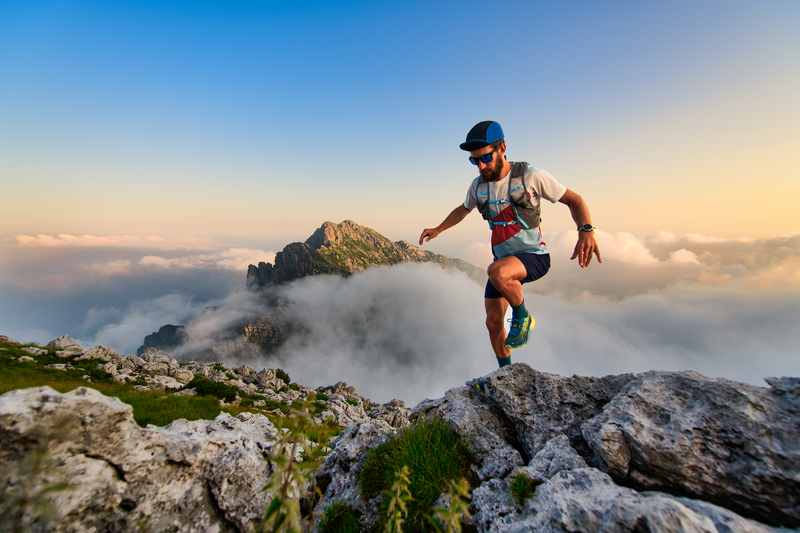
94% of researchers rate our articles as excellent or good
Learn more about the work of our research integrity team to safeguard the quality of each article we publish.
Find out more
REVIEW article
Front. Cardiovasc. Med. , 26 March 2021
Sec. Cardiovascular Imaging
Volume 8 - 2021 | https://doi.org/10.3389/fcvm.2021.613400
This article is part of the Research Topic Advances in Intravascular Imaging View all 10 articles
Intravascular optical coherence tomography (IVOCT) that produces images with 10 μm resolution has emerged as a significant technology for evaluating coronary architectural morphology. Yet, many features that are relevant to coronary plaque pathogenesis can only be seen at the cellular level. This issue has motivated the development of a next-generation form of OCT imaging that offers higher resolution. One such technology that we review here is termed micro-OCT (μOCT) that enables the assessment of the cellular and subcellular morphology of human coronary atherosclerotic plaques. This chapter reviews recent advances and ongoing works regarding μOCT in the field of cardiology. This new technology has the potential to provide researchers and clinicians with a tool to better understand the natural history of coronary atherosclerosis, increase plaque progression prediction capabilities, and better assess the vessel healing process after revascularization therapy.
In the early 1990's, intravascular optical coherence tomography (IVOCT) (1) commenced with the understanding that OCT (2) could be clinically applied beyond ophthalmology. Conventional IVOCT employs broadband near-infrared light centered at a wavelength of 1,300 nm (3), providing it with a spatial resolution of about 10 μm that is an order of magnitude higher than that of intravascular ultrasound (IVUS) (4). The roughly 10-μm-resolution of IVOCT provides detailed information on treated and untreated coronary plaque morphology by resolving varying arterial microscopic architectural structures (5–10). Similarly to the circumferential view of IVUS, depth information provided by IVOCT makes it possible to display coronary artery lumen cross-sections (9, 10), luminal narrowing (11), and intimal thickening (12). Its higher resolution enables other features to be clearly identified, including fibrous cap thickness (4–6, 13), lipid (4, 13), cholesterol crystals (4), thrombus (4), dissections (4), macrophage accumulations (4, 7), calcium (4–6), and intimal neo-vasculature (4). Hence, IVOCT has improved diagnostic accuracy for human coronary plaques (5, 7), and its feasibility for guiding coronary intervention has been consistently demonstrated (14–19). Over the past two decades, interventional cardiologists and engineers have worked together to make tremendous progress to develop and validate IVOCT as a useful instrument for visualizing the detailed morphology of coronary plaque and stents.
Despite the potential importance of physiological assessment of myocardial ischemia due to significant organic coronary stenosis (20), recent studies have highlighted that the initial interventional strategy with percutaneous coronary intervention or coronary artery bypass does not necessarily result in better clinical outcomes in stable (chronic) coronary artery disease (CAD) patients when compared to optimal medical therapy (21). These results have raised questions regarding what coronary morphological features bestow high risk of a future clinical event, regardless of the severity of the luminal narrowing. To address these questions, OCT with even higher resolution could illuminate the roles of coronary microstructures heretofore unseen, such as individual coronary endothelial cells (22, 23), inflammatory cells, cholesterol crystals (24), vascular smooth muscle cells (25), fibroblasts, (micro-)calcifications (26), and components of thrombi such as platelets and fibrin, all thought to play roles in natural history of coronary atherosclerosis and the clinical manifestations of high risk lesions (27).
In 2011, a new mode of OCT termed micro-OCT (μOCT) was demonstrated with a resolution of 1–2 μm (28). The initial μOCT technology was implemented using a bench-top microscope system and has shown broad utility for a variety of in vitro and ex vivo studies and applications (28–32). Recently, to implement μOCT clinically, a single fiber optic μOCT probe and intracoronary catheter have been created (33, 34)—the technology is now poised to be used in coronaries in vivo (35). In this article, we review the developments in μOCT technology and describe its potential clinical implications for intracoronary imaging.
