- 1Department of Cardiology, New York Presbyterian Brooklyn Methodist Hospital, Brooklyn, NY, United States
- 2VA New York Harbor Healthcare System and State University of New York Downstate Medical Center, Brooklyn, NY, United States
- 3Department of Medical Sciences, Surgery and Neurosciences, University of Siena, Siena, Italy
- 4NYU School of Medicine, New York, NY, United States
Patients with autoimmune diseases are at increased risk for developing cardiovascular diseases, and abnormal electrocardiographic findings are common. Voltage-gated calcium channels play a major role in the cardiovascular system and regulate cardiac excitability and contractility. Particularly, by virtue of their localization and expression in the heart, calcium channels modulate pace making at the sinus node, conduction at the atrioventricular node and cardiac repolarization in the working myocardium. Consequently, emerging evidence suggests that calcium channels are targets to autoantibodies in autoimmune diseases. Autoimmune-associated cardiac calcium channelopathies have been recognized in both sinus node dysfunction atrioventricular block in patients positive for anti-Ro/La antibodies, and ventricular arrhythmias in patients with dilated cardiomyopathy. In this review, we discuss mechanisms of autoimmune-associated calcium channelopathies and their relationship with the development of cardiac electrical abnormalities.
Introduction
Voltage gated calcium channels (VGCCs) are macromolecular complexes which include the main pore forming α1-subunits, the accessory β, α2δ, and γ-subunits (1–4). In the heart, VGCCs mediate calcium (Ca) influx in response to membrane depolarization and modulate excitability, contraction, hormonal secretion and gene transcription (1–6). There are many pathologies, both genetic and acquired, involving VGCCs. Mutations in VGCCs cause dysfunctions of Ca channels, resulting in abnormal excitation of the cardiomyocyte, and cardiac arrhythmias (2, 6–8), which contribute substantially to morbidity and mortality. Among the different pathophysiological mechanisms of arrhythmogenesis, a new area of interest has recently emerged and is related to autoimmune-associated Ca channel dysfunction (autoimmune Ca channelopathies) in cardiac arrhythmias (9–12). This review summarizes the recent findings on the roles of cardiac Ca channels in autoantibodies-associated cardiac arrhythmias.
Voltage-Gated Calcium Channels in the Heart
L-type and T-type Ca channels are the two major classes of VGCCs in the heart. The L-type Ca channel is a high voltage-activated, long-lasting, and the T-type channel is characterized by a low voltage-activated, transient-type channel (2, 3, 5, 6, 13, 14). There are 10 isoforms of mammalian genes encoding the α1 subunit. (5, 15–18). CACNA1S, CACNA1C, CACNA1D, and CACNA1F encode α1S, α1C, α1D, and α1F subunits (L-type Ca channels) respectively. CACNA1A, CACNA1B, and CACNA1E encode α1A, α1B, and α1E subunits (P/Q-, N-, and R-types), respectively, (19–21). The T-type Ca channels α1G, α1H, and α1I subunits are encoded by CACNA1G, CACNA1H, and CACNA1I, respectively, (22–24). Among these channels, the L-type Ca channels α1C and α1D isoforms and the T-type Ca channels α1G and α1H isoforms are the major VGCCs expressed in the heart (25–27). The features and tissue distribution of the L-type and T-type Ca channels are summarized in Table 1.
L-type Ca Channels in the Heart
α1C L-type Ca Channel
Cardiac α1C L-type VGCC is a protein complex comprised of α1C, β2, and α2/δ subunits. The α1 subunit is the pore-forming subunit, which determines the major features of the channel, such as ion selectivity, activation-inactivation and the sensitivity to Ca channel blockers (3, 6, 15, 16). The β2 and α2/δ accessory subunits play important roles in the regulation of the biophysical properties of Ca channels (36). The α1C VGCC is universally expressed in the heart and plays a critical role in excitation–contraction coupling, impulse generation in sinus node (SAN) and its conduction in the atrioventricular node (AVN). The Ca ions entering the cardiomyocytes through α1C VGCCs also shape the plateau phase of the ventricular action potential and induce the release of Ca from the sarcoplasmic reticulum (calcium induced-calcium release) which initiates the myocardial contraction (1, 6, 36).
α1D L-type Ca Channel
In contrast to the ubiquitously expressed α1C VGCCs in the heart, α1D VGCCs are restricted to the supraventricular tissue of the adult heart, with the highest expression in the atria, SAN, and AVN, but they are not expressed in the normal adult ventricles (5, 28, 37–42). In the fetal heart, however, α1D VGCCs are expressed throughout the heart including the ventricles, atria, SAN, and AVN (39). While α1C VGCCs activate at more positive (−40 and −30 mV) potentials, α1D VGCCs activate between −60 and −40 mV at a range of diastolic depolarization of the SAN (28, 42). This unique feature allows α1D VGCCs to play an important role in the automaticity of SAN pacemaker cells (29, 43, 44). The unexpected SAN dysfunction reported in mice lacking α1D VGCCs was the first evidence of their importance in heart automaticity (28, 42, 44). Deletion of the α1D VGCC gene impairs pace making in the SAN and atrioventricular conduction in the AVN but has no effect on myocardial contractility (42, 44).
T-type Ca Channels in the Heart
There are 3 isoforms of T-type VGCC: α1G (23, 45), α1H (24), and α1I (45, 46). Among them, α1G and α1H are the major isoforms in the myocardium and their expression is developmentally regulated (17, 30, 31). While α1H T-type VGCC constitutes the predominant isoform in embryonic heart tissue (32); α1G T-type VGCC expression increases during the perinatal period and reaches its maximal level in adulthood. In adult SAN, α1G expression is higher than α1H T-type VGCC (26, 27, 33). In contrast to α1D L-type VGCC, which requires accessary subunits for normal gating, α1G or α1H subunits expression alone exhibit native T-type Ca channel properties (17, 47, 48). In addition, T-type VGCCs open at significantly more negative membrane potentials that overlap the pacemaker potentials of SAN cells (30, 49). The threshold for activation is −70 to −60 mV, and the channel is fully activated at −30 to −10 mV (17, 31, 49). T-type VGCCs are expressed in the SAN (34), the AVN (50), and the Purkinje fibers (51, 52), supporting their roles in the generation of the diastolic depolarization, the automaticity of SAN and the impulse conduction of the heart (30, 31, 53, 54). Indeed, homozygous transgenic mice lacking α1G VGCC exhibit first-degree atrioventricular block (AVB) and bradycardia (25). Collectively, both L-type, and T-type Ca channels by virtue of their tissue-specific localization can modulate automaticity, conduction and repolarization, and as such, agents and compounds like autoantibodies (discussed below) which interact and target these channels are expected to affect the electrical activity of the heart.
Autoantibodies-Associated Cardiac Calcium Channelopathies
Autoimmune disorders and cardiovascular disorders are associated with significant morbidity and mortality and are a major health problem both in the USA and worldwide. While the field of “cardio-immunology” is being formally established, recent and emerging advances in this area indicate that autoantibodies play an important role in the development of cardiac arrhythmias.
Autoantibodies Against Ca Channel and Ventricular Arrhythmias: Anti-α1C Subunit Antibody
Autoimmunity is one of the main mechanisms involved in the pathogenesis of dilated cardiomyopathy (DCM) (55–57). Sudden death caused by ventricular arrhythmias is one of the leading causes of death in patients with DCM (58–60). Results from previous studies indicated that the VGCC plays an important role in the pathogenesis of DCM (11, 61, 62). The function of VGCCs in DCM is affected either by autoantibodies directed against the regulatory pathway/accessary subunits or autoantibodies targeting the pore forming α1 subunit itself. Several autoantibodies indirectly affecting the L-type VGCCs have been identified in patients with DCM (63–65). The presence of antibody against the β-adrenoceptor was first reported in a patient with Chagas' disease by Sterin-Borda et al. (66). Ten years later, Wallukat and Wollenberger demonstrated the presence of an agonist-like anti-β1 adrenoceptor in DCM patients (67). Subsequent studies showed that these autoantibodies in DCM target the second extra-cellular loop of the β1-adrenoreptor (68), resulting in a positive chronotropic effect. Autoantibodies against β1-adrenoceptors were closely related to ventricular arrhythmias in patients with DCM (69). Anti-β1-adrenoceptor antibodies induced in an animal model caused action potential duration prolongation, with higher propensity for induction of early repolarization, promoting the development of ventricular arrhythmias which increased the risk of sudden death (69–71). Notably, Christ et al. (72) demonstrated that anti-β1 adrenoceptor antibodies increased L-Type Ca current, ICa−L in adult rat ventricular cells in concordance with the prolongation of the action potential duration. Autoantibodies against adenine nucleotide translocators, which cross-react with VGCCs, increases the Ca inflow which causes myocyte damage by Ca overload in DCM (73–75).
