- Section of Cardiac Surgery, Department of Cardiac Sciences, Cumming School of Medicine, Libin Cardiovascular Institute of Alberta, University of Calgary, Calgary, AB, Canada
The epicardial surface of the heart is readily accessible during cardiac surgery and presents an opportunity for therapeutic intervention for cardiac repair and regeneration. As an important anatomic niche for endogenous mechanisms of repair, targeting the epicardium using decellularized extracellular matrix (ECM) bioscaffold therapy may provide the necessary environmental cues to promote functional recovery. Following ischemic injury to the heart caused by myocardial infarction (MI), epicardium derived progenitor cells (EPDCs) become activated and migrate to the site of injury. EPDC differentiation has been shown to contribute to endothelial cell, cardiac fibroblast, cardiomyocyte, and vascular smooth muscle cell populations. Post-MI, it is largely the activation of cardiac fibroblasts and the resultant dysregulation of ECM turnover which leads to maladaptive structural cardiac remodeling and loss of cardiac function. Decellularized ECM bioscaffolds not only provide structural support, but have also been shown to act as a bioactive reservoir for growth factors, cytokines, and matricellular proteins capable of attenuating maladaptive cardiac remodeling. Targeting the epicardium post-MI using decellularized ECM bioscaffold therapy may provide the necessary bioinductive cues to promote differentiation toward a pro-regenerative phenotype and attenuate cardiac fibroblast activation. There is an opportunity to leverage the clinical benefits of this innovative technology with an aim to improve the prognosis of patients suffering from progressive heart failure. An enhanced understanding of the utility of decellularized ECM bioscaffolds in epicardial repair will facilitate their growth and transition into clinical practice. This review will provide a summary of decellularized ECM bioscaffolds being developed for epicardial infarct repair in coronary artery bypass graft (CABG) surgery.
Introduction to Heart Failure
Heart failure is a chronic and progressive condition characterized by maladaptive structural cardiac remodeling and poor cardiac pump function. The most common cause of heart failure is damage to the cardiac muscle caused by ischemic injury, otherwise known as myocardial infarction (MI) (1). There are an increasing number of individuals living with heart failure, with 960,000 new cases reported each year in the US alone (2). As a result, an estimated >8 million individuals will be living with heart failure in the US by 2030 (3). Surgical revascularization remains the primary treatment modality for patients who have suffered from an MI. However, nearly one in every five heart failure patients is readmitted for heart failure or other related causes within 30 days (1). Therefore, heart failure is often referred to as a “revolving door condition” due to high rates of readmission. Given its increasing prevalence and high rate of readmission, it is necessary to improve our understanding of the surgical management of heart failure. Extracellular matrix (ECM) bioscaffolds may be the key to unlocking the potential of the epicardial surface of the heart with the ultimate goal of driving endogenous mechanisms of repair and attenuating progressive heart failure following ischemic injury.
The Current Surgical Management of Heart Failure
According to current guidelines, the primary objective in the surgical management of MI is to restore blood flow to the infarct region in order to preserve myocardial viability and alleviate symptoms (4, 5). Revascularization may be achieved by coronary artery bypass graft (CABG) surgery or percutaneous coronary intervention (PCI), and has been shown to improve survival in patients (4–8). A recent meta-analysis including twenty-one studies and 16,191 patients found revascularization by CABG or PCI was beneficial compared to medical treatment alone in patients suffering from ischemic heart disease and reduced left ventricular ejection fraction (LVEF) (9). The survival benefits of surgical revascularization are clear, and the completeness of revascularization is integral to preserving myocardial viability (4). Despite surgical intervention, studies have documented 12 and 22% readmission rates in patients who have undergone CABG or PCI, respectively, due to heart failure (6, 10).
For patients who progress to end-stage clinical heart failure, cardiac transplantation remains the gold standard treatment modality (11, 12). However, limited donor supply and an increasing number of eligible patients for cardiac transplants have driven the need for innovative alternative strategies. Mechanical circulatory support (MCS), specifically the left ventricular assist device (LVAD), has vastly improved since the seminal work of Dr. Michael E. DeBakey, Dr. Denton A. Cooley, and others (13–16). MCS may be utilized as a bridge to recovery, bridge to transplantation, or as a destination therapy in patients who are ineligible for cardiac transplantation (17, 18). INTERMACS (Interagency Registry for Mechanically Assisted Circulatory Support) includes >15,000 patients from 158 hospitals; it reports a 1-year survival rate of 80% and 2-year survival rate of 70% in patients receiving a continuous-flow device (17, 19). Importantly, despite the improvements made in MSC technology, a number of complications are still associated with its use (19–22). Adverse events reported at 2-year follow up of 133 patients treated using a continuous flow LVAD include, bleeding requiring blood transfusion (81%), cardiac arrhythmia (56%), right-sided heart failure (20%), LVAD-related infection (35%), stroke (18%), LVAD-thrombosis (4%), and pump replacement (9%) (23). Therefore, an opportunity exists to better understand and address the underlying cellular and molecular causes of heart failure in order to improve the prognosis of patients with heart failure.
Taking a Stem Cell-Based Approach
An array of stem cell-based approaches have emerged in the past two decades with the aim of restoring myocardial function and preventing the progression of heart failure. Despite the numerous clinical trials conducted and their important contribution to our current understanding of the treatment of heart failure following MI, an efficacious stem cell-based therapy with clinically relevant outcomes has yet to emerge (24–26). Clinical trial data regarding the use of a stem cell-based approach in patients suffering from acute myocardial infarction or ischemic cardiomyopathy remains variable and inconclusive (24, 25, 27).
In the case of acute myocardial infarction, earlier trials such as BOOST (BOne marrOw transfer to enhance ST-elevation infarct regeneration) and REPAIR-AMI (Reinfusion of Enriched Progenitor Cells and Infarct Remodeling in Acute Myocardial Infarction) showed improved LVEF in patients treated with bone marrow mononuclear cells (BM-MNCs) (28–30). However, these improvements were not replicated in future studies using BM-MNCs, including but not limited to, BOOST-2 (BOne marrOw transfer to enhance ST-elevation infarct regeneration-2), LATE-TIME (Late Timing in Myocardial Infarction Evaluation), and SWISS-AMI (SWiss multicenter Intracoronary Stem cells Study in Acute Myocardial Infarction) (24, 31–33).
Similarly, in the case of ischemic cardiomyopathy, patients with advanced heart failure who received BM-MNC therapy displayed a 9% improvement in LVEF at 4-months follow-up compared to baseline (34). However, the FOCUS-CCTRN (First Mononuclear Cells injected in the United States conducted by the CCTRN) trial showed a modest 2.7% LVEF improvement in patients treated with BM-MNC compared to placebo, alongside no significant improvement in infarct size (35). Furthermore, CHART-1 (Congestive Heart Failure Cardiopoietic Regenerative Therapy) found no significant difference amongst advanced heart failure patients (n = 157) receiving cardiopoietic cell therapy compared to sham control (n = 158) at 39-weeks follow-up (24, 36).
In addition to the variable clinical trial results discussed, poor stem cell engraftment at the site of delivery and the inherent conflict of introducing stem cells to an injured and hostile cardiac environment present significant hurdles to directing optimal cell function and tissue recovery (37, 38). The recent controversy surrounding c-kit+ cardiac stem cells and interruption of the CONCERT-HF (Combination of Mesenchymal and C-kit+ Cardiac Stem Cells as Regenerative Therapy for Heart Failure) trial has significantly contributed to uncertainty regarding the clinical efficacy of this approach (39–45).
The Paracrine Hypothesis
Despite the challenges associated with a stem cell-based approach, it has become clear that stem cells largely exert their effects in a paracrine fashion (41, 46–53). The ability of bone marrow-derived cells to produce a potent angiogenic growth factor response, enhance endothelial cell proliferation, and improve perfusion and function in models of ischemic injury has been described (49, 53–55). Specifically, autologous bone marrow cells are capable of producing a vascular endothelial growth factor (VEGF)-dependent response that drives angiogenesis and improves perfusion in a porcine model of MI (54). Moreover, human mesenchymal stem cell conditioned media (MSC-CM) has been shown to reduce MI size, enhance capillary density, and improve overall cardiac function compared to control media using a porcine model of MI (56, 57). Overall, it is the paracrine factors produced that play a fundamental role in mediating the effects of stem cell therapy.