Endothelial cells act as gatekeepers for the passage of low-density lipoprotein (LDL) and leukocytes into the intima, and thus endothelial disruption/dysfunction is considered to be an important catalyst of coronary atherogenesis (36, 37). Previous ultrastructural studies have demonstrated that endothelial cells cover the intima in a “cobblestone” pattern, also known as “endothelial pavementing” on en-face SEM (22). It has been recognized that coronary plaque erosion characterized by lesions with loss of endothelial cells beneath thrombus is the second most prevalent histopathological cause of acute coronary syndrome (ACS) (27, 38, 39).
μOCT has been shown to visualize swine and human coronary endothelial cells ex vivo (40). The capability of μOCT to visualize endothelial cells was validated with the current gold standard scanning electron microscopy (SEM) (22). The histological validation study included a visual comparison of swine coronary endothelial pavementing seen by μOCT, volume-rendered in three-dimension (3D-μOCT), with that seen by en-face SEM (Figure 1) (40). 3D-μOCT images clearly showed the uneven endothelial surface corresponding to coronary endothelial pavementing seen on corresponding SEM. After endothelial stripping (41), the surface roughness disappeared from the 3D-μOCT image, indicating the absence of endothelial cells. Quantitative analysis was performed by calculating surface roughness on a μOCT data-set and the corresponding SEM (40), demonstrating a high degree of correlation between μOCT and the SEM gold standard (R2 = 0.99, P < 0.01).
Figure 1. μOCT images of endothelial pavementing and corresponding SEM in swine and human coronary arteries ex vivo. Upper panels: Swine coronary endothelial pavementing visualized by three-dimensionally (3D) volume-rendered μOCT is similar to that seen by scanning electron microscopy (SEM). Lower panels: Findings were consistent in human coronary fibrous plaque. Scale bars, 25 μm. Figure and capture reprinted with permission from Nishimiya et al. (40).
μOCT has also been used to visualize endothelial cells in human cadaver coronaries ex vivo. Endothelial pavementing was confirmed in early coronary lesions with intimal thickening and fibrous plaque (4) while lesions with superficial nodular calcification and necrotic core (4) lacked endothelial cells. Indeed, the results of surface roughness measurements indicated that endothelial cell distributions diminished over fibroatheromatous and fibrocalcific coronary plaques as compared with intimal-thickening and fibrous lesions (40). 3D-μOCT images of drug-eluting stents (DES) implanted in coronary segments also showed variable presence or absence of the endothelial cell coverage, which standard OCT was unable to identify (Figure 2A).
Figure 2. 3D-μOCT of stent and endothelial coverage morphology in the human cadaver coronary artery ex vivo. (A) 3D-μOCT image of a drug-eluting stent (DES) implanted in a human coronary artery, showing tissue coverage with low intensity signal (red asterisks) and the surface of polymer coating as highly reflective regions (green asterisks). Scale bars, 100 μm. Unpublished data, obtained at the Massachusetts General Hospital. (B) DES struts showing polymers (red dashed box and inset) overlying the stent strut reflections. Scale bars, 30 μm. Figure and capture reprinted with permission from Liu et al. (28).
Studies have suggested that fluid shear stress induces spindle-shaped endothelial morphology that is aligned in the direction of flow while those exposed to low endothelial shear stress (ESS) are nonuniformly oriented (42). Furthermore, low and turbulent induce increased vascular permeability (36, 42) that may increase the probability of LDL and leukocyte influx. Because, it can be performed on fresh tissue and over large areas in three-dimensions, μOCT assessment of endothelial morphology's could increase our understanding of coronary regions altered by shear stress, such as bifurcations and segments at myocardial bridges (43).
Since ruptured coronary plaques account for the majority of ACS (44), an improved understanding of the role of endothelial cells in the progression of atherosclerosis and early identification of plaques at high risk are anticipated to have considerable clinical impact. A number of seminal studies have suggested that certain OCT features of thin cap fibroatheromas (TCFA), such as the thickness of fibrous caps, are critical (13, 45). However, OCT cut off values for high risk cap thicknesses are still undetermined (23). Evidence suggests that in TCFA lesions, apoptotic macrophages are not efficiently cleared by efferocytosis and are therefore prone to secondary necrosis, contributing to expansion of the necrotic core and further thinning of the fibrous cap (46). It is thus conceivable that endothelial cell wall border alignment can vary at the weakest point of these caps of TCFA. In this manner, 3D-μOCT visualization and calculation of endothelial surface roughness may augment precision definition of plaque vulnerability in humans.
Since the endothelial monolayer is below the resolution of OCT, the current OCT diagnostic criteria for a coronary erosion is defined as the presence of an intact fibrous cap at the culprit site with overlying thrombus (47). This criterion is a retrospective definition, as thrombus is required to demarcate this entity. In addition, clinically insignificant plaque erosion may occur without increased thrombogenicity resulting in healed plaque (48). Thus, there is a need for a prospective definition of a site that is at high risk of erosion and subsequent thrombus formation. Owing to its capacity to directly visualize the endothelium, μOCT may bridge these gaps in our diagnostic capabilities. Data has shown that μOCT is capable of imaging white thrombus containing fibrin (the type that is common in erosion), small platelets and multiple entrapped cells (28). Whether μOCT can clearly visualize the endothelium beneath thrombus remains an open question.
