- 1Department of Cardiovascular Surgery, Inselspital, Bern University Hospital and Department for BioMedical Research (DBMR), University of Bern, Bern, Switzerland
- 2Experimental Surgery Facility (ESF), Department for BioMedical Research (DBMR), University of Bern, Bern, Switzerland
Introduction: Donation after circulatory death (DCD) could substantially improve donor heart availability. However, warm ischemia prior to procurement is of particular concern for cardiac graft quality. We describe a rat model of DCD with in-situ ischemia in order to characterize the physiologic changes during the withdrawal period before graft procurement, to determine effects of cardioplegic graft storage, and to evaluate the post-ischemic cardiac recovery in comparison with an established ex-situ ischemia model.
Methods: Following general anesthesia in male, Wistar rats (404 ± 24 g, n = 25), withdrawal of life-sustaining therapy was simulated by diaphragm transection. Hearts underwent no ischemia or 27 min in-situ ischemia and were explanted. Ex situ, hearts were subjected to a cardioplegic flush and 15 min cold storage or not, and 60 min reperfusion. Cardiac recovery was determined and compared to published results of an entirely ex-situ ischemia model (n = 18).
Results: In donors, hearts were subjected to hypoxia and hemodynamic changes, as well as increased levels of circulating catecholamines and free fatty acids prior to circulatory arrest. Post-ischemic contractile recovery was significantly lower in the in-situ ischemia model compared to the ex-situ model, and the addition of cardioplegic storage improved developed pressure-heart rate product, but not cardiac output.
Conclusion: The in-situ model provides insight into conditions to which the heart is exposed before procurement. Compared to an entirely ex-situ ischemia model, hearts of the in-situ model demonstrated a lower post-ischemic functional recovery, potentially due to systemic changes prior to ischemia, which are partially abrogated by cardioplegic graft storage.
Introduction
Grafts obtained from donation after circulatory death (DCD) could substantially improve donor heart availability, which is a major issue in heart transplantation. However, concerns related to graft quality persist as they are subjected to a period of warm global ischemia before procurement. Despite excellent clinical outcomes in DCD heart transplantation (1, 2), further expansion of the donor pool requires the optimization of protocols to guarantee optimal graft quality.
In order to evaluate strategies for improving current DCD protocols, the choice of the most appropriate and clinically relevant animal models is essential. The ex-situ working rat heart system is a well-established experimental model that has been used in several studies to investigate cardioprotective strategies and ischemic tolerance in the context of DCD (3–8). With this model, the pre-ischemic and ischemic phases are carried out in the ex-situ heart preparation. This model allows tight control of experimental conditions, such as the precise timing of warm ischemic onset, and pre-ischemic energy substrate availability. Further, the ex-situ preparation permits detailed functional and biochemical assessment prior to warm ischemia and reperfusion. However, this model lacks the physiological changes that occur after the withdrawal of life sustaining therapy (WLST) in the donor. (9, 10). This is of particular importance in DCD, as the heart is not only exposed to warm ischemia, but also to a potentially damaging pre-ischemic phase prior to procurement.
The consideration of this pre-ischemic phase and the simulation of the DCD process in a donor is receiving more and more attention in the field, and several in-situ rat models have recently been developed (11–20). However, no standard protocol is available; for example, initiation of the DCD process can be simulated in different ways; by simply terminating the ventilatory support (14, 15, 17), by terminating ventilatory support in combination with a tracheal clamp (12, 13), with or without the addition of respiratory muscle paralysis (11, 16), or by the transection of the diaphragm (19, 20). Furthermore, not all protocols employ the same definition of the start of warm ischemia. For example, some measure cardiac asystole by electrocardiogram (11) while others define the beginning of ischemia when the peak systolic pressure drops below 30 mmHg or asystole (12, 13), or when the blood pressure was non-pulsatile or the mean arterial pressure less than 30 mmHg (14, 17). As such, strict comparison among varying pre-clinical models requires careful interpretation, but when possible, it may aid in advancing our understanding of the pathophysiologic processes involved.
Post-ischemic cardiac function, as well as the efficacy of protective strategies may not only be influenced by ischemia itself, but also by pre-ischemic conditions (9), which can alter cardiac metabolism upon reperfusion. Studies in pre-clinical models have shown that the phase between WLST and ischemia is characterized by hemodynamic instability, pressure and volume overload, significant drop up to cessation of arterial pulsatility and subsequent systemic responses that lead to catecholamine release (9, 10, 17). Besides influencing cardiac rhythm and contractility, catecholamines are also known to trigger the release of free fatty acids (FFA) into the circulation (21). Although increased pre-ischemic concentrations of FFA have not yet been measured in DCD, our group has demonstrated that pre-ischemic substrate availability, e.g., acute expose to high circulating fatty acids, can negatively affect post-ischemic cardiac recovery (22).
As interventions prior to circulatory death are limited for ethical reasons, strategies to reduce post-ischemic cardiac dysfunction that are applied after heart procurement hold much promise, particularly the initial reperfusion after warm ischemia. The majority of centers performing DCD heart transplantation are currently using St. Thomas' N°2 cardioplegia or Bretschneider histidine–tryptophan–ketoglutarate (HTK) crystalloid solution (Custodiol) supplemented with erythropoietin and glyceryl-trinitrate (23). However, the impact of perfusion with cold preservation solutions and this brief period of cold ischemia on post-ischemic functional recovery has not yet been investigated.
With the current study, we aimed to establish a clinically relevant in-situ ischemia rat model of DCD in our lab, to characterize the period between WLST until the end of functional, warm ischemia, and to evaluate the effects of cold cardioplegic storage in the in-situ model. Furthermore, we evaluated and compared post-ischemic cardiac recovery with isolated hearts from a published ex-situ ischemia model (6), using new measurements and analyses.
Materials and Methods
Ethics Statement
All experimental procedures were performed in compliance with the European Convention for Animal Care and approved by the Swiss animal welfare authorities and the Ethics Committee for Animal Experimentation, Berne, Switzerland (Veterinärdienst des Kantons Bern; BE40/15, BE103/17 and BE104/18). Surgery was performed under anesthesia, and all efforts were made to minimize animal suffering.
Animals
Male Wistar rats (Janvier Labs, Le Genest-Saint-Isle, France) were housed in groups under standard conditions with a 12 h light-dark cycle at a controlled room temperature, humidity and ad libitum access to water and food (KLIBA NAFAG, GRANOVIT AG, Kaiseraugst, Switzerland). Rats weighing 375–425 g and aged 11–12 weeks were chosen to represent young-adult, human DCD heart donors and to ensure mature cardiac metabolism (24, 25). A total of 43 rats were used in this study, and 7 rats over 6 experimental groups were excluded due to technical issues in cardiac function recording or long episodes of fibrillation (Supplementary Figure 3). The total number of rats included in each group are reported in Tables 1, 2.
Experimental Set Up
We established and characterized an in-situ rat model of DCD in our lab. With this model, four different experimental conditions were investigated: 0 and 27 min in-situ ischemia (IS), and 0 and 27 min in-situ ischemia with the addition of cardioplegia and cold storage (IS+). Post-ischemic cardiac recovery was determined following heart explantation and perfusion under normothermic conditions for 60 min. Cardiac recovery was also compared to recently published corresponding ischemic groups generated with a purely ex-situ ischemia model (ES) (6) following further measurements and analyses. The protocols of all six experimental groups are presented in Figure 1. Hearts of all six experimental groups were then reperfused ex situ to determine post-ischemic cardiac recovery.