The question, therefore, becomes “is the delivery of cells necessary for a therapeutic effect?” Given the challenges associated with a stem cell-based approach, our research group alongside many others have directed their attention toward modulating the local cardiac microenvironment and paracrine response, without the administration of stem cells, in order to direct endogenous mechanisms of cardiac repair (48, 51, 57–62). In particular, our research group has shown the benefits of using acellular bioactive extracellular matrix (ECM) scaffolds for epicardial infarct repair (63–66). By providing an optimal ECM microenvironment with the necessary paracrine growth factors, the aim is to limit infarct expansion by attenuating cardiac fibrosis and to promote vasculogenesis in order to improve blood flow to the infarcted myocardium (63, 65, 67) (Figure 1).
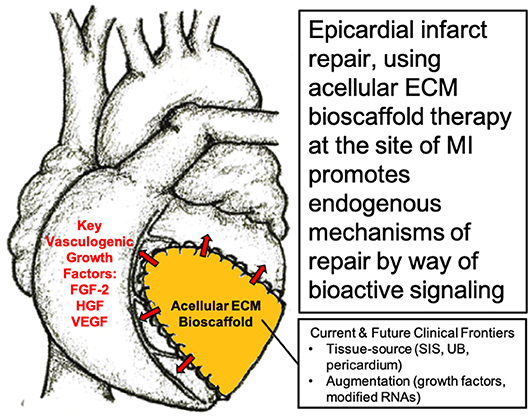
Figure 1. Surgical implantation of acellular extracellular matrix (ECM) bioscaffold on the epicardial surface of the heart promotes endogenous mechanisms of repair by way of bioactive paracrine signaling. Image, in part, provided courtesy of Dr. Holly Mewhort, Section of Cardiac Surgery, Department of Cardiac Sciences, Cumming School of Medicine, Libin Cardiovascular Institute of Alberta, University of Calgary, Calgary, Alberta, Canada.
Targeting the Epicardium in Cardiac Surgery
A unique opportunity exists to enhance endogenous repair mechanisms of the heart at its epicardial surface. In the case of routine cardiac surgery, surgeons gain access to the heart by way of sternotomy followed by cardiotomy, at which point they are presented with the epicardial surface of the heart. The epicardium is a promising anatomic niche that is involved in early cardiac development, the production and regulation of ECM components, paracrine signaling, and response to ischemic injury (68).
This thin outermost mesothelial layer of the heart contributes to normal cardiac development as it gives rise to multipotent cardiac progenitor cells, called epicardium derived progenitor cells (EPDCs) (68, 69). EPDCs have been found to differentiate to coronary vascular smooth muscle cells and cardiac fibroblasts by way of epithelial to mesenchymal transition (EMT) (70–73) (Figure 2). Studies have also reported EPDC differentiation to endothelial cell and cardiomyocyte populations (74–77). However, the contribution of EPDCs to endothelial cell and cardiomyocyte populations remains highly debatable and further lineage tracing is warranted to ascertain the extent of this contribution (71, 73, 77–82) (Figure 2).
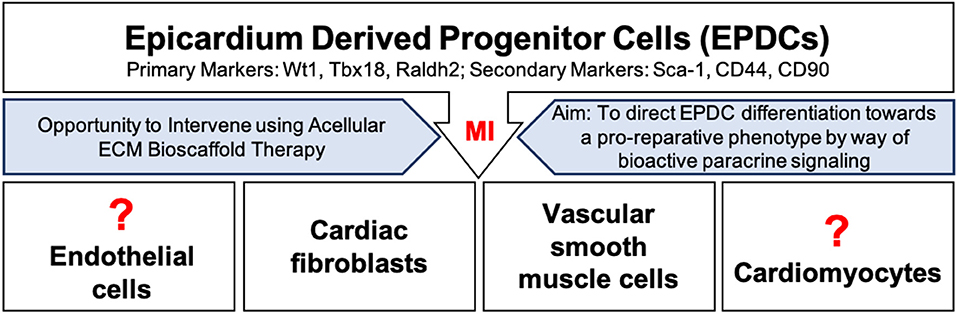
Figure 2. Epicardium derived progenitor cell (EPDC) activation, mobilization, and differentiation following myocardial infarction. EPDCs may differentiate into cardiac fibroblasts, vascular smooth muscle cells, or possibly into endothelial cells or cardiomyocytes. Intervention using acellular ECM bioscaffold therapy may be used to direct EDPCs toward a pro-reparative, vasculogenic fate.
During early cardiac development, the epicardium dictates myocardial maturation and compaction, and the formation of the coronary vasculature and Purkinje fibers (68, 83–85). Beyond its contribution to various cell populations, the epicardium has been shown to be a key player involved in paracrine signaling and the modulation of ECM components (82, 86–90). This makes the epicardium an ideal candidate that may be targeted to manipulate ECM remodeling and promote endogenous repair and regeneration of the adult human heart.
Ischemic Injury Leads to ECM Remodeling
Normally, the epicardium is quiescent in the healthy adult human heart, yet it may hold great regenerative capacity (68, 83, 91, 92). Following an MI, EPDCs become activated and migrate to the site of injury, where they have been shown to largely differentiate into vascular smooth muscle cells or fibroblasts (70, 92, 93) (Figure 2). The expression of markers including, Wilms tumor protein (Wt1), T-box transcription factor 18 (Tbx18), and retinaldehyde dehydrogenase 2 (Raldh2), has been reported in activated EPDC populations (69, 82, 92). Recent findings have further highlighted the heterogeneity within EPDC populations following an MI based on the differential expression of stem-cell antigen 1 (Sca-1), CD44, and CD90; these subpopulations may present clinically relevant targets (94). Overall, activation of the epicardium has been shown to play an important role in supporting the development of new vasculature by way of a robust paracrine response (79, 92). EPDC derived conditioned media has been reported to induce functional recovery in a mouse model of MI by way of a robust fibroblast growth factor-2 (FGF-2) and VEGF mediated angiogenic response (92). Clearly, an opportunity exists to direct EPDCs toward a pro-reparative or pro-vasculogenic fate, and away from a pro-fibrotic fate. As mentioned above, the completeness of revascularization is critical to the preservation of myocardial viability following an MI (4). Therefore, the aim is to promote vasculogenesis in order to improve blood flow to the infarcted myocardium, and to limit infarct scar expansion by attenuating the activity of cardiac fibroblasts.
Cardiac fibroblasts play an essential role in normal heart function, not only structurally and mechanically, but with regards to the biochemical and electrical properties of the cardiac environment as well (95–99). In the event of an ischemic injury and the resulting disruption of the local microenvironment of the infarcted myocardium, these fibroblasts become activated. Activated fibroblasts, known as myofibroblasts, are the key mediators of ECM remodeling (96, 98, 100, 101). Fibrotic remodeling of the infarcted myocardium is exacerbated by the migration and activation of additional cardiac fibroblasts at the site of injury. Of note, the epicardium is a significant source of these migratory cardiac fibroblasts (79, 82, 92, 93, 102–104). Myofibroblast activity at the site of MI leads to dysregulation of ECM homoeostasis, resulting in the deposition of a collagenous scar (98, 104, 105). Initially, this response is crucial in preventing ventricular free wall rupture at the site of an MI. However, persistent myofibroblast activation when left unchecked leads to infarct scar expansion, diastolic, and systolic dysfunction due to structural cardiac remodeling, and eventually end-stage clinical heart failure (97, 98, 105–108).
Therefore, targeting the epicardium post-MI by providing the necessary bioinductive cues capable of promoting a pro-reparative phenotype rather than a pro-fibrotic phenotype is compelling. Our research group and others believes that acellular ECM bioscaffolds offer an ideal approach by which the post-MI cardiac environment may be directed toward a pro-reparative phenotype by way of bioactive signaling (Figures 1, 2).