Compared to conventional OCT, which is incapable of distinctly visualizing endothelial cells, μOCT could make it possible to definitively assess endothelial coverage of stent struts and this information could be potentially used to shorten antiplatelet therapy treatment durations. In the emerging era of biodegradable-polymer DES (49) and bioresorbable scaffolds (50), μOCT should be capable of evaluating standalone stent polymers or polymer-coating overlying metal stents (Figure 2B). The use of μOCT technology to assess DES strut endothelial coverage may help resolve current questions and controversies regarding novel stent healing responses, potentially leading to a means for determining optimal antiplatelet therapy durations.
Inflammatory cells, such as leukocytes, monocytes, and macrophages play key roles in developing coronary atherosclerotic lesions (51). Because of its exquisite resolution, μOCT is capable of typing leukocytes based on cellular and intracellular morphology (28, 52). Additionally, μOCT has been shown to be quite capable of imaging pseudopods that inform on the activity of these cells (Figure 3A) (28). For example, compared to smaller cells with scant cytoplasm, consistent with lymphocytes, some of large cells seen on the surface had bean-shaped nucleus inside, presumably corresponding to monocytes (Figure 3B) (28). Macrophages are also seen clearly by μOCT as highly scattering, flocculent, round or ellipsoidal cells (28, 52), and are frequently observed over and within necrotic core lesions (Figure 3C) (28, 52). Some of these features have been recently demonstrated in the nasal airways in vivo, as μOCT was shown to be able to clearly visualize granulocytes in the mucus and epithelium of patients with cystic fibrosis (32).
Figure 3. μOCT for inflammatory cell morphology in human cadaver coronaries ex vivo. (A) Multiple leukocytes tethered to the endothelial surface by linear structures, suggestive of pseudopodia (white arrows). (B) A cell with an indented, bean-shaped nucleus (green arrow) suggestive of a monocyte. (C) Necrotic core fibroatheroma with highly scattering lipid-laden macrophages or foam cells (white arrows) infiltrating the fibrous-cap that were similarly seen in the corresponding histology (top left inset). An intracellular region of low signal, a suggestive of the nucleus, was shown within the cytoplasm of a foam cell (bottom left inset, denoted by blue arrow). Scale bars, 30 μm. Figure and capture reprinted with permission from Liu et al. (28).
Inflammatory cells play a pivotal role in all phases of coronary atherosclerosis. The compromised endothelial barrier permits them to invade into the tunica intima and initiate arterial wall thickening. Macrophages contribute to plaque vulnerability by producing proteolytic enzymes that digest extracellular matrix and destroy the integrity of the fibrous cap (46) and through their accumulation and death that form biomechanically unstable lipid deposits. For these and many other reasons, it is important to explore macrophage behavior in atherosclerotic lesions in vivo. Owing to its 3D imaging capabilities, μOCT makes it possible to observe such morphologic phenomena that are rarely seen in 2D cross sections. Due to the cellular resolution capabilities of μOCT, this technology could potentially also identify plaques with neutrophil extracellular trap (NETs) accumulations that induce endothelial cell apoptosis and resultant plaque erosion (53).
In in vitro cell culture experiments, macrophages containing cholesterol crystals demonstrated higher cytoplasmic scattering in μOCT images when compared to those without cholesterol crystals (Figure 4) (52). Of note, there was a discrepancy that cholesterol crystals were detected by the gold-standard polarization microscopy but were not seen on the μOCT image (Figures 4A,B). Using polarization microscopy, the accuracy of cholesterol crystal inclusions in macrophages relied on the size of cholesterol crystals (the size ≥100 mm2, 52% vs. <100 mm2, 36%, P < 0.05). 3D-μOCT clearly visualized a macrophage cell with the high scattering cholesterol crystal within its cytoplasm (Figure 4C).
Figure 4. μOCT for macrophages containing cholesterol crystals in vitro and ex vivo. (A) En-face and (B,C) cross-sections of the macrophage demonstrated highly scattering constituents inside its cytoplasm (yellow arrow). (D) Corresponding polarization microscopy (PLM) confirming the cholesterol crystal inclusions (red arrow). (E) Cholesterol crystals that was determined by PLM (F) was not definitive on the μOCT image (yellow arrow). (G,H) Representative μOCT images of the macrophage cell in human coronary artery ex vivo contained highly scattering cholesterol inclusions within their cytoplasm [yellow arrow in panel (G); red in panel (H)]. Scale bars, 50 μm. Figure and capture reprinted with permission from Kashiwagi et al. (52).
Cholesterol crystal protrusion toward the lumen has recently been proposed as a possible cause of thrombosis and resultant ACS (54). 3D-μOCT has shown clear delineation of multilayered cholesterol crystal sheets in human cadaver coronary arteries (Figure 5A) (28) and their protrusions that were similar to what seen by SEM (Figure 5B).
Figure 5. 3D-μOCT for visualizing cholesterol crystals and their protrusion in the human cadaver coronary artery ex vivo. (A) Fibroatheroma with large necrotic core (NC) showing multilayered cholesterol crystals (CC), characterized by reflections from their top and bottom surfaces. Scale bars, 30 μm. Figure and capture reprinted with permission from Liu et al. (28). (B) 3D-μOCT for a fibroatheromatous human coronary plaque with manually segmenting a protruding cholesterol crystal (yellow area). (B) Corresponding SEM image showing cholesterol crystals protruding from the arterial wall. Unpublished data, obtained at the Massachusetts General Hospital.
