- 1Department of Pharmacology and Toxicology, American University of Beirut, Beirut, Lebanon
- 2Department of Biochemistry and Molecular Genetics, American University of Beirut, Beirut, Lebanon
- 3Department of Pharmacology and Therapeutics, Beirut Arab University, Beirut, Lebanon
- 4Department of Pharmacology and Therapeutics, Faculty of Medicine, American University of Beirut, Beirut, Lebanon
- 5Department of Basic Medical Sciences, College of Medicine, Qatar University, Doha, Qatar
- 6Biomedical and Pharmaceutical Research Unit, QU Health, Qatar University, Doha, Qatar
- 7Department of Pharmacology and Toxicology, Faculty of Pharmacy, Alexandria University, Alexandria, Egypt
Adipose tissue is a critical regulator of systemic metabolism and bodily homeostasis as it secretes a myriad of adipokines, including inflammatory and anti-inflammatory cytokines. As the main storage pool of lipids, subcutaneous and visceral adipose tissues undergo marked hypertrophy and hyperplasia in response to nutritional excess leading to hypoxia, adipokine dysregulation, and subsequent low-grade inflammation that is characterized by increased infiltration and activation of innate and adaptive immune cells. The specific localization, physiology, susceptibility to inflammation and the heterogeneity of the inflammatory cell population of each adipose depot are unique and thus dictate the possible complications of adipose tissue chronic inflammation. Several lines of evidence link visceral and particularly perivascular, pericardial, and perirenal adipose tissue inflammation to the development of metabolic syndrome, insulin resistance, type 2 diabetes and cardiovascular diseases. In addition to the implication of the immune system in the regulation of adipose tissue function, adipose tissue immune components are pivotal in detrimental or otherwise favorable adipose tissue remodeling and thermogenesis. Adipose tissue resident and infiltrating immune cells undergo metabolic and morphological adaptation based on the systemic energy status and thus a better comprehension of the metabolic regulation of immune cells in adipose tissues is pivotal to address complications of chronic adipose tissue inflammation. In this review, we discuss the role of adipose innate and adaptive immune cells across various physiological and pathophysiological states that pertain to the development or progression of cardiovascular diseases associated with metabolic disorders. Understanding such mechanisms allows for the exploitation of the adipose tissue-immune system crosstalk, exploring how the adipose immune system might be targeted as a strategy to treat cardiovascular derangements associated with metabolic dysfunctions.
Introduction
Over the past two decades, the traditional view of adipose tissue (AT) as a passive store of excess calories evolved to implicate an endocrine role that is particularly pertinent to glucose and lipid homeostasis (1). This endocrine function is the result of a complex interaction between adipocytes and cells of the stromal vascular fraction of AT, which modulate the type of mediators produced in different conditions of health and disease. Importantly, this endocrine role is ascribed to the white adipose tissue (WAT); one of the two major types of AT. While, WAT comprises unilocular adipocytes that specialize in the storage of energy and the regulation of metabolic homeostasis by the production of adipokines, brown AT (BAT) is formed of mitochondria-rich multilocular adipocytes whose main function is energy dissipation through thermogenesis (2). Interestingly, accumulating evidence shows that both endocrine and thermogenic functions are modulated by resident and infiltrating immune cells. In fact, AT harbors a plethora of immune cells belonging to both the innate and adaptive immune systems, which either exert a pro- or an anti-inflammatory role depending on the microenvironmental stimulation and metabolic rewiring. Obese AT represents a state of chronic inflammation due to increased adipocyte hypertrophy, hyperplasia and apoptosis accompanied by an alteration in the production of adipokines and inflammatory mediators. This has been linked to the development of insulin resistance (IR), metabolic syndrome (MetS) and type 2 diabetes (T2D) (3). The manifestations of AT inflammation are proposed to alter the phenotype and gene expression profile of adipose immune cells, which was proposed to underlie major comorbidities of obesity including cardiovascular diseases (CVDs) (4).
In this review, we elaborate on the metabolic rewiring of AT-resident and infiltrating immune cells in health and disease and their participation in the inflammatory phenotype of AT relevant to the development of metabolic and cardiovascular disorders. We also touch upon recent evidence implicating AT-resident and infiltrating immune cells in the induction or suppression of AT thermogenesis and its possible outcomes. Finally, we discuss how several interventions immuno-modulate AT function and the exciting future perspectives in the field of AT immunometabolism.
Obesity, at Inflammation and the Metabolic Syndrome
AT Inflammation and Remodeling
The incidence of obesity is increasing globally at an alarming rate with a parallel increase in the associated conditions including IR, CVD, and T2D (5, 6). Obesity is considered a chronic inflammatory disease that is linked to metabolic disorders (7). In this context, AT chronic low-grade inflammation and the progressive infiltration of immune cells into the AT contribute to IR (5, 8). The precise triggers of obesity-correlated inflammation are not fully understood. However, it is widely accepted that overnutrition drives a state of hyperinsulinemia, which participates in AT inflammation by inducing adipocyte hypertrophy followed by hypoxia, adipocyte death, lipotoxicity, and altered extracellular matrix (ECM).
WAT is a poorly vascularized tissue that exhibits a further decreased blood supply during AT expansion resulting in hypoxia. This hypoxic atmosphere is stimulated by increased adipocyte dimensions beyond the oxygen diffusing-ability, increased oxygen demand and lack of proper compensatory vascularization (9, 10). Infiltrating immune cells and ECM alterations also contribute to this hypoxic phenotype (11). Indeed, hypoxia induces the release of pro-inflammatory cytokines, chemokines, and angiogenic as well as fibrotic factors from adipocytes, which favor AT inflammation, vasculature remodeling, and AT dysfunction (9, 12). Hypoxia-induced AT dysfunction is characterized by an extensive lipolytic activity and free fatty acids (FAs) release leading to lipotoxicity, which was shown to exacerbate AT inflammation and participate in the pathogenesis of IR by promoting endoplasmic reticulum (ER) stress, adipocyte apoptosis, and inflammation (8, 13, 14). Hypoxia also causes necrosis-like adipocyte death, which initiates inflammation via interacting with macrophages (15). Nevertheless, AT reacts to adipocyte death by initiating a self-limiting wound healing response, which is characterized by intensive infiltration of immune cells, especially macrophages, that encircle dead fat cells, creating histological crown-like structures (CLS) (15). These macrophages generate toxic reactive oxygen species (ROS) and nitric oxide (NO), which further damage neighboring cells and support fibrosis (16). On the other hand, as the injury signal sustains in obesity, the chronic stimulation of myofibroblasts and immune cells causes additional damage, fibrosis, ECM remodeling and eventually AT dysfunction as well as IR (17, 18).
AT low-grade inflammation is driven by the excessive production of inflammatory cytokines such as tumor necrosis factor (TNF)-α and interleukin (IL)-1β, which activate and recruit immune cells to AT, promoting its remodeling and causing an imbalance between homeostatic AT-resident immune cells and infiltrating inflammatory immune cells (19). The latter cells consist of macrophages, neutrophils, mast cells and T and B lymphocytes among others that secrete cytokines promoting the recruitment and polarization of other inflammatory cells in the AT. Moreover, dendritic cells (DCs), macrophages, and B cells induce the expansion of CD4 and CD8 T cells in the AT (20–22). In case of obese AT, macrophages exceed 50% of the immune cell population compared to lean AT (23, 24), and the production of CXCL12, CCR5, and MCP-1 by the AT tends to recruit and activate macrophages, making macrophages the major producers of cytokines in the AT (25–27).
Differences in Inflammation Susceptibility of Different AT Depots
Various molecular, physiological, and metabolic differences exist among adipose depots (28). Differences found in the microenvironment of WAT depots lead to unequal AT expansion and inflammation susceptibility under metabolic stress. Indeed, BAT is less prone to inflammation in comparison to WAT (29–31). Another good example is the difference in inflammation susceptibility between PVAT and other VAT depots. We have shown that PVAT localized inflammation, which was associated with uncoupling protein 1 (UCP1)-mediated hypoxic preconditioning, occurs in isolation of systemic inflammation in a prediabetic rat model (32). Moreover, EpiCAT has small adipocyte size, high protein content and high rate of FA synthesis compared to other adipose depots making it susceptible to a metabolic profile shift (33). Additionally, during EpiCAT expansion, a quick proinflammatory microenvironment is generated due to the extensive inflammatory immune cell infiltration (34).
Adipokine Profile Dysregulation
Adipokines, which encompass endocrine and other biologically-active proteins, are released by WAT and function as hormones that regulate insulin sensitivity, energy balance, immune system functions and whole-body homeostasis (35). Metabolically healthy individuals possess a balance between proinflammatory and anti-inflammatory adipokines. This balance shifts in favor of proinflammatory mediators as the AT expands in the course of metabolic syndrome and obesity. This adipokine profile dysregulation has been associated with an increased risk of metabolic dysfunction, T2D and CVDs. Importantly, these adipokines profoundly influence the activation state, differentiation, and proliferation of AT-resident and infiltrating immune cells. Anti-inflammatory adipokines include adiponectin, C1q/TNF-related proteins (CTRPs), omentin, and secreted frizzled-related protein 5 (SFRP5) (36–38). Proinflammatory adipokines include leptin, resistin, chemerin, visfatin, retinol binding protein 4 (RBP4), and lipocalin 2 (LCN2) (35).
Adiponectin
Adiponectin is the best-known and most abundant adipokine found in human serum with insulin-sensitizing and cardioprotective actions (39, 40). Adiponectin serum levels decrease in obesity, T2D, and in states of high oxidative stress (41, 42). Total plasma adiponectin levels are also inversely correlated with MI risk (43, 44). Adiponectin-deficient mice exhibit an exacerbated myocardial ischemic injury, and adiponectin supplementation protects the heart against ischemia/reperfusion injury (45, 46). In circulation, adiponectin forms low, intermediate, and high molecular weight complexes where the high molecular weight complex was shown to block NF-κB activation and the production of proinflammatory cytokines (47, 48). Adiponectin exerts its effects by binding to its tissue-specific receptors, AdipoR1 and AdipoR2, which results in the downstream activation of AMPK, Akt-eNOS phosphorylation, and NO production (49–51). Moreover, adiponectin exerts an antioxidant (oxidative and nitrative stress) activity that is AMPK-independent and that is largely mediated via PKA-dependent NF-κB inhibition (52). Importantly, adiponectin modulates the activity of several immune cells in the AT including macrophages (53, 54), eosinophils (55), and mast cells (56). Indeed, profound mechanistic frameworks for this modulation are still lacking and require further investigation.
CTRPs
CTRPs are structurally similar, paralogs of adiponectin, with at least 15 isoforms being described to date where they exhibit broadly diverse effects (57, 58). For example, CTRP1 plays an important role in regulating body energy homeostasis and insulin sensitivity (59). Plasma CTRP1 was higher and negatively correlated with insulin resistance in diabetic subjects (60, 61). A recent study highlighted a significant association between increased CTRP1 levels and metabolic syndrome, obesity, T2D and non-alcoholic fatty liver disease (62). It was suggested that CTRP1 improves insulin resistance by reducing the phosphorylation of IRS-1 Ser1101 (61). In line with that, it was shown that elevated concentrations of CTRP1 reduce weight gain and diet-induced insulin resistance (59). Moreover, CTRP1 was shown to enhance glucose uptake through an increased GLUT4 translocation to the plasma membrane and enhanced glycolysis in HFD-fed CTRP1 transgenic mice (63). Moreover, CTRP1 promoted fatty acid oxidation and therefore, CTRP1 seems to perform a defensive catabolic effect in response to nutritional challenges. Interestingly, CTRP1-deficient mice fed a low-fat diet developed insulin resistance and hepatic steatosis (64). At the level of the cardiovascular system, CTRP1 was shown to regulate blood pressure through the induction of vasoconstriction (65). As such, mice overexpressing CTRP1 are hypertensive and hypertensive patients display a higher CTRP1 levels in comparison to healthy individuals (65). Moreover, CTRP1 was demonstrated to limit the extent of ischemia-reperfusion injury in acute myocardial infarction (59). The level of CTRP1 was also significantly increased in CAD patients and was suggested as a superior biomarker for the diagnosis of severity of vessel-lesion in CAD patients (66, 67). Interestingly, CRTP1 levels positively correlated with concentrations of IL-6 and TNF-α in CAD patients (66). In congestive heart failure patients, the levels of CTRP1 in serum and EpiCAT were higher than in controls, which was associated with a worse prognosis (68). Nevertheless, the implication of CTRP1 serum levels alteration on the activity of immune cells in models of metabolic and cardiovascular diseases has not yet been assessed.
CTRP3 (also known as cartducin) regulates adiponectin secretion from adipocytes (69, 70). CTRP3 was also shown to regulate glucose homeostasis (71), to stimulate in vitro endothelial cell proliferation and migration (58), and to inhibit TLR4 signaling and cytokine production in LPS- and FFA-stimulated adipocytes and monocytes (58). Importantly, CTRP3 serum level decrease following myocardial infarction and its restoration post-MI attenuates post-ischemic pathological remodeling (72).
Plasma CTRP9 levels are decreased in rodent models of obesity and diabetes (73, 74). Importantly, CTRP9 heterodimerizes with adiponectin and shares AdipoR1 stimulation in cultured cardiomyocytes and endothelial cells (73, 75, 76). CTRP9 promotes eNOS activity and NO production via AdipoR1-mediated activation of AMPK, resulting in endothelium-dependent vasorelaxation of aortic rings (76). Moreover, CTRP9 attenuates inflammation in TNF-α-stimulated endothelial cells via AMPK activation and inhibits inflammatory responses in ox-LDL-stimulated macrophages (77, 78). Indeed, CTRP9-deficient mice are obese and insulin resistant (79). Importantly, several studies demonstrated a cardioprotective effect of CTRP9 (73, 74, 80, 81).
Adipolin (CTRP12) is an insulin-sensitizing adipokine that is abundantly produced by AT and whose expression levels decrease in rodent models of obesity (82, 83). The systemic administration of adipolin ameliorated glucose intolerance and insulin resistance in HFD-fed obese mice (82). Adipolin administration also attenuated macrophage infiltration and proinflammatory genes expression in AT of obese mice (82). Importantly, it was demonstrated that adipolin levels increase in response to hyperinsulinemia induction in healthy lean human subjects or following PPARγ agonism (84). This indicates that adipolin, as a novel anti-inflammatory adipokine, increases in the early stages of the metabolic insult to curb metabolic derangements and these levels are not sustained following prolonged metabolic disease induction. Importantly, adipolin levels were found to be lower in CAD patients compared to healthy controls (85). Moreover, adipolin levels were inversely correlated with HOMA-IR and TNF-α and positively correlated with adiponectin expression levels (85). Another study highlighted that adipolin levels decrease in acute myocardial infarction patients and that these levels are negatively associated with epicardial fat thickness (86). Indeed, adipolin-deficient mice exhibited an exacerbated neointimal thickening following vascular injury which was accompanied by enhanced inflammation and vascular cell proliferation (87). Adipolin-treated LPS-stimulated macrophages in vitro exhibited a reduced expression of IL-6 and TNF-α. Moreover, adipolin-deficient MI mice had increased myocardial apoptosis, cardiomyocyte hypertrophy, and perivascular fibrosis at the remote zone of infarct heart through an Akt-dependent mechanism (88). This indicates that adipolin exerts a protective effect against pathological processes of vascular and cardiac remodeling.
The adipokine CTRP6 regulates metabolism and inflammation (89, 90). CTRP6 improves cardiac function and ameliorates ventricular remodeling post-MI (91). CTRP13 was also shown to improve insulin sensitivity and inhibit the inflammation of lipid-loaded hepatocytes (92).
Omentin
Omentin is a novel adipokine whose levels decrease in obese subjects and negatively correlate with carotid intima media thickness (93–95). Moreover, omentin expression is negatively associated with the prevalence and the angiographic severity of coronary artery disease (96). Omentin inhibits TNF-α-induced endothelial COX2 expression and induces the activity of eNOS (97). Moreover, omentin enhances isolated aortic rings dilation in mice in an eNOS-dependent manner (98). Omentin systemic delivery also attenuated neointimal thickening and vascular smooth muscle proliferation in an AMPK-dependent mechanism (99). Therefore, omentin functions as an anti-atherogenic and anti-inflammatory adipokine similar to adiponectin and the CTRPs.
SFRP5
SFRP5 has anti-inflammatory effects in AT and in macrophages where it was shown to suppress the noncanonical Wnt5a/JNK signaling which inhibits the synthesis of macrophage TNF-α, IL-1β, and CCL2-MCP1 (100).
Leptin
First described as a satiety hormone, leptin was shown to bind to long form of leptin receptor expressed in nearly all immune cells to initiate innate immune responses (101). Leptin enhances the production of proinflammatory cytokines in peripheral blood monocytes and tissue-resident macrophages in mice and humans (102–105). Leptin also induces ROS production in macrophages, neutrophils, and endothelial cells and potentiate the expression of INFγ-induced nitric oxide synthase (106–108). Leptin also enhances Th1 and Th17 immune responses and prevents T cell apoptosis (109).
Resistin
Resistin was first characterized as a mediator of insulin resistance, metabolic syndrome, and T2D in mice (110). Although WAT represents the primary source of resistin in mice, monocytes and macrophages are the most important source of resistin in humans (111). The proinflammatory actions of resistin are mediated by CAP-1, a resistin receptor, with downstream activation of NF-κB in human monocytes (112). Resistin binds to TLR4 and regulates the production of TNF-α and IL-6 in macrophages through the activation of NF-κB signaling (113). Importantly, resistin levels are elevated in obese humans and are associated with an increased risk of CVDs (114).
Visfatin
Visfatin, also known as pre-B cell colony-enhancing factor (PBEF), is a novel, highly conserved adipokine that is abundantly expressed in visceral fat (115, 116). Visfatin plays a determinant role in the pathophysiology of metabolic and cardiovascular diseases (117). Visfatin elicits insulomimetic effects in adipocytes and an increased blood glucose level prompts an increase of serum visfatin (115, 118). Nevertheless, it was suggested that the effects of visfatin do not involve the classical insulin signaling pathways in skeletal muscles (115, 119). Indeed, several studies demonstrated an association between increased plasma visfatin level and diabetes (120, 121). In contrast, other studies reported opposite or no association between visfatin plasma levels and diabetes (122, 123). Similar controversy was also documented when correlating visfatin plasma levels with obesity (124–126). Despite the role of visfatin in metabolic disorders remaining debatable (127), it does not rule out visfatin implication in these disorders and its participation in metabolic dysfunction-associated cardiovascular diseases. Several studies suggested a pro-inflammatory role of visfatin in both VAT and scWAT (128). In fact, visfatin was shown to enhance monocyte-mediated recruitment of T cells and B cells by increasing the expression of CD80, CD40, and ICAM-1 (129). Moreover, visfatin-stimulated human leukocytes exhibit a dose-dependent induction in the expression of IL-1β, IL-1Ra, IL-10, and IL-6 (129).
LCN2 and RBP4
LCN2, also known as neutrophil gelatinase-associated lipocalin (NGAL) is upregulated in the presence of IFN-γ and TNF-α in obese individuals (130, 131). Similarly, RBP4, which is mostly complexed with retinol in circulation, was shown to promote IR and increases the risk of T2D (132, 133). RBP4 activates antigen presenting cells and is suggested as a cardiometabolic marker in MetS (134).
Alongside the above-mentioned changes observed in the adipokine profile with the induction and progression of metabolic disease, a bi-directional interaction proceeds within the AT microenvironment among adipocytes and different types of resident and infiltrating immune cells. Details and outcomes of this interaction will be discussed for each of the cell types below. A summary of the different pathways and mediators involved is provided in Figure 1.
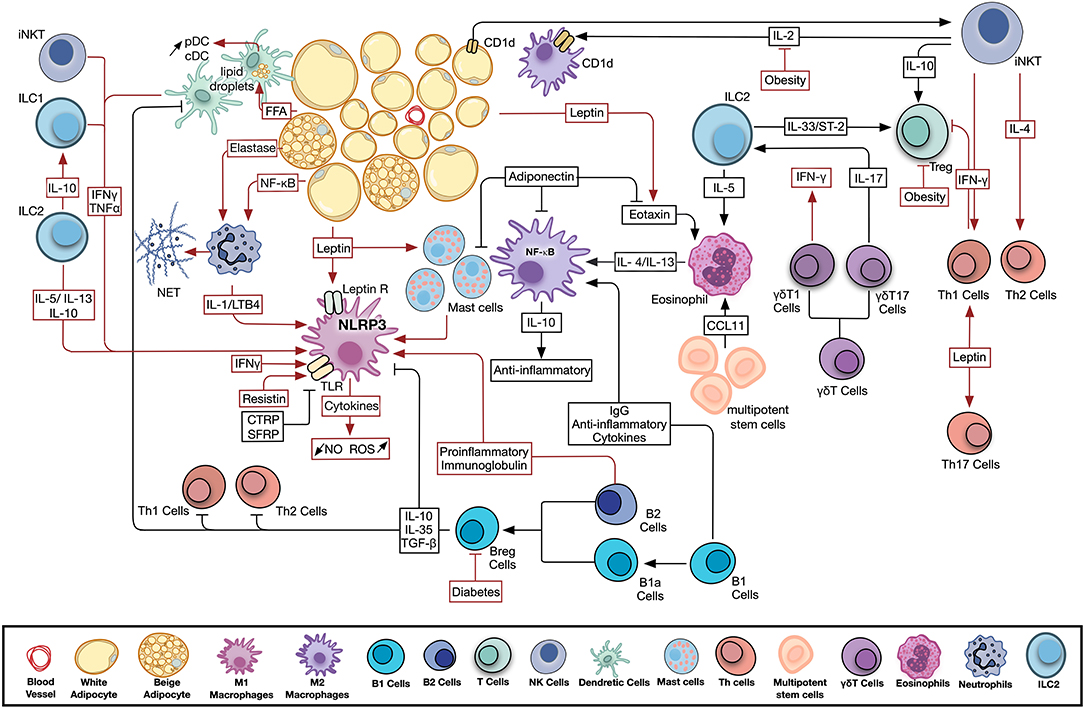
Figure 1. Adipose tissue resident and infiltrating immune cells activity in health and disease. Significant crosstalk exists among adipocytes, adipokines, and resident and infiltrating, innate and adaptive immune cells. Metabolic disease conditions modulate the adipokine profile and immune cell activity leading to the observed chronic low-grade inflammation. Pathways involved in AT homeostasis are depicted in black arrows, while those activated by metabolic dysfunction are shown in red. B Cell, B Lymphocyte; Breg Cells, Regulatory B Lymphocyte; CCL11, C-C motif chemokine 11; CD1d, Cluster of Differentiation 1d; cDC, Conventional Dendritic Cell; FFA, Free Fatty Acids; IFNγ, Interferon Gamma; IgG, Immunoglobulin G; IL, Interleukin; ILC, Innate Lymphoid Cell; iNKT Cell, Invariant Natural Killer T Cell; LTB4, Leukotriene B4; NET, Neutrophil Extracellular Trap; NF-κB, Nuclear Factor Kapp-light-chain-enhancer of Activated B cells; NLRP3, NLR Family Pyrin Domain Containing 3; NO, Nitric Oxide; pDC, Plasmacytoid Dendritic Cell; ROS, Reactive Oxygen Species; T Cell, T Lymphocyte; TGF-β, Transforming Growth Factor Beta; Th Cell, Helper T Lymphocyte; TLR, Toll Like Receptor; TNFα, Tumor Necrosis Factor Alpha; Treg, Regulatory T Lymphocyte.
Metabolic Regulation and Adaptation of Tissue Resident and Infiltrating Myeloid Cells
Macrophages
Tissue-resident macrophages are highly heterogeneous with distinct, localization-dependent transcriptomes (135). Classically-activated M1 macrophages, which drive CLS formation, can be induced by LPS, toll-like receptor (TLR) ligands or interferon (IFN)-γ, secrete pro-inflammatory cytokines and upregulate the production of ROS and NO following activation (136). Conversely, alternatively-activated M2 macrophages, which contribute to AT homeostasis, are induced by IL-4 and IL-13, are implicated in the resolution of inflammation, and produce anti-inflammatory cytokines such as IL-10 (137). Although being useful to highlight the inflammatory state of tissues in health and disease, the M1/M2 macrophage classification paradigm is now considered an oversimplification as it does not consider microenvironmental factors.
Macrophages exhibit differential metabolic profiles based on their specific polarization and microenvironmental factors (138). These metabolic alterations are summarized in Table 1. Indeed, the dynamic inflammatory milieu of obese AT drives ATMs metabolic profile modifications. ATMs in obese mice exhibit an increased activation of OXPHOS and glycolysis (140). In addition, the activation of the NLRP3 inflammasome in macrophages by the increasing exogenous FAs in obesity contributes to the emergence of M1 macrophages (155–157). Moreover, monocytes and macrophages express the leptin receptor, which induces the proliferation of macrophages and the production of pro-inflammatory cytokines in response to leptin (158). In contrast to leptin, adiponectin suppresses the NF-κB-dependent expression of pro-inflammatory cytokines and promotes M2 polarization (53, 54). Nevertheless, another study argued that adiponectin induced the production of pro-inflammatory cytokines in M2 macrophages without interfering with their polarization (159).
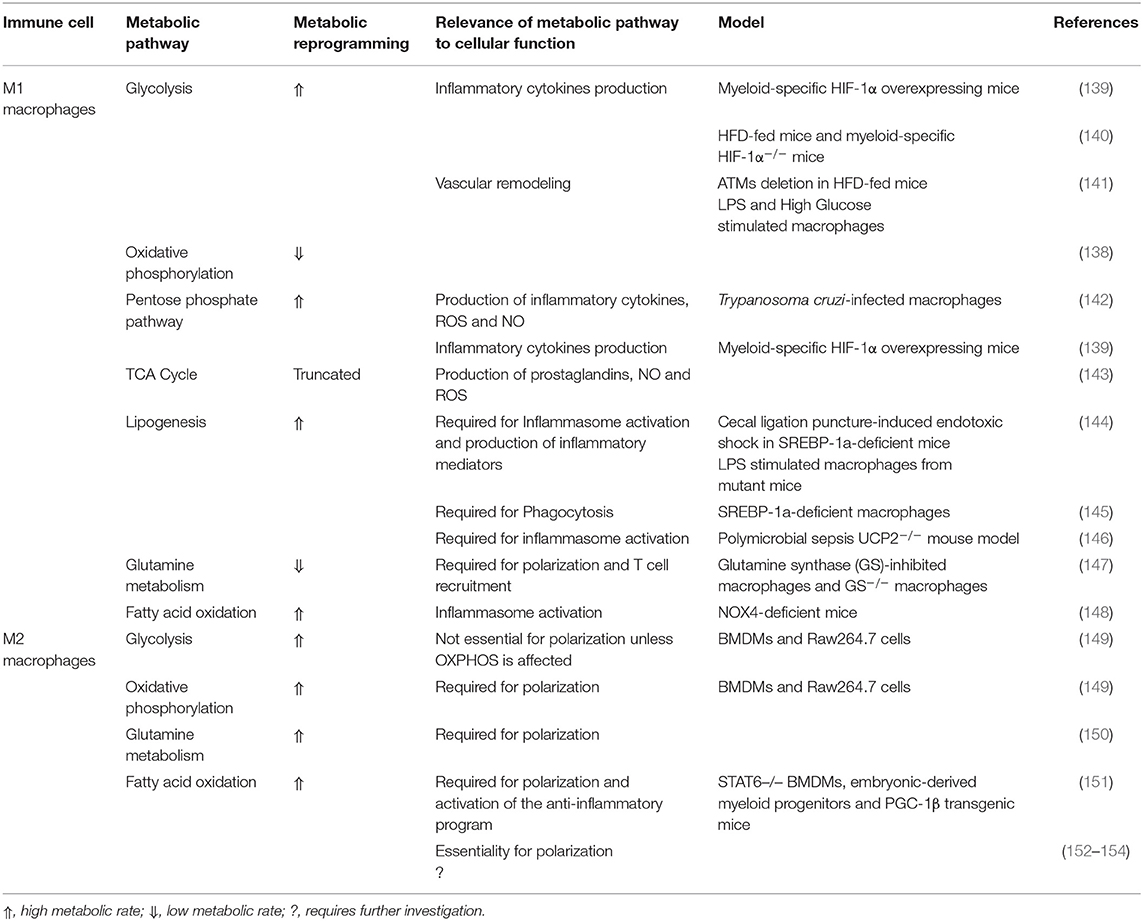
Table 1. Metabolic pathways of classically activated M1 macrophages and alternatively activated M2 macrophages.
Dendritic Cells
Dendritic cells (DCs) are professional antigen-presenting cells that either instigate or suppress immune responses based on their maturation state. DCs are divided into two categories, plasmacytoid DC (pDC) and myeloid or conventional DC (cDC). Accumulating evidence implicates DCs and particularly cDCs in the regulation of AT inflammation. The DC population expands, promotes macrophage recruitment and induce a Th17-driven inflammatory response in HFD-fed mice (160–162). Indeed, HFD-fed mice exhibited an increased number of CD11c+ DCs in the AT whose ablation attenuated visceral AT inflammation (160, 161, 163). The accumulation of cDC during obesity was also attenuated in CCR7-deficient mice, which was associated with decreased AT inflammation (164, 165). Conversely, cDCs in AT were shown to acquire a tolerogenic phenotype through the activation of β-catenin and PPARγ without affecting weight gain (166). pDCs were also shown to accumulate in AT and to have detrimental effects in mice and humans (167, 168). DCs also influence the normal expansion of lean AT, where increased adiposity was accompanied by a reduction of CD11c+ AT DCs (169). Moreover, the uptake and accumulation of FAs in DCs and the formation of lipid droplets (LDs) were associated with increased DC immunogenicity (170, 171). Due to the lipid-rich environment of WAT especially in obesity, AT DCs are expected to acquire more LDs. Nevertheless, the functional impacts of this remain to be investigated. Different DC subsets exhibit distinct metabolic programs (172). In fact, resting and stimulated DCs have different metabolic requirements and thus, employ differential metabolic pathways. These pathways are highlighted in Table 2.
Neutrophils
Neutrophils are relatively rare in WAT of lean mice, where they are suggested to maintain tissue homeostasis (178). Neutrophils are among the first immune cells to be recruited to the AT of HFD-fed mice with a sustained infiltration. Neutrophils drive AT inflammation and IR through the production of inflammatory mediators and the formation of neutrophil extracellular traps (NETs) (179–181). Neutrophils accumulation in AT is dependent on the production of elastase, whose activity is enhanced in the AT of HFD-fed mice (179). WAT-infiltrating neutrophils exhibit an upregulation of IL-1β expression via NF-κB activation in an adipocyte contact-dependent manner (182). Adipocyte lipolysis and LTB4 production in WAT also accumulates neutrophils prior to macrophages and increases the production of IL-1β, which enhances macrophage recruitment into the AT (182). Nevertheless, it was proposed that neutrophils, similar to macrophages, exhibit phenotypic heterogeneity by which N1 neutrophils are pro-inflammatory and N2 neutrophils are anti-inflammatory (183, 184).
Neutrophils were believed not to require extensive metabolic networks and to solely depend on glycolysis as they exhibit a relatively low transcriptional and translational activity where (185). Nevertheless, novel evidence suggests the implication of the TCA cycle, OXPHOS, PPP, FAO, and glutaminolysis in neutrophil metabolism, demonstrating a broad metabolic plasticity (185) (Table 3).
Eosinophils
Eosinophils are multifunctional phagocytic granulocytes that are typically associated with helminth infection and allergic disorders (206). A growing body of evidence suggests a homeostatic role for AT-resident eosinophils (178). AT-resident eosinophils are sustained by AT multipotent stromal cells-derived CCL11 and ILC2-derived IL-5 (207, 208). Indeed, AT-resident eosinophils produce IL-4 and IL-13 that drive macrophage M2 polarization, trigger Th2 differentiation, enhance B cell activation and promote metabolic homeostasis (209, 210). Eosinophil-deficient HFD-fed mice showed pronounced IR (209, 211). Moreover, it was shown that HFD-induced adiposity can be inhibited by increasing the number of eosinophils in mice (207, 209). Conversely, another study demonstrated an increase in gonadal AT eosinophils in HFD-fed mice, which was supposed to be regulated by increased CCL11 expression (211). Indeed, HFD-fed Δdb1GATA and IL-5-KO mice lacking eosinophils or almost having no gonadal AT eosinophils, exhibited impaired insulin sensitivity (207, 212). The forced increase of AT eosinophils in different models demonstrated an enhanced metabolic homeostasis (209, 213, 214). Indeed, IL-4-stimulated eosinophils induced M2 macrophage polarization, while oxidized LDL-mediated induction promoted M1 macrophage polarization (209, 215, 216). HFD-fed transgenic mice overexpressing eotaxin2 specifically in AT exhibited an increased eosinophil migration into AT that was accompanied by enhanced glucose tolerance (217). It is also worth mentioning that leptin promotes while adiponectin attenuates eotaxin-induced human eosinophil adhesion and chemotaxis (55, 218, 219). Nevertheless, enhancing AT eosinophil abundance is debated since several studies demonstrated no beneficial or even negative outcomes of this approach (220).
It was suggested that circulating eosinophils display a greater metabolic flexibility in comparison to neutrophils (200, 201). Further investigation into the metabolic rewiring of eosinophils (shown in Table 3) is required as emerging roles of eosinophils suggest a central modulatory function in AT homeostasis.
Mast Cells
Mast cells (MCs) are innate immune cells that originate from multipotent hematopoietic stem cells, then migrate to peripheral organs, where they undergo maturation giving rise to heterogeneous populations of mature MCs (21). MCs are enriched in visceral AT of mice and humans and are increased in settings of obesity and T2D, where they drive AT inflammation partly by enhancing macrophage infiltration (21, 221, 222). Indeed, the increased abundance of MCs in sub-cutaneous WAT of MetS subjects positively correlated with IR and markers of fibrosis and angiogenesis linking them to AT fibrosis and remodeling (223, 224). It was also suggested that MCs infiltration precedes the development of overt obesity (225). Indeed, the genetic ablation of MCs or manipulations impairing their function in HFD-fed mice resulted in decreased weight gain and reduced IR (221, 226, 227). Nevertheless, other studies utilizing different mouse models could not find a correlation between MC deficiency and the amelioration of AT inflammation (228, 229).
The activation of MCs is accompanied by a major metabolic reprogramming (Table 3). Indeed, these metabolic processes regulate MCs inflammatory cytokines and ROS production and IGE-mediated degranulation (203, 204, 230). A role for adipokines in the regulation of MC function has been recently revealed (56). Leptin and adiponectin were shown to exert opposite effects on MC polarization to promote a pro-inflammatory or an anti-inflammatory cytokine profile, respectively.
Metabolic Regulation and Adaptation of Tissue Resident and Infiltrating Lymphoid Cells
T Cells
T lymphocytes play major immunoregulatory and immunometabolic roles in AT homeostasis and dysfunction. Indeed, T cells were increased in VAT of obese mice and humans (231). Different effector T cells including helper T (Th) cells (T-bet-regulated Th1, GATA3-regulated Th2 and ROR-γt-regulated Th17) and cytotoxic T lymphocytes (CTLs) were shown to actively participate in obesity-associated WAT inflammation (178). Conversely, anti-inflammatory T cells such as regulatory T (Treg) cells and invariant natural killer T (iNKT) cells that reside in the AT under physiological conditions were reduced in obesity (232, 233). Based on the composition of the T-cell antigen receptors (TCR), T cells are categorized into two populations, αβ T and γδ T cells. αβ T cells are further classified, based on their surface markers, into CD4+ T cells and CD8+ T cells that upon activation, differentiate into Th cells and CTLs, respectively. Tregs emerge as a subset of CD4+ T cells that negatively regulate immune responses with a characteristic signature CD4+ CD25+ Foxp3+.