Bio-Engineered Materials for Epicardial Infarct Repair
Collagen is commonly studied for ECM bioscaffold-based infarct repair as it is the primary component of the cardiac ECM (109–111). Acellular type I collagen cardiac bioscaffold has been shown to preserve contractility, reduce cardiac fibrosis, and attenuate LV remodeling using a murine model of MI (112, 113). Additionally, acellular type I collagen cardiac bioscaffold therapy has been reported to promote a pro-vasculogenic response, which is accompanied by increased vessel density in the injured heart (112–115) Other natural bioscaffolds, such as fibrin, gelatin, Matrigel, alginate, and chitosan-based scaffolds, have also been investigated for infarct repair and regeneration (111, 116–119). Acellular fibrin-based scaffolds leverage the blood clotting cascade to polymerize in situ, and have been reported to preserve cardiac function and improve neovascularization in a rat model of MI (111, 120, 121). Despite the potential of these acellular strategies, there are challenges associated with the use of natural bioscaffolds, such as rapid degradation and poor mechanical performance (111, 122).
Promising results have also been found using synthetic scaffold solutions, which typically include the use of polylactic acid (PLA), polyglycolic acid (PGA), poly-ε-caprolactone (PCL), polyester urethane urea (PEUU), polytetrafluoroethylene (PTFE), or varying combinations of the aforementioned materials (110, 122, 123). For example, polyester urethane urea (PEUU) scaffold implantation in a rat model of MI is capable of improving overall contractile function and cardiac remodeling (124). While the mechanical properties of synthetic scaffold solutions are highly tunable, they lack the biological complexity required to target the epicardial surface of the heart by way of paracrine signaling and bioactive factors.
As such, the development of decellularized tissue-derived ECM bioscaffolds remains a focal point within the field. These acellular ECM bioscaffolds facilitate directing endogenous mechanisms of repair and regeneration at the site of ischemic injury by way of bioactive paracrine signaling (63–65, 109, 125–128) (Figure 1). Acellular ECM bioscaffolds retain the native ECM architecture and composition, including a variety of embedded growth factors, of the tissue from which they were derived (125, 126, 129). These complex bioscaffolds may be exploited to provide an optimal microenvironment capable of enhancing blood flow and attenuating cardiac myofibroblast activity at the site of an MI. Of the tissue-derived ECM bioscaffolds, acellular porcine-derived small intestinal submucosa (SIS) ECM is best characterized in the literature with regards to epicardial infarct repair. We have previously described the collection of acellular ECM bioscaffolds available for a variety of indications in cardiac surgery (98).
The small intestine is a highly vascularized organ and therefore the composition and structure of SIS-derived ECM bioscaffold is proposed to be highly conducive to revascularization itself (109, 126). Specifically, 90% of SIS-ECM bioscaffold is type I collagen, fibronectin, laminin, and glycosaminoglycans (GAGs) ECM components (109, 130). The role of fibronectin and laminin in endothelial cell adhesion and the maintenance of vascular structures, respectively, has been characterized (109, 131–133). Additionally, GAGs play an important role in binding the growth factors and cytokines within the ECM and therefore present a possible target for modifying tissue-derived ECM bioscaffolds with signaling factors (109, 134). Finally, SIS-ECM itself has been shown to naturally contain essential growth factors, both bound by GAGs and embedded within the ECM itself, including fibroblast growth factor-2 (FGF-2), VEGF, and hepatocyte growth factor (HGF), which play key roles in vasculogenesis (109, 135).
In the context of epicardial infarct repair, our research group has highlighted the promise of acellular SIS-ECM bioscaffold, CorMatrix® ECM (CorMatrix Cardiovascular Inc., USA) (63–66). We have shown that the interaction of human cardiac fibroblasts with CorMatrix® ECM results in a robust fibroblast growth factor-2 (FGF-2) dependent cell-mediated paracrine response capable of stimulating new blood vessel assembly (65). This is recapitulated in vivo, using a rat MI model: animals treated via surgical implantation of CorMatrix® ECM post-MI compared to animals treated with sham or inactivated CorMatrix® ECM displayed an FGF-2-dependent increase in vascularity, reduced LV dilatation, improved ejection fraction, and improved contractility (65). Further studies using a large pre-clinical porcine ischemia-reperfusion model have similarly demonstrated that surgical implantation of CorMatrix® ECM improves vascularity and functional recovery of the infarct region (63, 64) (Figure 3). Other groups have assessed SIS-ECM bioscaffold in surgical reconstruction of septal defects, vascular or outflow tract augmentation, and valve reconstruction, and have yielded positive results (136). However, long-term patient follow up is required to truly understand the impact of this intervention. Notably, our research group is characterizing the clinical use of commercially-available CorMatrix® ECM in an on-going first-in-human phase I clinical trial (NCT02887768) for epicardial infarct repair at the time of surgical revascularization (CABG surgery).
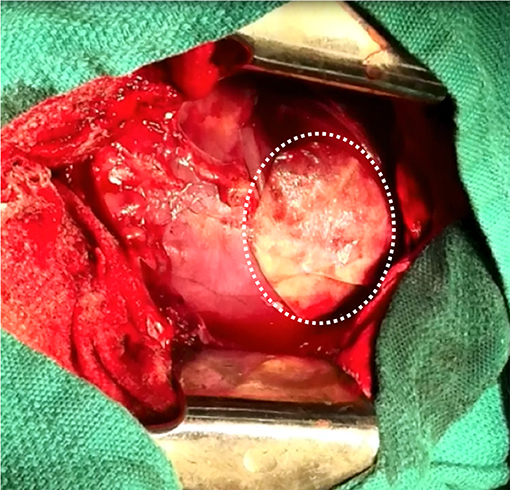
Figure 3. Surgical implantation of porcine-derived acellular small intestine submucosa (SIS) extracellular matrix (ECM) bioscaffold (CorMatrix® ECM, CorMatrix Cardiovascular Inc., USA) over the site of ischemia-reperfusion injury on the anterior wall of the left ventricle, in a porcine model. Image provided courtesy of the Fedak Research Group (Dr. Holly Mewhort and Jeannine Turnbull) Campbell Family Cardiac Translational Laboratory, Libin Cardiovascular Institute of Alberta, University of Calgary, Calgary, Alberta, Canada.
Beyond SIS-ECM bioscaffold therapy for epicardial infarct repair, acellular ECM bioscaffolds derived from other tissue sources, such as the urinary bladder (UB), amniotic membrane (AM), and cardiac tissue have been investigated (137–140). Given the fact that ECM bioscaffolds are a function of the physiological requirements of the tissue from which they are derived, the tissue source will influence each bioscaffold's ability to direct cardiac repair. UB-ECM bioscaffold has been reported to outperform PTFE synthetic scaffold, and displays favorable tissue integration and replacement in a porcine MI model (139). More recently, an acellular pericardium-derived ECM bioscaffold was shown to support neovascularization and neoinnervation, alongside improved LVEF, cardiac output, and reduced infarct size in a porcine MI model at 30-day follow-up (137). Future work should continue to investigate acellular ECM bioscaffolds derived from various tissue sources in the context of epicardial infarct repair.
Additionally, augmentation of the aforementioned bioscaffolds with additional growth factors and/or modified RNAs, may also play an important role in modulating the local paracrine environment and enhancing their therapeutic effect. While the specific details and challenges are beyond the scope of this review, modified RNAs may be utilized to promote angiogenesis following an MI (141–143). Intramyocardial injection of VEGF-A modified RNA has been reported to enhance Wt1+-EPDC to endothelial cell differentiation and to promote functional vessel formation in a mouse model of MI (143). Similarly, our research group has shown that enhancement of SIS-ECM with additional FGF-2 further improves ECM homeostasis and cardiac function in a rat model of MI (63, 66). Overall, acellular tissue-derived ECM bioscaffolds are a promising therapeutic strategy for the modulation of cardiac repair at the site of an MI. The important role that bioactive paracrine signaling plays in directing endogenous mechanisms of repair at the site of an MI should remain a focal point moving forward.
Conclusion
A unique opportunity exists to augment surgical revascularization via CABG surgery using acellular ECM bioscaffold therapy. The epicardial surface is readily accessible in open heart surgery. As it is an anatomic niche responsible for normal cardiac development and paracrine signaling, which becomes activated in response to ischemic injury, we can leverage the epicardium to direct endogenous repair at the site of an MI. Acellular ECM bioscaffold therapy has been shown to improve vascularization and attenuate cardiac fibroblast-mediated ventricular remodeling following an MI by way of its bioactive signaling. By providing an optimal ECM bioscaffold signaling environment the goal is to shift the damaged cardiac tissue toward a pro-reparative phenotype, and away from a pro-fibrotic phenotype, and to improve overall revascularization of the tissue. Overall, by targeting the underlying cellular and molecular causes of heart failure using acellular ECM bioscaffold therapy, this innovative strategy may be able to significantly improve the prognosis of patients who have suffered from an MI.
