- 1Urogenital Stem Cell Research Center, Shahid Beheshti University of Medical Sciences, Tehran, Iran
- 2Department of Medical Genetics, Shahid Beheshti University of Medical Sciences, Tehran, Iran
- 3Urology and Nephrology Research Center, Shahid Beheshti University of Medical Sciences, Tehran, Iran
Coronary artery disease (CAD) is a common disorder caused by atherosclerotic processes in the coronary arteries. This condition results from abnormal interactions between numerous cell types in the artery walls. The main participating factors in this process are accumulation of lipid deposits, endothelial cell dysfunction, macrophage induction, and changes in smooth muscle cells. Several lines of evidence underscore participation of long non-coding RNAs (lncRNAs) and circular RNAs (circRNAs) in the pathogenesis of CAD. Several lncRNAs such as H19, ANRIL, MIAT, lnc-DC, IFNG-AS1, and LEF1-AS1 have been shown to be up-regulated in the biological materials obtained from CAD patients. On the other hand, Gas5, Chast, HULC, DICER1-AS1, and MEG3 have been down-regulated in CAD patients. Meanwhile, a number of circRNAs have been demonstrated to influence function of endothelial cells or vascular smooth muscle cells, thus contributing to the pathogenesis of CAD. In the current review, we summarize the function of lncRNAs and circRNAs in the development and progression of CAD.
Introduction
Coronary artery disease (CAD) is a common disorder caused by atherosclerotic processes in the coronary arteries. This condition can be asymptomatic or can result in fatal situations. In fact, CAD is the main cause of the mortality associated with coronary heart disorders (1). Atherosclerosis is regarded as a progressive inflammatory condition during which oxidative, hemodynamic, and biochemical factors destruct the function of endothelial cells (2). Subsequent alterations in the permeability of endothelial cells, accumulation of macrophages, production of inflammatory substances, and activation of smooth muscle cells are additional steps in the development of atherosclerosis (3, 4). Two classes of regulatory non-coding RNAs, namely, long non-coding RNAs (lncRNAs) and circular RNAs (circRNAs), have been shown to affect the process of atherosclerosis and CAD development (5, 6). Although both having regulatory effects on the expression of genes, they vary in terms of biogenesis and mechanism of action. LncRNAs have sizes of more than 200 nucleotides (7) and can function as signal, sequester, scaffold, guide, or enhancer RNAs to influence genomic organization or gene expression (8). They share several features with mRNAs such as the presence of RNA polymerase II binding sites, 3′ poly A tails and 5′ caps (9). On the other hand, circRNAs are single-stranded covalently enclosed molecules made via back-splicing of linear precursor transcripts (10). Both classes of transcript can influence function of endothelial cells or smooth muscle cells in the process of atherosclerosis. In the current review, we summarize the function of lncRNAs and circRNAs in the development and progression of CAD.
LncRNAS and CAD
Role of LncRNAs in CAD
LncRNAs can affect CAD pathogenesis through regulation of immune responses, modulation of function of endothelial cells and vascular smooth muscles, and changing lipid metabolism. In some cases, a certain lncRNA can affect more than one route.
LncRNAs Regulate Immune Responses
H19 is a transcript encoded by a conserved imprinted gene cluster containing the insulin-like growth factor 2 gene (11). H19 has been shown to function as a molecular sponge for let-7. Expression of this lncRNA has been shown to be reduced in the muscle of patients with type-2 diabetes, as well as animal model of this disorder. The consequent up-regulation of let-7 decreases expression levels let-7 targets (12). This lncRNA has been overexpressed in patients with CAD despite its normal expression in other forms of cardiovascular disorders and therefore has been suggested as a marker for prediction of CAD. Notably, its expression levels have been associated with duration of CAD and serum concentrations of transforming growth factor β1 (TGF-β1). In vitro studies verified the effects of H19 overexpression in enhancement of TGF-β1 secretion (13). The lncRNA CoroMarker has been shown to be functionally clustered with genes, which are related with signal transduction, transmembrane transport, synaptic communication, and innate immune responses, while having negative correlation with inflammation-related genes. Small interfering RNA–mediated silencing of CoroMarker has reduced the production of proinflammatory cytokines (14). IFNG-AS1 is another overexpressed lncRNA in CAD patients whose expression has been considerably associated with Gensini score, as well as levels of inflammatory markers high sensitivity C-reactive protein (hs-CRP), tumor necrosis factor α (TNF-α), and interleukin 6 (IL-6). On the other hand, IFNG-AS1 levels have been inversely associated with the levels of anti-inflammatory cytokine IL-10 level (15). Cho et al. (16) have demonstrated DQ485454 as the main ANRIL transcript in the endothelial cells. Expression of this transcript has been significantly higher in endothelial cells compared with THP-1 monocytes. Notably, they reported down-regulation of DQ485454 in CAD coronary arteries as compared with samples obtained from non-CAD arteries. Forced up-regulation of this transcript has attenuated cellular processes participating in CAD initiation as it decreased monocyte adhesion to endothelial cells, transendothelial monocyte migration, and endothelial cell migration. Moreover, expression of several CAD-related genes were altered after DQ485454 silencing (16). A microarray-based study has demonstrated down-regulation of NEXN-AS1 in human atherosclerotic plaques. This lncRNA interacts with the chromatin modifier BAZ1A and the 5′ part of the NEXN gene. Overexpression of NEXN-AS1 suppressed TLR4 oligomerization and nuclear factor κB (NF-κB) function, decreased endothelial production of adhesion proteins and inflammatory cytokines, and repressed adhesion of monocyte to endothelial cells (17). Another study in CAD patients demonstrated down-regulation of Chast, HULC, and DICER1-AS1 in the peripheral blood samples (18). Expression of CASC11 has also been decreased in patients with CAD parallel with overexpression of TGF-β1. Functional studies revealed the impact of this lncRNA in the suppression of TGF-β1 expression in endothelial cells (19).
