- 1Cardiovascular Research Center (ICCC) and CiberCV, Sant Pau Biomedical Research Institute (IIB-Sant Pau), Barcelona, Spain
- 2Cardiovascular Research Chair, UAB, Barcelona, Spain
Atherosclerosis (AT) is a progressive chronic disease involving lipid accumulation, fibrosis, and inflammation in medium and large-sized arteries, and it is the main cause of cardiovascular disease (CVD). AT is caused by dyslipidemia and mediated by both innate and adaptive immune responses. Despite lipid-lowering drugs have shown to decrease the risk of cardiovascular events (CVEs), there is a significant burden of AT-related morbidity and mortality. Identification of subjects at increased risk for CVE as well as discovery of novel therapeutic targets for improved treatment strategies are still unmet clinical needs in CVD. Microvesicles (MVs), small extracellular plasma membrane particles shed by activated and apoptotic cells have been widely linked to the development of CVD. MVs from vascular and resident cells by facilitating exchange of biological information between neighboring cells serve as cellular effectors in the bloodstream and play a key role in all stages of disease progression. This article reviews the current knowledge on the role of MVs in AT and CVD. Attention is focused on novel aspects of MV-mediated regulatory mechanisms from endothelial dysfunction, vascular wall inflammation, oxidative stress, and apoptosis to coagulation and thrombosis in the progression and development of atherothrombosis. MV contribution to vascular remodeling is also discussed, with a particular emphasis on the effect of MVs on the crosstalk between endothelial cells and smooth muscle cells, and their role regulating the active process of AT-driven angiogenesis and neovascularization. This review also highlights the latest findings and main challenges on the potential prognostic, diagnostic, and therapeutic value of cell-derived MVs in CVD. In summary, MVs have emerged as new regulators of biological functions in atherothrombosis and might be instrumental in cardiovascular precision medicine; however, significant efforts are still needed to translate into clinics the latest findings on MV regulation and function.
Introduction
Despite significant advances in prevention, diagnosis, and therapeutic intervention focused on strategies for preventing cardiovascular disease (CVD), coronary artery disease (CAD) remains the leading cause of mortality and morbidity worldwide. Since not every individual has the same risk of developing future cardiovascular events (CVEs), an important challenge in cardiovascular medicine is to accurately predict who will develop atherosclerosis (AT)-related complications, such as acute coronary syndromes (ACS). Current tools to predict atherosclerotic vascular complications perform only poor to moderate. This emphasizes that there is an unmet need for novel biomarkers to stratify the risk of atherosclerotic complications in the intermediate and high-risk population on top of classical risk factors.
Atherosclerosis, the hallmark sign of CVD, is a silent hypercholesterolemia-triggered chronic systemic inflammatory process of the artery wall that is characterized by deposition of lipids within the intima of elastic arteries. This leads to structural damage and formation of fatty streaks with subsequent loss of elasticity, which can develop to fiber and atheromatous plaques, resulting in thrombus formation and narrowing of the luminal. Damage to arterial endothelial cells (ECs) is considered the earliest event in atherogenesis. Development of atherosclerotic plaques also includes the continuous crosstalk between EC, smooth muscle cells (SMCs), inflammatory cells, and inflammatory mediators (1) acting through mechanisms that have not yet been completely revealed.
Microvesicles (MVs), with a diameter ranging from 0.10 to 1.00 μm, are small plasma membrane vesicle fragments, also known as microparticles (MPs), that are released by many cell types, such as platelets, leukocytes, ECs, erythrocytes, and SMCs, into different bodily fluids, including plasma, urine, saliva, milk, sweat, semen, and tears, as well as in conditioned media from cell culture experiments (2, 3) (Figure 1). Depending on the generation mechanism, there are distinct types of extracellular vesicles, including MVs, apoptotic bodies, and exosomes (4), being MVs the most heterogeneous and studied population so far. The present review particularly focuses on MVs, which are specifically formed by budding of the plasma membrane, a releasing process that is driven by calcium-dependent signaling, activity of several enzymes, cytoskeleton remodeling, and externalization of phosphatidylserine (PS). MVs are shed under basal conditions and their release increases with various stimuli and pathological settings. In contrast to MVs, apoptotic bodies are larger permeable membrane vesicles with a diameter >1 μm containing apoptotic nuclear material while exosomes constitute the smallest extracellular vesicle type (ranging from 40 to 100 nm in diameter), highly enriched in lipids and tetraspanins, and actively shed from intracellular multivesicular bodies upon fusion with the cell membrane.
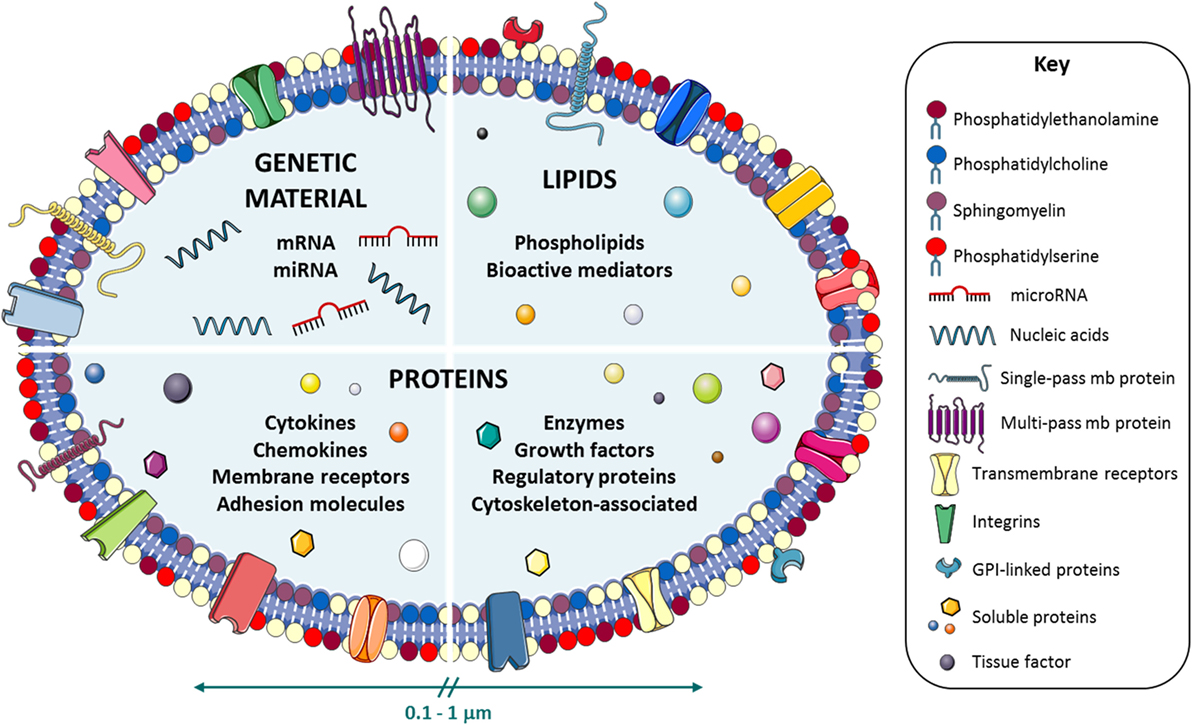
Figure 1. Microvesicle (MV) composition. Schematic representation of the molecular repertoire of the cell-derived MVs. MVs are loaded with distinct components of genetic material [nucleic acids, mRNAs, microRNAs (miRNAs)], lipids (phospholipids and bioactive mediators), and proteins (cytokines, chemokines, membrane receptors, adhesion molecules, enzymes, growth factors, and cytoskeleton-associated and regulatory proteins) to mediate intercellular communication processes.
Microvesicles are specifically composed of lipids, genetic material, such as mRNA, non-coding ribonucleic acids (RNAs) [microRNA (miRNA)], or even small amounts of DNA, and proteins such as transcription factors, cytokines, and growth factors (Figure 1). Interestingly, the packaging of distinct biomolecules into MVs seems to occur in a non-randomly fashion. Thus, specific miRNAs were seen to be preferentially sorted into MVs. Blood cells and cultured monocytic THP1 cells actively and selectively secreted MV-loaded miRNAs into the circulation in response to various stimuli (5). Nevertheless, further efforts are needed toward a complete understanding of this regulated sorting mechanism. MVs have been characteristically recognized by the externalization of PS on the outer membrane leaflet. However, this property has recently been a matter of debate. New evidence suggests that some MVs can express cell markers without annexin V binding (6, 7). Interestingly, MVs harbor on their surface transmembrane and receptor proteins from the parental cells from which they derived from. This property, important for specific cell–cell interactions, is also used in MV identification and characterization by high-sensitivity flow cytometry. MVs can deliver their cargo to cells nearby or in remote locations, perpetuating the intercellular communication process. Since their content fluctuates depending on the pathological context, MVs have drawn the attention as a potential source of biomarkers for disease identification (8).
Flow cytometry has been the gold standard methodological choice for MV measurements. Recently, some new methods (9) such as atomic force microscopy have been developed. Today there is still a general need of establishing preanalytical steps for MV isolation and of validating novel techniques. Recent efforts (10–12) are addressed to standardize MV analytical procedures between instruments and laboratories (13).
Microvesicles promote the development and progression of AT, by inducing endothelial dysfunction (ED) and initial lesion formation, influencing cell communication, promoting inflammatory reactions and participation in lipid deposition, neovascularization, calcification and unstable plaque progression, and injured plaque clotting and thrombosis after rupture. Here, we review the current and last data on the role of MVs in AT and CVD, highlighting their relevance for vascular remodeling and neovascularization. In addition, we discuss the emerging interest of MVs as prognostic and diagnostic biomarkers of disease and their potential use as therapeutic agents.
MV-Mediated Regulatory Mechanisms in the Development and Progression of AT
Several evidences support a direct functional role for circulating MVs in atherothrombosis. They span from the early stages related to the presence of classical cardiovascular risk factors (CVRF) (e.g., hyperlipidemia, diabetes, and hypertension) to acute cardiovascular and cerebrovascular events as result of their effect on intercellular communication processes transferring proteins, non-coding RNAs, and even mRNAs (14) to target cells. Specifically, MVs are able to (1) fuse with plasma membrane of target cells and transfer their cargo into the recipient cells, (2) interact with target cells via a receptor-mediated signaling mechanism, and (3) be internalized via endocytosis and fused with either endosomes to release their content into the cytosol or lysosomes to be degraded (2). To bridge the gap between atherosclerotic lesion initiation, plaque rupture, and CVEs, multifaceted MV-driven molecular mechanisms of AT progression linked to endothelial homeostasis, inflammation, and thrombosis will be discussed.
