- 1Department of Molecular Biomedical Sciences and Comparative Medicine Institute, North Carolina State University, Raleigh, NC, United States
- 2Joint Department of Biomedical Engineering, University of North Carolina at Chapel Hill and North Carolina State University, Raleigh, NC, United States
For the past decades, heart diseases remain the leading cause of death worldwide. In the adult mammalian heart, damaged cardiomyocytes will be replaced by non-contractile fibrotic scar tissues due to the poor regenerative ability of heart, causing heart failure subsequently. The development of tissue engineering has launched a new medical innovation for heart regeneration. As one of the most outstanding technology, cardiac patches hold the potential to restore cardiac function clinically. Consisted of two components: therapeutic ingredients and substrate scaffolds, the fabrication of cardiac patches requires both advanced bioactive molecules and biomaterials. In this review, we will present the most state-of-the-art cardiac patches and analysis their compositional details. The therapeutic ingredients will be discussed from cell sources to bioactive molecules. In the meanwhile, the recent advances to obtain scaffold biomaterials will be highlighted, including synthetic and natural materials. Also, we have focused on the challenges and potential strategies to fabricate clinically applicable cardiac patches.
Introduction
Heart disease remains a leading problem threatening millions of people worldwide (1–3). Due to the lack of regeneration ability, cardiomyocytes (CMs) in adult mammalian heart can hardly recover from ischemic injuries, like myocardial infarction (MI) (4–7). Suffered from such an irreversible cardiac muscle death, CMs will be gradually replaced by fibrotic scar tissues (8–10). The loss of the contractile capacity leads to the dysfunction of heart and causes heart failure eventually (11, 12). Thus, it is still a challenge to explore novel therapeutic methods for myocardium regeneration.
Preclinical studies have demonstrated the therapeutic performance of several approaches to treat MI, such as the injection of stem cells (13, 14), genes (15, 16) and growth factors (17). However, these therapeutics always suffer from low stability and short half-lifetime. Hence, delivery methods are highly demanded to achieve better therapeutic performance. In virtue of the engineered biomaterials, cardiac patches show promising potential in promoting cardiac function (18, 19). The component of a cardiac patch can be simply divided into two parts: substrate and therapeutic ingredients (20). With the development of fabrication technologies, more and more cardiac patches with excellent therapeutic performance have been developed (21) (Figure 1). The therapeutic ingredients for cardiac patches range from cells (such as skeletal myoblasts, mesenchymal stem cells and human pluripotent stem cells) to bioactive molecules (including growth factors, microRNA and extracellular vesicles) (22–24). A large number of biomaterials used to fabricate cardiac patches have emerged during the last decade (25–28). Whether natural or synthesized, these scaffolding materials offer a suitable environment for therapeutic ingredients (29–31). Polymers are the most used materials for cardiac patch fabrication (32, 33). Some patches are generated from in vivo sources like collagen (34), fibrin (35), decellularized ECM (36) and even cell sheets (37), providing outstanding biocompatibility compared to synthesized materials (38). In this review, both the therapeutic ingredients and biomaterials will be discussed (Table 1), but topics such as disease modeling (58), bioreactor stimulation (59), microphysiologic systems (60) will not be included. In addition, we will focus on the limitations of current cardiac patches to clinical application.
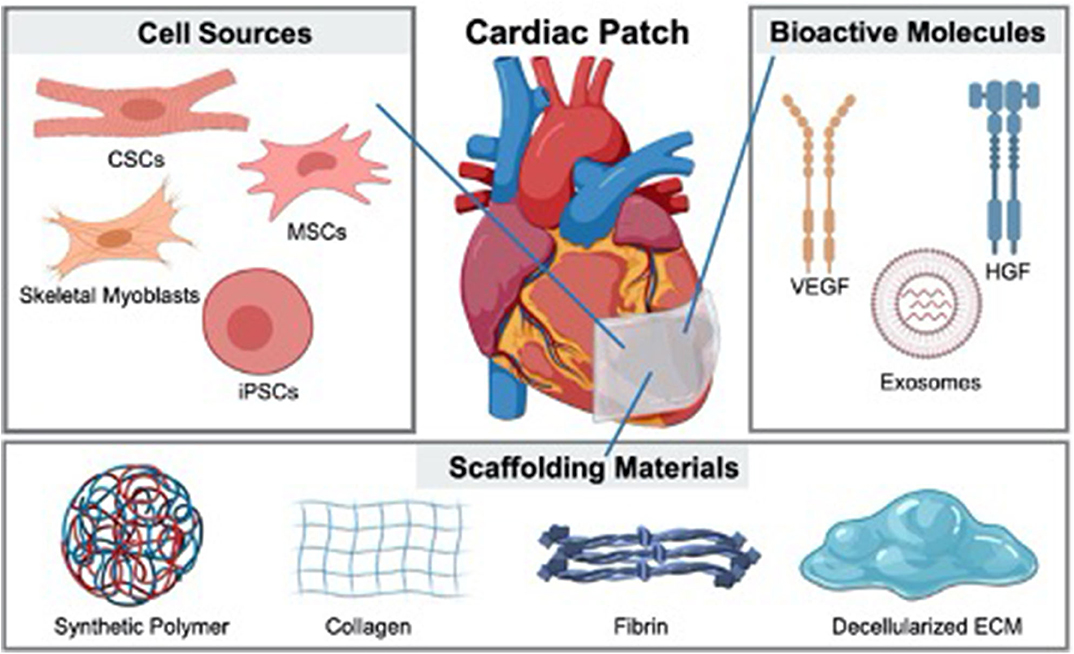
Figure 1. Cardiac patches fabricated from different types of cells and bioactive molecules with various scaffolding materials.
Cell Sources
The first type of cell reported for heart regeneration was skeletal myoblasts (61, 62). Up to now, the functional benefits of skeletal myoblasts to treat ischemic heart diseases have been proved in clinical trials (63, 64). Since then, cell therapy for cardiac regeneration has attracted wide interest (65). More and more types of cells have been studied, including cardiac stem/stromal cells (66), mesenchymal stem cells (MSCs) (67) and human pluripotent stem cells (68). To enhance the cellular retention and survival ratio, cell based cardiac patches have been developed (69–72).
Cardiac Stem/Stromal Cells
Cardiac stem/stromal cells, including cardiosphere-derived cells (CDCs) (73), stem cell antigen-1+ (Sca1+) cells (74) and lslet-1+ (Isl-1+) cells (75), have shown preclinical and clinical evidence in ischemic tissue preservation and anti-remodeling. Nowadays, the transplantation of CSCs for heart repairing has been achieved clinically, however, the adverse immunoreaction severely hampers the treatment performance (76, 77). One of the strategies to enhance the safety during CSCs transplantation is to introduce nanogel for encapsulation. In a recent work by Tang et al. (78), human CSCs (hCSCs) were encapsulated in thermosensitive P(NIPAM-AA) nanogel. It was found that with the injection of nanogel, barely systemic inflammation or local T cell infiltrations was elicited by hCSCs in immunocompetent mice. Compared to xenogeneic hCSCs injection in saline, these nanogel-encapsulated hCSCs exhibited largely reduced adverse effect, while still preserving cardiac function and reducing scar sizes in mouse and pig models. Another limitation of CSCs therapy in cardiac diseases is the low cellular retention and survival ratio (79). Cardiac patch provides an excellent platform for cell engraftment improvement. For example, a vascularized cardiac patch was recently developed, which shows promising benefits to treat ischemic heart injury (80). This patch was fabricated by encapsulating the biomimetic microvessels (BMVs) and CSCs in a fibrin gel. As tested in a rat MI model, the myocardial capillary density was improved significantly after BMV–CSC patch therapy, which can be ascribed to the paracrine factors release.
Mesenchymal Stem Cells
With the strong ability of differentiation and immunoprivileged nature, MSCs have been the most studied cell type for cardiac cell therapy in clinical trials (81, 82). MSCs can be derived from various organs and tissues, especially bone marrow and adipose tissue (83, 84). According to current researches, the therapeutic effects of MSCs are achieved through the secretion of paracrine effectors (such as growth factors) (85). To achieve better treatment, various methods have been developed to extend the therapeutic potential of current MSCs (86). Primed MSCs through genetically engineered hepatocyte growth factor–expressing MSCs (HGF-eMSCs) was developed by Park et al. (45), showing largely improved vasculogenic ability and enhanced cell viability. After encapsulated in a cardiac patch, this kind of MSCs promoted vascular regeneration and repaired cardiac function within MI hearts. Another issue for the clinical use of MSCs is their restricted retention ability after transplantation into failing hearts. Recently, a minimally invasive approach was developed by Lee et al. (87), which utilized an array of microneedles with an outer shell [poly(lactic-co-glycolic) acid] and an internal gelatin methacryloyl (GelMA)-MSC mixture (GMM). The mechanical strength and the regenerative ability were demonstrated in vitro and in vivo. Apart from bone marrow, other tissues also exhibit MSCs derived potential, including adipose, placenta, and amnion (88). Cells derived from different sources present diverse treatment performance after transplantation into MI animals (89).