αβT Cells
αβ T cells represent the second largest immune population in WAT (178). In obesity, T cells are enriched in visceral AT of mice and humans, and are possibly recruited through a CCR5-CCL5-mediated interaction (231, 234). It was proposed that T cells infiltration precedes that of macrophages (235). However, this is debated as other studies did not arrive at a similar result (236, 237). CD4+ T cells represent the more abundant subtype in visceral AT and are further enriched in obesity (234, 238). In addition to their recruitment from the general circulation, both CD4+ and CD8+ T cells undergo clonal expansion in epicardial WAT (239, 240). MHCII expression was increased post-HFD feeding and mice deficient in MHCII exhibited greater insulin sensitivity (241). Nevertheless, inhibiting MHCII in HFD-fed mice did not improve glucose tolerance, an improvement seen with conventional T cells deficiency (242–244). The depletion of CD8+ cells in HFD-fed mice decreased the expression of TNF-α and IL-6 in epicardial WAT, which was accompanied by an enhanced glucose and insulin tolerance (235). Similarly, CD4+ Th1 cells were shown to drive AT inflammation and glucose intolerance (245). In fact, Th1 cells are similar in proportion to Tregs in lean conditions, while they occur at a higher frequency in comparison to other CD4+ cell subtypes in obesity (242, 246). Similarly, Th17 cells accumulated in sub-cutaneous WAT of insulin resistant individuals (247). IL-17-deficient mice displayed a better insulin and glucose tolerance, this was however abrogated by HFD feeding (248). Th2 cells were shown to have a beneficial effect on AT inflammation. Rag-deficient HFD-fed mice showed marked obesity and IR in comparison to their WT counterparts, a phenotype that was abrogated by the adoptive transfer of CD4+, but not CD8+ T cells (242). Indeed, most of CD4+ cells that homed to epicardial WAT expressed GATA3 (242).
Increasing evidence suggests a pivotal role for metabolic pathways in naïve and activated T cells maintenance and function (249). On activation, naïve T cells undergo major metabolic reprogramming, which highly depends on the duration and strength of TCR stimulation, including glucose metabolism, glutamine metabolism, and biosynthetic pathways (249). These metabolic alterations are summarized in Table 4.
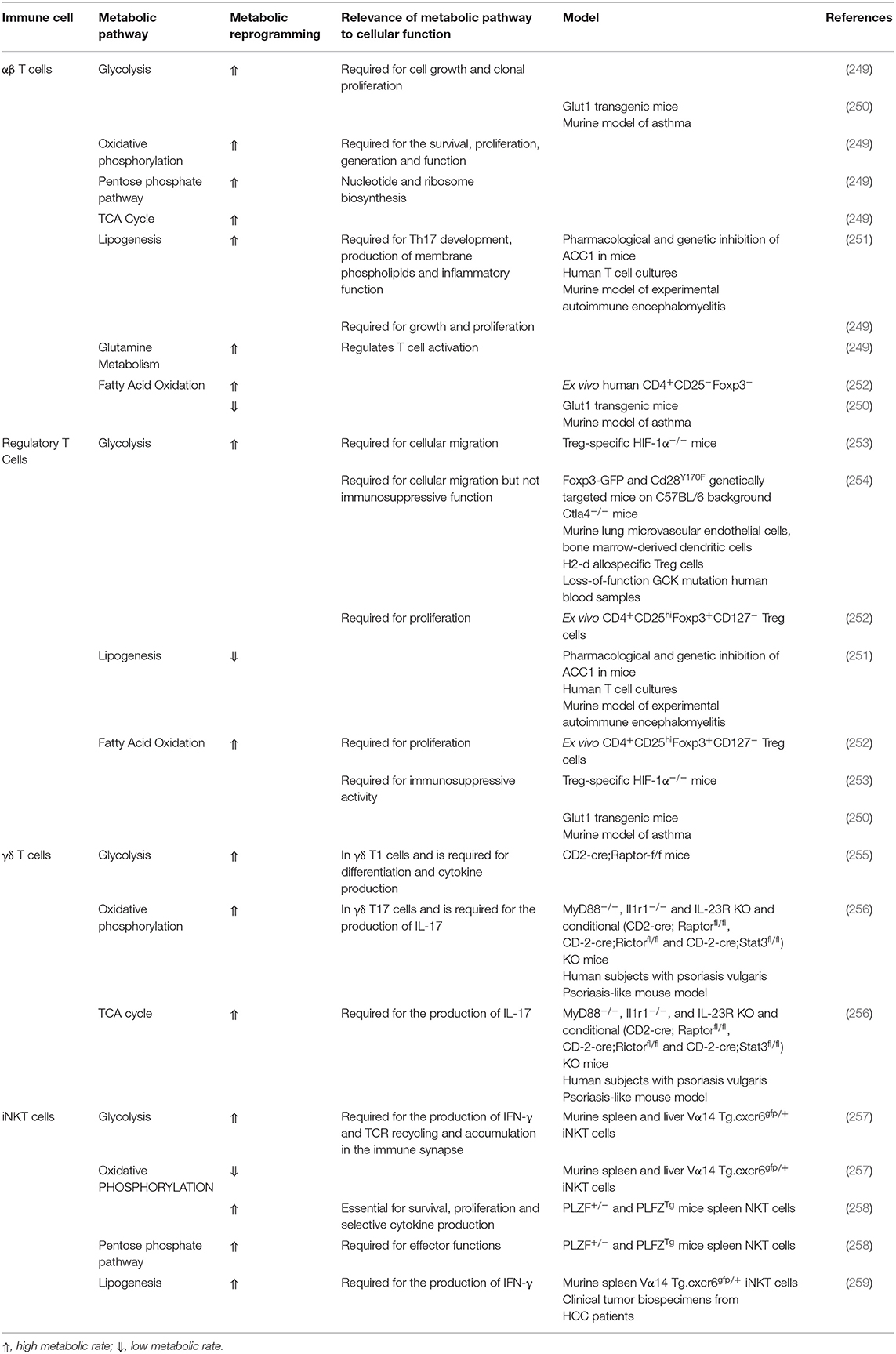
Table 4. Metabolic pathways required for T lymphocyte proliferation, differentiation, function, and activity.
Regulatory T Cells
Phenotypically-distinct AT-resident Tregs were reported to be enriched in visceral AT of lean mice, where they originate from enhanced proliferation rather than circulating Tregs infiltration (178). Visceral AT Tregs are markedly reduced in obese mice and humans, which promotes inflammation (232, 260, 261). Conversely, expanding Tregs in HFD-fed mice improved metabolic parameters (262). Indeed, PPARγ is essential for the accumulation and function of Tregs in AT of lean mice, where it collaborates with Foxp3 to induce their distinct phenotype, a phenotype abrogated by obesity through phosphorylating PPARγ at position Ser273 (263–265). Moreover, it was shown that IL-33/ST-2 axis amplified Tregs in visceral AT, which was accompanied by an attenuation of inflammation in obese mice (266, 267). Indeed, Tregs are highly enriched in visceral AT and to a lesser extent in sub-cutaneous WAT, where IL-33 is expressed (268–270). Other immune cells including γδ T cells, ILC2s and iNKT cells were also shown to regulate AT Tregs accumulation promoting insulin sensitivity (178). Conversely, IFN-γ producing Th1 cells inhibited AT Tregs and thus promoted IR (271).
The development, function, and phenotype stabilization of Tregs is metabolically regulated by several pathways highlighted in Table 4 (272). Moreover, leptin metabolism was shown to partially induce Tregs in vitro and mice deficient in leptin exhibited an increased proliferative ability of Tregs (273). Several lines of evidence suggest that Treg deficiency or insufficiency can lead to both T1D and T2D (274). Although studies demonstrated no difference in Treg frequency in diabetes, Treg phenotype and suppressive function were altered (275).
γδT Cells
γδ T cells can be classified into two major functional groups, IFN-γ-producing γδ T1 cells and IL-17-producing γδ T17 cells (178). In comparison to αβ T cells, γδ T cells harbor a restricted TCR repertoire, and the antigens recognized by these cells remain largely unknown. γδ T cells are as abundant as MCs, neutrophils, and CD8+ T cells in lean WAT, and are increased in response to HFD consumption (268, 276). Earlier investigations into the role of γδ T cells in AT demonstrated a pro-inflammatory function in HFD-fed mice (276). Nevertheless, circulating γδ T cells were decreased in obese subjects and were negatively correlated with BMI (277). More recently, γδ T cells of epicardial WAT were shown to comprise two distinct populations; PLZF−, CD3εlow, CD27+, RORγT−, T-bet+ γδ T cells that produce IFN-γ and PLZF+, CD3εhigh, CD27−, RORγT+, T-bet− cells that produce TNF-α and IL-17A (268). Indeed, mice deficient in PLZF+ γδ T cells or IL-17A KO mice exhibited reduced IL-33 levels and failed to accumulate ILC2s and Tregs in AT, suggesting that PLZF+ γδ T cell-produced cytokines modulate the number of IL-33-producing stromal cells. Moreover, PPARβ overexpressing mice, which exhibited lower αβ T cells and higher γδ T cells, were protected from HFD-induced AT inflammation and IR (278). Intriguingly, it was demonstrated that γδ T cells were initially increased in AT of ketogenic diet-fed mice but then decreased following the development of obesity (279). Table 4 highlights the fact that different γδ T cell subtypes exhibit a differential metabolic profile depending on their polarization (256).
iNKT Cells
iNKT cells represent a subset of the innate-like T lymphocytes, NKT cells, that recognize glycolipids presented on MHC-I-like family protein CD1d and express a conserved semi-invariant TCR that recognizes the prototypic ligand α-galactosylceramide (178). Indeed, adipose iNKT cells exhibit a distinct transcriptome from that of iNKT cells residing in other tissues with both anti-inflammatory and pro-inflammatory characteristics, secreting Th1-recruiting IFN-γ and Th2-recruiting IL-4 (280, 281). iNKT cells are suggested to modulate WAT immunity in setting of leanness and obesity (282, 283). iNKT cells were enriched in visceral AT of humans and mice and in mouse sub-cutaneous WAT, where CD1d-expressing M2 macrophages and adipocytes promptly activate iNKT cells (283–285). WAT iNKT cells contribute to metabolic homeostasis through the secretion of IL-2 and IL-10, which regulate M2 macrophages and Tregs function, respectively (283). In obesity, the number of AT iNKT cells decline with WAT inflammation (284–288). Also, iNKT cells were shown to be dysfunctional in patients suffering from obesity or T2D exhibited by a diminished capacity to secrete IL-2 (289). Alternatively, HFD-fed mice deficient in AT iNKT cells were prone to obesity and IR which were reversed upon the adoptive transfer of iNKT cells (290, 291). Importantly, the hypoxic condition of expanding AT favors the upregulation of HIF-1α (10, 292). iNKT cells respond to hypoxia by upregulating the CD1d-mediated cytokine response (293). Furthermore, leptin activates iNKT cells resulting in their anergy and PD-1 upregulation (294, 295). Moreover, the inhibition of the synthesis of glucosylceramide, which can be presented on CD1d, in adipocytes was shown to impair iNKT cell activity and cytokine production (296). Several lines of evidence suggest that iNKT cell metabolism contributes to their development and functioning (shown in Table 4).
Innate Lymphoid Cells
Innate lymphoid cells have been previously regarded as enigmatic lymphocyte-like cells that possess the morphological features of a lymphocyte in the immature state but lack its surface markers, and are thus described as “lineage negative.” ILCs include three transcriptionally-defined groups; Tbet-dependent ILC1s (which include NK cells) that secrete IFN-γ and TNF-α, GATA3-dependent ILC2s that secrete IL-5/IL-13 and IL-10, ROR-γt-dependent ILC3s that secrete IL-17A/IL-22 and finally Id3-dependent ILCregs that produce IL-10 and require autocrine TGF-β1 (297). Importantly, recent evidence demonstrated the presence of all ILC subsets in different AT depots, where they are implicated in AT immune responses (298).
Adipose Tissue ILC1s and NK Cells
AT-resident ILC1s and NK cells are highly enriched in WAT in both humans and mice, and further increase at the setting of obesity and T2D, where they positively correlated with IR (299, 300). ILC1s and NK cells drive AT inflammation in obesity by secreting IFN-γ and promoting M1 macrophage polarization (299, 301). However, the enrichment of ILC1s and NK cells in WAT at steady state suggests homeostatic roles (302). Indeed, ILC1s and NK cells were shown to regulate the survival of ATMs by killing AT M2 macrophages (302). Nevertheless, the physiological relevance of this regulation is questioned since mice and human deficient in ILC1s do not display major metabolic derangements (303, 304). ILC1s and NK cells exhibit a distinct metabolic program following activation. These alterations drive cellular functions, cytotoxicity, and inflammatory cytokines production (305–307). Table 5 highlights metabolic pathways implicated in ILC1s and NK cells activity and function.
Adipose Tissue ILC2s
ILC2 are key regulators of lean AT homeostasis (298). ILC2s are enriched in visceral AT, where they represent the predominant producers of IL-5 and IL-13 which are essential for the recruitment of eosinophils (207). Indeed, the recruitment and proliferation of AT ILC2s is driven by IL-33 whose origin is still debated (297, 316). Moreover, AT ILC2s express ICOSL, which signals to Tregs through ICOS and drive their accumulation in visceral AT at steady state, a process abrogated in obesity by IFN-γ (317). Moreover, ILC2s upregulate OX40L following their stimulation by IL-33 which is essential for the recruitment of Treg cells into the AT (318). The exact mechanisms leading to the reduction of AT ILC2s number in obesity is not well-understood. Nevertheless, one possible mechanism includes the expansion of ILC1s where ILC1-derived IFN-γ antagonizes ILC2s (317). Another mechanism implicates IL-12 in driving the conversion of ILC2s to ILC1s in the context of diet-induced obesity (DIO) (319). Metabolic pathways in ILC2s govern their proliferation and function. Shifting the balance between OXPHOS and glycolysis toward glycolysis impairs the development and function of ILC2s (311, 312). Metabolic pathways implicated in ILC2s metabolism are summarized in Table 5.
B Cells
B lymphocytes are further subdivided into 2 major classes; B-1 and B-2 depending on their developmental origin, microenvironmental niches and the requirement of Th cells to produce antibodies (320). B-1 cells are further stratified to B1-a and B1-b cells, which differ by their surface expression of CD5. B cell-secreted IL-10, IL-35 and TGF-β are characteristics of the functionally-distinct Breg cells that can derive from both B-1 and B-2 cells. Breg cells suppress Th1 and Th2 polarization, and inhibit macrophage and dendritic cell activation and cytokine production. B-1 and B-2 cells coexist in perivascular AT, epicardial WAT and BAT, where the B1:B2 ratio is higher than that in sub-cutaneous WAT but yet varies greatly in a depot-specific manner (321, 322). B cells are among the first immune cells to infiltrate AT following the consumption of a HFD consistent with an increased IR, where AT B-2 cells are thought to promote inflammation (323–327). B cell abundance also increases in BAT following the consumption of a HFD, where their role is poorly understood (328). Indeed, B cells global deficiency in mice attenuated HFD-induced AT inflammation and reduced IR (324, 325). Consistently, circulating B cells from obese, diabetic and obese diabetic individuals produced higher amounts of pro-inflammatory cytokines in comparison to healthy individuals (329, 330). In addition, B cell-produced pro-inflammatory immunoglobulins were elevated in visceral AT of obese mice activating macrophages to secrete inflammatory cytokines (324, 331). Contrary to B-2 cells, B-1 cell-derived natural IgG and anti-inflammatory cytokines block AT inflammation and improve glucose tolerance through inducing M2 macrophage polarization and increasing their production of IL-10, while reducing their production of IL-6 and TNF-α (321, 322, 332–335). The number of Breg cells, which are present in AT, is reduced in diabetic patients in comparison to healthy donors (336, 337). Moreover, Breg cells from diabetic patients secrete less IL-10 (329, 330). Indeed, the adoptive transfer of Breg cells ameliorated AT inflammation and IR in DIO mice (336). Different B cell subsets exhibit distinctive metabolic profiles depending on their particular microenvironments and thus, careful interpretation of B cell metabolic data is pivotal (338). The metabolic pathways implicated in B cell metabolism are summarized in Table 6. Metabolic rewiring of different subsets of B cells and plasma cells have also been discussed in details elsewhere (338).
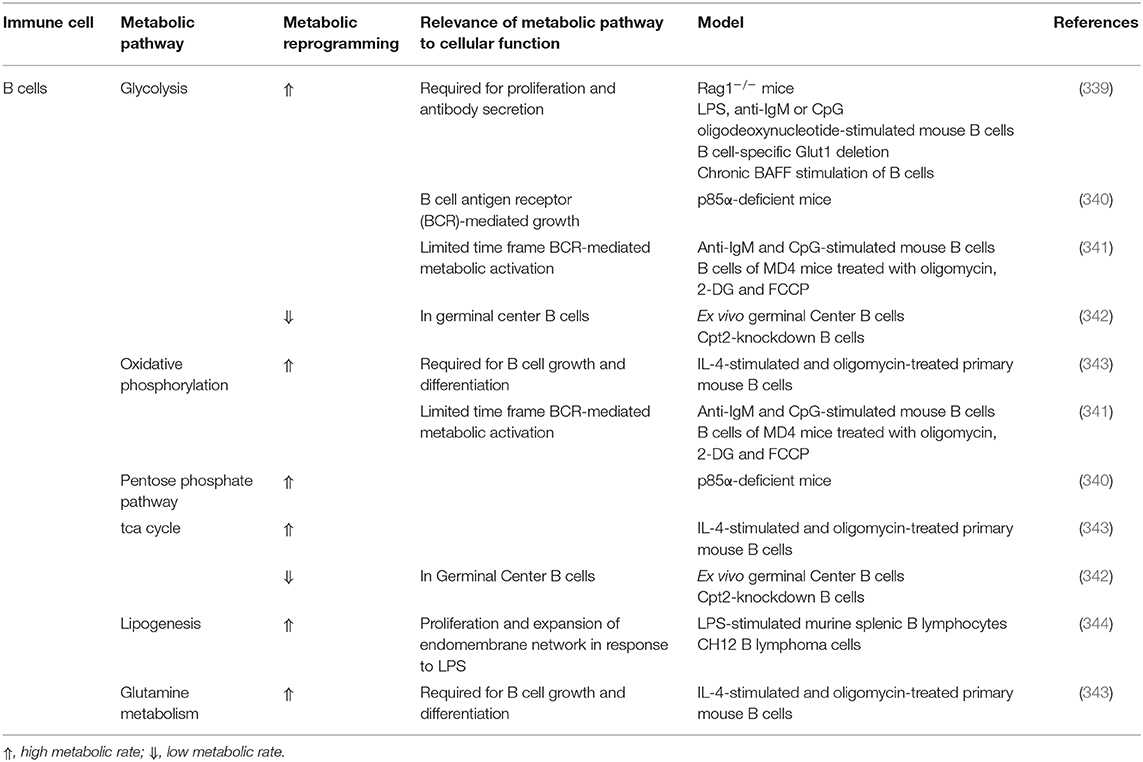
Table 6. Metabolic pathways implicated in B lymphocyte proliferation, differentiation, activation, and function.
Immune Cell Contribution to Depot-Specific Adipose Tissue Inflammation
While AT is broadly classified in WAT and BAT, WAT is further divided into several distinct depots that differ in their properties and microenvironments including subcutaneous (scWAT) and visceral WAT (VAT) depots. The latter includes epicardial (EpiCAT), perivascular (PVAT), epidydimal (EpiWAT), mesenteric (MAT) and perirenal (PRAT) AT. VAT has been extensively studied due to the association between visceral obesity and the emergence of CVD risks (345, 346). It was demonstrated that scWAT exhibits a greater potential than VAT to undergo beiging, a process by which white adipocytes become brown-like and participate in energy dissipation (2, 347). Indeed, the induction of VAT beiging has been largely regarded as an approach to curb obesity and its accompanying metabolic and cardiovascular derangements (348, 349). Nevertheless, several visceral adipose depots including PVAT and EpiCAT intrinsically possess a beige phenotype and the implications of thermogenic induction in these particular tissues on cardiovascular functioning is not yet well-characterized, especially that the immune landscape of these tissues is less known (33). In the below sections, an overview of changes in immune cell function, population, and activity, as well as the alterations in adipokine and cytokine profile across different depots will be provided with particular emphasis on PVAT and EpiCAT due to their relevance to CVD in metabolic impairment.
Subcutaneous Adipose Tissue
The mass of scWAT is positively correlated with BMI in obese subjects (350). A dysregulated scWAT in patients with MetS exhibits higher macrophage infiltration and CLS formation, which is accompanied by a dysregulated adipokine profile (351). Indeed, scWAT inflammation is linked to the development of IR (214, 352). Interestingly, a sustained scWAT low-grade inflammation extending beyond weight loss was reported (353, 354). This sustained inflammation has been attributed to the accumulation of effector memory T cells (354). This contradicts with recent reports that demonstrated VAT but not scWAT inflammation as a manifestation of obesity (355, 356). Such discrepancies may arise from differences in diet composition and the duration of HFD-feeding. The general consensus however is that scWAT inflammation participates in driving IR and the MetS in obese subjects with the implication of various immune cells.
It was shown that an increased abundance of eosinophils in scWAT of MetS patients, is accompanied with IR, tissue fibrosis, and adipokine dysregulation (357). Macrophage infiltration and CLS formation are also elevated in scWAT of obese and diabetic patients (351, 358). Interestingly, an accumulation of M2 macrophages in scWAT but not in VAT of obese patients is associated with inflammation limitation (359). Moreover, the abundance of MCs is increased in scWAT of MetS subjects and is significantly correlated with IR, leptin, IL-1β, IL-6, and the activities of MAPK and NF-κB in circulating monocytes (224). Similar to eosinophils, scWAT MCs are correlated with markers of AT fibrosis and angiogenesis (224). The number of total dendritic cells is reduced but that of pDC increases in the scWAT of subjects with T2D implicating pDCs in scWAT low-grade inflammation (360).
Epidydimal Adipose Tissue
EpiWAT is a metabolically active visceral fat pad, which is anatomically attached to the testis and epididymis, then stretches out toward the diaphragm (361). EpiWAT low-grade inflammation is thought to contribute to the initiation of IR, MetS and its related cardiovascular derangements (362). Indeed, macrophage infiltration and accumulation in EpiWAT is at the core of this inflammation (222, 362). Interestingly, Chronic DIO eventually leads to decreased EpiWAT mass, which correlated negatively with body weight and was associated with a widespread of CLSs and MCs, together with an impaired adipokines gene expression, which could be attributed to the increased abundance of dysfunctional or dead fat cells (361).
EpiWAT neutrophils of HFD-fed mice exhibited an increased IL-6 expression in EpiWAT due to an adipocyte-contact-dependent activation of NF-κB (182). Moreover, it was suggested that HFD-induced EpiWAT fibrosis was attributed to macrophages and MCs (222). MCs were also shown to have a role in HFD-stimulated adipocytes senescence in EpiWAT (222). It has also been shown that the consumption of a HFD induces an elevation of NK count and the production of pro-inflammatory cytokines in EpiWAT at an early phase of obesity induction, which was linked to increased fasting glucose, insulin levels and ATMs count (301). Interestingly, NK-mediated EpiWAT derangements were IFN-γ-dependent at early stages and then became TNFα-dependent (363). Moreover, studies revealed an increase in B cells in EpiWAT of HFD-fed mice, which was associated with IR (320). These B cells were shown to drive VAT inflammation by regulating the activity of VAT T cells and macrophages through the production of pro-inflammatory cytokines including IL-6 and INF-γ (320). HFD induces an increase of the immature DCs count in EpiWAT, which has a role in adipose tissue inflammation through triggering a parallel increased production of Th17 cells via promoting an excessive production of IL-6, TGF-β, and IL-23 (162).
Perivascular Adipose Tissue
PVAT environs most of the blood vessels including the aorta and coronary and subcutaneous small arteries. PVAT play a crucial role in supporting blood vessels by maintaining vasomotor tone and insulating them from surrounding environment (364). It communicates with neighboring VSMCs and ECs in a paracrine manner through the production of various adipokines influencing the vascular tone (365). Indeed, PVAT-derived adipokines infiltrate into vasculature and serve as either vasodilators or vasoconstrictors. As such, an adipokine profile imbalance in PVAT toward proinflammatory adipokines is suggested to drive vascular derangements in metabolic disorders through the disruption of PVAT anticontractile activity. Furthermore, PVAT inflammation has been reported in states of nutritional excess through the recruitment of pro-inflammatory cells. This interaction provides the framework by which PVAT inflammation impairs vascular function (366–369).
PVAT infiltrating immune cells include macrophages, T cells, NK cells, and DCs that produce both inflammatory and anti-inflammatory cytokines, depending on the adipokine profile shifts (363, 370–373). Interestingly, both B cell subtypes occur in PVAT, where B-2s promote the development of diet-induced atherosclerosis and B-1s inhibit it by reducing MCP-1 and TNF-α production (334, 374, 375). However, PVAT possesses a higher B1:B2 ratio and thus, B cells have an anti-inflammatory role in PVAT (322). Recent studies done on rat PVAT inflammation demonstrated that the increased production of IL-1β and TGF-β1 was correlated with a reduced AMPK activity and endothelial relaxation impairment (32). On the other hand, there was an increase in AT hypertrophy, oxidative stress, and Rho-associated kinase (ROCK)-mediated Ca2+ sensitivity (32, 376). Other factors associated with PVAT inflammation include adipocyte derived MCP-1 and low-density lipoprotein receptor related protein-1 (377, 378).
On the other hand, several PVAT adipokines were reported to have an ameliorative effect on vascular function. Indeed, the treatment with the adipokine irisin normalizes the reduced anti-contractile properties of aortic PVAT in obese mice (379). Adiponectin was also shown to attenuate vascular inflammation and atherosclerosis possibly through blocking NF-κB signaling and downregulating the expression of VCAM-1 and ICAM-1 (380–383). Moreover, PVAT-derived adiponectin normalizes endothelial function partly through enhancing endothelial eNOS phosphorylation (384). Similarly, omentin was suggested to have an anti-atherogenic potential in concert with circulating adiponectin (385). In fact, omentin was demonstrated to restore endothelium-dependent relaxation by inhibiting ROS and enhancing NO production in high glucose-treated endothelial cells (386, 387).
Other PVAT-derived adipokines include vaspin, which modulates ER stress by upregulating the phosphorylation of Akt and AMPK (388), and apelin, which maintains vascular structure by upregulating endothelial NO (389, 390). Despite the positive correlation between serum leptin levels and vascular calcification (391), PVAT-derived leptin was reported to exert an anticontractile effect when in synergy with other vasorelaxing factors (392, 393). Nevertheless, PVAT-derived leptin also promotes VSMC phenotypic switch by increasing the phosphorylation of p38 MAPK (394–396). Chemerin was demonstrated to promote aortic atherosclerosis by promoting NF-κB signaling and p38 MAPK phosphorylation (397, 398). Additionally, and via the activation of NLRP3 signaling, the adipokine visfatin was shown to induce vascular endothelial dysfunction and tissue inflammation (399, 400). Finally, resistin was demonstrated to activate the renin-angiotensin system inducing hypertension (401, 402). Changes in PVAT adipokine environment and immune cell activity brought about by metabolic dysfunction is depicted in Figure 2.
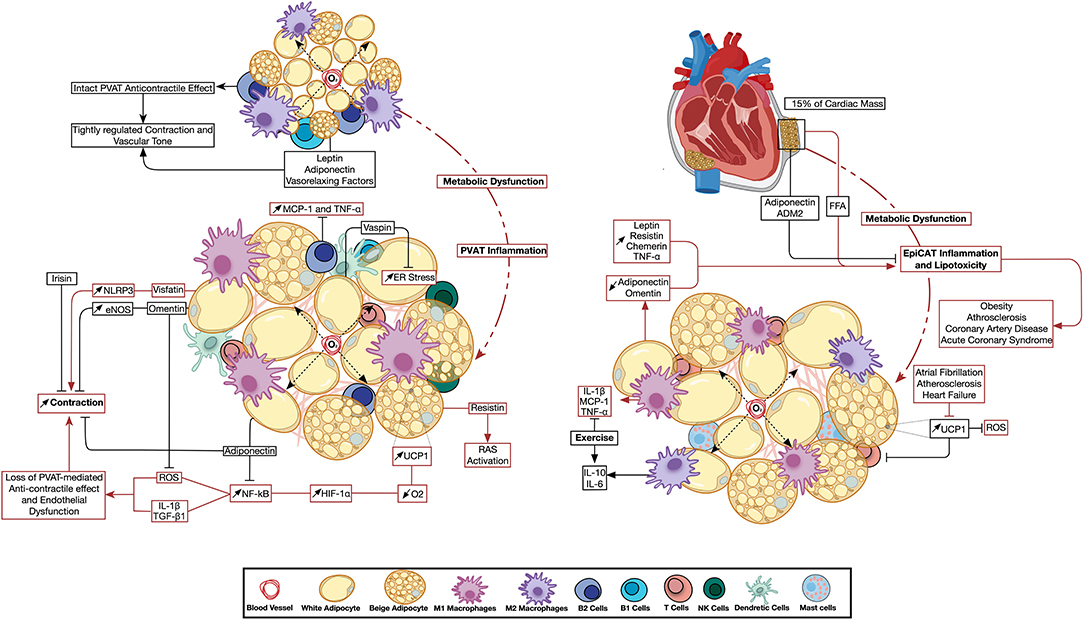
Figure 2. Perivascular and epicardiac adipose tissue dysfunction: the emerging role of immune cell and adipokine profile dysregulation. Metabolic impairment triggers changes in PVAT (left) and EpiCAT (right) adipokine environment and immune cell activity. Inflammation has a direct effect on the neighboring vascular and cardiac tissue. Changes in UCP1 expression were reported to have opposite effects in either depot. Pathways active in basal PVAT and EpiCAT homeostasis are depicted in black arrows, while those activated during inflammation are shown in red. ADM2, Adrenomedullin-2; eNOS, Endothelial Nitric Oxide Synthase; EpiCAT, Epicardial Adipose Tissue; ER Stress, Endoplasmic Reticulum Stress; HIF-1α, Hypoxia-induced Factor 1 Alpha; IL, Interleukin; MCP-1, Monocyte Chemoattractant Protein 1; NF-κB, Nuclear Factor Kapp-light-chain-enhancer of Activated B cells; NLRP3, NLR Family Pyrin Domain Containing 3; O2, Oxygen; PVAT, Perivascular Adipose Tissue; RAS, Renin Angiotensin System; ROS, Reactive Oxygen Species; TGF-β, Transforming Growth Factor Beta; TNFα, Tumor Necrosis Factor Alpha; UCP1, Uncoupling Protein 1.
Epicardial Adipose Tissue
EpiCAT is located in the atrioventricular and interventricular heart grooves and plays a role in providing FA to the myocardium. Indeed, EpiCAT represents 15% of the cardiac mass, and as the epicardial fat increases, the ventricles and the epicardial surfaces get covered by EpiCAT. Moreover, EpiCAT surrounds the adventitia of coronary arteries and plays a cardioprotective role during metabolic and mechanical insults (33). The endocrine function of EpiCAT has witnessed extensive investigation as EpiCAT dysfunction was implicated in cardiovascular diseases. It regulates FA homeostasis to prevent lipotoxicity, while secreting anti-inflammatory and anti-atherogenic adipokines under healthy conditions (403, 404). However, EpiCAT alters its adipokines to release FA and pro-inflammatory cytokines under metabolic insults (404, 405). Several studies reported the expression of numerous adipokines in EpiCAT including adiponectin, omentin, adipsin, leptin, resistin, visfatin, chemerin and adrenomedullin (406). While the EpiCAT expression of resistin, leptin and TNF-α increase in obesity, the expression of adiponectin is markedly reduced (407, 408). In addition, EpiCAT adiponectin expression is also decreased while that of leptin increased in CAD patients (409, 410). Importantly, the administration of recombinant adiponectin can reverse the harmful effects of dysfunctional EpiCAT-derived factors (409). Another study demonstrated a differential expression of adiponectin, visfatin, leptin, chemerin and vaspin in periaortic, pericoronary and apical EpiCAT, where these adipokines were correlated with either aortic or coronary atherosclerosis (411). Furthermore, the expression level of omentin was decreased in CAD patients (412). Importantly, it was suggested that exogenous omentin supplementation might support a cardioprotective role through its anti-inflammatory effect on EpiCAT (413). Increased levels of resistin in EpiCAT were also reported in patients with advanced coronary atherosclerosis and patients with acute coronary syndrome (414, 415). Similarly, higher chemerin levels were observed in CAD patients which was correlated with an increased EpiCAT volume (416, 417). Interestingly, the level of chemerin was positively correlated with the severity of coronary atherosclerosis in CAD patients (416).
Immunohistochemistry done on EpiCAT confirmed the presence of infiltrating CD3+ T cells, tryptase+ mast cells, and CD68+ macrophages. These immune cells have been shown to be unique to EpiCAT when compared to scWAT (418). A study showed that patients with coronary artery disease (CAD) had a significant increase in macrophage infiltration into EpiCAT compared to individuals without CAD (419). Furthermore, the levels of IL-6, IL-1β, MCP-1, and TNF-α were higher in EpiCAT compared to scWAT (418). Changes in EpiCAT adipokine environment and immune cell activity brought about by metabolic dysfunction is depicted in Figure 2.
Mesenteric Adipose Tissue
MAT is located between the gut and the liver. Several lines of evidence associate MAT expansion to an elevated risk for the development of peripheral and central IR as well as CVDs (327, 420). In response to HFD consumption, MAT adipocytes secrete high amounts of MCP-1, which intensifies the inflammatory response by modulating macrophage infiltration driving IR and atherosclerosis (421). Similar to MCP-1, GM-CSF is highly expressed in MAT of obese animals. It has been shown that both GM-CSF and B cells play an important role in the activation and accumulation of macrophages besides the production of pro-inflammatory cytokines in MAT of HFD-fed animals (327, 422). It is worth noting that B cells are among the earliest immune cells infiltrating MAT in HFD-induced AT inflammation models (327). Likewise, an increased accumulation of mast cells was shown in MAT of HFD-fed mice, which was associated with tissue fibrosis and IR. These alterations occurred coincidently with the progression of obesity and diabetes (225).
Perirenal Adipose Tissue
PRAT, the AT surrounding the kidney, was previously assumed to merely mechanically support the kidneys. However, several studies postulated that not only PRAT has a pronounced role in regulating kidney function but is also associated with cardiometabolic risk factors. Clinical studies suggest that excess PRAT is associated with higher risk of CVDs (423, 424). The weight of PRAT has the highest partial correlation coefficient with CVDs among other AT (425). Indeed, excess PRAT is believed to contribute to the decrease in kidney function, regardless of obesity, in hypertensive patients (426). A recent study reviewed the possible mechanisms of PRAT in regulating CVDs including neural, humoral, and direct kidney related regulation (425). PRAT is shown to synthesize and secrete adipokines and several pro-inflammatory cytokines (425, 427). PRAT in pigs with obesity-related metabolic dysfunction showed elevated levels of pro-inflammatory macrophage infiltration and TNF-α expression (428). Moreover, excess PRAT secrets leptin which in turn activates MAPK pathway and further exacerbates renal vascular and endothelial damage (429). An interesting study in rats have shown that injecting Leptin directly into PRAT activated the adipose afferent reflex without changing the systemic sympathetic activity, indicating a direct regulation of cardiovascular function by PRAT (430). Interestingly a study on diabetic fatty rats found that the inhibition of PRAT inflammation, mainly inhibiting IL-6, IL-1b, and TNF-α, reduced renal inflammation and alleviated diabetic nephropathy (431). As such PRAT inflammation is assorted with adverse cardiometabolic risk factors and is a main predictor of CVD.