The evidence of the presence of agonist-like autoantibodies directly against the L-type VGCC α1C subunits in DCM was demonstrated by Liao et al. (76) and Xiao et al. (11) subsequently demonstrated that autoantibodies against α1C Ca channel are arrhythmogenic and lead to sudden cardiac death in patients with DCM. In a prospective study, the authors compared ventricular arrhythmias and sudden death in 80 patients with DCM and age- and gender-matched controls for 32 months. Autoantibodies against L-type α1C subunits (anti-α1C) were detected by ELISA in 39 patients with DCM (48.8%) and 5 controls (6.3%). Higher incidence of ventricular arrhythmias and sudden cardiac death was observed in anti-α1C antibody-positive patients as compared to the antibody-negative patients. The presence of anti-α1C antibodies was identified as the strongest independent predictor for sudden death in DCM (11). The arrhythmogenic effect of anti-α1C antibodies was reproduced in a rat model (11). Perfusion of affinity purified anti-α1C antibodies lead to ventricular arrhythmias by action potential duration prolongation and triggered activity (11). This effect was blocked by pre-incubating the anti-α1C antibodies with its specific peptide and Ca channel blockers, indicating the specificity of the arrhythmogenic effect of the anti-α1C antibodies (11). To further investigate the underlying mechanism of the anti-α1C antibodies, Xiao et al. using immunofluorescent approach demonstrated that anti-α1C antibodies were able specifically to bind to the Ca channel on the myocyte, enhancing the channel's activities (hence the agonist-like effect). In a prospective study, Yu et al. (62) recruited 2096 patients with congestive heart failure, of which 841 dilated cardiomyopathy patients (DMC) 1,255 ischemic cardiomyopathy (ICM) patients, and 834 controls. By the end of a median follow up of 52 months, 102 cases of DCM had sudden cardiac death. Interestingly, the rate of anti-Ca channel antibody in DCM was significantly higher in DCM patients compared to controls. After adjusting for risk factor including age, left ventricular ejection fraction (LVEF), hypertension, diabetes, New York Heart Association (NYHA) functional classification, QTc, and medications, Cox regression analysis revealed that the presence of anti-Ca channel antibodies still remains an independent risk factor for sudden cardiac death in DCM patients. In conclusion, there are novel agonist-like anti-α1C Ca channel antibodies in patients with DCM, which prolong action potential duration and QT interval, induce early after depolarizations, and ventricular tachycardia, eventually leading to sudden cardiac death. These antibodies could serve as novel clinical markers and as positive predictor of sudden death in DCM (Figure 1) (61, 62).
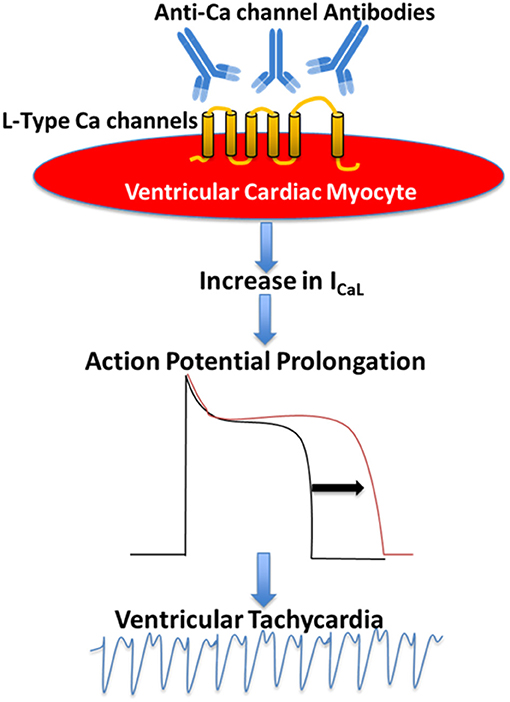
Figure 1. Proposed mechanism of the pathogenic role of anti-Ca channels autoantibodies in Dilated Cardiomyopathy. Anti-Ca channels autoantibodies target L-type Ca channels in the ventricular myocyte resulting in an increase in L-type Ca current (ICaL) which in turn leads to action potential prolongation and ventricular arrhythmias.
Autoimmune-Associated Brady-Arrhythmias and Conduction Abnormalities: Cardiac L-type Ca Channels and Anti-ro Antibodies
While presence of the anti-α1C Ca channel antibody is identified as a strong predictor for ventricular arrhythmias and sudden cardiac death in DCM (11), its role has not been well-established in other autoimmune-associated cardiac electrical abnormalities. The best studied disease caused by autoantibody related L-type Ca channel dysfunction is autoimmune-associated congenital heart block (CHB) characterized by AVB, and sinus bradycardia (10, 35, 77–80). CHB is a conduction abnormality that affects structurally normal hearts of fetuses and/or newborn to mothers with autoantibodies against the intracellular ribonucleoproteins SSA-Ro and SSB-La (10, 79, 80). The hallmark of CHB is various degrees of AVB, with complete AVB being the most common, for which more than 60% of affected children require lifelong pacemakers (81), and carries mortality rate up to 30% (81, 82). Because anti-Ro antibodies are the most prevalent autoantibodies in CHB (83–85), anti-La antibodies are not discussed in this review. There are 2 subtypes of anti-Ro autoantibodies: anti-52 and anti-60 kD SSA/Ro (collectively termed anti-Ro antibodies in this review). Anti-Ro antibodies result from an autoimmune response to the SSA-Ro antigen, which is an intracellular ribonucleoprotein that is not accessible to the circulating anti-Ro antibodies in the normal cardiac myocyte, likely because of their large size. Anti-Ro antibodies are more prevalent in certain autoimmune diseases including Sjögren's syndrome, systemic lupus erythematosus, scleroderma, rheumatoid arthritis, systemic sclerosis, and myositis (86, 87). Intriguingly, these anti-Ro antibodies are also present in the general healthy population (87–89). The incidence of CHB is about 1:11,000 (81, 90); however, this incidence dramatically increases to about 5% in anti-Ro positive mothers and up to 18% in subsequent pregnancies thereby affecting the decision to have a second child (79, 81). The causal relationship of anti-Ro antibodies to the development of CHB was reproduced in both active and passive mice models of CHB (81, 91–93). Various degree of AVB developed in pups born to female mice immunized with recombinant 52 SSA/Ro protein (active immunization) (81, 93, 94). Transfer of anti-Ro antibodies from mothers with CHB children (anti-Ro antibody positive IgG) directly into timely pregnant mice also resulted in first degree AVB and, surprisingly, sinus bradycardia in about 70% of the pups (passive immunization) (91). Similarly, clinical data (95, 96) also confirmed similar sinus bradycardia in newborns of mothers with anti-Ro antibody positive IgG, indicating that the spectrum of CHB extends beyond AVN to also affect SAN.
Anti-Ro Antibody Positive IgG Inhibits Both α1C and α1D Ca Currents
As mentioned above, the hallmark of CHB is AVB. The conduction of the impulse through the AVN depends critically on α1C Ca current, ICa−L, which activates at more positive (−40 and −30 mV) potentials (97). It is logical to speculate that anti-Ro antibody positive IgG might target α1C Ca channel to disturb the electrical conduction at AVN as seen in CHB. Anti-Ro antibody positive IgG and affinity purified anti-52 Ro antibodies from mothers with CHB children, but not anti-Ro antibody negative IgG from healthy mothers, inhibited ICa−L in isolated SAN, AVN cells, Purkinje fibers and in ventricular cells by 50–59% (77, 78, 98–100). In addition, anti-Ro antibody positive IgG had no effect on K currents (the transient outward current, Ito and the inward rectifier, IK1), or the Na current (INa), indicating its specificity toward Ca channels (98). To exclude the possibility of potential contamination from other ion currents, α1C Ca channels expressed in Xenopus oocytes were similarly inhibited about 50% by anti-Ro antibody positive IgG (92, 99, 100).
While inhibition of α1C ICa−L could account for the AVB seen in CHB, the contribution of α1C ICa−L to diastolic depolarization of the SA node is generally considered to be minor. SAN pacemaker depolarization occurs between −60 and −40 mV; however α1C ICa−L activates at more positive (−40 and −30 mV) potentials (101). Knockout of the α1D Ca channel, which activates at −60 and −40 mV in mice, results in significant sinus bradycardia and AVB (28, 42, 102), a phenotype reminiscent to that seen in CHB. Mangoni et al. (44) showed ICa−L in SAN cells was decreased by 75% in α1D Ca channel knockout mice compared with wild-type mice, which indicates that the contribution of the α1D Ca channel to total ICa−L is significant in the mouse SA node cell. Furthermore, our previous studies demonstrated that both α1D Ca channel transcripts and proteins are expressed in human fetal heart and in adult rabbit SAN (39, 40). Collectively, these data suggest that α1D, along with α1C, contribute to form ICa−L, playing a critical role in pace making activity in SAN and are a potential target by anti-Ro antibodies. Because there are no biophysical methods or specific blockers to separate α1D from α1C ICaL in native cells, the specific effect of anti-Ro antibodies on α1D ICa−L has been challenging. Initial studies were carried out in expression systems to allow individual expression of α1D ICa−L to characterize the effect of anti-Ro antibody positive IgG. Anti-Ro antibody positive IgG from mothers with CHB children inhibited α1D ICa−L by about 43% in tsA201 cells and about 33% in Xenopus oocytes (40, 77, 78, 92, 99, 100). To overcome this limitation of using expression systems, our group has tested the effect of anti-Ro antibodies on α1D ICa−L in native neonatal cardiomyocytes, in which the α1C gene was effectively silenced by lentivirus. Adding anti-Ro antibody positive IgG resulted in 35% reduction of α1D ICa−L in naïve cardiomyocytes (103), similar to the results seen using expression systems.