Several recent studies have highlighted that anti-inflammatory pharmacotherapeutic strategies (e.g., an interleukin-1β neutralizing human monoclonal antibody, colchicine) (55, 56) may have a high potential to eliminate residual risk of CAD. Intimal crystals have been identified as a possible therapeutic target for cardiovascular disease, due to the potential of these crystals to exacerbate inflammation through inflammasome-mediated cytokine production/activation (57, 58). Identification of localized vascular inflammation as intimal crystals surrounded by inflammatory changes using μOCT would be helpful for assessing the effects of these novel therapeutic agents in patients in vivo.
Recently, the optical imaging elements required to conduct intravascular μOCT were demonstrated (33, 34) and integrated into a catheter that had a size that was suitable for human coronary imaging (35). The imaging capability of the intravascular μOCT catheter was shown in human cadaver coronary arteries ex vivo and atherosclerotic rabbit aortae in vivo (35). μOCT circumferential views displayed cellular and subcellular coronary structures that were not readily identified by the standard OCT. For instance, small or large cholesterol crystal sheets were consistently noted in human lipid-rich plaques ex vivo (Figure 6) that were sometimes difficult to interpret in corresponding convention IVOCT images. As with the ex vivo bench top studies, smooth muscle cells could be clearly visualized as low-intensity, slit-like structures within the intima. Likewise, cross-sectional μOCT showed macrophage diapedesis in human coronaries ex vivo. 3D-rendering of μOCT images exhibited that individual macrophages residing on the surface of fibroatheromatous plaques that appeared to be transmigrating through the endothelium toward a deposit of intimal cholesterol crystals (Figures 7A,B) or with their pseudopods opposing each other (Figures 7C,D). Thrombus could be noted with cells that were consistent with leucocytes embedded in the intraluminal mass. The findings of this study (35) indicate that we are on the threshold of conducting intracoronary μOCT in vivo and await the development of clinical versions of these devices for the first-in-human studies.
Figure 6. Catheter-based intravascular μOCT for cholesterol crystals in the human cadaver coronary ex vivo. Circumferential image showing that μOCT was capable of resolving small cholesterol crystals at distances close to the sheath (a couple hundred microns, orange inset) and far from the sheath (~1 mm, red inset) simultaneously. Scale bars, 100 μm. Figure and capture reprinted with permission from Yin et al. (35).
Figure 7. Intravascular μOCT for inflammatory cells in human cadaver coronaries ex vivo. (A) Individual macrophages (yellow arrows) residing on the surface of a fibroatheromatous plaque that (B) appeared to be transmigrating through the endothelium toward a deposit of intimal cholesterol crystals (red arrows). (C) 3D-μOCT image showing a pair of macrophages tethered to the surface, (D) polarized toward one another with extended pseudopodia (blue arrow). Scale bars, 50 μm. Figure and capture reprinted with permission from Yin et al. (35).
To achieve clinical intracoronary μOCT in the cardiac catheterization lab, several limitations and barriers still need to be resolved. First, to attain such high resolution images, μOCT is currently conducted at a shorter light wavelength (centered at 800 nm) that is lower than that of standard OCT (1,300 nm). The use of this shorter wavelength decreases the penetration depth of light in tissue, potentially further compromising its ability to assess intimal thickness and thus plaque remodeling. Second, imaging with μOCT collects ~3 orders of magnitude more data than imaging with standard OCT, and so the image sizes of a μOCT pullback will be 1,000 times greater than those of a standard OCT pullback. This 1,000-fold increase in data puts a great strain on data acquisition sensitivity and electronics, so currently the frame rate of acquiring μOCT images is significantly slower than that of standard OCT. With today's technology, imaging the entire length of a coronary artery with isotropic 1–2 μm resolution would require many pullbacks, each needing a radiocontrast flush for blood clearance. Interpreting the immense amount of information provided by μOCT may be difficult for interventional cardiologist; it is likely that artificial intelligence will be needed to aid image analysis. 3D visualization of μOCT will also likely facilitate image understanding in real time. The development of machine learning algorithms and rapid 3D rendering are ongoing topics of investigation in the μOCT field. Future technological developments will be focused on addressing these limitations to enable practical application of μOCT in the cath lab.
Clinical applications of μOCT for coronary imaging has the potential to be extensive. In the very beginning of coronary plaque development, altered shear-stress affects the endothelial cell alignment and effectuates endothelial dysfunction (42). Although such a relationship between shear-stress and endothelial cell orientation has been known for a long while, the finding has not been demonstrated in living patients in vivo. Furthermore, visualization of ongoing inflammatory cell adhesion, plaque disruption and blood coagulation remain elusive in humans in vivo. For interventional cardiology, μOCT will provide precise information for acute thrombotic formation around stent struts (23) at a microscopic level. A longitudinal view of stent architecture by 3D-μOCT has been demonstrated in rabbit aorta in vivo (Figure 8) (35). The detailed information regarding stent malapposition (59) or stent fracture (60) could help in the early detection of a precursor of procedure-related stent thrombosis. Visualization of DES polymer cracking (61) and the tissue response to anti-proliferative agents delivered by drug-coated balloons (62) could help clinicians to predict the arterial healing process.
Figure 8. Intravascular 3D-μOCT of a drug-eluting stent implanted in the atherosclerotic rabbit iliac artery in vivo. The artery was imaged immediately after the stent implantation. Stent struts are denoted by the purple and red arrows. Scale bar, 100 μm. Figure and capture reprinted with permission from Yin et al. (35).