Endothelial Dysfunction
Endothelial cells exert natural barrier functions in the vessel wall to prevent pathogen invasion and to maintain vascular integrity, through the balanced release of cell and molecular components acting at paracrine and autocrine level. Vascular ECs change their phenotype and function in response to mechanical injury and systemic factors, such as dyslipidemia, smoking, and other stimuli and/or risk factors.
Recent studies support a direct relationship between the levels of circulating endothelial-derived MVs (eMVs) and the degree of ED in patients with end-stage renal failure (15), congenital heart disease (16), CAD (17, 18), type 2 diabetes (T2D) (19), and obesity and hypertension (20). Indeed, MVs are able to interact with the vascular endothelium and promote cellular dysfunction (Figure 2). Thus, MVs from T2D patients have shown to attenuate eNOS expression in cultured EC (21). Furthermore, MVs of endothelial origin have shown to decrease nitric oxide (NO) production in vitro (22, 23) and under high glucose conditions through changes in oxidative stress (24) or inducing a temporal cross talk between mitochondria and endoplasmic reticulum (25). These effects have been obtained in ex vivo studies (23, 26) and with patient-derived MVs in pathologies such as myocardial infarction (MI) (27), end-stage renal failure (15), metabolic syndrome (28), valvular heart disease and cardiac injury (29), and undergoing percutaneous coronary intervention (30). As a result, vascular tone and endothelial repair capacity are altered. Studies in vitro using rat aortic rings provided evidence that eMVs lead to impaired acetylcholine-mediated vasorelaxation (22), and studies ex vivo with eMVs from ACS patients induced premature ED, senescence, and thrombogenicity through an angiotensin II-dependent redox activation of phosphoinositide 3-kinase/Akt and mitogen-activated protein kinases pathways (31). In addition, when MVs obtained from plasma of subjects with metabolic syndrome, were injected intravenously in mice, a severe impairment of endothelium-triggered vasorelaxation with decreased NO-synthase expression was detected (28). Horn et al. reported that MVs carry functionally active NO synthase that induce NO production within the MVs, a function downregulated in MVs from patients with ED (32). These results indicate that eMVs have a functional role in the control of vascular homeostasis.
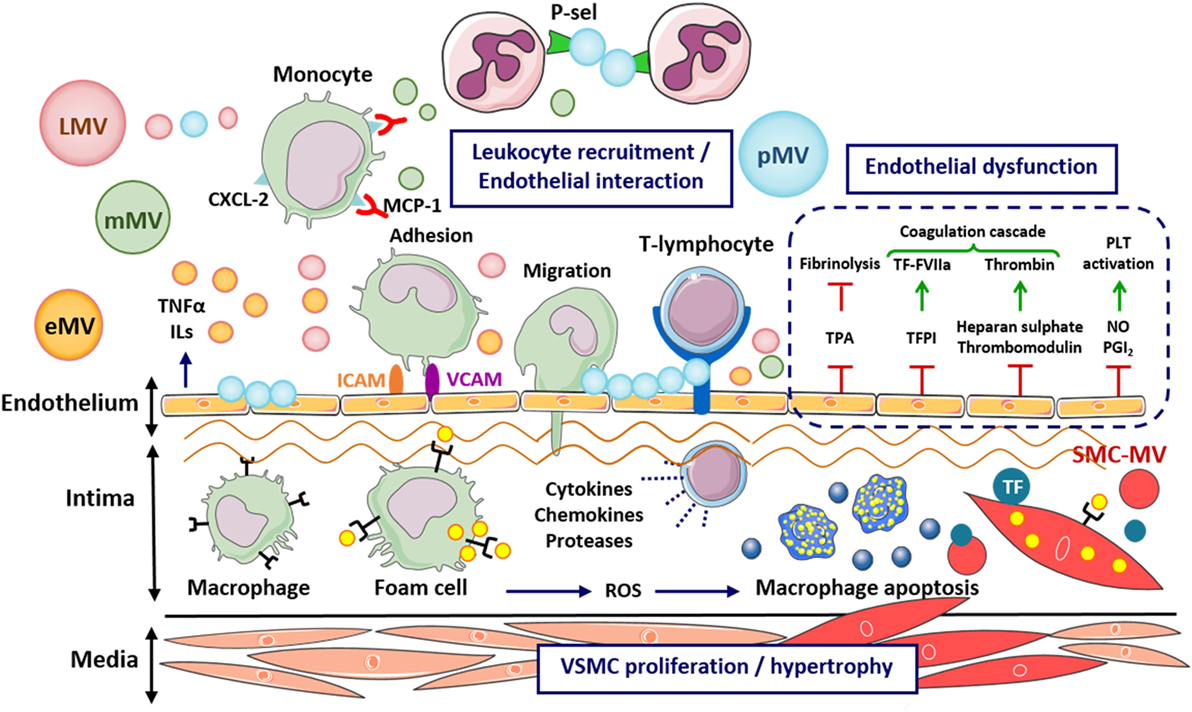
Figure 2. Effects of microvesicles (MVs) on the early stages of atherosclerosis development. Cell-derived MVs are able to interact with the subendothelial matrix, induce endothelial dysfunction, stimulate proinflammatory response, enhance the adhesion and infiltration of leukocytes, as well as oxidative stress, apoptosis, and vascular remodeling, promoting the inflammation and injury of the vessel wall and the progression of atherosclerotic lesions. CXCL-2, C–X–C motif chemokine ligand 2; eMV, endothelial cell-derived microvesicle; FVIIa, coagulation factor VIIa; ICAM, intercellular cell adhesion molecule; LMV, leukocyte-derived microvesicle; mMV, monocyte-derived microvesicle; MCP-1, monocyte chemotactic protein 1; NO, nitric oxide; PGI, prostacyclin; pMV, platelet-derived microvesicle; P-Sel, P-selectin; ROS, reactive oxygen species; SMC-MV, smooth muscle cell-derived microvesicle; VCAM, vascular cell adhesion molecule; TF, tissue factor; TFPI, tissue factor pathway inhibitor; TPA, tissue plasminogen activator; TNF, tumor necrosis factor.
The physiopathological conditions stimulating MVs formation have an impact on MV phenotype and functional activity. Interestingly, Mahmoud and colleagues reported that MVs from healthy individuals or shed under basal conditions had no effects on endothelial function, while in vitro generated eMVs exert a protective role on endothelial function in a free-fatty acid-induced model, via eNOS/Akt signaling and reduced oxidative stress (33). This recent study reflects on how MVs may behave as different biological effectors in health and disease, highlighting the relevance of the environment and pathological stimuli triggering MV release on their phenotype and biological activity.
Injured endothelium beyond promoting the recruitment of activated platelets to repair damaged ECs, also facilitates adherence of platelet-derived MVs (pMVs) to the vascular wall (Figure 2), inducing in turn the permeability and apoptosis of ECs, the latter by MV transfer of caspase-3 and Rho-kinase enzymes according to the in vitro findings reported by Edrissi et al. (34). More specifically, these authors have proved that plasma-derived MVs from rats submitted to chronic cerebral ischemia contain factors involved in the activation of the tumor necrosis factor (TNF)-α pathway that delivered to ECs regulate endothelial permeability in vitro.
Vascular Wall Atheroinflammation
The role of MVs on the propagation of endothelial proinflammatory response was initially reported in in vitro studies showing that blood-derived MVs (obtained from either plasma and/or cell cultures, and also induced by high-shear stress) raise the release of cytokines and cell adhesion molecules in ECs (35, 36) and in leukocytes (37). In addition, circulating MVs obtained from healthy volunteers after infusion of a chemotactic peptide induced cytokine and chemokine release in in vitro EC cultures (38). Similar findings have been reported using MVs from both animal models and patients (39, 40). Thus, MVs obtained from a high-fat diet-fed obese murine model of insulin resistance and T2D induced the expression of vascular cell adhesion molecule-1 and reactive oxygen species (ROS) production in rat cardiac ECs (41). Furthermore, MVs have also shown to increase the monocyte expression of cell surface antigens such as CD11a/CD18 and CD11b/CD18 αM/β2 (37) and the endothelial expression of intercellular cell adhesion molecule-1 (ICAM-1) (42, 43). Increasing evidences suggest that regulation of protein expression by MVs is dependent on their miRNA-cargo. Thus, MV-mediated regulation of ICAM-1 expression in ECs is dependent on their content in miRNA-222 and its transfer to the target cells (44). MV-associated inflammatory cascade promotes the binding of monocytes to the endothelium and their infiltration into the atherosclerotic plaque (Figure 2) (24, 37, 45–47). Indeed, in vitro studies demonstrated that monocyte and leukocyte activation and adhesion is regulated by eMVs transferring miRNA-10 to the monocyte and thereby targeting nuclear factor-κB inflammatory pathway (48) and by MVs that carry arachidonic acid as well as oxidize phospholipids (45). Another smart study showed that pMVs transfer proatherogenic C–C motif chemokine 5 [regulated on activation, normal T cell expressed and secreted (RANTES)] to either activated ECs or murine atherosclerotic carotid arteries enabling monocyte adherence (46). Thus, MVs might contribute to the early atherosclerotic lesions development by promoting leukocyte adhesion to the endothelium.
Oxidative Stress, Apoptosis, and Vascular Remodeling
The seminal study of Forlow et al. showed that pMVs expressing P-selectin (P-Sel) promoted monocyte infiltration by facilitating leukocyte–leukocyte interactions under detrimental flow conditions (Figure 2) (49). Besides, lipid-containing MVs derived from various cell types promote foam cell formation and programmed cell death by inducing lipid and cholesterol accumulation in macrophages through a toll-like receptor induced mechanism in vitro and in a human skin model (50). TNF-α-induced eMVs contributed to apoptosis and inflammation of ECs in vitro (51). Delivery of the proapoptotic enzymes caspase-1 and caspase-3 to target cells by eMVs, pMVs, monocyte-derived MVs (mMVs), T-lymphocyte-derived MVs (ℓMVs), and erythrocyte-derived MVs trigger macrophage cell apoptosis in vitro (52–56), which in turn causes more MV release, amplifying the process and facilitating progression of the atherosclerotic lesion. Thus, human atherosclerotic lesions contain MVs (57, 58) being those of monocyte and macrophage origin the most abundant. Apoptosis cell-derived MVs amplify the initiation and maintenance of inflammation in human monocytes together with interferon γ (59) in contrast to a recent study pointing out that MVs released from apoptotic cells provoke less inflammatory response than MVs from viable cells in monocytes, relating this effect with the specific miRNAs pattern found in these MVs (60). Furthermore, atherogenic low-density lipoprotein (LDL) modified by aggregation induce the release MVs enriched in tissue factor (TF) from SMCs (61) (Figures 2 and 3). pMVs increased SMC proliferation (62) by a mechanism independent of platelet-derived growth factor (PDGF) (63) whereas mMVs induced SMC death by caspase-1 (56).