Because anti-Ro antibodies inhibit both α1C and α1D ICa−L, it is anticipated that anti-Ro antibodies will cause both sinus bradycardia and AVB. Further experimental evidence using isolated multicellular AVN preparations (Figures 2A,B) and Langendorff-perfused whole hearts (Figures 2C–E) demonstrated that anti-Ro antibody positive IgG resulted in bradycardia associated with 2:1 AVB then complete third degree AVB as recorded by surface EG. In contrast, perfusion of the AVN preparation or whole heart with control anti-Ro antibody negative IgG had no effect on ECG parameters (78). The sinus bradycardia and AVB were also demonstrated in Langendorff-perfused human hearts by our group (77) and by others (104, 105). Similar findings were obtained using the optical mapping technique, which allows simultaneous recording of voltage action potentials at multiple areas of the heart including the AVN area. Perfusion of hearts with anti-Ro antibody positive IgG revealed the sites of conduction abnormalities at the sinoatrial junction and AVN, thereby confirming the site of action for these autoantibodies (106).
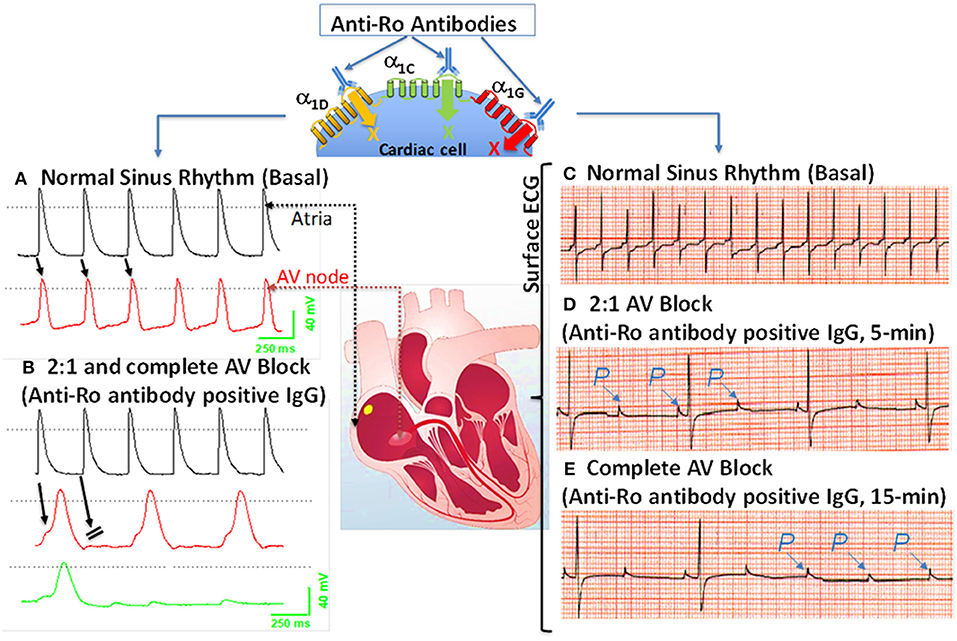
Figure 2. Effects of anti-Ro antibodies from mothers of children with congenital heart block on an isolated multicellular AV nodal preparation (left) and Langendorff perfused whole heart (Right). (A) Simultaneous control action potentials from the crista terminalis (black tracing) and the AV node area (red tracing). (B) Superfusion of the preparation with positive IgG (800 μg/mL) for 10 min resulted in 2:1 AV block (indicated by the arrows) which progressed to near complete inhibition of the AV node action potential by 15 min (B), (green tracing). (C) ECG was recorded by the conventional ECG machine in lead I, except for the use of silver wires at the recording end of the leads. One lead was inserted in the atrium, the second in the left ventricle near the apex, and the third in Tyrode's solution (ground). “P” indicates, the P wave and on the ECG. Regular sinus rhythm (horizontal scale, 50 mm/s and vertical scale: 5 mm/mV) at 300 beats/min in Tyrode's solution. (D) After 5 min of perfusion with positive IgG (800 μg/mL), there was bradycardia associated with a 2:1 second degree AV block that degenerated into complete AV block by 15 min of IgG perfusion (E). The sectioned heart in the middle panel illustrates the location of the microelectrode recordings.
In summary, α1D and α1C Ca channels both contribute to total ICa−L in the heart, with α1D Ca channels playing a more critical role in the SAN and α1C Ca channels in the AVN. Anti-Ro antibodies inhibit ICa−L emanating from both α1D and α1C, resulting in AVB and sinus bradycardia seen in CHB. This causal relationship was confirmed by reproducing active and passive mice CHB models by induction of anti-Ro antibodies (active immunization) or passive transfer of the anti-Ro positive maternal IgG into pregnant mice (passive immunization). Altogether, anti-Ro autoantibodies' inhibition of Ca channels are causally related to the development of CHB, but the low incidence of CHB children born to anti-Ro antibodies positive mothers suggest that additional factor(s) may be necessary to contribute to the full spectrum of CHB.
Anti-ro Antibody Positive IgG Inhibits Ca Currents by Binding Directly to the Pore Forming Subunit of the Ca Channels
As pointed out earlier, anti-Ro antibody positive IgG cannot cross the sarcolemma of a normal fetal cardiac myocyte, and hence one can suspect that its effects are not directly mediated through its antigen, SSA/Ro, but rather via sarcolemma targets such as Ca channels. Evidence for direct interaction between anti-Ro antibodies and Ca channels is provided by the direct binding of anti-Ro antibodies on the pore forming α1 subunit of VGCC, resulting in inhibition of ICaL. Indeed, using immunostaining and Western blots, it was demonstrated that anti-Ro antibody positive IgG binds directly to the Ca channels' α1 subunit (99, 107). In a subsequent study, purified GST fusion proteins corresponding to the extracellular loop S5–S6 of each of the four domains that form the pore of the α1D subunit were expressed and their reactivity to anti-Ro antibody positive IgG was tested. Fourteen percent of anti-Ro antibody positive IgG reacted specifically with the extracellular loop S5-S6 of the first domains of the α1D subunit, as demonstrated by both ELISA and Western blots (108). L-type Ca channels' inhibition by anti-Ro antibodies is one of the mechanisms for the electrocardiographic abnormalities seen in CHB. The resulting formulation of the “Ca channel hypothesis” was based on the above experimental findings and was driven by the fact that AVN electrogenesis depends on the L-type Ca channels. Inhibition of this channel will ultimately lead to AVB, as seen in CHB. The “Ca channel hypothesis” states that circulating maternal antibodies directly cross react with L-type Ca channel pore forming protein α1-subunit, inhibiting the currents and leading to the development of AVB (97).
T-type Ca Channel and Autoimmune-Associated Congenital Heart Block
T-type α1G VGCCs subtype participates with α1H in regulating electrical conduction through the AVN (18, 27, 31, 34). α1G VGCC is highly expressed in the AVN in human hearts (27, 31, 32). Homozygous α1G knockout mice exhibit first-degree AVB and bradycardia, a phenotype seen in CHB (25). These findings suggest α1G VGCC as an additional potential cross-reactive target with anti-Ro antibody positive IgG in the development of CHB. Hu et al. demonstrated that anti-Ro antibody positive IgG decreased both ICaL and T-type Ca current (ICa−T) without affecting the delayed rectifier K current, IK, and the funny current, If, in rabbit SAN cells (98). The average inhibition of ICa−T by anti-Ro antibody positive IgG was 31.4% at −40 mV and 44.1% at −20 mV in rabbit SAN cells (98). In addition, although anti-Ro antibody positive IgG inhibited the α1H ICaT expressed in the Xenopus oocyte (100), α1H Ca channel knockout mice have no ECG changes (109), likely secondary to the low level of α1H expression in the human neonatal AVN cells (107). These findings support the conclusion that the α1G Ca channel is the target for anti-Ro antibody positive IgG. Strindberg et al. demonstrated α1G mRNA and proteins in human fetal hearts and that α1G ICa−T rather than α1H ICa−T is the dominant current in the AVN in newborns (107). Experimental data using immunoprecipitation, Western blot and immunofluorescent staining have demonstrated accessibility of anti-Ro antibody positive IgG to the α1G epitope on the surfaces on the cardiomyocytes in the human fetal heart (107). Reactivity to α1G T-type VGCC was significantly higher in CHB maternal sera compared to controls. Binding epitope of anti-Ro antibody positive IgG was mapped to the extracellular S5–S6 portion of repeat I of α1G subunit (aa305–319; designated as p305). Using the patch-clamp technique, the authors also demonstrated that anti-Ro antibody positive IgG inhibited ICa−T in isolated mice SAN cells (107). Taken together, these results indicate that anti-Ro antibody positive IgG readily target an extracellular epitope of α1G T-type VGCC and inhibit the current in human fetal cardiomyocytes, thus contributing to the development of AVB as seen in CHB.
Anti-52kD Ro antibodies are present in 80% of mothers of children with CHB; however, the risk of having CHB children is low, with only 1–2% in single anti-Ro antibody positive pregnancies (84). Markham et al. investigated if reactivity with p305 (anti-Ro/p305) can be used clinically to more accurately predict CHB in anti-Ro antibody positive patients (110). Using anti-Ro antibody positive IgG and with multiple control groups, reactivity was determined and compared for binding to anti-Ro/p305. In mothers carrying anti-Ro antibodies, positive anti-Ro/p305 antibodies were detected in 3/59 (5%) CHB pregnancies, 4/30 (13%) unaffected pregnancies with a CHB-sibling, and 0/42 (0%) of unaffected pregnancies with no CHB-sibling. Similarly, using umbilical blood from 61 CHB and 41 healthy with CHB-sibling, in which reactivity would unambiguously substantiate exposure to maternal antibody, no association of anti-Ro/p305 with CHB was detected. These data indicate that anti-Ro/p305 reactivity in pregnant anti-Ro antibody-positive patients is not a robust maternal marker for assessing increased risk of CHB (110).