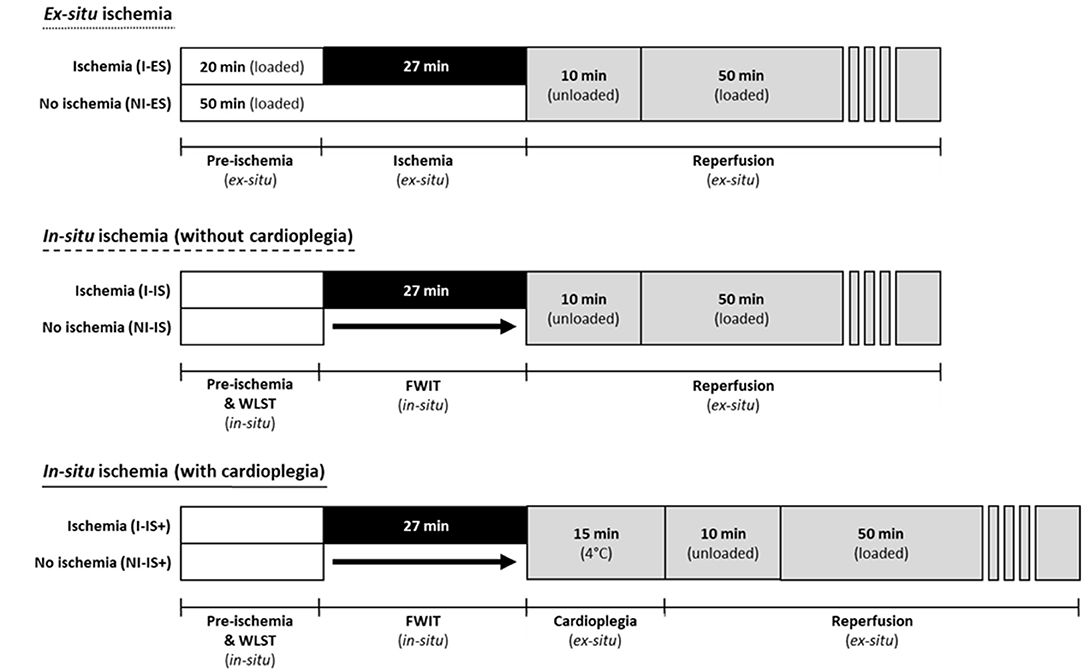
Figure 1. Perfusion protocols for all experimental groups using the ex-situ model and the in-situ model with and without cardioplegic storage. Ex-situ ischemia was induced by clamping pre- and afterload lines. In-situ ischemia/functional warm ischemia (FWIT) was induced by simulating withdrawal of life sustaining therapy (WLST). FWIT start was defined as peak systolic pressure <50 mmHg and FWIT end was defined as initial coronary perfusion (with cardioplegia or physiologic buffer).
Heart Preparation for Ischemia in-situ
Surgical anesthesia was induced in rats with an intraperitoneal injection of 78 mg/kg ketamine (Narketan®, Vetoquinol AG, Bern, Switzerland), 7.2 mg/kg xylazine (Xylapan®, Vetoquinol AG, Bern, Switzerland), and 1.2 mg/kg acepromazine (Prequillan, Fatro AG, Bologna, Italy). Arterial oxygen saturation (SaO2) was monitored using a toe-clip pulse oximeter (AD Instruments, Spechbach, Germany). Animals received 100% oxygen via a face mask and flow adjustments were made to keep SaO2 between 95 and 99%. When the hind-paw withdrawal reflex could not be elicited after toe pinch, a catheter was inserted into the right common carotid artery and connected to a pressure transducer for continuous recording of blood pressure and heart rate. Seven hundred and fifty U.I./kg of sodium heparin (Liquemin®, Drossapharm AG, Arlesheim, Switzerland) were injected through this catheter, which also served as blood sampling port. Withdrawal of life sustaining therapy was simulated by asphyxiation through transection of the diaphragm. Functional warm ischemic time (FWIT) was considered to start when the peak systolic pressure dropped below 50 mmHg. Circulatory arrest was declared when the pulse pressure was lower than 3 mmHg. Body temperature was measured by introducing a sensor tip thermometer into the thoracic cavity and temperature was kept between 36.6 and 37.2°C using a heating lamp (if required). After 27 min of ischemia, all hearts were explanted, and aortae cannulated in the same isolated heart system used with the ex-situ ischemia model for the reperfusion period where cardioplegia was administered following the current clinical practice: hearts were flushed with 7 mL ice cold St. Thomas' N°2 cardioplegic solution supplemented with 100 mg/mL glyceryl trinitrate (GTN; Nitroglycerin Bioren, Sintetica, Mendrisio, Switzerland) and 5,000 U/L erythropoietin (EPO; Eprex, Janssen, Berchem, Belgium) (26) at a constant pressure of 60 mmHg and then statically stored for a total of 15 min immersed in ice-cold St. Thomas' N°2 cardioplegia. FWIT was considered to end with the initiation of the cardioplegic flush.
Heart Preparation for Ischemia ex-situ
Surgical anesthesia was induced in rats with an intraperitoneal injection of 100 mg/kg ketamine (Narketan®, Vetoquinol AG, Bern, Switzerland), and 10 mg/kg xylazine (Xylapan®, Vetoquinol AG, Bern, Switzerland). Adequate depth of anesthesia was confirmed by absence of the hind-paw withdrawal reflex. Hearts were then rapidly excised, placed in ice-cold phosphate-buffered saline, and working- mode preparations were established. Isolated, working rat hearts were prepared as previously described (5, 6). Briefly, hearts were aerobically perfused with a modified Krebs-Henseleit buffer containing 1.25 mM Ca2+, 11 mM glucose, 1.2 mM palmitate bound to 3% BSA and 0.5 mM lactate oxygenated with 95% O2-5% CO2 for a pre-ischemic period of 20 min at a constant pressure of 80 mmHg. Ischemia of 27 min was initiated and terminated by clamping and unclamping of the pre- and after- load perfusion lines, respectively. During the ischemic period, the heart was immersed in a glucose- and palmitate-free Krebs-Henseleit buffer at 37°C.
Protocol for Post-ischemic Heart Perfusions (Both Models)
Ischemic and non-ischemic hearts from both models were reperfused for a total of 60 min using a modified Krebs-Henseleit buffer containing 1.25 mM Ca2+ and 11 mM glucose. Hearts remained unloaded during the first 10 min. During this time, the left atria of hearts from the in-situ model were cannulated. The perfusion was then switched to working mode for another 50 min. During the 60 min reperfusion, perfusate was recirculating, except the first minute of reperfusion in hearts obtained with the in-situ model, when cardioplegia or blood was washed out and discarded. All hearts were maintained at 37°C with an aortic pressure of 60 mmHg. At the end of the protocol, hearts were immediately frozen in liquid nitrogen and stored at −80°C.
Assessment of Cardiac Function
Intraventricular cardiac pressure was measured using a micro-tip pressure catheter (Millar, Houston, USA) placed in the left ventricle. Perfusate flow was measured using flow probes (Transonic Systems Inc., Ithaca, USA) placed in pre- and after- load lines. Data were continuously recorded using a PowerLab data acquisition system (ADInstruments, Spechbach, Germany).
Blood and Perfusate Sampling
During the in-situ ischemia protocol, blood analysis was performed before withdrawal, during the withdrawal phase (FWIT and cardiac arrest time points) and, for the ischemic hearts, at the end of the 27 min in-situ ischemia. Blood samples were obtained from the carotid artery (arterial blood) prior to heart procurement, and from the chest cavity after heart procurement at end-ischemia (mixed arterial-venous blood). Samples were used for blood gas analysis (see details in the Supplementary Material) and the remainder was transferred to lithium heparin tubes and centrifuged at 2,000g for 5 min. Plasma was stored at −80°C.
During post-ischemic heart perfusions, coronary effluent and circulating perfusate were sampled at multiple time points. Samples were stored at −80°C.
Additional Methods
The following methods and materials are described in detail in the Supplementary Material: measurement of blood gas, circulating factors and measurement of tissue water content.