High-risk plaques have a large lipid content covered by a thin fibrous cap penetrated by proinflammatory cells and a diffuse pattern of calcification (64, 65). The level of calcification has associated either with plaque burden (66) and lesion destabilization (67) or with less prone to rupture plaques (68). It seems plausible that the effect of calcification on atherosclerotic lesions evolves from a destabilizing effect in early lesions to a potential stabilizing effect of larger calcium burden in more advanced plaques (69). MVs from ECs, VSMCs, and macrophages seem to be recruited at the site of atherosclerotic plaque calcification and have been associated with the calcification process (70–73). Regarding MV-associated modulation of fibrous cap weakening, several cell-derived MVs can influence the progressive depletion of VSMCs and extracellular matrix degradation through metalloprotease interaction. Specifically, the involved molecular effectors are matrix metalloproteinase 9 (MMP-9), a disintegrin and metalloprotease-10 (ADAM-10) and -17 (ADAM-17) in neutrophil-derived MVs [(74, 75), respectively], MMP-9, matrix metalloproteinase-2 (MMP-2), and MMP-10 in eMVs [(76–78), respectively], and the metalloprotease TNF-α converting enzyme (TACE/ADAM-17) of human atherosclerotic plaques-derived MVs (79). A relevant finding is that the shedding of matrix MVs from arterial intimal SMCs was found higher in athero-prone areas of the human aorta than in athero-resistant areas at the preatherosclerotic disease stage with quantitative electron microscopic analyses (80). Shear stress-induced eMVs-containing miRNA-143 and miRNA-145 prevented VSMC dedifferentiation (81). Likewise, circulating MVs bearing miRNA-223 could penetrate the vascular wall and inhibit VSMC proliferation and migration, resulting in a decreased plaque size (82).
Coagulation and Thrombosis
The potential role of MVs in atherothrombosis is supported by a large number of studies. Procoagulant MVs are located within human advance vulnerable plaques (83). Upon atherosclerotic plaque erosion or rupture, the high-risk vulnerable plaque exposes their vascular contents to the blood flow, the coagulation cascade is activated and, concomitantly, there is recruitment and activation of circulating platelets that may lead to thrombus formation. Thrombosis may compromise arterial blood flow supply leading to the presentation of oxygen deficiency and the presence of MI.
First described as platelet dust (84), MVs are procoagulant biological effectors due to the surface content of negatively charged PS, which confers them high binding capacity for coagulating factors, being prominently higher in MVs than cell surface membrane in the case of platelets (85). The presence of other molecules and receptors including TF (83), factor VIII and Va (86), P-selectin glycoprotein ligand-1 (PSGL-1) (87), glycoprotein IIb/IIIa (88), and protein disulfide isomerase (PDI) (89) on MV surface might further enhance clot formation and thrombosis. TF can be functionally transferred via MVs to monocytes and other cells (90, 91). Protease-activated receptor 2 activation favors the shedding of TF-rich MVs through a process involving filamin A. Specifically, the interaction of TF with filamin A translocate cell surface TF to cholesterol-rich lipid rafts, promoting its activity and release into MVs (92). Indeed, procoagulant TF-rich MVs are increased by hyperinsulinemia (93) and found increased in patients with T2D (94). Recently, it has been shown that proinflammatory cytokine IL-33 induces differential TF expression and activity in intermediate monocyte subset as well as the release of procoagulant MVs rich in TF (95), emphasizing the interplay between inflammation and thrombosis (Figure 3).
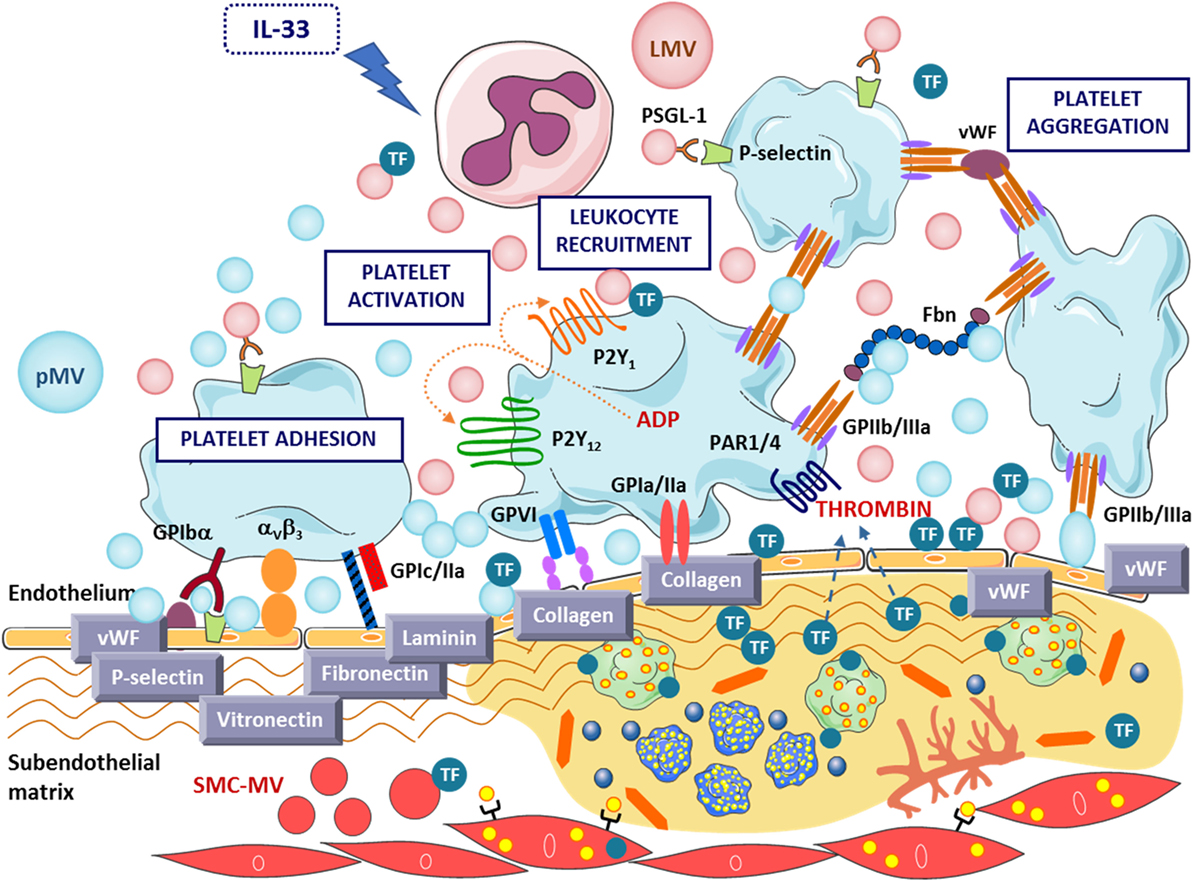
Figure 3. Key prothrombotic mechanisms exerted by microvesicles (MVs) at sites of vascular injury. MVs’ adhesion to the endothelial layer enhances platelet adhesion and thrombus formation and contributes to TF release at the injured atherosclerotic plaque. IL-33, interleukin-33; LMV, leukocyte-derived microvesicle; mMV, monocyte-derived microvesicle; pMV, platelet-derived microvesicle; PSGL-1, P-selectin glycoprotein ligand-1; SMC-MV, smooth muscle cell-derived microvesicle; TF, tissue factor; vWF, von Willebrand factor.
Several key studies have addressed how MVs affect the clotting process (Figure 3). Upon endothelial injury, pMVs can bind to subcellular matrix to enhance clotting (96). Noteworthy, we reported that circulating and platelet-derived MVs exert direct effects on atherothrombosis by promoting platelet and fibrin deposition on atherosclerotic arterial wall (97). We performed a proof-of-concept study by perfusing blood with and without exogenously added pMVs to injured atherosclerotic vessel wall and demonstrated that elevated levels of pMVs were able to enhance platelet and fibrin adhesion under conditions of high-shear stress (97, 98). Indeed, a decreased level of pMVs bearing surface epitopes of adhesion and activation was found in blood perfusing prothrombotic surfaces, and also in ST-segment elevation myocardial infarction (STEMI) patients (99), supporting the high tendency to adhere of pMVs (49). High-shear stress-induced pMVs induce enhanced expression of cell adhesion molecules in ECs and monocytes (36) and regulate monocytes involvement in AT and inflammation (37). Interestingly, MVs from healthy subjects support low-grade thrombin generation (100) and are able to activate a stress signaling pathway in ECs leading to increased procoagulant activity (35); and PDI-bearing pMVs promote platelet hyperaggregability and insulin degradation in patients with T2D (89). Furthermore, interactions between platelet P-selection with PSGL-1 of leukocyte-derived MVs (LMVs) are required to concentrate TF activity at the thrombus edge to promote thrombus formation (87). MVs carrying functional TF might enable the growth of unregulated thrombus generation and fibrin formation and thrombus propagation ultimately leading to thrombotic complications (101).
Potential Prognostic, Diagnostic, and Therapeutic Value of Cell-Derived MVs in AT
CV diseases reflect a continuum of mechanisms underlying the gradually progressing AT. In addition to the pathogenic effects of MVs in AT and thrombosis, circulating levels of MVs (cMVs) of different cellular origin are increased in CVDs and reflect the severity of the different stages of the pathophysiology (102), thus cMVs might serve as potential diagnosis and prognosis biomarkers, which would be valuable tools for cardiovascular risk prediction as well as for evaluating the pharmacological response to therapeutic interventions.
Circulating microvesicles exist in the blood of normal healthy individuals released upon activation and in some cases apoptosis of vascular cells. Indeed, MV levels show gender-specific differences (103, 104) and changes are observed with age (105), during pregnancy (106), after exercise (107–109) and after a high-fat meal (110). It is important to recognize that differences may arise depending on age, gender, body mass index, lipid, hormone levels, smoking status and other confounding variables in apparently healthy subjects when evaluating cMVs for pathogenic potential (111). Another relevant issue to bear in mind when studying cMVs is their clearance mechanism, which might influence the levels of cMVs and could also be impaired by CVRF.
Cardiovascular Risk Factors
The number and phenotype of cMVs has been associated to major CVRF, such as smoking, diabetes mellitus, obesity, hypertension, dyslipidemia, and metabolic syndrome (Table 1). Within hyperlipidemia, it has been shown that in patients with heterozygous familial hypercholesterolemia (FH), pMVs carrying TF identify subclinical atherosclerotic plaque burden (112), and ℓMPs are able to discriminate between lipid-rich and fibrous atherosclerotic plaques in the same patients (113), thus reflecting that chronic exposure to high levels of LDL activates the vascular compartment by distinct mechanisms. Therefore, CVRF correlate with increased numbers of MVs indicating that MVs might be active in triggering thrombosis and further contributing to an increased risk of CVEs.