μOCT is a next-generation form of OCT that provides an order of magnitude increase in axial and lateral resolution. Our group has demonstrated that μOCT enables the visualization of structures relevant to coronary atherosclerosis pathogenesis and stent healing at cellular/subcellular levels. The recently developed μOCT coronary catheter brings this technology close to clinial use. Clinical studies will be conducted with intracoronary μOCT in the near future. Results will potentially change the landscape of coronary imaging and our understanding of coronary disease and its treatment.
All authors listed have made a substantial, direct and intellectual contribution to the work, and approved it for publication.
This work was supported by the US National Institutes of Health (contracts R01HL076398, R01HL122388, and R01HL137913), John and Dottie Remondi Family Foundation, the Mike and Sue Hazard Family Foundation, and the MGH Research Scholars program, the Cystic Fibrosis Foundation (contract TEARNE07XX0).
GT receives catheter materials from Terumo Corporation. Massachusetts General Hospital has a licensing arrangement with Terumo Corporation. He has the rights to receive royalties from this licensing arrangement and he receives sponsored research funding pertaining to coronary OCT from Vivolight, Canon Inc., and CN USA Biotech Holdings and AstraZeneca sponsor intracoronary μOCT research in GT's lab. GT has a financial/fiduciary interest in SpectraWave, a company developing an OCT-NIRS intracoronary imaging system and catheter. His financial/fiduciary interest was reviewed and is managed by the Massachusetts General Hospital and Partners HealthCare in accordance with their conflict of interest policies. He also has a consulting arrangement with SpectraWave.
The remaining author declares that the research was conducted in the absence of any commercial or financial relationships that could be construed as a potential conflict of interest.
We wish to acknowledge all laboratory members and collaborators worked for the μOCT project at the Wellman Center for Photomedicine, the Massachusetts General Hospital, Harvard Medical School. KN would like to thank for the supports by a Research Fellowship Programs from the MSD Life Science Foundation, and the Uehara Memorial Foundation (Japan). We also gratefully acknowledge the Hazard Family Foundation and John and Dottie Remondi Foundation for their generous support of μOCT work.
1. Tearney GJ, Brezinski ME, Bouma BE, Boppart SA, Pitris C, Southern JF, et al. In vivo endoscopic optical biopsy with optical coherence tomography. Science. (1997) 276:2037–9. doi: 10.1126/science.276.5321.2037
2. Huang D, Swanson EA, Lin CP, Schuman JS, Stinson WG, Chang W, et al. Optical coherence tomography. Science. (1991) 254:1178–81. doi: 10.1126/science.1957169
3. Yun SH, Tearney GJ, Vakoc BJ, Shishkov M, Oh WY, Desjardins AE, et al. Comprehensive volumetric optical microscopy in vivo. Nat Med. (2006) 12:1429–33. doi: 10.1038/nm1450
4. Tearney GJ, Regar E, Akasaka T, Adriaenssens T, Barlis P, Bezerra HG, et al. Consensus standards for acquisition, measurement, and reporting of intravascular optical coherence tomography studies: a report from the International Working Group for Intravascular Optical Coherence Tomography Standardization and Validation. J Am Coll Cardiol. (2012) 59:1058–72. doi: 10.1016/j.jacc.2011.09.079
5. Yabushita H, Bouma BE, Houser SL, Aretz HT, Jang IK, Schlendorf KH, et al. Characterization of human atherosclerosis by optical coherence tomography. Circulation. (2002) 106:1640–5. doi: 10.1161/01.CIR.0000029927.92825.F6
6. Jang IK, Bouma BE, Kang DH, Park SJ, Park SW, Seung KB, et al. Visualization of coronary atherosclerotic plaques in patients using optical coherence tomography comparison with intravascular ultrasound. J Am Coll Cardiol. (2002) 39:604–9. doi: 10.1016/S0735-1097(01)01799-5
7. Tearney GJ, Yabushita H, Houser SL, Aretz HT, Jang IK, Schlendorf KH, et al. Quantification of macrophage content in atherosclerotic plaques by optical coherence tomography. Circulation. (2003) 107:113–9. doi: 10.1161/01.CIR.0000044384.41037.43
8. Bouma BE, Tearney GJ, Yabushita H, Shishkov M, Kauffman CR, DeJoseph Gauthier D, et al. Evaluation of intracoronary stenting by intravascular optical coherence tomography. Heart. (2003) 89:317–20. doi: 10.1136/heart.89.3.317
9. Jang IK, Tearney GJ, MacNeill B, Takano M, Moselewski F, Iftima N, et al. In vivo characterization of coronary atherosclerotic plaque by use of optical coherence tomography. Circulation. (2005) 111:1551–5. doi: 10.1161/01.CIR.0000159354.43778.69