Pluripotent Stem Cells
Human pluripotent stem cells, including embryonic stem cells (ESCs) and induced-pluripotent stem cells (iPSCs), have been proved to generate various types of cells in the area of regeneration (90). ESCs, derived from inner cell mass of pre-implantation blastocyst, show the potential of differentiation into cardiomyocytes (91). Compared to adult cardiomyocytes, these differentiated cardiomyocytes have similar physiological characteristics and can beat spontaneously (92). ESCs can also differentiate into other types of heart cells, such as endothelium and vascular smooth muscle (93). Moreover, these cells show excellent ability in releasing paracrine factors for heart repair, including growth factors, antifibrotic and antiapoptotic (94). Another technology to fabricate ESCs is parthenogenesis-asexual, which has successfully achieved in mice and non-human primates through chemically stimulated (95).
Similar to the chemical stimulation process in producing ESCs, the generation of iPSCs can be achieved by introducing different transcriptional regulators (such as Oct4, Sox2, Klf4 and Myc) (96). Taking the advantage of derived patients' cells, the utilization of iPSCs in treating heart diseases benefits a lot from immunological aspect (97). The clinical potential of human iPSCs (hiPSCs) derived cells encapsulated patches have been explored. By suspending three different cardiac cells (cardiomyocytes, smooth-muscle cells, and endothelial cells) derived from hiPSCs in fibrin scaffold, a clinically relevant size (4 × 2 cm × 1.25 mm) human cardiac muscle patches (hCMPs) was fabricated (48). It was found that the infarct region was significantly reduced after the transplantation of this patch. Because of the reduction in LV wall stress, cardiac function was largely restored while the arrhythmogenicity showed little change. The safety of hiPSCs in clinical trial has been confirmed, while the enhancement of therapeutic efficacy is still on the way (98, 99). A dual approach was explored in recent study (100). Cardiomyocytes derived from human induced pluripotent stem cells (hiPSC-CMs) along with a hMSCs patch were applied in the meantime in MI rat model. Since the hMSCs patch provided a microenvironment for hiPSC-CMs to survive, the cardiac function of rat was better restored then hiPSC-CMs along. Because of the ethical controversy and immunological issues, it has been reported that iPSCs takes more advantages in heart regeneration than ESCs (101). To better understand the bioactivity of iPSCs, more specific characterization like genetic programming is highly demanded.
Acellular Patch
Instead of the direct interact of cells with host cardiomyocytes, paracrine factors released by cells play a key role in heart repair (102). Among these factors, growth factors (GFs), extracellular vesicles (EVs) and microRNAs are mostly studied (103). Different from cellular patches, acellular patches integrated with paracrine factors exhibit more direct therapeutic effects (104). In addition, patches fabricated with only biomaterials also show passive restrained ability toward injured myocardium (105).
Growth Factors
As one of the paracrine effectors, the significant role of growth factors in multiple cellular processes have been determined. Accumulating evidence supports that growth factors, such as vascular endothelial growth factor (VEGF), hepatocyte growth factor (HGF) and insulin-like growth factor-1 (IGF-1), regulate the growth, survival and migration of cardiomyocytes (106). To protect growth factors (mostly proteins) during the delivery process as well as target the tissue site, growth factors encapsulated cardiac patches have attracted tremendous attention (107). Before clinical trials, the efficiency of growth factors integrated cardiac patches have been evaluated via various animal models. For example, a growth factor embedded nanofibrous patch was developed and tested in a rabbit MI model (107). With the rising death rate of cardiac diseases, the on-demand release of therapeutic factors shows significant in clinical treatment. Cardiac patches integrated with complex electronics were fabricated, allowing the on demand releasing of growth factors (31). With the electronics, this patch also performed cellular electrical activities recording ability, facilitating the electrically triggered cell contraction. Cardiac patches provide a protection for growth factors from easy elimination. Due to the pleiotropic functions of growth factors (108), local delivery through cardiac patch shows significance to avoid unexpected adverse effects.
Extracellular Vesicles
Extracellular vesicles (EVs) are miniscule vesicles with a diameter between 30 and 150 nm (109). It is highlighted that EVs play a key role in cell communication, regulating various intercellular activities (110). The certain EVs markers are still under exploration because of their complex overlapping surface properties. Based on the surface proteins, EVs can be generally divided into three types: apoptotic bodies, microvesicles, and exosomes (111).
Among all the EVs, exosomes secreted by stem cells have been studied extensively (112). Exosomes are phospholipid bilayer-enclosed vesicles with contents inside, such as proteins, mRNA and miRNA (113). Recent studies have illustrated that it is paracrine factors rather than MSCs themselves contribute to treating cardiovascular diseases (114). Moreover, lots of pre-clinical evidence confirmed the cardiac function restored ability through exosomes that extracted from MSC conditioned media, demonstrating the potential cardiac repair performance of exosomes (115). In addition to MSCs, exosomes secreted by other cell sources, including iPSCs derived CMs, hESCs derived CMs and monocyte, also help with cardiac repairing. For example, a hydrogel patch encapsulated with EVs derived from iPSCs derived CMs was developed by Liu et al. (104), which allowed continuous treatment during the whole phase of heart injury. In another work, PGSA-g-EG polymer was investigated for the fabrication of cardiac patch (116). With the loading of EVs, this adhesive cardiac patch exhibited a controlled release behavior for more than 14 days. As evidenced by the miRNA analysis, exosomes secreted by iPSCs derived CMs and hESCs derived CMs have the same functional miRNAs for heart repair (117). Because of the short half-life, current therapies utilizing exosomes lack long-term effect. Although recent studies have developed various delivery methods for exosomes (118), explorations should still focus on fabrication technology to meet clinical demands.
MicroRNAs
MicroRNAs (miR) are a kind of single-stranded RNA with a small number of nucleotides (usually 20–25) (119). Previous researches have shown that miRs play an important role in regulating downstream pathways of messenger RNA through RNA interference, indicating effective cell modification ability (120). In particular, both the survival and apoptosis of cardiomyocyte are under the control of the miR network, such as miR-24, -199a, and -590 (121). Thus, miR related therapy for myocardial infarction (MI) has been identified as a promising treatment method (24). Despite the feasibility of miR therapy, several issues need to be addressed before further practical studies. Since naked miRs are unstable during in vivo circulation, the leading challenge would be how to deliver miRs to the targeted site with enhanced retention (122).
To overcome these obstacles, several methods have been investigated, including chemical modification and carrier development (123). For example, a recent study showed that with the assistance of a tissue-engineered three-dimensional (3D) culture environment, the reprogramming efficiency of miRs from cardiac fibroblasts into functional cardiomyocytes was enhanced (124). Such an improvement was confirmed from both gene and protein levels by the assessment of cardiac differentiation markers, which provided a fundamental basis for the fabrication of cardiac patch with miRs. Given that several human miRs, such as hsa-miR-199a-3p, exhibited therapeutic potential in cardiac regeneration, a pig MI model was constructed for more clinically relevant research (15). In this 1-month study, the de-differentiation and proliferation of cardiomyocyte were observed, indicating the expression of human microRNA-199a contributed to restoring cardiac function. However, unexpected sudden arrhythmic death occurred among treated pigs, which required further careful control on the dosage. The delivery of miRs with cardiac patches is appealing for cardiac restoration, but more detailed studies are required to better understand this emerging technology.
Acellular Patch Without Biomolecules
In spite of providing a pathway for cellular or biomolecule delivery, cardiac patches fabricated with only acellular biomaterial matrices have shown potential to treat MI (125). Served as mechanical-structural supports, such cardiac patches without any external therapeutic agents exhibited passively restraining ability toward infarcted ventricle, protecting left ventricular from remodeling and dilation (126). To understand the mechanisms as well as maximize the therapeutic efficiency, a simulation-guided strategy was carried out recently (21). In this study, finite-element simulations were applied to simulate the remodeling of left ventricular, which accounts for the balanced material properties between fluid and solid. Benefit from such design, a viscoelastic adhesive patch was fabricated, which not only restrained dilatation but also restored the cardiac function after MI. To match the mechanical properties of heart tissue, technologies such as excimer laser microablation was applied for the fabrication of mechanical support cardiac patch (127). By virtue of such micropattern strategy, the patch was determined with a negative Poisson's ratio, showing priority in conforming to the mechanics of the heart. Although the design of such matrices only cardiac patches is to provide mechanical support to reduce pathological cardiac remodeling, their contributes to providing a favorable physiological environment for biomolecule delivery cannot be ignored (105).
Materials for Patch Fabrication
The exploration of materials used to fabricate cardiac patches never stops. Due to the unstable bioactivity of cells or other biological molecules, a substrate is highly needed to provide cellular microenvironment as well as enough mechanical support (128). Some key points should be taken into consideration when select suitable materials, such as biocompatibility, biodegradability and mechanical strength (129). In the meantime, materials whether synthetic or natural, must be deliberated carefully to meet the demands of clinical applicability (130), such as easy to acquire and long-term storage. In this section, biomaterials for cardiac patch fabrication will be discussed from synthetic to natural ones.