LncRNAs Alter Function of Endothelial Cells and Vascular Smooth Muscle Cells
ANRIL is another up-regulated lncRNA in CAD patients as well as animal model of this disorder. Overexpression of ANRIL decreases expression of miR-181b and is associated with risk of CAD in the subpopulations of elderly patients with history of smoking, hypertension, and hyperlipidemia. Overexpression of ANRIL in human coronary endothelial cells has down-regulated miR-181b, increased p50/p65 expressions, enhanced viability of human coronary endothelial cells, and promoted release of inflammatory molecules and vascular-protecting proteins (20). Another study has revealed overexpression of ANRIL in patients with acute coronary syndrome in association with levels of monocyte chemoattractant protein-1 and IL-10. Notably, these proinflammatory cytokines are produced in reaction to dysfunction of endothelial cells. ANRIL silencing has enhanced cell proliferation and tubule development and suppressed induction of inflammatory responses and apoptosis of endothelial cells. Such effects were linked to ANRIL-mediated suppression of let-7b and its impacts on the TGF-βR1/Smad signaling (21). Another study has demonstrated higher levels of ANRIL and MIAT in the atherosclerotic arteries when compared to the non-atherosclerotic ones (22). ANRIL can also regulate growth of vascular smooth muscle cells via modulation of CDKN2A/B locus, which has a direct effect on the pathobiology of atherosclerosis (23). H19 in addition to its role in the regulation of immune responses can influence vascular smooth muscle cells. Expression of this lncRNA has been increased in the injured neointima and in human atherosclerotic plaques but is scarcely deceted in normal vessels. H19 sequesters let-7 family microRNAs (miRNAs), which are known to shield vascular smooth muscle cells from oxidative damage (12, 24). Expression of lnc-DC has been higher in patients with type 2 diabetes and CAD compared with diabetic patients without CAD. Such up-regulation has been accompanied by overexpression of STAT3. Yet, expression of these genes was not associated with the severity of CAD. Based on the observed correlations between expression of genes, authors have suggested the importance of JAK/STAT-related-lncRNAs in the pathogenesis of CAD (25). Expression of FAL1 has been shown to be elevated in CAD tissues and TNF-α-stimulated endothelial cells compared with normal and unstimulated cells. Up-regulation of FAL1 in endothelial cells has enhanced cell cycle progression, proliferation, and migration via modulation of PTEN/AKT pathway (26). In addition, HIF1a-AS1 partakes in the pathology of atherosclerosis via modulating apoptosis of vascular smooth muscle cells and endothelial cells (27). Expression of CASC11 has also been decreased in patients with CAD parallel with overexpression of TGF-β1. Functional studies revealed the impact of this lncRNA in the suppression of TGF-β1 expression in endothelial cells (19). Expression of LEF1-AS1 has been elevated in plasma and tissue samples of CAD patients, whereas expression of its target miRNA, i.e., miR-544a, has been decreased. LEF1-AS1 modulates proliferation and migration of smooth muscle cells via the miR-544a/PTEN route (28). Figure 1 shows the molecular cascade of contribution of LEF1-AS1 and MEG3 in CAD.
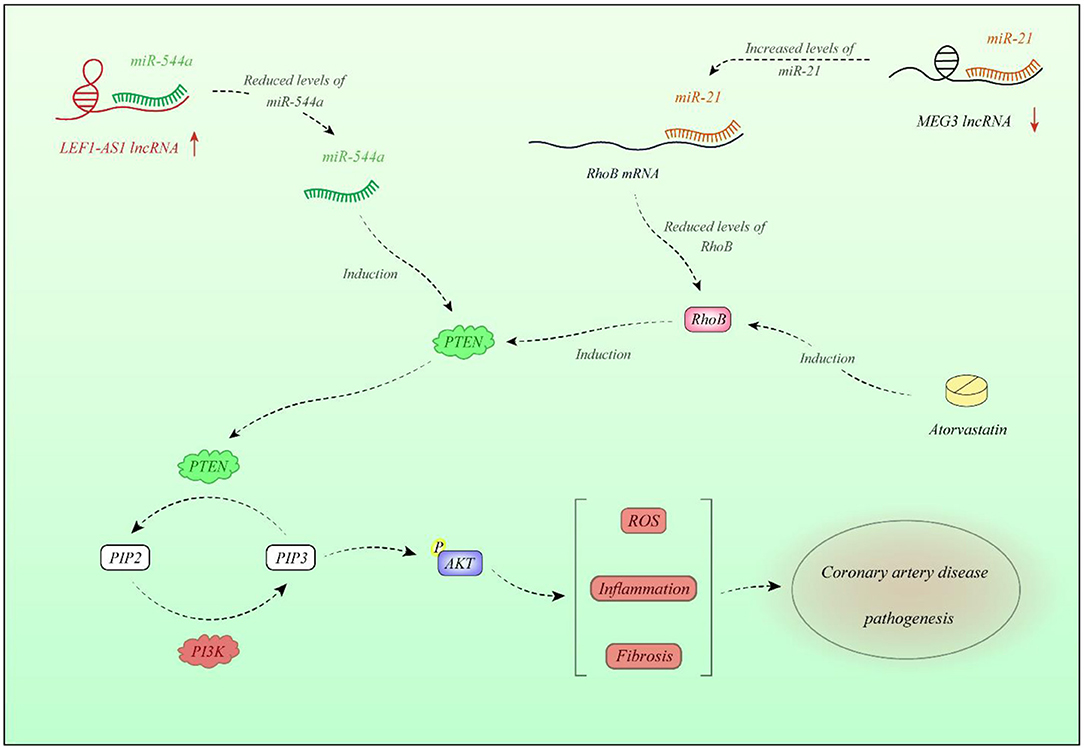
Figure 1. Expression of LEF1-AS1 is increased in plasma and tissues of CAD patients. LEF1-AS1 inhibits miR-544a, thus decreasing PTEN. This is because miR-544a increases PTEN levels. PTEN diminishes AKT activity. LEF1-AS1 contributes in enhancement of reactive oxygen species (ROS) formation, elevation of inflammatory responses, and fibrosis through miR-544a/PTEN axis (28). On the other hand, expression of MEG3 is decreased in CAD tissues compared with normal tissues. Down-regulation of MEG3 is associated with up-regulation of miR-21. miR-21 binds with 3′-UTR of RhoB and decreases its expression. RhoB has a role in activation of PTEN; therefore, MEG3 down-regulation is associated with the lower activity of PTEN (29). Atorvastatin has a regulatory role on PTEN through modulation of RhoB (30).
LncRNAs Regulate Lipid Metabolism
LincRNA-DYNLRB2-2 is an lncRNA whose expression is stimulated by Ox-LDL. This transcript enhances ABCA1-associated cholesterol efflux and suppresses inflammatory responses via GPR119 in macrophage originated foam cells (31). CHROME is another up-regulated lncRNA in CAD patients whose expression is altered by nutritional and cellular cholesterol levels via the sterol-activated liver X receptor transcription factors. This lncRNA enhances cholesterol secretion and HDL synthesis through suppression of the activity of a number of miRNAs. CHROME silencing in human hepatocytes and macrophages enhances expressions of miR-27b, miR-33a, miR-33b, and miR-128, thus decreasing the levels of their shared target genes, particularly ABCA1, which controls de novo synthesis of HDL (Figure 2) (32). Expression of FAL1 has been shown to be elevated in CAD tissues and TNF-α-stimulated endothelial cells compared with normal and unstimulated cells. Up-regulation of FAL1 in endothelial cells has enhanced cell cycle progression, proliferation, and migration via modulation of PTEN/AKT pathway (26). GAS5 is another down-regulated lncRNA in CAD. Enforced up-regulation of GAS5 in animal models of CAD has improved hyperlipidemia, reduced myocardial damage, suppressed apoptosis of cardiac cells, and diminished oxidative stress, inflammatory damage, and aberrant induction of the Wnt/β-catenin pathway in cardiac tissue (33). The function of up-regulated and down-regulated lncRNAs in CAD is summarized in Tables 1, 2, respectively.
