268
16. Chen MY, Skewes J, Woodruff MA, Dasgupta P, Rukin NJ. Multi-colour extrusion fused deposition modelling: a low-cost 3D printing method for anatomical prostate cancer models. Sci Rep. (2020) 10:1–5. doi: 10.1038/s41598-020-67082-7
17. Bücking TM, Hill ER, Robertson JL, Maneas E, Plumb AA, Nikitichev DI. From medical imaging data to 3D printed anatomical models. PLoS ONE. (2017) 12:e0178540. doi: 10.1371/journal.pone.0178540
18. Lau I, Wong YH, Yeong CH, Abdul Aziz YF, Md Sari NA, Hashim SA, et al. Quantitative and qualitative comparison of low- and high-cost 3D-printed heart models. Quant Imaging Med Surg. (2019) 9:107–14. doi: 10.21037/qims.2019.01.02
19. Vukicevic M, Mosadegh B, Min JK, Little SH. Cardiac 3D printing and its future directions. JACC Cardiovas Imaging. (2017) 10:171–84. doi: 10.1016/j.jcmg.2016.12.001
20. Bartel T, Rivard A, Jimenez A, Mestres CA, Müller S. Medical three-dimensional printing opens up new opportunities in cardiology and cardiac surgery. Europ Heart J. (2018) 38:1246–54. doi: 10.1093/eurheartj/ehx016
21. Valverde I, Gomez G, Coserria JF, Suarez-Mejias C, Uribe S, Sotelo J, et al. 3D printed models for planning endovascular stenting in transverse aortic arch hypoplasia. Catheter Cardiovasc Interv. (2015) 85:1006–12. doi: 10.1002/ccd.25810
22. Hussain T, Velasco Forte M, Valverde I, Tandon A, Byrne N. A systematic review of image segmentation methodology, used in the additive manufacture of patient-specific 3D printed models of the cardiovascular system. JRSM Cardiovasc Dis. (2016) 5:204800401664546. doi: 10.1177/2048004016645467
23. Weissheimer A, Menezes LM De, Sameshima GT, Enciso R, Pham J, Grauer D. Imaging software accuracy for 3-dimensional analysis of the upper airway. Am J Orthod Dentofac Orthop. (2012) 142:801–13. doi: 10.1016/j.ajodo.2012.07.015
24. Mitsouras D, Liacouras P, Imanzadeh A, Giannopoulos AA, Cai T, Kumamaru KK, et al. Medical 3D printing for the radiologist. RadioGraphics. (2015) 35:1965–88. doi: 10.1148/rg.2015140320
25. Dimitrov D, Schreve K, De Beer N. Rapid Prototyping Journal Advances in three dimensional printing – state of the art and future perspectives. Rapid Prototyp J Assem Autom Iss Rapid Prototyp J. (2013) 12:136–47. doi: 10.1108/13552540610637264
26. Hussain T, Lossnitzer D, Bellsham-Revell H, Valverde I, Beerbaum P, Razavi R, et al. Three-dimensional dual-phase whole-heart mr imaging: clinical implications for congenital heart disease. Radiology. (2012) 263:547–54. doi: 10.1148/radiol.12111700
27. Gosnell J, Pietila T, Samuel BP, Kurup HKN, Haw MP, Vettukattil JJ. Integration of computed tomography and three-dimensional echocardiography for hybrid three-dimensional printing in congenital heart disease. J Digit Imaging. (2016) 29:665–9. doi: 10.1007/s10278-016-9879-8
28. Mahmood F, Owais K, Taylor C, Montealegre-Gallegos M, Manning W, Matyal R, et al. Three-dimensional printing of mitral valve using echocardiographic data [Internet]. JACC Cardiovasc Imaging. (2015) 8:227–9. doi: 10.1016/j.jcmg.2014.06.020
29. Valverde I, Gomez G, Gonzalez A, Suarez-Mejias C, Adsuar A, Coserria JF, et al. Three-dimensional patient-specific cardiac model for surgical planning in Nikaidoh procedure. Cardiol Young. (2015) 25:698–704. doi: 10.1017/S1047951114000742
30. Vaquerizo B, Escabias C, Dubois D, Gómez G, Barreiro-Pérez M, Cruz-González I. Patient-specific 3D-printed cardiac model for percutaneous left atrial appendage occlusion. Revista Espanola de Cardiologia. (2018) 71:762–4. doi: 10.1016/j.rec.2017.05.028
31. Valverde I, Gómez T, Gómez G y, Suarez C. Patent ES2608203B1. Customizable Simulation Device for Interventions. (2015) Available online at: https://patents.google.com/patent/ES2608203B1/en?q=gorka&q=gomez&oq=gorka+gomez (accessed February 4, 2019).
Keywords: affordable 3d printing, surgical planning, interventional planning, heart models, medical education
Citation: Gómez-Ciriza G, Gómez-Cía T, Rivas-González JA, Velasco Forte MN and Valverde I (2021) Affordable Three-Dimensional Printed Heart Models. Front. Cardiovasc. Med. 8:642011. doi: 10.3389/fcvm.2021.642011
Received: 15 December 2020; Accepted: 29 April 2021;
Published: 04 June 2021.
Edited by:
Ruth Heying, University Hospital Leuven, BelgiumReviewed by:
Zhonghua Sun, Curtin University, AustraliaCopyright © 2021 Gómez-Ciriza, Gómez-Cía, Rivas-González, Velasco Forte and Valverde. This is an open-access article distributed under the terms of the Creative Commons Attribution License (CC BY). The use, distribution or reproduction in other forums is permitted, provided the original author(s) and the copyright owner(s) are credited and that the original publication in this journal is cited, in accordance with accepted academic practice. No use, distribution or reproduction is permitted which does not comply with these terms.
*Correspondence: Gorka Gómez-Ciriza, Z29ya2EuZ29tZXpAanVudGFkZWFuZGFsdWNpYS5lcw==
Disclaimer: All claims expressed in this article are solely those of the authors and do not necessarily represent those of their affiliated organizations, or those of the publisher, the editors and the reviewers. Any product that may be evaluated in this article or claim that may be made by its manufacturer is not guaranteed or endorsed by the publisher.
Research integrity at Frontiers
Learn more about the work of our research integrity team to safeguard the quality of each article we publish.