Synthetic Materials
Taking advantages of reproducible synthesis processes, synthetic materials with stable physical and mechanical properties have shown potential to meet clinical requirements (131). A lot of synthetic materials for tissue engineering have been well-studied, such as polymer poly(vinyl alcohol) (PVA), poly(lactic-co-glycolic) acid (PLGA), poly-(L-lactic) acid (PLLA) and polyurethanes (PU) (132).
With the strong mechanical properties, it is possible to make a linker between cells and host myocardium through a synthetic polymer patch (133). Currently, how to deliver secreted factors efficiently to MI region remains a challenge in cardiac stem cell therapy. Microneedle patch provides such an opportunity for drug delivery. Recently, a cardiac stromal cell-encapsulated microneedle patch (MN-CSCs) was fabricated through micromolding approach, using biocompatible PVA (39). Microneedle played a role as the channels between cells and the host myocardium, from which cells got sufficient nutrients to survive and released more paracrine factors to restore the heart function.
Due to the strong mechanical strength, acellular epicardial patches also show excellent therapeutic efficacy to help rebuilt damaged cardiac tissues. A viscoelastic adhesive patch was developed, exhibiting LV remodeling reversing ability in both acute and subacute MI rats model (21). This patch was made from ionically crosslinked transparent hydrogel with a low dynamic modulus. Through finite-element simulations, this acellular epicardial patch was designed at the “gel point,” contributing to the balance between fluid and solid properties. Without biomolecules or cells, cardiac patches made from synthetic materials have priorities than natural materials for clinical application, such as long-term storage, stable quality and easy manufacturing process.
Natural Materials
Different from synthetic materials, natural materials such as collagen, fibrin, alginate, hyaluronic acid, gelatin, and decellularized extra-cellular matrix (ECM) show superior biocompatibility (134). Whether derived from in vivo sources or naturally occurring, these materials retain the structure to mimic originally cellular microenvironment. Notably, materials generated from biological sources offer extra protection for therapeutics to escape from immune inflammation, allowing improved therapeutic function (135).
Collagen is the most widely used natural material for cardiac patch fabrication, which mainly exists in cardiac ECM. Due to the minimal antigenicity and chemotactic properties, collagen can provide a tissue-like environment for cells (136). The latest technology to fabricate collagen patches including electrospinning, which requires electrically charging. Different cells can be seeded into electrospun nanofibrous collagen scaffolds, such as iPSCs and MSCs (39). Benefit from paracrine signaling and force transmission, collagen scaffold patches show potential therapeutic performance in treating both MI and dilated cardiomyopathy (DCM). The development of conductive collagen cardiac patch has become a tendency. With the addition of conductive components, such as carbon nanotubes, metal nanoparticles and graphene oxide, the online monitoring of tissue statues can be achieved (127). Other in vivo source natural materials, like fibrin, HA and alginate, also exhibit therapeutic potential for clinical studies.
Currently, the most studied biomaterials for cardiac patch fabrication is decellularized ECM (137). Either derived from cardiac sources (such as myocardium and pericardium) or non-cardiac sources (small intestinal submucosa and urinary bladder matrix), decellularized ECM provides an ideal environment to support cardiac restore processes (138). In addition, recent studies also demonstrated the vital roles of decellularized ECM in cardiac repair. Inspired by the excellent regeneration ability of neonatal mouse heart, researchers found that one of the proteins in neonatal extracellular matrix named agrin, played a key role in promoting heart regeneration (139). This kind of protein helped with the disassembly of the dystrophin–glycoprotein complex, thus promoted the division of cardiomyocytes in vitro. For cardiac stem therapy with patches, the difficulty in cell viability retaining becomes the limitation for clinical trials. To solve this problem, a strategy using synthetic cardiac stromal cells (synCSCs) was generated to fabricate an off-the-shelf therapeutic cardiac patch (40). Through the encapsulating of synCSCs on to the decellularized ECM, the potency of this fully acellular artificial cardiac patch (artCP) was well-maintained for a long time. The cardiac repair function of the artCP was confirmed in a rat MI model. Furthermore, the clinical potential of this artCP was revealed in a porcine model with MI.
The strong physical properties of decellularized ECM allow the application of novel technologies during fabrication process, such as 3D Printing. For cardiac tissue engineering, immunological problem remains an unmet gap for patients. Recently, personalized bioink for 3D printing was developed from ECM hydrogel (47). Since this ECM was generated from the patient's personalized omental tissue, the biocompatibility of this bioink was guaranteed. With the addition of two different types of cells, cardiac patch with enhanced vascularization ability was printed. Moreover, cellularized hearts with a natural blood vessel architecture was printed via this kind of bioink. Such technology extended the further potential of ECM for tissue engineering in personalized therapy.
Scaffold-Less Cardiac Patches
Patches made from scaffold materials have shown priority in improving cell engraftment, however, problems remain in the cause of undesirable arrhythmogenicity and immune rejection toward xenogeneic materials (140). To avoid these drawbacks, the strategy to develop scaffold-less cardiac patches has been developed. As one of the most promising scaffold-less cardiac patches, cell sheets have demonstrated some advantages, including a more biomimetic microenvironment and better cell-cell communication (37). Currently, the fabrication of cell sheets involves a specially coated cell culture dish, which is covered with a temperature-responsive polymer named poly-N-isopropylacrylamide (141). When temperature changes from 37 to 20°C, the surface of this polymer will turn from hydrophobic to hydrophilic because of the conformation transformation. Cells only adhere to the hydrophobic surface and detach from the hydrophilic surface. During the fabrication process, cells are firstly cultured under 37°C until the formation of a confluent film. Afterwards, cells are placed at 20°C until detached spontaneously. Eventually, the cell sheets can be obtained in the upper layer of the media.
The first in-man study of cell sheets was reported in 2012 (142), in which a male with idiopathic dilated cardiomyopathy received the transplantation of cell sheets made from his skeletal myoblasts. Showing the treatment efficiency and safety, cell sheets made from different cell types have been reported, like myoblasts, cardiomyocytes and stem cells. Recent studies have demonstrated the advantages of adipose-derived stem cells (ASCs) in heart regeneration (143). The transplantation of ASCs sheet was developed, which showed restoration ability toward the injured heart. It has been proved that the enhanced secretion of VEGF induced by norepinephrine was the functional process for ASCs sheet therapy. In addition to the secretion of paracrine factors, heart therapy via cell sheets also benefits from the prevention of arrhythmogenicity. It was demonstrated for the first time by Suzuki et al., that skeletal myoblasts (SMBs) sheets not only restored heart function but also prevented ventricular arrhythmias by maintaining the regular electrical conductance (144). To better understand the therapeutic effects of cell sheets, the mechanism studies remain urgent. In addition, it is necessary to seek novel fabrication technology to meet the complicated requirement of clinical trials.
Challenges and Further Direction
Although cardiac patches have shown promising performance in pre-clinical studies in cardiac repair, problems still remain before their clinical implementation (145). Therapeutic integrates benefit a lot from cardiac patches with enhanced delivery efficiency, however, the implementation of most cardiac patches requires open-chest surgery. As known to all, patients with MI may be not strong enough to recover from the surgical caused damage and inflammation, which brings panic to patients with psychological anxiety (146). Thus, minimally invasive delivery of cardiac patches is highly needed. Not only the implantation method should be improved, novel fabrication technology as well as materials should be the strong back support. More precious technologies, such as 3D printing and photoetching, are highly recommended for patch fabrication (147). Materials with shape memory ability and stronger mechanical property would be the next generation of biomaterials for cardiac patches.
Although it has been confirmed in animal models, the biocompatibility of current cardiac patches is still far below satisfaction and unable to meet the clinical requirement (148). On the one hand, the integration ability of patches with host myocardium is important in enhancing therapeutic efficiency, like improving cardiomyogenesis and angiogenesis at injured heart. On the other hand, it should be highly addressed that the tissue adhesion after cardiac patch transplantation appears commonly, leading to severe side effects (149). It is an immune response since patches are still foreign constituents. This would be the vital concern before cardiac patches can be applied clinically. Currently, researchers have discovered that surface modification will largely reduce the tissue adhesion (150), which may be good candidates for polymer cardiac patches. The biodegradation should also be taken into consideration, as immune rejection will last unless the patches can be degraded after treatment (151). Such improvement requires the biodegradable of materials themselves, while having little impact on therapeutic period.
In addition, long-term storage is necessary for clinical application. Because of the special requirements for cell culture, current cell therapy with cardiac patches are time-consuming and mostly can only be achieved in laboratory (152). How to retain the viability and functionality of cells remains a block for the large-scale production of such therapeutic patches. Although various studies have found that cell retention and engraftment are enhanced to some extent with cardiac patches (153), the therapeutic efficiency is still far below clinical requirement. Artificial materials have a similar function to cells that are highly needed, which requires more mechanism studies of cell therapy for heart regeneration. In conclusion, the development of cardiac patches paves the way for cardiac repair and gives inspiration for heart regeneration.