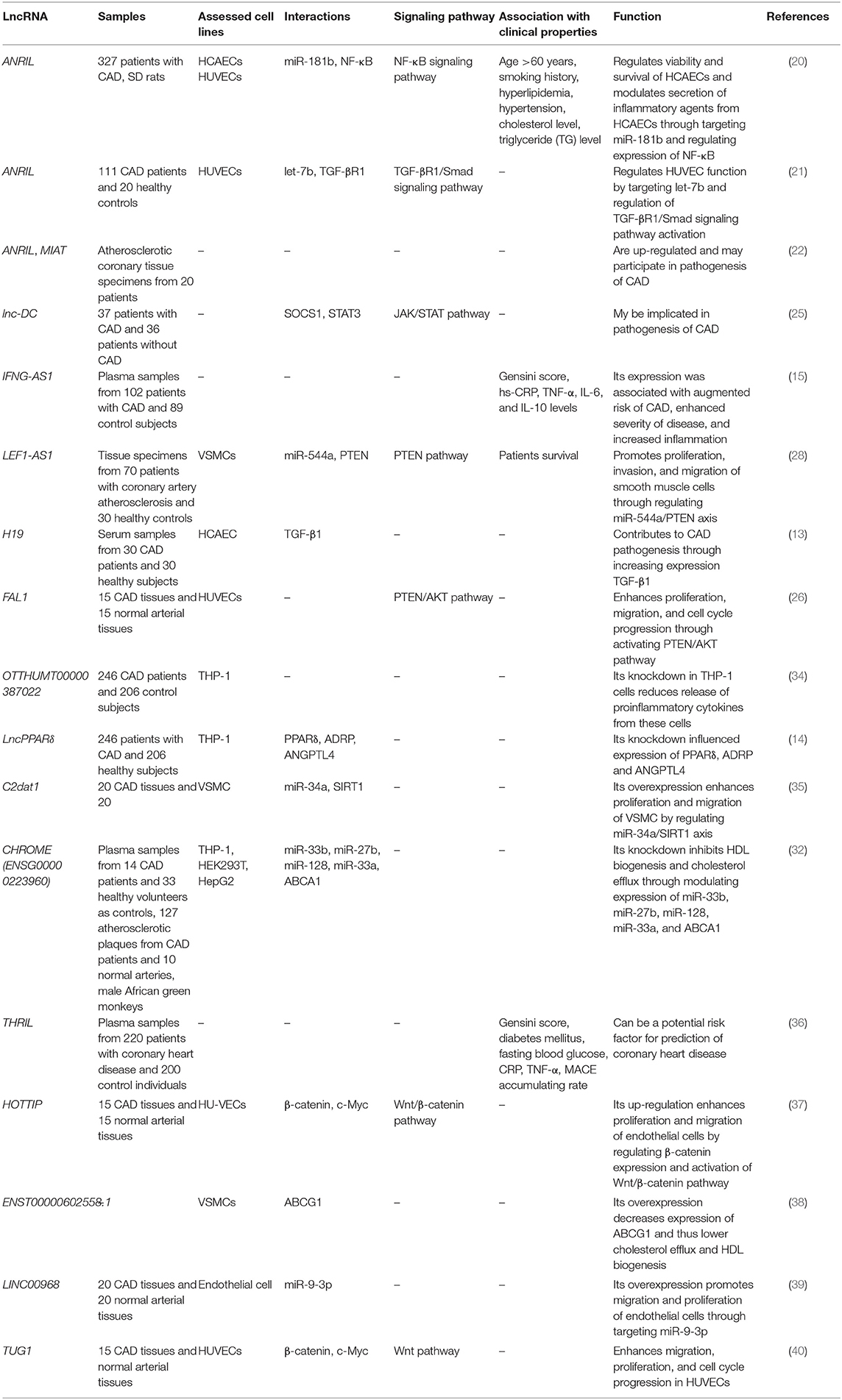
Table 1. List of up-regulated lncRNAs in CAD (HCAECs, human coronary endothelial cells; HUVECs, human umbilical vein endothelial cells; VSMCs, vascular smooth muscle cells).
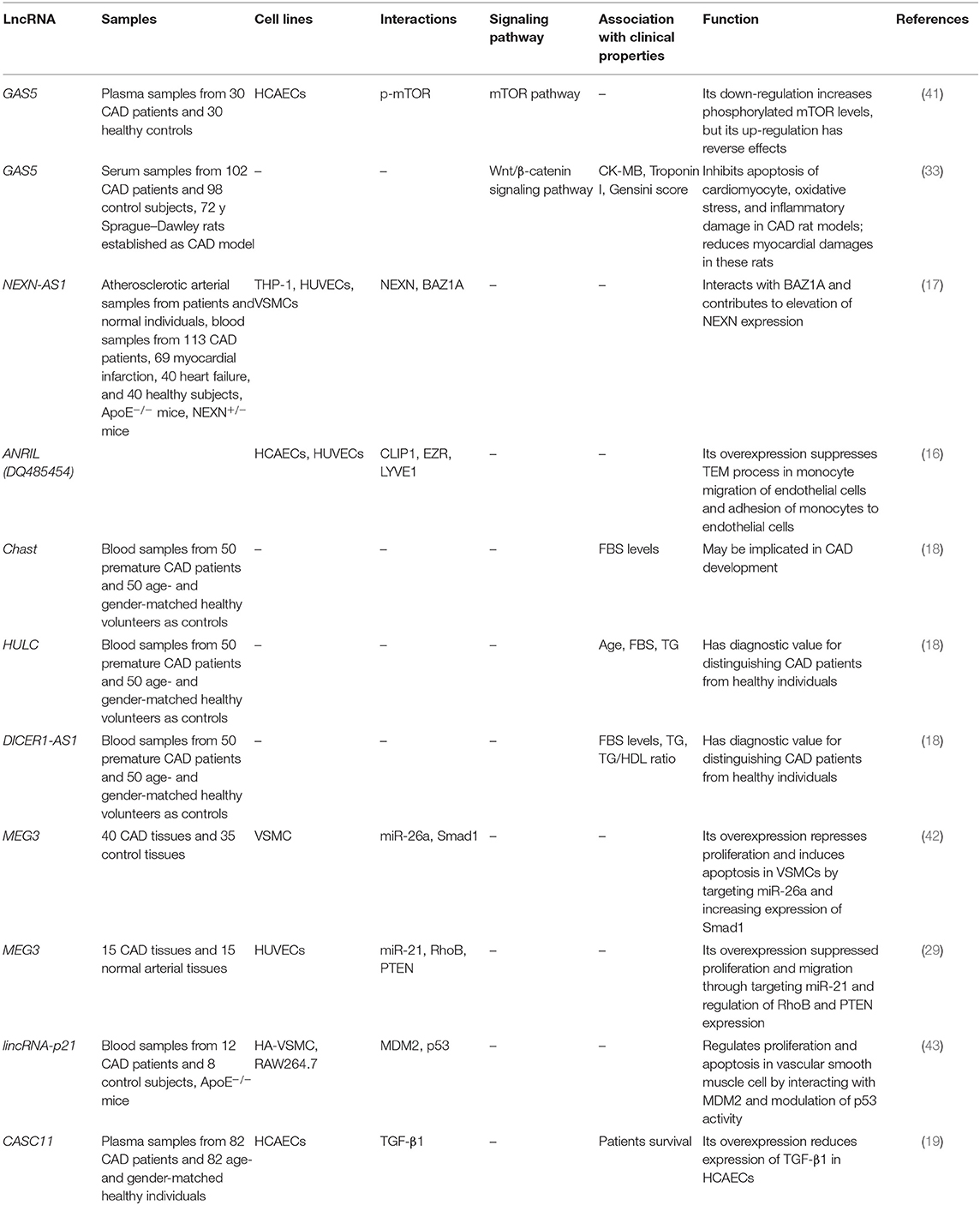
Table 2. List of down-regulated lncRNAs in CAD (HCAEC, human coronary endothelial cells; HUVEC, human umbilical vein endothelial cells).