As described above, it is well-recognized that maternal anti-Ro antibody is associated with the development of the congenial AVB, at least in part resulting from an inhibitory cross-reaction with L- and T-type Ca channels. More recent, studies demonstrated that 10–60% of anti-Ro-positive subjects are at increased risk of developing QTc prolongation as a result of anti-Ro antibodies' interference with K channels, (111–115) resulting in complex ventricular arrhythmia, (116, 117) including Torsade's de Pointes (TdP) (118, 119). Lazzerini et al. (119) recently evaluated 25 consecutive patients who experienced TdP, where anti-Ro antibody was present in 15 out of 25 patients. Purified anti-Ro positive IgG from TdP patients cross-reacted with the Human Ether-a-go-go-related Gene (hERG) K channel and significantly inhibited the resulting current, IKr. This observation indicates that anti-Ro antibodies may represent a novel, clinically silent risk factors for TdP. To date, studies on the association of anti-Ro antibodies and atrial fibrillation are scarce. In our previous study (120), we were able to induce atrial fibrillation in the α1D knockout mice but not in the wild-type mice. One can speculate that the unique atrial specific distribution of α1D Ca channel, together with the documented inhibitory effect of the anti-Ro antibodies on the α1D Ca channels, may suggest that anti-Ro positive patients might be at increased risk of having atrial fibrillation, warranting further investigations.
Conclusions and Future Directions
Cardiac Ca channels, including both L- and T-type Ca channels, play critical roles in the impulse generation in the SAN, the conduction through the AVN and the development of arrhythmias. Autoantibodies targeting Ca channels have been identified in 2 major pathologies, DCM and CHB. In addition, several autoantibodies are directly related to sudden death in patients with DCM, including anti-N/K-ATPase, anti-M2 muscarinic acetylcholine receptors, and anti-β1 receptor antibodies, indirectly affecting the L-type VGCCs. Early risk stratification to effectively prevent adverse outcomes in DCM has been challenging. Recent studies confirmed the presence of autoantibodies directly against Ca channel α1C subunit in DCM, which was identified as a strong predictor for ventricular arrhythmias and sudden cardiac death, indicating that anti-α1C Ca channel antibodies might be a valuable biomarker to predict sudden death in DCM.
The association of anti-Ro autoantibodies with CHB is generally accepted, but the predictive value of these autoantibodies is still low despite overwhelming experimental data demonstrating causality between anti-Ro antibodies and electrocardiographic abnormalities seen in CHB (Figure 2). This indicates that anti-Ro antibodies are necessary, but not sufficient, for inducing the clinical electrocardiographic phenotype. To date, two hypotheses have been proposed to explain the molecular mechanism(s) by which maternal anti-Ro antibodies lead to the development of CHB in the fetal hearts (79, 121). The “apoptosis hypothesis” (Figure 3) suggests that intracellular antigens translocate to the surface of cardiomyocytes undergoing apoptosis during physiological remodeling, thereby exposing the antigens to the circulating maternal anti-Ro antibodies. Binding of anti-Ro antibodies to the cell surface antigens promotes pro-inflammatory and pro-fibrotic responses (122, 123), causing the fibrosis of the AVN, which eventually leads to the development of the irreversible AVB (124, 125). The “Ca channel hypothesis” explained in this review is based on molecular mimicry, whereby anti-Ro antibodies directly cross-react and subsequently inhibit the cardiac Ca channels' activity, thereby causing sinus bradycardia and AVB (77, 78, 108) (Figure 4). This occurs by anti-Ro autoantibodies binding to Ca channels and the resulting inhibition of ICaL (Acute effect, Figure 4). The subsequent cross-linkage and downregulation of Ca channels and lysis by lysosomes followed by intracellular Ca dysregulation leads to cell death/apoptosis, inflammation, and fibrosis of the AVN (Figure 4). The ultimate proof of direct autoantibodies' involvement in CHB is provided by the identification of the site of action on the different subunits of cardiac Ca channels (126–128), including α1C and α1D subunits of L-type VGCCs and α1G subunit of T-type VGCCs (Figure 4). Although autoantibodies are utilized as diagnostic or prognostic markers in other pathologies, unfortunately, to date, there is no specific maternal marker for assessing the increased risk of having CHB children during an anti-Ro positive pregnancy. It is possible that, instead of having a single CHB-inducing antibody specificity, future studies may focus on several different specificities that may act synergistically to induce AVB in fetal hearts.
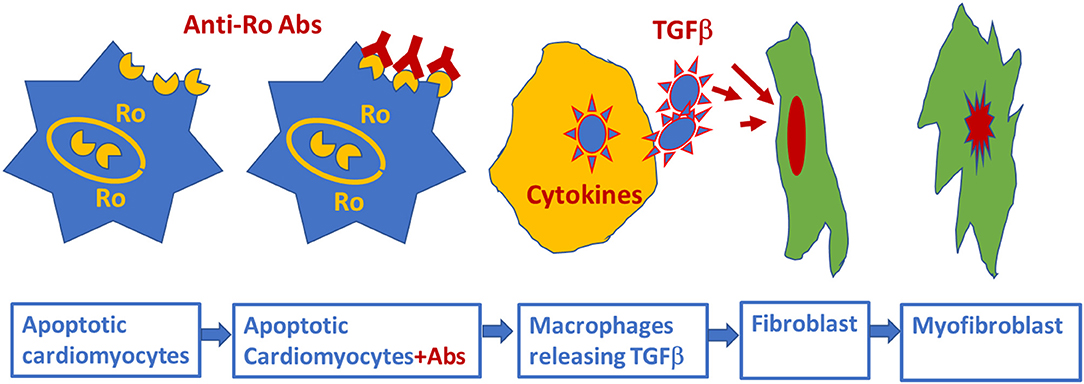
Figure 3. Schematic representation of alternative mechanism of linking anti-Ro antibodies to the development of atrioventricular block: fetal cardiomyocytes undergoing “physiological” apoptosis cause the surface translocation of the intracellular located Ro antigens. Circulating maternal anti-Ro antibodies which can cross the placenta, subsequently bind to the translocated Ro antigens at the cell surface; provoke the secretion of proinflammatory cytokines such as TGFβ from macrophages. Excessive TGFβ secretion activates fibroblasts leading to scars promoting myofibroblasts in the Atrioventricular node, resulting in atrioventricular block.
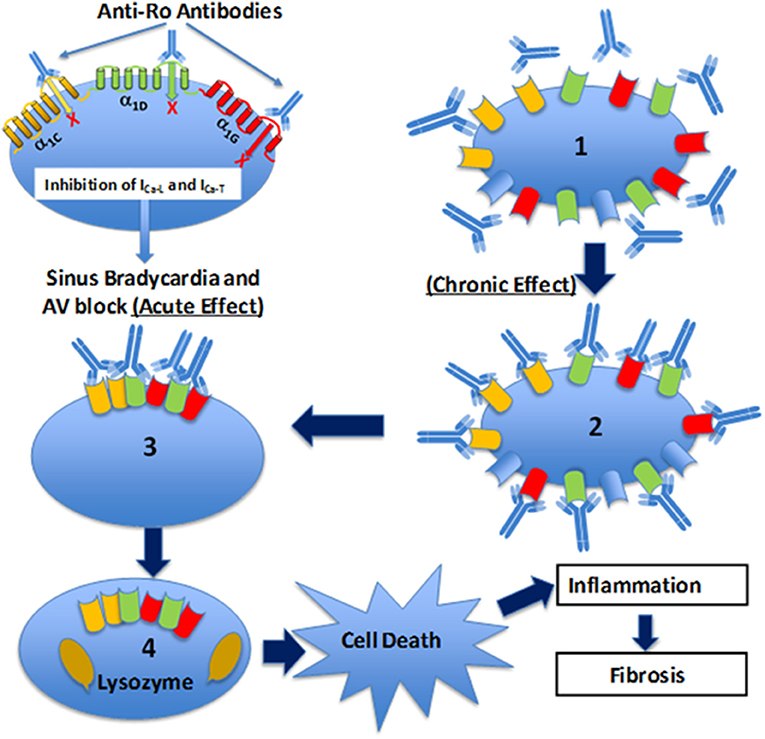
Figure 4. Schematic illustration of the Ca channel hypothesis. Maternal anti-Ro antibodies cross react and bind to α1C (yellow), α1D (green), and α1G (red) Ca channels in the fetal human heart, inhibit all three Ca currents leading to sinus bradycardia and atrioventricular (AV) block (acute effect). Furthermore, fetal heart Ca channels are exposed chronically (chronic effect) (1) to maternal anti-Ro antibodies during pregnancy. Binding of anti-Ro antibodies to Ca channels (2), can cause cross-linking of the adjacent ion channels by the two Fab arms of IgG (3) to increase the internalization of the channel/antibody complex and thereby decrease of the channel density on the cell membrane. Internalized Ca channels are lysed by lysosomes (4). If the number of Ca channels on cell surface decreased to a critical level, then cell death will occur. Cell death, per se, could trigger inflammation subsequent to leukocytic influx resulting in damage of the surrounding healthy myocytes such as in sinoatrial node and AV node which can cause permanent sinus bradycardia and AV block.