Author Contributions
SP, AF, and PF designed, drafted, and revised the manuscript.
Funding
This research was funded by the Heart and Stroke Foundation of Canada to PF.
Conflict of Interest Statement
The authors declare that the research was conducted in the absence of any commercial or financial relationships that could be construed as a potential conflict of interest.
References
1. Heart and Stroke Foundation. The Burden of Heart Failure: 2016 Report on the Health of Canadians. (2016). Available online at: https://www.heartandstroke.ca/-/media/pdf-files/canada/2017-heart-month/heartandstroke-reportonhealth-2016.ashx?la=en (accessed November 25, 2017).
2. Benjamin EJ, Blaha MJ, Chiuve SE, Cushman M, Das SR, Deo R, et al. Heart disease and stroke statistics-−2017 update: a report from the American heart association. Circulation. (2017) 135:e146–603. doi: 10.1161/CIR.0000000000000485
3. Benjamin EJ, Muntner P, Alonso A, Bittencourt MS, Callaway CW, Carson AP, et al. Heart disease and stroke statistics-−2019 update: a report from the american heart association. Circulation. (2019) 139:e56–28. doi: 10.1161/CIR.0000000000000659
4. Neumann FJ, Sousa-Uva M, Ahlsson A, Alfonso F, Banning AP, Benedetto U, et al. 2018 ESC/EACTS Guidelines on myocardial revascularization. Eur Heart J. (2018) 76:1585–664. doi: 10.5603/KP.2018.0228
5. Yancy CW, Jessup M, Bozkurt B, Butler J, Casey DE, Drazner MH, et al. 2013 ACCF/AHA guideline for the management of heart failure. Circulation. (2013) 128:e240–327. doi: 10.1161/CIR.0b013e31829e8776
6. Marui A, Kimura T, Nishiwaki N, Komiya T, Hanyu M, Shiomi H, et al. Three-year outcomes after percutaneous coronary intervention and coronary artery bypass grafting in patients with heart failure: from the CREDO-Kyoto percutaneous coronary intervention/coronary artery bypass graft registry cohort-2 The CREDO-Kyoto PCI/CAB. Eur J Cardio-thoracic Surg. (2014) 47:316–21. doi: 10.1093/ejcts/ezu131
7. Velazquez EJ, Lee KL, Jones RH, Al-Khalidi HR, Hill JA, Panza JA, et al. Coronary-artery bypass surgery in patients with ischemic cardiomyopathy. N Engl J Med. (2016) 374:1511–20. doi: 10.1056/NEJMoa1602001
8. Yusuf S, Zucker D, Passamani E, Peduzzi P, Takaro T, Fisher L, et al. Effect of coronary artery bypass graft surgery on survival: overview of 10-year results from randomised trials by the Coronary Artery Bypass Graft Surgery Trialists Collaboration. Lancet. (1994) 344:563–70. doi: 10.1016/S0140-6736(94)91963-1
9. Wolff G, Dimitroulis D, Andreotti F, Kołodziejczak M, Jung C, Scicchitano P, et al. Survival benefits of invasive versus conservative strategies in heart failure in patients with reduced ejection fraction and coronary artery disease. Circ Hear Fail. (2017) 10:e003255. doi: 10.1161/CIRCHEARTFAILURE.116.003255
10. Hannan EL, Zhong Y, Lahey SJ, Culliford AT, Gold JP, Smith CR, et al. 30-day readmissions after coronary artery bypass graft surgery in New York State. JACC Cardiovasc Interv. (2011) 4:569–76. doi: 10.1016/j.jcin.2011.01.010
11. Kittleson MM, Kobashigawa JA. Cardiac transplantation. JACC Hear Fail. (2017) 5:857–68. doi: 10.1016/j.jchf.2017.08.021
12. Mehra MR, Canter CE, Hannan MM, Semigran MJ, Uber PA, Baran DA, et al. The 2016 international society for heart lung transplantation listing criteria for heart transplantation: a 10-year update. J Hear Lung Transplant. (2016) 35:1–23. doi: 10.1016/j.healun.2015.10.023
14. Cooley DA, Liotta D, Hallman GL, Bloodwell RD, Leachman RD, Milam JD. Orthotopic cardiac prosthesis for two-staged cardiac replacement. Am J Cardiol. (1969) 24:723–30. doi: 10.1016/0002-9149(69)90460-3
15. DeBakey ME. Development of mechanical heart devices. Ann Thorac Surg. (2005) 79:S2228–31. doi: 10.1016/j.athoracsur.2005.03.029
16. Liotta D, Hall CW, Henly WS, Cooley DA, Crawford ES, DeBakey ME. Prolonged assisted circulation during and after cardiac or aortic surgery: prolonged partial left ventricular bypass by means of intracorporeal circulation. Am J Cardiol. (1963) 12:399–405. doi: 10.1016/0002-9149(63)90235-2
17. Cook JL, Colvin M, Francis GS, Grady KL, Hoffman TM, Jessup M, et al. Recommendations for the use of mechanical circulatory support: ambulatory and community patient care: a scientific statement from the american heart association. Circulation. (2017) 135:e1145–58. doi: 10.1161/CIR.0000000000000507
18. Wilson SR, Givertz MM, Stewart GC, Mudge GH. Ventricular assist devices. J Am Coll Cardiol. (2009) 54:1647–59. doi: 10.1016/j.jacc.2009.06.035
19. Kirklin JK, Naftel DC, Pagani FD, Kormos RL, Stevenson LW, Blume ED, et al. Seventh INTERMACS annual report: 15,000 patients and counting. J Hear Lung Transplant. (2015) 34:1495–504. doi: 10.1016/j.healun.2015.10.003
20. Dang NC, Topkara VK, Mercando M, Kay J, Kruger KH, Aboodi MS, et al. Right heart failure after left ventricular assist device implantation in patients with chronic congestive heart failure. J Hear Lung Transplant. (2006) 25:1–6. doi: 10.1016/j.healun.2005.07.008
21. Lima B, Bansal A, Abraham J, Rich JD, Lee SS, Soleimani B, et al. Controversies and challenges of ventricular assist device therapy. Am J Cardiol. (2018) 121:1219–24. doi: 10.1016/j.amjcard.2018.01.034
22. Nguyen AB, Uriel N, Adatya S. New challenges in the treatment of patients with left ventricular support: LVAD thrombosis. Curr Heart Fail Rep. (2016) 13:302–9. doi: 10.1007/s11897-016-0310-z
23. Slaughter MS, Rogers JG, Milano CA, Russell SD, Conte JV, Feldman D, et al. Advanced heart failure treated with continuous-flow left ventricular assist device. N Engl J Med. (2009) 361:2241–51. doi: 10.1056/NEJMoa0909938
24. Banerjee MN, Bolli R, Hare JM. Clinical studies of cell therapy in cardiovascular medicine. Circ Res. (2018) 123:266–87. doi: 10.1161/CIRCRESAHA.118.311217
25. Fisher SA, Zhang H, Doree C, Mathur A, Martin-Rendon E. Stem cell treatment for acute myocardial infarction. Cochrane Database Syst Rev. (2015) 30:CD006536. doi: 10.1002/14651858.CD006536.pub4
26. Mathur A, Fernández-Avilés F, Dimmeler S, Hauskeller C, Janssens S, Menasche P, et al. The consensus of the Task Force of the European Society of Cardiology concerning the clinical investigation of the use of autologous adult stem cells for the treatment of acute myocardial infarction and heart failure: update 2016. Eur Heart J. (2017) 38:2930–5. doi: 10.1093/eurheartj/ehw640