Prognostic Value of LncRNAs in CAD
LncRNAs can be used for evaluation of prognosis of CAD patients. For instance, a long-term follow-up study has demonstrated correlation between down-regulation of CASC11 and poor survival of patients with CAD (19). On the other hand, overexpression of LEF1-AS1 and ANRIL has been shown to be correlated with poor clinical outcome of patients with CAD (28, 44). Kaplan–Meier analysis has also demonstrated association between ANRIL and LEF1-AS1 overexpression and short overall survival in CAD patients (28, 44). Table 3 reviews the studies that appraised the prognostic role of lncRNAs in CAD.
Diagnostic Value of LncRNAs in CAD
Blood or serum levels of some lncRNAs can be used as diagnostic markers in CAD. The best diagnostic value has been reported for H19 where the receiver operating characteristic (ROC) curves showed the diagnostic power of 0.9367, signifying H19 as a suitable marker for CAD (13). Cai et al. (14) have profiled lncRNAs in circulating peripheral blood monocytes and plasma samples of CAD patients and healthy subjects. Their preliminary results demonstrated possible biomarker role for CoroMarker, BAT5, and IL21R-AS1 lncRNAs. The verification step in the larger cohort of CAD patients supported the biomarker role of CoroMarker. This lncRNA could differentiate CAD patients from healthy subjects with accuracy of 0.920 and in an independent manner from identified CAD risk factors and other cardiovascular disorders (14). Another study demonstrated the accuracy of 0.90 and 0.87 for HULC and DICER1-AS1, respectively, in differentiation between CAD patients and healthy individuals (18). In addition, up-regulation of IFNG-AS1 in CAD patients could be used to forecast the risk of CAD with accuracy of 0.755 (15). Table 4 summarizes the points regarding the diagnostic significance of lncRNAs in CAD.
LncRNAs Polymorphisms and CAD
A number of functional single-nucleotide polymorphisms (SNPs) in lncRNAs have been associated with susceptibility to CAD. ANRIL has been the mostly assessed lncRNA in this regard. For instance, rs1330049, rs2383206, rs10757278, and rs10757274 SNPs have been associated with risk of CAD in Asians (52). On the other hand, rs2383207 and rs1333049 SNPs of ANRIL have not been associated with CAD risk in Han Chinese (53). In addition, rs1333040 and rs1004638 SNPs of ANRIL have not been associated with this disorder in Iranian population (54). H19 is another lncRNA whose association with risk of CAD has been assessed in some populations. Hu et al. (55) have reported an association between H19 rs2735971 and rs3024270 SNPs and susceptibility to CAD in a Chinese population, suggesting the significance of these SNPs as markers for prediction of risk of CAD in this population. Other SNPs within LINC00841, MALAT1, and lincRNA-p21 have been associated with risk of CAD in some ethnic groups (Table 5).
CircRNAs and CAD
Role of CircRNAs in CAD
A comprehensive circRNA profiling in CAD patients has shown up-regulation of 624 circRNAs and down-regulation of 171 circRNAs in these patients compared with healthy subjects. Subsequent validation in a larger cohort of patients supported up-regulation of hsa_circ_0001879 and hsa_circ_0004104 in CAD patients. Remarkably, up-regulation of hsa_circ_0004104 has led to aberrant expression of atherosclerosis-associated genes in macrophages (63). Another high-throughput study of circRNAs expression in CAD patients has shown up-regulation of 18 circRNAs, whereas down-regulation has been shown in six circRNAs. Subsequently, authors have reported the role of nine circRNAs in the enhancement of TRPM3 expression through suppression of hsa-miR-130a-3p (64). Expression of circ-SATB2 has been shown to be increased in proliferative vascular smooth muscle cells in association with down-regulation of miR-939. Circ-SATB2 was able to augment expression of a target of miR-939, namely, STIM1. Up-regulation of circ-SATB2 decreases expression of SM22-α, a marker of contractile vascular smooth muscle cells. Functional studies verified the role of this circRNA in the regulation of differentiation, proliferation, apoptosis, and migration of vascular smooth muscle cells through enhancing STIM1 expression (65). Expression of circZNF609 has been shown to be reduced in peripheral blood leukocytes of CAD patients in correlation with levels of CRP and lymphocyte counts. Forced overexpression of circZNF609 has enhanced production of inflammatory cytokines IL-6 and TNF-α, while enhancing expression of IL-10. These effects are possibly mediated through sponging miRNAs (66). In an eminent study, Guo et al. (67) have assessed levels of hsa_circ_0002984, hsa_circ_0010283, and hsa_circ_0029589 in human peripheral blood mononuclear cell–originated macrophages from CAD patients and evaluated the consequences of overexpression or silencing of these circRNAs. Authors have reported down-regulation of hsa_circ_0029589 in macrophages, whereas up-regulation of the N6-methyladenosine levels of hsa_circ_0029589 in macrophages of patients with acute coronary syndrome has been shown. Notably, up-regulation of IRF-1 has diminished the expression of hsa_circ_0029589, but surged its m6A levels. Therefore, IRF-1 has been shown to enhance macrophage pyroptosis and inflammatory responses in acute coronary syndrome and atherosclerotic patients by obstructing circ_0029589 via increasing its m6A modifications (67). Tables 6, 7 show the list and function of up-regulated and down-regulated circRNAs in CAD, respectively.