Peptide-based therapeutic approaches are one of the growing classes of novel therapeutic agents. The development of short non-immunogenic peptides and their use as decoy targets for pathogenic autoantibodies is expected to minimize and/or prevent autoantibody association with ion channels and their functions. This therapeutic path awaits further development and progress.
Author Contributions
All authors listed have made a substantial, direct and intellectual contribution to the work, and approved it for publication.
Conflict of Interest Statement
The authors declare that the research was conducted in the absence of any commercial or financial relationships that could be construed as a potential conflict of interest.
References
1. Rougier JS, Abriel H. Cardiac voltage-gated calcium channel macromolecular complexes. Biochim Biophys Acta. (2016) 1863:1806–12. doi: 10.1016/j.bbamcr.2015.12.014
2. Betzenhauser MJ, Pitt GS, Antzelevitch C. Calcium channel mutations in cardiac arrhythmia syndromes. Curr Mol Pharmacol. (2015) 8:133–42. doi: 10.2174/1874467208666150518114857
3. Dolphin AC. Calcium channel diversity: multiple roles of calcium channel subunits. Curr Opin Neurobiol. (2009) 19:237–44. doi: 10.1016/j.conb.2009.06.006
4. Catterall WA. Signaling complexes of voltage-gated sodium and calcium channels. Neurosci Lett. (2010) 486:107–16. doi: 10.1016/j.neulet.2010.08.085
5. Mesirca P, Torrente AG, Mangoni ME. Functional role of voltage gated Ca(2+) channels in heart automaticity. Front Physiol. (2015) 6:19. doi: 10.3389/fphys.2015.00019
6. Zamponi GW, Striessnig J, Koschak A, Dolphin AC. The physiology, pathology, and pharmacology of voltage-gated calcium channels and their future therapeutic potential. Pharmacol Rev. (2015) 67:821–70. doi: 10.1124/pr.114.009654
7. Zhang Q, Chen J, Qin Y, Wang J, Zhou L. Mutations in voltage-gated L-type calcium channel: implications in cardiac arrhythmia. Channels. (2018) 12:201–18. doi: 10.1080/19336950.2018.1499368
8. Venetucci L, Denegri M, Napolitano C, Priori SG. Inherited calcium channelopathies in the pathophysiology of arrhythmias. Nat Rev Cardiol. (2012) 9:561–75. doi: 10.1038/nrcardio.2012.93
9. Lee HC, Huang KT, Wang XL, Shen WK. Autoantibodies and cardiac arrhythmias. Heart Rhythm. (2011) 8:1788–95. doi: 10.1016/j.hrthm.2011.06.032
10. Lazzerini PE, Capecchi PL, Laghi-Pasini F, Boutjdir M. Autoimmune cardiac channelopathies: the heart of the matter. Nat Rev Cardiol. (2017) 14:566. doi: 10.1038/nrcardio.2017.111
11. Xiao H, Wang M, Du Y, Yuan J, Cheng X, Chen Z, et al. Arrhythmogenic autoantibodies against calcium channel lead to sudden death in idiopathic dilated cardiomyopathy. Eur J Heart Fail. (2011) 13:264–70. doi: 10.1093/eurjhf/hfq198
12. Durante A, Bronzato S. The increased cardiovascular risk in patients affected by autoimmune diseases: review of the various manifestations. J Clin Med Res. (2015) 7:379–84. doi: 10.14740/jocmr2122w
13. Nilius B, Hess P, Lansman JB, Tsien RW. A novel type of cardiac calcium channel in ventricular cells. Nature. (1985) 316:443–6. doi: 10.1038/316443a0
14. Bean BP. Two kinds of calcium channels in canine atrial cells. differences in kinetics, selectivity, and pharmacology. J Gen Physiol. (1985) 86:1–30. doi: 10.1085/jgp.86.1.1
15. Catterall WA. Voltage-gated calcium channels. Cold Spring Harb Perspect Biol. (2011) 3:a003947. doi: 10.1101/cshperspect.a003947
16. Dolphin AC. Voltage-gated calcium channels and their auxiliary subunits: physiology and pathophysiology and pharmacology. J Physiol. (2016) 594:5369–90. doi: 10.1113/JP272262
17. Perez-Reyes E. Molecular physiology of low-voltage-activated t-type calcium channels. Physiol Rev. (2003) 83:117–61. doi: 10.1152/physrev.00018.2002
18. Cribbs L. T-type calcium channel expression and function in the diseased heart. Channels. (2010) 4:447–52. doi: 10.4161/chan.4.6.12870
19. Nowycky MC, Fox AP, Tsien RW. Three types of neuronal calcium channel with different calcium agonist sensitivity. Nature. (1985) 316:440–3. doi: 10.1038/316440a0
20. Mintz IM, Adams ME, Bean BP. P-type calcium channels in rat central and peripheral neurons. Neuron. (1992) 9:85–95. doi: 10.1016/0896-6273(92)90223-Z
21. Namkung Y, Smith SM, Lee SB, Skrypnyk NV, Kim HL, Chin H, et al. Targeted disruption of the Ca2+ channel beta3 subunit reduces N- and L-type Ca2+ channel activity and alters the voltage-dependent activation of P/Q-type Ca2+ channels in neurons. Proc. Natl. Acad. Sci. U.S.A. (1998) 95:12010–5. doi: 10.1073/pnas.95.20.12010
22. Lambert RC, McKenna F, Maulet Y, Talley EM, Bayliss DA, Cribbs LL, et al. Low-voltage-activated Ca2+ currents are generated by members of the CavT subunit family (alpha1G/H) in rat primary sensory neurons. J Neurosci. (1998) 18:8605–13. doi: 10.1523/JNEUROSCI.18-21-08605.1998
23. Perez-Reyes E, Cribbs LL, Daud A, Lacerda AE, Barclay J, Williamson MP, et al. Molecular characterization of a neuronal low-voltage-activated T-type calcium channel. Nature. (1998) 391:896–900. doi: 10.1038/36110
24. Cribbs LL, Lee JH, Yang J, Satin J, Zhang Y, Daud A, et al. Cloning and characterization of alpha1H from human heart, a member of the T-type Ca2+ channel gene family. Circ Res. (1998) 83:103–9. doi: 10.1161/01.RES.83.1.103
25. Mangoni ME, Traboulsie A, Leoni AL, Couette B, Marger L, Le Quang K, et al. Bradycardia and slowing of the atrioventricular conduction in mice lacking CaV3.1/alpha1G T-type calcium channels. Circ Res. (2006) 98:1422–30. doi: 10.1161/01.RES.0000225862.14314.49
26. Mizuta E, Shirai M, Arakawa K, Hidaka K, Miake J, Ninomiya H, et al. Different distribution of Cav3.2 and Cav3.1 transcripts encoding T-type Ca(2+) channels in the embryonic heart of mice. Biomed Res. (2010) 31:301–5. doi: 10.2220/biomedres.31.301
27. Monteil A, Chemin J, Bourinet E, Mennessier G, Lory P, Nargeot J. Molecular and functional properties of the human alpha(1G) subunit that forms T-type calcium channels. J Biol Chem. (2000) 275:6090–100. doi: 10.1074/jbc.275.9.6090
28. Koschak A, Reimer D, Huber I, Grabner M, Glossmann H, Engel J, et al. alpha 1D (Cav1.3) subunits can form l-type Ca2+ channels activating at negative voltages. J Biol Chem. (2001) 276:22100–6. doi: 10.1074/jbc.M101469200
29. Baig SM, Koschak A, Lieb A, Gebhart M, Dafinger C, Nurnberg G, et al. Loss of Ca(v)1.3 (CACNA1D) function in a human channelopathy with bradycardia and congenital deafness. Nat Neurosci. (2011) 14:77–84. doi: 10.1038/nn.2694
30. Ono K, Iijima T. Pathophysiological significance of T-type Ca2+ channels: properties and functional roles of T-type Ca2+ channels in cardiac pacemaking. J Pharmacol Sci. (2005) 99:197–204. doi: 10.1254/jphs.FMJ05002X2
31. Vassort G, Talavera K, Alvarez JL. Role of T-type Ca2+ channels in the heart. Cell Calcium. (2006) 40:205–20. doi: 10.1016/j.ceca.2006.04.025
32. Ferron L, Capuano V, Deroubaix E, Coulombe A, Renaud JF. Functional and molecular characterization of a T-type Ca(2+) channel during fetal and postnatal rat heart development. J Mol Cell Cardiol. (2002) 34:533–46. doi: 10.1006/jmcc.2002.1535
33. Bohn G, Moosmang S, Conrad H, Ludwig A, Hofmann F, Klugbauer N. Expression of T- and L-type calcium channel mRNA in murine sinoatrial node. FEBS Lett. (2000) 481:73–6. doi: 10.1016/S0014-5793(00)01979-7