Author Contributions
XM wrote the text of this review paper with guidance from KC. Both authors have reviewed the final version and approve of the content in this manuscript.
Funding
This work was supported by grants from the National Institutes of Health (R01 HL123920, HL137093, HL144002, HL146153, and HL147357 to KC) and the American Heart Association (18TPA34230092 and 19EIA34660286 to KC).
Conflict of Interest
The authors declare that the research was conducted in the absence of any commercial or financial relationships that could be construed as a potential conflict of interest.
References
1. Mahmoudi M, Yu M, Serpooshan V, Wu JC, Langer R, Lee RT, et al. Multiscale technologies for treatment of ischemic cardiomyopathy. Nat Nanotechnol. (2017) 12:845–55. doi: 10.1038/nnano.2017.167
2. Molero-Díez YB, Sánchez-Tabernero Á, Ruiz-Simón FA, Sanchis-Dux R. Fourth universal definition of myocardial infarction: perioperative anesthetic considerations. Colomb J Anesthesiol. (2019) 47:175–7. doi: 10.1097/CJ9.0000000000000120
3. Thygesen K, Alpert JS, Jaffe AS, Simoons ML, Chaitman BR, White HD, et al. Third universal definition of myocardial infarction. Eur Heart J. (2012) 33:2551–67. doi: 10.1016/j.jacc.2012.08.001
4. Tardif JC Kouz S Waters DD Bertrand OF Diaz R Maggioni AP . Efficacy and safety of low-dose colchicine after myocardial infarction. N Engl J Med. (2019) 381:2497–505. doi: 10.1056/NEJMoa1912388
5. Agewall S, Beltrame JF, Reynolds HR, Niessner A, Rosano G, Caforio ALP, et al. ESC working group position paper on myocardial infarction with non-obstructive coronary arteries. Eur Heart J. (2017) 38:143–53. doi: 10.1093/eurheartj/ehw149
6. Lellouche F, Simon M, L'Her E. Oxygen therapy in suspected acute myocardial infarction. N Engl J Med. (2018) 378:201. doi: 10.1056/NEJMc1714937
7. Chapman AR, Shah ASV, Lee KK, Anand A, Francis O, Adamson P, et al. Long-term outcomes in patients with type 2 myocardial infarction and myocardial injury. Circulation. (2018) 137:1236–45. doi: 10.1161/CIRCULATIONAHA.117.031806
8. Parikh SS, Blackwell DJ, Gomez-Hurtado N, Frisk M, Wang L, Kim K, et al. Thyroid and glucocorticoid hormones promote functional T-tubule development in human-induced pluripotent stem cell-derived cardiomyocytes. Circ Res. (2017) 121:1323–30. doi: 10.1161/CIRCRESAHA.117.311920
9. Mattera R, Benvenuto M, Giganti MG, Tresoldi I, Pluchinotta FR, Bergante S, et al. Effects of polyphenols on oxidative stress-mediated injury in cardiomyocytes. Nutrients. (2017) 9:523. doi: 10.3390/nu9050523
10. Jones PP, MacQuaide N, Louch WE. Dyadic plasticity in cardiomyocytes. Front Physiol. (2018) 9:1773. doi: 10.3389/fphys.2018.01773
11. Rikhtegar R, Pezeshkian M, Dolati S, Safaie N, Afrasiabi Rad A, Mahdipour M, et al. Stem cells as therapy for heart disease: iPSCs, ESCs, CSCs, and skeletal myoblasts. Biomed Pharmacother. (2019) 109:304–13. doi: 10.1016/j.biopha.2018.10.065
12. Robison P, Caporizzo MA, Ahmadzadeh H, Bogush AI, Chen CY, Margulies KB, et al. Detyrosinated microtubules buckle and bear load in contracting cardiomyocytes. Science. (2016) 352:aaf0659. doi: 10.1126/science.aaf0659
13. Qiao L, Hu S, Liu S, Zhang H, Ma H, Huang K, et al. MicroRNA-21-5p dysregulation in exosomes derived from heart failure patients impairs regenerative potential. J Clin Invest. (2019) 129:2237–50. doi: 10.1172/JCI123135
14. Kimbrel EA, Lanza R. Next-generation stem cells-ushering in a new era of cell-based therapies. Nat Rev Drug Discov. (2020) 19:463–79. doi: 10.1038/s41573-020-0064-x
15. Gabisonia K, Prosdocimo G, Aquaro GD, Carlucci L, Zentilin L, Secco I, et al. MicroRNA therapy stimulates uncontrolled cardiac repair after myocardial infarction in pigs. Nature. (2019) 569:418–22. doi: 10.1038/s41586-019-1191-6
16. Scimia MC, Gumpert AM, Koch WJ. Cardiovascular gene therapy for myocardial infarction. Expert Opin Biol Ther. (2014) 14:183–95. doi: 10.1517/14712598.2014.866085
17. Torella D, Rota M, Nurzynska D, Musso E, Monsen A, Shiraishi I, et al. Cardiac stem cell and myocyte aging, heart failure, and insulin-like growth factor-1 overexpression. Circ Res. (2004) 94:514–24. doi: 10.1161/01.RES.0000117306.10142.50
18. Boopathy A V., Mandal A, Kulp DW, Menis S, Bennett NR, Watkins HC, et al. Enhancing humoral immunity via sustained-release implantable microneedle patch vaccination. Proc Natl Acad Sci USA. (2019) 116:16473–8. doi: 10.1073/pnas.1902179116
19. Chen H, Ma X, Wu S, Tian H. A rapidly self-healing supramolecular polymer hydrogel with photostimulated room-temperature phosphorescence responsiveness. Angew Chemie Int Ed. (2014) 53:14149–52. doi: 10.1002/anie.201407402
20. Yang SY, O'Cearbhaill ED, Sisk GC, Park KM, Cho WK, Villiger M, et al. A bio-inspired swellable microneedle adhesive for mechanical interlocking with tissue. Nat Commun. (2013) 4:1–10. doi: 10.1038/ncomms2715
21. Lin X, Liu Y, Bai A, Cai H, Bai Y, Jiang W, et al. A viscoelastic adhesive epicardial patch for treating myocardial infarction. Nat Biomed Eng. (2019) 3:632–43. doi: 10.1038/s41551-019-0380-9
22. Mirvakili SM, Sim D, Hunter IW, Langer R. Actuation of untethered pneumatic artificial muscles and soft robots using magnetically induced liquid-to-gas phase transitions. Sci Robot. (2020) 5:eaaz4239. doi: 10.1126/scirobotics.aaz4239
23. Squillaro T, Peluso G, Galderisi U. Clinical trials with mesenchymal stem cells: an update. Cell Transplant. (2016) 25:829–48. doi: 10.3727/096368915X689622
24. Wang LL, Liu Y, Chung JJ, Wang T, Gaffey AC, Lu M, et al. Sustained miRNA delivery from an injectable hydrogel promotes cardiomyocyte proliferation and functional regeneration after ischaemic injury. Nat Biomed Eng. (2017) 1:983–92. doi: 10.1038/s41551-017-0157-y
25. Bejleri D, Streeter B, Nachlas A, Brown M, Gaetani R, Christman K, et al. A bioprinted cardiac patch composed of cardiac-specific extracellular matrix and progenitor cells for heart repair. Adv Healthcare Mater. (2018) 7:1800672. doi: 10.1002/adhm.201800672
26. Izadifar M, Chapman D, Babyn P, Chen X, Kelly ME. UV-assisted 3D bioprinting of nanoreinforced hybrid cardiac patch for myocardial tissue engineering. Tissue Eng Part C Methods. (2018) 24:74–88. doi: 10.1089/ten.tec.2017.0346
27. Fung E, Järvelin MR, Doshi RN, Shinbane JS, Carlson SK, Grazette LP, et al. Electrocardiographic patch devices and contemporary wireless cardiac monitoring. Front Physiol. (2015) 6:149. doi: 10.3389/fphys.2015.00149
28. Gaetani R, Feyen DAM, Verhage V, Slaats R, Messina E, Christman KL, et al. Epicardial application of cardiac progenitor cells in a 3D-printed gelatin/hyaluronic acid patch preserves cardiac function after myocardial infarction. Biomaterials. (2015) 61:339–48. doi: 10.1016/j.biomaterials.2015.05.005
29. Khan M, Xu Y, Hua S, Johnson J, Belevych A, Janssen PML, et al. Erratum: evaluation of changes in morphology and function of human induced pluripotent stem cell derived cardiomyocytes (HiPSC-CMs) cultured on an aligned-nanofiber cardiac patch. PLoS ONE. (2015) 10:e0126338. doi: 10.1371/journal.pone.0126338
30. Wendel JS, Ye L, Tao R, Zhang J, Zhang J, Kamp TJ, et al. Functional effects of a tissue-engineered cardiac patch from human induced pluripotent stem cell-derived cardiomyocytes in a rat infarct model. Stem Cells Transl Med. (2015) 4:1324–32. doi: 10.5966/sctm.2015-0044