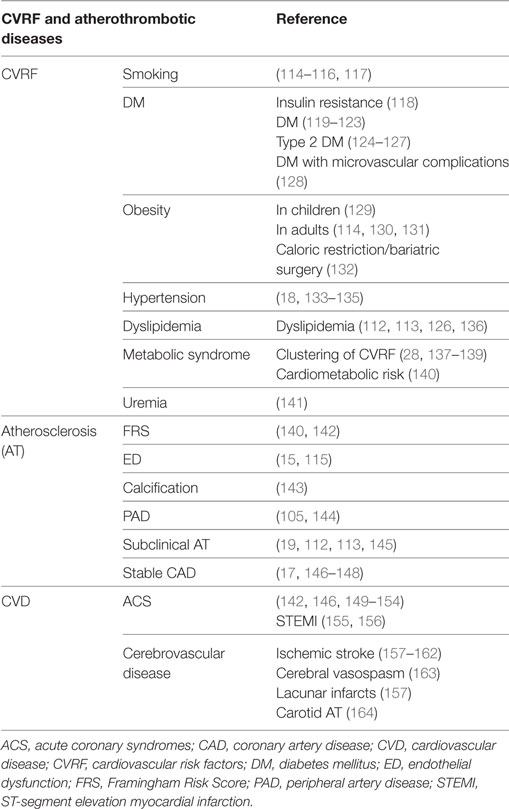
Table 1. Microvesicle-associated prognostic and diagnostic value in cardiovascular risk stratification and CVDs.
Atherosclerosis
Several studies have shown an association between cMVs and the Framingham Risk Score (used to predict CVD risk), ED, coronary calcification, peripheral artery disease, and subclinical AT (Table 1). Our group has recently reported that subjects at high cardiovascular risk who were about to develop a major CVE have increased levels of blood CD3+/CD45+ cMV (165). This is in agreement with our previous findings demonstrating that FH patients with subclinical lipid-rich atherosclerotic plaques have significantly higher levels blood-derived circulating ℓMVs than those with fibrous plaques (113). Besides, levels of circulating MVs have been found increased in patients with stable CAD, being MVs of endothelial origin (CD144+, CD31+/Annexin V+) and bearing miRNA-199a and miRNA-126 the most associated to major adverse CVEs (17, 146–148).
Acute Coronary Syndromes
Cell-derived cMV levels are profoundly increased in patients with ACS (Table 1). In a study in patients with STEMI, our group has demonstrated increased levels of pan-LMPs (CD45+), including lymphocytes (CD3+) and monocytes (CD14+) within the first hours of the ACS onset, with partial reductions after 72 h, likely because the inflammatory burst occurred at STEMI onset (155). These data are strongly supported by the fact that platelet- and monocyte-derived cMVs also associate with AMI severity (156) and CVD mortality (166), reflecting the sustained underlying endothelial injury and leukocyte and platelet activation in CVD progression after a CVE.
Cerebrovascular Disease
Circulating microvesicles have been associated with ischemic stroke, cerebral vasospasm, lacunar infarcts and multi-infarct dementia, and carotid AT (Table 1). Besides, a recent case-control pilot study suggests that circulating MVs derived from monocytes of M2 phenotype, but not those of M1-phenotype are significantly increased in patients with intracerebral hemorrhage within 12 h of symptom onset (167).
AT-Driven Angiogenesis and Neovascularization: MVs as Therapeutic Vectors
Angiogenesis is an active fine-tuning process of vessel sprouting and growth that depends on a precise interplay between stimulatory and inhibitory signals of ECs, SMCs, and pericytes (168). Notably, recent evidence outlines the importance of EC metabolism for angiogenesis in the context of atherogenesis and AT progression (169). Thus, angiogenic processes have severe consequences on vascular remodeling and plaque stability within vulnerable atherosclerotic plaques (plaque rupture and intra-plaque hemorrhage). The generation of new delicate and frail vessels within the growing atherosclerotic lesion contributes to increase the vulnerability of the plaque to rupture. Intriguingly, it is not fully understood whether an atherosclerotic plaque in the arterial tree will rupture and trigger thrombotic cascade or will stabilize. The highest accumulation of neovessels was found in ACS-related human coronary plaques (170). Paracrine intercellular communication exerted by MVs might play a role in the adaptive response of ischemic tissue to hypoxic stress caused by CVD (171). Interestingly, MVs have been postulated as both pro- and antiangiogenic factors (172–177) (Table 2). Indeed, low levels of eMVs-containing β1-integrin and the enzymatically active MMP-2 and MMP-9 showed to promote angiogenesis whereas high levels abolished the angiogenic effects (76). The effect of MVs on angiogenesis is also highly dependent on the quantity, the parental cell type and cell surface content.
Microvesicles from apoptotic ECs (24, 188), endothelial progenitor cells (EPCs) (188), platelets (172, 181, 182, 193, 194), skin wound myofibroblasts (187), and ischemic muscle (183) stimulate endothelial proliferation, survival, migration, repair, and tube formation in vitro by activating pro-angiogenic signaling cascades, such as ERK and PI3K/Akt pathways, or through upregulation of MMP-2 and MMP-9 expression in ECs (185) (Table 2). Leroyer et al. (186) elegantly demonstrated that carotid plaque MVs stimulate both endothelial proliferation and in vivo angiogenesis in a CD40 ligand-dependent manner, which could be modulated by the fibrinolytic activity of eMVs and LMVs (184). Indeed, eMVs play an important role in plasmin formation, which can influence in vitro the tube formation of EPCs in a dose-dependent manner (184).
Other cell-specific MVs have also shown proangiogenic potential (Table 2). pMVs from atherosclerotic patients increased the neovascularization capacity of circulating angiogenic cells through a RANTES-mediated mechanism (178). pMVs not only were shown to stimulate vascular endothelial growth factor (VEGF)-dependent revascularization after chronic cardiac ischemia (172) but also stem cell repair mechanisms after brain ischemia in rats by increasing angiogenesis and neurogenesis at the infarct zone (179). In addition, the remote conditioning protective effect of pMVs was further proved against cerebral ischemic reperfusion injury (180). Increased levels of MVs from ischemic muscle showed to promote postischemic neovascularization in mouse after hindlimb ischemia (HLI) (183). T-lymphocyte-derived ℓMVs enriched with the morphogen sonic hedgehog increased neoangiogenesis and restored endothelial function after injection in mice by stimulating the NO synthesis pathway (175, 195). Endothelial colony-forming cell-derived MVs were demonstrated to be pro-angiogenic both in vitro and in vivo through eNOS and the PI3K/AKT pathway (188). Moreover, MVs from bone marrow-derived mesenchymal stem cells (MSCs) showed proangiogenic activities in a rat MI model contributing to cardiac repair (189) and improved postischemic neuroangiogenesis in a stroke model (190) while MSC–derived MVs from umbilical cord enhanced tube-like structure development and further rescued blood flow in a rat model of HLI (191). Indeed, umbilical cord MSC-derived MVs were shown to enhance angiogenesis in a rat model of kidney ischemia (192), suggesting that MSC-derived MVs might exert cardioprotective effects.
In contrast to the findings reported above, placental MVs have been implicated in antiangiogenic processes contributing to impaired perfusion of placenta in patients with preeclampsia (196). In line with these studies, high levels of eMVs inhibited angiogenesis in the cultured segments of hearts by hampering endothelial nitric oxide synthase (eNOS) regulation (176). Oxidative stress is also involved in the antiangiogenic effect of MVs. Lymphocyte-MVs inhibited angiogenesis regulating negatively VEGF pathway (197) whereas eMVs inhibited in vitro angiogenesis by impairing acetylcholine-induced endothelial vasorelaxation and NO production in rat aortic rings (22, 173). Recently, eMVs were reported to inhibit capillary-like branch structure formation by microvascular ECs (mECs) in vitro and in vivo triggered by ROS via the expression of CD36 on the target EC (198).
Interestingly, the stimulating environment influencing the generation of MVs is able to switch their angiogenic phenotype. Treatment of MSCs with PDGF generated proangiogenic MVs (199), showing that the cargoes of MVs greatly impact on their effects. In agreement, cavin-2 that is released in eMVs and required for eMV biogenesis acts as a regulator of angiogenesis by producing NO and controlling the stability and activity of eNOS (200). Moreover, we found that C-reactive protein (CRP) is pro-angiogenic (201) and interestingly CRP is carried by circulating MVs in ischemic patients (202). eMVs from patients with chronic thromboembolic pulmonary hypertension increased EC endoglin concentration and stimulated endothelial angiogenesis showing a protective mechanism for ED and vascular occlusion (203). Of note, exercise intensity increases the levels of circulating pMVs with proangiogenic potential, which stimulate EC proliferation, migration, and tubule formation (204). Besides, antihypertensive drugs have shown to regulate both eMV generation and angiogenesis (205). Therefore, MVs might be considered as dual-purpose mediators of cell–cell communication in health and disease, greatly depending on the surrounding environment in which they are formed, their content and the pathophysiological context where they exert their functions.
Similarly, MVs from preconditioned adipose-derived stem cells (ASC) showed proangiogenic potential through the release of miR-31 targeting factor-inhibiting HIF-1 and enhancing ASC therapeutic efficacy (206). Indeed, miRNA delivery is a key mechanism involved in the effects of MV-driven angiogenesis such as EPC-MVs promoting neovascularization and impairing muscle damage after HLI (207) or inducing the survival of human islet transplants (208). Thus, eMVs released upon interleukin-3 activation transferred miRNA-126-3p and pSTAT5 into ECs thereby promoting angiogenesis (209). In this regard, MVs containing miRNA-126 stimulate reendothelialization after vascular injury (24) and administration of vesicles containing miR-126 decreased atherosclerotic plaque formation and favored plaque stability in mice (210). Besides, transfer of miRNA-150 to ECs by mMVs also promoted angiogenesis both in vitro and in vivo (117).
Tissue factor, the primary cellular initiator of blood coagulation, is also involved in angiogenic processes (211–213). Proangiogenic signaling through TF-dependent MV-mediated activation of PAR-2 has been reported in hypoxic ECs (214). Besides, TF+-MVs were shown to bind to β1-integrin in the surface of ECs to induce proliferation through ERK1/2 (215). In the same line, we have demonstrated that TF-containing eMVs from microvascular ECs (meMVs) interacted via paracrine signaling with other mECs and triggered angiogenesis ex vivo and postischemic collateral vessel growth in vivo (216). The meMVs proangiogenic potential was shown to be regulated by β1-integrin–EC interactions inducing Rac1–ERK1/2–ETS signaling and CCL2 production (Figure 4). Taken together, MVs can overwhelm the effects of arterial occlusion and tissue ischemia by stimulating postischemic neovascularization together with tissue reperfusion. Beyond being a promising therapeutic strategy for treating ischemic diseases (217), angiogenesis has also a role in the context of tumor progression and cancer, in which distinct types of MVs by means of cell-cell communication have also a regulatory function (14, 218–228). It is important to join efforts toward the ultimate goal of reaching therapeutical applications of MVs into the clinical arena.