34. Hagiwara N, Irisawa H, Kameyama M. Contribution of two types of calcium currents to the pacemaker potentials of rabbit sino-atrial node cells. J Physiol. (1988) 395:233–53. doi: 10.1113/jphysiol.1988.sp016916
35. Benitah JP, Gomez AM, Fauconnier J, Kerfant BG, Perrier E, Vassort G, et al. Voltage-gated Ca2+ currents in the human pathophysiologic heart: a review. Basic Res Cardiol. (2002) 97 (Suppl. 1):I11–8. doi: 10.1007/s003950200023
36. Striessnig J, Pinggera A, Kaur G, Bock G, Tuluc P. L-type Ca(2+) channels in heart and brain. Wiley Interdiscip Rev Membr Transp Signal. (2014) 3:15–38. doi: 10.1002/wmts.102
37. Pinggera A, Lieb A, Benedetti B, Lampert M, Monteleone S, Liedl KR, et al. CACNA1D de novo mutations in autism spectrum disorders activate Cav1.3 L-type calcium channels. Biol Psychiatry. (2015) 77:816–22. doi: 10.1016/j.biopsych.2014.11.020
38. Srivastava U, Aromolaran AS, Fabris F, Lazaro D, Kassotis J, Qu Y, et al. Novel function of alpha1D L-type calcium channel in the atria. Biochem Biophys Res Commun. (2017) 482:771–6. doi: 10.1016/j.bbrc.2016.11.109
39. Qu Y, Karnabi E, Ramadan O, Yue Y, Chahine M, Boutjdir M. Perinatal and postnatal expression of Cav1.3 alpha1D Ca(2)(+) channel in the rat heart. Pediatr Res. (2011) 69:479–84. doi: 10.1203/PDR.0b013e318217a0df
40. Qu Y, Baroudi G, Yue Y, Boutjdir M. Novel molecular mechanism involving alpha1D (Cav1.3) L-type calcium channel in autoimmune-associated sinus bradycardia. Circulation. (2005) 111:3034–41. doi: 10.1161/CIRCULATIONAHA.104.517326
41. Marionneau C, Couette B, Liu J, Li H, Mangoni ME, Nargeot J, et al. Specific pattern of ionic channel gene expression associated with pacemaker activity in the mouse heart. J Physiol. (2005) 562:223–34. doi: 10.1113/jphysiol.2004.074047
42. Platzer J, Engel J, Schrott-Fischer A, Stephan K, Bova S, Chen H, et al. Congenital deafness and sinoatrial node dysfunction in mice lacking class D L-type Ca2+ channels. Cell. (2000) 102:89–97. doi: 10.1016/S0092-8674(00)00013-1
43. Striessnig J, Koschak A. Exploring the function and pharmacotherapeutic potential of voltage-gated Ca2+ channels with gene knockout models. Channels. (2008) 2:233–51. doi: 10.4161/chan.2.4.5847
44. Mangoni ME, Couette B, Bourinet E, Platzer J, Reimer D, Striessnig J, et al. Functional role of L-type Cav1.3 Ca2+ channels in cardiac pacemaker activity. Proc. Natl. Acad. Sci. U.S.A. (2003) 100:5543–8. doi: 10.1073/pnas.0935295100
45. Lee JH, Daud AN, Cribbs LL, Lacerda AE, Pereverzev A, Klockner U, et al. Cloning and expression of a novel member of the low voltage-activated T-type calcium channel family. J Neurosci. (1999) 19:1912–21. doi: 10.1523/JNEUROSCI.19-06-01912.1999
46. Catterall WA, Perez-Reyes E, Snutch TP, Striessnig J. International Union of Pharmacology. XLVIII. Nomenclature and structure-function relationships of voltage-gated calcium channels. Pharmacol Rev. (2005) 57:411–25. doi: 10.1124/pr.57.4.5
47. Dolphin AC, Wyatt CN, Richards J, Beattie RE, Craig P, Lee JH, et al. The effect of alpha2-delta and other accessory subunits on expression and properties of the calcium channel alpha1G. J Physiol. (1999) 519:35–45. doi: 10.1111/j.1469-7793.1999.0035o.x
48. Dubel SJ, Altier C, Chaumont S, Lory P, Bourinet E, Nargeot J. Plasma membrane expression of T-type calcium channel alpha(1) subunits is modulated by high voltage-activated auxiliary subunits. J Biol Chem. (2004) 279:29263–9. doi: 10.1074/jbc.M313450200
49. Chiang CS, Huang CH, Chieng H, Chang YT, Chang D, Chen JJ, et al. The Ca(v)3.2 T-type Ca(2+) channel is required for pressure overload-induced cardiac hypertrophy in mice. Circ Res. (2009) 104:522–30. doi: 10.1161/CIRCRESAHA.108.184051
50. Liu DQ, Zhou SS, Zhao DH, Sheng BH. Effects of furyl-dihydropyridine on action potential ventricular myocardium of rabbit in vivo and isolated guinea pig left atrium in vitro. Zhongguo Yao Li Xue Bao. (1993) 14:164–7.
51. Hirano Y, Fozzard HA, January CT. Characteristics of L- and T-type Ca2+ currents in canine cardiac Purkinje cells. Am J Physiol. (1989) 256:H1478–92. doi: 10.1152/ajpheart.1989.256.5.H1478
52. Tseng GN, Boyden PA. Multiple types of Ca2+ currents in single canine Purkinje cells. Circ Res. (1989) 65:1735–50. doi: 10.1161/01.RES.65.6.1735
53. Nilius B. Possible functional significance of a novel type of cardiac Ca channel. Biomed Biochim Acta. (1986) 45:K37–45.
54. Nilius B, Talavera K, Verkhratsky A. T-type calcium channels: the never ending story. Cell Calcium. (2006) 40:81–8. doi: 10.1016/j.ceca.2006.04.011
55. Liao YH, Fu M. Autoimmunity in the pathogenesis of cardiomyopathy. J Autoimmun. (2001) 16:1–2. doi: 10.1006/jaut.2000.0466
56. MacLellan WR, Lusis AJ. Dilated cardiomyopathy: learning to live with yourself. Nat Med. (2003) 9:1455–6. doi: 10.1038/nm1203-1455
57. Jahns R, Boivin V, Schwarzbach V, Ertl G, Lohse MJ. Pathological autoantibodies in cardiomyopathy. Autoimmunity. (2008) 41:454–61. doi: 10.1080/08916930802031603
58. Felker GM, Thompson RE, Hare JM, Hruban RH, Clemetson DE, Howard DL, et al. Underlying causes and long-term survival in patients with initially unexplained cardiomyopathy. N Engl J Med. (2000) 342:1077–84. doi: 10.1056/NEJM200004133421502
59. Braunwald E. Expanding indications for beta-blockers in heart failure. N Engl J Med. (2001) 344:1711–2. doi: 10.1056/NEJM200105313442210
60. Klein GJ, Krahn AD, Skanes AC, Yee R, Gula LJ. Primary prophylaxis of sudden death in hypertrophic cardiomyopathy, arrhythmogenic right ventricular cardiomyopathy, and dilated cardiomyopathy. J Cardiovas Electrophys. (2005) 16 (Suppl. 1):S28–34. doi: 10.1111/j.1540-8167.2005.50116.x
61. Xiao H, Wang M, Du Y, Yuan J, Zhao G, Tu D, et al. Agonist-like autoantibodies against calcium channel in patients with dilated cardiomyopathy. Heart Vessels. (2012) 27:486–92. doi: 10.1007/s00380-011-0176-7
62. Yu H, Pei J, Liu X, Chen J, Li X, Zhang Y, et al. Calcium channel autoantibodies predicted sudden cardiac death and all-cause mortality in patients with ischemic and nonischemic chronic heart failure. Dis Markers. (2014) 2014:796075. doi: 10.1155/2014/796075
63. Deubner N, Berliner D, Schlipp A, Gelbrich G, Caforio AL, Felix SB, et al. Cardiac beta1-adrenoceptor autoantibodies in human heart disease: rationale and design of the Etiology, Titre-Course, and Survival (ETiCS) Study. Eur J Heart Fail. (2010) 12:753–62. doi: 10.1093/eurjhf/hfq072
64. Jahns R. Autoantibodies against cardiac troponin I: friend or foe? Eur J Heart Fail. (2010) 12:645–8. doi: 10.1093/eurjhf/hfq098
65. Heymans S, Hirsch E, Anker SD, Aukrust P, Balligand JL, Cohen-Tervaert JW, et al. Inflammation as a therapeutic target in heart failure? a scientific statement from the translational research committee of the heart failure association of the European Society of Cardiology. Eur J Heart Fail. (2009) 11:119–29. doi: 10.1093/eurjhf/hfn043
66. Sterin-Borda L, Cossio PM, Gimeno MF, Gimeno AL, Diez C, Laguens RP, et al. Effect of chagasic sera on the rat isolated atrial preparation: immunological, morphological and function aspects. Cardiovasc Res. (1976) 10:613–22. doi: 10.1093/cvr/10.6.613
67. Wallukat G, Wollenberger A. Effects of the serum gamma globulin fraction of patients with allergic asthma and dilated cardiomyopathy on chronotropic beta adrenoceptor function in cultured neonatal rat heart myocytes. Biomed Biochim Acta. (1987) 46:S634–9.