27. Gyöngyösi M, Wojakowski W, Lemarchand P, Lunde K, Tendera M, Bartunek J, et al. Meta-analysis of cell-based cardiac studies (ACCRUE) in patients with acute myocardial infarction based on individual patient data. Circ Res. (2015) 116:1346–60. doi: 10.1161/CIRCRESAHA.116.304346
28. Schachinger V, Erbs S, Elsasser A, Haberbosch W, Hambrecht R, Holschermann H, et al. Improved clinical outcome after intracoronary administration of bone-marrow-derived progenitor cells in acute myocardial infarction: final 1-year results of the REPAIR-AMI trial. Eur Heart J. (2006) 27:2775–83. doi: 10.1093/eurheartj/ehl388
29. Schächinger V, Erbs S, Elsässer A, Haberbosch W, Hambrecht R, Hölschermann H, et al. Intracoronary bone marrow–derived progenitor cells in acute myocardial infarction. N Engl J Med. (2006) 355:1210–21. doi: 10.1056/NEJMoa060186
30. Wollert KC, Meyer GP, Lotz J, Ringes Lichtenberg S, Lippolt P, Breidenbach C, et al. Intracoronary autologous bone-marrow cell transfer after myocardial infarction: the BOOST randomised controlled clinical trial. Lancet. (2004) 364:141–8. doi: 10.1016/S0140-6736(04)16626-9
31. Sürder D, Manka R, Moccetti T, Lo Cicero V, Emmert MY, Klersy C, et al. Effect of bone marrow–derived mononuclear cell treatment, early or late after acute myocardial infarction. Circ Res. (2016) 119:481–90. doi: 10.1161/CIRCRESAHA.116.308639
32. Traverse JH, Henry TD, Ellis SG, Pepine CJ, Willerson JT, Zhao DXM, et al. Effect of intracoronary delivery of autologous bone marrow mononuclear cells 2 to 3 weeks following acute myocardial infarction on left ventricular function. JAMA. (2011) 306:2110. doi: 10.1001/jama.2011.1670
33. Wollert KC, Meyer GP, Müller-Ehmsen J, Tschöpe C, Bonarjee V, Larsen AI, et al. Intracoronary autologous bone marrow cell transfer after myocardial infarction: the BOOST-2 randomised placebo-controlled clinical trial. Eur Heart J. (2017) 38:2936–43. doi: 10.1093/eurheartj/ehx188
34. Perin EC, Dohmann HFR, Borojevic R, Silva SA, Sousa ALS, Mesquita CT, et al. Transendocardial, autologous bone marrow cell transplantation for severe, chronic ischemic heart failure. Circulation. (2003) 107:2294–302. doi: 10.1161/01.CIR.0000070596.30552.8B
35. Perin EC, Willerson JT, Pepine CJ, Henry TD, Ellis SG, Zhao DXM, et al. Effect of transendocardial delivery of autologous bone marrow mononuclear cells on functional capacity, left ventricular function, and perfusion in chronic heart failure. JAMA. (2012) 307:1717–26. doi: 10.1001/jama.2012.418
36. Bartunek J, Terzic A, Davison BA, Filippatos GS, Radovanovic S, Beleslin B, et al. Cardiopoietic cell therapy for advanced ischemic heart failure: results at 39 weeks of the prospective, randomized, double blind, sham-controlled CHART-1 clinical trial. Eur Heart J. (2016) 38:ehw543. doi: 10.1093/eurheartj/ehw543
37. Kanda P, Davis DR. Cellular mechanisms underlying cardiac engraftment of stem cells. Expert Opin Biol Ther. (2017) 17:1127–43. doi: 10.1080/14712598.2017.1346080
38. Li X, Tamama K, Xie X, Guan J. Improving cell engraftment in cardiac stem cell therapy. Stem Cells Int. (2016) 2016:1–11. doi: 10.1155/2016/2470351
39. Balsam LB, Wagers AJ, Christensen JL, Kofidis T, Weissman IL, Robbins RC. Haematopoietic stem cells adopt mature haematopoietic fates in ischaemic myocardium. Nature. (2004) 428:668–73. doi: 10.1038/nature02460
40. Bolli R, Hare JM, March KL, Pepine CJ, Willerson JT, Perin EC, et al. Rationale and design of the CONCERT-HF trial (Combination of mesenchymal and c-kit cardiac stem cells as regenerative therapy for heart failure). Circ Res. (2018) 122:1703–15. doi: 10.1161/CIRCRESAHA.118.312978
41. Chien KR, Frisén J, Fritsche-Danielson R, Melton DA, Murry CE, Weissman IL. Regenerating the field of cardiovascular cell therapy. Nat Biotechnol. (2019) 37:232–7. doi: 10.1038/s41587-019-0042-1
42. Curfman G. Stem Cell Therapy for Heart Failure. JAMA. (2019) 321:1186. doi: 10.1001/jama.2019.2617
43. Davis DR. Cardiac stem cells in the post-Anversa era. Eur Heart J. (2019) 40:1039–41. doi: 10.1093/eurheartj/ehz098
44. Murry CE, Soonpaa MH, Reinecke H, Nakajima H, Nakajima HO, Rubart M, et al. Haematopoietic stem cells do not transdifferentiate into cardiac myocytes in myocardial infarcts. Nature. (2004) 428:664–8. doi: 10.1038/nature02446
45. Sultana N, Zhang L, Yan J, Chen J, Cai W, Razzaque S, et al. Resident c-kit+ cells in the heart are not cardiac stem cells. Nat Commun. (2015) 6:8701. doi: 10.1038/ncomms9701
46. Braunwald E. Cell-based therapy in cardiac regeneration. Circ Res. (2018) 123:132–7. doi: 10.1161/CIRCRESAHA.118.313484
47. D'amore A, Yoshizumi T, Luketich SK, Wolf MT, Gu X, Cammarata M, et al. Bi-layered polyurethane e Extracellular matrix cardiac patch improves ischemic ventricular wall remodeling in a rat model. Biomaterials. (2016) 107:1–14. doi: 10.1016/j.biomaterials.2016.07.039
48. Fedak PWM. Paracrine effects of cell transplantation: modifying ventricular remodeling in the failing heart. Semin Thorac Cardiovasc Surg. (2008) 20:87–93. doi: 10.1053/j.semtcvs.2008.04.001
49. Gnecchi M, He H, Liang OD, Melo LG, Morello F, Mu H, et al. Paracrine action accounts for marked protection of ischemic heart by Akt-modified mesenchymal stem cells. Nat Med. (2005) 11:367–8. doi: 10.1038/nm0405-367
50. Gnecchi M, Zhang Z, Ni A, Dzau VJ. Paracrine mechanisms in adult stem cell signaling and therapy. Circ Res. (2008) 103:1204–19. doi: 10.1161/CIRCRESAHA.108.176826
51. Hodgkinson CP, Bareja A, Gomez JA, Dzau VJ. Emerging concepts in paracrine mechanisms in regenerative cardiovascular medicine and biology. Circ Res. (2016) 118:95–107. doi: 10.1161/CIRCRESAHA.115.305373
52. Malliaras K, Marban E. Cardiac cell therapy: where we've been, where we are, and where we should be headed. Br Med Bull. (2011) 98:161–85. doi: 10.1093/bmb/ldr018
53. Yoshioka T, Ageyama N, Shibata H, Yasu T, Misawa Y, Takeuchi K, et al. Repair of infarcted myocardium mediated by transplanted bone marrow-derived CD34+ stem cells in a nonhuman primate model. Stem Cells. (2005) 23:355–64. doi: 10.1634/stemcells.2004-0200
54. Fuchs S, Baffour R, Zhou YF, Shou M, Pierre A, Tio FO, et al. Transendocardial delivery of autologous bone marrow enhances collateral perfusion and regional function in pigs with chronic experimental myocardial ischemia. J Am Coll Cardiol. (2001) 37:1726–32. doi: 10.1016/S0735-1097(01)01200-1