Diagnostic Value of CircRNAs in CAD
ROC curve analysis has shown that hsa_circ_0001879 and hsa_circ_0004104 could differentiate CAD patients from healthy subjects with diagnostic values of 0.703 and 0.700, respectively. Notably, combination of expression levels of these circRNAs, in conjunction with CAD risk factors, could enhance the diagnostic power (63). Expression of circZNF609 has been shown to be reduced in peripheral blood leukocytes of CAD patients in correlation with levels of CRP and lymphocyte counts. In addition, down-regulation of circZNF609 has been associated with higher risks of CAD with accuracy of 0.761 (66). In a high-throughput circRNA profiling, Zhang et al. have demonstrated differential expression of 22 circRNAs between CAD patients and healthy subjects. Among these circRNAs, hsa_circ_0124644 has been shown to have the highest AUC value. Validation of their results in a larger cohort of CAD patients showed the diagnostic power of 0.769 for this circRNA (69). Combination of expression profile of certain circRNAs with conventional CAD risk factors has enhanced the diagnostic power (Table 8).
CircRNA Polymorphisms and CAD
Finally, SNPs within circRNAs can alter the risk of CAD. Zhou et al. (73) have appraised the impact of two SNPs at the circFOXO3 flanking introns in the development of CAD in a Chinese population. They reported the association between the rs12196996 G allele and elevated susceptibility to CAD. Moreover, such association was more remarkable among younger individuals and non-smokers. The haplotype rs12196996G-rs9398171C has also been associated with susceptibility to CAD. Functionally, the rs12196996 GG genotype conferred lower amounts of circFOXO3 expression (73).
Discussion
CAD is a pathogenic condition in which several cell types and molecules are involved. In fact, this condition results from abnormal interactions between numerous cell types in the artery walls. The main participating factors in this process are accumulation of lipid deposits, endothelial cell dysfunction, macrophage induction, and changes in smooth muscle cells (5). Non-coding RNAs can influence almost every aspect of this pathogenic process. Dysregulation of several lncRNAs and circRNAs has been noted in CAD. ANRIL has been among the most assessed lncRNAs in CAD. Whereas, most studies have demonstrated up-regulation of ANRIL in patients with CAD or animal models of CAD (20, 22), a single study has reported down-regulation of certain transcript of ANRIL in CAD coronary arteries as compared with samples obtained from non-CAD arteries (16). Therefore, transcript variants of lncRNAs might have different tissue specificity and diverse functional roles. Such detailed analysis of transcript variants has not been performed for other lncRNAs in the context of CAD.
Dysregulated lncRNAs in CAD patients have functional interactions with Wnt/β-catenin, NF-κB, TGF-βR1/Smad, JAK/STAT, PTEN/AKT, and mTOR signaling pathways. Therefore, these signaling pathways are putative targets for therapeutic manipulations in CAD. Comprehensive studies are needed to explore the interactions between all mentioned lncRNAs and these pathways to find the most appropriate lncRNA for therapeutic interventions. The lncRNAs with the most robust interactions with higher numbers of these pathways are probably the most suitable targets. Moreover, CAD-related lncRNAs have interactions with a number of miRNAs such as miR-181b, let-7b, miR-544a, miR-34a, miR-33b, miR-26a, miR-27b, miR-21, miR-128, and miR-33a, suggesting the complicated interactions between diverse non-coding RNAs in the context of CAD. Identification of this multifaceted interaction network is a prerequisite for the development of anti-CAD strategies. Such network has a practical significance in the design of prognostic or diagnostic panels.
Functional studies have confirmed the causal effects of lncRNAs/circRNAs in the pathogenesis of CAD, as the ectopic expression of these transcripts in human endothelial cells has led to dysregulation of proliferation, cell cycle transition, and migration in the favor of CAD development. Moreover, altered expression of these transcripts in immune cells has provoked immune responses and suppressed anti-inflammatory cytokines. A number of additional lncRNAs/circRNAs have functional impact on deposition of lipid in the vessels as demonstrated by in vivo experiments. The synergic effects of these transcripts in the pathobiology of CAD should also be evaluated in functional studies through establishment of double-knockout models.
Some SNPs within lncRNAs have been related with risk of CAD in certain populations. However, the results of these studies have not been replicated in other populations. Therefore, these data are not sufficient to propose these SNPs as markers for CAD in a pan-ethnic scale. Moreover, assessment of haplotypes of these SNPs and their association with risk of CAD would provide more reliable results in this regard.
In addition, circRNAs could partake in the development of CAD via modulation of proliferation, differentiation, or apoptosis in CAD-related cells such as vascular smooth muscle cells. Such roles may be mediated through modulation of expression of several target genes, particularly miRNAs. Similar to lncRNAs, circRNAs can serve as molecular sponges for miRNAs. Expression levels of some circRNAs can be used as diagnostic markers in CAD.
In brief, CAD has been associated with dysregulation of numerous lncRNAs and circRNAs. Moreover, these kinds of non-coding transcripts can be used as markers for prediction of risk of CAD and disease course. The potential of these transcripts as therapeutic targets should be appraised in upcoming investigations. The most important limitation of studies that assessed the functional role of lncRNAs/circRNAs in the pathogenesis of CAD is that they are mostly dependent on cell line studies or animal studies whose generalization to human subjects is not easy. Moreover, the consequences of the observed altered expression of lncRNAs/circRNAs in human subjects should be assessed in follow-up studies. Finally, the association between lncRNAs/circRNAs signature and response to the therapeutic option in CAD patients including coronary artery bypass graft, percutaneous coronary intervention, and medical therapies should be assessed in upcoming studies.
Author Contributions
MT and SG-F wrote the draft and revised it. MG collected the data and designed the tables. MT designed the figures. All the authors approved the submitted version.
Conflict of Interest
The authors declare that the research was conducted in the absence of any commercial or financial relationships that could be construed as a potential conflict of interest.