Statistical Analysis
Values are reported as mean ± standard deviation or as median, 25–75 percentiles and range (box-and-whiskers). Statistical analyses were performed with GraphPad Prism software (GraphPad Software, Inc., La Jolla, CA). The Kruskal-Wallis test was performed for an overview of differences between experimental groups and, when significant overall results were observed, comparisons between groups of interest were performed with Mann-Whitney analyses. Two-tailed p-values were adjusted for multiple comparisons (modified, sequential, rejective Bonferroni procedure) (27). Corrected p-values are reported and considered statistically significant if <0.05.
Results
Baseline Characteristics
Baseline characteristics for the ischemic ex-situ and in-situ groups are represented in Tables 1, 2, respectively. No difference in baseline characteristics among experimental groups was observed in either the ex-situ or the in-situ model.
Withdrawal Phase (in-situ Ischemia Model)
The average time from WLST to FWIT start was 1.0 ± 0.6 min. For hearts that underwent ischemia, mean time from FWIT start to circulatory arrest was 1.9 ± 0.9 min. No difference among experimental groups was observed (Supplementary Table 1).
Peak systolic pressure in rats dropped significantly after WLST and was, per definition, 50 mmHg at FWIT start (p < 0.05; Figure 2A). A hyperdynamic phase was observed for heart rate and pulse pressure. Heart rate increased after WLST (p < 0.05) before it finally dropped (Figure 2B). Similarly, pulse pressure appeared to increase after WLST, however this change did not reach statistical significance. Subsequently, pulse pressure continuously dropped to 3 mmHg, at which time circulatory arrest was declared (p < 0.05 hyperdynamic phase vs. FWIT and FWIT vs. cardiac arrest; Figure 2C).
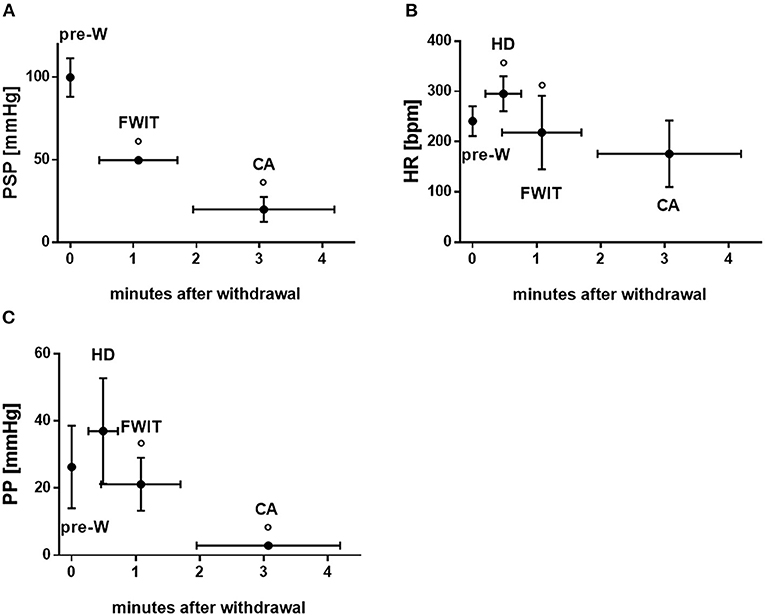
Figure 2. Functional parameters during in-situ/withdrawal phase. (A) Peak systolic pressure (PSP) (B) heart rate (HR) (C) pulse pressure (PP). CA, circulatory arrest; FWIT, functional warm ischemia start; HD, hyperdynamic phase; pre-W, pre-withdrawal of life sustaining therapy. Data are expressed as mean ± standard deviation. Statistical analyses were only performed between consecutive time points. °p < 0.05 vs. corresponding previous time point. n = 10–21/time point.
Blood O2 saturation immediately dropped after WLST and was below the detection level (<30%) for all subsequent measurement time points (Figure 3A). A similar steep decline was observed for the partial pressure of oxygen (PO2), as shown in Figure 3B. Partial pressure of carbon dioxide (PCO2) at the pre-withdrawal of life sustaining therapy time point already indicated hypercapnia (63.3 ± 16.4 mmHg). PCO2 after WLST increased and reached the upper detection threshold (>150 mmHg) at the end of ischemia (p < 0.05 cardiac arrest vs. end-ischemia; Figure 3B).
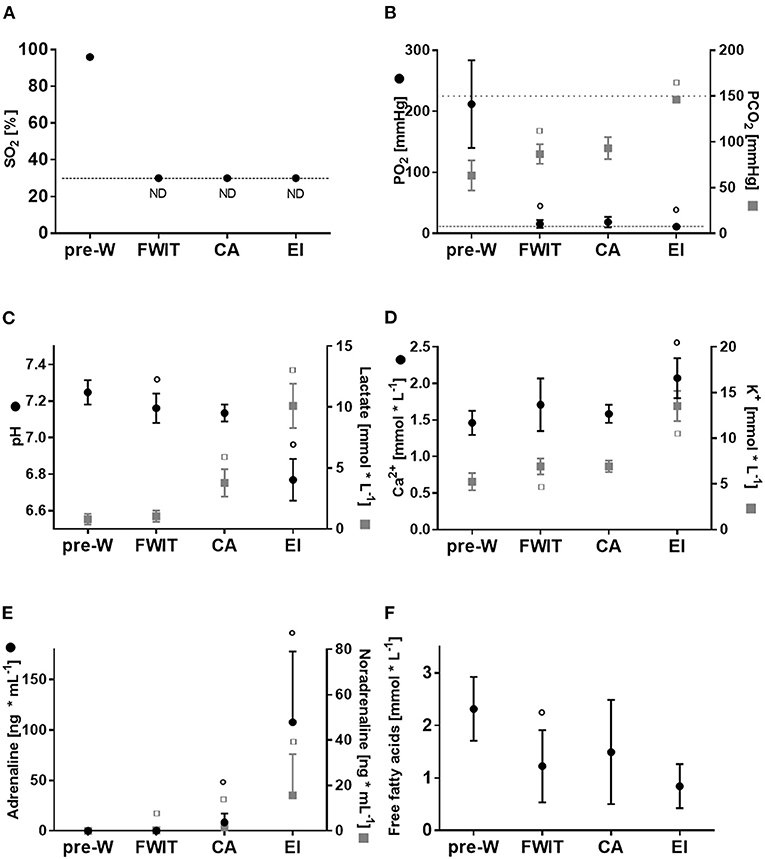
Figure 3. Blood parameters during in-situ/withdrawal phase. (A) Oxygen saturation (SO2) (B) partial oxygen and carbon dioxide pressure (PO2 and PCO2) (C) blood pH and lactate (D) blood calcium (Ca2+) and potassium (K+) (E) blood adrenaline and noradrenaline (F) blood free fatty acids. CA, circulatory arrest; EI, end-ischemia; FWIT, functional warm ischemia start; ND, below detection limit; pre-W, pre-withdrawal of life sustaining therapy. Data are expressed as mean ± standard deviation. Statistical analyses were only performed between consecutive time points. ° or □ p < 0.05 vs. corresponding previous time point. n = 8–21/time point.
Blood pH dropped significantly from pre-withdrawal of life sustaining therapy to FWIT (p < 0.05), and remained stable from FWIT to cardiac arrest. At the end of 27 min ischemia, pH further decreased to a profound acidosis (p < 0.05; Figure 3C) with a concomitant increase in blood lactate concentration (Figure 3C).
Both blood calcium and potassium concentrations increased, being highest at end ischemia (Figure 3D). Blood sodium, chloride and glucose concentrations are presented in Supplementary Figure 1.