55. Kinnaird T, Stabile E, Burnett MS, Shou M, Lee CW, Barr S, et al. Local delivery of marrow-derived stromal cells augments collateral perfusion through paracrine mechanisms. Circulation. (2004) 109:1543–9. doi: 10.1161/01.CIR.0000124062.31102.57
56. Timmers L, Lim SK, Arslan F, Armstrong JS, Hoefer IE, Doevendans PA, et al. Reduction of myocardial infarct size by human mesenchymal stem cell conditioned medium. Stem Cell Res. (2008) 1:129–37. doi: 10.1016/j.scr.2008.02.002
57. Timmers L, Lim SK, Hoefer IE, Arslan F, Lai RC, van Oorschot AAM, et al. Human mesenchymal stem cell-conditioned medium improves cardiac function following myocardial infarction. Stem Cell Res. (2011) 6:206–14. doi: 10.1016/j.scr.2011.01.001
58. Hynes B, Kumar AHS, O'Sullivan J, Klein Buneker C, Leblond A-L, Weiss S, et al. Potent endothelial progenitor cell-conditioned media-related anti-apoptotic, cardiotrophic, and pro-angiogenic effects post-myocardial infarction are mediated by insulin-like growth factor-1. Eur Heart J. (2013) 34:782–9. doi: 10.1093/eurheartj/ehr435
59. Kervadec A, Bellamy V, El Harane N, Arakélian L, Vanneaux V, Cacciapuoti I, et al. Cardiovascular progenitor–derived extracellular vesicles recapitulate the beneficial effects of their parent cells in the treatment of chronic heart failure. J Hear Lung Transplant. (2016) 35:795–807. doi: 10.1016/j.healun.2016.01.013
60. Menasché P. The future of stem cells: Should we keep the “stem” and skip the “cells”? J Thorac Cardiovasc Surg. (2016) 152:345–9. doi: 10.1016/j.jtcvs.2016.02.058
61. Tseliou E, Fouad J, Reich H, Slipczuk L, de Couto G, Aminzadeh M, et al. Fibroblasts rendered antifibrotic, antiapoptotic, and angiogenic by priming with cardiosphere-derived extracellular membrane vesicles. J Am Coll Cardiol. (2015) 66:599–611. doi: 10.1016/j.jacc.2015.05.068
62. Wei K, Serpooshan V, Hurtado C, Diez-Cuñado M, Zhao M, Maruyama S, et al. Epicardial FSTL1 reconstitution regenerates the adult mammalian heart. Nature. (2015) 525:479–85. doi: 10.1038/nature15372
63. Mewhort HEM, Turnbull JD, Meijndert HC, Ngu JMC, Fedak PWM. Epicardial infarct repair with basic fibroblast growth factor–enhanced CorMatrix-ECM biomaterial attenuates postischemic cardiac remodeling. J Thorac Cardiovasc Surg. (2014) 147:1650–9. doi: 10.1016/j.jtcvs.2013.08.005
64. Mewhort HEM, Turnbull JD, Satriano A, Chow K, Flewitt JA, Andrei AC, et al. Epicardial infarct repair with bioinductive extracellular matrix promotes vasculogenesis and myocardial recovery. J Hear Lung Transplant. (2016) 35:661–70. doi: 10.1016/j.healun.2016.01.012
65. Mewhort HEM, Svystonyuk DA, Turnbull JD, Teng G, Belke DD, Guzzardi DG, et al. Bioactive extracellular matrix scaffold promotes adaptive cardiac remodeling and repair. JACC Basic Transl Sci. (2017) 2:450–64. doi: 10.1016/j.jacbts.2017.05.005
66. Park D, Mewhort H, Teng G, Belke D, Turnbull J, Svystonyuk D, et al. Heparin augmentation enhances bioactive properties of acellular extracellular matrix scaffold. Tissue Eng Part A. (2017) 24:128–34. doi: 10.1089/ten.TEA.2017.0004
67. Mewhort HEM, Lipon BD, Svystonyuk DA, Teng G, Guzzardi DG, Silva C, et al. Monocytes increase human cardiac myofibroblast-mediated extracellular matrix remodeling through TGF-β 1. Am J Physiol Hear Circ Physiol. (2016) 310:H716–24. doi: 10.1152/ajpheart.00309.2015
68. Smart N, Riley PR. The epicardium as a candidate for heart regeneration. Future Cardiol. (2012). 8:53–69. doi: 10.2217/fca.11.87
69. Masters M, Riley PR. The epicardium signals the way towards heart regeneration. Stem Cell Res. (2014) 13:683–92. doi: 10.1016/j.scr.2014.04.007
70. Dettman RW, Denetclaw W, Ordahl CP, Bristow J. Common epicardial origin of coronary vascular smooth muscle, perivascular fibroblasts, and intermyocardial fibroblasts in the avian heart. Dev Biol. (1998) 193:169–81. doi: 10.1006/dbio.1997.8801
71. Gittenberger-de Groot AC, Vrancken Peeters MP, Mentink MM, Gourdie RG, Poelmann RE. Epicardium-derived cells contribute a novel population to the myocardial wall and the atrioventricular cushions. Circ Res. (1998) 82:1043–52. doi: 10.1161/01.RES.82.10.1043
72. Mikawa T, Gourdie RG. Pericardial mesoderm generates a population of coronary smooth muscle cells migrating into the heart along with ingrowth of the epicardial organ. Dev Biol. (1996) 174:221–32. doi: 10.1006/dbio.1996.0068
73. Wessels A, Pérez-Pomares JM. The epicardium and epicardially derived cells (EPDCs) as cardiac stem cells. Anat Rec Part A Discov Mol Cell Evol Biol. (2004) 276A:43–57. doi: 10.1002/ar.a.10129
74. Cai C-L, Martin JC, Sun Y, Cui L, Wang L, Ouyang K, et al. A myocardial lineage derives from Tbx18 epicardial cells. Nature. (2008) 454:104–8. doi: 10.1038/nature06969
75. Cano E, Carmona R, Ruiz-Villalba A, Rojas A, Chau YY, Wagner KD, et al. Extracardiac septum transversum/proepicardial endothelial cells pattern embryonic coronary arterio–venous connections. Proc Natl Acad Sci USA. (2016) 113:656–61. doi: 10.1073/pnas.1509834113
76. Smart N, Bollini S, Dubé KN, Vieira JM, Zhou B, Davidson S, et al. De novo cardiomyocytes from within the activated adult heart after injury. Nature. (2011) 474:640–4. doi: 10.1038/nature10188
77. Zhou B, Ma Q, Rajagopal S, Wu SM, Domian I, Rivera-Feliciano J, et al. Epicardial progenitors contribute to the cardiomyocyte lineage in the developing heart. Nature. (2008) 454:109–13. doi: 10.1038/nature07060
78. Christoffels VM, Grieskamp T, Norden J, Mommersteeg MTM, Rudat C, Kispert A. Tbx18 and the fate of epicardial progenitors. Nature. (2009) 458:E8–9. doi: 10.1038/nature07916
79. Dubé KN, Thomas TM, Munshaw S, Rohling M, Riley PR, Smart N. Recapitulation of developmental mechanisms to revascularize the ischemic heart. JCI Insight. (2017) 2:e96800. doi: 10.1172/jci.insight.96800
80. Manner J. Does the subepicardial mesenchyme contribute myocardioblasts to the myocardium of the chick embryo heart? A quail-chick chimera study tracing the fate of the epicardial primordium. Anat Rec. (1999) 255:212–26. doi: 10.1002/(SICI)1097-0185(19990601)255:2andlt;212::AID-AR11andgt;3.0.CO;2-X
81. Pérez-Pomares JM, Carmona R, González-Iriarte M, Atencia G, Wessels A, Muñoz-Chápuli R. Origin of coronary endothelial cells from epicardial mesothelium in avian embryos. Int J Dev Biol. (2002) 46:1005–13.