References
1. Sanchis-Gomar F, Perez-Quilis C, Leischik R, Lucia A. Epidemiology of coronary heart disease and acute coronary syndrome. Ann Transl Med. (2016) 4:256. doi: 10.21037/atm.2016.06.33
2. Matsuzawa Y, Lerman A. Endothelial dysfunction and coronary artery disease: assessment, prognosis, and treatment. Coron Artery Dis. (2014) 25:713–24. doi: 10.1097/MCA.0000000000000178
3. Ding L, Su XX, Zhang WH, Xu YX, Pan XF. Gene expressions underlying mishandled calcium clearance and elevated generation of reactive oxygen species in the coronary artery smooth muscle cells of chronic heart failure rats. Chin Med J. (2017) 130:460–9. doi: 10.4103/0366-6999.199825
4. Nurnberg ST, Cheng K, Raiesdana A, Kundu R, Miller CL, Kim JB, et al. Coronary artery disease associated transcription factor TCF21 regulates smooth muscle precursor cells that contribute to the fibrous cap. PLoS Genet. (2015) 11:e1005155. doi: 10.1371/journal.pgen.1005155
5. Zhang Y, Zhang L, Wang Y, Ding H, Xue S, Qi H, et al. MicroRNAs or long noncoding RNAs in diagnosis and prognosis of coronary artery disease. Aging Dis. (2019) 10:353–66. doi: 10.14336/AD.2018.0617
6. Pan RY, Zhao CH, Yuan JX, Zhang YJ, Jin JL, Gu MF, et al. Circular RNA profile in coronary artery disease. Am J Transl Res. (2019) 11:7115–25. doi: 10.2139/ssrn.3398517
7. Esteller M. Non-coding RNAs in human disease. Nat Rev Genet. (2011) 12:861–74. doi: 10.1038/nrg3074
8. Fang Y, Fullwood MJ. Roles, functions, and mechanisms of long non-coding RNAs in cancer. Genom Proteom Bioinform. (2016) 14:42–54. doi: 10.1016/j.gpb.2015.09.006
9. Kashi K, Henderson L, Bonetti A, Carninci P. Discovery and functional analysis of lncRNAs: methodologies to investigate an uncharacterized transcriptome. Biochim Biophys Acta Gene Regul Mechan. (2016) 1859:3–15. doi: 10.1016/j.bbagrm.2015.10.010
10. Lasda E, Parker R. Circular RNAs: diversity of form and function. RNA. (2014) 20:1829–42. doi: 10.1261/rna.047126.114
11. Gabory A, Jammes H, Dandolo L. The H19 locus: role of an imprinted non-coding RNA in growth and development. Bioessays. (2010) 32:473–80. doi: 10.1002/bies.200900170
12. Gao Y, Wu F, Zhou J, Yan L, Jurczak MJ, Lee HY, et al. The H19/let-7 double-negative feedback loop contributes to glucose metabolism in muscle cells. Nucleic Acids Res. (2014) 42:13799–81. doi: 10.1093/nar/gku1160
13. Yao Y, Xiong G, Jiang X, Song T. The overexpression of lncRNA H19 as a diagnostic marker for coronary artery disease. Rev Assoc Méd Bras. (2019) 65:110–7. doi: 10.1590/1806-9282.65.2.110
14. Cai Y, Yang Y, Chen X, He D, Zhang X, Wen X, et al. Circulating “LncPPARδ” from monocytes as a novel biomarker for coronary artery diseases. Medicine. (2016) 95:e2360. doi: 10.1097/MD.0000000000002360
15. Xu Y, Shao B. Circulating lncRNA IFNG-AS1 expression correlates with increased disease risk, higher disease severity and elevated inflammation in patients with coronary artery disease. J Clin Lab Anal. (2018) 32:e22452. doi: 10.1002/jcla.22452
16. Cho H, Shen GQ, Wang X, Wang F, Archacki S, Li Y, et al. Long noncoding RNA ANRIL regulates endothelial cell activities associated with coronary artery disease by up-regulating CLIP1, EZR, and LYVE1 genes. J Biol Chem. (2019) 294:3881–98. doi: 10.1074/jbc.RA118.005050
17. Hu YW, Guo FX, Xu YJ, Li P, Lu ZF, McVey DG, et al. Long noncoding RNA NEXN-AS1 mitigates atherosclerosis by regulating the actin-binding protein NEXN. J Clin Invest. (2019) 129:1115–28. doi: 10.1172/JCI98230
18. Ebadi N, Ghafouri-Fard S, Taheri M, Arsang-Jang S, Parsa SA, Omrani MD. Dysregulation of autophagy-related lncRNAs in peripheral blood of coronary artery disease patients. Eur J Pharmacol. (2020) 867:172852. doi: 10.1016/j.ejphar.2019.172852
19. Chen J, Dang J. LncRNA CASC11 was downregulated in coronary artery disease and inhibits transforming growth factor-β 1. J Int Med Res. (2020) 48:300060519889187. doi: 10.1177/0300060519889187
20. Guo F, Tang C, Li Y, Liu Y, Lv P, Wang W, et al. The interplay of Lnc RNA ANRIL and miR-181b on the inflammation-relevant coronary artery disease through mediating NF-κB signalling pathway. J Cell Mol Med. (2018) 22:5062–75. doi: 10.1111/jcmm.13790
21. Liu X, Li S, Yang Y, Sun Y, Yang Q, Gu N, et al. The lncRNA ANRIL regulates endothelial dysfunction by targeting the let-7b/TGF-βR1 signalling pathway. J Cell Physiol. (2020) 236:2058–69. doi: 10.1002/jcp.29993
22. Arslan S, Berkan Ö, Lalem T, Özbilüm N, Göksel S, Korkmaz Ö, et al. Long non-coding RNAs in the atherosclerotic plaque. Atherosclerosis. (2017) 266:176–81. doi: 10.1016/j.atherosclerosis.2017.10.012
23. Zhuang J, Peng W, Li H, Wang W, Wei Y, Li W, et al. Methylation of p15 INK4b and expression of ANRIL on chromosome 9p21 are associated with coronary artery disease. PLoS ONE. (2012) 7:e47193. doi: 10.1371/journal.pone.0047193
24. Kallen AN, Zhou XB, Xu J, Qiao C, Ma J, Yan L, et al. The imprinted H19 lncRNA antagonizes let-7 microRNAs. Mol Cell. (2013) 52:101–12. doi: 10.1016/j.molcel.2013.08.027
25. Alikhah A, Kakhki MP, Ahmadi A, Dehghanzad R, Boroumand MA, Behmanesh M. The role of lnc-DC long non-coding RNA and SOCS1 in the regulation of STAT3 in coronary artery disease and type 2 diabetes mellitus. J. Diabetes Complicat. (2018) 32:258–65. doi: 10.1016/j.jdiacomp.2017.12.001
26. Shang J, Li Q, Zhang J, Yuan H. FAL1 regulates endothelial cell proliferation in diabetic arteriosclerosis through PTEN/AKT pathway. Eur Rev Med Pharmacol Sci. (2018) 22:6492–9. doi: 10.26355/eurrev_201810_16063
27. Zhao Y, Feng G, Wang Y, Yue Y, Zhao W. Regulation of apoptosis by long non-coding RNA HIF1A-AS1 in VSMCs: implications for TAA pathogenesis. Int J Clin Exp Pathol. (2014) 7:7643–52.
28. Zhang L, Zhou C, Qin Q, Liu Z, Li P. LncRNA LEF1-AS1 regulates the migration and proliferation of vascular smooth muscle cells by targeting miR-544a/PTEN axis. J Cell Biochem. (2019) 120:14670–8. doi: 10.1002/jcb.28728
29. Wu Z, He Y, Li D, Fang X, Shang T, Zhang H, et al. Long noncoding RNA MEG3 suppressed endothelial cell proliferation and migration through regulating miR-21. Am J Transl Res. (2017) 9:3326–35.