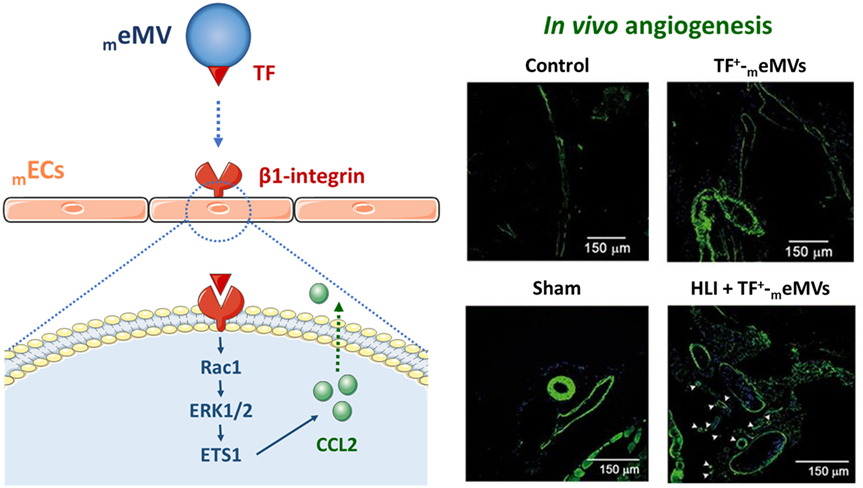
Figure 4. Microvesicle (MV)-mediated neovascularization. TF-positive microvascular endothelial-derived MVs (TF+-meMVs) were shown to interact with endothelial cell surface β1-integrin to induce a Rac1–ERK1/2–ETS signaling cascade that leads to CCL2 production and angiogenesis (216). Representative immunofluorescence images demonstrate that TF+-meMVs enhance collateral capillary formation and angiogenesis in vivo after ischemic hindlimbs femoral arteriectomy with antibody against α-actin (green) and nuclear staining (blue). HLI, hindlimb ischemia; meMV, microvascular endothelial-derived microvesicle; TF, tissue factor.
Pharmacological and Non-Pharmacological Intervention
A deep understanding of the role of MVs in AT might be fundamental for both CVD risk factor control and therapeutic treatment (130). Non-pharmacological interventions lie in overcoming CVRF by lifestyle modifications, such as exercise and diet. In primary prevention, a recent clinical trial showed the benefit of the adherence to the Mediterranean diet in patients at high cardiovascular risk in relation to the incidence of severe CVEs (229). Within the same population, our group has recently demonstrated that decreased levels of cMVs derived from ECs, leukocytes and activated platelets could signal for a reduced rate of major CVEs in high-risk patients under state-of-the-art treatment and receiving a controlled MedDiet supplemented with nuts (230). Dietary modulation of MV release is a relatively new field of study based mainly on short-term studies; further large-scale studies will help to better understand the complex relationship between diet and CVD.
Several drugs have shown to influence cMVs levels (231, 232), their cargoes (233) and even their function (Table 3). For instance, berberine improved endothelial function by reducing CD31+/CD42− eMVs and thereby levels of oxidative stress in humans (234, 235) and the adhesion of monocytes to ECs was partially prevented by eMVs after lipid-lowering and antihypertensive treatments (236). Since MV biogenesis and release is not fully understood, distinct therapeutic options are under investigation. Reduced levels of pMVs are associated with the use of distinct anti-platelet drugs such as GPIIb/IIIa inhibitors (237, 238), clopidogrel (239, 240), ticlopidine (121, 122), and acetylsalicylic acid (ASA) (241). In patients under antithrombotic therapy, P-Sel and TF-containing pMVs remain high 6 months after treatment initiation (242), likely due to the fact that low doses of ASA might not be potent enough to prevent the release of pMVs into the microcirculation (243). Our group has reported that ASA intake in patients with diabetes in primary prevention has no effect on pMVs (244). Similarly, antihypertensive drugs like angiotensin II receptor antagonists (245) and calcium channel blockers (246), antioxidants (247), peroxisome proliferator-activator receptor activators (248, 249), hydroxyurea in sickle cell disease (250), and eculizumab in paroxysmal nocturnal hemoglobinuria (251) have also shown influence on MV shedding. Up to now the effect of statins, the cornerstone drugs for lipid-lowering treatment (LLT) in CVD prevention, has been highly debated. While some authors demonstrated that statins may enhance the shedding of MVs (252, 253) many studies have found that statin treatment promote MV inhibition (88, 135, 254–256). Thus, pravastatin and simvastatin decreased pMVs in hypertensive (257) and type-2 diabetic (88, 120) patients. Similarly, atorvastatin diminished the formation of thrombin and the expression of P-Sel, TF, and GPIIIa on pMVs in patients with peripheral artery disease (233) and with dyslipidemia and type-1 diabetes (258). Indeed, in a study focused on the effects of LLT on levels of cMV in atherosclerotic patients in primary prevention, we have reported that the plasma of LLT-treated patients presented lower quantity of MVs and lower content of cell surface activation markers than the plasma from untreated patients with the same blood cholesterol levels (255), indicating a direct benefit of LLT with statins in reducing cell membrane shedding, which may have effects in the beneficial protection against AT characteristic of statins by inhibiting MV generation and the triggering of MV-dependent mechanisms. These data are in agreement to results pointing out the broader use of statins decreasing inflammation and suppressing MV release, an effect that is not shown neither with ezetimibe alone (259) nor with ezetimibe combined with statins (260). Furthermore, several inhibitors of MV shedding such as ROCK inhibitors or calpain, among others, are currently under study. Nevertheless, whether the clinical benefit of these pharmacological strategies is directly related to MV decrease deserves further research.
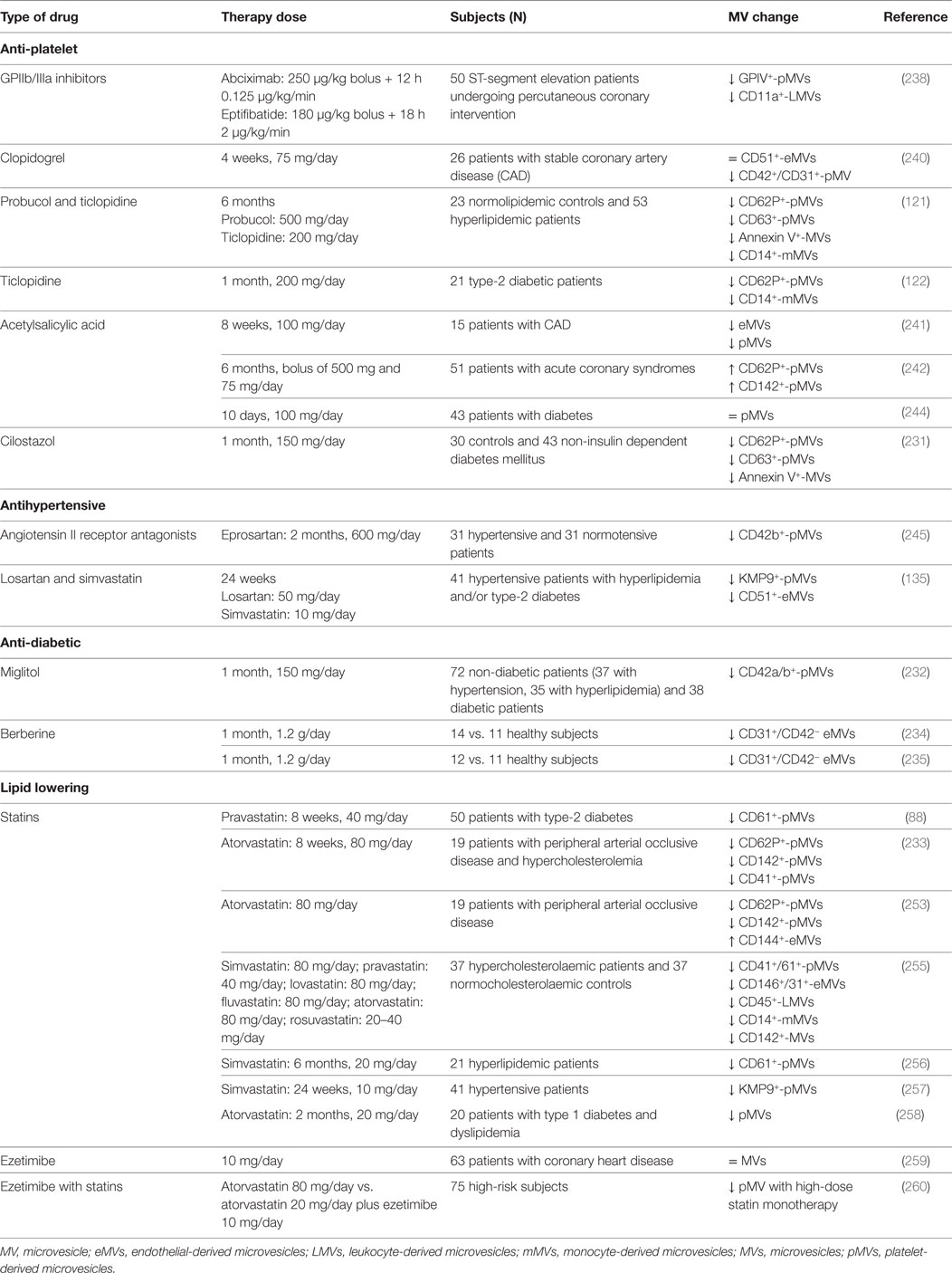
Table 3. Main studies evaluating the effects of pharmacological therapies on circulating microvesicles.