The Adipose Immune System as O Regulator of Adaptive Thermogenesis
UCP1-Dependent and Independent Thermogenesis
Uncoupling protein 1 (UCP1) is an inner mitochondrial membrane protein that uncouples oxidative phosphorylation from the production of ATP through a FA/H+ symport mechanism (2). UCP1 expression is mainly driven through β3-adrenoreceptors (β3-AR) stimulation by sympathetically and non-sympathetically produced norepinephrine in thermogenically active adipocytes. Although being the most efficient and qualitatively significant thermogenic effector, it was demonstrated that UCP1 is dispensable for cold-induced and diet-induced thermogenesis. Therefore, it was proposed that less-efficient thermogenic pathways downstream of β3-ARs also contribute to adaptive thermogenesis (432).
Creatine cycling, that is the phosphorylation of creatine by creatine kinase and its subsequent hydrolysis, participates in energy transfer from ATP-rich to ATP-poor cellular regions (2). Creatine futile cycling appears to occur in all fat depots and blocking creatine cycling promotes obesity in HFD-fed mice (2). Lipolysis/re-esterification cycling has also been proposed to mediate adaptive thermogenesis based on the ATP demand of triacylglycerol synthesis (2). This pathway proposes that adipocytes break down fat and subsequently re-esterifies FAs by way of glycerol 3-phosphate. Importantly, It was also shown that triglyceride/FA cycling is induced in WAT upon HFD feeding (433).
A role for calcium transport in non-shivering thermogenesis has also been proposed (2). Calcium sequestration in the sarcoplasmic (SR) and endoplasmic (ER) reticulum is mediated by the SR/ER calcium ATPase (SERCA) pump. SERCA activity in the AT is regulated by phospholamban (PLB) (434). Interestingly, it was shown that PLB is upregulated in UCP1-deficient beige fat with no difference in the expression of SERCA suggesting compensatory thermogenesis (435).
Finally, the UCP1-independent proton leak by the ubiquitously expressed inner membrane protein, mitochondrial ADP/ATP carrier (ACC), that is initiated at high membrane potential, contributes to adaptive thermogenesis (2).
Adaptive Thermogenesis Across Adipose Depots
Brown Adipose Tissue
The first insights into the implication of BAT in thermogenesis and its contribution to energy expenditure started with the demonstration of a reduced GDP binding to BAT mitochondria of cold-exposed obese ob/ob mice relative to lean siblings (436). Then, Rothwell and Stock observed an increased sympathetic activity in BAT following overnutrition in rats (437). The identification of human BAT and the subsequent observations that a reduced BAT level induces obesity ignited investigation into BAT-mediated non-shivering thermogenesis. In comparison to WAT, which is more prone to inflammation than BAT (31), relatively little is known about the processes driving BAT chronic inflammation. However, increasing evidence suggests that BAT inflammation alters its thermogenic activity through the induction of IR (438, 439). Although mainly composed of brown adipocytes and their precursors, BAT also contains a variety of immune cells such as neutrophils, macrophages and lymphocytes (440, 441). Chronic inflammation of BAT was associated with a shift of BAT immune cells where M1 macrophages drive BAT whitening (442, 443).
Subcutaneous Adipose Tissue
Cold exposure and β3-AR stimulation induced the expression of UCP1 in scWAT of humans (444, 445). Nevertheless, despite the increased UCP1 expression in scWAT, cold acclimation was shown to reduce mitochondrial uncoupling-mediated fat oxidation in inguinal scWAT, while increasing the capacity to export FAs (446). Indeed, the consumption of HFD induced scWAT inflammatory and immune responses (447). These derangements were reversed by intermittent fasting, which increased the expression of UCP1, β3-ARs and adiponectin, while it attenuated the expression of pro-inflammatory and pro-apoptotic markers in scWAT (448). In an AMPK gain of function mutant mice, scWAT exhibited a morphological similarity to brown adipocytes with no detectable UCP1 expression but increased energy expenditure suggesting the activation of UCP1-independent thermogenesis (449). It was demonstrated that PPARγ agonism induced scWAT browning, while PPARγ deletion in inguinal scWAT inhibited thermogenesis and was associated with IR (450, 451).
Perivascular Adipose Tissue
The peculiarity of PVAT, being a hybrid AT and especially the resemblance of aortic PVAT to classical BAT in morphology and UCP1 expression, suggests that PVAT possesses a similar thermogenic potential (369). Indeed, it was shown that PVAT deletion resulted in a reduction of whole body temperature (452). The proximity of PVAT to the vascular wall suggests a possible implication of PVAT thermogenic processes on the pathophysiology of vascular diseases (33). We recently identified an increased expression of UCP1 in PVAT of HFD-fed rats, which was associated with localized PVAT inflammation contributing to MetS-associated vascular dysfunction (32). The targeting of PVAT UCP1 was also put forward as means to limit its detrimental effect on PVAT hypoxic predisposition (33). Such a proposition was made based on an assumed exaggerated oxygen consumption triggered by increased UCP1 expression and further complicated by the observed adipocyte hypertrophy in a combination of events less likely to occur in other adipose depots. However, increased UCP1 expression is typically viewed as beneficial where it serves as a route of energy assimilation that might be of value in diabetes and obesity. Yet, many of the tools shown to increase adipocyte glucose consumption and increased UCP1 expression in vitro failed to produce any effect when used in vivo, and even resulted in an opposite effect of decreased UCP1 expression (453, 454).
Epicardial Adipose Tissue
EpiCAT adipocytes express genes and secrete adipokines that are involved in thermogenesis (455). Adult human EpiCAT was shown to possess molecular features characteristic of beige adipocytes with relatively abundant expression of UCP1 (456). Opposite to findings in PVAT, an increased expression of UCP1 in EpiCAT was associated with a downregulation of ROS production and immune response (457, 458). Indeed, EpiCAT thermogenic activity was impaired in patients suffering from atrial fibrillation and heart failure with reduced ejection fraction (459, 460). Moreover, during the progression of atherosclerosis, EpiCAT was shown to undergo a phenotypic conversion from BAT to WAT, which further promoted the development of atherosclerosis (461). Nevertheless, exploiting EpiCAT browning for the treatment of CVDs remains controversial (33). As such, detailed examination of the role of thermogenesis modulation in PVAT and EpiCAT is required since both depots are particularly pertinent to the development of CVD in metabolic dysfunction.
Perirenal Adipose Tissue
Human PRAT has been shown to possess unilocular and multilocular UCP1+ adipocytes (462, 463). Indeed, several studies associated PRAT browning with aging and the female sex (464, 465). Also, bigger unilocular adipocytes with reduced UCP1 expression were detected in the PRAT of hypertensive patients (466).
Epidydimal Adipose Tissue
EpiWAT expresses UCP1 in rats age-dependently (467). The chronic agonism of PPARγ in EpiWAT promoted UCP1 expression and WAT browning (347). Indeed, the ectopic expression of very low levels of UCP1 in EpiWAT was shown to reverse IR in obese mice and epididymal beige adipocytes were shown to employ prominent creatine cycling (468, 469). Cold exposure improved metabolic dysfunction in obese mice through activating BAT thermogenesis and inducing EpiWAT browning (470). Moreover, cold-induced browning of VAT and improvement of insulin sensitivity were blunted following the knockdown of UCP1 in EpiWAT (471). Additionally, housing mice at room temperature induced EpiWAT thermogenesis, which was associated with a decreased M1 macrophage infiltration and improved insulin sensitivity (472). It was also shown that infused M2 macrophages in obese rats homed to EpiWAT reversing the M1 macrophage-dominant phenotype, enhancing UCP1 expression and ameliorating IR (473).
Mesenteric Adipose Tissue
It was demonstrated that β3-AR agonism in HFD-fed rats not only decreased the mass of WAT but also induced the appearance of multilocular, UCP1+ adipocytes in MAT (474, 475). These early observations indicated that MAT can be thermogenically induced. Indeed, cold exposure induced a sympathetic response in MAT of rats, evidenced by an increased level of tyrosine hydroxylase (476). Importantly, chronic cold exposure induces non-sympathetic catecholamine production leading to an increased level of NE in addition to the stimulation of M2 macrophage infiltration, pro-inflammatory cytokines reduction, and UCP1 induction (476, 477).
Adipose Immune System and Adaptive Thermogenesis
Adipose Immune Cells and β3-AR Stimulation
β-AR stimulation is pivotal to the induction of thermogenesis. Sympathetically-released NE stimulates the release of adipokines and FGF21 from adipocytes, promoting PGC1α and UCP1 expression, oxidative metabolism, and mitochondrial biogenesis (478, 479). FGF21 also induces the release of CCL11 in murine scWAT, which promotes the recruitment of IL-4-secreting eosinophils and the proliferation of PDGFRα+ beige adipocytes in an IL-4Rα-dependent manner (480, 481). Moreover, eosinophils and ILC2s were shown to induce β3-AR signaling through IL-4/IL-13-dependent induction of tyrosine hydroxylase expression in ATMs, promoting the release of NE (215, 482). Also, the selective deletion of Mecp2 in BAT macrophages reduces UCP1 expression as a result of impaired innervation (441). Nevertheless, recent evidence suggests that ATMs are not likely to contribute to the induction of adaptive thermogenesis by directly producing NE (483). Sympathetic neuron-associated macrophages increased in HFD-fed mice AT and were recently shown to express the NE transporter, SLC6A2 and the NE degrader, monoamine oxidase (MAO), where the inhibition of SLC6A2 increased AT thermogenesis (484). Conversely, CLS-associated ATMs were shown to phagocytose white adipocytes and secrete chemokines that drive the recruitment of beige adipocyte precursors (263). Tregs were also shown to enhance β3-AR signaling in scWAT but not in VAT of female and to a lesser extent in male mice by suppressing M1 and inducing M2 macrophages (485). γδT cells were also shown to promote AT innervation by driving the expression of TGFβ1 in parenchymal cells via the IL-17 receptor C (IL-17RC), where the ablation of IL-17RC signaling pathway or γδT cells impaired sympathetic innervation and thermogenesis (486). The interaction among immune cells, adipocytes, and sympathetic nerve terminals is summarized in Figure 3.
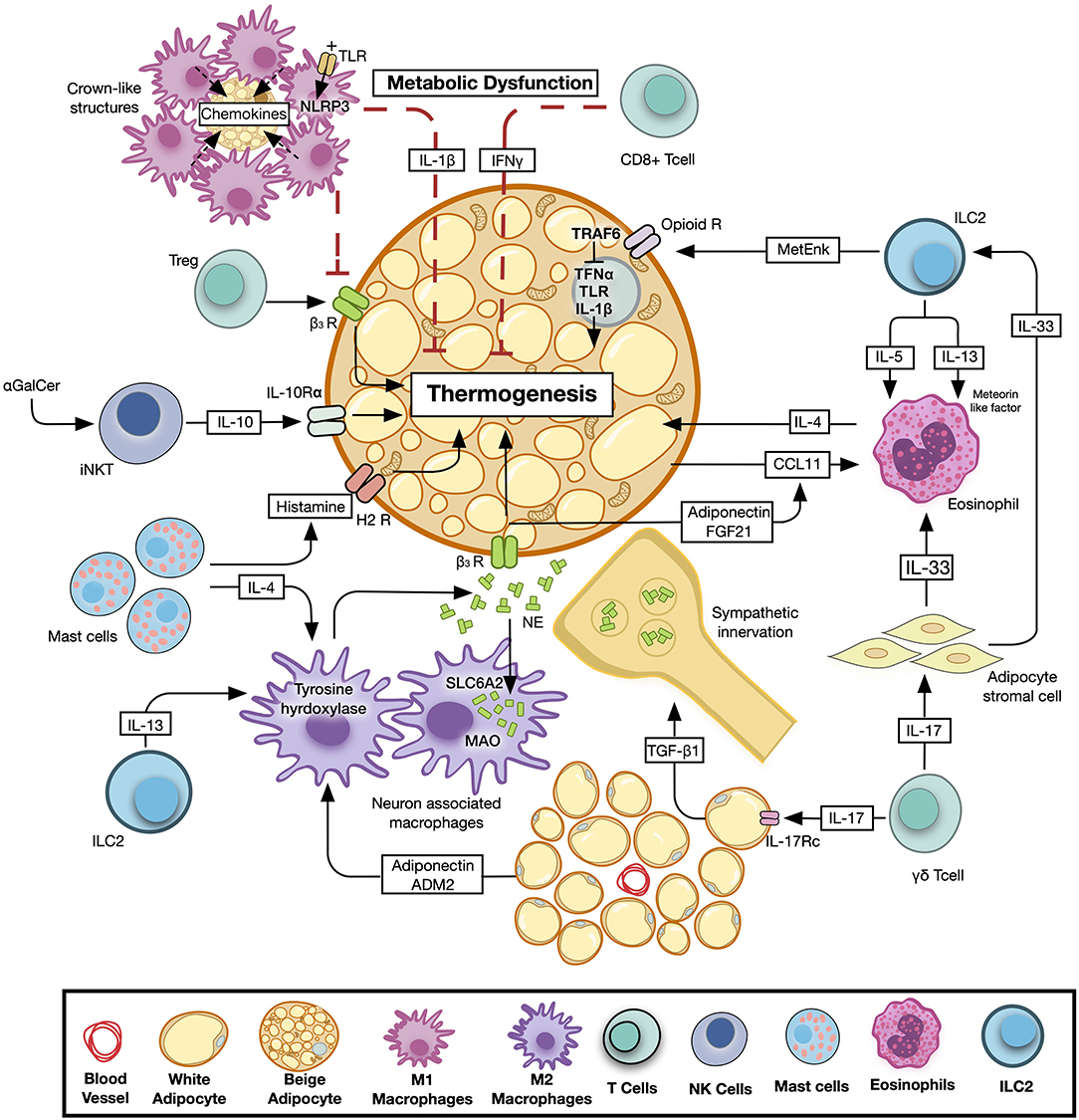
Figure 3. Immune cells-mediated regulation of adaptive thermogenesis. Different types of immune cells exert various modes of control on thermogenesis by either directly modulating the adipocyte function or affecting sympathetic nerve activity and norepinephrine turn-over. Pathways promoting thermogenesis are depicted in black, while inhibitory pathways are shown in red. ADM2, Adrenomedullin-1; β3-AR, Beta 3-adrenergic Receptor; CCL11, C-C motif chemokine 11; FGF21, Fibroblast Growth Factor 21; H2R, Histamine 2 Receptor; γGalCer, Alpha-galactosylceramide; IL, Interleukin; ILC, Innate Lymphoid Cell; MAO, Monoamine Oxidase; NE, Norepinephrine; Opioid R, Opioid Receptor; SCL6A2, Solute Carrier Family 6 Member 2; TGF-β, Transforming Growth Factor Beta; Treg, Regulatory T Lymphocyte.
Macrophages
M1 macrophages suppress the induction of thermogenic adipocytes in obese AT of mice (487). Conversely, adiponectin-induced M2 macrophages drive scWAT thermogenesis in cold-exposed mice and the depletion of either the macrophages or adiponectin reduces scWAT browning (488). The browning effect of adrenomedullin 2 (ADM2), a white adipocyte-produced factor that increases UCP1 expression, is also mediated by M2 macrophages (489).
The activation of pattern recognition receptors in AT-infiltrating macrophages was shown to suppress thermogenesis. LPS-activated TLR4 receptors of macrophages repressed β3-AR-induced adipocyte browning, caused mitochondrial dysfunction, and increased ROS production (490). Moreover, the activation of NLRP3 inflammasome in macrophages attenuated UCP1 induction in cultured adipocytes in an IL-1β-dependent manner (490). Furthermore, adipocyte-specific deletion of transforming growth factor-activated kinase 1 (TAK1) but not TNF receptor associated factor 6 (TRAF6), increased the expression of beige markers in WAT. TAK1 deletion in WAT increases AMPK phosphorylation, PGC-1α abundance, non-canonical NF-κB signaling, and markers of M2 macrophages while inhibiting canonical NF-κB signaling (491). Conversely, the deletion of TRAF1, an inhibitory adapter of TNFα, IL-1β, and TLRs enhanced leukocyte accumulation and potentiated the proinflammatory signaling of macrophages in HFD-fed mice (492). Nevertheless, TRAF1-deficient mice were protected from metabolic derangements and exhibited an improved IR partially by β3-AR-mediated induction of UCP1-dependent thermogenesis (492).
ILC2s
Activation of murine ILC2s with IL-33 induced the proliferation of beige adipocyte progenitors and increased WAT browning through an IL-4/IL-13-dependent pathway involving eosinophils (480). The recruitment of IL-4+ eosinophils was driven by ILC2-secreted IL-5 and IL-13. ILC2-produced BMP7 was also demonstrated to induced the differentiation of adipocyte progenitors into brown adipocytes (493). ILC2s also induce thermogenesis through the production of the opioid-like peptide methionine enkephalin (MetEnk) (316, 494). The stimulation of ILC2s with IL-33 induced the production of MetEnk that signaled through opioid receptors in scWAT and BAT to promote thermogenesis (316). Mice either treated with MetEnk or adoptive transfer of IL-33-activated ILC2s increased the expression of UCP1 in scWAT even in mice deficient in eosinophils or IL-4Rα demonstrating a direct activity of ILC2s on opioid receptors to induce thermogenesis (316).
γδT Cells
AT-resident γδT cells were recently shown to regulate body temperature through the production of IL-17A upon cold exposure, which regulated IL-33 production by adipose stromal cells (268). Mice deficient in γδT cells or IL-17A exhibited decreases in both ST2+ Tregs and IL-33 abundance in VAT and dysregulated core body temperature at thermoneutrality and upon cold exposure (268). Given the critical role of IL-33 in regulating insulin homeostasis and thermogenesis (495), IL-17-deficient mice were cold-intolerant (268). γδT cell-deficient mice also exhibited a reduced UCP1 expression and energy expenditure upon cold exposure (268).
iNKT Cells
The selective loss of IL-10Rα in adipocytes as well as the global depletion of IL-10 enhanced thermogenesis (496). Early reports demonstrated that the activation of adipose iNKT cells with αGalCer induced potent weight loss in obese mice (282, 284, 286, 497, 498). It was recently shown that iNKT cell-induced weight loss occur through the induction of FGF21-dependent adaptive thermogenesis. The intraperitoneal administration of αGalCer into obese mice induced a significant reduction of AT mass under thermoneutral conditions, which was accompanied by an increased WAT browning and energy expenditure (499). FGF21-deficient mice exhibited a blunted, but not fully ablated response toward αGalCer suggesting that iNKT cells drive thermogenesis through an FGF21-independent mechanism (499).
Mast Cells
BAT MC-released histamine is thought to play a β3-AR-independent role in thermogenesis through its interaction with H2 receptors (500). Upon cold exposure, MCs were recruited to WAT and exhibited an enhanced histamine degranulation in both lean and obese subjects, which was positively correlated with UCP1 expression and thermogenesis (501). Indeed, in response to cold, MCs also release IL-4 along with other factors driving UCP1 expression and WAT browning (502). Nevertheless, it was also proposed that MC deficiency in mice increases WAT browning by promoting adipocyte differentiation as MC-derived serotonin inhibited WAT browning (503). Nevertheless, these findings were based on a murine model in which c-kit tyrosine kinase is mutated and thus, a careful interpretation of the results is required. Furthermore, several other genetic models of MC depletion showed no association between MC function and obesity (228, 229).
T and B Lymphocytes
Several studies revealed a potential function of Tregs of scWAT and BAT in regulating thermogenic homeostasis. Systemic deletion of Tregs impaired oxygen consumption upon cold exposure (504). Additionally, the T cell-specific STAT6/PTEN axis is thought to mediate the link between β3-AR stimulation and Treg cell induction in both BAT and scWAT (505, 506). Indeed, UCP1-deficient mice exhibited reduced Tregs in BAT and scWAT (506). B and T lymphocytes were also shown to play a role in thermoregulation. Rag1-deficient HFD-fed mice, lacking both T and B lymphocytes, exhibited decreased UCP1 expression (507). Conversely, deleting Rag1 in lean mice housed at room temperature resulted in an increased UCP1 expression and energy expenditure (508). Moreover, a decreased CD8+ but not CD4+ T cells is believed to contribute to adipocyte browning mainly due to a decreased IFN-γ secretion (508).
Eosinophils
Eosinophil-derived IL-4 drives M2 macrophage polarization, promoting the secretion of catecholamines that drive WAT browning (215). As mentioned before, the role of M2 macrophages in local catecholamine production has been questioned. Nevertheless, this does not preclude the implication of eosinophil-derived catecholamines in WAT browning. Indeed, PVAT eosinophils were shown to promote PVAT browning by locally producing catecholamines (509). Moreover, mice lacking eosinophils exhibited an impaired thermogenic capacity of scWAT following cold exposure (215). Meteorin-like is another factor linking eosinophils to WAT browning, where it was shown to stimulate IL-4 secretion from eosinophils and macrophage M2 polarization in AT following cold exposure (510). In addition to ILC2-derived IL-33-dependent eosinophils recruitment to AT, IL-33 was also shown to recruit eosinophils in the absence of ILC2s (178). It was recently demonstrated that the transcriptional repressor krüppel-like factor 3 (KLF3)-deficient mice exhibited profound WAT beiging, which was accompanied by an accumulation of AT eosinophils (511).
Immunomodulating Adipose Tissue Inflammation in Metabolic Disorders and Cardiovascular Diseases
Strategies to modulate AT inflammation are multi-faceted, they include physical exercise, lifestyle modifications, in addition to several pharmacological and non-pharmacological interventions. Likewise, treatment of CVDs focuses on similar strategies that impact AT. In this section, we will tackle the contribution of different modalities on AT inflammation in CVDs.
Exercise and Lifestyle Modifications
One of the first strategies to decrease the severity and complications of CVDs is to limit food intake and increase energy expenditure, this is mainly due to the fact that most patients with CVDs are overweight or obese. Exercise and lifestyle modifications lower the mortality risk, improve quality of life and have been extensively studied. Physical activity improves insulin sensitivity and alters AT adipokine expression, which affect whole-body metabolic health in human subjects (512, 513). Recent studies highlighted the mechanistic pathways, linking those two interventions with decreasing AT inflammation. The protective effects of regular physical activity is accompanied with reduction of visceral fat mass along with an anti-inflammatory pathway (514). Physical exercise exerts a direct anti-inflammatory effect by inducing an acute elevation in IL-6 and IL-10 and an inhibition of TNF-α (515). The anti-inflammatory effect of exercise also comprises the inhibition of macrophage infiltration and the induction of ATMs phenotypic switch toward the M2 phenotype in obese mice (515, 516). Zielger et al. demonstrated that exercise enhances the anti-inflammatory phenotype in VATs of old mice (517). Endurance training regardless of weight loss induced an increase in M2 macrophages in scWAT (513, 518). The role of physical activity in thermogenesis and WAT browning is debated as some reported scWAT browning with bicycle training programs while another study failed to find a correlation between aerobic exercises and recruitment of beige adipocytes (519, 520). Nevertheless, more studies should target the exact role of AT remodeling following physical activity. Indeed, long-term anti-inflammatory effects of chronic physical activity could be required for a pronounced AT remodeling required to decrease CVD risks.
Diet Modification and Weight Loss
Fasting
Several fasting regimens were introduced as an alternative or a complementary intervention to restricted caloric diets in improving cardiometabolic endpoints in related diseases (521, 522). Indeed, several studies on experimental animals and recent human investigations highlighted the importance of fasting in metabolic activity regulation, blood pressure, and atherosclerosis reduction, as well as health optimization (522–524). The most common types of fasting regimens include intermittent fasting (IF), periodic fasting (PF), short term fasting, and religious fasting. IF has a crucial role in adaptive cellular responses being able to reduce inflammation, oxidative stress, optimize energy metabolism, and cellular bioenergetics (524). Fasting is an effective strategy for improving cardiometabolic profile in cases of IR, stroke, prediabetes, and diabetes (525, 526). Moreover, IF modulates the susceptibility of inflammatory diseases by decreasing peripheral monocyte pools and modifying their metabolic activity through AMPK and PPARα pathways (527). On the AT level, fasting enhances mitochondrial biogenesis in visceral adipocytes. Short term fasting suppressed thermogenesis in inguinal WAT and iBAT in a mouse model (528). Another fasting regimen, every other day fasting, was shown to induce beiging of WAT thus reversing HFD-induced obesity and associated metabolic disorders in mice (529). The metabolic effects of IF are largely mediated by adipose thermogenesis; fasting-induced adipose VEGF, which is thought to act on eosinophils, Th2, and ILC2 to promote M2 polarization was linked to WAT browning (530). Moreover, fasting induces a reduction of IL-1 and IL-6 in VAT and scWAT and IL-6 in intraperitoneal WAT (531). Moreover, biomarkers of inflammation in EpiWAT and BAT were reduced in mice following IF. The latter study presented IF as a preventive and therapeutic intervention to protect mice against MetS and obesity (532). On the other hand, fasting reduces leptin levels triggering a profound metabolic state as well as regulating T lymphocytes and cytokine production in obese animal models and human trials (533–535). In addition to that, a new randomized control trial has also linked fasting to reduction of leptin levels (536).
Dietary Modifications
Dietary modification is the cornerstone in preventing cardiometabolic diseases. Weight loss approaches as well as initiating certain diet regimens lower CVD events significantly and reduce mortality (537). Reports correlating dietary manipulation, such as in high protein diets, phosphate diet, and ketogenic diet, to AT inflammation suggest that certain diet regimens can play a critical role in modulating cardiometabolic diseases.
Long term intake of high protein diet in obesity-prone rats reduced food intake and WAT mass while improving basal blood sugar, insulin levels, leptin, and triglyceride levels in addition to glucose tolerance (538). On the other hand, a clinical study suggested that phosphorus supplementation is involved in modulating glucose and insulin serum levels (539). Another study reported that high dietary intake of phosphate in rats can influence lipid and glucose metabolism by upregulating lipolytic gene expression and reducing WAT accumulation (540).
Ketogenic diet (KD), which consists mainly of ingesting healthy fats, improved long-term blood glucose control and subsequently decreased the use of anti-diabetic agents in human studies (541, 542). KD also improved the CVD biomarkers in T2DM patients (543). Moreover, short term feeding of KD was shown to modulate AT immune cells, where it reduced macrophage infiltration and the expansion of γδ T cells in VAT (279).
Mediterranean Diet (MD) is composed of a balanced combination of fruits, vegetables, fibers, fish, poly saturated fats as well as low intake of meat and dairy products in addition to moderate intake of red wine (544). The adherence to MD is known to protect humans against CVDs, MetS, onset of various types of cancer, and aging (545, 546). Several studies documented that certain typical food of the MD including olive oil, tomato, and red wine induce anti-inflammatory properties and could be even insulin-sensitizing (547, 548). For example, tomato juice mitigates AT inflammation; a 20-days duration of consumption could decrease TNF-α, IL-6, and IL-8 (549, 550). Moreover, tomato juice supplementation could reduce body weight, blood cholesterol levels as well MCP-1 (551).
Anti-diabetic Drugs
Metformin
Besides being widely used for DM2 treatment, Metformin reduces CVD risk, induces weight loss, and improves insulin sensitivity (552). Metformin has been proposed to reduce adipocyte stores and initiate a metabolically healthy adipocytes distribution (553). The beneficial effects of metformin also include reducing visceral AT, a mechanism that is thought to be related to FA oxidation and an upregulation of adaptive thermogenesis (554). Emerging body of evidence, including work done by our team, documented that metformin can exhibit immunomodulatory features, an anti-inflammatory effect that is shown to be independent on glycemic control (32, 555, 556). Metformin activates the anti-inflammatory macrophage polarization; it lowers the pro-inflammatory cytokine production through elevating M2 macrophage and lowering M1 macrophages (557).
Metformin also decreases MCP-1 in isolated human AT cultures, suggesting an improved low-grade inflammation (558). Moreover, metformin has been shown to alter CRP, NF-κB expression in addition to reducing advanced glycation end products (559–561). All in all, the anti-inflammatory effects of metformin and its ability to reduce several inflammatory related illnesses is becoming more apparent (552).
Thiazolidinediones (TZDs)
Besides the use of TZDs in T2DM, a beneficial role of Pioglitazone lies in reducing cardiovascular events (562). In fact, TZDs activation of PPARγ not only enhances adipogenesis but also reduces fat deposition in tissues, and attenuates the inflammatory cytokines release in obesity (563, 564). Moreover, TZDs repress NF-kB thus restore M2 macrophage phenotype, and prompt the recruitment of regulatory T cells in AT (263, 565, 566). Therefore, this reveals a possible involvement of TZDs in an immunomodulatory mechanism in AT that may benefit patients with CVDs, yet the exact definitive pathway has not been established. Expanding on the beneficial effects of PPARγ agonism in limiting AT chronic low-grade inflammation in metabolic disorders, glitazones-like, multi-targeting drug ligands (MTDLs) were rationally designed (567). Importantly, these drugs were partial PPARγ agonists, potent COX-2 antagonists and moderate 15-LOX inhibitors. This balanced modulation of the three inflammatory targets allows for a more effective targeting of AT inflammation and possibly limit its cardiovascular complications (568).
Glucagon-Like Peptide-1 (GLP-1) Receptor Agonists
Several GLP-1 receptor agonists, as Liraglutide, have been developed to mimic the glucose-lowering and anorexic effects of Glucagon-like peptide-1 (GLP-1) to treat obesity and T2DM. As AT express GLP-1, Liraglutide has been effective in controlling glucose levels, promoting weight loss, and reducing total adiposity (569–571). In addition, in a clinical trial, Liraglutide has been shown to decrease the risk of myocardial infarction in patients with T2DM and high CVD risk (572). More studies on the effect of GLP-1 agonists documented their protective roles against endothelial cell dysfunction, and therefore atherosclerosis by reducing CRP and plasma lipids (573, 574). As such, GLP-1 agonists could provide protection against CVDs through AT mass reduction and inflammation.
Sodium-Glucose Cotransporter (SGLT2) Inhibitors
Similar to GLP-1 receptor agonists, SGLT-2 inhibitors are shown to reduce blood glucose levels and CVDs risk and mortality (575, 576). Treatment with Empagliflozin, an SGLT-2 inhibitor, has been shown to induce weight loss when given in combination with other anti-diabetic medication (577, 578). In obese mice, Empagliflozin was shown to promote utilization and browning of AT as well as reduction of IR and inflammation, a pathway linked to M2 macrophage polarization (579). As such, beside the anti-diabetic effects of SGLT-2 inhibitors, strong evidence appears in their effect on AT remodeling and anti-inflammatory pathway; yet the exact mechanism is to be elucidated.
Surgical Interventions
Bariatric surgery is the most effective treatment option in obese patients for weight loss whether due to food restriction, malabsorption, or both (580). Following the surgery and independent on weight loss; IR, CVDs, and mortality rates are all reduced (537, 581, 582). Importantly, it is expected that a late phase reduction of AT inflammation could be in favor of all the metabolic consequences of the surgery (583). However, when looking back to literature, contradictory results are revealed. Some have documented a decrease in inflammatory mediators of AT after the surgery-induced weight loss, and others have reported no further change (584–587). Reports generally assess subcutaneous AT depot, as it is easier in sampling. However, more studies should be warned to confirm or elucidate the effects of bariatric surgery on AT inflammation. Sampling from visceral AT and other sites should be done as it is more prone to inflammatory changes.
New Avenues for Adipose Tissue Immunomodulation in Metabolic Disorders and Cardiovascular Diseases
AT inflammation is associated with an increased production of pro-inflammatory cytokines including IL-1β, TNF-α, IL-6, and IFN-γ. Anti-inflammatory treatments were proposed to contribute to the treatment of diabetes and its vascular complications (588). The antagonism of IL-1R improved IR in T1D patients and DIO mice (589, 590), and in patients with impaired glucose tolerance (591). One study however highlighted the importance of combining IL-1R antagonism treatment with proper dieting for the treatment of obesity (592). Moreover, inhibiting TNF-α in psoriasis patients with MetS decreased macrophage infiltration and pro-inflammatory cytokines levels in umbilical fat (593). Interestingly, a combined inhibition of IL-1β and TNF-α was more effective in improving IR in T2D rats (594). Similarly targeting IL-6 improved IR and normalized adipokine levels in MetS and fructose-fed rats (595, 596). Nevertheless, this mechanistic link was not evident in clinical trials (597). Indeed, IL-6 was shown to drive exercise-induced weight loss in subjects with visceral obesity (598). The complexity of the AT immune landscape driving AT inflammation in the MetS and the response of these cells to the various pro-inflammatory cytokines dictate the efficacy of these approaches. However, simply targeting pro-inflammatory cytokines with either receptor inhibitors or monoclonal antibodies for the treatment of metabolic and cardiovascular diseases is not yet a valid therapeutic strategy and requires further investigation.
The metabolic reprogramming in response to nutritional excess and scarcity of the various immune cells is not universal. Indeed, metabolic modulation emerged as a novel concept in cancer immunotherapy (599). Th2 immunity in AT supports metabolic health and thus, targeting Th2 immunometabolism represents a valuable therapeutic strategy in metabolic disorders. Several reports on immunometabolism-targeted treatments in cancer and autoimmunity can be repurposed for metabolic diseases. For example, in a model of allograft rejection, blocking glycolysis and glutamine metabolism inhibited CD4+ and CD8+ T cell induction and promoted the generation of allospecific Treg cells (600). Indeed, expanding AT Treg cells hold much promise for the treatment of metabolic disorders and their cardiovascular complications. Additionally, nanoparticles, liposomes, and glucan-shells carrying siRNA or specific drugs can be engineered to tissue-specifically target specific immune cells as exemplified by ATMs (601). Nevertheless, a profound knowledge of metabolic profile shifts of immune cells within the AT is still lacking and thus, direct interpretations of such shifts in other tissues cannot be extrapolated, particularly in the complex, dynamically-evolving AT. Emerging technologies such as RNA sequencing, metabolomics, proteomics and phage display will surely allow for the identification of novel peptide targets (602). Moreover, adaptive immunity and the acquisition of memory T cells in HFD-fed mice suggests an effect on subsequent episodes of weight gain following weight loss (603, 604). Modulating T cell memory has been achieved by targeting antigen presentation in conventional and non-conventional APCs (271) and checkpoint co-inhibitory interactions (605). Finally, emerging evidence indicates that immunometabolism is controlled epigenetically and through miRNAs, which affects cellular differentiation and polarization (606, 607). Nevertheless, further research is required to determine how metabolic dysfunction drives alterations in epigenetic histone modifications and how miRNAs affects the AT immune profile.
Accumulating evidence suggests a role for the gut microbiota in modulating metabolic homeostasis. Indeed, it was shown that the adoptive transfer of Th 17 cells contributed to metabolic homeostasis through an IL-17-dependent microbiota development (608). Moreover, it was demonstrated that the M2 macrophage-mediated helminth-associated Th2/Treg responses induce alterations in microbiota composition which was accompanied by protection against obesity (609, 610).
We have highlighted a key role for physical activity, different fasting regimens, and dietary modifications in limiting AT inflammation and IR. Nevertheless, their implications on different adipose depots, especially those of cardiovascular interest are not well-characterized. In fact, a depot-specific metabolic profiling is pivotal to delineate their differential effects. Moreover, anti-diabetic drugs and surgical procedures showed a favorable outcome on metabolic parameters and CVDs. In fact, these approaches reduced AT inflammation through mechanisms being revealed only recently. Therefore, it is pivotal that AT immune profiles in different depots be characterized following the administration of anti-diabetic drugs.