30. Ma Q, Gao Y, Xu P, Li K, Xu X, Gao J, et al. Atorvastatin inhibits breast cancer cells by downregulating PTEN/AKT pathway via promoting ras homolog family member B (RhoB). Biomed Res Int. (2019) 2019:3235021. doi: 10.1155/2019/3235021
31. Hu YW, Yang JY, Ma X, Chen ZP, Hu YR, Zhao JY, et al. A lincRNA-DYNLRB2-2/GPR119/GLP-1R/ABCA1-dependent signal transduction pathway is essential for the regulation of cholesterol homeostasis. J Lipid Res. (2014) 55:681–97. doi: 10.1194/jlr.M044669
32. Hennessy EJ, van Solingen C, Scacalossi KR, Ouimet M, Afonso MS, Prins J, et al. The long noncoding RNA CHROME regulates cholesterol homeostasis in primates. Nat Metab. (2019) 1:98–110. doi: 10.1038/s42255-018-0004-9
33. Li X, Hou L, Cheng Z, Zhou S, Qi J, Cheng J. Overexpression of GAS5 inhibits abnormal activation of Wnt/β-catenin signaling pathway in myocardial tissues of rats with coronary artery disease. J Cell Physiol. (2019) 234:11348–59. doi: 10.1002/jcp.27792
34. Cai Y, Yang Y, Chen X, Wu G, Zhang X, Liu Y, et al. Circulating ‘lncRNA OTTHUMT00000387022'from monocytes as a novel biomarker for coronary artery disease. Cardiovasc Res. (2016) 112:714–24. doi: 10.1093/cvr/cvw022
35. Wang H, Jin Z, Pei T, Song W, Gong Y, Chen D, et al. Long noncoding RNAs C2dat1 enhances vascular smooth muscle cell proliferation and migration by targeting MiR-34a-5p. J Cell Biochem. (2019) 120:3001–8. doi: 10.1002/jcb.27070
36. Qi H, Shen J, Zhou W. Up-regulation of long non-coding RNA THRIL in coronary heart disease: prediction for disease risk, correlation with inflammation, coronary artery stenosis, and major adverse cardiovascular events. J Clin Lab Anal. (2020) 34:e23196. doi: 10.1002/jcla.23196
37. Liao B, Chen R, Lin F, Mai A, Chen J, Li H, et al. Long noncoding RNA HOTTIP promotes endothelial cell proliferation and migration via activation of the Wnt/β-catenin pathway. J Cell Biochem. (2018) 119:2797–805. doi: 10.1002/jcb.27284
38. Cai C, Zhu H, Ning X, Li L, Yang B, Chen S, et al. LncRNA ENST00000602558. 1 regulates ABCG1 expression and cholesterol efflux from vascular smooth muscle cells through a p65-dependent pathway. Atherosclerosis. (2019) 285:31–9. doi: 10.1016/j.atherosclerosis.2019.04.204
39. Wang X, Zhao Z, Zhang W, Wang Y. Long noncoding RNA LINC00968 promotes endothelial cell proliferation and migration via regulating miR-9-3p expression. J Cell Biochem. (2019) 120:8214–21. doi: 10.1002/jcb.28103
40. Yan H, Bu S, Zhou W, Mai Y. TUG1 promotes diabetic atherosclerosis by regulating proliferation of endothelial cells via Wnt pathway. Eur Rev Med Pharmacol Sci. (2018) 22:6922–9. doi: 10.26355/eurrev_201810_16162
41. Yin Q, Wu A, Liu M. Plasma long non-coding RNA (lncRNA) GAS5 is a new biomarker for coronary artery disease. Med Sci Monit. (2017) 23:6042–8. doi: 10.12659/MSM.907118
42. Bai Y, Zhang Q, Su Y, Pu Z, Li K. Modulation of the proliferation/apoptosis balance of vascular smooth muscle cells in atherosclerosis by lncRNA-MEG3 via regulation of miR-26a/Smad1 axis. Int Heart J. (2019) 60:444–50. doi: 10.1536/ihj.18-195
43. Wu G, Cai J, Han Y, Chen J, Huang ZP, Chen C, et al. LincRNA-p21 regulates neointima formation, vascular smooth muscle cell proliferation, apoptosis, and atherosclerosis by enhancing p53 activity. Circulation. (2014) 130:1452–65. doi: 10.1161/CIRCULATIONAHA.114.011675
44. Hu Y, Hu J. Diagnostic value of circulating lncRNA ANRIL and its correlation with coronary artery disease parameters. Braz J Med Biol Res. (2019) 52:e8309. doi: 10.1590/1414-431x20198309
45. Yari M, Bitarafan S, Broumand MA, Fazeli Z, Rahimi M, Ghaderian SMH, et al. Association between long noncoding RNA ANRIL expression variants and susceptibility to coronary artery disease. Int J Mol Cell Med. (2018) 7:1. doi: 10.1590/1414-431X20198309
46. Yang Y, Cai Y, Wu G, Chen X, Liu Y, Wang X, et al. Plasma long non-coding RNA, CoroMarker, a novel biomarker for diagnosis of coronary artery disease. Clin Sci. (2015) 129:675–85. doi: 10.1042/CS20150121
47. Lai Z, Lin P, Weng X, Su J, Chen Y, He Y, et al. MicroRNA-574-5p promotes cell growth of vascular smooth muscle cells in the progression of coronary artery disease. Biomed Pharmacother. (2018) 97:162–7. doi: 10.1016/j.biopha.2017.10.062
48. Zhang Z, Gao W, Long QQ, Zhang J, Li YF, Yan JJ, et al. Increased plasma levels of lncRNA H19 and LIPCAR are associated with increased risk of coronary artery disease in a Chinese population. Sci Rep. (2017) 7:7491. doi: 10.1038/s41598-017-07611-z
49. Zhang Y, Zhang L, Wang Y, Ding H, Xue S, Yu H, et al. KCNQ 1 OT 1, HIF 1A-AS 2 and APOA 1-AS are promising novel biomarkers for diagnosis of coronary artery disease. Clin Exp Pharmacol Physiol. (2019) 46:635–42. doi: 10.1111/1440-1681.13094
50. Li X, Zhao Z, Gao C, Rao L, Hao P, Jian D, et al. Identification of a peripheral blood Long non-coding RNA (Upperhand) as a potential diagnostic marker of coronary artery disease. Cardiol J. (2018) 25:393–402. doi: 10.5603/CJ.a2017.0133
51. Toraih EA, El-Wazir A, Alghamdi SA, Alhazmi AS, El-Wazir M, Abdel-Daim MM, et al. Association of long non-coding RNA MIAT and MALAT1 expression profiles in peripheral blood of coronary artery disease patients with previous cardiac events. Genet Mol Biol. (2019) 42:509–18. doi: 10.1590/1678-4685-gmb-2018-0185
52. Shanker J, Arvind P, Jambunathan S, Nair J, Kakkar VV. Genetic analysis of the 9p21. 3 CAD risk locus in Asian Indians. Thromb Haemost. (2014) 112:960–9. doi: 10.1160/TH13-08-0706
53. Mangalarapu M, Vinukonda S, Komaravalli PL, Nagula P, Koduganti RR, Korripally P, et al. Association of CDKN2BAS gene polymorphism with periodontitis and coronary artery disease from south Indian population. Gene. (2019) 710:324–32. doi: 10.1016/j.gene.2019.06.002