82. van Wijk B, Gunst QD, Moorman AFM, van den Hoff MJB. Cardiac regeneration from activated epicardium. PLoS ONE. (2012) 7:e44692. doi: 10.1371/journal.pone.0044692
83. Cao J, Poss KD. The epicardium as a hub for heart regeneration. Nat Rev Cardiol. (2018) 15:631–47. doi: 10.1038/s41569-018-0046-4
84. Olivey HE, Svensson EC. Epicardial–myocardial signaling directing coronary vasculogenesis. Circ Res. (2010) 106:818–32. doi: 10.1161/CIRCRESAHA.109.209197
85. Pérez-Pomares JM, de la Pompa JL. Signaling during epicardium and coronary vessel development. Circ Res. (2011) 109:1429–42. doi: 10.1161/CIRCRESAHA.111.245589
86. Chablais F, Jazwinska A. The regenerative capacity of the zebrafish heart is dependent on TGF signaling. Development. (2012) 139:1921–30. doi: 10.1242/dev.078543
87. Kikuchi K, Holdway JE, Major RJ, Blum N, Dahn RD, Begemann G, et al. Retinoic acid production by endocardium and epicardium is an injury response essential for zebrafish heart regeneration. Dev Cell. (2011) 20:397–404. doi: 10.1016/j.devcel.2011.01.010
88. Lavine KJ, Yu K, White AC, Zhang X, Smith C, Partanen J, et al. Endocardial and epicardial derived FGF signals regulate myocardial proliferation and differentiation in vivo. Dev Cell. (2005) 8:85–95. doi: 10.1016/j.devcel.2004.12.002
89. Wang J, Karra R, Dickson AL, Poss KD. Fibronectin is deposited by injury-activated epicardial cells and is necessary for zebrafish heart regeneration. Dev Biol. (2013) 382:427–35. doi: 10.1016/j.ydbio.2013.08.012
90. Lepilina A, Coon AN, Kikuchi K, Holdway JE, Roberts RW, Burns CG, et al. A dynamic epicardial injury response supports progenitor cell activity during zebrafish heart regeneration. Cell. (2006) 127:607–19. doi: 10.1016/j.cell.2006.08.052
91. Simões FC, Riley PR. The ontogeny, activation and function of the epicardium during heart development and regeneration. Development. (2018) 145:dev155994. doi: 10.1242/dev.155994
92. Zhou B, Honor LB, He H, Ma Q, Oh J-H, Butterfield C, et al. Adult mouse epicardium modulates myocardial injury by secreting paracrine factors. J Clin Invest. (2011) 121:1894–904. doi: 10.1172/JCI45529
93. Ruiz-Villalba A, Simón AM, Pogontke C, Castillo MI, Abizanda G, Pelacho B, et al. Interacting resident epicardium-derived fibroblasts and recruited bone marrow cells form myocardial infarction scar. J Am Coll Cardiol. (2015) 65:2057–66. doi: 10.1016/j.jacc.2015.03.520
94. Bollini S, Vieira JMN, Howard S, Dubè KN, Balmer GM, Smart N, et al. Re-activated adult epicardial progenitor cells are a heterogeneous population molecularly distinct from their embryonic counterparts. Stem Cells Dev. (2014) 23:1719–30. doi: 10.1089/scd.2014.0019
95. Baum J, Duffy HS. Fibroblasts and myofibroblasts: what are we talking about? J Cardiovasc Pharmacol. (2011) 57:376–9. doi: 10.1097/FJC.0b013e3182116e39
96. Dixon IMC, Wigle J. Cardiac fibrosis and heart failure : cause or effect? In: Cardiac Fibrosis and Heart Failure: Cause or Effect? Springer. (2015). Available online at: https://books.google.ca/books?id=0D0PCgAAQBAJanddq=ian+dixon+cardiac+fibroblast+sentinel+cellandsource=gbsnavlinkss (accessed February 14, 2019).
97. Fedak PWM, Verma S, Weisel RD, Li R-K. Cardiac remodeling and failure: from molecules to man (Part I). Cardiovasc Pathol. (2005) 14:1–11. doi: 10.1016/j.carpath.2004.12.002
98. Pattar SS, Fatehi Hassanabad A, Fedak PWM. Acellular extracellular matrix bioscaffolds for cardiac repair and regeneration. Front Cell Dev Biol. (2019) 7:63. doi: 10.3389/fcell.2019.00063
99. Souders CA, Bowers SLK, Baudino TA. Cardiac fibroblast: the renaissance cell. Circ Res. (2009) 105:1164–76. doi: 10.1161/CIRCRESAHA.109.209809
100. Fan D, Takawale A, Lee J, Kassiri Z. Cardiac fibroblasts, fibrosis and extracellular matrix remodeling in heart disease. Fibrogenesis Tissue Repair. (2012) 5:15. doi: 10.1186/1755-1536-5-15
101. Fang M, Xiang F-L, Braitsch CM, Yutzey KE. Epicardium-derived fibroblasts in heart development and disease. J Mol Cell Cardiol. (2016) 91:23–7. doi: 10.1016/j.yjmcc.2015.12.019
102. Braitsch CM, Kanisicak O, van Berlo JH, Molkentin JD, Yutzey KE. Differential expression of embryonic epicardial progenitor markers and localization of cardiac fibrosis in adult ischemic injury and hypertensive heart disease. J Mol Cell Cardiol. (2013) 65:108–19. doi: 10.1016/j.yjmcc.2013.10.005
103. Kennedy-Lydon T, Rosenthal N. Cardiac regeneration: epicardial mediated repair. Proc R Soc B Biol Sci. (2015) 282:20152147. doi: 10.1098/rspb.2015.2147
104. Travers JG, Kamal FA, Robbins J, Yutzey KE, Blaxall BC. Cardiac fibrosis: the fibroblast awakens. Circ Res. (2016) 118:1021–40. doi: 10.1161/CIRCRESAHA.115.306565
105. Brown RD, Ambler SK, Mitchell MD, Long CS. THE CARDIAC FIBROBLAST: therapeutic target in myocardial remodeling and failure. Annu Rev Pharmacol Toxicol. (2005) 45:657–87. doi: 10.1146/annurev.pharmtox.45.120403.095802
106. Beltrami CA, Finato N, Rocco M, Feruglio GA, Puricelli C, Cigola E, et al. Structural basis of end-stage failure in ischemic cardiomyopathy in humans. Circulation. (1994) 89:151–63. doi: 10.1161/01.CIR.89.1.151
107. Krenning G, Zeisberg EM, Kalluri R. The origin of fibroblasts and mechanism of cardiac fibrosis. J Cell Physiol. (2010) 225:631–7. doi: 10.1002/jcp.22322
108. Richardson WJ, Holmes JW. Why is infarct expansion such an elusive therapeutic target? J Cardiovasc Transl Res. (2015) 8:421–30. doi: 10.1007/s12265-015-9652-2
109. Badylak SF. The extracellular matrix as a scaffold for tissue reconstruction. Semin Cell Dev Biol. (2002) 13:377–83. doi: 10.1016/S1084952102000940
110. Cui Z, Yang B, Li RK. Application of biomaterials in cardiac repair and regeneration. Engineering. (2016) 2016:141–8. doi: 10.1016/J.ENG.2016.01.028
111. Domenech M, Polo-Corrales L, Ramirez-Vick JE, Freytes DO. Tissue engineering strategies for myocardial regeneration: acellular versus cellular scaffolds? Tissue Eng Part B Rev. (2016) 22:438–58. doi: 10.1089/ten.teb.2015.0523
112. Gaballa MA, Sunkomat JNE, Thai H, Morkin E, Ewy G, Goldman S. Grafting an acellular 3-dimensional collagen scaffold onto a non-transmural infarcted myocardium induces neo-angiogenesis and reduces cardiac remodeling. J Hear Lung Transplant. (2006) 25:946–54. doi: 10.1016/j.healun.2006.04.008
113. Serpooshan V, Zhao M, Metzler SA, Wei K, Shah PB, Wang A, et al. The effect of bioengineered acellular collagen patch on cardiac remodeling and ventricular function post myocardial infarction. Biomaterials. (2013) 34:9048–55. doi: 10.1016/j.biomaterials.2013.08.017
114. Callegari A, Bollini S, Iop L, Chiavegato A, Torregrossa G, Pozzobon M, et al. Neovascularization induced by porous collagen scaffold implanted on intact and cryoinjured rat hearts. Biomaterials. (2007) 28:5449–61. doi: 10.1016/j.biomaterials.2007.07.022
115. Xiang Z, Liao R, Kelly MS, Spector M. Collagen-GAG scaffolds grafted onto myocardial infarcts in a rat model: a delivery vehicle for mesenchymal stem cells. Tissue Eng. (2006) 12:2467–78. doi: 10.1089/ten.2006.12.2467
116. Christman KL, Lee RJ. Biomaterials for the treatment of myocardial infarction. J Am Coll Cardiol. (2006) 48:907–13. doi: 10.1016/j.jacc.2006.06.005