31. Feiner R, Engel L, Fleischer S, Malki M, Gal I, Shapira A, et al. Engineered hybrid cardiac patches with multifunctional electronics for online monitoring and regulation of tissue function. Nat Mater. (2016) 15:679–85. doi: 10.1038/nmat4590
32. Parameswaran R, Koehler K, Rotenberg MY, Burke MJ, Kim J, Jeong KY, et al. Optical stimulation of cardiac cells with a polymer-supported silicon nanowire matrix. Proc Natl Acad Sci USA. (2019) 116:413–21. doi: 10.1073/pnas.1816428115
33. Dvir T, Timko BP, Brigham MD, Naik SR, Karajanagi SS, Levy O, et al. Nanowired three-dimensional cardiac patches. Nat Nanotechnol. (2011) 6:720–5. doi: 10.1038/nnano.2011.160
34. Querejeta R, López B, González A, Sánchez E, Larman M, Martínez Ubago JL, et al. Increased collagen type I synthesis in patients with heart failure of hypertensive origin: relation to myocardial fibrosis. Circulation. (2004) 110:1263–8. doi: 10.1161/01.CIR.0000140973.60992.9A
35. Roura S, Soler-Botija C, Bagó JR, Llucià-Valldeperas A, Férnandez MA, Gálvez-Montón C, et al. Postinfarction functional recovery driven by a three-dimensional engineered fibrin patch composed of human umbilical cord blood-derived mesenchymal stem cells. Stem Cells Transl Med. (2015) 4:956–66. doi: 10.5966/sctm.2014-0259
36. Das S, Jang J. 3D bioprinting and decellularized ECM-based biomaterials for in vitro CV tissue engineering. J 3D Print Med. (2018) 2:69–87. doi: 10.2217/3dp-2018-0002
37. Sekine H, Shimizu T, Yang J, Kobayashi E, Okano T. Pulsatile myocardial tubes fabricated with cell sheet engineering. Circulation. (2006) 114:I87–93. doi: 10.1161/CIRCULATIONAHA.105.000273
38. da Silva D, Kaduri M, Poley M, Adir O, Krinsky N, Shainsky-Roitman J, et al. Biocompatibility, biodegradation and excretion of polylactic acid (PLA) in medical implants and theranostic systems. Chem Eng J. (2018) 340:9–14. doi: 10.1016/j.cej.2018.01.010
39. Tang J, Wang J, Huang K, Ye Y, Su T, Qiao L, et al. Cardiac cell–integrated microneedle patch for treating myocardial infarction. Sci Adv. (2018) 4:eaat9365. doi: 10.1126/sciadv.aat9365
40. Huang K, Ozpinar EW, Su T, Tang J, Shen D, Qiao L, et al. An off-the-shelf artificial cardiac patch improves cardiac repair after myocardial infarction in rats and pigs. Sci Transl Med. (2020) 12:eaat9683. doi: 10.1126/scitranslmed.aat9683
41. Dong Y, Hong M, Dai R, Wu H, Zhu P. Engineered bioactive nanoparticles incorporated biofunctionalized ECM/silk proteins based cardiac patches combined with MSCs for the repair of myocardial infarction: in vitro and in vivo evaluations. Sci Total Environ. (2020) 707:135976. doi: 10.1016/j.scitotenv.2019.135976
42. Kang K, Chuai J bo, Xie B dong, Li J zhong, Qu H, Wu H, et al. Mesenchymal stromal cells from patients with cyanotic congenital heart disease are optimal candidate for cardiac tissue engineering. Biomaterials. (2020) 230:119574. doi: 10.1016/j.biomaterials.2019.119574
43. Olvera D, Sohrabi Molina M, Hendy G, Monaghan MG. Electroconductive melt electrowritten patches matching the mechanical anisotropy of human myocardium. Adv Funct Mater. (2020) 30:1909880. doi: 10.1002/adfm.201909880
44. Kobayashi K, Ichihara Y, Sato N, Umeda N, Fields L, Fukumitsu M, et al. On-site fabrication of Bi-layered adhesive mesenchymal stromal cell-dressings for the treatment of heart failure. Biomaterials. (2019) 209:41–53. doi: 10.1016/j.biomaterials.2019.04.014
45. Park BW, Jung SH, Das S, Lee SM, Park JH, Kim H, et al. In vivo priming of human mesenchymal stem cells with hepatocyte growth factor–engineered mesenchymal stem cells promotes therapeutic potential for cardiac repair. Sci Adv. (2020) 6:eaay6994. doi: 10.1126/sciadv.aay6994
46. Melhem MR, Park J, Knapp L, Reinkensmeyer L, Cvetkovic C, Flewellyn J, et al. 3D printed stem-cell-laden, microchanneled hydrogel patch for the enhanced release of cell-secreting factors and treatment of myocardial infarctions. ACS Biomater Sci Eng. (2017) 3:1980–7. doi: 10.1021/acsbiomaterials.6b00176
47. Noor N, Shapira A, Edri R, Gal I, Wertheim L, Dvir T. 3D printing of personalized thick and perfusable cardiac patches and hearts. Adv Sci. (2019) 6:1900344. doi: 10.1002/advs.201900344
48. Gao L, Gregorich ZR, Zhu W, Mattapally S, Oduk Y, Lou X, et al. Large cardiac muscle patches engineered from human induced-pluripotent stem cell-derived cardiac cells improve recovery from myocardial infarction in swine. Circulation. (2018) 137:1712–30. doi: 10.1161/CIRCULATIONAHA.117.030785
49. Yeung E, Fukunishi T, Bai Y, Bedja D, Pitaktong I, Mattson G, et al. Cardiac regeneration using human-induced pluripotent stem cell-derived biomaterial-free 3D-bioprinted cardiac patch in vivo. J Tissue Eng Regen Med. (2019) 13:2031–9. doi: 10.1002/term.2954
50. Schaefer JA, Guzman PA, Riemenschneider SB, Kamp TJ, Tranquillo RT. A cardiac patch from aligned microvessel and cardiomyocyte patches. J Tissue Eng Regen Med. (2018) 12:546–56. doi: 10.1002/term.2568
51. Kaiser NJ, Kant RJ, Minor AJ, Coulombe KLK. Optimizing blended collagen-fibrin hydrogels for cardiac tissue engineering with human iPSC-derived cardiomyocytes. ACS Biomater Sci Eng. (2019) 5:887–99. doi: 10.1021/acsbiomaterials.8b01112
52. Yang B, Lui C, Yeung E, Matsushita H, Jeyaram A, Pitaktong I, et al. A net mold-based method of biomaterial-free three-dimensional cardiac tissue creation. Tissue Eng Part C Methods. (2019) 25:243–52. doi: 10.1089/ten.tec.2019.0003
53. Stevens KR, Pabon L, Muskheli V, Murry CE. Scaffold-free human cardiac tissue patch created from embryonic stem cells. Tissue Eng Part A. (2009) 15:1211–22. doi: 10.1089/ten.tea.2008.0151
54. Chen Q-Z, Ishii H, Thouas GA, Lyon AR, Wright JS, Blaker JJ, et al. An elastomeric patch derived from poly (glycerol sebacate) for delivery of embryonic stem cells to the heart. Biomaterials. (2010) 31:3885–93. doi: 10.1016/j.biomaterials.2010.01.108
55. O'Neill HS, O'Sullivan J, Porteous N, Ruiz-Hernandez E, Kelly HM, O'Brien FJ, et al. A collagen cardiac patch incorporating alginate microparticles permits the controlled release of hepatocyte growth factor and insulin-like growth factor-1 to enhance cardiac stem cell migration and proliferation. J Tissue Eng Regen Med. (2018) 12:e384–94. doi: 10.1002/term.2392
56. Han C, Zhou J, Liang C, Liu B, Pan X, Zhang Y, et al. Human umbilical cord mesenchymal stem cell derived exosomes encapsulated in functional peptide hydrogels promote cardiac repair. Biomater Sci. (2019) 7:2920–33. doi: 10.1039/C9BM00101H
57. Han C, Zhou J, Liu B, Liang C, Pan X, Zhang Y, et al. Delivery of miR-675 by stem cell-derived exosomes encapsulated in silk fibroin hydrogel prevents aging-induced vascular dysfunction in mouse hindlimb. Mater Sci Eng C. (2019) 99:322–32. doi: 10.1016/j.msec.2019.01.122
58. Niederer SA, Lumens J, Trayanova NA. Computational models in cardiology. Nat Rev Cardiol. (2019) 16:100–11. doi: 10.1038/s41569-018-0104-y
59. Tsimbouri PM, Childs PG, Pemberton GD, Yang J, Jayawarna V, Orapiriyakul W, et al. Stimulation of 3D osteogenesis by mesenchymal stem cells using a nanovibrational bioreactor. Nat Biomed Eng. (2017) 1:758–70. doi: 10.1038/s41551-017-0127-4