10. Kawasaki M, Bouma BE, Bressner J, Houser SL, Nadkarni SK, MacNeill BD, et al. Diagnostic accuracy of optical coherence tomography and integrated backscatter intravascular ultrasound images for tissue characterization of human coronary plaques. J Am Coll Cardiol. (2006) 48:81–8. doi: 10.1016/j.jacc.2006.02.062
11. Gerbaud E, Weisz G, Tanaka A, Kashiwagi M, Shimizu T, Wang L, et al. Multi-laboratory inter-institute reproducibility study of IVOCT and IVUS assessments using published consensus document definitions. Eur Heart J Cardiovasc Imaging. (2016) 17:756–64. doi: 10.1093/ehjci/jev229
12. Kume T, Akasaka T, Kawamoto T, Watanabe N, Toyota E, Neishi Y, et al. Assessment of coronary intima-media thickness by optical coherence tomography: comparison with intravascular ultrasound. Circ J. (2005) 69:903–7. doi: 10.1253/circj.69.903
13. Kume T, Akasaka T, Kawamoto T, Okura H, Watanabe N, Toyota E, et al. Measurement of the thickness of the fibrous cap by optical coherence tomography. Am Heart J. (2006) 152:755.e1-4. doi: 10.1016/j.ahj.2006.06.030
14. Ali ZA, Maehara A, Généreux P, Shlofmitz RA, Fabbiocchi F, Nazif TM, et al. Optical coherence tomography compared with intravascular ultrasound and with angiography to guide coronary stent implantation (ILUMIEN III: OPTIMIZEPCI): a randomized controlled trial. Lancet. (2016) 388:2618–28. doi: 10.1016/S0140-6736(16)31922-5
15. Meneveau N, Souteyrand G, Motreff P, Caussin C, Amabile N, Ohlmann P, et al. Optical coherence tomography to optimize results of percutaneous coronary intervention in patients with non-ST-elevation acute coronary syndrome: results of the multicenter, randomized DOCTORS study (does optical coherence tomography optimize results of stenting). Circulation. (2016) 134:906–17. doi: 10.1161/CIRCULATIONAHA.116.024393
16. Prati F, Guagliumi G, Mintz GS, Costa M, Regar E, Akasaka T, et al. Expert review document part 2: methodology, terminology and clinical applications of optical coherence tomography for the assessment of interventional procedures. Eur Heart J. (2012) 33:2513–20. doi: 10.1093/eurheartj/ehs095
17. Maehara A, Matsumura M, Ali ZA, Mintz GS, Stone GW. IVUS-Guided versus OCT-guided coronary stent implantation: a critical appraisal. J Am Coll Cardiol Img. (2017) 10:1487–503. doi: 10.1016/j.jcmg.2017.09.008
18. Ali ZA, Karimi Galougahi K, Maehara A, Shlofmitz RA, Ben-Yehuda O, Mintz GS, et al. Intracoronary optical coherence tomography 2018: current status and future directions. J Am Coll Cardiol Intv. (2017) 10:2473–87. doi: 10.1016/j.jcin.2017.09.042
19. Kubo T, Shinke T, Okamura T, Hibi K, Nakazawa G, Morino Y, et al. Optical frequency domain imaging vs. intravascular ultrasound in percutaneous coronary intervention (OPINION trial): one-year angiographic and clinical results. Eur Heart J. (2017) 38:3139–47. doi: 10.1093/eurheartj/ehx351
20. De Bruyne B, Pijls NH, Kalesan B, Barbato E, Tonino PA, Piroth Z, et al. Fractional flow reserve-guided PCI versus medical therapy in stable coronary disease. N Engl J Med. (2012) 367:991–1001. doi: 10.1056/NEJMoa1205361
21. Maron DJ, Hochman JS, Reynolds HR, Bangalore S, O'Brien SM, Boden WE, et al. Initial invasive or conservative strategy for stable coronary disease. N Engl J Med. (2020) 382:1395–407. doi: 10.1056/NEJMoa1915922
22. Pasternak RC, Baughman KL, Fallon JT, Block PC. Scanning electron microscopy after coronary transluminal angioplasty of normal canine coronary arteries. Am J Cardiol. (1980) 45:591–8. doi: 10.1016/S0002-9149(80)80009-9
23. Otsuka F, Finn AV, Yazdani SK, Nakano M, Kolodgie FD, Virmani R. The importance of the endothelium in atherothrombosis and coronary stenting. Nat Rev Cardiol. (2012) 9:439–53. doi: 10.1038/nrcardio.2012.64
24. Janoudi A, Shamoun FE, Kalavakunta JK, Abela GS. Cholesterol crystal induced arterial inflammation and destabilization of atherosclerotic plaque. Eur Heart J. (2016) 37:1959–67. doi: 10.1093/eurheartj/ehv653
25. Kolodgie FD, Burke AP, Nakazawa G, Virmani R. Is pathologic intimal thickening the key to understanding early plaque progression in human atherosclerotic disease? Arterioscler Thromb Vasc Biol. (2007) 27:986–9. doi: 10.1161/ATVBAHA.0000258865.44774.41
26. De Maria GL, Scarsini R, Banning AP. Management of calcific coronary artery lesions: is it time to change our interventional therapeutic approach? J Am Coll Cardiol Intv. (2019) 12:1465–78. doi: 10.1016/j.jcin.2019.03.038
27. Otsuka F, Joner M, Prati F, Virmani R, Narula J. Clinical classification of plaque morphology in coronary disease. Nat Rev Cardiol. (2014) 11:379–89. doi: 10.1038/nrcardio.2014.62