Therapeutic Potential
In addition to pharmacological modulation, the advantageous characteristics of cell-derived MVs, which are a naturally produced therapeutic agents with potential to be used as delivery drugs to specific cell types (261), open up their potential therapeutic application, especially in cardiac cell therapy. Preclinical studies demonstrated that treatment with the vesicular fraction of the conditioned media of hypoxic MSCs decreased infarct size and improved cardiac function by decreasing oxidative stress, enhancing myocardial viability, and preventing damage to the heart after MI in mouse and pig models (262–264). The mechanism of action might involve the transfer of specific RNAs through embryonic stem cell MVs (265). MSC-derived MVs were able to face the detrimental effects of ischemia and reperfusion (I/R) injury in the kidney (266). Similarly, MVs derived from myocardial ischemia could protect hearts from I/R injury in rats through calcium regulatory proteins to alleviate intrinsic myocardial mitochondrial and endoplasmic reticulum apoptotic pathways (267). Interestingly, the effect of cardiosphere-derived cells (CDC) on the therapeutic regeneration of the infarcted myocardium shown in a clinical trial (268) seems to be mediated by CDC-derived extracellular vesicles (269). ECs have also been shown to be atheroprotective by transferring miRNAs via MVs to SMCs (81). Specifically, MVs transported functional miR-143/145 into SMCs after activation by shear stress and they reduced atherosclerotic lesion formation in the aorta. Furthermore, a promising therapeutic application of MVs is the use of synthetic MVs mimicking the natural ones. MV delivery could be clinically useful in several conditions such as MI and inflammatory pathologies. Indeed, a recent work reported that infusion of artificially produced MVs can improve inflammation and ameliorate symptoms in different mouse models of MI, multiple sclerosis, and kidney injury (270). Therapeutic innovation of MVs is still in its infancy, and its applicability is hampered by many shortcomings, such as the in vivo biodistribution of MVs that depends on their cellular origin, their half-life and the route of administration (271). Further understanding of the biological mechanisms, efficiency and feasibility of these MV-based therapies is warranted.
Conclusion and Future Perspectives
Microvesicles actively contribute to AT progression and complication due to their implicit role in cell-to-cell communication. cMVs not only play a direct biological role in AT and neoangiogenensis but also might be susceptible targets for pharmacological modulation and emerge as potential prognostic and diagnostic biomarkers of atherothrombotic CVD. However, limitations and technological constraints have precluded the complete understanding of mechanisms of MV formation and pathophysiological relevance. Most functional effects of MVs have been evidenced in in vitro studies with a predetermined MV concentration that is not always comparable to the pathophysiological situation while their exact role in the clinical setting is dismissed. Despite cMVs emerge as promising biomarker candidates, clinical studies in larger cohorts are required to clearly delineate their role as diagnostic and prognostic markers of disease. Until now, the lack of standardization in preanalytical phase guidelines, the difficult implementation as routine in the clinical laboratory as well as the high cost of cMV measurements have curbed their full clinical characterization. However, recent and advanced sensitive technologies together with consensus within the scientific community will undoubtedly shed light on the cMV potential as biomarkers. Besides, exploring the cMV specific targeting to a selective tissue, how they are produced in vivo and their specific genomic, transcriptomic, metabolomic and proteomic content is essential to design efficient therapeutically strategies involving MVs. In the next coming years, we will witness advances and breakthroughs in the area of MVs that will translate into their use in diagnostic and therapeutic innovation.
Author Contributions
LB, RS, and TP conceived and coordinated the design of the review and wrote the paper. RS made figures. LB and RS edited the paper. All the authors wrote part of the manuscript, provided critical comments, revised the manuscript, and approved the final version of the manuscript.
Conflict of Interest Statement
The authors declare that the research was conducted in the absence of any commercial or financial relationships that could be construed as a potential conflict of interest.
Acknowledgments
The continuous support of Fundación Investigación Cardiovascular—F. Jesus Serra (Spain) to the Cardiovascular Research Chair-UAB (LB) is gratefully acknowledged.
Funding
This work was supported by the Spanish Ministry of Economy and Competitiveness Plan Estatal I+D+I [SAF2016-76819-R], the Institute of Health Carlos III-ISCIII [CIBERCV CN16/11/00411 and TERCEL RD16/0011/018 to LB, FIS PI16/01915 to TP], and by the Fondo Europeo de Desarrollo Regional (FEDER—“Una manera de hacer Europa”). The authors thank the continuous support of the Generalitat of Catalunya (Secretaria d’Universitats i Recerca del Departament d’Economia i Coneixement de la Generalitat; 2014SGR1303). RS is a postdoctoral fellow funded by Daniel Bravo Andreu Foundation.
Abbreviations
AT, atherosclerosis; CAD, coronary artery disease; cMV, circulating microvesicle; CVD, cardiovascular disease; CVE, cardiovascular event; EC, endothelial cell; eMV, endothelial-derived microvesicle; EPC, endothelial progenitor cell; ErMV, erythrocyte-derived microvesicle; ICAM, intercellular cell adhesion molecule; I/R, ischemia and reperfusion; LDL, low-density lipoprotein; ℓMV, lymphocyte-derived microvesicle; LMV, leukocyte-derived microvesicle; LLT, lipid-lowering treatment; mEC, microvascular endothelial cell; meMV, microvascular endothelial-derived microvesicle; MetDiet, Mediterranean diet; miRNA, microRNA; MMP, matrix metalloproteinase; mMV, monocyte-derived microvesicle; MP, microparticle; MSC, mesenchymal stem cell; MV, microvesicle; NO, nitric oxide; pMV, platelet-derived microvesicle; PS, phosphatidylserine; RNA, ribonucleic acid; ROS, reactive oxygen species; SMC, smooth muscle cell; TF, tissue factor; T2D, type 2 diabetes; VCAM, vascular cell adhesion molecule.
References
1. Santos-Gallego CG, Picatoste B, Badimon JJ. Pathophysiology of acute coronary syndrome. Curr Atheroscler Rep (2014) 16:401. doi:10.1007/s11883-014-0401-9
2. Suades R, Padro T, Badimon L. The role of blood-borne microparticles in inflammation and hemostasis. Semin Thromb Hemost (2015) 41:590–606. doi:10.1055/s-0035-1556591
3. Badimon L, Suades R, Fuentes E, Palomo I, Padro T. Role of platelet-derived microvesicles as crosstalk mediators in atherothrombosis and future pharmacology targets: a link between inflammation, atherosclerosis, and thrombosis. Front Pharmacol (2016) 7:293. doi:10.3389/fphar.2016.00293
4. van der pol E, Boing AN, Gool EL, Nieuwland R. Recent developments in the nomenclature, presence, isolation, detection and clinical impact of extracellular vesicles. J Thromb Haemost (2016) 14:48–56. doi:10.1111/jth.13190
5. Zhang Y, Liu D, Chen X, Li J, Li L, Bian Z, et al. Secreted monocytic miR-150 enhances targeted endothelial cell migration. Mol Cell (2010) 39:133–44. doi:10.1016/j.molcel.2010.06.010
6. Connor DE, Exner T, Ma DD, Joseph JE. The majority of circulating platelet-derived microparticles fail to bind annexin V, lack phospholipid-dependent procoagulant activity and demonstrate greater expression of glycoprotein Ib. Thromb Haemost (2010) 103:1044–52. doi:10.1160/TH09-09-0644
7. Arraud N, Linares R, Tan S, Gounou C, Pasquet JM, Mornet S, et al. Extracellular vesicles from blood plasma: determination of their morphology, size, phenotype and concentration. J Thromb Haemost (2014) 12:614–27. doi:10.1111/jth.12554
8. Gaceb A, Martinez MC, Andriantsitohaina R. Extracellular vesicles: new players in cardiovascular diseases. Int J Biochem Cell Biol (2014) 50:24–8. doi:10.1016/j.biocel.2014.01.018
9. Coumans FAW, Gool EL, Nieuwland R. Bulk immunoassays for analysis of extracellular vesicles. Platelets (2017) 28:242–8. doi:10.1080/09537104.2016.1265926
10. Coumans FAW, Brisson AR, Buzas EI, Dignat-George F, Drees EEE, El-Andaloussi S, et al. Methodological guidelines to study extracellular vesicles. Circ Res (2017) 120:1632–48. doi:10.1161/CIRCRESAHA.117.309417
11. Ridger VC, Boulanger CM, Angelillo-Scherrer A, Badimon L, Blanc-Brude O, Bochaton-Piallat ML, et al. Microvesicles in vascular homeostasis and diseases. Position Paper of the European Society of Cardiology (ESC) working group on atherosclerosis and vascular biology. Thromb Haemost (2017) 117:1296–316. doi:10.1160/TH16-12-0943
12. Szatanek R, Baj-Krzyworzeka M, Zimoch J, Lekka M, Siedlar M, Baran J. The methods of choice for extracellular vesicles (EVs) characterization. Int J Mol Sci (2017) 18(6):ii:E1153. doi:10.3390/ijms18061153