60. Mathur A, Loskill P, Shao K, Huebsch N, Hong S, Marcus SG, et al. Human iPSC-based cardiac microphysiological system for drug screening applications. Sci Rep. (2015) 5:8883. doi: 10.1038/srep08883
61. Murray IR, Baily JE, Chen WCW, Dar A, Gonzalez ZN, Jensen AR, et al. Skeletal and cardiac muscle pericytes: functions and therapeutic potential. Pharmacol Ther. (2017) 171:65–74. doi: 10.1016/j.pharmthera.2016.09.005
62. Juhas M, Abutaleb N, Wang JT, Ye J, Shaikh Z, Sriworarat C, et al. Incorporation of macrophages into engineered skeletal muscle enables enhanced muscle regeneration. Nat Biomed Eng. (2018) 2:942–54. doi: 10.1038/s41551-018-0290-2
63. Hagège AA, Marolleau JP, Vilquin JT, Alhéritière A, Peyrard S, Duboc D, et al. Skeletal myoblast transplantation in ischemic heart failure: long-term follow-up of the first phase I cohort of patients. Circulation. (2006) 114:I108–13. doi: 10.1161/CIRCULATIONAHA.105.000521
64. Smits PC, Van Geuns RJM, Poldermans D, Bountioukos M, Onderwater EEM, Lee CH, et al. Catheter-based intramyocardial injection of autologous skeletal myoblasts as a primary treatment of ischemic heart failure clinical experience with six-month follow-up. J Am Coll Cardiol. (2003) 42:2063–9. doi: 10.1016/j.jacc.2003.06.017
65. Shen D, Cheng K, Marbán E. Dose-dependent functional benefit of human cardiosphere transplantation in mice with acute myocardial infarction. J Cell Mol Med. (2012) 16:2112–6. doi: 10.1111/j.1582-4934.2011.01512.x
66. Giacomelli E, Meraviglia V, Campostrini G, Cochrane A, Cao X, van Helden RWJ, et al. Human-iPSC-derived cardiac stromal cells enhance maturation in 3D cardiac microtissues and reveal non-cardiomyocyte contributions to heart disease. Cell Stem Cell. (2020) 26:862–879.e11. doi: 10.1016/j.stem.2020.05.004
67. Majumdar MK, Thiede MA, Mosca JD, Moorman M, Gerson SL. Phenotypic and functional comparison of cultures of marrow-derived mesenchymal stem cells (MSCs) and stromal cells. J Cell Physiol. (1998) 176:57–66. doi: 10.1002/(SICI)1097-4652(199807)176:1<57::AID-JCP7>3.0.CO;2-7
68. Mei Y, Saha K, Bogatyrev SR, Yang J, Hook AL, Kalcioglu ZI, et al. Combinatorial development of biomaterials for clonal growth of human pluripotent stem cells. Nat Mater. (2010) 9:768–78. doi: 10.1038/nmat2812
69. Zhang G, Wang X, Wang Z, Zhang J, Suggs L. A PEGylated fibrin patch for mesenchymal stem cell delivery. Tissue Eng. (2006) 12:9–19. doi: 10.1089/ten.2006.12.9
70. Liau B, Christoforou N, Leong KW, Bursac N. Pluripotent stem cell-derived cardiac tissue patch with advanced structure and function. Biomaterials. (2011) 32:9180–7. doi: 10.1016/j.biomaterials.2011.08.050
71. Da Cruz L, Fynes K, Georgiadis O, Kerby J, Luo YH, Ahmado A, et al. Phase 1 clinical study of an embryonic stem cell-derived retinal pigment epithelium patch in age-related macular degeneration. Nat Biotechnol. (2018) 36:1–10. doi: 10.1038/nbt.4114
72. Braunwald E. Cell-based therapy in cardiac regeneration: an overview. Circ Res. (2018) 123:132–7. doi: 10.1161/CIRCRESAHA.118.313484
73. Smith RR, Barile L, Cho HC, Leppo MK, Hare JM, Messina E, et al. Regenerative potential of cardiosphere-derived cells expanded from percutaneous endomyocardial biopsy specimens. Circulation. (2007) 115:896–908. doi: 10.1161/CIRCULATIONAHA.106.655209
74. Noseda M, Harada M, McSweeney S, Leja T, Belian E, Stuckey DJ, et al. PDGFRα demarcates the cardiogenic clonogenic Sca1+ stem/progenitor cell in adult murine myocardium. Nat Commun. (2015) 6:1–16. doi: 10.1038/ncomms7930
75. Eberhardt M, Salmon P, von Mach MA, Hengstler JG, Brulport M, Linscheid P, et al. Multipotential nestin and Isl-1 positive mesenchymal stem cells isolated from human pancreatic islets. Biochem Biophys Res Commun. (2006) 345:1167–76. doi: 10.1016/j.bbrc.2006.05.016
76. Herberts CA, Kwa MSG, Hermsen HPH. Risk factors in the development of stem cell therapy. J Transl Med. (2011) 9:29. doi: 10.1186/1479-5876-9-29
77. Tang J, Cores J, Huang K, Cui X, Luo L, Zhang J, et al. Concise review: is cardiac cell therapy dead? embarrassing trial outcomes and new directions for the future. Stem Cells Transl Med. (2018) 7:354–9. doi: 10.1002/sctm.17-0196
78. Tang J, Cui X, Caranasos TG, Hensley MT, Vandergriff AC, Hartanto Y, et al. Heart repair using nanogel-encapsulated human cardiac stem cells in mice and pigs with myocardial infarction. ACS Nano. (2017) 11:9738–49. doi: 10.1021/acsnano.7b01008
79. Mayfield AE, Tilokee EL, Latham N, McNeill B, Lam BK, Ruel M, et al. The effect of encapsulation of cardiac stem cells within matrix-enriched hydrogel capsules on cell survival, post-ischemic cell retention and cardiac function. Biomaterials. (2014) 35:133–42. doi: 10.1016/j.biomaterials.2013.09.085
80. Su T, Huang K, Daniele MA, Hensley MT, Young AT, Tang J, et al. Cardiac stem cell patch integrated with microengineered blood vessels promotes cardiomyocyte proliferation and neovascularization after acute myocardial infarction. ACS Appl Mater Interfaces. (2018) 10:33088–96. doi: 10.1021/acsami.8b13571
81. Ankrum JA, Ong JF, Karp JM. Mesenchymal stem cells: immune evasive, not immune privileged. Nat Biotechnol. (2014) 32:252–60. doi: 10.1038/nbt.2816
82. Salem HK, Thiemermann C. Mesenchymal stromal cells: current understanding and clinical status. Stem Cells. (2010) 28:585–96. doi: 10.1002/stem.269
83. Lee RH Kim BC Choi IS Kim H Choi HS Suh KT . Characterization and expression analysis of mesenchymal stem cells from human bone marrow and adipose tissue. Cell Physiol Biochem. (2004) 14:311–24. doi: 10.1159/000080341
84. Xu L, Liu Y, Sun Y, Wang B, Xiong Y, Lin W, et al. Tissue source determines the differentiation potentials of mesenchymal stem cells: a comparative study of human mesenchymal stem cells from bone marrow and adipose tissue. Stem Cell Res Ther. (2017) 8:275. doi: 10.1186/s13287-017-0716-x
85. Anderson JD, Johansson HJ, Graham CS, Vesterlund M, Pham MT, Bramlett CS, et al. Comprehensive proteomic analysis of mesenchymal stem cell exosomes reveals modulation of angiogenesis via nuclear factor-kappaB signaling. Stem Cells. (2016) 34:601–13. doi: 10.1002/stem.2298
86. Liang H, Huang K, Su T, Li Z, Hu S, Dinh P-U, et al. Mesenchymal stem cell/red blood cell-inspired nanoparticle therapy in mice with carbon tetrachloride-induced acute liver failure. ACS Nano. (2018) 12:6536–44. doi: 10.1021/acsnano.8b00553
87. Lee KJ, Xue Y, Lee J, Kim HJ, Liu Y, Tebon P, et al. A patch of detachable hybrid microneedle depot for localized delivery of mesenchymal stem cells in regeneration therapy. Adv Funct Mater. (2020) 30:2000086. doi: 10.1002/adfm.202000086
88. Lee P-H, Tu C-T, Hsiao C-C, Tsai M-S, Ho C-M, Cheng N-C, et al. Antifibrotic activity of human placental amnion membrane-derived CD34+ mesenchymal stem/progenitor cell transplantation in mice with thioacetamide-induced liver injury. Stem Cells Transl Med. (2016) 5:1473–84. doi: 10.5966/sctm.2015-0343
89. Araújo AB, Salton GD, Furlan JM, Schneider N, Angeli MH, Laureano ÁM, et al. Comparison of human mesenchymal stromal cells from four neonatal tissues: amniotic membrane, chorionic membrane, placental decidua and umbilical cord. Cytotherapy. (2017) 19:577–85. doi: 10.1016/j.jcyt.2017.03.001