28. Liu L, Gardecki JA, Nadkarni SK, Toussaint JD, Yagi Y, Bouma BE, et al. Imaging the subcellular structure of human coronary atherosclerosis using micro-optical coherence tomography. Nat Med. (2011) 17:1010–4. doi: 10.1038/nm.2409
29. Iyer JS, Batts SA, Chu KK, Sahin MI, Leung HM, Tearney GJ, et al. Micro-optical coherence tomography of the mammalian cochlea. Sci Rep. (2016) 6:33288. doi: 10.1038/srep33288
30. Chu KK, Kusek ME, Liu L, Som A, Yonker LM, Leung H, et al. Illuminating dynamic neutrophil trans-epithelial migration with micro-optical coherence tomography. Sci Rep. (2017) 8:45789. doi: 10.1038/srep45789
31. Gardecki JA, Singh K, Wu CL, Tearney GJ. Imaging the human prostate gland using 1-μm-resolution optical coherence tomography. Arch Pathol Lab Med. (2019) 143:314–8. doi: 10.5858/arpa.2018-0135-OA
32. Leung HM, Birket SE, Hyun C, Ford TN, Cui D, Solomon GM, et al. Intranasal micro-optical coherence tomography imaging for cystic fibrosis studies. Sci Transl Med. (2019) 11:eaav3505. doi: 10.1126/scitranslmed.aav3505
33. Yin B, Chu KK, Liang CP, Singh K, Reddy R, Tearney GJ. μOCT imaging using depth of focus extension by self-imaging wavefront division in a common-path fiber optic probe. Opt Express. (2016) 24:5555–64. doi: 10.1364/OE.24.005555
34. Yin B, Hyun C, Gardecki JA, Tearney GJ. Extended depth of focus for coherence-based cellular imaging. Optica. (2017) 4:959–65. doi: 10.1364/OPTICA.4.000959
35. Yin B, Piao Z, Nishimiya K, Hyun C, Gardecki JA, Mauskapf A, et al. 3D cellular-resolution imaging in arteries using few-mode interferometry. Light Sci Appl. (2019) 8:104. doi: 10.1038/s41377-019-0211-5
36. Aird WC. Phenotypic heterogeneity of the endothelium: I. Structure, function, and mechanisms. Circ Res. (2007) 100:158–73. doi: 10.1161/01.RES.0000255691.76142.4a
37. Vanhoutte PM, Shimokawa H, Feletou M, Tang EH. Endothelial dysfunction and vascular disease - a 30th anniversary update. Acta Physiol. (2017) 219:22–9. doi: 10.1111/apha.12646
38. Vergallo R, Crea F. Atherosclerotic plaque healing. N Engl J Med. (2020) 383:846–39. doi: 10.1056/NEJMra2000317
39. Farb A, Burke AP, Tang AL, Liang TY, Mannan P, Smialek J, et al. Coronary plaque erosion without rupture into a lipid core. A frequent cause of coronary thrombosis in sudden coronary death. Circulation. (1996) 93:1354–63. doi: 10.1161/01.CIR.93.7.1354
40. Nishimiya K, Yin B, Piao Z, Ryu J, Osman H, Leung HM, et al. Micro-optical coherence tomography for endothelial cell visualization in the coronary arteries. J Am Coll Cardiol Img. (2019) 12:1878–80. doi: 10.1016/j.jcmg.2019.01.021
41. Jelev L, Surchev L. A novel simple technique for en face endothelial observations using water-soluble media -'thinned-wall' preparations. J Anat. (2008) 212:192–7. doi: 10.1111/j.1469-7580.2007.00844.x
42. Malek AM, Alper SL, Izumo S. Hemodynamic shear stress and its role in atherosclerosis. JAMA. (1999) 282:2035–42. doi: 10.1001/jama.282.21.2035
43. Herrmann J, Higano ST, Lenon RJ, Rihal CS, Lerman A. Myocardial bridging is associated with alteration in coronary vasoreactivity. Eur Heart J. (2004) 25:2134 42. doi: 10.1016/j.ehj.2004.08.015
44. Jia H, Abtahian F, Aguirre AD, Lee S, Chia S, Lowe H, et al. In vivo diagnosis of plaque erosion and calcified nodule in patients with acute coronary syndrome by intravascular optical coherence tomography. J Am Coll Cardiol. (2013) 62:1748–58. doi: 10.1016/j.jacc.2013.05.071
45. Prati F, Regar E, Mintz GS, Arbustini E, Di Mario C, Jang IK, et al. Expert review document on methodology, terminology, and clinical applications of optical coherence tomography: physical principles, methodology of image acquisition, and clinical application for assessment of coronary arteries and atherosclerosis. Eur Heart J. (2010) 31:401–15. doi: 10.1093/eurheartj/ehp433
46. Tabas I. Macrophage death and defective inflammation resolution in atherosclerosis. Nat Rev Immunol. (2010) 10:36–46. doi: 10.1038/nri2675
47. Partida RA, Libby P, Crea F, Jang IK. Plaque erosion: a new in vivo diagnosis and a potential major shift in the management of patients with acute coronary syndromes. Eur Heart J. (2018) 39:2070–6. doi: 10.1093/eurheartj/ehx786
48. Yahagi K, Kolodgie FD, Otsuka F, Finn AV, Davis HR, Joner M, et al. Pathophysiology of native coronary, vein graft, and in stent atherosclerosis. Nat Rev Cardiol. (2016) 13:79–98. doi: 10.1038/nrcardio.2015.164
49. Nishimiya K, Matsumoto Y, Uzuka H, Ogata T, Hirano M, Shindo T, et al. Beneficial effects of a novel bioabsorbable polymer coating on enhanced coronary vasoconstricting responses after drug-eluting stent implantation in pigs in vivo. J Am Coll Cardiol Intv. (2016) 9:281–91. doi: 10.1016/j.jcin.2015.09.041