Finally, the induction of BAT activity and WAT browning has been proposed as a mean to curb obesity and combat CVDs. Indeed, the induction of different AT depots browning resulted in either favorable or detrimental outcomes. Targeting UCP1 was even proposed as the induction of browning in PVAT and EpiCAT was supposed to deteriorate vascular and cardiac functionality (33). Indeed, this strategy is still debated as clinical trials have not shown a significant improvement of metabolic parameters following the induction of thermogenesis (33). In addition to the non-selective impact on all adipose depots, the available UCP1 inhibitors possess a fairly high IC50 value (~20 μM) (611) precluding systemic administration without significant off-target and adverse effects. As such, immune modulation of thermogenesis might constitute a lucrative target for depot-specific intervention. As different depots possess variable intrinsic brown-like character, it is pivotal to further characterize this phenotype, the manner by which it is affected by the immune system in states of health and disease, and how increased energy expenditure leads to clinical significance. Significantly, the relative impact of the activation of different thermogenic pathways on AT inflammation in various adipose depots requires systematic examination. Whether the selection of one pathway over the other modulates activity and/or recruitment of disparate immune cells remains unknown. As well, the ability of a specific immune cell product/function to favor one pathway over the other has not been investigated.
Conclusion
Metabolic and Cardiovascular diseases are multifactorial disorders to which contributes the inflammation of the AT. Several pharmacological and non-pharmacological interventions have been shown to exert their positive effects in these diseases, at least in part by modulating AT inflammation. Accumulating evidence implicates different immune cells in the regulation of AT inflammation and its consequences including IR. The metabolic reprogramming of AT immune cells and the alteration of the AT immune landscape are believed to drive AT inflammation, BAT thermogenesis and WAT browning. Further investigation is required to delineate the exact role of different immune cells and the consequences of their metabolic profile alteration in different adipose depots inflammation. A better comprehension of the mechanisms driving AT inflammation allows for the emergence of novel therapeutic strategies aimed at immunomodulating the AT.
Author Contributions
IA, SH, HA-K, and AG participated in literature review and screening and contributed to manuscript writing. IA wrote the first draft of the manuscript. AE helped in overseeing and coordinating the work and participated in manuscript draft review. AE-Y developed the idea, supervised the work, reviewed and modified manuscript draft, and provided research funding support. All authors contributed to the article and approved the submitted version.
Funding
This work was supported by AUB-Faculty of Medicine Medical Practice Plan Grant No. 320148 and an AUB President Collaborative Research Stimulus Grant to AE-Y.
Conflict of Interest
The authors declare that the research was conducted in the absence of any commercial or financial relationships that could be construed as a potential conflict of interest.
References
1. Guilherme A, Virbasius JV, Puri V, Czech MP. Adipocyte dysfunctions linking obesity to insulin resistance and type 2 diabetes. Nat Rev Mol Cell Biol. (2008) 9:367–77. doi: 10.1038/nrm2391
2. Chouchani ET, Kazak L, Spiegelman BM. New advances in adaptive thermogenesis: UCP1 and beyond. Cell Metab. (2019) 29:27–37. doi: 10.1016/j.cmet.2018.11.002
3. Zatterale F, Longo M, Naderi J, Raciti GA, Desiderio A, Miele C, et al. Chronic adipose tissue inflammation linking obesity to insulin resistance and type 2 diabetes. Front Physiol. (2020) 10:1607. doi: 10.3389/fphys.2019.01607
4. Lumeng CN, Saltiel AR. Inflammatory links between obesity and metabolic disease. J Clin Invest. (2011) 121:2111–7. doi: 10.1172/JCI57132
5. Kunath A, Klöting N. Adipocyte biology and obesity-mediated adipose tissue remodeling. Obes Med. (2016) 4:15–20. doi: 10.1016/j.obmed.2016.10.001
6. Tune JD, Goodwill AG, Sassoon DJ, Mather KJ. Cardiovascular consequences of metabolic syndrome. Transl Res. (2017) 183:57–70. doi: 10.1016/j.trsl.2017.01.001
7. Stolarczyk E. Adipose tissue inflammation in obesity: a metabolic or immune response? Curr Opin Pharmacol. (2017) 37:35–40. doi: 10.1016/j.coph.2017.08.006
8. Choe SS, Huh JY, Hwang IJ, Kim JI, Kim JB. Adipose tissue remodeling: its role in energy metabolism and metabolic disorders. Front Endocrinol. (2016) 7:30. doi: 10.3389/fendo.2016.00030
9. Trayhurn P, Wood IS. Signalling role of adipose tissue: adipokines and inflammation in obesity. Biochem Soc Trans. (2005) 33:1078–81. doi: 10.1042/BST0331078
10. Lee YS, Kim JW, Osborne O, Sasik R, Schenk S, Chen A, et al. Increased adipocyte O2 consumption triggers HIF-1α, causing inflammation and insulin resistance in obesity. Cell. (2014) 157:1339–52. doi: 10.1016/j.cell.2014.05.012
11. Engin A. The pathogenesis of obesity-associated adipose tissue inflammation. Adv Exp Med Biol. (2017) 960:221–45. doi: 10.1007/978-3-319-48382-5_9
12. Halberg N, Khan T, Trujillo ME, Wernstedt-Asterholm I, Attie AD, Sherwani S, et al. Hypoxia-inducible factor 1α induces fibrosis and insulin resistance in white adipose tissue. Mol Cell Biol. (2009) 29:4467–83. doi: 10.1128/MCB.00192-09
13. Legrand-Poels S, Esser N, L'homme L, Scheen A, Paquot N, Piette J. Free fatty acids as modulators of the NLRP3 inflammasome in obesity/type 2 diabetes. Biochem Pharmacol. (2014) 92:131–41. doi: 10.1016/j.bcp.2014.08.013
14. Morigny P, Houssier M, Mouisel E, Langin D. Adipocyte lipolysis and insulin resistance. Biochimie. (2016) 125:259–66. doi: 10.1016/j.biochi.2015.10.024
15. Kuroda M, Sakaue H. Adipocyte death and chronic inflammation in obesity. J Med Invest. (2017) 64:193–6. doi: 10.2152/jmi.64.193
16. Kruglikov IL, Scherer PE. Dermal adipocytes: from irrelevance to metabolic targets? Trends Endocrinol Metab. (2016) 27:1–10. doi: 10.1016/j.tem.2015.11.002
17. Lin D, Chun TH, Kang L. Adipose extracellular matrix remodelling in obesity and insulin resistance. Biochem Pharmacol. (2016) 119:8–16. doi: 10.1016/j.bcp.2016.05.005
18. Crewe C, An YA, Scherer PE. The ominous triad of adipose tissue dysfunction: inflammation, fibrosis, and impaired angiogenesis. J Clin Invest. (2017) 127:74–82. doi: 10.1172/JCI88883
19. Kane H, Lynch L. Innate immune control of adipose tissue homeostasis. Trends Immunol. (2019) 40:857–72. doi: 10.1016/j.it.2019.07.006
20. Winer DA, Winer S, Chng MH, Shen L, Engleman EG. B lymphocytes in obesity-related adipose tissue inflammation and insulin resistance. Cell Mol Life Sci. (2014) 71:1033–43. doi: 10.1007/s00018-013-1486-y
21. Zelechowska P, Agier J, Kozłowska E, Brzezińska-Błaszczyk E. Mast cells participate in chronic low-grade inflammation within adipose tissue. Obes Rev. (2018) 19:686–97. doi: 10.1111/obr.12670
22. Lu J, Zhao J, Meng H, Zhang X. Adipose tissue-resident immune cells in obesity and type 2 diabetes. Front Immunol. (2019) 10:1173. doi: 10.3389/fimmu.2019.01173
23. Weisberg SP, Mccann D, Desai M, Rosenbaum M, Leibel RL, Ferrante AW. Obesity is associated with macrophage accumulation in adipose tissue. J Clin Invest. (2003) 112:1796–808. doi: 10.1172/JCI200319246
24. Lumeng CN, Deyoung SM, Bodzin JL, Saltiel AR. Increased inflammatory properties of adipose tissue macrophages recruited during diet-induced obesity. Diabetes. (2007) 56:16–23. doi: 10.2337/db06-1076
25. Olefsky JM, Glass CK. Macrophages, inflammation, and insulin resistance. Annu Rev Physiol. (2010) 72:219–46. doi: 10.1146/annurev-physiol-021909-135846
26. Kitade H, Sawamoto K, Nagashimada M, Inoue H, Yamamoto Y, Sai Y, et al. CCR5 plays a critical role in obesity-induced adipose tissue inflammation and insulin resistance by regulating both macrophage recruitment and M1/M2 status. Diabetes. (2012) 61:1680–90. doi: 10.2337/db11-1506
27. Amano SU, Cohen JL, Vangala P, Tencerova M, Nicoloro SM, Yawe JC, et al. Local proliferation of macrophages contributes to obesity-associated adipose tissue inflammation. Cell Metab. (2014) 19:162–71. doi: 10.1016/j.cmet.2013.11.017
28. Ibrahim MM. Subcutaneous and visceral adipose tissue: structural and functional differences. Obes Rev. (2010) 11:11–8. doi: 10.1111/j.1467-789X.2009.00623.x
29. Fitzgibbons TP, Kogan S, Aouadi M, Hendricks GM, Straubhaar J, Czech MP. Similarity of mouse perivascular and brown adipose tissues and their resistance to diet-induced inflammation. Am J Physiol Heart Circ Physiol. (2011) 301:H1425–37. doi: 10.1152/ajpheart.00376.2011
30. Roberts-Toler C, O'neill BT, Cypess AM. Diet-induced obesity causes insulin resistance in mouse brown adipose tissue. Obesity. (2015) 23:1765–70. doi: 10.1002/oby.21134
31. Dowal L, Parameswaran P, Phat S, Akella S, Majumdar ID, Ranjan J, et al. Intrinsic properties of brown and white adipocytes have differential effects on macrophage inflammatory responses. Mediators Inflamm. (2017) 2017:9067049. doi: 10.1155/2017/9067049
32. Elkhatib MW, Mroueh A, Rafeh RW, Sleiman F, Fouad H, Saad EI, et al. Amelioration of perivascular adipose inflammation reverses vascular dysfunction in a model of nonobese prediabetic metabolic challenge: potential role of antidiabetic drugs. Transl Res. (2019) 214:121–43. doi: 10.1016/j.trsl.2019.07.009
33. Rafeh R, Viveiros A, Oudit GY, El-Yazbi AF. Targeting perivascular and epicardial adipose tissue inflammation: therapeutic opportunities for cardiovascular disease. Clin Sci. (2020) 134:827–51. doi: 10.1042/CS20190227
34. Guzik TJ, Skiba DS, Touyz RM, Harrison DG. The role of infiltrating immune cells in dysfunctional adipose tissue. Cardiovasc Res. (2017) 113:1009–23. doi: 10.1093/cvr/cvx108
35. Mancuso P. The role of adipokines in chronic inflammation. Immun Ther. (2016) 5:47. doi: 10.2147/ITT.S73223
36. Auguet T, Quintero Y, Riesco D, Morancho B, Terra X, Crescenti A, et al. New adipokines vaspin and omentin. Circulating levels and gene expression in adipose tissue from morbidly obese women. BMC Med Genet. (2011) 12:60. doi: 10.1186/1471-2350-12-60
37. Ohashi K, Shibata R, Murohara T, Ouchi N. Role of anti-inflammatory adipokines in obesity-related diseases. Trends Endocrinol Metab. (2014) 25:348–55. doi: 10.1016/j.tem.2014.03.009
38. Tan YL, Zheng XL, Tang CK. The protective functions of omentin in cardiovascular diseases. Clin Chim Acta. (2015) 448:98–106. doi: 10.1016/j.cca.2015.05.019
39. Scherer PE, Williams S, Fogliano M, Baldini G, Lodish HF. A novel serum protein similar to C1q, produced exclusively in adipocytes. J Biol Chem. (1995) 270:26746–9. doi: 10.1074/jbc.270.45.26746
40. Lau WB, Ohashi K, Wang Y, Ogawa H, Murohara T, Ma XL, et al. Role of adipokines in cardiovascular disease. Circ J. (2017) 81:920–8. doi: 10.1253/circj.CJ-17-0458
41. Yu JG, Javorschi S, Hevener AL, Kruszynska YT, Norman RA, Sinha M, et al. The effect of thiazolidinediones on plasma adiponectin levels in normal, obese, and type 2 diabetic subjects. Diabetes. (2002) 51:2968–74. doi: 10.2337/diabetes.51.10.2968
42. Combs TP, Berg AH, Rajala MW, Klebanov S, Iyengar P, Jimenez-Chillaron JC, et al. Sexual differentiation, pregnancy, calorie restriction, and aging affect the adipocyte-specific secretory protein adiponectin. Diabetes. (2003) 52:268–76. doi: 10.2337/diabetes.52.2.268
43. Kumada M, Kihara S, Sumitsuji S, Kawamoto T, Matsumoto S, Ouchi N, et al. Association of hypoadiponectinemia with coronary artery disease in men. Arterioscler Thromb Vasc Biol. (2003) 23:85–9. doi: 10.1161/01.ATV.0000048856.22331.50
44. Pischon T, Girman CJ, Hotamisligil GS, Rifai N, Hu FB, Rimm EB. Plasma adiponectin levels and risk of myocardial infarction in men. JAMA. (2004) 291:1730–7. doi: 10.1001/jama.291.14.1730
45. L'abbate A, Neglia D, Vecoli C, Novelli M, Ottaviano V, Baldi S, et al. Beneficial effect of heme oxygenase-1 expression on myocardial ischemia-reperfusion involves an increase in adiponectin in mildly diabetic rats. Am J Physiol Heart Circ Physiol. (2007) 293:H3532–41. doi: 10.1152/ajpheart.00826.2007
46. Goldstein BJ, Scalia RG, Ma XL. Protective vascular and myocardial effects of adiponectin. Nat Clin Pract Cardiovasc Med. (2009) 6:27–35. doi: 10.1038/ncpcardio1398
47. Yamaguchi N, Argueta JG, Masuhiro Y, Kagishita M, Nonaka K, Saito T, et al. Adiponectin inhibits Toll-like receptor family-induced signaling. FEBS Lett. (2005) 579:6821–6. doi: 10.1016/j.febslet.2005.11.019
48. Chandrasekar B, Boylston WH, Venkatachalam K, Webster NJ, Prabhu SD, Valente AJ. Adiponectin blocks interleukin-18-mediated endothelial cell death via APPL1-dependent AMP-activated protein kinase (AMPK) activation and IKK/NF-kappaB/PTEN suppression. J Biol Chem. (2008) 283:24889–98. doi: 10.1074/jbc.M804236200
49. Chen H, Montagnani M, Funahashi T, Shimomura I, Quon MJ. Adiponectin stimulates production of nitric oxide in vascular endothelial cells. J Biol Chem. (2003) 278:45021–6. doi: 10.1074/jbc.M307878200
50. Yamauchi T, Kamon J, Ito Y, Tsuchida A, Yokomizo T, Kita S, et al. Cloning of adiponectin receptors that mediate antidiabetic metabolic effects. Nature. (2003) 423:762–9. doi: 10.1038/nature01705
51. Ouchi N, Kobayashi H, Kihara S, Kumada M, Sato K, Inoue T, et al. Adiponectin stimulates angiogenesis by promoting cross-talk between AMP-activated protein kinase and Akt signaling in endothelial cells. J Biol Chem. (2004) 279:1304–9. doi: 10.1074/jbc.M310389200
52. Alvarez P, Tapia L, Mardones L, Pedemonte J, Farías J, Castillo R. Cellular mechanisms against ischemia reperfusion injury induced by the use of anesthetic pharmacological agents. Chem Biol Interact. (2014) 218:89–98. doi: 10.1016/j.cbi.2014.04.019
53. Ajuwon KM, Spurlock ME. Adiponectin inhibits LPS-induced NF-κB activation and IL-6 production and increases PPARγ2 expression in adipocytes. Am J Physiol Regul Integr Compar Physiol. (2005) 288:R1220–5. doi: 10.1152/ajpregu.00397.2004
54. Ohashi K, Parker JL, Ouchi N, Higuchi A, Vita JA, Gokce N, et al. Adiponectin promotes macrophage polarization toward an anti-inflammatory phenotype. J Biol Chem. (2010) 285:6153–60. doi: 10.1074/jbc.M109.088708
55. Yamamoto R, Ueki S, Moritoki Y, Kobayashi Y, Oyamada H, Konno Y, et al. Adiponectin attenuates human eosinophil adhesion and chemotaxis: implications in allergic inflammation. J Asthma. (2013) 50:828–35. doi: 10.3109/02770903.2013.816725
56. Zelechowska P, Brzezińska-Błaszczyk E, Wiktorska M, Rózalska S, Wawrocki S, Kozłowska E, et al. Adipocytokines leptin and adiponectin function as mast cell activity modulators. Immunology. (2019) 158:3–18. doi: 10.1111/imm.13090
57. Wong GW, Wang J, Hug C, Tsao TS, Lodish HF. A family of Acrp30/adiponectin structural and functional paralogs. Proc Natl Acad Sci USA. (2004) 101:10302–7. doi: 10.1073/pnas.0403760101
58. Kopp A, Bala M, Buechler C, Falk W, Gross P, Neumeier M, et al. C1q/TNF-related protein-3 represents a novel and endogenous lipopolysaccharide antagonist of the adipose tissue. Endocrinology. (2010) 151:5267–78. doi: 10.1210/en.2010-0571
59. Janowska JD. C1q/TNF-related protein 1, a multifunctional adipokine: an overview of current data. Am J Med Sci. (2020) 360:222–8. doi: 10.1016/j.amjms.2020.05.036
60. Bai B, Ban B, Liu Z, Zhang MM, Tan BK, Chen J. Circulating C1q complement/TNF-related protein (CTRP) 1, CTRP9, CTRP12 and CTRP13 concentrations in type 2 diabetes mellitus: in vivo regulation by glucose. PLoS ONE. (2017) 12:e0172271. doi: 10.1371/journal.pone.0172271
61. Xin Y, Zhang D, Fu Y, Wang C, Li Q, Tian C, et al. C1qENF-related protein 1 improve insulin resistance by reducing phosphorylation of serine 1101 in insulin receptor substrate 1. Endocr J. (2017) 64:787–96. doi: 10.1507/endocrj.EJ17-0128
62. Muendlein A, Leiherer A, Saely C, Ebner J, Geiger K, Brandtner EM, et al. The novel adipokine CTRP1 is significantly associated with the incidence of major adverse cardiovascular events. Atherosclerosis. (2019) 286:1–6. doi: 10.1016/j.atherosclerosis.2019.04.222
63. Han S, Park JS, Lee S, Jeong AL, Oh KS, Ka HI, et al. CTRP1 protects against diet-induced hyperglycemia by enhancing glycolysis and fatty acid oxidation. J Nutr Biochem. (2016) 27:43–52. doi: 10.1016/j.jnutbio.2015.08.018
64. Rodriguez S, Lei X, Petersen PS, Tan SY, Little HC, Wong GW. Loss of CTRP1 disrupts glucose and lipid homeostasis. Am J Physiol Endocrinol Metab. (2016) 311:E678–97. doi: 10.1152/ajpendo.00087.2016
65. Han S, Yang Y. A novel blood pressure modulator C1q/TNF-α-related protein 1 (CTRP1). BMB Rep. (2018) 51:611. doi: 10.5483/BMBRep.2018.51.12.268
66. Shen L, Wang S, Ling Y, Liang W. Association of C1q/TNF-related protein-1 (CTRP1) serum levels with coronary artery disease. J Int Med Res. (2019) 47:2571–9. doi: 10.1177/0300060519847372
67. Zhang Y, Liu C, Liu J, Guo R, Yan Z, Liu W, et al. Implications of C1q/TNF-related protein superfamily in patients with coronary artery disease. Sci Rep. (2020) 10:1–11. doi: 10.1038/s41598-020-57877-z
68. Yang Y, Liu S, Zhang RY, Luo H, Chen L, He WF, et al. Association between C1q/TNF-related protein-1 levels in human plasma and epicardial adipose tissues and congestive heart failure. Cell Physiol Biochem. (2017) 42:2130–43. doi: 10.1159/000479915
69. Akiyama H, Furukawa S, Wakisaka S, Maeda T. CTRP3/cartducin promotes proliferation and migration of endothelial cells. Mol Cell Biochem. (2007) 304:243. doi: 10.1007/s11010-007-9506-6
70. Wölfing B, Buechler C, Weigert J, Neumeier M, Aslanidis C, Schöelmerich J, et al. Effects of the new C1q/TNF-related protein (CTRP-3)“cartonectin” on the adipocytic secretion of adipokines. Obesity. (2008) 16:1481–6. doi: 10.1038/oby.2008.206
71. Peterson JM, Wei Z, Wong GW. C1q/TNF-related protein-3 (CTRP3), a novel adipokine that regulates hepatic glucose output. J Biol Chem. (2010) 285:39691–701. doi: 10.1074/jbc.M110.180695
72. Yi W, Sun Y, Yuan Y, Lau WB, Zheng Q, Wang X, et al. C1q/tumor necrosis factor-related protein-3, a newly identified adipokine, is a novel antiapoptotic, proangiogenic, and cardioprotective molecule in the ischemic mouse heart. Circulation. (2012) 125:3159–69. doi: 10.1161/CIRCULATIONAHA.112.099937
73. Kambara T, Ohashi K, Shibata R, Ogura Y, Maruyama S, Enomoto T, et al. CTRP9 protein protects against myocardial injury following ischemia-reperfusion through AMP-activated protein kinase (AMPK)-dependent mechanism. J Biol Chem. (2012) 287:18965–73. doi: 10.1074/jbc.M112.357939
74. Su H, Yuan Y, Wang XM, Lau WB, Wang Y, Wang X, et al. Inhibition of CTRP9, a novel and cardiac-abundantly expressed cell survival molecule, by TNFα-initiated oxidative signaling contributes to exacerbated cardiac injury in diabetic mice. Basic Res Cardiol. (2013) 108:315. doi: 10.1007/s00395-012-0315-z
75. Wong GW, Krawczyk SA, Kitidis-Mitrokostas C, Ge G, Spooner E, Hug C, et al. Identification and characterization of CTRP9, a novel secreted glycoprotein, from adipose tissue that reduces serum glucose in mice and forms heterotrimers with adiponectin. FASEB J. (2009) 23:241–58. doi: 10.1096/fj.08-114991
76. Zheng Q, Yuan Y, Yi W, Lau WB, Wang Y, Wang X, et al. C1q/TNF-related proteins, a family of novel adipokines, induce vascular relaxation through the adiponectin receptor-1/AMPK/eNOS/nitric oxide signaling pathway. Arterioscler Thromb Vasc Biol. (2011) 31:2616–23. doi: 10.1161/ATVBAHA.111.231050
77. Jung CH, Lee MJ, Kang YM, La Lee Y, Seol SM, Yoon HK, et al. C1q/TNF-related protein-9 inhibits cytokine-induced vascular inflammation and leukocyte adhesiveness via AMP-activated protein kinase activation in endothelial cells. Mol Cell Endocrinol. (2016) 419:235–43. doi: 10.1016/j.mce.2015.10.023
78. Zhang P, Huang C, Li J, Li T, Guo H, Liu T, et al. Globular CTRP9 inhibits oxLDL-induced inflammatory response in RAW 264.7 macrophages via AMPK activation. Mol Cell Biochem. (2016) 417:67–74. doi: 10.1007/s11010-016-2714-1
79. Yi W, Sun Y, Gao E, Wei X, Lau WB, Zheng Q, et al. Reduced cardioprotective action of adiponectin in high-fat diet–induced type II diabetic mice and its underlying mechanisms. Antioxid Redox Signal. (2011) 15:1779–88. doi: 10.1089/ars.2010.3722
80. Sun Y, Yi W, Yuan Y, Lau WB, Yi D, Wang X, et al. C1q/tumor necrosis factor–related protein-9, a novel adipocyte-derived cytokine, attenuates adverse remodeling in the ischemic mouse heart via protein kinase A activation. Circulation. (2013) 128:S113–20. doi: 10.1161/CIRCULATIONAHA.112.000010
81. Yuan Y, Lau WB, Su H, Sun Y, Yi W, Du Y, et al. C1q-TNF-related protein-9, a novel cardioprotetcive cardiokine, requires proteolytic cleavage to generate a biologically active globular domain isoform. Am J Physiol Endocrinol Metab. (2015) 308:E891–8. doi: 10.1152/ajpendo.00450.2014
82. Enomoto T, Ohashi K, Shibata R, Higuchi A, Maruyama S, Izumiya Y, et al. Adipolin/C1qdc2/CTRP12 protein functions as an adipokine that improves glucose metabolism. J Biol Chem. (2011) 286:34552–8. doi: 10.1074/jbc.M111.277319
83. Enomoto T, Shibata R, Ohashi K, Kambara T, Kataoka Y, Uemura Y, et al. Regulation of adipolin/CTRP12 cleavage by obesity. Biochem Biophys Res Commun. (2012) 428:155–9. doi: 10.1016/j.bbrc.2012.10.031
84. Tan BK, Lewandowski KC, O'hare JP, Randeva HS. Insulin regulates the novel adipokine adipolin/CTRP12: in vivo and ex vivo effects. J Endocrinol. (2014) 221:111–9. doi: 10.1530/JOE-13-0537
85. Fadaei R, Moradi N, Kazemi T, Chamani E, Azdaki N, Moezibady SA, et al. Decreased serum levels of CTRP12/adipolin in patients with coronary artery disease in relation to inflammatory cytokines and insulin resistance. Cytokine. (2019) 113:326–31. doi: 10.1016/j.cyto.2018.09.019
86. Babapour B, Doustkami H, Avesta L, Moradi A, Saadat S, Piralaei K, et al. Correlation of serum adipolin with epicardial fat thickness and severity of coronary artery diseases in acute myocardial infarction and stable angina pectoris patients. Med Princ Pract. (2020). doi: 10.1159/000508834. [Epub ahead of print].
87. Ogawa H, Ohashi K, Ito M, Shibata R, Kanemura N, Yuasa D, et al. Adipolin/CTRP12 protects against pathological vascular remodelling through suppression of smooth muscle cell growth and macrophage inflammatory response. Cardiovasc Res. (2020) 116:237–49. doi: 10.1093/cvr/cvz074
88. Takikawa T, Ohashi K, Fang L, Kawanishi H, Otaka N, Ogawa H, et al. P5390 Adipolin/C1q/Tnf-related protein 12 attenuates adverse cardiac remodeling in a mouse model of myocardial infarction. Eur Heart J. (2019) 40:ehz746.0350. doi: 10.1093/eurheartj/ehz746.0350
89. Wong GW, Krawczyk SA, Kitidis-Mitrokostas C, Revett T, Gimeno R, Lodish HF. Molecular, biochemical and functional characterizations of C1q/TNF family members: adipose-tissue-selective expression patterns, regulation by PPAR-γ agonist, cysteine-mediated oligomerizations, combinatorial associations and metabolic functions. Biochem J. (2008) 416:161–77. doi: 10.1042/BJ20081240
90. Xu W, Chen J, Lin J, Liu D, Mo L, Pan W, et al. Exogenous H2S protects H9c2 cardiac cells against high glucose-induced injury and inflammation by inhibiting the activation of the NF-κB and IL-1β pathways. Int J Mol Med. (2015) 35:177–86. doi: 10.3892/ijmm.2014.2007
91. Wu D, Lei H, Wang JY, Zhang CL, Feng H, Fu FY, et al. CTRP3 attenuates post-infarct cardiac fibrosis by targeting Smad3 activation and inhibiting myofibroblast differentiation. J Mol Med. (2015) 93:1311–25. doi: 10.1007/s00109-015-1309-8
92. Wei Z, Peterson JM, Wong GW. Metabolic regulation by C1q/TNF-related Protein-13 (CTRP13) activation of AMP-activated protein kinase and suppression of fatty acid-induced JNK signaling. J Biol Chem. (2011) 286:15652–65. doi: 10.1074/jbc.M110.201087
93. De Souza Batista CM, Yang RZ, Lee MJ, Glynn NM, Yu DZ, Pray J, et al. Omentin plasma levels and gene expression are decreased in obesity. Diabetes. (2007) 56:1655–61. doi: 10.2337/db06-1506
94. Shibata R, Takahashi R, Kataoka Y, Ohashi K, Ikeda N, Kihara S, et al. Association of a fat-derived plasma protein omentin with carotid artery intima-media thickness in apparently healthy men. Hypertens Res. (2011) 34:1309–12. doi: 10.1038/hr.2011.130
95. Yoo HJ, Hwang SY, Hong HC, Choi HY, Yang SJ, Seo JA, et al. Association of circulating omentin-1 level with arterial stiffness and carotid plaque in type 2 diabetes. Cardiovasc Diabetol. (2011) 10:103. doi: 10.1186/1475-2840-10-103
96. Shang FJ, Wang JP, Liu XT, Zheng QS, Xue YS, Wang B, et al. Serum omentin-1 levels are inversely associated with the presence and severity of coronary artery disease in patients with metabolic syndrome. Biomarkers. (2011) 16:657–62. doi: 10.3109/1354750X.2011.622789
97. Yamawaki H, Kuramoto J, Kameshima S, Usui T, Okada M, Hara Y. Omentin, a novel adipocytokine inhibits TNF-induced vascular inflammation in human endothelial cells. Biochem Biophys Res Commun. (2011) 408:339–43. doi: 10.1016/j.bbrc.2011.04.039
98. Yamawaki H, Tsubaki N, Mukohda M, Okada M, Hara Y. Omentin, a novel adipokine, induces vasodilation in rat isolated blood vessels. Biochem Biophys Res Commun. (2010) 393:668–72. doi: 10.1016/j.bbrc.2010.02.053
99. Uemura Y, Shibata R, Kanemura N, Ohashi K, Kambara T, Hiramatsu-Ito M, et al. Adipose-derived protein omentin prevents neointimal formation after arterial injury. FASEB J. (2015) 29:141–51. doi: 10.1096/fj.14-258129
100. Nakamura K, Sano S, Fuster JJ, Kikuchi R, Shimizu I, Ohshima K, et al. Secreted frizzled-related protein 5 diminishes cardiac inflammation and protects the heart from ischemia/reperfusion injury. J Biol Chem. (2016) 291:2566–75. doi: 10.1074/jbc.M115.693937
101. La Cava A, Matarese G. The weight of leptin in immunity. Nat Rev Immunol. (2004) 4:371–9. doi: 10.1038/nri1350
102. Gainsford T, Willson TA, Metcalf D, Handman E, Mcfarlane C, Ng A, et al. Leptin can induce proliferation, differentiation, and functional activation of hemopoietic cells. Proc Natl Acad Sci USA. (1996) 93:14564–8. doi: 10.1073/pnas.93.25.14564
103. Loffreda S, Yang SQ, Lin HZ, Karp CL, Brengman ML, Wang DJ, et al. Leptin regulates proinflammatory immune responses. FASEB J. (1998) 12:57–65. doi: 10.1096/fsb2fasebj.12.1.57
104. Yamagishi SI, Edelstein D, Du XL, Kaneda Y, Guzmán M, Brownlee M. Leptin induces mitochondrial superoxide production and monocyte chemoattractant protein-1 expression in aortic endothelial cells by increasing fatty acid oxidation via protein kinase A. J Biol Chem. (2001) 276:25096–100. doi: 10.1074/jbc.M007383200
105. Mancuso P, Canetti C, Gottschalk A, Tithof PK, Peters-Golden M. Leptin augments alveolar macrophage leukotriene synthesis by increasing phospholipase activity and enhancing group IVC iPLA2 (cPLA2gamma) protein expression. Am J Physiol Lung Cell Mol Physiol. (2004) 287:L497–502. doi: 10.1152/ajplung.00010.2004
106. Bouloumie A, Marumo T, Lafontan M, Busse R. Leptin induces oxidative stress in human endothelial cells. FASEB J. (1999) 13:1231–8. doi: 10.1096/fasebj.13.10.1231
107. Raso GM, Pacilio M, Esposito E, Coppola A, Di Carlo R, Meli R. Leptin potentiates IFN-gamma-induced expression of nitric oxide synthase and cyclo-oxygenase-2 in murine macrophage J774A.1. Br J Pharmacol. (2002) 137:799–804. doi: 10.1038/sj.bjp.0704903
108. Caldefie-Chezet F, Poulin A, Vasson MP. Leptin regulates functional capacities of polymorphonuclear neutrophils. Free Radic Res. (2003) 37:809–14. doi: 10.1080/1071576031000097526
109. Reis BS, Lee K, Fanok MH, Mascaraque C, Amoury M, Cohn LB, et al. Leptin receptor signaling in T cells is required for Th17 differentiation. J Immunol. (2015) 194:5253–60. doi: 10.4049/jimmunol.1402996
110. Steppan CM, Bailey ST, Bhat S, Brown EJ, Banerjee RR, Wright CM, et al. The hormone resistin links obesity to diabetes. Nature. (2001) 409:307–12. doi: 10.1038/35053000
111. Qatanani M, Szwergold NR, Greaves DR, Ahima RS, Lazar MA. Macrophage-derived human resistin exacerbates adipose tissue inflammation and insulin resistance in mice. J Clin Invest. (2009) 119:531–9. doi: 10.1172/JCI37273
112. Lee S, Lee HC, Kwon YW, Lee SE, Cho Y, Kim J, et al. Adenylyl cyclase-associated protein 1 is a receptor for human resistin and mediates inflammatory actions of human monocytes. Cell Metab. (2014) 19:484–97. doi: 10.1016/j.cmet.2014.01.013
113. Benomar Y, Taouis M. Molecular mechanisms underlying obesity-induced hypothalamic inflammation and insulin resistance: pivotal role of resistin/TLR4 pathways. Front Endocrinol. (2019) 10:140. doi: 10.3389/fendo.2019.00140
114. Drolet R, Richard C, Sniderman A, Mailloux J, Fortier M, Huot C, et al. Hypertrophy and hyperplasia of abdominal adipose tissues in women. Int J Obes. (2008) 32:283–91. doi: 10.1038/sj.ijo.0803708
115. Fukuhara A, Matsuda M, Nishizawa M, Segawa K, Tanaka M, Kishimoto K, et al. Visfatin: a protein secreted by visceral fat that mimics the effects of insulin. Science. (2005) 307:426–30. doi: 10.1126/science.1097243
116. Chen H, Xia T, Zhou L, Chen X, Gan L, Yao W, et al. Gene organization, alternate splicing and expression pattern of porcine visfatin gene. Domest Anim Endocrinol. (2007) 32:235–45. doi: 10.1016/j.domaniend.2006.03.004
117. Hasmann M, Schemainda I. FK866, a highly specific noncompetitive inhibitor of nicotinamide phosphoribosyltransferase, represents a novel mechanism for induction of tumor cell apoptosis. Cancer Res. (2003) 63:7436–42.