54. Khademi KG, Foroughmand AM, Galehdari H, Yazdankhah S, Borujeni MP, Shahbazi Z, et al. Association study of rs1333040 and rs1004638 polymorphisms in the 9p21 locus with coronary artery disease in Southwest of Iran. Iran Biomed J. (2016) 20:122–7. doi: 10.7508/ibj.2016.02.008
55. Hu WN, Ding HX, Xu Q, Zhang XY, Yang DT, Jin YZ. Relationship between long noncoding RNA H19 Polymorphisms and risk of coronary artery disease in a chinese population: a case-control study. Dis Markers. (2020) 2020:9839612. doi: 10.1155/2020/9839612
56. Yang J, Gu L, Guo X, Huang J, Chen Z, Huang G, et al. LncRNA ANRIL expression and ANRIL gene polymorphisms contribute to the risk of ischemic stroke in the Chinese han population. Cell Mol Neurobiol. (2018) 38:1253–69. doi: 10.1007/s10571-018-0593-6
57. AbdulAzeez S, Al-Nafie AN, Al-Shehri A, Borgio JF, Baranova EV, Al-Madan MS, et al. Intronic polymorphisms in the CDKN2B-AS1 gene are strongly associated with the risk of myocardial infarction and coronary artery disease in the Saudi population. Int J Mol Sci. (2016) 17:395. doi: 10.3390/ijms17030395
58. Gao W, Zhu M, Wang H, Zhao S, Zhao D, Yang Y, et al. Association of polymorphisms in long non-coding RNA H19 with coronary artery disease risk in a Chinese population. Mutat Res. (2015) 772:15–22. doi: 10.1016/j.mrfmmm.2014.12.009
59. Tarighi S, Alipoor B, Zare A, Ghaedi H, Shanaki M. Association of the rs1870634 variant in long intergenic non-protein coding RNA 841 with coronary artery disease: a GWAS-replication study in an Iranian population. Biochem Genet. (2018) 56:522–32. doi: 10.1007/s10528-018-9859-4
60. Wang G, Li Y, Peng Y, Tang J, Li H. Association of polymorphisms in MALAT1 with risk of coronary atherosclerotic heart disease in a Chinese population. Lipids Health Dis. (2018) 17:75. doi: 10.1007/978-981-13-0620-4
61. Tang SS, Cheng J, Cai MY, Yang XL, Liu XG, Zheng BY, et al. Association of lincRNA-p21 haplotype with coronary artery disease in a Chinese Han population. Dis Markers. (2016) 2016:9109743. doi: 10.1155/2016/9109743
62. Shahmoradi N, Nasiri M, Kamfiroozi H, Kheiry MA. Association of the rs555172 polymorphism in SENCR long non-coding RNA and atherosclerotic coronary artery disease. J Cardiovasc Thorac Res. (2017) 9:170–4. doi: 10.15171/jcvtr.2017.29
63. Wang L, Shen C, Wang Y, Zou T, Zhu H, Lu X, et al. Identification of circular RNA Hsa_circ_0001879 and Hsa_circ_0004104 as novel biomarkers for coronary artery disease. Atherosclerosis. (2019) 286:88–96. doi: 10.1016/j.atherosclerosis.2019.05.006
64. Pan RY, Liu P, Zhou HT, Sun WX, Song J, Shu J, et al. Circular RNAs promote TRPM3 expression by inhibiting hsa-miR-130a-3p in coronary artery disease patients. Oncotarget. (2017) 8:60280–90. doi: 10.18632/oncotarget.19941
65. Mao YY, Wang JQ, Guo XX, Bi Y, Wang CX. Circ-SATB2 upregulates STIM1 expression and regulates vascular smooth muscle cell proliferation and differentiation through miR-939. Biochem Biophys Res Commun. (2018) 505:119–25. doi: 10.1016/j.bbrc.2018.09.069
66. Wang M, Li J, Cai J, Cheng L, Wang X, Xu P, et al. Overexpression of microRNA-16 alleviates atherosclerosis by inhibition of inflammatory pathways. Biomed Res Int. (2020) 2020:8504238. doi: 10.1155/2020/8504238
67. Guo M, Yan R, Ji Q, Yao H, Sun M, Duan L, et al. IFN regulatory Factor-1 induced macrophage pyroptosis by modulating m6A modification of circ_0029589 in patients with acute coronary syndrome. Int Immunopharmacol. (2020) 86:106800. doi: 10.1016/j.intimp.2020.106800
68. Sun J, Zhang Z, Yang S. Circ_RUSC2 upregulates the expression of miR-661 target gene SYK and regulates the function of vascular smooth muscle cells. Biochem Cell Biol. (2019) 97:709–14. doi: 10.1139/bcb-2019-0031
69. Zhao Z, Li X, Gao C, Jian D, Hao P, Rao L, et al. Peripheral blood circular RNA hsa_circ_0124644 can be used as a diagnostic biomarker of coronary artery disease. Sci Rep. (2017) 7:39918. doi: 10.1038/srep39918
70. Gao L, Zeng H, Zhang T, Mao C, Wang Y, Han Z, et al. MicroRNA-21 deficiency attenuated atherogenesis and decreased macrophage infiltration by targeting Dusp-8. Atherosclerosis. (2019) 291:78–86. doi: 10.1016/j.atherosclerosis.2019.10.003
71. Wu WZ, Pan YH, Cai MY, Cen JM, Chen C, Zheng L, et al. Plasma-derived exosomal circular RNA hsa_circ_0005540 as a novel diagnostic biomarker for coronary artery disease. Dis Markers. (2020) 2020:3178642. doi: 10.1155/2020/3178642
72. Miao L, Yin RX, Zhang QH, Liao PJ, Wang Y, Nie RJ, et al. A novel circRNA-miRNA-mRNA network identifies circ-YOD1 as a biomarker for coronary artery disease. Sci Rep. (2019) 9:18314. doi: 10.1038/s41598-019-54603-2
Keywords: long non-coding RNA, circRNA, coronary artery disorder, expression, biomarkers
Citation: Ghafouri-Fard S, Gholipour M and Taheri M (2021) The Emerging Role of Long Non-coding RNAs and Circular RNAs in Coronary Artery Disease. Front. Cardiovasc. Med. 8:632393. doi: 10.3389/fcvm.2021.632393
Received: 23 November 2020; Accepted: 15 January 2021;
Published: 23 February 2021.
Edited by:
En-Zhi Jia, Nanjing Medical University, ChinaReviewed by:
Mingxian Chen, Central South University, ChinaShujie Guo, Shanghai Institute of Hypertension, China
Copyright © 2021 Ghafouri-Fard, Gholipour and Taheri. This is an open-access article distributed under the terms of the Creative Commons Attribution License (CC BY). The use, distribution or reproduction in other forums is permitted, provided the original author(s) and the copyright owner(s) are credited and that the original publication in this journal is cited, in accordance with accepted academic practice. No use, distribution or reproduction is permitted which does not comply with these terms.
*Correspondence: Mohammad Taheri, bW9oYW1tYWRfODIzQHlhaG9vLmNvbQ==