117. Esmaeili Pourfarhangi K, Mashayekhan S, Asl SG, Hajebrahimi Z. Construction of scaffolds composed of acellular cardiac extracellular matrix for myocardial tissue engineering. Biologicals. (2018) 53:10–8. doi: 10.1016/j.biologicals.2018.03.005
118. Rane AA, Christman KL, Jolla L. Biomaterials for the treatment of myocardial infarction A 5-year update. J Am Coll Cardiol. (2011) 58:2615–29. doi: 10.1016/j.jacc.2011.11.001
119. Venugopal JR, Prabhakaran MP, Mukherjee S, Ravichandran R, Dan K, Ramakrishna S. Biomaterial strategies for alleviation of myocardial infarction. J R Soc Interface. (2012) 9:1–19. doi: 10.1098/rsif.2011.0301
120. Christman KL, Fok HH, Sievers RE, Fang Q, Lee RJ. Fibrin glue alone and skeletal myoblasts in a fibrin scaffold preserve cardiac function after myocardial infarction. Tissue Eng. (2004) 10:403–9. doi: 10.1089/107632704323061762
121. Christman KL, Vardanian AJ, Fang Q, Sievers RE, Fok HH, Lee RJ. Injectable fibrin scaffold improves cell transplant survival, reduces infarct expansion, and induces neovasculature formation in ischemic myocardium. J Am Coll Cardiol. (2004) 44:654–60. doi: 10.1016/j.jacc.2004.04.040
122. Arnal-Pastor M, Chachques JC, Monleón Pradas M, Vallés-Lluch A. Biomaterials for cardiac tissue engineering. In: Andrades JA, editor. Regenerative Medicine and Tissue Engineering. IntechOpen (2013). Available online at: https://www.intechopen.com/books/regenerative-medicine-and-tissue-engineering/biomaterials-for-cardiac-tissue-engineering
123. Lister Z, Rayner KJ, Suuronen EJ. How biomaterials can influence various cell types in the repair and regeneration of the heart after myocardial infarction. Front Bioeng Biotechnol. (2016) 4:62. doi: 10.3389/fbioe.2016.00062
124. Fujimoto KL, Tobita K, Merryman WD, Guan J, Momoi N, Stolz DB, et al. An elastic, biodegradable cardiac patch induces contractile smooth muscle and improves cardiac remodeling and function in subacute myocardial infarction. J Am Coll Cardiol. (2007) 49:2292–300. doi: 10.1016/j.jacc.2007.02.050
125. Badylak SF. The extracellular matrix as a biologic scaffold material. Biomaterials. (2007) 28:3587–93. doi: 10.1016/j.biomaterials.2007.04.043
126. Badylak SF, Freytes DO, Gilbert TW. Reprint of: extracellular matrix as a biological scaffold material: structure and function. Acta Biomater. (2015) 23(Suppl):S17–26. doi: 10.1016/j.actbio.2015.07.016
127. Svystonyuk DA, Mewhort HEM, Fedak PWM. Using acellular bioactive extracellular matrix scaffolds to enhance endogenous cardiac repair. Front Cardiovasc Med. (2018) 5:35. doi: 10.3389/fcvm.2018.00035
128. Taylor DA, Chandler AM, Gobin AS, Sampaio LC. Maximizing cardiac repair. Circ Res. (2017) 120:30–2. doi: 10.1161/CIRCRESAHA.116.309959
129. Swinehart IT, Badylak SF. Extracellular matrix bioscaffolds in tissue remodeling and morphogenesis. Dev Dyn. (2016) 245:351–60. doi: 10.1002/dvdy.24379
130. Mosala Nezhad Z, Poncelet A, de Kerchove L, Gianello P, Fervaille C, El Khoury G. Small intestinal submucosa extracellular matrix (CorMatrix®) in cardiovascular surgery: a systematic review. Interact Cardiovasc Thorac Surg. (2016) 22:839–50. doi: 10.1093/icvts/ivw020
131. Hodde J, Record R, Tullius R, Badylak S. Fibronectin peptides mediate HMEC adhesion to porcine-derived extracellular matrix. Biomaterials. (2002) 23:1841–8. doi: 10.1016/S0142-9612(01)00310-6
132. McPherson TB, Badylak SF. Characterization of fibronectin derived from porcine small intestinal submucosa. Tissue Eng. (1998) 4:75–83. doi: 10.1089/ten.1998.4.75
133. Ponce ML, Nomizu M, Delgado MC, Kuratomi Y, Hoffman MP, Powell S, et al. Identification of endothelial cell binding sites on the laminin gamma 1 chain. Circ Res. (1999) 84:688–94. doi: 10.1161/01.RES.84.6.688
134. Hodde JP, Badylak SF, Brightman AO, Voytik-Harbin SL. Glycosaminoglycan content of small intestinal submucosa: a bioscaffold for tissue replacement. Tissue Eng. (1996) 2:209–17. doi: 10.1089/ten.1996.2.209
135. Seghezzi G, Patel S, Ren CJ, Gualandris A, Pintucci G, Robbins ES, et al. Fibroblast growth factor-2 (FGF-2) induces vascular endothelial growth factor (VEGF) expression in the endothelial cells of forming capillaries: an autocrine mechanism contributing to angiogenesis. J Cell Biol. (1998) 141:1659–73. doi: 10.1083/jcb.141.7.1659
136. Witt RG, Raff G, Van Gundy J, Rodgers-Ohlau M, Si M-S. Short-term experience of porcine small intestinal submucosa patches in paediatric cardiovascular surgery. Eur J Cardio-Thoracic Surg. (2013) 44:72–6. doi: 10.1093/ejcts/ezs638
137. Gálvez-Montón C, Fernandez-Figueras MT, Martí M, Soler-Botija C, Roura S, Perea-Gil I, et al. Neoinnervation and neovascularization of acellular pericardial-derived scaffolds in myocardial infarcts. Stem Cell Res Ther. (2015) 6:108. doi: 10.1186/s13287-015-0101-6
138. Khorramirouz R, Kameli SM, Fendereski K, Daryabari SS, Kajbafzadeh A-M. Evaluating the efficacy of tissue-engineered human amniotic membrane in the treatment of myocardial infarction. Regen Med. (2019) 14:113–26. doi: 10.2217/rme-2018-0024
139. Robinson KA, Li J, Mathison M, Redkar A, Cui J, Chronos NAF, et al. Extracellular matrix scaffold for cardiac repair. Circulation. (2005) 112(Suppl 9):I135–43. doi: 10.1161/CIRCULATIONAHA.104.525436
140. Sarig U, Sarig H, de-Berardinis E, Chaw SY, Nguyen EBV, Ramanujam VS, et al. Natural myocardial ECM patch drives cardiac progenitor based restoration even after scarring. Acta Biomater. (2016) 44:209–20. doi: 10.1016/j.actbio.2016.08.031
141. Hadas Y, Katz MG, Bridges CR, Zangi L. Modified mRNA as a therapeutic tool to induce cardiac regeneration in ischemic heart disease. Wiley Interdiscip Rev Syst Biol Med. (2017) 9:e1367. doi: 10.1002/wsbm.1367
142. Hulot JS, Ishikawa K, Hajjar RJ. Gene therapy for the treatment of heart failure: promise postponed. Eur Heart J. (2016) 37:1651–8. doi: 10.1093/eurheartj/ehw019
Keywords: extracellular matrix, biomaterials, epicardium, heart failure, cardiac surgery, bioscaffold, myocardial infarction
Citation: Pattar SS, Fatehi Hassanabad A and Fedak PWM (2019) Application of Bioengineered Materials in the Surgical Management of Heart Failure. Front. Cardiovasc. Med. 6:123. doi: 10.3389/fcvm.2019.00123
Received: 17 June 2019; Accepted: 06 August 2019;
Published: 20 August 2019.
Edited by:
Sharan Ramaswamy, Florida International University, United StatesReviewed by:
Sveva Bollini, University of Genoa, ItalyAlexander Von Gise, Hannover Medical School, Germany
Copyright © 2019 Pattar, Fatehi Hassanabad and Fedak. This is an open-access article distributed under the terms of the Creative Commons Attribution License (CC BY). The use, distribution or reproduction in other forums is permitted, provided the original author(s) and the copyright owner(s) are credited and that the original publication in this journal is cited, in accordance with accepted academic practice. No use, distribution or reproduction is permitted which does not comply with these terms.
*Correspondence: Paul W. M. Fedak, cGF1bC5mZWRhayYjeDAwMDQwO2dtYWlsLmNvbQ==