118. Haider DG, Schaller G, Kapiotis S, Maier C, Luger A, Wolzt M. The release of the adipocytokine visfatin is regulated by glucose and insulin. Diabetologia. (2006) 49:1909–14. doi: 10.1007/s00125-006-0303-7
119. Harasim E, Chabowski A, Gorski J. Lack of downstream insulin-mimetic effects of visfatin/eNAMPT on glucose and fatty acid metabolism in skeletal muscles. Acta Physiol. (2011) 202:21–8. doi: 10.1111/j.1748-1716.2011.02254.x
120. Chen MP, Chung FM, Chang DM, Tsai JCR, Huang HF, Shin SJ, et al. Elevated plasma level of visfatin/pre-B cell colony-enhancing factor in patients with type 2 diabetes mellitus. J Clin Endocrinol Metab. (2006) 91:295–9. doi: 10.1210/jc.2005-1475
121. Retnakaran R, Youn BS, Liu Y, Hanley AJ, Lee NS, Park JW, et al. Correlation of circulating full-length visfatin (PBEF/NAMPT) with metabolic parameters in subjects with and without diabetes: a cross-sectional study. Clin Endocrinol. (2008) 69:885–93. doi: 10.1111/j.1365-2265.2008.03264.x
122. Ingelsson E, Larson MG, Fox CS, Yin X, Wang TJ, Lipinska I, et al. Clinical correlates of circulating visfatin levels in a community-based sample. Diabetes Care. (2007) 30:1278–80. doi: 10.2337/dc06-2353
123. Takebayashi K, Suetsugu M, Wakabayashi S, Aso Y, Inukai T. Association between plasma visfatin and vascular endothelial function in patients with type 2 diabetes mellitus. Metabolism. (2007) 56:451–8. doi: 10.1016/j.metabol.2006.12.001
124. Berndt J, Klöting N, Kralisch S, Kovacs P, Fasshauer M, Schön MR, et al. Plasma visfatin concentrations and fat depot–specific mRNA expression in humans. Diabetes. (2005) 54:2911–6. doi: 10.2337/diabetes.54.10.2911
125. Jian WX, Luo TH, Gu YY, Zhang HL, Zheng S, Dai M, et al. The visfatin gene is associated with glucose and lipid metabolism in a Chinese population. Diabet Med. (2006) 23:967–73. doi: 10.1111/j.1464-5491.2006.01909.x
126. Pagano C, Pilon C, Olivieri M, Mason P, Fabris R, Serra R, et al. Reduced plasma visfatin/pre-B cell colony-enhancing factor in obesity is not related to insulin resistance in humans. J Clin Endocrinol Metab. (2006) 91:3165–70. doi: 10.1210/jc.2006-0361
127. Sethi JK. Is PBEF/visfatin/Nampt an authentic adipokine relevant to the metabolic syndrome? Curr Hypertens Rep. (2007) 9:33. doi: 10.1007/s11906-007-0007-5
128. Chang YC, Chang TJ, Lee WJ, Chuang LM. The relationship of visfatin/pre–B-cell colony-enhancing factor/nicotinamide phosphoribosyltransferase in adipose tissue with inflammation, insulin resistance, and plasma lipids. Metab Clin Exp. (2010) 59:93–9. doi: 10.1016/j.metabol.2009.07.011
129. Laight DW, Kengatharan K, Gopaul NK, Änggård EE, Carrier MJ. Investigation of oxidant stress and vasodepression to glyceryl trinitrate in the obese Zucker rat in vivo. Br J Pharmacol. (1998) 125:895–901. doi: 10.1038/sj.bjp.0702132
130. Guo H, Bazuine M, Jin D, Huang MM, Cushman SW, Chen X. Evidence for the regulatory role of lipocalin 2 in high-fat diet-induced adipose tissue remodeling in male mice. Endocrinology. (2013) 154:3525–38. doi: 10.1210/en.2013-1289
131. Apostolopoulos V, De Courten MP, Stojanovska L, Blatch GL, Tangalakis K, De Courten B. The complex immunological and inflammatory network of adipose tissue in obesity. Mol Nutr Food Res. (2016) 60:43–57. doi: 10.1002/mnfr.201500272
132. Ortega-Senovilla H, De Oya M, Garcés C. Relationship of NEFA concentrations to RBP4 and to RBP4/retinol in prepubertal children with and without obesity. J Clin Lipidol. (2019) 13:301–7. doi: 10.1016/j.jacl.2019.01.006
133. Satish M, Saxena SK, Agrawal DK. Adipokine dysregulation and insulin resistance with atherosclerotic vascular disease: metabolic syndrome or independent sequelae? J Cardiovasc Transl Res. (2019) 12:415–24. doi: 10.1007/s12265-019-09879-0
134. Tabak O, Simsek G, Erdenen F, Sozer V, Hasoglu T, Gelisgen R, et al. The relationship between circulating irisin, retinol binding protein-4, adiponectin and inflammatory mediators in patients with metabolic syndrome. Archiv Endocrinol Metab. (2017) 61:515–23. doi: 10.1590/2359-3997000000289
135. Thapa B, Lee K. Metabolic influence on macrophage polarization and pathogenesis. BMB Rep. (2019) 52:360. doi: 10.5483/BMBRep.2019.52.6.140
136. Pirzgalska RM, Domingos AI. Macrophages in obesity. Cell Immunol. (2018) 330:183–7. doi: 10.1016/j.cellimm.2018.04.014
137. Shapouri-Moghaddam A, Mohammadian S, Vazini H, Taghadosi M, Esmaeili SA, Mardani F, et al. Macrophage plasticity, polarization, and function in health and disease. J Cell Physiol. (2018) 233:6425–40. doi: 10.1002/jcp.26429
138. Caslin HL, Bhanot M, Bolus WR, Hasty AH. Adipose tissue macrophages: unique polarization and bioenergetics in obesity. Immunol Rev. (2020) 295:101–13. doi: 10.1111/imr.12853
139. Wang T, Liu H, Lian G, Zhang SY, Wang X, Jiang C. HIF1α-induced glycolysis metabolism is essential to the activation of inflammatory macrophages. Mediators Inflamm. (2017) 2017:9029327. doi: 10.1155/2017/9029327
140. Boutens L, Hooiveld GJ, Dhingra S, Cramer RA, Netea MG, Stienstra R. Unique metabolic activation of adipose tissue macrophages in obesity promotes inflammatory responses. Diabetologia. (2018) 61:942–53. doi: 10.1007/s00125-017-4526-6
141. Onogi Y, Wada T, Okekawa A, Matsuzawa T, Watanabe E, Ikeda K, et al. Pro-inflammatory macrophages coupled with glycolysis remodel adipose vasculature by producing platelet-derived growth factor-B in obesity. Sci Rep. (2020) 10:670. doi: 10.1038/s41598-019-57368-w
142. Koo SJ, Szczesny B, Wan X, Putluri N, Garg NJ. Pentose phosphate shunt modulates reactive oxygen species and nitric oxide production controlling Trypanosoma cruzi in macrophages. Front Immunol. (2018) 9:202. doi: 10.3389/fimmu.2018.00202
143. Ryan DG, O'neill LA. Krebs cycle rewired for macrophage and dendritic cell effector functions. FEBS Lett. (2017) 591:2992–3006. doi: 10.1002/1873-3468.12744
144. Im SS, Yousef L, Blaschitz C, Liu JZ, Edwards RA, Young SG, et al. Linking lipid metabolism to the innate immune response in macrophages through sterol regulatory element binding protein-1a. Cell Metab. (2011) 13:540–9. doi: 10.1016/j.cmet.2011.04.001
145. Lee JH, Phelan P, Shin M, Oh BC, Han X, Im SS, et al. SREBP-1a–stimulated lipid synthesis is required for macrophage phagocytosis downstream of TLR4-directed mTORC1. Proc Natl Acad Sci USA. (2018) 115:E12228–34. doi: 10.1073/pnas.1813458115
146. Moon JS, Lee S, Park MA, Siempos II, Haslip M, Lee PJ, et al. UCP2-induced fatty acid synthase promotes NLRP3 inflammasome activation during sepsis. J Clin Invest. (2015) 125:665–80. doi: 10.1172/JCI78253
147. Palmieri EM, Menga A, Martín-Pérez R, Quinto A, Riera-Domingo C, De Tullio G, et al. Pharmacologic or genetic targeting of glutamine synthetase skews macrophages toward an M1-like phenotype and inhibits tumor metastasis. Cell Rep. (2017) 20:1654–66. doi: 10.1016/j.celrep.2017.07.054
148. Moon JS, Nakahira K, Chung KP, Denicola GM, Koo MJ, Pabón MA, et al. NOX4-dependent fatty acid oxidation promotes NLRP3 inflammasome activation in macrophages. Nat Med. (2016) 22:1002–12. doi: 10.1038/nm.4153
149. Wang F, Zhang S, Vuckovic I, Jeon R, Lerman A, Folmes CD, et al. Glycolytic stimulation is not a requirement for M2 macrophage differentiation. Cell Metab. (2018) 28:463–75.e464. doi: 10.1016/j.cmet.2018.08.012
150. Ren W, Xia Y, Chen S, Wu G, Bazer FW, Zhou B, et al. Glutamine metabolism in macrophages: a novel target for obesity/type 2 diabetes. Adv Nutr. (2019) 10:321–30. doi: 10.1093/advances/nmy084
151. Vats D, Mukundan L, Odegaard JI, Zhang L, Smith KL, Morel CR, et al. Oxidative metabolism and PGC-1beta attenuate macrophage-mediated inflammation. Cell Metab. (2006) 4:13–24. doi: 10.1016/j.cmet.2006.05.011
152. Huang SCC, Everts B, Ivanova Y, O'sullivan D, Nascimento M, Smith AM, et al. Cell-intrinsic lysosomal lipolysis is essential for alternative activation of macrophages. Nat Immunol. (2014) 15:846–55. doi: 10.1038/ni.2956
153. Namgaladze D, Brüne B. Fatty acid oxidation is dispensable for human macrophage IL-4-induced polarization. Biochim Biophys Acta. (2014) 1841:1329–35. doi: 10.1016/j.bbalip.2014.06.007
154. Nomura M, Liu J, Rovira II, Gonzalez-Hurtado E, Lee J, Wolfgang MJ, et al. Fatty acid oxidation in macrophage polarization. Nat Immunol. (2016) 17:216–7. doi: 10.1038/ni.3366
155. Wen H, Gris D, Lei Y, Jha S, Zhang L, Huang MT-H, et al. Fatty acid–induced NLRP3-ASC inflammasome activation interferes with insulin signaling. Nat Immunol. (2011) 12:408–15. doi: 10.1038/ni.2022
156. Gianfrancesco MA, Dehairs J, L'homme L, Herinckx G, Esser N, Jansen O, et al. Saturated fatty acids induce NLRP3 activation in human macrophages through K+ efflux resulting from phospholipid saturation and Na, K-ATPase disruption. Biochim Biophys Acta. (2019) 1864:1017–30. doi: 10.1016/j.bbalip.2019.04.001
157. Korbecki J, Bajdak-Rusinek K. The effect of palmitic acid on inflammatory response in macrophages: an overview of molecular mechanisms. Inflamm Res. (2019) 68:915–32. doi: 10.1007/s00011-019-01273-5
158. Fernández-Riejos P, Najib S, Santos-Alvarez J, Martín-Romero C, Pérez-Pérez A, González-Yanes C, et al. Role of leptin in the activation of immune cells. Mediators Inflamm. (2010) 2010:568343. doi: 10.1155/2010/568343
159. Tsatsanis C, Zacharioudaki V, Androulidaki A, Dermitzaki E, Charalampopoulos I, Minas V, et al. Adiponectin induces TNF-α and IL-6 in macrophages and promotes tolerance to itself and other pro-inflammatory stimuli. Biochem Biophys Res Commun. (2005) 335:1254–63. doi: 10.1016/j.bbrc.2005.07.197
160. Bertola A, Ciucci T, Rousseau D, Bourlier V, Duffaut C, Bonnafous S, et al. Identification of adipose tissue dendritic cells correlated with obesity-associated insulin-resistance and inducing Th17 responses in mice and patients. Diabetes. (2012) 61:2238–47. doi: 10.2337/db11-1274
161. Stefanovic-Racic M, Yang X, Turner MS, Mantell BS, Stolz DB, Sumpter TL, et al. Dendritic cells promote macrophage infiltration and comprise a substantial proportion of obesity-associated increases in CD11c+ cells in adipose tissue and liver. Diabetes. (2012) 61:2330–9. doi: 10.2337/db11-1523
162. Chen Y, Tian J, Tian X, Tang X, Rui K, Tong J, et al. Adipose tissue dendritic cells enhances inflammation by prompting the generation of Th17 cells. PLoS ONE. (2014) 9:e92450. doi: 10.1371/journal.pone.0092450
163. Patsouris D, Li PP, Thapar D, Chapman J, Olefsky JM, Neels JG. Ablation of CD11c-positive cells normalizes insulin sensitivity in obese insulin resistant animals. Cell Metab. (2008) 8:301–9. doi: 10.1016/j.cmet.2008.08.015
164. Cho KW, Zamarron BF, Muir LA, Singer K, Porsche CE, Delproposto JB, et al. Adipose tissue dendritic cells are independent contributors to obesity-induced inflammation and insulin resistance. J Immunol. (2016) 197:3650–61. doi: 10.4049/jimmunol.1600820
165. Hellmann J, Sansbury BE, Holden CR, Tang Y, Wong B, Wysoczynski M, et al. CCR7 maintains nonresolving lymph node and adipose inflammation in obesity. Diabetes. (2016) 65:2268–81. doi: 10.2337/db15-1689
166. Macdougall CE, Wood EG, Loschko J, Scagliotti V, Cassidy FC, Robinson ME, et al. Visceral adipose tissue immune homeostasis is regulated by the crosstalk between adipocytes and dendritic cell subsets. Cell Metab. (2018) 27:588–601.e584. doi: 10.1016/j.cmet.2018.02.007
167. Ghosh AR, Bhattacharya R, Bhattacharya S, Nargis T, Rahaman O, Duttagupta P, et al. Adipose recruitment and activation of plasmacytoid dendritic cells fuel metaflammation. Diabetes. (2016) 65:3440–52. doi: 10.2337/db16-0331
168. Hannibal TD, Schmidt-Christensen A, Nilsson J, Fransén-Pettersson N, Hansen L, Holmberg D. Deficiency in plasmacytoid dendritic cells and type I interferon signalling prevents diet-induced obesity and insulin resistance in mice. Diabetologia. (2017) 60:2033–41. doi: 10.1007/s00125-017-4341-0
169. Pamir N, Liu NC, Irwin A, Becker L, Peng Y, Ronsein GE, et al. Granulocyte/macrophage colony-stimulating factor-dependent dendritic cells restrain lean adipose tissue expansion. J Biol Chem. (2015) 290:14656–67. doi: 10.1074/jbc.M115.645820
170. Ibrahim J, Nguyen AH, Rehman A, Ochi A, Jamal M, Graffeo CS, et al. Dendritic cell populations with different concentrations of lipid regulate tolerance and immunity in mouse and human liver. Gastroenterology. (2012) 143:1061–72. doi: 10.1053/j.gastro.2012.06.003
171. Den Brok MH, Raaijmakers TK, Collado-Camps E, Adema GJ. Lipid droplets as immune modulators in myeloid cells. Trends Immunol. (2018) 39:380–92. doi: 10.1016/j.it.2018.01.012
172. Wculek SK, Khouili SC, Priego E, Heras-Murillo I, Sancho D. Metabolic control of dendritic cell functions: digesting information. Front Immunol. (2019) 10:775. doi: 10.3389/fimmu.2019.00775
173. Márquez S, Fernández JJ, Terán-Cabanillas E, Herrero C, Alonso S, Azogil A, et al. Endoplasmic reticulum stress sensor IRE1α enhances IL-23 expression by human dendritic cells. Front Immunol. (2017) 8:639. doi: 10.3389/fimmu.2017.00639
174. Everts B, Amiel E, Huang SCC, Smith AM, Chang CH, Lam WY, et al. TLR-driven early glycolytic reprogramming via the kinases TBK1-IKKε supports the anabolic demands of dendritic cell activation. Nat Immunol. (2014) 15:323–32. doi: 10.1038/ni.2833
175. Guak H, Al Habyan S, Ma EH, Aldossary H, Al-Masri M, Won SY, et al. Glycolytic metabolism is essential for CCR7 oligomerization and dendritic cell migration. Nat Commun. (2018) 9:2463. doi: 10.1038/s41467-018-04804-6
176. Williams NC, O'neill LA. A role for the krebs cycle intermediate citrate in metabolic reprogramming in innate immunity and inflammation. Front Immunol. (2018) 9:141. doi: 10.3389/fimmu.2018.00141
177. Pearce EJ, Everts B. Dendritic cell metabolism. Nat Rev Immunol. (2015) 15:18–29. doi: 10.1038/nri3771
178. Weinstock A, Moura Silva H, Moore KJ, Schmidt AM, Fisher EA. Leukocyte heterogeneity in adipose tissue, including in obesity. Circ Res. (2020) 126:1590–612. doi: 10.1161/CIRCRESAHA.120.316203
179. Talukdar S, Oh DY, Bandyopadhyay G, Li D, Xu J, Mcnelis J, et al. Neutrophils mediate insulin resistance in mice fed a high-fat diet through secreted elastase. Nat Med. (2012) 18:1407–12. doi: 10.1038/nm.2885
180. Casanova-Acebes M, Nicolás-Ávila JA, Li JL, García-Silva S, Balachander A, Rubio-Ponce A, et al. Neutrophils instruct homeostatic and pathological states in naive tissues. J Exp Med. (2018) 215:2778–95. doi: 10.1084/jem.20181468
181. Arivazhagan L, Ruiz HH, Wilson RA, Manigrasso MB, Gugger PF, Fisher EA, et al. An eclectic cast of cellular actors orchestrates innate immune responses in the mechanisms driving obesity and metabolic perturbation. Circ Res. (2020) 126:1565–89. doi: 10.1161/CIRCRESAHA.120.315900
182. Watanabe Y, Nagai Y, Honda H, Okamoto N, Yanagibashi T, Ogasawara M, et al. Bidirectional crosstalk between neutrophils and adipocytes promotes adipose tissue inflammation. FASEB J. (2019) 33:11821–35. doi: 10.1096/fj.201900477RR
183. Fridlender ZG, Sun J, Kim S, Kapoor V, Cheng G, Ling L, et al. Polarization of tumor-associated neutrophil phenotype by TGF-β: “N1” versus “N2” TAN. Cancer Cell. (2009) 16:183–94. doi: 10.1016/j.ccr.2009.06.017
184. Ma Y, Yabluchanskiy A, Iyer RP, Cannon PL, Flynn ER, Jung M, et al. Temporal neutrophil polarization following myocardial infarction. Cardiovasc Res. (2016) 110:51–61. doi: 10.1093/cvr/cvw024
185. Kumar S, Dikshit M. Metabolic insight of neutrophils in health and disease. Front Immunol. (2019) 10:2099. doi: 10.3389/fimmu.2019.02099
186. Borregaard N, Herlin T. Energy metabolism of human neutrophils during phagocytosis. J Clin Invest. (1982) 70:550–7. doi: 10.1172/JCI110647
187. Rodríguez-Espinosa O, Rojas-Espinosa O, Moreno-Altamirano MMB, López-Villegas EO, Sánchez-García FJ. Metabolic requirements for neutrophil extracellular traps formation. Immunology. (2015) 145:213–24. doi: 10.1111/imm.12437
188. Six E, Lagresle-Peyrou C, Susini S, De Chappedelaine C, Sigrist N, Sadek H, et al. AK2 deficiency compromises the mitochondrial energy metabolism required for differentiation of human neutrophil and lymphoid lineages. Cell Death Dis. (2015) 6:e1856. doi: 10.1038/cddis.2015.211
189. Fossati G, Moulding DA, Spiller DG, Moots RJ, White MR, Edwards SW. The mitochondrial network of human neutrophils: role in chemotaxis, phagocytosis, respiratory burst activation, and commitment to apoptosis. J Immunol. (2003) 170:1964–72. doi: 10.4049/jimmunol.170.4.1964
190. Zmijewski JW, Lorne E, Banerjee S, Abraham E. Participation of mitochondrial respiratory complex III in neutrophil activation and lung injury. Am J Physiol Lung Cell Mol Physiol. (2009) 296:L624–34. doi: 10.1152/ajplung.90522.2008
191. Zhou W, Cao L, Jeffries J, Zhu X, Staiger CJ, Deng Q. Neutrophil-specific knockout demonstrates a role for mitochondria in regulating neutrophil motility in zebrafish. Dis Models Mech. (2018) 11:dmm033027. doi: 10.1242/dmm.033027
192. Azevedo EP, Rochael NC, Guimarães-Costa AB, De Souza-Vieira TS, Ganilho J, Saraiva EM, et al. A metabolic shift toward pentose phosphate pathway is necessary for amyloid fibril-and phorbol 12-myristate 13-acetate-induced neutrophil extracellular trap (NET) formation. J Biol Chem. (2015) 290:22174–83. doi: 10.1074/jbc.M115.640094
193. Ham M, Choe SS, Shin KC, Choi G, Kim JW, Noh JR, et al. Glucose-6-phosphate dehydrogenase deficiency improves insulin resistance with reduced adipose tissue inflammation in obesity. Diabetes. (2016) 65:2624–38. doi: 10.2337/db16-0060
194. Pesce S, Greppi M, Tabellini G, Rampinelli F, Parolini S, Olive D, et al. Identification of a subset of human natural killer cells expressing high levels of programmed death 1: a phenotypic and functional characterization. J Allergy Clin Immunol. (2017) 139:335–46.e333. doi: 10.1016/j.jaci.2016.04.025
195. Amankulor NM, Kim Y, Arora S, Kargl J, Szulzewsky F, Hanke M, et al. Mutant IDH1 regulates the tumor-associated immune system in gliomas. Genes Dev. (2017) 31:774–86. doi: 10.1101/gad.294991.116
196. RoŽman S, Yousefi S, Oberson K, Kaufmann T, Benarafa C, Simon H-U. The generation of neutrophils in the bone marrow is controlled by autophagy. Cell Death Differ. (2015) 22:445–56. doi: 10.1038/cdd.2014.169
197. Riffelmacher T, Clarke A, Richter FC, Stranks A, Pandey S, Danielli S, et al. Autophagy-dependent generation of free fatty acids is critical for normal neutrophil differentiation. Immunity. (2017) 47:466–80.e465. doi: 10.1016/j.immuni.2017.08.005
198. Lodhi IJ, Wei X, Yin L, Feng C, Adak S, Abou-Ezzi G, et al. Peroxisomal lipid synthesis regulates inflammation by sustaining neutrophil membrane phospholipid composition and viability. Cell Metab. (2015) 21:51–64. doi: 10.1016/j.cmet.2014.12.002
199. Rice CM, Davies LC, Subleski JJ, Maio N, Gonzalez-Cotto M, Andrews C, et al. Tumour-elicited neutrophils engage mitochondrial metabolism to circumvent nutrient limitations and maintain immune suppression. Nat Commun. (2018) 9:5099. doi: 10.1038/s41467-018-07505-2
200. Porter L, Toepfner N, Bashant KR, Guck J, Ashcroft M, Farahi N, et al. Metabolic profiling of human eosinophils. Front Immunol. (2018) 9:1404. doi: 10.3389/fimmu.2018.01404
201. Jones N, Vincent EE, Felix LC, Cronin JG, Scott LM, Hole PS, et al. Interleukin-5 drives glycolysis and reactive oxygen species-dependent citric acid cycling by eosinophils. Allergy. (2020) 75:1361–70. doi: 10.1111/all.14158
202. Chakravarty N. Further observations on the inhibition of histamine release by 2-deoxyglucose. Acta Physiol Scand. (1968) 72:425–32. doi: 10.1111/j.1365-201X.1968.tb10852.x
203. Kitahata Y, Nunomura S, Terui T, Ra C. Prolonged culture of mast cells with high-glucose medium enhances the Fc epsilon RI-mediated degranulation response and leukotriene C4 production. Int Arch Allergy Immunol. (2010) 152:22–31. doi: 10.1159/000312122
204. Michaeloudes C, Bhavsar PK, Mumby S, Xu B, Hui CKM, Chung KF, et al. Role of metabolic reprogramming in pulmonary innate immunity and its impact on lung diseases. J Innate Immun. (2020) 12:31–46. doi: 10.1159/000504344
205. Ryu H, Walker JK, Kim S, Koo N, Barak LS, Noguchi T, et al. Regulation of M2-type pyruvate kinase mediated by the high-affinity IgE receptors is required for mast cell degranulation. Br J Pharmacol. (2008) 154:1035–46. doi: 10.1038/bjp.2008.148
206. Van Der Zande HJ, Zawistowska-Deniziak A, Guigas B. Immune regulation of metabolic homeostasis by Helminths and their molecules. Trends Parasitol. (2019) 35:795–808. doi: 10.1016/j.pt.2019.07.014
207. Molofsky AB, Nussbaum JC, Liang HE, Van Dyken SJ, Cheng LE, Mohapatra A, et al. Innate lymphoid type 2 cells sustain visceral adipose tissue eosinophils and alternatively activated macrophages. J ExpMed. (2013) 210:535–49. doi: 10.1084/jem.20121964
208. Rana BM, Jou E, Barlow JL, Rodriguez-Rodriguez N, Walker JA, Knox C, et al. A stromal cell niche sustains ILC2-mediated type-2 conditioning in adipose tissue. J Exp Med. (2019) 216:1999–2009. doi: 10.1084/jem.20190689
209. Wu D, Molofsky AB, Liang HE, Ricardo-Gonzalez RR, Jouihan HA, Bando JK, et al. Eosinophils sustain adipose alternatively activated macrophages associated with glucose homeostasis. Science. (2011) 332:243–7. doi: 10.1126/science.1201475
210. Yoon J, Um HN, Jang J, Bae YA, Park WJ, Kim HJ, et al. Eosinophil activation by toll-like receptor 4 ligands regulates macrophage polarization. Front Cell Dev Biol. (2019) 7:329. doi: 10.3389/fcell.2019.00329
211. Lee EH, Itan M, Jang J, Gu HJ, Rozenberg P, Mingler MK, et al. Eosinophils support adipocyte maturation and promote glucose tolerance in obesity. Sci Rep. (2018) 8:9894. doi: 10.1038/s41598-018-28371-4
212. Yu C, Cantor AB, Yang H, Browne C, Wells RA, Fujiwara Y, et al. Targeted deletion of a high-affinity GATA-binding site in the GATA-1 promoter leads to selective loss of the eosinophil lineage in vivo. J Exp Med. (2002) 195:1387–95. doi: 10.1084/jem.20020656
213. Berbudi A, Surendar J, Ajendra J, Gondorf F, Schmidt D, Neumann AL, et al. Filarial infection or antigen administration improves glucose tolerance in diet-induced obese mice. J Innate Immun. (2016) 8:601–16. doi: 10.1159/000448401
214. Van Der Kolk BW, Kalafati M, Adriaens M, Van Greevenbroek MMJ, Vogelzangs N, Saris WHM, et al. Subcutaneous adipose tissue and systemic inflammation are associated with peripheral but not hepatic insulin resistance in humans. Diabetes. (2019) 68:2247–58. doi: 10.2337/db19-0560
215. Qiu Y, Nguyen KD, Odegaard JI, Cui X, Tian X, Locksley RM, et al. Eosinophils and type 2 cytokine signaling in macrophages orchestrate development of functional beige fat. Cell. (2014) 157:1292–308. doi: 10.1016/j.cell.2014.03.066
216. Qin M, Wang L, Li F, Yang M, Song L, Tian F, et al. Oxidized LDL activated eosinophil polarize macrophage phenotype from M2 to M1 through activation of CD36 scavenger receptor. Atherosclerosis. (2017) 263:82–91. doi: 10.1016/j.atherosclerosis.2017.05.011
217. Weller PF, Spencer LA. Functions of tissue-resident eosinophils. Nat Rev Immunol. (2017) 17:746–60. doi: 10.1038/nri.2017.95
218. Wong CK, Cheung PFY, Lam CW. Leptin-mediated cytokine release and migration of eosinophils: implications for immunopathophysiology of allergic inflammation. Eur J Immunol. (2007) 37:2337–48. doi: 10.1002/eji.200636866
219. Kato H, Ueki S, Kamada R, Kihara J, Yamauchi Y, Suzuki T, et al. Leptin has a priming effect on eotaxin-induced human eosinophil chemotaxis. Int Archiv Allergy Immunol. (2011) 155:335–44. doi: 10.1159/000321195
220. Calco GN, Fryer AD, Nie Z. Unraveling the connection between eosinophils and obesity. J Leukoc Biol. (2020) 108:123–8. doi: 10.1002/JLB.5MR0120-377R
221. Liu J, Divoux A, Sun J, Zhang J, Clément K, Glickman JN, et al. Genetic deficiency and pharmacological stabilization of mast cells reduce diet-induced obesity and diabetes in mice. Nat Med. (2009) 15:940–5. doi: 10.1038/nm.1994
222. Kumar D, Pandya SK, Varshney S, Shankar K, Rajan S, Srivastava A, et al. Temporal immmunometabolic profiling of adipose tissue in HFD-induced obesity: manifestations of mast cells in fibrosis and senescence. Int J Obes. (2019) 43:1281–94. doi: 10.1038/s41366-018-0228-5
223. Divoux A, Moutel S, Poitou C, Lacasa D, Veyrie N, Aissat A, et al. Mast cells in human adipose tissue: link with morbid obesity, inflammatory status, and diabetes. J Clin Endocrinol Metabol. (2012) 97:E1677–85. doi: 10.1210/jc.2012-1532
224. Gurung P, Moussa K, Adams-Huet B, Devaraj S, Jialal I. Increased mast cell abundance in adipose tissue of metabolic syndrome: relevance to the proinflammatory state and increased adipose tissue fibrosis. Am J Physiol Endocrinol Metab. (2019) 316:E504–9. doi: 10.1152/ajpendo.00462.2018
225. Hirai S, Ohyane C, Kim YI, Lin S, Goto T, Takahashi N, et al. Involvement of mast cells in adipose tissue fibrosis. Am J Physiol Endocrinol Metabol. (2014) 306:E247–55. doi: 10.1152/ajpendo.00056.2013
226. Tanaka A, Nomura Y, Matsuda A, Ohmori K, Matsuda H. Mast cells function as an alternative modulator of adipogenesis through 15-deoxy-delta-12:14-prostaglandin J2. Am J Physiol Cell Physiol. (2011) 301:C1360–7. doi: 10.1152/ajpcell.00514.2010
227. Ishijima Y, Ohmori SY, Ohneda K. Mast cell deficiency results in the accumulation of preadipocytes in adipose tissue in both obese and non-obese mice. FEBS Open Bio. (2014) 4:18–24. doi: 10.1016/j.fob.2013.11.004
228. Gutierrez DA, Muralidhar S, Feyerabend TB, Herzig S, Rodewald H-R. Hematopoietic kit deficiency, rather than lack of mast cells, protects mice from obesity and insulin resistance. Cell Metabol. (2015) 21:678–91. doi: 10.1016/j.cmet.2015.04.013
229. Chmelar J, Chatzigeorgiou A, Chung KJ, Prucnal M, Voehringer D, Roers A, et al. No role for mast cells in obesity-related metabolic dysregulation. Front Immunol. (2016) 7:524. doi: 10.3389/fimmu.2016.00524
230. Caslin HL, Abebayehu D, Abdul Qayum A, Haque TT, Taruselli MT, Paez PA, et al. Lactic acid inhibits lipopolysaccharide-induced mast cell function by limiting glycolysis and ATP availability. J Immunol. (2019) 203:453–64. doi: 10.4049/jimmunol.1801005
231. Wu H, Ghosh S, Perrard XD, Feng L, Garcia GE, Perrard JL, et al. T-cell accumulation and regulated on activation, normal T cell expressed and secreted upregulation in adipose tissue in obesity. Circulation. (2007) 115:1029. doi: 10.1161/CIRCULATIONAHA.106.638379
232. Feuerer M, Herrero L, Cipolletta D, Naaz A, Wong J, Nayer A, et al. Lean, but not obese, fat is enriched for a unique population of regulatory T cells that affect metabolic parameters. Nat Med. (2009) 15:930–9. doi: 10.1038/nm.2002
233. Schipper HS, Prakken B, Kalkhoven E, Boes M. Adipose tissue-resident immune cells: key players in immunometabolism. Trends Endocrinol Metabol. (2012) 23:407–15. doi: 10.1016/j.tem.2012.05.011
234. Kintscher U, Hartge M, Hess K, Foryst-Ludwig A, Clemenz M, Wabitsch M, et al. T-lymphocyte infiltration in visceral adipose tissue: a primary event in adipose tissue inflammation and the development of obesity-mediated insulin resistance. Arterioscler Thromb Vasc Biol. (2008) 28:1304–10. doi: 10.1161/ATVBAHA.108.165100
235. Nishimura S, Manabe I, Nagasaki M, Eto K, Yamashita H, Ohsugi M, et al. CD8+ effector T cells contribute to macrophage recruitment and adipose tissue inflammation in obesity. Nat Med. (2009) 15:914–20. doi: 10.1038/nm.1964
236. Strissel KJ, Defuria J, Shaul ME, Bennett G, Greenberg AS, Obin MS. T-cell recruitment and Th1 polarization in adipose tissue during diet-induced obesity in C57BL/6 mice. Obesity. (2010) 18:1918–25. doi: 10.1038/oby.2010.1
237. Jaitin DA, Adlung L, Thaiss CA, Weiner A, Li B, Descamps H, et al. Lipid-associated macrophages control metabolic homeostasis in a Trem2-dependent manner. Cell. (2019) 178:686–98.e614. doi: 10.1016/j.cell.2019.05.054
238. Shirakawa K, Yan X, Shinmura K, Endo J, Kataoka M, Katsumata Y, et al. Obesity accelerates T cell senescence in murine visceral adipose tissue. J Clin Invest. (2016) 126:4626–39. doi: 10.1172/JCI88606
239. Yang H, Youm YH, Vandanmagsar B, Ravussin A, Gimble JM, Greenway F, et al. Obesity increases the production of proinflammatory mediators from adipose tissue T cells and compromises TCR repertoire diversity: implications for systemic inflammation and insulin resistance. J Immunol. (2010) 185:1836–45. doi: 10.4049/jimmunol.1000021
240. Mcdonnell WJ, Koethe JR, Mallal SA, Pilkinton MA, Kirabo A, Ameka MK, et al. High CD8 T-cell receptor clonality and altered CDR3 properties are associated with elevated isolevuglandins in adipose tissue during diet-induced obesity. Diabetes. (2018) 67:2361–76. doi: 10.2337/db18-0040
241. Pavlova NN, Thompson CB. The emerging hallmarks of cancer metabolism. Cell Metab. (2016) 23:27–47. doi: 10.1016/j.cmet.2015.12.006
242. Winer S, Chan Y, Paltser G, Truong D, Tsui H, Bahrami J, et al. Normalization of obesity-associated insulin resistance through immunotherapy. Nat Med. (2009) 15:921–9. doi: 10.1038/nm.2001
243. Morris DL, Cho KW, Delproposto JL, Oatmen KE, Geletka LM, Martinez-Santibanez G, et al. Adipose tissue macrophages function as antigen-presenting cells and regulate adipose tissue CD4+ T cells in mice. Diabetes. (2013) 62:2762–72. doi: 10.2337/db12-1404
244. Neumann JT, Tzikas S, Funke-Kaiser A, Wilde S, Appelbaum S, Keller T, et al. Association of MR-proadrenomedullin with cardiovascular risk factors and subclinical cardiovascular disease. Atherosclerosis. (2013) 228:451–9. doi: 10.1016/j.atherosclerosis.2013.03.006
245. Rocha VZ, Folco EJ, Sukhova G, Shimizu K, Gotsman I, Vernon AH, et al. Interferon-γ, a Th1 cytokine, regulates fat inflammation: a role for adaptive immunity in obesity. Circ Res. (2008) 103:467–76. doi: 10.1161/CIRCRESAHA.108.177105