68. Magnusson Y, Marullo S, Hoyer S, Waagstein F, Andersson B, Vahlne A, et al. Mapping of a functional autoimmune epitope on the beta 1-adrenergic receptor in patients with idiopathic dilated cardiomyopathy. J Clin Invest. (1990) 86:1658–63. doi: 10.1172/JCI114888
69. Iwata M, Yoshikawa T, Baba A, Anzai T, Mitamura H, Ogawa S. Autoantibodies against the second extracellular loop of beta1-adrenergic receptors predict ventricular tachycardia and sudden death in patients with idiopathic dilated cardiomyopathy. J Am Coll Cardiol. (2001) 37:418–24. doi: 10.1016/S0735-1097(00)01109-8
70. Magnusson Y, Wallukat G, Waagstein F, Hjalmarson A, Hoebeke J. Autoimmunity in idiopathic dilated cardiomyopathy. characterization of antibodies against the beta 1-adrenoceptor with positive chronotropic effect. Circulation. (1994) 89:2760–7. doi: 10.1161/01.CIR.89.6.2760
71. Magnusson Y, Hjalmarson A, Hoebeke J. Beta 1-adrenoceptor autoimmunity in cardiomyopathy. Int J Cardiol. (1996) 54:137–41. doi: 10.1016/0167-5273(96)02590-9
72. Christ T, Wettwer E, Dobrev D, Adolph E, Knaut M, Wallukat G, et al. Autoantibodies against the beta1 adrenoceptor from patients with dilated cardiomyopathy prolong action potential duration and enhance contractility in isolated cardiomyocytes. J Mol Cell Cardiol. (2001) 33:1515–25. doi: 10.1006/jmcc.2001.1414
73. Schulze K, Heineman FW, Schultheiss HP, Balaban RS. Impairment of myocardial calcium homeostasis by antibodies against the adenine nucleotide translocator. Cell Calcium. (1999) 25:361–70. doi: 10.1054/ceca.1999.0039
74. Liao YH. Functional analysis of autoantibodies against ADP/ATP carrier from dilated cardiomyopathy. Int J Cardiol. (1996) 54:165–9. doi: 10.1016/0167-5273(96)02594-6
75. Liao YH, Cheng LX, Dai SP, Tu YS. Autoantibodies against ADP/ATP carrier from patients with dilated cardiomyopathy increase activity of voltage-dependent Ca channels in isolated cardiac myocytes. Blood Press Supple. (1996) 3:41–4.
76. Liao YH, Yuan J. Progress in the research of targets for the molecular immunotherapy in dilated cardiomyopathy. Zhonghua yi xue za zhi. (2006) 86:1158–60. doi: 10.3760/j:issn:0376-2491.2006.17.003
77. Boutjdir M, Chen L, Zhang ZH, Tseng CE, DiDonato F, Rashbaum W, et al. Arrhythmogenicity of IgG and anti-52-kD SSA/Ro affinity-purified antibodies from mothers of children with congenital heart block. Circ Res. (1997) 80:354–62. doi: 10.1161/01.RES.80.3.354
78. Boutjdir M, Chen L, Zhang ZH, Tseng CE, El-Sherif N, Buyon JP. Serum and immunoglobulin G from the mother of a child with congenital heart block induce conduction abnormalities and inhibit L-type calcium channels in a rat heart model. Pediatr Res. (1998) 44:11–9. doi: 10.1203/00006450-199807000-00002
79. Boutjdir M. Molecular and ionic basis of congenital complete heart block. Trends Cardiovasc Med. (2000) 10:114–22. doi: 10.1016/S1050-1738(00)00059-1
80. Lazzerini PE, Capecchi PL, Guideri F, Acampa M, Selvi E, Bisogno S, et al. Autoantibody-mediated cardiac arrhythmias: mechanisms and clinical implications. Basic Res Cardiol. (2008) 103:1–11. doi: 10.1007/s00395-007-0686-8
81. Miranda-Carus ME, Boutjdir M, Tseng CE, DiDonato F, Chan EK, Buyon JP. Induction of antibodies reactive with SSA/Ro-SSB/La and development of congenital heart block in a murine model. J Immunol. (1998) 161:5886–92.
82. Brucato A, Franceschini F, Buyon JP. Neonatal lupus: long-term outcomes of mothers and children and recurrence rate. Clin Exp Rheumatol. (1997) 15:467–73.
83. Buyon JP, Winchester RJ, Slade SG, Arnett F, Copel J, Friedman D, et al. Identification of mothers at risk for congenital heart block and other neonatal lupus syndromes in their children. comparison of enzyme-linked immunosorbent assay and immunoblot for measurement of anti-SS-A/Ro and anti-SS-B/La antibodies. Arthritis Rheum. (1993) 36:1263–73. doi: 10.1002/art.1780360911
84. Brucato A, Frassi M, Franceschini F, Cimaz R, Faden D, Pisoni MP, et al. Risk of congenital complete heart block in newborns of mothers with anti-Ro/SSA antibodies detected by counterimmunoelectrophoresis: a prospective study of 100 women. Arthritis Rheum. (2001) 44:1832–5. doi: 10.1002/1529-0131(200108)44:8<1832::AID-ART320>3.0.CO;2-C
85. Gordon P, Khamashta MA, Rosenthal E, Simpson JM, Sharland G, Brucato A, et al. Anti-52 kDa Ro, anti-60 kDa Ro, and anti-La antibody profiles in neonatal lupus. J Rheumatol. (2004) 31:2480–7.
86. Franceschini F, Cavazzana I. Anti-Ro/SSA and La/SSB antibodies. Autoimmunity. (2005) 38:55–63. doi: 10.1080/08916930400022954
87. Satoh M, Chan EK, Ho LA, Rose KM, Parks CG, Cohn RD, et al. Prevalence and sociodemographic correlates of antinuclear antibodies in the United States. Arthritis Rheum. (2012) 64:2319–27. doi: 10.1002/art.34380
88. Hayashi N, Koshiba M, Nishimura K, Sugiyama D, Nakamura T, Morinobu S, et al. Prevalence of disease-specific antinuclear antibodies in general population: estimates from annual physical examinations of residents of a small town over a 5-year period. Mod Rheumatol. (2008) 18:153–60. doi: 10.1007/s10165-008-0028-1
89. Guo YP, Wang CG, Liu X, Huang YQ, Guo DL, Jing XZ, et al. The prevalence of antinuclear antibodies in the general population of china: a cross-sectional study. Curr Ther Res Clin Exp. (2014) 76:116–9. doi: 10.1016/j.curtheres.2014.06.004
90. Siren MK, Julkunen H, Kaaja R. The increasing incidence of isolated congenital heart block in Finland. J Rheumatol. (1998) 25:1862–4.
91. Mazel JA, El-Sherif N, Buyon J, Boutjdir M. Electrocardiographic abnormalities in a murine model injected with IgG from mothers of children with congenital heart block. Circulation. (1999) 99:1914–8. doi: 10.1161/01.CIR.99.14.1914
92. Xiao GQ, Qu Y, Hu K, Boutjdir M. Down-regulation of L-type calcium channel in pups born to 52 kDa SSA/Ro immunized rabbits. FASEB J. (2001) 15:1539–45. doi: 10.1096/fj.01-0052com
93. Salomonsson S, Sonesson SE, Ottosson L, Muhallab S, Olsson T, Sunnerhagen M, et al. Ro/SSA autoantibodies directly bind cardiomyocytes, disturb calcium homeostasis, and mediate congenital heart block. J Exp Med. (2005) 201:11–7. doi: 10.1084/jem.20041859
94. Suzuki H, Silverman ED, Wu X, Borges C, Zhao S, Isacovics B, et al. Effect of maternal autoantibodies on fetal cardiac conduction: an experimental murine model. Pediatr Res. (2005) 57:557–62. doi: 10.1203/01.PDR.0000155947.82365.E4
95. Menon A, Silverman ED, Gow RM, Hamilton RM. Chronotropic competence of the sinus node in congenital complete heart block. Am J Cardiol. (1998) 82:1119–21. doi: 10.1016/S0002-9149(98)00569-4
96. Brucato A, Cimaz R, Catelli L, Meroni P. Anti-Ro-associated sinus bradycardia in newborns. Circulation. (2000) 102:E88–9. doi: 10.1161/01.CIR.102.11.e88
97. Mendez C, Zipes DP. Possible ionic mechanism of the action potentials of the atrioventricular node of rabbits. Bol Estud Med Biol. (1974) (Suppl 1):67–80.