Concentrations of catecholamines in blood are represented in Figure 3E; adrenaline and noradrenaline both increased at FWIT to cardiac arrest (p < 0.05 for both; Figure 3E). Peak concentrations were reached at end ischemia.
Blood free fatty acid concentrations peaked before withdrawal and dropped significantly by FWIT (p < 0.05; Figure 3F).
Post-ischemic Functional Recovery
Post-ischemic functional recovery is presented in Figure 4 and Supplementary Figure 2. In general, left ventricular work at the end of reperfusion (Figure 4A) was lower after ischemia compared to the corresponding non-ischemic groups, reaching statistical significance for IS and ES groups (p < 0.05 for both). Furthermore, among non-ischemic hearts, left ventricular work tended to be lower in ES vs. IS and IS+ hearts, reaching statistical significance between IS and ES groups (p < 0.05). However, among ischemic hearts, left ventricular work was significantly decreased in IS vs. ES hearts (p < 0.05). Thus, recovery expressed as percentage of corresponding non-ischemic group, was significantly lower in IS vs. ES (45 ± 13% vs. 78 ± 9%, respectively, p < 0.05). Interestingly, no differences were observed between IS+ and ES hearts for both absolute values and percentage recovery of left ventricular work.
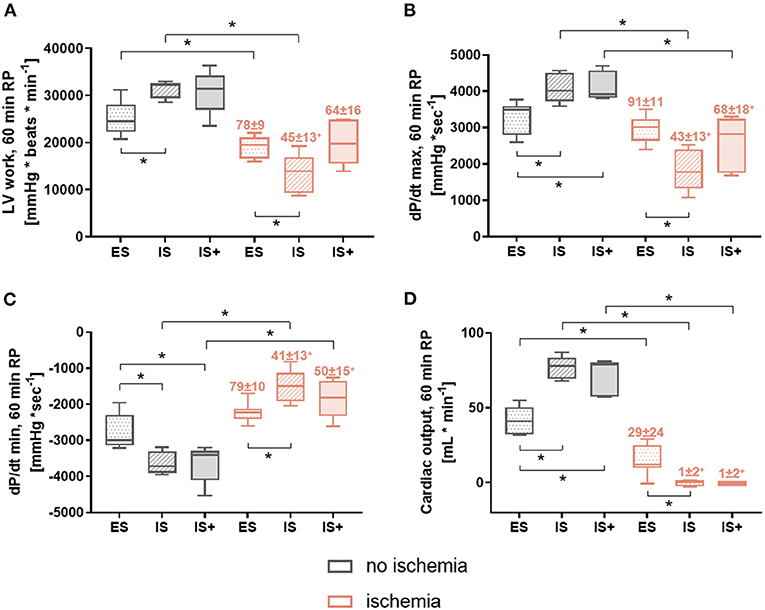
Figure 4. Post-ischemic cardiac function at 60 min reperfusion. (A) LV work (left ventricular work: heart rate*developed pressure) (B) dP/dt max (maximum first derivative of LV pressure) (C) dP/dt min (minimum first derivative of LV pressure) (D) CO (cardiac output). Data are expressed as median, 25–75 percentiles, and range; numbers indicate percentage of corresponding non-ischemic value. *p < 0.05, +p < 0.05 vs. ES. ES, ex-situ model; IS, in-situ model; IS+, in-situ model with cardioplegia; RP, reperfusion; n = 5–8 per group.
Similar patterns were observed for contractility rate (dP/dt max) and relaxation rate (dP/dt min) at 60 min of reperfusion (Figures 4B,C); however, although a tendency for improved recovery was observed with IS+ compared to IS hearts, the percent recovery for these hearts remained significantly lower than that of ES hearts.
The results of cardiac output (Figure 4D) were also similar; however, the addition of cardioplegia did not appear to improve recovery.
Vascular Parameters
During early reperfusion (5 min), coronary flow was significantly lower in ischemic vs. corresponding non-ischemic hearts in the in-situ model (p < 0.05 for IS and IS+), but not in the ex-situ model (Figure 5A). Among non-ischemic groups, coronary flow was significantly increased in the IS+ hearts compared to the ES hearts (p < 0.05; Figure 5A), suggesting greater vasodilation. While in ischemic hearts, coronary flow was significantly decreased in IS and IS+ hearts compared with ES hearts (p < 0.05 for both; Figure 5A), and cardioplegic storage did not affect coronary flow.
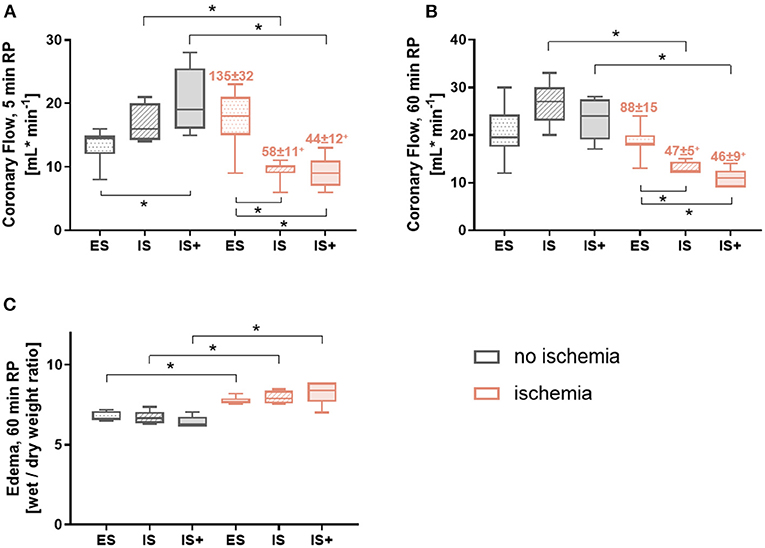
Figure 5. Vascular parameters. (A) Coronary flow at 5 min reperfusion (B) Coronary flow at 60 min reperfusion (C) Tissue water content at 60 min reperfusion. Data are expressed as median, 25–75 percentiles, and range; numbers indicate percentage of corresponding non-ischemic value. *p < 0.05, +p < 0.05 vs. ES. ES, ex-situ model; IS, in-situ model; IS+, in-situ model with cardioplegia; RP, reperfusion; n = 5–8 per group.
Similarly, after 60 min reperfusion, coronary flow was significantly lower in ischemic vs. the corresponding non-ischemic hearts in the in-situ model (p < 0.05 for IS and IS+), but not in the ex-situ model (Figure 5B). Among ischemic hearts, coronary flow was significantly lower in the in-situ model compared with the ex-situ model (p < 0.05 for both; Figure 5A), and cardioplegic storage did not affect coronary flow.
Organ edema was significantly increased with ischemia in all the groups compared to their corresponding non-ischemic groups (p < 0.05 for all; Figure 5C). No differences among either ischemic groups or non-ischemic groups were observed.
Circulating Factors
At 60 min reperfusion, cardiac troponin I was significantly higher in all ischemic groups compared to their corresponding non-ischemic groups (p < 0.05 for all), and no differences were observed among ischemic or non-ischemic groups (Figure 6A).