246. Mclaughlin T, Liu LF, Lamendola C, Shen L, Morton J, Rivas H, et al. T-cell profile in adipose tissue is associated with insulin resistance and systemic inflammation in humans. Arterioscler Thromb Vasc Biol. (2014) 34:2637–43. doi: 10.1161/ATVBAHA.114.304636
247. Fabbrini E, Cella M, Mccartney SA, Fuchs A, Abumrad NA, Pietka TA, et al. Association between specific adipose tissue CD4+ T-cell populations and insulin resistance in obese individuals. Gastroenterology. (2013) 145:366–74.e363. doi: 10.1053/j.gastro.2013.04.010
248. Zúñiga LA, Shen WJ, Joyce-Shaikh B, Pyatnova EA, Richards AG, Thom C, et al. IL-17 regulates adipogenesis, glucose homeostasis, and obesity. J Immunol. (2010) 185:6947–59. doi: 10.4049/jimmunol.1001269
249. Chapman NM, Boothby MR, Chi H. Metabolic coordination of T cell quiescence and activation. Nat Rev Immunol. (2020) 20:55–70. doi: 10.1038/s41577-019-0203-y
250. Michalek RD, Gerriets VA, Jacobs SR, Macintyre AN, Maciver NJ, Mason EF, et al. Cutting edge: distinct glycolytic and lipid oxidative metabolic programs are essential for effector and regulatory CD4+ T cell subsets. J Immunol. (2011) 186:3299–303. doi: 10.4049/jimmunol.1003613
251. Berod L, Friedrich C, Nandan A, Freitag J, Hagemann S, Harmrolfs K, et al. De novo fatty acid synthesis controls the fate between regulatory T and T helper 17 cells. Nat Med. (2014) 20:1327–33. doi: 10.1038/nm.3704
252. Procaccini C, Carbone F, Di Silvestre D, Brambilla F, De Rosa V, Galgani M, et al. The proteomic landscape of human ex vivo regulatory and conventional T cells reveals specific metabolic requirements. Immunity. (2016) 44:406–21. doi: 10.1016/j.immuni.2016.01.028
253. Miska J, Lee-Chang C, Rashidi A, Muroski ME, Chang AL, Lopez-Rosas A, et al. HIF-1α is a metabolic switch between glycolytic-driven migration and oxidative phosphorylation-driven immunosuppression of Tregs in glioblastoma. Cell Rep. (2019) 27:226–37.e224. doi: 10.1016/j.celrep.2019.03.029
254. Kishore M, Cheung KCP, Fu H, Bonacina F, Wang G, Coe D, et al. Regulatory T cell migration is dependent on glucokinase-mediated glycolysis. Immunity. (2017) 47:875–89.e810. doi: 10.1016/j.immuni.2017.10.017
255. Yang Q, Liu X, Liu Q, Guan Z, Luo J, Cao G, et al. Roles of mTORC1 and mTORC2 in controlling γδ T1 and γδ T17 differentiation and function. Cell Death Differ. (2020) 27:2248–62. doi: 10.1038/s41418-020-0500-9
256. Cai Y, Xue F, Qin H, Chen X, Liu N, Fleming C, et al. Differential roles of the mTOR-STAT3 signaling in dermal γδ T cell effector function in skin inflammation. Cell Rep. (2019) 27:3034–48.e5. doi: 10.1016/j.celrep.2019.05.019
257. Fu S, Zhu S, Tian C, Bai S, Zhang J, Zhan C, et al. Immunometabolism regulates TCR recycling and iNKT cell functions. Sci Signal. (2019) 12:eaau1788. doi: 10.1126/scisignal.aau1788
258. Kumar A, Pyaram K, Yarosz EL, Hong H, Lyssiotis CA, Giri S, et al. Enhanced oxidative phosphorylation in NKT cells is essential for their survival and function. Proc Natl Acad Sci USA. (2019) 116:7439–48. doi: 10.1073/pnas.1901376116
259. Fu S, He K, Tian C, Sun H, Zhu C, Bai S, et al. Impaired lipid biosynthesis hinders anti-tumor efficacy of intratumoral iNKT cells. Nat Commun. (2020) 11:438. doi: 10.1038/s41467-020-14332-x
260. Deiuliis J, Shah Z, Shah N, Needleman B, Mikami D, Narula V, et al. Visceral adipose inflammation in obesity is associated with critical alterations in tregulatory cell numbers. PLoS ONE. (2011) 6:e16376. doi: 10.1371/journal.pone.0016376
261. Gyllenhammer LE, Lam J, Alderete TL, Allayee H, Akbari O, Katkhouda N, et al. Lower omental t-regulatory cell count is associated with higher fasting glucose and lower β-cell function in adults with obesity. Obesity. (2016) 24:1274–82. doi: 10.1002/oby.21507
262. Ilan Y, Maron R, Tukpah AM, Maioli TU, Murugaiyan G, Yang K, et al. Induction of regulatory T cells decreases adipose inflammation and alleviates insulin resistance in ob/ob mice. Proc Natl Acad Sci USA. (2010) 107:9765–70. doi: 10.1073/pnas.0908771107
263. Cipolletta D, Feuerer M, Li A, Kamei N, Lee J, Shoelson SE, et al. PPAR-γ is a major driver of the accumulation and phenotype of adipose tissue Treg cells. Nature. (2012) 486:549–53. doi: 10.1038/nature11132
264. Cipolletta D, Cohen P, Spiegelman BM, Benoist C, Mathis D. Appearance and disappearance of the mRNA signature characteristic of Treg cells in visceral adipose tissue: age, diet, and PPARγ effects. Proc Natl Acad Sci USA. (2015) 112:482–7. doi: 10.1073/pnas.1423486112
265. Li C, Dispirito JR, Zemmour D, Spallanzani RG, Kuswanto W, Benoist C, et al. TCR transgenic mice reveal stepwise, multi-site acquisition of the distinctive fat-Treg phenotype. Cell. (2018) 174:285–99.e212. doi: 10.1016/j.cell.2018.05.004
266. Han JM, Wu D, Denroche HC, Yao Y, Verchere CB, Levings MK. IL-33 reverses an obesity-induced deficit in visceral adipose tissue ST2+ T regulatory cells and ameliorates adipose tissue inflammation and insulin resistance. J Immunol. (2015) 194:4777–83. doi: 10.4049/jimmunol.1500020
267. Vasanthakumar A, Moro K, Xin A, Liao Y, Gloury R, Kawamoto S, et al. The transcriptional regulators IRF4, BATF and IL-33 orchestrate development and maintenance of adipose tissue–resident regulatory T cells. Nat Immunol. (2015) 16:276–85. doi: 10.1038/ni.3085
268. Kohlgruber AC, Gal-Oz ST, Lamarche NM, Shimazaki M, Duquette D, Koay HF, et al. γδ T cells producing interleukin-17A regulate adipose regulatory T cell homeostasis and thermogenesis. Nat Immunol. (2018) 19:464–74. doi: 10.1038/s41590-018-0094-2
269. Mahlakõiv T, Flamar AL, Johnston L, Moriyama S, Putzel G, Bryce P, et al. Stromal cells maintain immune cell homeostasis in adipose tissue via production of interleukin-33. Sci Immunol. (2019) 4:eaax0416. doi: 10.1126/sciimmunol.aax0416
270. Spallanzani RG, Zemmour D, Xiao T, Jayewickreme T, Li C, Bryce PJ, et al. Distinct immunocyte-promoting and adipocyte-generating stromal components coordinate adipose tissue immune and metabolic tenors. Sci Immunol. (2019) 4:eaaw3658. doi: 10.1126/sciimmunol.aaw3658
271. Deng T, Liu J, Deng Y, Minze L, Xiao X, Wright V, et al. Adipocyte adaptive immunity mediates diet-induced adipose inflammation and insulin resistance by decreasing adipose Treg cells. Nat Commun. (2017) 8:1–11. doi: 10.1038/ncomms15725
272. Pacella I, Piconese S. Immunometabolic checkpoints of treg dynamics: adaptation to microenvironmental opportunities and challenges. Front Immunol. (2019) 10:1889. doi: 10.3389/fimmu.2019.01889
273. De Rosa V, Procaccini C, Calì G, Pirozzi G, Fontana S, Zappacosta S, et al. A key role of leptin in the control of regulatory T cell proliferation. Immunity. (2007) 26:241–55. doi: 10.1016/j.immuni.2007.01.011
274. Zeng C, Shi X, Zhang B, Liu H, Zhang L, Ding W, et al. The imbalance of Th17/Th1/Tregs in patients with type 2 diabetes: relationship with metabolic factors and complications. J Mol Med. (2012) 90:175–86. doi: 10.1007/s00109-011-0816-5
275. Van Exel E, Gussekloo J, De Craen AJ, Frölich M, Bootsma-Van Der Wiel A, Westendorp RG. Low production capacity of interleukin-10 associates with the metabolic syndrome and type 2 diabetes: the leiden 85-plus study. Diabetes. (2002) 51:1088–92. doi: 10.2337/diabetes.51.4.1088
276. Mehta P, Nuotio-Antar AM, Smith CW. γδ T cells promote inflammation and insulin resistance during high fat diet-induced obesity in mice. J Leukoc Biol. (2015) 97:121–34. doi: 10.1189/jlb.3A0414-211RR
277. Costanzo AE, Taylor KR, Dutt S, Han PP, Fujioka K, Jameson JM. Obesity impairs γδ T cell homeostasis and antiviral function in humans. PLoS ONE. (2015) 10:e0120918. doi: 10.1371/journal.pone.0120918
278. Le Menn G, Sibille B, Murdaca J, Rousseau AS, Squillace R, Vergoni B, et al. Decrease in αβ/γδ T-cell ratio is accompanied by a reduction in high-fat diet-induced weight gain, insulin resistance, and inflammation. FASEB J. (2019) 33:2553–62. doi: 10.1096/fj.201800696RR
279. Goldberg EL, Shchukina I, Asher JL, Sidorov S, Artyomov MN, Dixit VD. Ketogenesis activates metabolically protective γδ T cells in visceral adipose tissue. Nat Metabol. (2020) 2:50–61. doi: 10.1038/s42255-019-0160-6
280. Hogquist K, Georgiev H. Recent advances in iNKT cell development. F1000Res. (2020) 9:F1000 Faculty Rev-127. doi: 10.12688/f1000research.21378.1
281. Lamarche NM, Kane H, Kohlgruber AC, Dong H, Lynch L, Brenner MB. Distinct iNKT cell populations use IFNγ or ER stress-induced IL-10 to control adipose tissue homeostasis. Cell Metab. (2020) 32:243–58.e6. doi: 10.1016/j.cmet.2020.05.017
282. Schipper HS, Rakhshandehroo M, Van De Graaf SF, Venken K, Koppen A, Stienstra R, et al. Natural killer T cells in adipose tissue prevent insulin resistance. J Clin Invest. (2012) 122:3343–54. doi: 10.1172/JCI62739
283. Lynch L, Michelet X, Zhang S, Brennan PJ, Moseman A, Lester C, et al. Regulatory i NKT cells lack expression of the transcription factor PLZF and control the homeostasis of T reg cells and macrophages in adipose tissue. Nat Immunol. (2015) 16:85–95. doi: 10.1038/ni.3047
284. Lynch L, Nowak M, Varghese B, Clark J, Hogan AE, Toxavidis V, et al. Adipose tissue invariant NKT cells protect against diet-induced obesity and metabolic disorder through regulatory cytokine production. Immunity. (2012) 37:574–87. doi: 10.1016/j.immuni.2012.06.016
285. Huh JY, Park YJ, Kim JB. Adipocyte CD1d determines adipose inflammation and insulin resistance in obesity. Adipocyte. (2018) 7:129–36. doi: 10.1080/21623945.2018.1440928
286. Lynch L, O'shea D, Winter DC, Geoghegan J, Doherty DG, O'farrelly C. Invariant NKT cells and CD1d+ cells amass in human omentum and are depleted in patients with cancer and obesity. Eur J Immunol. (2009) 39:1893–901. doi: 10.1002/eji.200939349
287. Ji Y, Sun S, Xu A, Bhargava P, Yang L, Lam KS, et al. Activation of natural killer T cells promotes M2 Macrophage polarization in adipose tissue and improves systemic glucose tolerance via interleukin-4 (IL-4)/STAT6 protein signaling axis in obesity. J Biol Chem. (2012) 287:13561–71. doi: 10.1074/jbc.M112.350066
288. Huh JY, Park J, Kim JI, Park YJ, Lee YK, Kim JB. Deletion of CD1d in adipocytes aggravates adipose tissue inflammation and insulin resistance in obesity. Diabetes. (2017) 66:835–47. doi: 10.2337/db16-1122
289. Li Y, Woods K, Parry-Strong A, Anderson RJ, Capistrano C, Gestin A, et al. Distinct dysfunctional states of circulating innate-like T cells in metabolic disease. Front Immunol. (2020) 11:448. doi: 10.3389/fimmu.2020.00448
290. Yu D, Rao S, Tsai LM, Lee SK, He Y, Sutcliffe EL, et al. The transcriptional repressor Bcl-6 directs T follicular helper cell lineage commitment. Immunity. (2009) 31:457–68. doi: 10.1016/j.immuni.2009.07.002
291. Huh JY, Kim JI, Park YJ, Hwang IJ, Lee YS, Sohn JH, et al. A novel function of adipocytes in lipid antigen presentation to iNKT cells. Mol Cell Biol. (2013) 33:328–39. doi: 10.1128/MCB.00552-12
292. Lee YS, Wollam J, Olefsky JM. An integrated view of immunometabolism. Cell. (2018) 172:22–40. doi: 10.1016/j.cell.2017.12.025
293. Webb TJ, Carey GB, East JE, Sun W, Bollino DR, Kimball AS, et al. Alterations in cellular metabolism modulate CD1d-mediated NKT-cell responses. Pathog Dis. (2016) 74:ftw055. doi: 10.1093/femspd/ftw055
294. Venken K, Seeuws S, Zabeau L, Jacques P, Decruy T, Coudenys J, et al. A bidirectional crosstalk between iNKT cells and adipocytes mediated by leptin modulates susceptibility for T cell mediated hepatitis. J Hepatol. (2014) 60:175–82. doi: 10.1016/j.jhep.2013.08.008
295. Favreau M, Menu E, Gaublomme D, Vanderkerken K, Faict S, Maes K, et al. Leptin receptor antagonism of iNKT cell function: a novel strategy to combat multiple myeloma. Leukemia. (2017) 31:2678–85. doi: 10.1038/leu.2017.146
296. Rakhshandehroo M, Van Eijkeren RJ, Gabriel TL, De Haar C, Gijzel SMW, Hamers N, et al. Adipocytes harbor a glucosylceramide biosynthesis pathway involved in iNKT cell activation. Biochim Biophys Acta. (2019) 1864:1157–67. doi: 10.1016/j.bbalip.2019.04.016
297. Bénézech C, Jackson-Jones LH. ILC2 orchestration of local immune function in adipose tissue. Front Immunol. (2019) 10:171. doi: 10.3389/fimmu.2019.00171
298. Rolot M, O'sullivan TE. Living with yourself: innate lymphoid cell immunometabolism. Cells. (2020) 9:334. doi: 10.3390/cells9020334
299. O'sullivan TE, Rapp M, Fan X, Weizman OE, Bhardwaj P, Adams NM, et al. Adipose-resident group 1 innate lymphoid cells promote obesity-associated insulin resistance. Immunity. (2016) 45:428–41. doi: 10.1016/j.immuni.2016.06.016
300. Wang H, Shen L, Sun X, Liu F, Feng W, Jiang C, et al. Adipose group 1 innate lymphoid cells promote adipose tissue fibrosis and diabetes in obesity. Nat Commun. (2019) 10:3254. doi: 10.1038/s41467-019-11270-1
301. Lee BC, Kim MS, Pae M, Yamamoto Y, Eberlé D, Shimada T, et al. Adipose natural killer cells regulate adipose tissue macrophages to promote insulin resistance in obesity. Cell Metab. (2016) 23:685–98. doi: 10.1016/j.cmet.2016.03.002
302. Boulenouar S, Michelet X, Duquette D, Alvarez D, Hogan AE, Dold C, et al. Adipose type one innate lymphoid cells regulate macrophage homeostasis through targeted cytotoxicity. Immunity. (2017) 46:273–86. doi: 10.1016/j.immuni.2017.01.008
303. Chung JJ, Markiewicz MA, Polić B, Shaw AS. Role of NKG2D in obesity-induced adipose tissue inflammation and insulin resistance. PLoS ONE. (2014) 9:e110108. doi: 10.1371/journal.pone.0110108
304. Vély F, Barlogis V, Vallentin B, Neven B, Piperoglou C, Ebbo M, et al. Evidence of innate lymphoid cell redundancy in humans. Nat Immunol. (2016) 17:1291–9. doi: 10.1038/ni.3553
305. Marçais A, Cherfils-Vicini J, Viant C, Degouve S, Viel S, Fenis A, et al. The metabolic checkpoint kinase mTOR is essential for IL-15 signaling during the development and activation of NK cells. Nat Immunol. (2014) 15:749–57. doi: 10.1038/ni.2936
306. Mah AY, Rashidi A, Keppel MP, Saucier N, Moore EK, Alinger JB, et al. Glycolytic requirement for NK cell cytotoxicity and cytomegalovirus control. JCI Insight. (2017) 2:e95128. doi: 10.1172/jci.insight.95128
307. Cong J, Wang X, Zheng X, Wang D, Fu B, Sun R, et al. Dysfunction of natural killer cells by FBP1-induced inhibition of glycolysis during lung cancer progression. Cell metabol. (2018) 28:243–255.e245. doi: 10.1016/j.cmet.2018.06.021
308. Keating SE, Zaiatz-Bittencourt V, Loftus RM, Keane C, Brennan K, Finlay DK, et al. Metabolic reprogramming supports IFN-γ production by CD56bright NK cells. J Immunol. (2016) 196:2552–60. doi: 10.4049/jimmunol.1501783
309. Nandagopal N, Ali AK, Komal AK, Lee S-H. The critical role of IL-15–PI3K–mTOR pathway in natural killer cell effector functions. Front Immunol. (2014) 5:187. doi: 10.3389/fimmu.2014.00187
310. Keppel MP, Saucier N, Mah AY, Vogel TP, Cooper MA. Activation-specific metabolic requirements for NK Cell IFN-γ production. J Immunol. (2015) 194:1954–62. doi: 10.4049/jimmunol.1402099
311. Bando JK, Nussbaum JC, Liang HE, Locksley RM. Type 2 innate lymphoid cells constitutively express arginase-I in the naive and inflamed lung. J Leukoc Biol. (2013) 94:877–84. doi: 10.1189/jlb.0213084
312. Monticelli LA, Buck MD, Flamar AL, Saenz SA, Tait Wojno ED, Yudanin NA, et al. Arginase 1 is an innate lymphoid-cell-intrinsic metabolic checkpoint controlling type 2 inflammation. Nat Immunol. (2016) 17:656–65. doi: 10.1038/ni.3421
313. Li Q, Li D, Zhang X, Wan Q, Zhang W, Zheng M, et al. E3 ligase VHL promotes group 2 innate lymphoid cell maturation and function via glycolysis inhibition and induction of interleukin-33 receptor. Immunity. (2018) 48:258–70.e255. doi: 10.1016/j.immuni.2017.12.013
314. Galle-Treger L, Hurrell BP, Lewis G, Howard E, Jahani PS, Banie H, et al. Autophagy is critical for group 2 innate lymphoid cell metabolic homeostasis and effector function. J Allergy Clin Immunol. (2020) 145:502–17.e505. doi: 10.1016/j.jaci.2019.10.035
315. Wilhelm C, Harrison OJ, Schmitt V, Pelletier M, Spencer SP, Urban JF, et al. Critical role of fatty acid metabolism in ILC2-mediated barrier protection during malnutrition and helminth infection. J Exp Med. (2016) 213:1409–18. doi: 10.1084/jem.20151448
316. Brestoff JR, Kim BS, Saenz SA, Stine RR, Monticelli LA, Sonnenberg GF, et al. Group 2 innate lymphoid cells promote beiging of white adipose tissue and limit obesity. Nature. (2015) 519:242–6. doi: 10.1038/nature14115
317. Molofsky AB, Van Gool F, Liang HE, Van Dyken SJ, Nussbaum JC, Lee J, et al. Interleukin-33 and interferon-γ counter-regulate group 2 innate lymphoid cell activation during immune perturbation. Immunity. (2015) 43:161–74. doi: 10.1016/j.immuni.2015.05.019
318. Halim TY, Rana BM, Walker JA, Kerscher B, Knolle MD, Jolin HE, et al. Tissue-restricted adaptive type 2 immunity is orchestrated by expression of the costimulatory molecule OX40L on group 2 innate lymphoid cells. Immunity. (2018) 48:1195–207.e6. doi: 10.1016/j.immuni.2018.05.003
319. Lim AI, Menegatti S, Bustamante J, Le Bourhis L, Allez M, Rogge L, et al. IL-12 drives functional plasticity of human group 2 innate lymphoid cells. J Exp Med. (2016) 213:569–83. doi: 10.1084/jem.20151750
320. Srikakulapu P, Mcnamara CA. B lymphocytes and adipose tissue inflammation. Arterioscler Thromb Vasc Biol. (2020) 40:1110–22. doi: 10.1161/ATVBAHA.119.312467
321. Harmon DB, Srikakulapu P, Kaplan JL, Oldham SN, Mcskimming C, Garmey JC, et al. Protective role for B-1b B cells and IgM in obesity-associated inflammation, glucose intolerance, and insulin resistance. Arterioscler Thromb Vasc Biol. (2016) 36:682–91. doi: 10.1161/ATVBAHA.116.307166
322. Srikakulapu P, Upadhye A, Rosenfeld SM, Marshall MA, Mcskimming C, Hickman AW, et al. Perivascular adipose tissue harbors atheroprotective IgM-producing B cells. Front Physiol. (2017) 8:719. doi: 10.3389/fphys.2017.00719
323. Duffaut C, Galitzky J, Lafontan M, Bouloumié A. Unexpected trafficking of immune cells within the adipose tissue during the onset of obesity. Biochem Biophys Res Commun. (2009) 384:482–5. doi: 10.1016/j.bbrc.2009.05.002
324. Winer DA, Winer S, Shen L, Wadia PP, Yantha J, Paltser G, et al. B cells promote insulin resistance through modulation of T cells and production of pathogenic IgG antibodies. Nat Med. (2011) 17:610–7. doi: 10.1038/nm.2353
325. Defuria J, Belkina AC, Jagannathan-Bogdan M, Snyder-Cappione J, Carr JD, Nersesova YR, et al. B cells promote inflammation in obesity and type 2 diabetes through regulation of T-cell function and an inflammatory cytokine profile. Proc Natl Acad Sci USA. (2013) 110:5133–8. doi: 10.1073/pnas.1215840110
326. Ying W, Wollam J, Ofrecio JM, Bandyopadhyay G, El Ouarrat D, Lee YS, et al. Adipose tissue B2 cells promote insulin resistance through leukotriene LTB4/LTB4R1 signaling. The J Clin Invest. (2017) 127:1019–30. doi: 10.1172/JCI90350
327. Wu Z, Xu J, Tan J, Song Y, Liu L, Zhang F, et al. Mesenteric adipose tissue B lymphocytes promote local and hepatic inflammation in non-alcoholic fatty liver disease mice. J Cell Mol Med. (2019) 23:3375–85. doi: 10.1111/jcmm.14232
328. Peterson KR, Flaherty DK, Hasty AH. Obesity alters B cell and macrophage populations in brown adipose tissue. Obesity. (2017) 25:1881–4. doi: 10.1002/oby.21982
329. Jagannathan M, Mcdonnell M, Liang Y, Hasturk H, Hetzel J, Rubin D, et al. Toll-like receptors regulate B cell cytokine production in patients with diabetes. Diabetologia. (2010) 53:1461–71. doi: 10.1007/s00125-010-1730-z
330. Zhai X, Qian G, Wang Y, Chen X, Lu J, Zhang Y, et al. Elevated B cell activation is associated with type 2 diabetes development in obese subjects. Cell Physiol Biochem. (2016) 38:1257–66. doi: 10.1159/000443073
331. Bergtold A, Desai DD, Gavhane A, Clynes R. Cell surface recycling of internalized antigen permits dendritic cell priming of B cells. Immunity. (2005) 23:503–14. doi: 10.1016/j.immuni.2005.09.013
332. Wong SC, Puaux AL, Chittezhath M, Shalova I, Kajiji TS, Wang X, et al. Macrophage polarization to a unique phenotype driven by B cells. Eur J Immunol. (2010) 40:2296–307. doi: 10.1002/eji.200940288
333. Wu L, Parekh VV, Hsiao J, Kitamura D, Van Kaer L. Spleen supports a pool of innate-like B cells in white adipose tissue that protects against obesity-associated insulin resistance. Proc Natl Acad Sci USA. (2014) 111:E4638–47. doi: 10.1073/pnas.1324052111
334. Rosenfeld SM, Perry HM, Gonen A, Prohaska TA, Srikakulapu P, Grewal S, et al. B-1b cells secrete atheroprotective IgM and attenuate atherosclerosis. Circ Res. (2015) 117:e28–39. doi: 10.1161/CIRCRESAHA.117.306044
335. Shen L, Chng MHY, Alonso MN, Yuan R, Winer DA, Engleman EG. B-1a lymphocytes attenuate insulin resistance. Diabetes. (2015) 64:593–603. doi: 10.2337/db14-0554
336. Nishimura S, Manabe I, Takaki S, Nagasaki M, Otsu M, Yamashita H, et al. Adipose natural regulatory B cells negatively control adipose tissue inflammation. Cell Metabol. (2013) 18:759–66. doi: 10.1016/j.cmet.2013.09.017
337. García-Hernández M, Rodríguez-Varela E, García-Jacobo R, Hernández-De La Torre M, Uresti-Rivera E, González-Amaro R, et al. Frequency of regulatory B cells in adipose tissue and peripheral blood from individuals with overweight, obesity and normal-weight. Obes Res Clin Pract. (2018) 12:513–9. doi: 10.1016/j.orcp.2018.07.001
338. Jellusova J. Cross-talk between signal transduction and metabolism in B cells. Immunol Lett. (2018) 201:1–13. doi: 10.1016/j.imlet.2018.11.003
339. Caro-Maldonado A, Wang R, Nichols AG, Kuraoka M, Milasta S, Sun LD, et al. Metabolic reprogramming is required for antibody production that is suppressed in anergic but exaggerated in chronically BAFF-exposed B cells. J Immunol. (2014) 192:3626–36. doi: 10.4049/jimmunol.1302062
340. Doughty CA, Bleiman BF, Wagner DJ, Dufort FJ, Mataraza JM, Roberts MF, et al. Antigen receptor-mediated changes in glucose metabolism in B lymphocytes: role of phosphatidylinositol 3-kinase signaling in the glycolytic control of growth. Blood. (2006) 107:4458–65. doi: 10.1182/blood-2005-12-4788
341. Akkaya M, Traba J, Roesler AS, Miozzo P, Akkaya B, Theall BP, et al. Second signals rescue B cells from activation-induced mitochondrial dysfunction and death. Nat Immunol. (2018) 19:871–84. doi: 10.1038/s41590-018-0156-5
342. Weisel FJ, Mullett SJ, Elsner RA, Menk AV, Trivedi N, Luo W, et al. Germinal center B cells selectively oxidize fatty acids for energy while conducting minimal glycolysis. Nat Immunol. (2020) 21:331–42. doi: 10.1038/s41590-020-0598-4
343. Waters LR, Ahsan FM, Wolf DM, Shirihai O, Teitell MA. Initial B cell activation induces metabolic reprogramming and mitochondrial remodeling. iScience. (2018) 5:99–109. doi: 10.1016/j.isci.2018.07.005
344. Dufort FJ, Gumina MR, Ta NL, Tao Y, Heyse SA, Scott DA, et al. Glucose-dependent de novo lipogenesis in B lymphocytes: a requirement for atp-citrate lyase in lipopolysaccharide-induced differentiation. J Biol Chem. (2014) 289:7011–24. doi: 10.1074/jbc.M114.551051
345. Kissebah AH, Vydelingum N, Murray R, Evans DJ, Hartz AJ, Kalkhoff RK, et al. Relation of body fat distribution to metabolic complications of obesity. J Clin Endocrinol Metab. (1982) 54:254–60. doi: 10.1210/jcem-54-2-254
346. Foster MT, Pagliassotti MJ. Metabolic alterations following visceral fat removal and expansion: beyond anatomic location. Adipocyte. (2012) 1:192–9. doi: 10.4161/adip.21756
347. Petrovic N, Walden TB, Shabalina IG, Timmons JA, Cannon B, Nedergaard J. Chronic peroxisome proliferator-activated receptor gamma (PPARgamma) activation of epididymally derived white adipocyte cultures reveals a population of thermogenically competent, UCP1-containing adipocytes molecularly distinct from classic brown adipocytes. J Biol Chem. (2010) 285:7153–64. doi: 10.1074/jbc.M109.053942
348. Chen KY, Brychta RJ, Sater ZA, Cassimatis TM, Cero C, Fletcher LA, et al. Opportunities and challenges in the therapeutic activation of human energy expenditure and thermogenesis to manage obesity. J Biol Chem. (2020) 295:1926–42. doi: 10.1074/jbc.REV119.007363
349. Pan R, Zhu X, Maretich P, Chen Y. Combating obesity with thermogenic fat: current challenges and advancements. Front Endocrinol. (2020) 11:185. doi: 10.3389/fendo.2020.00185
350. Van Mil SR, Vijgen G, Van Huisstede A, Klop B, Van De Geijn GM, Birnie E, et al. Discrepancies between BMI and classic cardiovascular risk factors. Obes Surg. (2018) 28:3484–91. doi: 10.1007/s11695-018-3359-9
351. Jialal I, Devaraj S. Subcutaneous adipose tissue biology in metabolic syndrome. Horm Mol Biol Clin Investig. (2018) 33:1–6. doi: 10.1515/hmbci-2017-0074
352. Pahwa R, Singh A, Huet B, Devaraj S, Jialal I. Increased inflammasome activity in subcutaneous adipose tissue of patients with metabolic syndrome. Diabetes Metab Res Rev. (2020). doi: 10.1002/dmrr.3383. [Epub ahead of print].
353. Ara I, Auerbach P, Larsen S, Mata E, Stallknecht B, Ploug T, et al. Low-grade inflammation is not present in former obese males but adipose tissue macrophage infiltration persists. Biomedicines. (2020) 8:123. doi: 10.3390/biomedicines8050123
354. Sbierski-Kind J, Mai K, Kath J, Jurisch A, Streitz M, Kuchenbecker L, et al. Association between subcutaneous adipose tissue inflammation, insulin resistance, and calorie restriction in obese females. J Immunol. (2020) 205:45–55. doi: 10.4049/jimmunol.2000108
355. Verboven K, Wouters K, Gaens K, Hansen D, Bijnen M, Wetzels S, et al. Abdominal subcutaneous and visceral adipocyte size, lipolysis and inflammation relate to insulin resistance in male obese humans. Sci Rep. (2018) 8:4677. doi: 10.1038/s41598-018-22962-x
356. Picarelli A, Borghini R, Marino M, Casale R, Di Tola M, Lubrano C, et al. Visceral and subcutaneous adipose tissue as markers of local and systemic inflammation: a comparison between celiac and obese patients using MRI. Tech Coloproctol. (2020) 24:553–62. doi: 10.1007/s10151-020-02173-1
357. Moussa K, Gurung P, Adams-Huet B, Devaraj S, Jialal I. Increased eosinophils in adipose tissue of metabolic syndrome. J Diabet Comp. (2019) 33:535–8. doi: 10.1016/j.jdiacomp.2019.05.010
358. Chylikova J, Dvorackova J, Cizkova K, Lacey H, Kamarad V. Macrophages of the subcutaneous and omental fatty tissue in obese patients: immunohistochemical phenotyping of M2 subtypes in relation to type 2 diabetes. Biomed Pap Med Fac Univ Palacky Olomouc Czech Repub. (2020) 164:133–7. doi: 10.5507/bp.2019.011
359. Giron-Ulloa A, Gonzalez-Dominguez E, Klimek RS, Patino-Martinez E, Vargas-Ayala G, Segovia-Gamboa NC, et al. Specific macrophage subsets accumulate in human subcutaneous and omental fat depots during obesity. Immunol Cell Biol. (2020). doi: 10.1111/imcb.12380. [Epub ahead of print].
360. Mráz M, Cinkajzlová A, Kloučková J, Lacinová Z, Kratochvílová H, Lipš M, et al. Dendritic cells in subcutaneous and epicardial adipose tissue of subjects with type 2 diabetes, obesity, and coronary artery disease. Mediators Inflamm. (2019) 2019:5481725. doi: 10.1155/2019/5481725
361. Altintas MM, Rossetti MA, Nayer B, Puig A, Zagallo P, Ortega LM, et al. Apoptosis, mastocytosis, and diminished adipocytokine gene expression accompany reduced epididymal fat mass in long-standing diet-induced obese mice. Lipids Health Dis. (2011) 10:198. doi: 10.1186/1476-511X-10-198
362. Martinez H, Quinones M, Jimenez F, Estrada C, Clark K, Muscogiuri G, et al. Critical role of chemokine (CC motif) receptor 2 (CCR2) in the KKAy+ Apoe−/− mouse model of the metabolic syndrome. Diabetologia. (2011) 54:2660–8. doi: 10.1007/s00125-011-2248-8
363. Wensveen FM, Jelenčić V, Valentić S, Šestan M, Wensveen TT, Theurich S, et al. NK cells link obesity-induced adipose stress to inflammation and insulin resistance. Nat Immunol. (2015) 16:376–85. doi: 10.1038/ni.3120
364. Hildebrand S, Stümer J, Pfeifer A. PVAT and its relation to brown, beige, and white adipose tissue in development and function. Front Physiol. (2018) 9:70. doi: 10.3389/fphys.2018.00070
365. Samuel OO. Review on multifaceted involvement of perivascular adipose tissue in vascular pathology. Cardiovasc Pathol. (2020) 49:107259. doi: 10.1016/j.carpath.2020.107259
366. Szasz T, Bomfim GF, Webb RC. The influence of perivascular adipose tissue on vascular homeostasis. Vasc Health And Risk Manag. (2013) 9:105. doi: 10.2147/VHRM.S33760
367. Omar A, Chatterjee TK, Tang Y, Hui DY, Weintraub NL. Proinflammatory phenotype of perivascular adipocytes. Arterioscler Thromb Vasc Biol. (2014) 34:1631–6. doi: 10.1161/ATVBAHA.114.303030
368. Numaguchi R, Furuhashi M, Matsumoto M, Sato H, Yanase Y, Kuroda Y, et al. Differential phenotypes in perivascular adipose tissue surrounding the internal thoracic artery and diseased coronary artery. J Am Heart Assoc. (2019) 8:e011147. doi: 10.1161/JAHA.118.011147
369. Saxton SN, Clark BJ, Withers SB, Eringa EC, Heagerty AM. Mechanistic links between obesity, diabetes, and blood pressure: role of perivascular adipose tissue. Physiol Rev. (2019) 99:1701–63. doi: 10.1152/physrev.00034.2018
370. Guzik TJ, Hoch NE, Brown KA, Mccann LA, Rahman A, Dikalov S, et al. Role of the T cell in the genesis of angiotensin II–induced hypertension and vascular dysfunction. J Exp Med. (2007) 204:2449–60. doi: 10.1084/jem.20070657
371. Xing T, Bautista T, Fong A, Pilowsky P. Acute intermittent hypoxia induced long-term facilitation of sympathetic nerve activity is serotonin dependent. In: Hypertension: Abstracts From the 33rd Annual Scientific Meeting of the HBPRCA. Philadelphia, PA: Lippincott Williams & Wilkins (2012). p. 502.