13. Valkonen S, Van Der Pol E, Boing A, Yuana Y, Yliperttula M, Nieuwland R, et al. Biological reference materials for extracellular vesicle studies. Eur J Pharm Sci (2017) 98:4–16. doi:10.1016/j.ejps.2016.09.008
14. Skog J, Wurdinger T, Van Rijn S, Meijer DH, Gainche L, Sena-Esteves M, et al. Glioblastoma microvesicles transport RNA and proteins that promote tumour growth and provide diagnostic biomarkers. Nat Cell Biol (2008) 10:1470–6. doi:10.1038/ncb1800
15. Amabile N, Guerin AP, Leroyer A, Mallat Z, Nguyen C, Boddaert J, et al. Circulating endothelial microparticles are associated with vascular dysfunction in patients with end-stage renal failure. J Am Soc Nephrol (2005) 16:3381–8. doi:10.1681/ASN.2005050535
16. Lin ZB, Ci HB, Li Y, Cheng TP, Liu DH, Wang YS, et al. Endothelial microparticles are increased in congenital heart diseases and contribute to endothelial dysfunction. J Transl Med (2017) 15:4. doi:10.1186/s12967-016-1087-2
17. Werner N, Wassmann S, Ahlers P, Kosiol S, Nickenig G. Circulating CD31+/annexin V+ apoptotic microparticles correlate with coronary endothelial function in patients with coronary artery disease. Arterioscler Thromb Vasc Biol (2006) 26:112–6. doi:10.1161/01.ATV.0000191634.13057.15
18. Chen Y, Feng B, Li X, Ni Y, Luo Y. Plasma endothelial microparticles and their correlation with the presence of hypertension and arterial stiffness in patients with type 2 diabetes. J Clin Hypertens (Greenwich) (2012) 14:455–60. doi:10.1111/j.1751-7176.2012.00631.x
19. Feng B, Chen Y, Luo Y, Chen M, Li X, Ni Y. Circulating level of microparticles and their correlation with arterial elasticity and endothelium-dependent dilation in patients with type 2 diabetes mellitus. Atherosclerosis (2010) 208:264–9. doi:10.1016/j.atherosclerosis.2009.06.037
20. Hu SS, Zhang HG, Zhang QJ, Xiu RJ. CD51+ endothelial microparticles as a biomarker of endothelial dysfunction in obese patients with hypertension. Endocrine (2015) 49:283–5. doi:10.1007/s12020-014-0423-7
21. Martin S, Tesse A, Hugel B, Martinez MC, Morel O, Freyssinet JM, et al. Shed membrane particles from T lymphocytes impair endothelial function and regulate endothelial protein expression. Circulation (2004) 109:1653–9. doi:10.1161/01.CIR.0000124065.31211.6E
22. Brodsky SV, Zhang F, Nasjletti A, Goligorsky MS. Endothelium-derived microparticles impair endothelial function in vitro. Am J Physiol Heart Circ Physiol (2004) 286:H1910–5. doi:10.1152/ajpheart.01172.2003
23. Densmore JC, Signorino PR, Ou J, Hatoum OA, Rowe JJ, Shi Y, et al. Endothelium-derived microparticles induce endothelial dysfunction and acute lung injury. Shock (2006) 26:464–71. doi:10.1097/01.shk.0000228791.10550.36
24. Jansen F, Yang X, Hoelscher M, Cattelan A, Schmitz T, Proebsting S, et al. Endothelial microparticle-mediated transfer of microRNA-126 promotes vascular endothelial cell repair via SPRED1 and is abrogated in glucose-damaged endothelial microparticles. Circulation (2013) 128:2026–38. doi:10.1161/CIRCULATIONAHA.113.001720
25. Safiedeen Z, Rodriguez-Gomez I, Vergori L, Soleti R, Vaithilingam D, Douma I, et al. Temporal cross talk between endoplasmic reticulum and mitochondria regulates oxidative stress and mediates microparticle-induced endothelial dysfunction. Antioxid Redox Signal (2017) 26:15–27. doi:10.1089/ars.2016.6771
26. Ishida K, Taguchi K, Hida M, Watanabe S, Kawano K, Matsumoto T, et al. Circulating microparticles from diabetic rats impair endothelial function and regulate endothelial protein expression. Acta Physiol (Oxf) (2016) 216:211–20. doi:10.1111/apha.12561
27. Boulanger CM, Scoazec A, Ebrahimian T, Henry P, Mathieu E, Tedgui A, et al. Circulating microparticles from patients with myocardial infarction cause endothelial dysfunction. Circulation (2001) 104:2649–52. doi:10.1161/hc4701.100516
28. Agouni A, Lagrue-Lak-Hal AH, Ducluzeau PH, Mostefai HA, Draunet-Busson C, Leftheriotis G, et al. Endothelial dysfunction caused by circulating microparticles from patients with metabolic syndrome. Am J Pathol (2008) 173:1210–9. doi:10.2353/ajpath.2008.080228
29. Fu L, Hu XX, Lin ZB, Chang FJ, Ou ZJ, Wang ZP, et al. Circulating microparticles from patients with valvular heart disease and cardiac surgery inhibit endothelium-dependent vasodilation. J Thorac Cardiovasc Surg (2015) 150:666–72. doi:10.1016/j.jtcvs.2015.05.069
30. Ye S, Shan XF, Han WQ, Zhang QR, Gao J, Jin AP, et al. Microparticles from patients undergoing percutaneous coronary intervention impair vasodilatation by uncoupling endothelial nitric oxide synthase. Shock (2017) 48:201–8. doi:10.1097/SHK.0000000000000823
31. Abbas M, Jesel L, Auger C, Amoura L, Messas N, Manin G, et al. Endothelial microparticles from acute coronary syndrome patients induce premature coronary artery endothelial cell aging and thrombogenicity: role of the Ang II/AT1 receptor/NADPH oxidase-mediated activation of MAPKs and PI3-kinase pathways. Circulation (2017) 135:280–96. doi:10.1161/CIRCULATIONAHA.116.017513
32. Horn P, Cortese-Krott MM, Amabile N, Hundsdorfer C, Kroncke KD, Kelm M, et al. Circulating microparticles carry a functional endothelial nitric oxide synthase that is decreased in patients with endothelial dysfunction. J Am Heart Assoc (2012) 2:e003764. doi:10.1161/JAHA.112.003764
33. Mahmoud AM, Wilkinson FL, Mccarthy EM, Moreno-Martinez D, Langford-Smith A, Romero M, et al. Endothelial microparticles prevent lipid-induced endothelial damage via Akt/eNOS signaling and reduced oxidative stress. FASEB J (2017) 31:4636–48. doi:10.1096/fj.201601244RR
34. Edrissi H, Schock SC, Hakim AM, Thompson CS. Microparticles generated during chronic cerebral ischemia increase the permeability of microvascular endothelial barriers in vitro. Brain Res (2016) 1634:83–93. doi:10.1016/j.brainres.2015.12.032
35. Mesri M, Altieri DC. Leukocyte microparticles stimulate endothelial cell cytokine release and tissue factor induction in a JNK1 signaling pathway. J Biol Chem (1999) 274:23111–8. doi:10.1074/jbc.274.33.23111
36. Nomura S, Tandon NN, Nakamura T, Cone J, Fukuhara S, Kambayashi J. High-shear-stress-induced activation of platelets and microparticles enhances expression of cell adhesion molecules in THP-1 and endothelial cells. Atherosclerosis (2001) 158:277–87. doi:10.1016/S0021-9150(01)00433-6
37. Barry OP, Pratico D, Savani RC, Fitzgerald GA. Modulation of monocyte-endothelial cell interactions by platelet microparticles. J Clin Invest (1998) 102:136–44. doi:10.1172/JCI2592
38. Mesri M, Altieri DC. Endothelial cell activation by leukocyte microparticles. J Immunol (1998) 161:4382–7.
39. Meziani F, Delabranche X, Asfar P, Toti F. Bench-to-bedside review: circulating microparticles – a new player in sepsis? Crit Care (2010) 14:236. doi:10.1186/cc9231
40. Wang JG, Williams JC, Davis BK, Jacobson K, Doerschuk CM, Ting JP, et al. Monocytic microparticles activate endothelial cells in an IL-1beta-dependent manner. Blood (2011) 118:2366–74. doi:10.1182/blood-2011-01-330878
41. Heinrich LF, Andersen DK, Cleasby ME, Lawson C. Long-term high fat feeding of rats results in increased numbers of circulating microvesicles with pro-inflammatory effects on endothelial cells. Br J Nutr (2015) 113:1704–11. doi:10.1017/S0007114515001117
42. Curtis AM, Wilkinson PF, Gui M, Gales TL, Hu E, Edelberg JM. p38 mitogen-activated protein kinase targets the production of proinflammatory endothelial microparticles. J Thromb Haemost (2009) 7:701–9. doi:10.1111/j.1538-7836.2009.03304.x
43. Fink K, Moebes M, Vetter C, Bourgeois N, Schmid B, Bode C, et al. Selenium prevents microparticle-induced endothelial inflammation in patients after cardiopulmonary resuscitation. Crit Care (2015) 19:58. doi:10.1186/s13054-015-0774-3
44. Jansen F, Yang X, Baumann K, Przybilla D, Schmitz T, Flender A, et al. Endothelial microparticles reduce ICAM-1 expression in a microRNA-222-dependent mechanism. J Cell Mol Med (2015) 19:2202–14. doi:10.1111/jcmm.12607
45. Huber J, Vales A, Mitulovic G, Blumer M, Schmid R, Witztum JL, et al. Oxidized membrane vesicles and blebs from apoptotic cells contain biologically active oxidized phospholipids that induce monocyte-endothelial interactions. Arterioscler Thromb Vasc Biol (2002) 22:101–7. doi:10.1161/hq0102.101525
46. Mause SF, Von Hundelshausen P, Zernecke A, Koenen RR, Weber C. Platelet microparticles: a transcellular delivery system for RANTES promoting monocyte recruitment on endothelium. Arterioscler Thromb Vasc Biol (2005) 25:1512–8. doi:10.1161/01.ATV.0000170133.43608.37
47. Rautou PE, Leroyer AS, Ramkhelawon B, Devue C, Duflaut D, Vion AC, et al. Microparticles from human atherosclerotic plaques promote endothelial ICAM-1-dependent monocyte adhesion and transendothelial migration. Circ Res (2011) 108:335–43. doi:10.1161/CIRCRESAHA.110.237420
48. Njock MS, Cheng HS, Dang LT, Nazari-Jahantigh M, Lau AC, Boudreau E, et al. Endothelial cells suppress monocyte activation through secretion of extracellular vesicles containing antiinflammatory microRNAs. Blood (2015) 125:3202–12. doi:10.1182/blood-2014-11-611046
49. Forlow SB, Mcever RP, Nollert MU. Leukocyte-leukocyte interactions mediated by platelet microparticles under flow. Blood (2000) 95(4):1317–23.