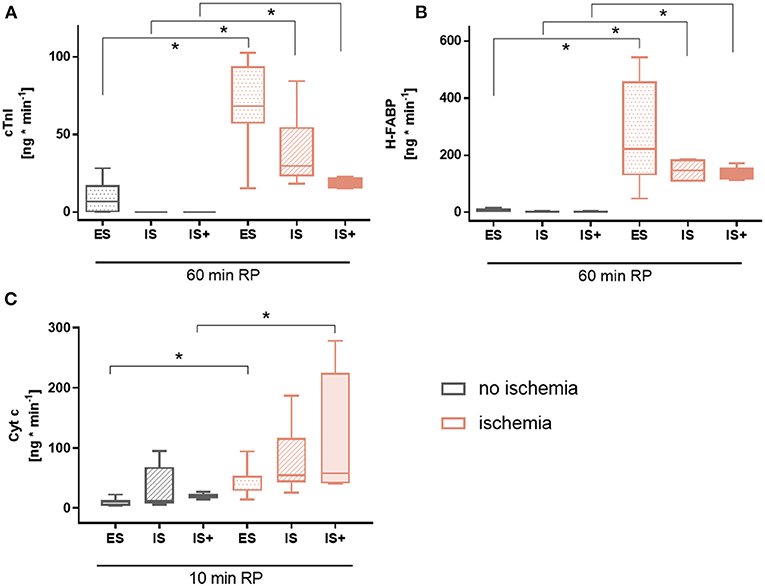
Figure 6. Circulating factors. (A) Cardiac troponin I (cTnI) release at 60 min reperfusion (B) Heart-type fatty acid binding protein (H-FABP) release at 60 min reperfusion (C) Cytochrome c (Cyt c) release at 10 min reperfusion. Data are expressed as median, 25–75 percentiles and range. *p < 0.05. ES, ex-situ model; IS, in-situ model; IS+, in-situ model with cardioplegia; RP, reperfusion; n = 5–8 per group.
A similar pattern was observed for heart-type fatty acid binding protein (p < 0.05 for all; Figure 6B).
Cytochrome c, a marker of mitochondrial damage, tended to be increased in all ischemic groups compared to their corresponding non-ischemic groups, but reached statistical significance only for ES and IS+ hearts (p < 0.05 for both; Figure 6C). No differences were observed among ischemic or non-ischemic groups.
Discussion
For the safe expansion of DCD heart transplantation, preclinical research models are required to help optimize current clinical protocols. Although large animal models, such as porcine models, are necessary to test clinical relevance, studies in smaller animal models, such as rats, are more easily manageable with respect to both technical and financial aspects, thereby allowing only the most promising approaches to proceed with testing in larger animal models. We describe an in-situ ischemia rat model of DCD, with characterization of the withdrawal phase, investigation of the effects of a brief period of cold cardioplegia, and comparison of cardiac recovery with a completely ex-situ ischemia model. Simulation of withdrawal of life sustaining therapy in the in-situ model of DCD induced a hyperdynamic cardiac phase and an elevation in plasma catecholamines before circulatory arrest, which is in agreement with other pre-clinical models of DCD (9, 10, 17). Interestingly, we report that circulating concentrations of FFA are also increased, which is of particular importance as high concentrations of pre-ischemic fatty acids are associated with substantially reduced contractile recovery and greater mitochondrial damage (22). Significantly lower post-ischemic contractile recovery was observed with in-situ ischemia (IS) compared to ex-situ ischemia (ES). However, the addition of a brief period of cold ischemia with cardioplegia did not further reduce recovery, rather it provided an improvement in left ventricular work compared to in-situ ischemia without cardioplegia. However, cardioplegia was not able to rescue decreased post-ischemic coronary flow, which was significantly lower in both in-situ conditions vs. ES, suggesting greater endothelial dysfunction/damage. Importantly, no difference between models was observed for markers of cell death or mitochondrial damage. In summary, we conclude that the choice of experimental model can affect cardiac recovery in a variable-specific manner and that models for cardiac DCD require careful selection according to the particular research focus (Table 3).
In the in-situ model of DCD, hearts are exposed to variable conditions prior to procurement, which can impact on cardiac recovery. Upon simulation of WLST, hearts developed a hyperdynamic phase characterized by increased heart rate and pulse pressure, whereas blood oxygen saturation and mean peak systolic pressure continuously decreased. This hyperdynamic phase was previously described in other preclinical models of DCD (9, 10, 17). As the donor heart initially works under rapidly developing hypotension and hypoxia, instantaneous systemic responses to restore blood pressure are stimulated, including catecholamine release. Accordingly, we detected increased concentrations of catecholamines and circulating FFA in plasma of rats at the end of the withdrawal, as well as the ischemic phase. Besides influencing cardiac rhythm, contractility and increasing intracranial pressure (28), catecholamines have been shown in experimental settings to cause lactate accumulation, ATP depletion, myocyte damage, and contraction band necrosis (29, 30). Furthermore, catecholamines also trigger adaptations in cardiac metabolism. Despite the tendency to decrease between withdrawal of treatment and heart procurement, concentrations of FFA remained high compared with physiologic values (0.4 mM) (31). Sympathetic stimulation induces lipolysis of stored glycerides in adipocytes and increases concentrations of circulating FFA. This is of particular importance as FFA have been demonstrated to exacerbate reperfusion injury (22). Upon reperfusion, as soon as oxygen is reintroduced to the tissue, oxidation of FFA is stimulated and increases mitochondrial ratios of NADH/NAD+ and acetyl-CoA/CoA, both of which activate pyruvate dehydrogenase kinase. Activated pyruvate dehydrogenase kinase inhibits pyruvate dehydrogenase, the rate limiting enzyme of glucose oxidation. Reduced glucose oxidation at the beginning of reperfusion results in uncoupling between glycolysis and glucose oxidation and to a greater conversion of pyruvate to lactate. Acidification of the cytoplasm can further aggravate calcium overload and reperfusion injury (32). Another cause of high concentrations of circulating FFA is heparin. In DCD, heparin concentrations used are several times higher compared with ordinary clinical anticoagulation (>300 units/kg vs. ~80 units/kg) (33). Furthermore, in agreement with other studies (34), heparinization was required in this model to achieve effective heart reperfusion and prevent from clot formation. In addition, heparin activates lipoprotein lipase at the endothelial surface and stimulates the release of FFA from circulating lipoproteins (31). Our results do not permit the distinction between effects of heparinization and catecholamine release on circulating FFA. However, as the circulating FFA were already high during pre-withdrawal of life sustaining therapy, when measured catecholamine concentrations were very low, it is likely that initial free fatty acid release was induced by heparin and that subsequent catecholamine-mediated lipolysis was masked. Additionally, the increasing pattern of catecholamines in parallel with the decreasing pattern of FFA during the withdrawal phase also suggest that FFA release was likely initially induced by heparin addition. It is technically difficult to determine circulating FFA accurately in heparinized blood as lipolysis may continue after sample collection, and an overestimation in our samples of 20–50% can be expected (35). However, taking this into consideration, the FFA levels measured during the withdrawal period are still substantially higher than physiologic levels.
As systemic changes during the withdrawal phase and ischemia in situ can influence post-ischemic cardiac recovery, it is essential that they are reflected in experimental models. Over the last years, we developed an ex-situ model that includes specific DCD-relevant conditions (3–6, 8) with, e.g., tight temperature control during warm ischemia and the use of supraphysiologic perfusate FFA concentrations (1.2 mM palmitate) prior to ischemia. Nonetheless, some differences in post-ischemic recovery between in-situ and ex-situ models persist and may be explained by systemic changes during withdrawal and ischemia in situ. In general, post-ischemic recovery was higher in the ex-situ vs. in-situ model. In previous work with our ex-situ model, functional recovery deteriorated only significantly after 27 min ischemia, with a steep decline between 27 and 33 min ischemia (5, 6). In the in-situ model, before 27 min FWIT (starting with peak systolic pressure <50 mmHg), the entire body and heart are exposed to hypoxia during the withdrawal period as demonstrated by low oxygen saturation, supraphysiologic concentrations of circulating lactate and CO2 at FWIT. Pre-ischemic hypoxia may be detrimental through the reduction of ATP production via oxidative phosphorylation, in parallel with increased lactate production and associated acidosis. It is known that hypoxia may also exert protective effects, as it could stimulate pro-survival pathways activated by pre-conditioning; however, these protocols generally require intermittent application by several cycles of ischemia and reperfusion (36). Therefore, the additional exposure to hypoxia/ischemia could be a reason for the lower post-ischemic recovery in IS vs. ES hearts. Further damage may also result from increased levels of catecholamines, as catecholamine storms have been associated with detrimental organ function in brain dead donors (37, 38).