90. Spence JR, Mayhew CN, Rankin SA, Kuhar MF, Vallance JE, Tolle K, et al. Directed differentiation of human pluripotent stem cells into intestinal tissue in vitro. Nature. (2011) 470:105–10. doi: 10.1038/nature09691
91. Kehat I, Khimovich L, Caspi O, Gepstein A, Shofti R, Arbel G, et al. Electromechanical integration of cardiomyocytes derived from human embryonic stem cells. Nat Biotechnol. (2004) 22:1282–9. doi: 10.1038/nbt1014
92. Kehat I, Kenyagin-Karsenti D, Snir M, Segev H, Amit M, Gepstein A, et al. Human embryonic stem cells can differentiate into myocytes with structural and functional properties of cardiomyocytes. J Clin Invest. (2001) 108:407–14. doi: 10.1172/JCI200112131
93. Patsch C, Challet-Meylan L, Thoma EC, Urich E, Heckel T, O'Sullivan JF, et al. Generation of vascular endothelial and smooth muscle cells from human pluripotent stem cells. Nat Cell Biol. (2015) 17:994–1003. doi: 10.1038/ncb3205
94. Moretti A, Caron L, Nakano A, Lam JT, Bernshausen A, Chen Y, et al. Multipotent embryonic Isl1+ progenitor cells lead to cardiac, smooth muscle, and endothelial cell diversification. Cell. (2006) 127:1151–65. doi: 10.1016/j.cell.2006.10.029
95. Kim K, Lerou P, Yabuuchi A, Lengerke C, Ng K, West J, et al. Histocompatible embryonic stem cells by parthenogenesis. Science. (2007) 315:482–6. doi: 10.1126/science.1133542
96. Yoshioka N, Gros E, Li HR, Kumar S, Deacon DC, Maron C, et al. Efficient generation of human iPSCs by a synthetic self-replicative RNA. Cell Stem Cell. (2013) 13:246–54. doi: 10.1016/j.stem.2013.06.001
97. Kilpinen H, Goncalves A, Leha A, Afzal V, Alasoo K, Ashford S, et al. Common genetic variation drives molecular heterogeneity in human iPSCs. Nature. (2017) 546:370–5. doi: 10.1038/nature22403
98. Kiskinis E, Eggan K. Progress toward the clinical application of patient-specific pluripotent stem cells. J Clin Invest. (2010) 120:51–9. doi: 10.1172/JCI40553
99. Andrews PW, Ben-David U, Benvenisty N, Coffey P, Eggan K, Knowles BB, et al. Assessing the safety of human pluripotent stem cells and their derivatives for clinical applications. Stem Cell Rep. (2017) 9:1–4. doi: 10.1016/j.stemcr.2017.05.029
100. Park SJ, Kim RY, Park BW, Lee S, Choi SW, Park JH, et al. Dual stem cell therapy synergistically improves cardiac function and vascular regeneration following myocardial infarction. Nat Commun. (2019) 10:1–12. doi: 10.1038/s41467-019-11091-2
101. Chen H, Zhang A, Wu JC. Harnessing cell pluripotency for cardiovascular regenerative medicine. Nat Biomed Eng. (2018) 2:392–8. doi: 10.1038/s41551-018-0244-8
102. Shafei AES, Ali MA, Ghanem HG, Shehata AI, Abdelgawad AA, Handal HR, et al. Mesenchymal stem cell therapy: a promising cell-based therapy for treatment of myocardial infarction. J Gene Med. (2017) 19:e2995. doi: 10.1002/jgm.2995
103. de Abreu RC, Fernandes H, da Costa Martins PA, Sahoo S, Emanueli C, Ferreira L. Native and bioengineered extracellular vesicles for cardiovascular therapeutics. Nat Rev Cardiol. (2020) 17:685–97. doi: 10.1038/s41569-020-0389-5
104. Liu B, Lee BW, Nakanishi K, Villasante A, Williamson R, Metz J, et al. Cardiac recovery via extended cell-free delivery of extracellular vesicles secreted by cardiomyocytes derived from induced pluripotent stem cells. Nat Biomed Eng. (2018) 2:293–303. doi: 10.1038/s41551-018-0229-7
105. D'Amore A, Yoshizumi T, Luketich SK, Wolf MT, Gu X, Cammarata M, et al. Bi-layered polyurethane–extracellular matrix cardiac patch improves ischemic ventricular wall remodeling in a rat model. Biomaterials. (2016) 107:1–14. doi: 10.1016/j.biomaterials.2016.07.039
106. Recchia AG, Filice E, Pellegrino D, Dobrina A, Cerra MC, Maggiolini M. Endothelin-1 induces connective tissue growth factor expression in cardiomyocytes. J Mol Cell Cardiol. (2009) 46:352–9. doi: 10.1016/j.yjmcc.2008.11.017
107. Lakshmanan R, Kumaraswamy P, Krishnan UM, Sethuraman S. Engineering a growth factor embedded nanofiber matrix niche to promote vascularization for functional cardiac regeneration. Biomaterials. (2016) 97:176–95. doi: 10.1016/j.biomaterials.2016.02.033
108. Kosaka N, Sakamoto H, Terada M, Ochiya T. Pleiotropic function of FGF-4: its role in development and stem cells. Dev Dyn. (2009) 238:265–76. doi: 10.1002/dvdy.21699
109. El Andaloussi S, Mäger I, Breakefield XO, Wood MJA. Extracellular vesicles: biology and emerging therapeutic opportunities. Nat Rev Drug Discov. (2013) 12:347–57. doi: 10.1038/nrd3978
110. Raposo G, Stoorvogel W. Extracellular vesicles: exosomes, microvesicles, and friends. J Cell Biol. (2013) 200:373–83. doi: 10.1083/jcb.201211138
111. György B, Szabó TG, Pásztói M, Pál Z, Misják P, Aradi B, et al. Membrane vesicles, current state-of-the-art: Emerging role of extracellular vesicles. Cell Mol Life Sci. (2011) 68:2667–88. doi: 10.1007/s00018-011-0689-3
112. Mathivanan S, Ji H, Simpson RJ. Exosomes: extracellular organelles important in intercellular communication. J Proteomics. (2010) 73:1907–20. doi: 10.1016/j.jprot.2010.06.006
113. Théry C, Zitvogel L, Amigorena S. Exosomes: composition, biogenesis and function. Nat Rev Immunol. (2002) 2:569–79. doi: 10.1038/nri855
114. Loyer X, Vion AC, Tedgui A, Boulanger CM. Microvesicles as cell-cell messengers in cardiovascular diseases. Circ Res. (2014) 114:345–53. doi: 10.1161/CIRCRESAHA.113.300858
115. Kuwabara Y, Ono K, Horie T, Nishi H, Nagao K, Kinoshita M, et al. Increased microRNA-1 and microRNA-133a levels in serum of patients with cardiovascular disease indicate myocardial damage. Circ Cardiovasc Genet. (2011) 4:446–54. doi: 10.1161/CIRCGENETICS.110.958975
116. Hamada T, Dubois JLN, Bellamy V, Pidial L, Hagège A, Pereira MN, et al. In vitro controlled release of extracellular vesicles for cardiac repair from poly(glycerol sebacate) acrylate-based polymers. Acta Biomater. (2020) 115:92–103. doi: 10.1016/j.actbio.2020.08.015
117. Hu GW, Li Q, Niu X, Hu B, Liu J, Zhou SM, et al. Exosomes secreted by human-induced pluripotent stem cell-derived mesenchymal stem cells attenuate limb ischemia by promoting angiogenesis in mice. Stem Cell Res Ther. (2015) 6:10. doi: 10.1186/scrt546
118. Kowal J, Tkach M, Théry C. Biogenesis and secretion of exosomes. Curr Opin Cell Biol. (2014) 29:116–25. doi: 10.1016/j.ceb.2014.05.004
119. Bheri S, Davis ME. Nanoparticle–hydrogel system for post-myocardial infarction delivery of microRNA. ACS Nano. (2019) 13:9702–6. doi: 10.1021/acsnano.9b05716
120. Bejerano T, Etzion S, Elyagon S, Etzion Y, Cohen S. Nanoparticle Delivery of miRNA-21 mimic to cardiac macrophages improves myocardial remodeling after myocardial infarction. Nano Lett. (2018) 18:5885–91. doi: 10.1021/acs.nanolett.8b02578
121. Yang H, Qin X, Wang H, Zhao X, Liu Y, Wo H-T, et al. An in vivo miRNA delivery system for restoring infarcted myocardium. ACS Nano. (2019) 13:9880–94. doi: 10.1021/acsnano.9b03343
122. Maegdefessel L, Azuma J, Toh R, Deng A, Merk DR, Raiesdana A, et al. MicroRNA-21 blocks abdominal aortic aneurysm development and nicotine-augmented expansion. Sci Transl Med. (2012) 4:122ra22. doi: 10.1126/scitranslmed.3003441