98. Hu K, Qu Y, Yue Y, Boutjdir M. Functional basis of sinus bradycardia in congenital heart block. Circ Res. (2004) 94:e32–8. doi: 10.1161/01.RES.0000121566.01778.06
99. Qu Y, Xiao GQ, Chen L, Boutjdir M. Autoantibodies from mothers of children with congenital heart block downregulate cardiac L-type Ca channels. J Mol Cell Cardiol. (2001) 33:1153–63. doi: 10.1006/jmcc.2001.1379
100. Xiao GQ, Hu K, Boutjdir M. Direct inhibition of expressed cardiac l- and t-type calcium channels by IGG from mothers whose children have congenital heart block. Circulation. (2001) 103:1599–604. doi: 10.1161/01.CIR.103.11.1599
101. Takimoto K, Li D, Nerbonne JM, Levitan ES. Distribution, splicing and glucocorticoid-induced expression of cardiac alpha 1C and alpha 1D voltage-gated Ca2+ channel mRNAs. J Mol Cell Cardiol. (1997) 29:3035–42. doi: 10.1006/jmcc.1997.0532
102. Matthes J, Yildirim L, Wietzorrek G, Reimer D, Striessnig J, Herzig S. Disturbed atrio-ventricular conduction and normal contractile function in isolated hearts from Cav1.3-knockout mice. Naunyn Schmiedebergs Arch Pharmacol. (2004) 369:554–62. doi: 10.1007/s00210-004-0940-7
103. Karnabi E, Qu Y, Mancarella S, Yue Y, Wadgaonkar R, Boutjdir M. Silencing of Cav1.2 gene in neonatal cardiomyocytes by lentiviral delivered shRNA. Biochem Biophys Res Commun. (2009) 384:409–14. doi: 10.1016/j.bbrc.2009.04.150
104. Hamilton RM, Lee-Poy M, Kruger K, Silverman ED. Investigative methods of congenital complete heart block. J Electrocardiol. (1998) 30:69–74. doi: 10.1016/S0022-0736(98)80035-6
105. Garcia S, Nascimento JH, Bonfa E, Levy R, Oliveira SF, Tavares AV, et al. Cellular mechanism of the conduction abnormalities induced by serum from anti-Ro/SSA-positive patients in rabbit hearts. J Clin Invest. (1994) 93:718–24. doi: 10.1172/JCI117025
106. Restivo M, Kozhevnikov DO, Boutjdir M. Optical mapping of activation patterns in an animal model of congenital heart block. Am J Physiol Heart Circ Physiol. (2001) 280:H1889–95. doi: 10.1152/ajpheart.2001.280.4.H1889
107. Strandberg LS, Cui X, Rath A, Liu J, Silverman ED, Liu X, et al. Congenital heart block maternal sera autoantibodies target an extracellular epitope on the alpha1G T-type calcium channel in human fetal hearts. PLoS ONE. (2013) 8:e72668. doi: 10.1371/journal.pone.0072668
108. Karnabi E, Qu Y, Wadgaonkar R, Mancarella S, Yue Y, Chahine M, et al. Congenital heart block: identification of autoantibody binding site on the extracellular loop (domain I, S5-S6) of alpha(1D) L-type Ca channel. J Autoimmun. (2010) 34:80–6. doi: 10.1016/j.jaut.2009.06.005
109. Chen CC, Lamping KG, Nuno DW, Barresi R, Prouty SJ, Lavoie JL, et al. Abnormal coronary function in mice deficient in alpha1H T-type Ca2+ channels. Science. (2003) 302:1416–8. doi: 10.1126/science.1089268
110. Markham AJ, Rasmussen SE, Salmon JE, Martinez-Ortiz W, Cardozo TJ, Clancy RM, et al. Reactivity to the p305 epitope of the alpha1G T-type calcium channel and autoimmune-associated congenital heart block. J Am Heart Assoc. (2015) 4:e001836. doi: 10.1161/JAHA.115.001836
111. Cimaz R, Stramba-Badiale M, Brucato A, Catelli L, Panzeri P, Meroni PL. QT interval prolongation in asymptomatic anti-SSA/Ro-positive infants without congenital heart block. Arthritis Rheum. (2000) 43:1049–53. doi: 10.1002/1529-0131(200005)43:5<1049::AID-ANR13>3.0.CO;2-X
112. Cimaz R, Meroni PL, Brucato A, Fesstova V, Panzeri P, Goulene K, et al. Concomitant disappearance of electrocardiographic abnormalities and of acquired maternal autoantibodies during the first year of life in infants who had QT interval prolongation and anti-SSA/Ro positivity without congenital heart block at birth. Arthritis Rheum. (2003) 48:266–8. doi: 10.1002/art.10700
113. Bourre-Tessier J, Clarke AE, Huynh T, Bernatsky S, Joseph L, Belisle P, et al. Prolonged corrected QT interval in anti-Ro/SSA-positive adults with systemic lupus erythematosus. Arthritis Care Res. (2011) 63:1031–7. doi: 10.1002/acr.20470
114. Lazzerini PE, Capecchi PL, Acampa M, Morozzi G, Bellisai F, Bacarelli MR, et al. Anti-Ro/SSA-associated corrected QT interval prolongation in adults: the role of antibody level and specificity. Arthritis Care Res. (2011) 63:1463–70. doi: 10.1002/acr.20540
115. Tufan AN, Sag S, Oksuz MF, Ermurat S, Coskun BN, Gullulu M, et al. Prolonged Tpeak-Tend interval in anti-Ro52 antibody-positive connective tissue diseases. Rheumatol Int. (2017) 37:67–73. doi: 10.1007/s00296-016-3488-1
116. Lazzerini PE, Capecchi PL, Guideri F, Bellisai F, Selvi E, Acampa M, et al. Comparison of frequency of complex ventricular arrhythmias in patients with positive versus negative anti-Ro/SSA and connective tissue disease. Am J Cardiol. (2007) 100:1029–34. doi: 10.1016/j.amjcard.2007.04.048
117. Duke C, Stuart G, Simpson JM. Ventricular tachycardia secondary to prolongation of the QT interval in a fetus with autoimmune mediated congenital complete heart block. Cardiol Young. (2005) 15:319–21. doi: 10.1017/S1047951105000673
118. Nakamura K, Katayama Y, Kusano KF, Haraoka K, Tani Y, Nagase S, et al. Anti-KCNH2 antibody-induced long QT syndrome: novel acquired form of long QT syndrome. J Am Coll Cardiol. (2007) 50:1808–9. doi: 10.1016/j.jacc.2007.07.037
119. Lazzerini PE, Yue Y, Srivastava U, Fabris F, Capecchi PL, Bertolozzi I, et al. Arrhythmogenicity of anti-Ro/SSA antibodies in patients with torsades de pointes. Circ Arrhythm Electrophysiol. (2016) 9:e003419. doi: 10.1161/CIRCEP.115.003419
120. Mancarella S, Yue Y, Karnabi E, Qu Y, El-Sherif N, Boutjdir M. Impaired Ca2+ homeostasis is associated with atrial fibrillation in the alpha1D L-type Ca2+ channel KO mouse. Am J Physiol Heart Circ Physiol. (2008) 295:H2017–24. doi: 10.1152/ajpheart.00537.2008
121. Izmirly P, Saxena A, Buyon JP. Progress in the pathogenesis and treatment of cardiac manifestations of neonatal lupus. Curr Opin Rheumatol. (2017) 29:467–72. doi: 10.1097/BOR.0000000000000414
122. Clancy RM, Neufing PJ, Zheng P, O'Mahony M, Nimmerjahn F, Gordon TP, et al. Impaired clearance of apoptotic cardiocytes is linked to anti-SSA/Ro and -SSB/La antibodies in the pathogenesis of congenital heart block. J Clin Invest. (2006) 116:2413–22. doi: 10.1172/JCI27803
123. Brito-Zerón P, Izmirly PM, Ramos-Casals M, Buyon JP, Khamashta MA. The clinical spectrum of autoimmune congenital heart block. Nat Rev Rheumatol. (2015) 11:301–12. doi: 10.1038/nrrheum.2015.29
124. Litsey SE, Noonan JA, O'Connor WN, Cottrill CM, Mitchell B. Maternal connective tissue disease and congenital heart block. demonstration of immunoglobulin in cardiac tissue. N Engl J Med. (1985) 312:98–100. doi: 10.1056/NEJM198501103120206
125. Ho SY, Esscher E, Anderson RH, Michaelsson M. Anatomy of congenital complete heart block and relation to maternal anti-Ro antibodies. Am J Cardiol. (1986) 58:291–4. doi: 10.1016/0002-9149(86)90064-0
126. Tonello M, Ruffatti A, Favaro M, Tison T, Del Ross T, Calligaro A, et al. Maternal autoantibody profiles at risk for autoimmune congenital heart block: a prospective study in high-risk patients. Lupus Sci Med. (2016) 3:e000129. doi: 10.1136/lupus-2015-000129
127. Reed JH, Clancy RM, Lee KH, Saxena A, Izmirly PM, Buyon JP. Umbilical cord blood levels of maternal antibodies reactive with p200 and full-length Ro 52 in the assessment of risk for cardiac manifestations of neonatal lupus. Arthritis Care Res. (2012) 64:1373–81. doi: 10.1002/acr.21704
Keywords: calcium channel, autoantibodies, autoimmune, channelopathy, cardiac electrical abnormalities
Citation: Qu YS, Lazzerini PE, Capecchi PL, Laghi-Pasini F, El Sherif N and Boutjdir M (2019) Autoimmune Calcium Channelopathies and Cardiac Electrical Abnormalities. Front. Cardiovasc. Med. 6:54. doi: 10.3389/fcvm.2019.00054
Received: 07 November 2018; Accepted: 16 April 2019;
Published: 02 May 2019.
Edited by:
Shimon Rosenheck, Meir Medical Center, IsraelReviewed by:
Daniel M. Johnson, University of Birmingham, United KingdomMartin Ibarrola, Independent Researcher, Bella Vista, Argentina
Thomas Hund, The Ohio State University, United States
Copyright © 2019 Qu, Lazzerini, Capecchi, Laghi-Pasini, El Sherif and Boutjdir. This is an open-access article distributed under the terms of the Creative Commons Attribution License (CC BY). The use, distribution or reproduction in other forums is permitted, provided the original author(s) and the copyright owner(s) are credited and that the original publication in this journal is cited, in accordance with accepted academic practice. No use, distribution or reproduction is permitted which does not comply with these terms.
*Correspondence: Yongxia Sarah Qu, eXNxOTAwMUBueXAub3Jn