Although the cold, cardioplegic storage used in this study adds a potentially damaging period of cold ischemia, our findings indicate that it may provide some cardioprotection, potentially via the cold, coronary flush and addition of protective agents. Although not significantly different, tendencies for improved contractile function and lower release of the cell death marker cardiac troponin I were visible in IS+ vs. IS groups. EPO, a component of the cardioplegia, has been shown to be cardioprotective, through the activation of the pro-survival Akt signaling pathway and decreased cell death (39–41). GTN is a nitric oxide donor and a widely used drug to treat ischemic heart disease (42). The addition of GTN and EPO in Celsior cardioplegia was cardioprotective in rat and pig heart models (43, 44). EPO and GTN may therefore contribute to the trend of improved recovery in IS+ vs. IS hearts. Although we see improved contractile function and reduced release of cell death markers in IS+ vs. IS groups, cardioprotection could potentially be further optimized with use of a preservation solution that is better suited for DCD organs than St. Thomas' N°2, for example with a normokalemic and hypocalcemic solution.
In this study, ischemia is associated with substantial changes in coronary flow. In the absence of ischemia, coronary flow at 5 min reperfusion was higher in IS+ vs. ES hearts. This higher coronary flow may result from the hyperemic response since IS+ hearts were subjected to a short period of ischemia during procurement and cannulation, and cold storage when used, immediately prior to reperfusion. During ischemia, the heart produces vasoactive compounds, such as adenosine, leading to the vasodilation of the arterioles, the reduction of vascular resistance and elevated coronary flow upon reperfusion, resulting in hyperemia (45). Furthermore, with the cardioplegia, IS+ hearts received both GTN, a potent vasodilator via donation of nitric oxide (46), and EPO, which has been shown to upregulate endothelial nitric oxide synthase -derived nitric oxide production by increasing phosphorylation and expression through PI3-kinase/Akt (47). Thus, hyperemia, as well as GTN and EPO supplementation, likely contribute to increased coronary flow in IS+ vs. ES hearts in the absence of warm ischemia. Post-ischemic coronary flow was significantly lower in IS and IS+ vs. ES hearts at both early and late reperfusion time points. Although the mechanism for this is not entirely clear, it is unlikely to result from differences in edema-induced flow reductions since all post-ischemic groups demonstrated similar levels of edema. Contractile function tended to be greater in ES hearts, potentially leading to a greater metabolic demand, which could stimulate coronary flow. Alternatively, one might speculate that reductions in coronary flow in IS and IS+ hearts may be a result of greater exposure to adhesion molecules promoting platelet aggregates, platelet-leukocyte aggregates and erythrocyte aggregates in hearts undergoing ischemia in situ, which could block the capillaries and lead to no-reflow (48).
Although the in-situ withdrawal period and ischemia represent major differences from the ex-situ model, additional differences are present between models that could influence outcomes. For example, our in-situ model requires ante-mortem heparinization, whereas no heparin was used in the ex-situ model. Pre-ischemic heparin has been reported to reduce ischemia-reperfusion injury in rabbit hearts (49, 50); however, we generally observed reduced recovery in hearts exposed to heparin. Furthermore, isolated heart preparations are recognized to deteriorate with time (51) and our in-situ ischemia hearts were maintained in the isolated system for approximately half the time of those from the ex-situ model, which would be expected to result in lower post-ischemic function in ES vs. IS and IS+ hearts, which is opposite to our observations. Lastly, due to the ante-mortem surgical intervention (cannulation of carotid artery), the anesthetic protocol for the in-situ model (ketamine, xylazine, acepromazine) differs from that of the ex-situ model (ketamine, xylazine).
Limitations
This study has several limitations. The models we used do not account for neurologic injury, which is almost always present in DCD donors, and could potentially lead to remote preconditioning. In our in-situ model, WLST is simulated by dissection of the animal's diaphragm (asphyxiation model of DCD), which may result in a shorter duration of the period between WLST and circulatory arrest compared with human DCD donors who may display remnants of spontaneous respiration (52). Additionally, due to the fact that rats did not undergo intermittent positive pressure ventilation during general anesthesia, hypercapnia and respiratory acidosis were present before WLST, which can be avoided in human DCD donors. The 15-min cold static period implemented in the group of hearts treated with St. Thomas' N°2 cardioplegic solution is a relatively short period in the clinical setting for preparation of the heart and/or the ex-situ perfusion machine. Future work could include additional studies to better characterize the loss of myocardial function in DCD by measuring, for example, infarct size; as well as studies with increased sample size to confirm the relevance of our results.
Conclusions
In this study, we characterized an in-situ rat model of DCD. DCD grafts were subjected to hypoxia, hemodynamic changes, acidosis, and increased concentrations of catecholamines and circulating FFA before and during ischemia, which highlights the suitability of this model for further research. In-situ conditions were more detrimental for hearts vs. ex-situ conditions in terms of post-ischemic contractility and relaxation rates, and cardiac output. The application of cardioplegia tended to abrogate differences compared to ES for cardiac function, with the exception of cardiac output and coronary flow. However, given the complexity of WLST and ischemia in situ, future studies toward the optimization of clinical DCD protocols will certainly benefit from preclinical models, which incorporates the effects of systemic, and blood-borne changes upon WLST.
Data Availability Statement
The raw data supporting the conclusions of this article will be made available by the authors, without undue reservation.
Ethics Statement
The animal study was reviewed and approved by Ethics Committee for Animal Experimentation, Berne, Switzerland (Veterinärdienst des Kantons Bern).
Author Contributions
MA, NM-C, and RW contributed to all aspects of this manuscript including study planning and design, model development, performing experiments, data collection and analysis, and preparation of the manuscript. AJ participated in study planning and design, model development, performing experiments, and data collection and analysis. DC and SL participated in study planning and design, model development, data analysis, and preparation of the manuscript. TC participated in data analysis and preparation of the manuscript. All authors contributed to the article and approved the submitted version.
Funding
This study was supported by a Project Grant from the Swiss Heart Foundation (FF18089) and the Stiftung zur Förderung der herzchirurgischen Forschung am Inselspital.
Conflict of Interest
The authors declare that the research was conducted in the absence of any commercial or financial relationships that could be construed as a potential conflict of interest.
Acknowledgments
We would like to thank Olgica Beslac from the Experimental Surgery Facility (ESF) of the University of Bern for the training of surgical skills.
Supplementary Material
The Supplementary Material for this article can be found online at: https://www.frontiersin.org/articles/10.3389/fcvm.2020.596883/full#supplementary-material
Abbreviations
DCD, donation after circulatory death; dP/dt max, maximum first derivative of left ventricular pressure; dP/dt min, minimum first derivative of left ventricular pressure; EPO, erythropoietin; ES, ischemic ex-situ model; FFA, free fatty acids; FWIT, functional warm ischemic time; GTN, glyceryl trinitrate; IS, ischemic in-situ model; IS+, ischemic in-situ model with cardioplegia; min, minutes; PCO2, partial carbon dioxide pressure; PO2, partial oxygen pressure; WLST, withdrawal of life sustaining therapy.