123. Liu HJ, Luan X, Feng HY, Dong X, Yang SC, Chen ZJ, et al. Integrated combination treatment using a “smart” chemotherapy and microrna delivery system improves outcomes in an orthotopic colorectal cancer model. Adv Funct Mater. (2018) 28:1801118. doi: 10.1002/adfm.201801118
124. Li Y, Dal-Pra S, Mirotsou M, Jayawardena TM, Hodgkinson CP, Bursac N, et al. Tissue-engineered 3-dimensional (3D) microenvironment enhances the direct reprogramming of fibroblasts into cardiomyocytes by microRNAs. Sci Rep. (2016) 6:38815. doi: 10.1038/srep38815
125. Cui H, Liu C, Esworthy T, Huang Y, Yu Z, Zhou X, et al. 4D physiologically adaptable cardiac patch: A 4-month in vivo study for the treatment of myocardial infarction. Sci Adv. (2020) 6:eabb5067. doi: 10.1126/sciadv.abb5067
126. Norahan MH, Pourmokhtari M, Saeb MR, Bakhshi B, Soufi Zomorrod M, Baheiraei N. Electroactive cardiac patch containing reduced graphene oxide with potential antibacterial properties. Mater Sci Eng C. (2019) 104:109921. doi: 10.1016/j.msec.2019.109921
127. Kapnisi M, Mansfield C, Marijon C, Guex AG, Perbellini F, Bardi I, et al. Auxetic cardiac patches with tunable mechanical and conductive properties toward treating myocardial infarction. Adv Funct Mater. (2018) 28:1800618. doi: 10.1002/adfm.201800618
128. Tse JR, Engler AJ. Preparation of hydrogel substrates with tunable mechanical properties. Curr Protoc Cell Biol. (2010) 47:10–16. doi: 10.1002/0471143030.cb1016s47
129. Cheng K, Kisaalita WS. Exploring cellular adhesion and differentiation in a micro-/nano-hybrid polymer scaffold. Biotechnol Prog. (2010) 26:838–46. doi: 10.1002/btpr.391
130. Singh A, Elisseeff J. Biomaterials for stem cell differentiation. J Mater Chem. (2010) 20:8832–47. doi: 10.1039/c0jm01613f
131. Dilauro AM, Robbins JS, Phillips ST. Reproducible and scalable synthesis of end-cap-functionalized depolymerizable poly(phthalaldehydes). Macromolecules. (2013) 46:2963–8. doi: 10.1021/ma4001594
132. Chan G, Mooney DJ. New materials for tissue engineering: towards greater control over the biological response. Trends Biotechnol. (2008) 26:382–92. doi: 10.1016/j.tibtech.2008.03.011
133. Pok S, Jacot JG. Biomaterials advances in patches for congenital heart defect repair. J Cardiovasc Transl Res. (2011) 4:646–54. doi: 10.1007/s12265-011-9289-8
134. Sharma S, Srivastava D, Grover S, Sharma V. Biomaterials in tooth tissue engineering: a review. J Clin Diagnostic Res. (2014) 8:309–15. doi: 10.7860/JCDR/2014/7609.3937
135. Sheikh Z, Hamdan N, Ikeda Y, Grynpas M, Ganss B, Glogauer M. Natural graft tissues and synthetic biomaterials for periodontal and alveolar bone reconstructive applications: a review. Biomater Res. (2017) 21:9. doi: 10.1186/s40824-017-0095-5
136. Knott L, Bailey AJ. Collagen cross-links in mineralizing tissues: a review of their chemistry, function, and clinical relevance. Bone. (1998) 22:181–7. doi: 10.1016/S8756-3282(97)00279-2
137. Saldin LT, Cramer MC, Velankar SS, White LJ, Badylak SF. Extracellular matrix hydrogels from decellularized tissues: structure and function. Acta Biomater. (2017) 49:1–15. doi: 10.1016/j.actbio.2016.11.068
138. Keane TJ Swinehart IT Badylak SF. Methods of tissue decellularization used for preparation of biologic scaffolds and in vivo relevance. Methods. (2015) 84:25–34. doi: 10.1016/j.ymeth.2015.03.005
139. Bassat E, Mutlak YE, Genzelinakh A, Shadrin IY, Baruch Umansky K, Yifa O, et al. The extracellular matrix protein agrin promotes heart regeneration in mice. Nature. (2017) 547:179–84. doi: 10.1038/nature22978
140. Yamato M, Okano T. Cell sheet engineering. Mater Today. (2004) 7:42–7. doi: 10.1016/S1369-7021(04)00234-2
141. Sekine H, Shimizu T, Dobashi I, Matsuura K, Hagiwara N, Takahashi M, et al. Cardiac cell sheet transplantation improves damaged heart function via superior cell survival in comparison with dissociated cell injection. Tissue Eng Part A. (2011) 17:2973–80. doi: 10.1089/ten.tea.2010.0659
142. Egami M, Haraguchi Y, Shimizu T, Yamato M, Okano T. Latest status of the clinical and industrial applications of cell sheet engineering and regenerative medicine. Arch Pharm Res. (2014) 37:96–106. doi: 10.1007/s12272-013-0299-8
143. Kronsteiner B, Wolbank S, Peterbauer A, Hackl C, Redl H, Van Griensven M, et al. Human mesenchymal stem cells from adipose tissue and amnion influence T-cells depending on stimulation method and presence of other immune cells. Stem Cells Dev. (2011) 20:2115–26. doi: 10.1089/scd.2011.0031
144. Narita T, Shintani Y, Ikebe C, Kaneko M, Harada N, Tshuma N, et al. The use of cell-sheet technique eliminates arrhythmogenicity of skeletal myoblast-based therapy to the heart with enhanced therapeutic effects. Int J Cardiol. (2013) 168:261–9. doi: 10.1016/j.ijcard.2012.09.081
145. Kitsara M, Agbulut O, Kontziampasis D, Chen Y, Menasché P. Fibers for hearts: a critical review on electrospinning for cardiac tissue engineering. Acta Biomater. (2017) 48:20–40. doi: 10.1016/j.actbio.2016.11.014
146. Sax H, Bloemberg G, Hasse B, Sommerstein R, Kohler P, Achermann Y, et al. Prolonged outbreak of mycobacterium chimaera infection after open-chest heart surgery. Clin Infect Dis. (2015) 61:67–75. doi: 10.1093/cid/civ198
147. Patra S, Young V. A review of 3D printing techniques and the future in biofabrication of bioprinted tissue. Cell Biochem Biophys. (2016) 74:93–8. doi: 10.1007/s12013-016-0730-0
148. Athanasiou KA, Niederauer GG, Agrawal CM. Sterilization, toxicity, biocompatibility and clinical applications of polylactic acid/polyglycolic acid copolymers. Biomaterials. (1996) 17:93–102. doi: 10.1016/0142-9612(96)85754-1
149. Leor J, Cohen S. Myocardial tissue engineering: creating a muscle patch for a wounded heart. Ann N Y Acad Sci. (2004) 1015:312–9. doi: 10.1196/annals.1302.026
150. Soon G, Pingguan-Murphy B, Lai KW, Akbar SA. Review of zirconia-based bioceramic: surface modification and cellular response. Ceram Int. (2016) 42:12543–55. doi: 10.1016/j.ceramint.2016.05.077
151. Li Z, Hu S, Cheng K. Chemical engineering of cell therapy for heart diseases. Acc Chem Res. (2019) 52:1687–96. doi: 10.1021/acs.accounts.9b00137
152. Huang K, Hu S, Cheng K. A new era of cardiac cell therapy: opportunities and challenges. Adv Healthc Mater. (2019) 8:1801011. doi: 10.1002/adhm.201801011
Keywords: biomaterials, cardiac patch, myocardial infarction, cell therapy, cardiac tissue regeneration
Citation: Mei X and Cheng K (2020) Recent Development in Therapeutic Cardiac Patches. Front. Cardiovasc. Med. 7:610364. doi: 10.3389/fcvm.2020.610364
Received: 25 September 2020; Accepted: 03 November 2020;
Published: 27 November 2020.
Edited by:
Huaxiao Adam Yang, University of North Texas, United StatesReviewed by:
Oscar J. Abilez, Stanford University, United StatesZhen Ma, Syracuse University, United States
Copyright © 2020 Mei and Cheng. This is an open-access article distributed under the terms of the Creative Commons Attribution License (CC BY). The use, distribution or reproduction in other forums is permitted, provided the original author(s) and the copyright owner(s) are credited and that the original publication in this journal is cited, in accordance with accepted academic practice. No use, distribution or reproduction is permitted which does not comply with these terms.
*Correspondence: Ke Cheng, a2NoZW5nM0BuY3N1LmVkdQ==