372. Moore JP, Vinh A, Tuck KL, Sakkal S, Krishnan SM, Chan CT, et al. M2 macrophage accumulation in the aortic wall during angiotensin II infusion in mice is associated with fibrosis, elastin loss, and elevated blood pressure. Am J Physiol Heart Circ Physiol. (2015) 309:H906–17. doi: 10.1152/ajpheart.00821.2014
373. Mikolajczyk TP, Nosalski R, Szczepaniak P, Budzyn K, Osmenda G, Skiba D, et al. Role of chemokine RANTES in the regulation of perivascular inflammation, T-cell accumulation, and vascular dysfunction in hypertension. FASEB J. (2016) 30:1987–99. doi: 10.1096/fj.201500088R
374. Kyaw T, Tay C, Krishnamurthi S, Kanellakis P, Agrotis A, Tipping P, et al. B1a B lymphocytes are atheroprotective by secreting natural IgM that increases IgM deposits and reduces necrotic cores in atherosclerotic lesions. Circ Res. (2011) 109:830–40. doi: 10.1161/CIRCRESAHA.111.248542
375. Choi YS, Dieter JA, Rothaeusler K, Luo Z, Baumgarth N. B-1 cells in the bone marrow are a significant source of natural IgM. Eur J Immunol. (2012) 42:120–9. doi: 10.1002/eji.201141890
376. Lefranc C, Friederich-Persson M, Braud L, Palacios-Ramirez R, Karlsson S, Boujardine N, et al. MR (mineralocorticoid receptor) induces adipose tissue senescence and mitochondrial dysfunction leading to vascular dysfunction in obesity. Hypertension. (2019) 73:458–68. doi: 10.1161/HYPERTENSIONAHA.118.11873
377. Manka D, Chatterjee TK, Stoll LL, Basford JE, Konaniah ES, Srinivasan R, et al. Transplanted perivascular adipose tissue accelerates injury-induced neointimal hyperplasia: role of monocyte chemoattractant protein-1. Arterioscler. Thromb Vasc Biol. (2014) 34:1723–30. doi: 10.1161/ATVBAHA.114.303983
378. Konaniah ES, Kuhel DG, Basford JE, Weintraub NL, Hui DY. Deficiency of LRP1 in mature adipocytes promotes diet-induced inflammation and atherosclerosis—brief report. Arterioscler Thromb Vasc Biol. (2017) 37:1046–9. doi: 10.1161/ATVBAHA.117.309414
379. Hou N, Liu Y, Han F, Wang D, Hou X, Hou S, et al. Irisin improves perivascular adipose tissue dysfunction via regulation of the heme oxygenase-1/adiponectin axis in diet-induced obese mice. J Mol Cell Cardiol. (2016) 99:188–96. doi: 10.1016/j.yjmcc.2016.09.005
380. Trayhurn P, Beattie JH. Physiological role of adipose tissue: white adipose tissue as an endocrine and secretory organ. Proc Nutr Soc. (2001) 60:329–39. doi: 10.1079/PNS200194
381. Viengchareun S, Zennaro MC, Pascual-Le Tallec L, Lombes M. Brown adipocytes are novel sites of expression and regulation of adiponectin and resistin. FEBS Lett. (2002) 532:345–50. doi: 10.1016/S0014-5793(02)03697-9
382. Li CJ, Sun HW, Zhu FL, Chen L, Rong YY, Zhang Y, et al. Local adiponectin treatment reduces atherosclerotic plaque size in rabbits. J Endocrinol. (2007) 193:137–45. doi: 10.1677/JOE-06-0173
383. Wang X, Chen Q, Pu H, Wei Q, Duan M, Zhang C, et al. Adiponectin improves NF-κB-mediated inflammation and abates atherosclerosis progression in apolipoprotein E-deficient mice. Lipids Health Dis. (2016) 15:33. doi: 10.1186/s12944-016-0202-y
384. Sena CM, Pereira A, Fernandes R, Letra L, Seiça RM. Adiponectin improves endothelial function in mesenteric arteries of rats fed a high-fat diet: role of perivascular adipose tissue. Br J Pharmacol. (2017) 174:3514–26. doi: 10.1111/bph.13756
385. Nishimura M, Morioka T, Hayashi M, Kakutani Y, Yamazaki Y, Kurajoh M, et al. Plasma omentin levels are inversely associated with atherosclerosis in type 2 diabetes patients with increased plasma adiponectin levels: a cross-sectional study. Cardiovasc Diabetol. (2019) 18:167. doi: 10.1186/s12933-019-0973-3
386. Watanabe T, Watanabe-Kominato K, Takahashi Y, Kojima M, Watanabe R. Adipose tissue-derived omentin-1 function and regulation. Compr Physiol. (2017) 7:765–81. doi: 10.1002/cphy.c160043
387. Liu F, Fang S, Liu X, Li J, Wang X, Cui J, et al. Omentin-1 protects against high glucose-induced endothelial dysfunction via the AMPK/PPARδ signaling pathway. Biochem Pharmacol. (2020) 174:113830. doi: 10.1016/j.bcp.2020.113830
388. Nakatsuka A, Wada J, Iseda I, Teshigawara S, Higashio K, Murakami K, et al. Vaspin is an adipokine ameliorating ER stress in obesity as a ligand for cell-surface GRP78/MTJ-1 complex. Diabetes. (2012) 61:2823–32. doi: 10.2337/db12-0232
389. Boucher J, Masri B, Daviaud D, Gesta S, Guigné C, Mazzucotelli A, et al. Apelin, a newly identified adipokine up-regulated by insulin and obesity. Endocrinology. (2005) 146:1764–71. doi: 10.1210/en.2004-1427
390. Chandra SM, Razavi H, Kim J, Agrawal R, Kundu RK, De Jesus Perez V, et al. Disruption of the apelin-APJ system worsens hypoxia-induced pulmonary hypertension. Arterioscler Thromb Vasc Biol. (2011) 31:814–20. doi: 10.1161/ATVBAHA.110.219980
391. Reilly MP, Iqbal N, Schutta M, Wolfe ML, Scally M, Localio AR, et al. Plasma leptin levels are associated with coronary atherosclerosis in type 2 diabetes. J Clin Endocrinol Metab. (2004) 89:3872–8. doi: 10.1210/jc.2003-031676
392. Gálvez-Prieto B, Somoza B, Gil-Ortega M, García-Prieto CF, De Las Heras AI, González MC, et al. Anticontractile effect of perivascular adipose tissue and leptin are reduced in hypertension. Front Pharmacol. (2012) 3:103. doi: 10.3389/fphar.2012.00103
393. Jamroz-Wiśniewska A, Gertler A, Solomon G, Wood ME, Whiteman M, Bełtowski J. Leptin-induced endothelium-dependent vasorelaxation of peripheral arteries in lean and obese rats: role of nitric oxide and hydrogen sulfide. PLoS ONE. (2014) 9:e86744. doi: 10.1371/journal.pone.0086744
394. Buyse M, Viengchareun S, Bado A, Lombès M. Insulin and glucocorticoids differentially regulate leptin transcription and secretion in brown adipocytes. FASEB J. (2001) 15:1357–66. doi: 10.1096/fj.00-0669com
395. Li H, Wang YP, Zhang LN, Tian G. Perivascular adipose tissue-derived leptin promotes vascular smooth muscle cell phenotypic switching via p38 mitogen-activated protein kinase in metabolic syndrome rats. Exp Biol Med. (2014) 239:954–65. doi: 10.1177/1535370214527903
396. Liu R, Chen B, Chen J, Lan J. Leptin upregulates smooth muscle cell expression of MMP-9 to promote plaque destabilization by activating AP-1 via the leptin receptor/MAPK/ERK signaling pathways. Exp Ther Med. (2018) 16:5327–33. doi: 10.3892/etm.2018.6853
397. Hansen IR, Jansson KM, Cannon B, Nedergaard J. Contrasting effects of cold acclimation versus obesogenic diets on chemerin gene expression in brown and brite adipose tissues. Biochim Biophys Acta. (2014) 1841:1691–9. doi: 10.1016/j.bbalip.2014.09.003
398. Liu H, Xiong W, Luo Y, Chen H, He Y, Cao Y, et al. Adipokine chemerin stimulates progression of atherosclerosis in Apoe(-/-) mice. Biomed Res Int. (2019) 2019:7157865. doi: 10.1155/2019/7157865
399. Curat CA, Wegner V, Sengenès C, Miranville A, Tonus C, Busse R, et al. Macrophages in human visceral adipose tissue: increased accumulation in obesity and a source of resistin and visfatin. Diabetologia. (2006) 49:744–7. doi: 10.1007/s00125-006-0173-z
400. Romacho T, Valencia I, Ramos-González M, Vallejo S, López-Esteban M, Lorenzo O, et al. Visfatin/eNampt induces endothelial dysfunction in vivo: a role for toll-like receptor 4 and NLRP3 inflammasome. Sci Rep. (2020) 10:5386. doi: 10.1038/s41598-020-62190-w
401. Patel SD, Rajala MW, Rossetti L, Scherer PE, Shapiro L. Disulfide-dependent multimeric assembly of resistin family hormones. Science. (2004) 304:1154–8. doi: 10.1126/science.1093466
402. Jiang Y, Lu L, Hu Y, Li Q, An C, Yu X, et al. Resistin induces hypertension and insulin resistance in mice via a TLR4-dependent pathway. Sci Rep. (2016) 6:22193. doi: 10.1038/srep22193
403. Fitzgibbons TP, Czech MP. Epicardial and perivascular adipose tissues and their influence on cardiovascular disease: basic mechanisms and clinical associations. J Am Heart Assoc. (2014) 3:e000582. doi: 10.1161/JAHA.113.000582
404. Talman AH, Psaltis PJ, Cameron JD, Meredith IT, Seneviratne SK, Wong DT. Epicardial adipose tissue: far more than a fat depot. Cardiovasc Diagn Ther. (2014) 4:416–29. doi: 10.3978/j.issn.2223-3652.2014.11.05
405. Cherian S, Lopaschuk GD, Carvalho E. Cellular cross-talk between epicardial adipose tissue and myocardium in relation to the pathogenesis of cardiovascular disease. Am J Physiol Endocrinol Metabol. (2012) 303:E937–49. doi: 10.1152/ajpendo.00061.2012
406. Matloch Z, Kotulák T, Haluzík M. The role of epicardial adipose tissue in heart disease. Physiol Res. (2016) 65:23–32. doi: 10.33549/physiolres.933036
407. Cheng KH, Chu CS, Lee KT, Lin TH, Hsieh CC, Chiu CC, et al. Adipocytokines and proinflammatory mediators from abdominal and epicardial adipose tissue in patients with coronary artery disease. Int J Obes. (2008) 32:268–74. doi: 10.1038/sj.ijo.0803726
408. Greif M, Becker A, Von Ziegler F, Lebherz C, Lehrke M, Broedl UC, et al. Pericardial adipose tissue determined by dual source CT is a risk factor for coronary atherosclerosis. Arterioscler Thromb Vasc Biol. (2009) 29:781–6. doi: 10.1161/ATVBAHA.108.180653
409. Karastergiou K, Evans I, Ogston N, Miheisi N, Nair D, Kaski JC, et al. Epicardial adipokines in obesity and coronary artery disease induce atherogenic changes in monocytes and endothelial cells. Arterioscler Thromb Vasc Biol. (2010) 30:1340–6. doi: 10.1161/ATVBAHA.110.204719
410. Gruzdeva OV, Akbasheva OE, Dyleva YA, Antonova LV, Matveeva VG, Uchasova EG, et al. Adipokine and cytokine profiles of epicardial and subcutaneous adipose tissue in patients with coronary heart disease. Bull Exp Biol Med. (2017) 163:608–11. doi: 10.1007/s10517-017-3860-5
411. Spiroglou SG, Kostopoulos CG, Varakis JN, Papadaki HH. Adipokines in periaortic and epicardial adipose tissue: differential expression and relation to atherosclerosis. J Atheroscler Thromb. (2010) 17:115–30. doi: 10.5551/jat.1735
412. Du Y, Ji Q, Cai L, Huang F, Lai Y, Liu Y, et al. Association between omentin-1 expression in human epicardial adipose tissue and coronary atherosclerosis. Cardiovasc Diabetol. (2016) 15:90. doi: 10.1186/s12933-016-0406-5
413. Fernández-Trasancos Á, Agra RM, García-Acuña JM, Fernández ÁL, González-Juanatey JR, Eiras S. Omentin treatment of epicardial fat improves its anti-inflammatory activity and paracrine benefit on smooth muscle cells. Obesity. (2017) 25:1042–9. doi: 10.1002/oby.21832
414. Langheim S, Dreas L, Veschini L, Maisano F, Foglieni C, Ferrarello S, et al. Increased expression and secretion of resistin in epicardial adipose tissue of patients with acute coronary syndrome. Am J Physiol Heart Circ Physiol. (2010) 298:H746–53. doi: 10.1152/ajpheart.00617.2009
415. Rachwalik M, Zyśko D, Diakowska D, Kustrzycki W. Increased content of resistin in epicardial adipose tissue of patients with advanced coronary atherosclerosis and history of myocardial infarction. Thorac Cardiovasc Surg. (2014) 62:554–60. doi: 10.1055/s-0034-1376403
416. Gao X, Mi S, Zhang F, Gong F, Lai Y, Gao F, et al. Association of chemerin mRNA expression in human epicardial adipose tissue with coronary atherosclerosis. Cardiovasc Diabetol. (2011) 10:87. doi: 10.1186/1475-2840-10-87
417. Wu Q, Chen Y, Chen S, Wu X, Nong W. Correlation between adiponectin, chemerin, vascular endothelial growth factor and epicardial fat volume in patients with coronary artery disease. Exp Ther Med. (2020) 19:1095–102. doi: 10.3892/etm.2019.8299
418. Mazurek T, Zhang L, Zalewski A, Mannion JD, Diehl JT, Arafat H, et al. Human epicardial adipose tissue is a source of inflammatory mediators. Circulation. (2003) 108:2460–6. doi: 10.1161/01.CIR.0000099542.57313.C5
419. Iacobellis G. Coronary artery disease and epicardial adipose tissue. In: Iacobellis G, editor. Epicardial Adipose Tissue. Contemporary Cardiology. Cham: Humana (2020). p. 77–90. doi: 10.1007/978-3-030-40570-0_8
420. Szablewski L. (editor). Introductory Chapter: Adipose Tissue - An Update. IntechOpen. (2019). doi: 10.5772/intechopen.88420
421. Yu R, Kim CS, Kwon BS, Kawada TJO. Mesenteric adipose tissue-derived monocyte chemoattractant protein-1 plays a crucial role in adipose tissue macrophage migration and activation in obese mice. Obesity. (2006) 14:1353–62. doi: 10.1038/oby.2006.153
422. Kim DH, Sandoval D, Reed JA, Matter EK, Tolod EG, Woods SC, et al. The role of GM-CSF in adipose tissue inflammation. Am J Physiol Endocrinol Metab. (2008) 295:E1038–46. doi: 10.1152/ajpendo.00061.2008
423. Grima P, Guido M, Zizza A, Chiavaroli R. Sonographically measured perirenal fat thickness: an early predictor of atherosclerosis in HIV-1-infected patients receiving highly active antiretroviral therapy? J Clin Ultrasound. (2010) 38:190–5. doi: 10.1002/jcu.20664
424. De Pergola G, Campobasso N, Nardecchia A, Triggiani V, Caccavo D, Gesualdo L, et al. Para- and perirenal ultrasonographic fat thickness is associated with 24-hours mean diastolic blood pressure levels in overweight and obese subjects. BMC Cardiovasc Disord. (2015) 15:108. doi: 10.1186/s12872-015-0101-6
425. Liu BX, Sun W, Kong XQ. Perirenal fat: a unique fat pad and potential target for cardiovascular disease. Angiology. (2019) 70:584–93. doi: 10.1177/0003319718799967
426. Geraci G, Zammuto MM, Mattina A, Zanoli L, Geraci C, Granata A, et al. Para-perirenal distribution of body fat is associated with reduced glomerular filtration rate regardless of other indices of adiposity in hypertensive patients. J Clin Hypertens. (2018) 20:1438–46. doi: 10.1111/jch.13366
427. Chau YY, Bandiera R, Serrels A, Martínez-Estrada OM, Qing W, Lee M, et al. Visceral and subcutaneous fat have different origins and evidence supports a mesothelial source. Nat Cell Biol. (2014) 16:367–75. doi: 10.1038/ncb2922
428. Ma S, Zhu XY, Eirin A, Woollard JR, Jordan KL, Tang H, et al. Perirenal fat promotes renal arterial endothelial dysfunction in obese swine through tumor necrosis factor-α. J Urol. (2016) 195:1152–9. doi: 10.1016/j.juro.2015.08.105
429. Li H, Li M, Liu P, Wang Y, Zhang H, Li H, et al. Telmisartan ameliorates nephropathy in metabolic syndrome by reducing leptin release from perirenal adipose tissue. Hypertension. (2016) 68:478–90. doi: 10.1161/HYPERTENSIONAHA.116.07008
430. Tanida M, Iwashita S, Ootsuka Y, Terui N, Suzuki M. Leptin injection into white adipose tissue elevates renal sympathetic nerve activity dose-dependently through the afferent nerves pathway in rats. Neurosci Lett. (2000) 293:107–10. doi: 10.1016/S0304-3940(00)01490-7
431. Ndisang JF, Jadhav A, Mishra M. The heme oxygenase system suppresses perirenal visceral adiposity, abates renal inflammation and ameliorates diabetic nephropathy in Zucker diabetic fatty rats. PLoS ONE. (2014) 9:e87936. doi: 10.1371/journal.pone.0087936
432. Roesler A, Kazak L. UCP1-independent thermogenesis. Biochem J. (2020) 477:709–25. doi: 10.1042/BCJ20190463
433. Newsholme EA, Arch JR, Brooks B, Surholt B. The role of substrate cycles in metabolic regulation. Biochem Soc Trans. (1983). 11:52–6. doi: 10.1042/bst0110052
434. Fujii J, Kadoma M, Tada M, Toda H, Sakiyama F. Characterization of structural unit of phospholamban by amino acid sequencing and electrophoretic analysis. Biochem Biophys Res Commun. (1986) 138:1044–50. doi: 10.1016/S0006-291X(86)80387-4
435. Ukropec J, Anunciado RP, Ravussin Y, Hulver MW, Kozak LP. UCP1-independent thermogenesis in white adipose tissue of cold-acclimated Ucp1−/− mice. J Biol Chem. (2006) 281:31894–908. doi: 10.1074/jbc.M606114200
436. Himms-Hagen J, Desautels M. A mitochondrial defect in brown adipose tissue of the obese (obob) mouse: reduced binding of purine nucleotides and a failure to respond to cold by an increase in binding. Biochem Biophys Res Commun. (1978) 83:628–34. doi: 10.1016/0006-291X(78)91036-7
437. Rothwell NJ, Stock MJ. A role for brown adipose tissue in diet-induced thermogenesis. Nature. (1979) 281:31–5. doi: 10.1038/281031a0
438. Rebiger L, Lenzen S, Mehmeti I. Susceptibility of brown adipocytes to pro-inflammatory cytokine toxicity and reactive oxygen species. Biosci Rep. (2016) 36:e00306. doi: 10.1042/BSR20150193
439. Van Den Berg SM, Van Dam AD, Rensen PC, De Winther MP, Lutgens E. Immune modulation of brown (ing) adipose tissue in obesity. Endocr Rev. (2017) 38:46–68. doi: 10.1210/er.2016-1066
440. Kooijman S, Van Den Heuvel JK, Rensen PC. Neuronal control of brown fat activity. Trends Endocrinol Metabol. (2015) 26:657–68. doi: 10.1016/j.tem.2015.09.008
441. Wolf Y, Boura-Halfon S, Cortese N, Haimon Z, Sar Shalom H, Kuperman Y, et al. Brown-adipose-tissue macrophages control tissue innervation and homeostatic energy expenditure. Nat Immunol. (2017) 18:665–74. doi: 10.1038/ni.3746
442. Alcalá M, Calderon-Dominguez M, Bustos E, Ramos P, Casals N, Serra D, et al. Increased inflammation, oxidative stress and mitochondrial respiration in brown adipose tissue from obese mice. Sci Rep. (2017) 7:16082. doi: 10.1038/s41598-017-16463-6
443. Kotzbeck P, Giordano A, Mondini E, Murano I, Severi I, Venema W, et al. Brown adipose tissue whitening leads to brown adipocyte death and adipose tissue inflammation. J Lipid Res. (2018) 59:784–94. doi: 10.1194/jlr.M079665
444. Contreras GA, Lee YH, Mottillo EP, Granneman JG. Inducible brown adipocytes in subcutaneous inguinal white fat: the role of continuous sympathetic stimulation. Am J Physiol Endocrinol Metab. (2014) 307:E793–9. doi: 10.1152/ajpendo.00033.2014
445. Kern PA, Finlin BS, Zhu B, Rasouli N, Mcgehee RE Jr, et al. The effects of temperature and seasons on subcutaneous white adipose tissue in humans: evidence for thermogenic gene induction. J Clin Endocrinol Metab. (2014) 99:E2772–9. doi: 10.1210/jc.2014-2440
446. Sepa-Kishi DM, Jani S, Da Eira D, Ceddia RB. Cold acclimation enhances UCP1 content, lipolysis, and triacylglycerol resynthesis, but not mitochondrial uncoupling and fat oxidation, in rat white adipocytes. Am J Physiol Cell Physiol. (2019) 316:C365–76. doi: 10.1152/ajpcell.00122.2018
447. Hageman RS, Wagener A, Hantschel C, Svenson KL, Churchill GA, Brockmann GA. High-fat diet leads to tissue-specific changes reflecting risk factors for diseases in DBA/2J mice. Physiol Genom. (2010) 42:55–66. doi: 10.1152/physiolgenomics.00072.2009
448. Marinho TS, Ornellas F, Aguila MB, Mandarim-De-Lacerda CA. Browning of the subcutaneous adipocytes in diet-induced obese mouse submitted to intermittent fasting. Mol Cell Endocrinol. (2020) 513:110872. doi: 10.1016/j.mce.2020.110872
449. Pollard AE, Martins L, Muckett PJ, Khadayate S, Bornot A, Clausen M, et al. AMPK activation protects against diet-induced obesity through Ucp1-independent thermogenesis in subcutaneous white adipose tissue. Nat Metabol. (2019) 1:340–9. doi: 10.1038/s42255-019-0036-9
450. Bartesaghi S, Hallen S, Huang L, Svensson PA, Momo RA, Wallin S, et al. Thermogenic activity of UCP1 in human white fat-derived beige adipocytes. Mol Endocrinol. (2015) 29:130–9. doi: 10.1210/me.2014-1295
451. Xu L, Ma X, Verma NK, Wang D, Gavrilova O, Proia RL, et al. Ablation of PPARγ in subcutaneous fat exacerbates age-associated obesity and metabolic decline. Aging Cell. (2018) 17:e12721. doi: 10.1111/acel.12721
452. Chang L, Villacorta L, Li R, Hamblin M, Xu W, Dou C, et al. Loss of perivascular adipose tissue on peroxisome proliferator–activated receptor-γ deletion in smooth muscle cells impairs intravascular thermoregulation and enhances atherosclerosis. Circulation. (2012) 126:1067–78. doi: 10.1161/CIRCULATIONAHA.112.104489
453. Burkey BF, Dong M, Gagen K, Eckhardt M, Dragonas N, Chen W, et al. Effects of pioglitazone on promoting energy storage, not expenditure, in brown adipose tissue of obese fa/fa Zucker rats: comparison to CL 316,243. Metabolism. (2000) 49:1301–8. doi: 10.1053/meta.2000.9524
454. Loh RKC, Formosa MF, Eikelis N, Bertovic DA, Anderson MJ, Barwood SA, et al. Pioglitazone reduces cold-induced brown fat glucose uptake despite induction of browning in cultured human adipocytes: a randomised, controlled trial in humans. Diabetologia. (2018) 61:220–30. doi: 10.1007/s00125-017-4479-9
455. Iacobellis G, Barbaro G. Epicardial adipose tissue feeding and overfeeding the heart. Nutrition. (2019) 59:1–6. doi: 10.1016/j.nut.2018.07.002
456. Sacks HS, Fain JN, Bahouth SW, Ojha S, Frontini A, Budge H, et al. Adult epicardial fat exhibits beige features. J Clin Endocrinol Metab. (2013) 98:E1448–55. doi: 10.1210/jc.2013-1265
457. Chechi K, Voisine P, Mathieu P, Laplante M, Bonnet S, Picard F, et al. Functional characterization of the Ucp1-associated oxidative phenotype of human epicardial adipose tissue. Sci Rep. (2017) 7:15566. doi: 10.1038/s41598-017-15501-7
458. Chechi K, Vijay J, Voisine P, Mathieu P, Bossé Y, Tchernof A, et al. UCP1 expression-associated gene signatures of human epicardial adipose tissue. JCI Insight. (2019) 4:e123618. doi: 10.1172/jci.insight.123618
459. He Y, Ma N, Tang M, Jiang Z, Liu H, Mei J. The differentiation of beige adipocyte in pericardial and epicardial adipose tissues induces atrial fibrillation development. Eur Rev Med Pharmacol Sci. (2017) 21:4398–405.
460. Pérez-Belmonte LM, Moreno-Santos I, Gómez-Doblas JJ, García-Pinilla JM, Morcillo-Hidalgo L, Garrido-Sánchez L, et al. Expression of epicardial adipose tissue thermogenic genes in patients with reduced and preserved ejection fraction heart failure. Int J Med Sci. (2017) 14:891–5. doi: 10.7150/ijms.19854
461. Wang J, Chen D, Cheng XM, Zhang QG, Peng YP, Wang LJ, et al. Influence of phenotype conversion of epicardial adipocytes on the coronary atherosclerosis and its potential molecular mechanism. Am J Transl Res. (2015) 7:1712–23.
462. Jespersen NZ, Feizi A, Andersen ES, Heywood S, Hattel HB, Daugaard S, et al. Heterogeneity in the perirenal region of humans suggests presence of dormant brown adipose tissue that contains brown fat precursor cells. Mol Metab. (2019) 24:30–43. doi: 10.1016/j.molmet.2019.03.005
463. Efremova A, Senzacqua M, Venema W, Isakov E, Di Vincenzo A, Zingaretti MC, et al. A large proportion of mediastinal and perirenal visceral fat of Siberian adult people is formed by UCP1 immunoreactive multilocular and paucilocular adipocytes. J Physiol Biochem. (2020) 76:185–92. doi: 10.1007/s13105-019-00721-4
464. Van Den Beukel JC, Grefhorst A, Hoogduijn MJ, Steenbergen J, Mastroberardino PG, Dor FJ, et al. Women have more potential to induce browning of perirenal adipose tissue than men. Obesity. (2015) 23:1671–9. doi: 10.1002/oby.21166
465. Sidlo J, Kvasnicka P, Sidlova H. Occurrence of periadrenal brown adipose tissue in adult Slovak population. Neuro Endocrinol Lett. (2019) 40:49–55.
466. Li X, Liu J, Wang G, Yu J, Sheng Y, Wang C, et al. Determination of UCP1 expression in subcutaneous and perirenal adipose tissues of patients with hypertension. Endocrine. (2015) 50:413–23. doi: 10.1007/s12020-015-0572-3
467. Oliver P, Picó C, Palou A. Differential expression of genes for uncoupling proteins 1:2 and 3 in brown and white adipose tissue depots during rat development. Cell Mol Life Sci. (2001) 58:470–6. doi: 10.1007/PL00000870
468. Yamada T, Katagiri H, Ishigaki Y, Ogihara T, Imai J, Uno K, et al. Signals from intra-abdominal fat modulate insulin and leptin sensitivity through different mechanisms: neuronal involvement in food-intake regulation. Cell Metab. (2006) 3:223–9. doi: 10.1016/j.cmet.2006.02.001
469. Bertholet AM, Kazak L, Chouchani ET, Bogaczyńska MG, Paranjpe I, Wainwright GL, et al. Mitochondrial patch clamp of beige adipocytes reveals UCP1-positive and UCP1-negative cells both exhibiting futile creatine cycling. Cell Metab. (2017) 25:811–22.e814. doi: 10.1016/j.cmet.2017.03.002
470. Jia R, Luo XQ, Wang G, Lin CX, Qiao H, Wang N, et al. Characterization of cold-induced remodelling reveals depot-specific differences across and within brown and white adipose tissues in mice. Acta Physiol. (2016) 217:311–24. doi: 10.1111/apha.12688
471. Yang X, Sui W, Zhang M, Dong M, Lim S, Seki T, et al. Switching harmful visceral fat to beneficial energy combustion improves metabolic dysfunctions. JCI Insight. (2017) 2:e89044. doi: 10.1172/jci.insight.89044
472. Van Der Stelt I, Hoevenaars F, Široká J, De Ronde L, Friedecký D, Keijer J, et al. Metabolic response of visceral white adipose tissue of obese mice exposed for 5 days to human room temperature compared to mouse thermoneutrality. Front Physiol. (2017) 8:179. doi: 10.3389/fphys.2017.00179
473. Zhang Q, Hao H, Xie Z, Cheng Y, Yin Y, Xie M, et al. M2 macrophages infusion ameliorates obesity and insulin resistance by remodeling inflammatory/macrophages' homeostasis in obese mice. Mol Cell Endocrinol. (2017) 443:63–71. doi: 10.1016/j.mce.2017.01.005
474. Himms-Hagen J, Cui J, Danforth E Jr, Taatjes DJ, Lang SS, et al. Effect of CL-316,243, a thermogenic beta 3-agonist, on energy balance and brown and white adipose tissues in rats. Am J Physiol. (1994) 266:R1371–82. doi: 10.1152/ajpregu.1994.266.4.R1371
475. Nagase I, Yoshida T, Kumamoto K, Umekawa T, Sakane N, Nikami H, et al. Expression of uncoupling protein in skeletal muscle and white fat of obese mice treated with thermogenic beta 3-adrenergic agonist. J Clin Invest. (1996) 97:2898–904. doi: 10.1172/JCI118748
476. Vargovic P, Manz G, Kvetnansky R. Continuous cold exposure induces an anti-inflammatory response in mesenteric adipose tissue associated with catecholamine production and thermogenin expression in rats. Endocr Regul. (2016) 50:137–44. doi: 10.1515/enr-2016-0015
477. Vargovic P, Laukova M, Ukropec J, Manz G, Kvetnansky R. Prior repeated stress attenuates cold-induced immunomodulation associated with “browning” in mesenteric fat of rats. Cell Mol Neurobiol. (2018) 38:349–61. doi: 10.1007/s10571-017-0531-z
478. Hondares E, Iglesias R, Giralt A, Gonzalez FJ, Giralt M, Mampel T, et al. Thermogenic activation induces FGF21 expression and release in brown adipose tissue. J Biol Chem. (2011) 286:12983–90. doi: 10.1074/jbc.M110.215889
479. Kleiner S, Douris N, Fox EC, Mepani RJ, Verdeguer F, Wu J, et al. FGF21 regulates PGC-1α and browning of white adipose tissues in adaptive thermogenesis. Genes Dev. (2012) 26:271–81. doi: 10.1101/gad.177857.111
480. Lee MW, Odegaard JI, Mukundan L, Qiu Y, Molofsky AB, Nussbaum JC, et al. Activated type 2 innate lymphoid cells regulate beige fat biogenesis. Cell. (2015) 160:74–87. doi: 10.1016/j.cell.2014.12.011
481. Huang Z, Zhong L, Lee JTH, Zhang J, Wu D, Geng L, et al. The FGF21-CCL11 axis mediates beiging of white adipose tissues by coupling sympathetic nervous system to type 2 immunity. Cell Metab. (2017) 26:493–508.e494. doi: 10.1016/j.cmet.2017.08.003
482. Nguyen KD, Qiu Y, Cui X, Goh YS, Mwangi J, David T, et al. Alternatively activated macrophages produce catecholamines to sustain adaptive thermogenesis. Nature. (2011) 480:104–8. doi: 10.1038/nature10653
483. Fischer K, Ruiz HH, Jhun K, Finan B, Oberlin DJ, Van Der Heide V, et al. Alternatively activated macrophages do not synthesize catecholamines or contribute to adipose tissue adaptive thermogenesis. Nat Med. (2017) 23:623. doi: 10.1038/nm.4316
484. Pirzgalska RM, Seixas E, Seidman JS, Link VM, Sanchez NM, Mahu I, et al. Sympathetic neuron–associated macrophages contribute to obesity by importing and metabolizing norepinephrine. Nat Med. (2017) 23:1309. doi: 10.1038/nm.4422
485. Fang W, Deng Z, Benadjaoud F, Yang D, Yang C, Shi GP. Regulatory T cells promote adipocyte beiging in subcutaneous adipose tissue. FASEB J. (2020) 34:9755–70. doi: 10.1096/fj.201902518R
486. Hu B, Jin C, Zeng X, Resch JM, Jedrychowski MP, Yang Z, et al. γδ T cells and adipocyte IL-17RC control fat innervation and thermogenesis. Nature. (2020) 578:610–4. doi: 10.1038/s41586-020-2028-z
487. Sakamoto T, Nitta T, Maruno K, Yeh YS, Kuwata H, Tomita K, et al. Macrophage infiltration into obese adipose tissues suppresses the induction of UCP1 level in mice. Am J Physiol Endocrinol Metab. (2016) 310:E676–87. doi: 10.1152/ajpendo.00028.2015
488. Hui X, Gu P, Zhang J, Nie T, Pan Y, Wu D, et al. Adiponectin enhances cold-induced browning of subcutaneous adipose tissue via promoting M2 macrophage proliferation. Cell Metab. (2015) 22:279–90. doi: 10.1016/j.cmet.2015.06.004
489. Lv Y, Zhang SY, Liang X, Zhang H, Xu Z, Liu B, et al. Adrenomedullin 2 enhances beiging in white adipose tissue directly in an adipocyte-autonomous manner and indirectly through activation of M2 macrophages. J Biol Chem. (2016) 291:23390–402. doi: 10.1074/jbc.M116.735563
490. Okla M, Zaher W, Alfayez M, Chung S. Inhibitory effects of toll-like receptor 4, NLRP3 inflammasome, and interleukin-1β on white adipocyte browning. Inflammation. (2018) 41:626–42. doi: 10.1007/s10753-017-0718-y
491. Gallot YS, Mcmillan JD, Xiong G, Bohnert KR, Straughn AR, Hill BG, et al. Distinct roles of TRAF6 and TAK1 in the regulation of adipocyte survival, thermogenesis program, and high-fat diet-induced obesity. Oncotarget. (2017) 8:112565–83. doi: 10.18632/oncotarget.22575
492. Anto Michel N, Colberg C, Buscher K, Sommer B, Pramod AB, Ehinger E, et al. Inflammatory pathways regulated by tumor necrosis receptor-associated factor 1 protect from metabolic consequences in diet-induced obesity. Circ Res. (2018) 122:693–700. doi: 10.1161/CIRCRESAHA.117.312055
493. Miyajima Y, Ealey KN, Motomura Y, Mochizuki M, Takeno N, Yanagita M, et al. Effects of BMP7 produced by group 2 innate lymphoid cells on adipogenesis. Int Immunol. (2020) 32:407–19. doi: 10.1093/intimm/dxaa013
494. Monticelli LA, Sonnenberg GF, Abt MC, Alenghat T, Ziegler CG, Doering TA, et al. Innate lymphoid cells promote lung-tissue homeostasis after infection with influenza virus. Nat Immunol. (2011) 12:1045–54. doi: 10.1038/ni.2131
495. Li W, Li Y, Jin J. The essential function of IL-33 in metabolic regulation. Acta Biochim Biophys Sin (Shanghai). (2020) 52:768–75. doi: 10.1093/abbs/gmaa045
496. Rajbhandari P, Arneson D, Hart SK, Ahn IS, Diamante G, Santos LC. Single cell analysis reveals immune cell-adipocyte crosstalk regulating the transcription of thermogenic adipocytes. Elife. (2019) 8:e21. doi: 10.7554/eLife.49501.021
497. Ji Y, Sun S, Xia S, Yang L, Li X, Qi L. Short term high fat diet challenge promotes alternative macrophage polarization in adipose tissue via natural killer T cells and interleukin-4. J Biol Chem. (2012) 287:24378–86. doi: 10.1074/jbc.M112.371807
498. Hams E, Locksley RM, Mckenzie AN, Fallon PG. Cutting edge: IL-25 elicits innate lymphoid type 2 and type II NKT cells that regulate obesity in mice. J Immunol. (2013) 191:5349–53. doi: 10.4049/jimmunol.1301176
499. Lynch L, Hogan AE, Duquette D, Lester C, Banks A, Leclair K, et al. iNKT cells induce FGF21 for thermogenesis and are required for maximal weight loss in GLP1 therapy. Cell Metab. (2016) 24:510–9. doi: 10.1016/j.cmet.2016.08.003
500. Desautels M, Wollin A, Halvorson I, Muralidhara D, Thornhill J. Role of mast cell histamine in brown adipose tissue thermogenic response to VMH stimulation. Am J Physiol Regul Integr Compar Physiol. (1994) 266:R831–7. doi: 10.1152/ajpregu.1994.266.3.R831
501. Finlin BS, Confides AL, Zhu B, Boulanger MC, Memetimin H, Taylor KW, et al. Adipose tissue mast cells promote human adipose beiging in response to cold. Sci Rep. (2019) 9:8658. doi: 10.1038/s41598-019-45136-9
502. Finlin BS, Zhu B, Confides AL, Westgate PM, Harfmann BD, Dupont-Versteegden EE, et al. Mast cells promote seasonal white adipose beiging in humans. Diabetes. (2017) 66:1237–46. doi: 10.2337/db16-1057
503. Zhang X, Wang X, Yin H, Zhang L, Feng A, Zhang QX, et al. Functional inactivation of mast cells enhances subcutaneous adipose tissue browning in mice. Cell Rep. (2019) 28:792–803.e794. doi: 10.1016/j.celrep.2019.06.044
504. Medrikova D, Sijmonsma TP, Sowodniok K, Richards DM, Delacher M, Sticht C, et al. Brown adipose tissue harbors a distinct sub-population of regulatory T cells. PLoS ONE. (2015) 10:e0118534. doi: 10.1371/journal.pone.0118534
505. Powell JD, Pollizzi KN, Heikamp EB, Horton MR. Regulation of immune responses by mTOR. Annu Rev Immunol. (2012) 30:39–68. doi: 10.1146/annurev-immunol-020711-075024
506. Kälin S, Becker M, Ott VB, Serr I, Hosp F, Mollah MM, et al. A Stat6/Pten axis links regulatory T cells with adipose tissue function. Cell Metab. (2017) 26:475–92.e477. doi: 10.1016/j.cmet.2017.08.008
507. Liu X, Huh J, Gong H, Chamberland J, Brinkoetter M, Hamnvik OR. Lack of mature lymphocytes results in obese but metabolically healthy mice when fed a high-fat diet. Int J Obes. (2015) 39:1548–57. doi: 10.1038/ijo.2015.93
508. Moysidou M, Karaliota S, Kodela E, Salagianni M, Koutmani Y, Katsouda A, et al. CD8+ T cells in beige adipogenesis and energy homeostasis. JCI insight. (2018) 3:e95456. doi: 10.1172/jci.insight.95456
509. Withers SB, Forman R, Meza-Perez S, Sorobetea D, Sitnik K, Hopwood T, et al. Eosinophils are key regulators of perivascular adipose tissue and vascular functionality. Sci Rep. (2017) 7:44571. doi: 10.1038/srep44571
510. Rao RR, Long JZ, White JP, Svensson KJ, Lou J, Lokurkar I, et al. Meteorin-like is a hormone that regulates immune-adipose interactions to increase beige fat thermogenesis. Cell. (2014) 157:1279–91. doi: 10.1016/j.cell.2014.03.065
511. Knights AJ, Vohralik EJ, Houweling PJ, Stout ES, Norton LJ, Alexopoulos SJ, et al. Eosinophil function in adipose tissue is regulated by Krüppel-like factor 3 (KLF3). Nat Commun. (2020) 11:2922. doi: 10.1038/s41467-020-16758-9
512. Polak J, Klimcakova E, Moro C, Viguerie N, Berlan M, Hejnova J, et al. Effect of aerobic training on plasma levels and subcutaneous abdominal adipose tissue gene expression of adiponectin, leptin, interleukin 6, and tumor necrosis factor alpha in obese women. Metabolism. (2006) 55:1375–81. doi: 10.1016/j.metabol.2006.06.008
513. Lee S, Norheim F, Langleite TM, Gulseth HL, Birkeland KI, Drevon CA. Effects of long-term exercise on plasma adipokine levels and inflammation-related gene expression in subcutaneous adipose tissue in sedentary dysglycaemic, overweight men and sedentary normoglycaemic men of healthy weight. Diabetologia. (2019) 62:1048–64. doi: 10.1007/s00125-019-4866-5
514. Mathur N, Pedersen BK. Exercise as a mean to control low-grade systemic inflammation. Mediators Inflamm. (2008) 2008:109502. doi: 10.1155/2008/109502
515. Kawanishi N, Mizokami T, Yano H, Suzuki K. Exercise attenuates M1 macrophages and CD8+ T cells in the adipose tissue of obese mice. Med Sci Sports Exerc. (2013) 45:1684–93. doi: 10.1249/MSS.0b013e31828ff9c6
516. Kawanishi N, Yano H, Yokogawa Y, Suzuki K. Exercise training inhibits inflammation in adipose tissue via both suppression of macrophage infiltration and acceleration of phenotypic switching from M1 to M2 macrophages in high-fat-diet-induced obese mice. Exerc Immunol Rev. (2010) 16:105–18.