References
1. Chew HC, Iyer A, Connellan M, Scheuer S, Villanueva J, Gao L, et al. Outcomes of donation after circulatory death heart transplantation in Australia. J Am Coll Cardiol. (2019) 73:1447–59. doi: 10.1016/j.jacc.2018.12.067
2. Messer S, Cernic S, Page A, Berman M, Kaul P, Colah S, et al. 5-Year single centre early experience of heart transplantation from donation after circulatory determined death (DCD) donors. J Heart Lung Transpl. (2020) 39:1463–75. doi: 10.1016/j.healun.2020.10.001
3. Farine E, Niederberger P, Wyss RK, Méndez-Carmona N, Gahl B, Fiedler GM, et al. Controlled reperfusion strategies improve cardiac hemodynamic recovery after warm global ischemia in an isolated, working rat heart model of donation after circulatory death (DCD). Front Physiol. (2016) 7:543. doi: 10.3389/fphys.2016.00543
4. Sanz MN, Farine E, Niederberger P, Méndez-Carmona N, Wyss RK, Arnold M, et al. Cardioprotective reperfusion strategies differentially affect mitochondria: studies in an isolated rat heart model of donation after circulatory death (DCD). Am J Transpl. (2019) 19:331–44. doi: 10.1111/ajt.15024
5. Wyss RK, Méndez-Carmona N, Sanz MN, Arnold M, Segiser A, Fiedler GM, et al. Mitochondrial integrity during early reperfusion in an isolated rat heart model of donation after circulatory death—consequences of ischemic duration. J Heart Lung Transpl. (2019) 38:647–57. doi: 10.1016/j.healun.2018.12.013
6. Méndez-Carmona N, Wyss RK, Arnold M, Joachimbauer A, Segiser A, Fiedler GM, et al. Differential effects of ischemia/reperfusion on endothelial function and contractility in donation after circulatory death. J Heart Lung Transpl. (2019) 38:767–77. doi: 10.1016/j.healun.2019.03.004
7. Bartkevics M, Huber S, Mathys V, Sourdon J, Dornbierer M, Carmona Mendez N, et al. Efficacy of mechanical postconditioning following warm, global ischaemia depends on circulating fatty acid levels in an isolated, working rat heart model. Eur J Cardio Thoracic Surg. (2016) 49:32–39. doi: 10.1093/ejcts/ezv008
8. Arnold M, Méndez-Carmona N, Gulac P, Wyss RK, Rutishauser N, Segiser A, et al. Mechanical postconditioning promotes glucose metabolism and AMPK activity in parallel with improved post-ischemic recovery in an isolated rat heart model of donation after circulatory death. Int J Mol Sci. (2020) 21:964. doi: 10.3390/ijms21030964
9. White CW, Lillico R, Sandha J, Hasanally D, Wang F, Ambrose E, et al. Physiologic changes in the heart following cessation of mechanical ventilation in a porcine model of donation after circulatory death: implications for cardiac transplantation: physiologic response to donor extubation. Am J Transpl. (2016) 16:783–93. doi: 10.1111/ajt.13543
10. Iyer A, Chew HC, Gao L, Villanueva J, Hicks M, Doyle A, et al. Pathophysiological trends during withdrawal of life support: implications for organ donation after circulatory death. Transplantation. (2016) 100:2621–9. doi: 10.1097/TP.0000000000001396
11. Quader M, Akande O, Toldo S, Cholyway R, Kang L, Lesnefsky EJ, et al. The commonalities and differences in mitochondrial dysfunction between ex vivo and in vivo myocardial global ischemia rat heart models: implications for donation after circulatory death research. Front Physiol. (2020) 11:681. doi: 10.3389/fphys.2020.00681
12. Aceros H, Der Sarkissian S, Borie M, Pinto Ribeiro RV, Maltais S, Stevens LM, et al. Novel heat shock protein 90 inhibitor improves cardiac recovery in a rodent model of donation after circulatory death. J Thoracic Cardiovasc Surg. (in press) doi: 10.1016/j.jtcvs.2020.03.042
13. Aceros H, Joulali L, Borie M, Ribeiro RVP, Badiwala MV, Sarkissian SD, et al. Pre-clinical model of cardiac donation after circulatory death. J. Vis Exp. (2019) 150:e59789. doi: 10.3791/59789
14. Kearns MJ, Miller SD, Kong HJ, Sirounis D, Cheung A, Bashir J, et al. Oligonucleotide-based preconditioning of DCD cardiac donors and its impact on cardiac viability. Transplantation. (2019) 103:2479–85. doi: 10.1097/TP.0000000000002849
15. Lan H, Su Y, Liu Y, Deng C, Wang J, Chen T, et al. Melatonin protects circulatory death heart from ischemia/reperfusion injury via the JAK2/STAT3 signalling pathway. Life Sci. (2019) 228:35–46. doi: 10.1016/j.lfs.2019.04.057
16. Quader M, Toldo S, Torrado J, Mishra S, Mauro AG, Mezzaroma E, et al. Determination of optimal coronary flow for the preservation of “Donation after circulatory death” in murine heart model. ASAIO J. (2018) 64:225–31. doi: 10.1097/MAT.0000000000000630
17. Kearns MJ, Miller SD, Cheung A, Bashir J, Wong S, Seidman MA, et al. A rodent model of cardiac donation after circulatory death and novel biomarkers of cardiac viability during ex vivo heart perfusion. Transplantation. (2017) 101:e231–9. doi: 10.1097/TP.0000000000001815
18. Yamada T, Chen F, Sakamoto J, Nakajima D, Ohsumi A, Bando T, et al. Impact of the cardiac arrest mode on cardiac death donor lungs. J Surg Res. (2015) 195:596–603. doi: 10.1016/j.jss.2015.02.029
19. Tolboom H, Olejníčková V, Reser D, Rosser B, Wilhelm MJ, Gassmann M, et al. Moderate hypothermia during ex vivo machine perfusion promotes recovery of hearts donated after cardiocirculatory death. Eur J Cardio Thoracic Surg. (2016) 49:25–31. doi: 10.1093/ejcts/ezv066
20. Schlegel A. Hypothermic oxygenated perfusion (HOPE) protects from biliary injury in a rodent model of DCD liver transplantation. J Hepatol. (2013) 59:984–91. doi: 10.1016/j.jhep.2013.06.022
21. Spitzer JJ, Gold M. Effect of catecholamines on the individual free fatty acids of plasma. Exp Biol Med. (1962) 110:645–7. doi: 10.3181/00379727-110-27604
22. Niederberger P, Farine E, Arnold M, Wyss RK, Sanz MN, Méndez-Carmona N, et al. High pre-ischemic fatty acid levels decrease cardiac recovery in an isolated rat heart model of donation after circulatory death. Metabolism. (2017) 71:107–17. doi: 10.1016/j.metabol.2017.03.007
23. White CW, Messer SJ, Large SR, Conway J, Kim DH, Kutsogiannis DJ, et al. Transplantation of hearts donated after circulatory death. Front Cardiovasc Med. (2018) 5:8. doi: 10.3389/fcvm.2018.00008
24. Fischer A, ten Hove M, Sebag-Montefiore L, Wagner H, Clarke K, Watkins H, et al. Changes in creatine transporter function during cardiac maturation in the rat. BMC Dev Biol. (2010) 10:70. doi: 10.1186/1471-213X-10-70
25. Anmann T, Varikmaa M, Timohhina N, Tepp K, Shevchuk I, Chekulayev V, et al. Formation of highly organized intracellular structure and energy metabolism in cardiac muscle cells during postnatal development of rat heart. Biochim Biophys Acta Bioener. (2014) 1837:1350–61. doi: 10.1016/j.bbabio.2014.03.015
26. Dhital KK, Iyer A, Connellan M, Chew HC, Gao L, Doyle A, et al. Adult heart transplantation with distant procurement and ex-vivo preservation of donor hearts after circulatory death: a case series. Lancet. (2015) 385:2585–91. doi: 10.1016/S0140-6736(15)60038-1
27. Holland BS, Copenhaver MD. An improved sequentially rejective bonferroni test procedure. Biometrics. (1987) 43:417. doi: 10.2307/2531823
28. Guglin M. How to increase the utilization of donor hearts? Heart Fail Rev. (2015) 20:95–105. doi: 10.1007/s10741-014-9434-y
29. Todd G, Baroldi G, Pieper G, Clayton F, Eliot R. Experimental catecholamine-induced myocardial necrosis. I. Morphology, quantification and regional distribution of acute contraction band lesions. J Mol Cell Cardiol. (1985) 17:317–38.