50. Ali ZA, Serruys PW, Kimura T, Gao R, Ellis SG, Kereiakes DJ, et al. 2-year outcomes with the absorb bioresorbable scaffold for treatment of coronary artery disease: a systematic review and meta-analysis of seven randomised trials with an individual patient data substudy. Lancet. (2017) 390:760–72. doi: 10.1016/S0140-6736(17)31470-8
51. Chinetti-Gbaguidi G, Colin S, Staels B. Macrophage subsets in atherosclerosis. Nat Rev Cardiol. (2015) 12:10–7. doi: 10.1038/nrcardio.2014.173
52. Kashiwagi M, Liu L, Chu KK, Sun CH, Tanaka A, Gardecki JA, et al. Feasibility of the assessment of cholesterol crystals in human macrophages using micro optical coherence tomography. PLoS One. (2014) 9:e102669. doi: 10.1371/journal.pone.0102669
53. Quillard T, Araújo HA, Franck G, Shvartz E, Sukhova G, Libby P. TLR2 and neutrophils potentiateendothelial stress, apoptosis and detachment: implications for superficial erosion. Eur Heart J. (2015) 36:1394–404. doi: 10.1093/eurheartj/ehv044
54. Katayama Y, Tanaka A, Taruya A, Kashiwagi M, Nishiguchi T, Ozaki Y, et al. Feasibility and clinical significance of in vivo cholesterol crystal detection using optical coherence tomography. Arterioscler Thromb Vasc Biol. (2020) 40:220–9. doi: 10.1161/ATVBAHA.119.312934
55. Ridker PM, Everett BM, Thuren T, MacFadyen JG, Chang WH, Ballantyne C, et al. Antiinflammatory therapy with canakinumab for atherosclerotic disease. N Engl J Med. (2017) 377:1119–31. doi: 10.1056/NEJMoa1707914
56. Tardif JC, Kouz S, Waters DD, Bertrand OF, Diaz R, Maggioni AP, et al. Efficacy and safety of low-dose colchicine after myocardial infarction. N Engl J Med. (2019) 381:2497–505. doi: 10.1056/NEJMoa1912388
57. Terkeltaub R. Update on gout: new therapeutic strategies and options. Nat Rev Rheumatol. (2010) 6:30–8. doi: 10.1038/nrrheum.2009.236
58. Duewell P, Kono H, Rayner KJ, Sirois CM, Vladimer G, Bauernfeind FG, et al. NLRP3 inflammasomes are required for atherogenesis and activated by cholesterol crystals. Nature. (2010) 464:1357–61. doi: 10.1038/nature08938
59. Lee SY, Im E, Hong SJ, Ahn CM, Kim JS, Kim BK, et al. Severe acute stent malapposition after drug-eluting stent implantation: effects on long-term clinical outcomes. J Am Heart Assoc. (2019) 8:e012800. doi: 10.1161/JAHA.119.012800
60. Chowdhury PS, Ramos RG. Images in clinical medicine. Coronary-stent fracture. N Engl J Med. (2002) 347:581. doi: 10.1056/NEJMicm020259
61. Rizas KD, Mehilli J. Stent polymers: Do they make a difference? Circ Cardiovasc Interv. (2016) 9:e002943. doi: 10.1161/CIRCINTERVENTIONS.115.002943
62. Chowdhury MM, Singh K, Albaghdadi MS, Khraishah H, Mauskapf A, Kessinger CW, et al. Paclitaxel drug-coated balloon angioplasty suppresses progression and inflammation of experimental atherosclerosis in rabbits. J Am Coll Cardiol Basic Trans Science. (2020) 5:685–95. doi: 10.1016/j.jacbts.2020.04.007
Keywords: optical coherence tomography, micro-OCT, endothelial cells, inflammatory cells, macrophage—cell, cholesterol crystals, necrotic core, plaque erosion
Citation: Nishimiya K and Tearney G (2021) Micro Optical Coherence Tomography for Coronary Imaging. Front. Cardiovasc. Med. 8:613400. doi: 10.3389/fcvm.2021.613400
Received: 02 October 2020; Accepted: 23 February 2021;
Published: 26 March 2021.
Edited by:
Patrick W. Serruys, Imperial College London, United KingdomReviewed by:
Antonios Karanasos, Hippokration General Hospital, GreeceCopyright © 2021 Nishimiya and Tearney. This is an open-access article distributed under the terms of the Creative Commons Attribution License (CC BY). The use, distribution or reproduction in other forums is permitted, provided the original author(s) and the copyright owner(s) are credited and that the original publication in this journal is cited, in accordance with accepted academic practice. No use, distribution or reproduction is permitted which does not comply with these terms.
*Correspondence: Guillermo Tearney, Z3RlYXJuZXlAcGFydG5lcnMub3Jn
Disclaimer: All claims expressed in this article are solely those of the authors and do not necessarily represent those of their affiliated organizations, or those of the publisher, the editors and the reviewers. Any product that may be evaluated in this article or claim that may be made by its manufacturer is not guaranteed or endorsed by the publisher.
Research integrity at Frontiers
Learn more about the work of our research integrity team to safeguard the quality of each article we publish.