517. Ziegler AK, Damgaard A, Mackey AL, Schjerling P, Magnusson P, Olesen AT, et al. An anti-inflammatory phenotype in visceral adipose tissue of old lean mice, augmented by exercise. Sci Rep. (2019) 9:12069. doi: 10.1038/s41598-019-48587-2
518. Auerbach P, Nordby P, Bendtsen LQ, Mehlsen JL, Basnet SK, Vestergaard H, et al. Differential effects of endurance training and weight loss on plasma adiponectin multimers and adipose tissue macrophages in younger, moderately overweight men. Am J Physiol Regul Integr Comp Physiol. (2013) 305:R490–8. doi: 10.1152/ajpregu.00575.2012
519. Otero-Díaz B, Rodríguez-Flores M, Sánchez-Muñoz V, Monraz-Preciado F, Ordoñez-Ortega S, Becerril-Elias V, et al. Exercise induces white adipose tissue browning across the weight spectrum in humans. Front Physiol. (2018) 9:1781. doi: 10.3389/fphys.2018.01781
520. Stinkens R, Brouwers B, Jocken JW, Blaak EE, Teunissen-Beekman KF, Hesselink MK, et al. Exercise training-induced effects on the abdominal subcutaneous adipose tissue phenotype in humans with obesity. J Appl Physiol (1985). (2018) 125:1585–93. doi: 10.1152/japplphysiol.00496.2018
521. Zubrzycki A, Cierpka-Kmiec K, Kmiec Z, Wronska A. The role of low-calorie diets and intermittent fasting in the treatment of obesity and type-2 diabetes. J Physiol Pharmacol. (2018) 69:663–83. doi: 10.26402/jpp.2018.5.02
522. Regmi P, Heilbronn LK. Time-restricted eating: benefits, mechanisms, and challenges in translation. iScience. (2020) 23:101161. doi: 10.1016/j.isci.2020.101161
523. Klempel MC, Kroeger CM, Bhutani S, Trepanowski JF, Varady KA. Intermittent fasting combined with calorie restriction is effective for weight loss and cardio-protection in obese women. Nutr J. (2012) 11:98. doi: 10.1186/1475-2891-11-98
524. Longo VD, Mattson MP. Fasting: molecular mechanisms and clinical applications. Cell Metab. (2014) 19:181–92. doi: 10.1016/j.cmet.2013.12.008
525. Sutton EF, Beyl R, Early KS, Cefalu WT, Ravussin E, Peterson CM. Early time-restricted feeding improves insulin sensitivity, blood pressure, and oxidative stress even without weight loss in men with prediabetes. Cell Metab. (2018) 27:1212–21.e1213. doi: 10.1016/j.cmet.2018.04.010
526. Stekovic S, Hofer SJ, Tripolt N, Aon MA, Royer P, Pein L, et al. Alternate day fasting improves physiological and molecular markers of aging in healthy, non-obese humans. Cell Metab. (2019) 30:462–76.e466. doi: 10.1016/j.cmet.2019.07.016
527. Jordan S, Tung N, Casanova-Acebes M, Chang C, Cantoni C, Zhang D, et al. Dietary intake regulates the circulating inflammatory monocyte pool. Cell. (2019) 178:1102–14.e1117. doi: 10.1016/j.cell.2019.07.050
528. Tang HN, Tang CY, Man XF, Tan SW, Guo Y, Tang J, et al. Plasticity of adipose tissue in response to fasting and refeeding in male mice. Nutr Metab (Lond). (2017) 14:3. doi: 10.1186/s12986-016-0159-x
529. Li G, Xie C, Lu S, Nichols RG, Tian Y, Li L, et al. Intermittent fasting promotes white adipose browning and decreases obesity by shaping the gut microbiota. Cell Metab. (2017) 26:672–85.e674. doi: 10.1016/j.cmet.2017.08.019
530. Kim KH, Kim YH, Son JE, Lee JH, Kim S, Choe MS, et al. Intermittent fasting promotes adipose thermogenesis and metabolic homeostasis via VEGF-mediated alternative activation of macrophage. Cell Res. (2017) 27:1309–26. doi: 10.1038/cr.2017.126
531. Speaker KJ, Paton MM, Cox SS, Fleshner M. A single bout of fasting (24 h) reduces basal cytokine expression and minimally impacts the sterile inflammatory response in the white adipose tissue of normal weight F344 rats. Mediators Inflamm. (2016) 2016:1698071. doi: 10.1155/2016/1698071
532. Chaix A, Zarrinpar A, Miu P, Panda S. Time-restricted feeding is a preventative and therapeutic intervention against diverse nutritional challenges. Cell Metab. (2014) 20:991–1005. doi: 10.1016/j.cmet.2014.11.001
533. Lord GM, Matarese G, Howard JK, Baker RJ, Bloom SR, Lechler RI. Leptin modulates the T-cell immune response and reverses starvation-induced immunosuppression. Nature. (1998) 394:897–901. doi: 10.1038/29795
534. O'doherty RM, Nguyen L. Blunted fasting-induced decreases in plasma and CSF leptin concentrations in obese rats: the role of increased leptin secretion. Int J Obes Relat Metab Disord. (2004) 28:173–5. doi: 10.1038/sj.ijo.0802477
535. Moro T, Tinsley G, Bianco A, Marcolin G, Pacelli QF, Battaglia G, et al. Effects of eight weeks of time-restricted feeding (16/8) on basal metabolism, maximal strength, body composition, inflammation, and cardiovascular risk factors in resistance-trained males. J Transl Med. (2016) 14:290. doi: 10.1186/s12967-016-1044-0
536. Trepanowski JF, Kroeger CM, Barnosky A, Klempel M, Bhutani S, Hoddy KK, et al. Effects of alternate-day fasting or daily calorie restriction on body composition, fat distribution, and circulating adipokines: Secondary analysis of a randomized controlled trial. Clin Nutr. (2018) 37:1871–8. doi: 10.1016/j.clnu.2017.11.018
537. Clifton PM, Keogh JB. Effects of different weight loss approaches on CVD risk. Curr Atheroscler Rep. (2018) 20:27. doi: 10.1007/s11883-018-0728-8
538. Lacroix M, Gaudichon C, Martin A, Morens C, Mathé V, Tomé D, et al. A long-term high-protein diet markedly reduces adipose tissue without major side effects in Wistar male rats. Am J Physiol Regul Integr Compar Physiol. (2004) 287:R934–42. doi: 10.1152/ajpregu.00100.2004
539. Khattab M, Abi-Rashed C, Ghattas H, Hlais S, Obeid O. Phosphorus ingestion improves oral glucose tolerance of healthy male subjects: a crossover experiment. Nutr J. (2015) 14:112. doi: 10.1186/s12937-015-0101-5
540. Imi Y, Yabiki N, Abuduli M, Masuda M, Yamanaka-Okumura H, Taketani Y. High phosphate diet suppresses lipogenesis in white adipose tissue. J Clin Biochem Nutr. (2018) 63:181–91. doi: 10.3164/jcbn.17-141
541. Hallberg SJ, Mckenzie AL, Williams PT, Bhanpuri NH, Peters AL, Campbell WW, et al. Effectiveness and safety of a novel care model for the management of type 2 diabetes at 1 year: an open-label, non-randomized, controlled study. Diabetes Ther. (2018) 9:583–612. doi: 10.1007/s13300-018-0373-9
542. Athinarayanan SJ, Adams RN, Hallberg SJ, Mckenzie AL, Bhanpuri NH, Campbell WW, et al. Long-term effects of a novel continuous remote care intervention including nutritional ketosis for the management of type 2 diabetes: a 2-year non-randomized clinical trial. Front Endocrinol (Lausanne). (2019) 10:348. doi: 10.3389/fendo.2019.00348
543. Bhanpuri NH, Hallberg SJ, Williams PT, Mckenzie AL, Ballard KD, Campbell WW, et al. Cardiovascular disease risk factor responses to a type 2 diabetes care model including nutritional ketosis induced by sustained carbohydrate restriction at 1 year: an open label, non-randomized, controlled study. Cardiovasc Diabetol. (2018) 17:56. doi: 10.1186/s12933-018-0698-8
544. Di Daniele N, Noce A, Vidiri MF, Moriconi E, Marrone G, Annicchiarico-Petruzzelli M, et al. Impact of Mediterranean diet on metabolic syndrome, cancer and longevity. Oncotarget. (2017) 8:8947–79. doi: 10.18632/oncotarget.13553
545. Anderson AL, Harris TB, Tylavsky FA, Perry SE, Houston DK, Hue TF, et al. Dietary patterns and survival of older adults. J Am Diet Assoc. (2011) 111:84–91. doi: 10.1016/j.jada.2010.10.012
546. Grosso G, Marventano S, Yang J, Micek A, Pajak A, Scalfi L, et al. A comprehensive meta-analysis on evidence of Mediterranean diet and cardiovascular disease: are individual components equal? Crit Rev Food Sci Nutr. (2017) 57:3218–32. doi: 10.1080/10408398.2015.1107021
547. Martínez-González MA, Sánchez-Villegas A. The emerging role of Mediterranean diets in cardiovascular epidemiology: monounsaturated fats, olive oil, red wine or the whole pattern? Eur J Epidemiol. (2004) 19:9–13. doi: 10.1023/B:EJEP.0000013351.60227.7b
548. Feng D, Ling WH, Duan RD. Lycopene suppresses LPS-induced NO and IL-6 production by inhibiting the activation of ERK, p38MAPK, and NF-kappaB in macrophages. Inflamm Res. (2010) 59:115–21. doi: 10.1007/s00011-009-0077-8
549. Ghavipour M, Saedisomeolia A, Djalali M, Sotoudeh G, Eshraghyan MR, Moghadam AM, et al. Tomato juice consumption reduces systemic inflammation in overweight and obese females. Br J Nutr. (2013) 109:2031–5. doi: 10.1017/S0007114512004278
550. Schwingshackl L, Christoph M, Hoffmann G. Effects of olive oil on markers of inflammation and endothelial function–a systematic review and meta-analysis. Nutrients. (2015) 7:7651–75. doi: 10.3390/nu7095356
551. Li YF, Chang YY, Huang HC, Wu YC, Yang MD, Chao PM. Tomato juice supplementation in young women reduces inflammatory adipokine levels independently of body fat reduction. Nutrition. (2015) 31:691–6. doi: 10.1016/j.nut.2014.11.008
552. Fung CS, Wan EY, Wong CK, Jiao F, Chan AK. Effect of metformin monotherapy on cardiovascular diseases and mortality: a retrospective cohort study on Chinese type 2 diabetes mellitus patients. Cardiovasc Diabetol. (2015) 14:137. doi: 10.1186/s12933-015-0304-2
553. Feng WH, Bi Y, Li P, Yin TT, Gao CX, Shen SM, et al. Effects of liraglutide, metformin and gliclazide on body composition in patients with both type 2 diabetes and non-alcoholic fatty liver disease: a randomized trial. J Diabetes Invest. (2019) 10:399–407. doi: 10.1111/jdi.12888
554. Tokubuchi I, Tajiri Y, Iwata S, Hara K, Wada N, Hashinaga T, et al. Beneficial effects of metformin on energy metabolism and visceral fat volume through a possible mechanism of fatty acid oxidation in human subjects and rats. PLoS ONE. (2017) 12:e0171293. doi: 10.1371/journal.pone.0171293
555. Caballero AE, Delgado A, Aguilar-Salinas CA, Herrera AN, Castillo JL, Cabrera T, et al. The differential effects of metformin on markers of endothelial activation and inflammation in subjects with impaired glucose tolerance: a placebo-controlled, randomized clinical trial. J Clin Endocrinol Metab. (2004) 89:3943–8. doi: 10.1210/jc.2004-0019
556. Al-Assi O, Ghali R, Mroueh A, Kaplan A, Mougharbil N, Eid AH, et al. Cardiac autonomic neuropathy as a result of mild hypercaloric challenge in absence of signs of diabetes: modulation by antidiabetic drugs. Oxid Med Cell Longev. (2018) 2018:9389784. doi: 10.1155/2018/9389784
557. Chiang CF, Chao TT, Su YF, Hsu CC, Chien CY, Chiu KC, et al. Metformin-treated cancer cells modulate macrophage polarization through AMPK-NF-κB signaling. Oncotarget. (2017) 8:20706–18. doi: 10.18632/oncotarget.14982
558. Bruun JM, Lihn AS, Pedersen SB, Richelsen B. Monocyte chemoattractant protein-1 release is higher in visceral than subcutaneous human adipose tissue (AT): implication of macrophages resident in the AT. J Clin Endocrinol Metab. (2005) 90:2282–9. doi: 10.1210/jc.2004-1696
559. Li SN, Wang X, Zeng QT, Feng YB, Cheng X, Mao XB, et al. Metformin inhibits nuclear factor kappaB activation and decreases serum high-sensitivity C-reactive protein level in experimental atherogenesis of rabbits. Heart Vessels. (2009) 24:446–53. doi: 10.1007/s00380-008-1137-7
560. Saisho Y. Metformin and inflammation: its potential beyond glucose-lowering effect. Endocr Metab Immune Disord Drug Targets. (2015) 15:196–205. doi: 10.2174/1871530315666150316124019
561. Adeshirlarijaney A, Zou J, Tran HQ, Chassaing B, Gewirtz AT. Amelioration of metabolic syndrome by metformin associates with reduced indices of low-grade inflammation independently of the gut microbiota. Am J Physiol Endocrinol Metab. (2019) 317:E1121–30. doi: 10.1152/ajpendo.00245.2019
562. Dormandy JA, Charbonnel B, Eckland DJ, Erdmann E, Massi-Benedetti M, Moules IK, et al. Secondary prevention of macrovascular events in patients with type 2 diabetes in the PROactive Study (PROspective pioglitAzone Clinical Trial In macroVascular Events): a randomised controlled trial. Lancet. (2005) 366:1279–89. doi: 10.1016/S0140-6736(05)67528-9
563. Miyazaki Y, Mahankali A, Matsuda M, Mahankali S, Hardies J, Cusi K, et al. Effect of pioglitazone on abdominal fat distribution and insulin sensitivity in type 2 diabetic patients. J Clin Endocrinol Metab. (2002) 87:2784–91. doi: 10.1210/jcem.87.6.8567
564. Pellegrinelli V, Carobbio S, Vidal-Puig A. Adipose tissue plasticity: how fat depots respond differently to pathophysiological cues. Diabetologia. (2016) 59:1075–88. doi: 10.1007/s00125-016-3933-4
565. Chawla A, Nguyen KD, Goh YP. Macrophage-mediated inflammation in metabolic disease. Nat Rev Immunol. (2011) 11:738–49. doi: 10.1038/nri3071
566. Esterson YB, Zhang K, Koppaka S, Kehlenbrink S, Kishore P, Raghavan P, et al. Insulin sensitizing and anti-inflammatory effects of thiazolidinediones are heightened in obese patients. J Investig Med. (2013) 61:1152–60. doi: 10.2310/JIM.0000000000000017
567. Elzahhar PA, Alaaeddine R, Ibrahim TM, Nassra R, Ismail A, Chua BSK, et al. Shooting three inflammatory targets with a single bullet: Novel multi-targeting anti-inflammatory glitazones. Eur J Med Chem. (2019) 167:562–82. doi: 10.1016/j.ejmech.2019.02.034
568. Alaaeddine RA, Elzahhar P, Alzaim I, Abou-Kheir W, Belal ASF, El-Yazbi AF. The emerging role of COX-2:15-LOX, and PPARγ in metabolic diseases and cancer: an introduction to novel multi-target directed ligands (MTDLs). Curr Med Chem. (2020) 27:1–39. doi: 10.2174/0929867327999200820173853
569. Kim Chung Le T, Hosaka T, Yoshida M, Harada N, Sakaue H, Sakai T, et al. Exendin-4, a GLP-1 receptor agonist, directly induces adiponectin expression through protein kinase A pathway and prevents inflammatory adipokine expression. Biochem Biophys Res Commun. (2009) 390:613–8. doi: 10.1016/j.bbrc.2009.10.015
570. Garber A, Henry RR, Ratner R, Hale P, Chang CT, Bode B. Liraglutide, a once-daily human glucagon-like peptide 1 analogue, provides sustained improvements in glycaemic control and weight for 2 years as monotherapy compared with glimepiride in patients with type 2 diabetes. Diabetes Obes Metab. (2011) 13:348–56. doi: 10.1111/j.1463-1326.2010.01356.x
571. Nauck M, Frid A, Hermansen K, Thomsen AB, During M, Shah N, et al. Long-term efficacy and safety comparison of liraglutide, glimepiride and placebo, all in combination with metformin in type 2 diabetes: 2-year results from the LEAD-2 study. Diabetes Obes Metab. (2013) 15:204–12. doi: 10.1111/dom.12012
572. Marso SP, Daniels GH, Brown-Frandsen K, Kristensen P, Mann JF, Nauck MA, et al. Liraglutide and cardiovascular outcomes in type 2 diabetes. N Engl J Med. (2016) 375:311–22. doi: 10.1056/NEJMoa1603827
573. Arakawa M, Mita T, Azuma K, Ebato C, Goto H, Nomiyama T, et al. Inhibition of monocyte adhesion to endothelial cells and attenuation of atherosclerotic lesion by a glucagon-like peptide-1 receptor agonist, exendin-4. Diabetes. (2010) 59:1030–7. doi: 10.2337/db09-1694
574. Ansar S, Koska J, Reaven PD. Postprandial hyperlipidemia, endothelial dysfunction and cardiovascular risk: focus on incretins. Cardiovasc Diabetol. (2011) 10:61. doi: 10.1186/1475-2840-10-61
575. Neal B, Perkovic V, Mahaffey KW, De Zeeuw D, Fulcher G, Erondu N, et al. Canagliflozin and cardiovascular and renal events in type 2 diabetes. N Engl J Med. (2017) 377:644–57. doi: 10.1056/NEJMoa1611925
576. Bray GA, Heisel WE, Afshin A, Jensen MD, Dietz WH, Long M, et al. The science of obesity management: an endocrine society scientific statement. Endocr Rev. (2018) 39:79–132. doi: 10.1210/er.2017-00253
577. Häring HU, Merker L, Seewaldt-Becker E, Weimer M, Meinicke T, Woerle HJ, et al. Empagliflozin as add-on to metformin plus sulfonylurea in patients with type 2 diabetes: a 24-week, randomized, double-blind, placebo-controlled trial. Diabetes Care. (2013) 36:3396–404. doi: 10.2337/dc12-2673
578. Kovacs CS, Seshiah V, Swallow R, Jones R, Rattunde H, Woerle HJ, et al. Empagliflozin improves glycaemic and weight control as add-on therapy to pioglitazone or pioglitazone plus metformin in patients with type 2 diabetes: a 24-week, randomized, placebo-controlled trial. Diabetes Obes Metab. (2014) 16:147–58. doi: 10.1111/dom.12188
579. Xu L, Nagata N, Nagashimada M, Zhuge F, Ni Y, Chen G, et al. SGLT2 inhibition by empagliflozin promotes fat utilization and browning and attenuates inflammation and insulin resistance by polarizing M2 macrophages in diet-induced obese mice. EBioMedicine. (2017) 20:137–49. doi: 10.1016/j.ebiom.2017.05.028
580. Pucci A, Batterham RL. Mechanisms underlying the weight loss effects of RYGB and SG: similar, yet different. J Endocrinol Invest. (2019) 42:117–28. doi: 10.1007/s40618-018-0892-2
581. Shah M, Simha V, Garg A. Review: long-term impact of bariatric surgery on body weight, comorbidities, and nutritional status. J Clin Endocrinol Metab. (2006) 91:4223–31. doi: 10.1210/jc.2006-0557
582. Debédat J, Amouyal C, Aron-Wisnewsky J, Clément K. Impact of bariatric surgery on type 2 diabetes: contribution of inflammation and gut microbiome? Semin Immunopathol. (2019) 41:461–75. doi: 10.1007/s00281-019-00738-3
583. Chait A, Den Hartigh LJ. Adipose tissue distribution, inflammation and its metabolic consequences, including diabetes and cardiovascular disease. Front Cardiovasc Med. (2020) 7:22. doi: 10.3389/fcvm.2020.00022
584. Viardot A, Lord RV, Samaras K. The effects of weight loss and gastric banding on the innate and adaptive immune system in type 2 diabetes and prediabetes. J Clin Endocrinol Metab. (2010) 95:2845–50. doi: 10.1210/jc.2009-2371
585. Trachta P, Dostálová I, Haluzíková D, Kasalický M, Kaválková P, Drápalová J, et al. Laparoscopic sleeve gastrectomy ameliorates mRNA expression of inflammation-related genes in subcutaneous adipose tissue but not in peripheral monocytes of obese patients. Mol Cell Endocrinol. (2014) 383:96–102. doi: 10.1016/j.mce.2013.11.013
586. Kratz M, Hagman DK, Kuzma JN, Foster-Schubert KE, Chan CP, Stewart S, et al. Improvements in glycemic control after gastric bypass occur despite persistent adipose tissue inflammation. Obesity (Silver Spring). (2016) 24:1438–45. doi: 10.1002/oby.21524
587. Hagman DK, Larson I, Kuzma JN, Cromer G, Makar K, Rubinow KB, et al. The short-term and long-term effects of bariatric/metabolic surgery on subcutaneous adipose tissue inflammation in humans. Metabolism. (2017) 70:12–22. doi: 10.1016/j.metabol.2017.01.030
588. Pollack RM, Donath MY, Leroith D, Leibowitz G. Anti-inflammatory agents in the treatment of diabetes and its vascular complications. Diabetes Care. (2016) 39:S244–52. doi: 10.2337/dcS15-3015
589. Osborn O, Brownell SE, Sanchez-Alavez M, Salomon D, Gram H, Bartfai T. Treatment with an Interleukin 1 beta antibody improves glycemic control in diet-induced obesity. Cytokine. (2008) 44:141–8. doi: 10.1016/j.cyto.2008.07.004
590. Van Asseldonk EJP, Van Poppel PCM, Ballak DB, Stienstra R, Netea MG, Tack CJ. One week treatment with the IL-1 receptor antagonist anakinra leads to a sustained improvement in insulin sensitivity in insulin resistant patients with type 1 diabetes mellitus. Clin Immunol. (2015) 160:155–62. doi: 10.1016/j.clim.2015.06.003
591. Van Poppel PC, Van Asseldonk EJ, Holst JJ, Vilsbøll T, Netea M, Tack C. The interleukin-1 receptor antagonist anakinra improves first-phase insulin secretion and insulinogenic index in subjects with impaired glucose tolerance. Diabetes Obes Metab. (2014) 16:1269–73. doi: 10.1111/dom.12357
592. Choi S. Interleukin-1 Receptor Antagonist as a Novel Therapeutic Target for Obesity. Canberra: Australian National University (2014).
593. Motolese A, Chiaravalloti V, Cerati M, Oldrini R, Mola F, Passi A. Interleukin levels and macrophagic density in periumbilical fat tissue in patients affected by moderate-to-severe psoriasis with metabolic syndrome, before and after etanercept treatment. G Ital Dermatol Venereol. (2016) 152:342–7. doi: 10.23736/S0392-0488.16.05235-4
594. Dik B, Bahcivan E, Eser Faki H, Uney K. Combined treatment with interleukin-1 and tumor necrosis factor-alpha antagonists improve type 2 diabetes in rats. Can J Physiol Pharmacol. (2018) 96:751–6. doi: 10.1139/cjpp-2017-0769
595. Abdelrahman AM, Al Suleimani YM, Ashique M, Manoj P, Ali BH. Effect of infliximab and tocilizumab on fructose-induced hyperinsulinemia and hypertension in rats. Biomed Pharmacother. (2018) 105:182–6. doi: 10.1016/j.biopha.2018.05.118
596. Yahia H, Hassan A, El-Ansary MR, Al-Shorbagy MY, El-Yamany MF. IL-6/STAT3 and adipokine modulation using tocilizumab in rats with fructose-induced metabolic syndrome. Naunyn Schmiedebergs Archiv Pharmacol. (2020). doi: 10.1007/s00210-020-01940-z. [Epub ahead of print].
597. Hoffman E, Rahat MA, Feld J, Elias M, Rosner I, Kaly L, et al. Effects of Tocilizumab, an anti-interleukin-6 receptor antibody, on serum lipid and Adipokine levels in patients with rheumatoid arthritis. Int J Mol Sci. (2019) 20:4633. doi: 10.3390/ijms20184633
598. Wedell-Neergaard AS, Lang Lehrskov L, Christensen RH, Legaard GE, Dorph E, Larsen MK, et al. Exercise-induced changes in visceral adipose tissue mass are regulated by IL-6 signaling: a randomized controlled trial. Cell Metab. (2019) 29:844–55.e843. doi: 10.1016/j.cmet.2018.12.007
599. Guerra L, Bonetti L, Brenner D. Metabolic modulation of immunity: a new concept in cancer immunotherapy. Cell Rep. (2020) 32:107848. doi: 10.1016/j.celrep.2020.107848
600. Lee CF, Lo YC, Cheng CH, Furtmüller GJ, Oh B, Andrade-Oliveira V, et al. Preventing allograft rejection by targeting immune metabolism. Cell Rep. (2015) 13:760–70. doi: 10.1016/j.celrep.2015.09.036
601. Peterson KR, Cottam MA, Kennedy AJ, Hasty AH. Macrophage-targeted therapeutics for metabolic disease. Trends Pharmacol Sci. (2018) 39:536–46. doi: 10.1016/j.tips.2018.03.001
602. Miettinen HM, Gripentrog JM, Lord CI, Nagy JO. CD177-mediated nanoparticle targeting of human and mouse neutrophils. PLoS ONE. (2018) 13:e0200444. doi: 10.1371/journal.pone.0200444
603. Anderson EK, Gutierrez DA, Kennedy A, Hasty AH. Weight cycling increases T-cell accumulation in adipose tissue and impairs systemic glucose tolerance. Diabetes. (2013) 62:3180–8. doi: 10.2337/db12-1076
604. Zou J, Lai B, Zheng M, Chen Q, Jiang S, Song A, et al. CD4+ T cells memorize obesity and promote weight regain. Cell Mol Immunol. (2018) 15:630–9. doi: 10.1038/cmi.2017.36
605. Chatzigeorgiou A, Seijkens T, Zarzycka B, Engel D, Poggi M, Van Den Berg S, et al. Blocking CD40-TRAF6 signaling is a therapeutic target in obesity-associated insulin resistance. Proc Natl Acad Sci USA. (2014) 111:2686–91. doi: 10.1073/pnas.1400419111
606. Raghuraman S, Donkin I, Versteyhe S, Barrès R, Simar D. The emerging role of epigenetics in inflammation and immunometabolism. Trends Endocrinol Metab. (2016) 27:782–95. doi: 10.1016/j.tem.2016.06.008
607. Caslin HL, Hasty AH. Extrinsic and intrinsic immunometabolism converge: perspectives on future research and therapeutic development for obesity. Curr Obes Rep. (2019) 8:210–9. doi: 10.1007/s13679-019-00344-2
608. Hong CP, Park A, Yang BG, Yun CH, Kwak MJ, Lee GW, et al. Gut-specific delivery of T-Helper 17 cells reduces obesity and insulin resistance in mice. Gastroenterology. (2017) 152:1998–2010. doi: 10.1053/j.gastro.2017.02.016
609. Su CW, Chen CY, Li Y, Long SR, Massey W, Kumar DV, et al. Helminth infection protects against high fat diet-induced obesity via induction of alternatively activated macrophages. Sci Rep. (2018) 8:4607. doi: 10.1038/s41598-018-22920-7
610. Su CW, Chen CY, Jiao L, Long SR, Mao T, Ji Q, et al. Helminth-induced and Th2-dependent alterations of the gut microbiota attenuate obesity caused by high-fat diet. Cell Mol Gastroenterol Hepatol. (2020) 10:763–78. doi: 10.1016/j.jcmgh.2020.06.010
Keywords: adipose tissue, adipose tissue inflammation-definition of metabolic syndrome-insulin resistance-myokines-systemic inflammation, immunometabolism, adipose tissue immunology, adipose tissue browning
Citation: AlZaim I, Hammoud SH, Al-Koussa H, Ghazi A, Eid AH and El-Yazbi AF (2020) Adipose Tissue Immunomodulation: A Novel Therapeutic Approach in Cardiovascular and Metabolic Diseases. Front. Cardiovasc. Med. 7:602088. doi: 10.3389/fcvm.2020.602088
Received: 02 September 2020; Accepted: 22 October 2020;
Published: 17 November 2020.
Edited by:
George W. Booz, University of Mississippi Medical Center School of Dentistry, United StatesReviewed by:
Rei Shibata, Nagoya University Hospital, JapanP. Trayhurn, University of Liverpool, United Kingdom
Copyright © 2020 AlZaim, Hammoud, Al-Koussa, Ghazi, Eid and El-Yazbi. This is an open-access article distributed under the terms of the Creative Commons Attribution License (CC BY). The use, distribution or reproduction in other forums is permitted, provided the original author(s) and the copyright owner(s) are credited and that the original publication in this journal is cited, in accordance with accepted academic practice. No use, distribution or reproduction is permitted which does not comply with these terms.
*Correspondence: Ahmed F. El-Yazbi, YWU4OCYjeDAwMDQwO2F1Yi5lZHUubGI=
†These authors have contributed equally to this work
‡These authors have contributed equally to this work