30. Todd G, Baroldi G, Pieper G, Clayton F, Eliot R. Experimental catecholamine-induced myocardial necrosis. II. Temporal development of isoproterenol-induced contraction band lesions correlated with ECG, hemodynamic and biochemical changes. J Mol Cell Cardiol. (1985) 17:647–56.
31. Grossman MI, Palm L, Becker GH, Moeller HC. Effect of lipemia and heparin on free fatty acid content of rat plasma. Exp Biol Med. (1954) 87:312–15. doi: 10.3181/00379727-87-21366
32. Oliver MF. Fatty acids and the risk of death during acute myocardial ischaemia. Clin Sci. (2015) 128:349–55. doi: 10.1042/CS20140404
33. Kramer AH, Doig CJ, Lauterio A, Di Sandro S, Ferla F, Zanierato M. Premortem heparin administration and location of withdrawal of life-sustaining interventions in DCD: lack of high-quality evidence precludes definitive conclusions. Transplantation. (2016) 100:e102–3. doi: 10.1097/TP.0000000000001419
34. Gao L, Doyle A, Villanueva J, Scheuer S, Chew HC, Hicks M, et al. Enhanced functional recovery of the heart donated after circulatory death determination with antemortem heparin. J Heart Lung Transpl. (2020) 39:607–9. doi: 10.1016/j.healun.2020.02.016
35. Krebs M, Stingl H, Nowotny P, Weghuber D, Bischof M, Waldhäusl W, et al. Prevention of in vitro lipolysis by tetrahydrolipstatin. Clin Chem. (2000) 46:950–4. doi: 10.1093/clinchem/46.7.950
36. Verges S, Chacaroun S, Godin-Ribuot D, Baillieul S. Hypoxic conditioning as a new therapeutic modality. Front Pediatrics. (2015) 3:58. doi: 10.3389/fped.2015.00058
37. Ryan JB, Hicks M, Cropper JR, Garlick SR, Kesteven SH, Wilson MK, et al. Functional evidence of reversible ischemic injury immediately after the sympathetic storm associated with experimental brain death. J Heart Lung Transpl. (2003) 22:922–8. doi: 10.1016/S1053-2498(02)00558-2
38. Floerchinger B, Oberhuber R, Tullius SG. Effects of brain death on organ quality and transplant outcome. Transpl Rev. (2012) 26:54–59. doi: 10.1016/j.trre.2011.10.001
39. Ohori K, Miura T, Tanno M, Miki T, Sato T, Ishikawa S, et al. Ser9 phosphorylation of mitochondrial GSK-3β is a primary mechanism of cardiomyocyte protection by erythropoietin against oxidant-induced apoptosis. Am J Physiol Heart Circ Physiol. (2008) 295:H2079–86. doi: 10.1152/ajpheart.00092.2008
40. Chong ZZ, Kang JQ, Maiese K. Erythropoietin is a novel vascular protectant through activation of Akt1 and mitochondrial modulation of cysteine proteases. Circulation. (2002) 106:2973–9. doi: 10.1161/01.CIR.0000039103.58920.1F
41. Cai Z, Manalo DJ, Wei G, Rodriguez ER, Fox-Talbot K, Lu H, et al. Hearts from rodents exposed to intermittent hypoxia or erythropoietin are protected against ischemia-reperfusion injury. Circulation. (2003) 108:79–85. doi: 10.1161/01.CIR.0000078635.89229.8A
42. Harrison DG, Bates JN. The nitrovasodilators. New ideas about old drugs. Circulation. (1993) 87:1461–7.
43. Watson AJ, Gao L, Sun L, Tsun J, Jabbour A, Ru Qiu M, et al. Enhanced preservation of the rat heart after prolonged hypothermic ischemia with erythropoietin-supplemented celsior solution. J Heart Lung Transpl. (2013) 32:633–40. doi: 10.1016/j.healun.2013.03.014
44. Iyer A, Gao L, Doyle A, Rao P, Jayewardene D, Wan B, et al. Increasing the tolerance of DCD hearts to warm ischemia by pharmacological postconditioning: increasing the tolerance of DCD hearts to warm ischemia. Am J Transpl. (2014) 14:1744–52. doi: 10.1111/ajt.12782
45. Klabunde RE. Organ blood flow. In: Taylor C, editor. Cardiovascular Physiology Concepts, 2nd edition. Philadelphia, PA: Lippincott Williams & Wilkins, Wolters Kluwer (2012), p. 156–157.
46. Hauerslev M, Mørk SR, Pryds K, Contractor H, Hansen J, Jespersen NR, et al. Influence of long-term treatment with glyceryl trinitrate on remote ischemic conditioning. Am J Physiol Heart Circ Physiol. (2018) 315:H150–8. doi: 10.1152/ajpheart.00114.2018
47. Burger D, Lei M, Geogheganmorphet N, Lu X, Xenocostas A, Feng Q. Erythropoietin protects cardiomyocytes from apoptosis via up-regulation of endothelial nitric oxide synthase. Cardiovasc Res. (2006) 72:51–9. doi: 10.1016/j.cardiores.2006.06.026
48. Heusch G. The coronary circulation as a target of cardioprotection. Circ Res. (2016) 118:1643–58. doi: 10.1161/CIRCRESAHA.116.308640
49. Friedrichs GS, Kilgore KS, Manley PJ, Gralinski MR, Lucchesi BR. Effects of heparin and N-acetyl heparin on ischemia/reperfusion-induced alterations in myocardial function in the rabbit isolated heart. Circ Res. (1994) 75:701–10. doi: 10.1161/01.RES.75.4.701
50. Kilgore KS, Tanhehco EJ, Naylor KB, Lucchesi BR. Ex vivo reversal of heparin-mediated cardioprotection by heparinase after ischemia and reperfusion. J Pharmcol Exp Ther. (1999) 290:1041–7.
51. Sutherland FJ, Hearse DJ. The isolated blood and perfusion fluid perfused heart. Pharmacol Res. (2000) 41:613–27. doi: 10.1006/phrs.1999.0653
Keywords: cardiac ischemia-reperfusion, experimental rat models, donation after circulatory death (DCD), ex-situ ischemia, in-situ ischemia, heart transplantation
Citation: Arnold M, Méndez-Carmona N, Wyss RK, Joachimbauer A, Casoni D, Carrel T and Longnus S (2021) Comparison of Experimental Rat Models in Donation After Circulatory Death (DCD): in-situ vs. ex-situ Ischemia. Front. Cardiovasc. Med. 7:596883. doi: 10.3389/fcvm.2020.596883
Received: 20 August 2020; Accepted: 14 December 2020;
Published: 13 January 2021.
Edited by:
Stephen Ralph Large, Papworth Hospital, United KingdomReviewed by:
Peter Simon Macdonald, St. Vincent's Hospital, AustraliaMohammed Quader, Virginia Commonwealth University, United States
Copyright © 2021 Arnold, Méndez-Carmona, Wyss, Joachimbauer, Casoni, Carrel and Longnus. This is an open-access article distributed under the terms of the Creative Commons Attribution License (CC BY). The use, distribution or reproduction in other forums is permitted, provided the original author(s) and the copyright owner(s) are credited and that the original publication in this journal is cited, in accordance with accepted academic practice. No use, distribution or reproduction is permitted which does not comply with these terms.
*Correspondence: Sarah Longnus, c2FyYWgubG9uZ251c0BpbnNlbC5jaA==
†These authors have contributed equally to this work