50. Keyel PA, Tkacheva OA, Larregina AT, Salter RD. Coordinate stimulation of macrophages by microparticles and TLR ligands induces foam cell formation. J Immunol (2012) 189:4621–9. doi:10.4049/jimmunol.1200828
51. Lee SK, Yang SH, Kwon I, Lee OH, Heo JH. Role of tumour necrosis factor receptor-1 and nuclear factor-kappaB in production of TNF-alpha-induced pro-inflammatory microparticles in endothelial cells. Thromb Haemost (2014) 112:580–8. doi:10.1160/TH13-11-0975
52. Abid Hussein MN, Nieuwland R, Hau CM, Evers LM, Meesters EW, Sturk A. Cell-derived microparticles contain caspase 3 in vitro and in vivo. J Thromb Haemost (2005) 3:888–96. doi:10.1111/j.1538-7836.2005.01240.x
53. Distler JH, Huber LC, Hueber AJ, Reich CF III, Gay S, Distler O, et al. The release of microparticles by apoptotic cells and their effects on macrophages. Apoptosis (2005) 10:731–41. doi:10.1007/s10495-005-2941-5
54. Huber LC, Jungel A, Distler JH, Moritz F, Gay RE, Michel BA, et al. The role of membrane lipids in the induction of macrophage apoptosis by microparticles. Apoptosis (2007) 12:363–74. doi:10.1007/s10495-006-0622-7
55. Boing AN, Hau CM, Sturk A, Nieuwland R. Platelet microparticles contain active caspase 3. Platelets (2008) 19:96–103. doi:10.1080/09537100701777295
56. Sarkar A, Mitra S, Mehta S, Raices R, Wewers MD. Monocyte derived microvesicles deliver a cell death message via encapsulated caspase-1. PLoS One (2009) 4:e7140. doi:10.1371/journal.pone.0007140
57. Leroyer AS, Isobe H, Leseche G, Castier Y, Wassef M, Mallat Z, et al. Cellular origins and thrombogenic activity of microparticles isolated from human atherosclerotic plaques. J Am Coll Cardiol (2007) 49:772–7. doi:10.1016/j.jacc.2006.10.053
58. Mayr M, Grainger D, Mayr U, Leroyer AS, Leseche G, Sidibe A, et al. Proteomics, metabolomics, and immunomics on microparticles derived from human atherosclerotic plaques. Circ Cardiovasc Genet (2009) 2:379–88. doi:10.1161/CIRCGENETICS.108.842849
59. Niessen A, Heyder P, Krienke S, Blank N, Tykocinski LO, Lorenz HM, et al. Apoptotic-cell-derived membrane microparticles and IFN-alpha induce an inflammatory immune response. J Cell Sci (2015) 128:2443–53. doi:10.1242/jcs.162735
60. Classen L, Tykocinski LO, Wiedmann F, Birr C, Schiller P, Tucher C, et al. Extracellular vesicles mediate intercellular communication: transfer of functionally active microRNAs by microvesicles into phagocytes. Eur J Immunol (2017) 47(9):1535–49. doi:10.1002/eji.201646595
61. Llorente-Cortes V, Otero-Vinas M, Camino-Lopez S, Llampayas O, Badimon L. Aggregated low-density lipoprotein uptake induces membrane tissue factor procoagulant activity and microparticle release in human vascular smooth muscle cells. Circulation (2004) 110:452–9. doi:10.1161/01.CIR.0000136032.40666.3D
62. Pakala R. Serotonin and thromboxane A2 stimulate platelet-derived microparticle-induced smooth muscle cell proliferation. Cardiovasc Radiat Med (2004) 5:20–6. doi:10.1016/j.carrad.2003.12.002
63. Weber A, Koppen HO, Schror K. Platelet-derived microparticles stimulate coronary artery smooth muscle cell mitogenesis by a PDGF-independent mechanism. Thromb Res (2000) 98:461–6. doi:10.1016/S0049-3848(00)00192-4
64. Kelly-Arnold A, Maldonado N, Laudier D, Aikawa E, Cardoso L, Weinbaum S. Revised microcalcification hypothesis for fibrous cap rupture in human coronary arteries. Proc Natl Acad Sci U S A (2013) 110:10741–6. doi:10.1073/pnas.1308814110
65. Badimon L, Vilahur G. Thrombosis formation on atherosclerotic lesions and plaque rupture. J Intern Med (2014) 276:618–32. doi:10.1111/joim.12296
66. Sangiorgi G, Rumberger JA, Severson A, Edwards WD, Gregoire J, Fitzpatrick LA, et al. Arterial calcification and not lumen stenosis is highly correlated with atherosclerotic plaque burden in humans: a histologic study of 723 coronary artery segments using nondecalcifying methodology. J Am Coll Cardiol (1998) 31:126–33. doi:10.1016/S0735-1097(97)00443-9
67. Otsuka F, Sakakura K, Yahagi K, Joner M, Virmani R. Has our understanding of calcification in human coronary atherosclerosis progressed? Arterioscler Thromb Vasc Biol (2014) 34:724–36. doi:10.1161/ATVBAHA.113.302642
68. Huang H, Virmani R, Younis H, Burke AP, Kamm RD, Lee RT. The impact of calcification on the biomechanical stability of atherosclerotic plaques. Circulation (2001) 103:1051–6. doi:10.1161/01.CIR.103.8.1051
69. Hsu JJ, Lim J, Tintut Y, Demer LL. Cell-matrix mechanics and pattern formation in inflammatory cardiovascular calcification. Heart (2016) 102:1710–5. doi:10.1136/heartjnl-2016-309667
70. Reynolds JL, Joannides AJ, Skepper JN, Mcnair R, Schurgers LJ, Proudfoot D, et al. Human vascular smooth muscle cells undergo vesicle-mediated calcification in response to changes in extracellular calcium and phosphate concentrations: a potential mechanism for accelerated vascular calcification in ESRD. J Am Soc Nephrol (2004) 15:2857–67. doi:10.1097/01.ASN.0000141960.01035.28
71. New SE, Goettsch C, Aikawa M, Marchini JF, Shibasaki M, Yabusaki K, et al. Macrophage-derived matrix vesicles: an alternative novel mechanism for microcalcification in atherosclerotic plaques. Circ Res (2013) 113:72–7. doi:10.1161/CIRCRESAHA.113.301036
72. Buendia P, Montes De Oca A, Madueno JA, Merino A, Martin-Malo A, Aljama P, et al. Endothelial microparticles mediate inflammation-induced vascular calcification. FASEB J (2015) 29:173–81. doi:10.1096/fj.14-249706
73. Goettsch C, Hutcheson JD, Aikawa M, Iwata H, Pham T, Nykjaer A, et al. Sortilin mediates vascular calcification via its recruitment into extracellular vesicles. J Clin Invest (2016) 126:1323–36. doi:10.1172/JCI80851
74. Gasser O, Hess C, Miot S, Deon C, Sanchez JC, Schifferli JA. Characterisation and properties of ectosomes released by human polymorphonuclear neutrophils. Exp Cell Res (2003) 285:243–57. doi:10.1016/S0014-4827(03)00055-7
75. Folkesson M, Li C, Frebelius S, Swedenborg J, Wagsater D, Williams KJ, et al. Proteolytically active ADAM10 and ADAM17 carried on membrane microvesicles in human abdominal aortic aneurysms. Thromb Haemost (2015) 114:1165–74. doi:10.1160/TH14-10-0899
76. Taraboletti G, D’Ascenzo S, Borsotti P, Giavazzi R, Pavan A, Dolo V. Shedding of the matrix metalloproteinases MMP-2, MMP-9, and MT1-MMP as membrane vesicle-associated components by endothelial cells. Am J Pathol (2002) 160:673–80. doi:10.1016/S0002-9440(10)64887-0
77. Lozito TP, Tuan RS. Endothelial cell microparticles act as centers of matrix metalloproteinsase-2 (MMP-2) activation and vascular matrix remodeling. J Cell Physiol (2012) 227:534–49. doi:10.1002/jcp.22744
78. Martinez de Lizarrondo S, Roncal C, Calvayrac O, Rodriguez C, Varo N, Purroy A, et al. Synergistic effect of thrombin and CD40 ligand on endothelial matrix metalloproteinase-10 expression and microparticle generation in vitro and in vivo. Arterioscler Thromb Vasc Biol (2012) 32:1477–87. doi:10.1161/ATVBAHA.112.248773
79. Canault M, Leroyer AS, Peiretti F, Leseche G, Tedgui A, Bonardo B, et al. Microparticles of human atherosclerotic plaques enhance the shedding of the tumor necrosis factor-alpha converting enzyme/ADAM17 substrates, tumor necrosis factor and tumor necrosis factor receptor-1. Am J Pathol (2007) 171:1713–23. doi:10.2353/ajpath.2007.070021
80. Bobryshev YV, Killingsworth MC, Orekhov AN. Increased shedding of microvesicles from intimal smooth muscle cells in athero-prone areas of the human aorta: implications for understanding of the predisease stage. Pathobiology (2013) 80:24–31. doi:10.1159/000339430
81. Hergenreider E, Heydt S, Treguer K, Boettger T, Horrevoets AJ, Zeiher AM, et al. Atheroprotective communication between endothelial cells and smooth muscle cells through miRNAs. Nat Cell Biol (2012) 14:249–56. doi:10.1038/ncb2441
82. Shan Z, Qin S, Li W, Wu W, Yang J, Chu M, et al. An endocrine genetic signal between blood cells and vascular smooth muscle cells: role of microRNA-223 in smooth muscle function and atherogenesis. J Am Coll Cardiol (2015) 65:2526–37. doi:10.1016/j.jacc.2015.03.570
83. Mallat Z, Hugel B, Ohan J, Leseche G, Freyssinet JM, Tedgui A. Shed membrane microparticles with procoagulant potential in human atherosclerotic plaques: a role for apoptosis in plaque thrombogenicity. Circulation (1999) 99:348–53. doi:10.1161/01.CIR.99.3.348
84. Wolf P. The nature and significance of platelet products in human plasma. Br J Haematol (1967) 13:269–88. doi:10.1111/j.1365-2141.1967.tb08741.x
85. Sinauridze EI, Kireev DA, Popenko NY, Pichugin AV, Panteleev MA, Krymskaya OV, et al. Platelet microparticle membranes have 50- to 100-fold higher specific procoagulant activity than activated platelets. Thromb Haemost (2007) 97(3):425–34. doi:10.1160/TH06-06-0313
86. Nomura S, Komiyama Y, Murakami T, Funatsu A, Kokawa T, Sugo T, et al. Flow cytometric analysis of surface membrane proteins on activated platelets and platelet-derived microparticles from healthy and thrombasthenic individuals. Int J Hematol (1993) 58:203–12.
87. Falati S, Liu Q, Gross P, Merrill-Skoloff G, Chou J, Vandendries E, et al. Accumulation of tissue factor into developing thrombi in vivo is dependent upon microparticle P-selectin glycoprotein ligand 1 and platelet P-selectin. J Exp Med (2003) 197:1585–98. doi:10.1084/jem.20021868
88. Sommeijer DW, Joop K, Leyte A, Reitsma PH, Ten Cate H. Pravastatin reduces fibrinogen receptor gpIIIa on platelet-derived microparticles in patients with type 2 diabetes. J Thromb Haemost (2005) 3:1168–71. doi:10.1111/j.1538-7836.2005.01403.x
89. Raturi A, Miersch S, Hudson JW, Mutus B. Platelet microparticle-associated protein disulfide isomerase promotes platelet aggregation and inactivates insulin. Biochim Biophys Acta (2008) 1778:2790–6. doi:10.1016/j.bbamem.2008.07.003
90. Scholz T, Temmler U, Krause S, Heptinstall S, Losche W. Transfer of tissue factor from platelets to monocytes: role of platelet-derived microvesicles and CD62P. Thromb Haemost (2002) 88(6):1033–8.
91. Del Conde I, Shrimpton CN, Thiagarajan P, Lopez JA. Tissue-factor-bearing microvesicles arise from lipid rafts and fuse with activated platelets to initiate coagulation. Blood (2005) 106:1604–11. doi:10.1182/blood-2004-03-1095
92. Collier M, Collier MEW, Ettelaie C, Goult BT, Maraveyas A, Goodall AH. Investigation of the filamin A-dependent mechanisms of tissue factor incorporation into microvesicles. Thromb Haemost (2017) 117(11):2034–44. doi:10.1160/TH17-01-0009
93. Boden G, Rao AK. Effects of hyperglycemia and hyperinsulinemia on the tissue factor pathway of blood coagulation. Curr Diab Rep (2007) 7:223–7. doi:10.1007/s11892-007-0035-1
94. Tsimerman G, Roguin A, Bachar A, Melamed E, Brenner B, Aharon A. Involvement of microparticles in diabetic vascular complications. Thromb Haemost (2011) 106:310–21. doi:10.1160/TH10-11-0712
95. Stojkovic S, Thulin A, Hell L, Thaler B, Rauscher S, Baumgartner J, et al. IL-33 stimulates the release of procoagulant microvesicles from human monocytes and differentially increases tissue factor in human monocyte subsets. Thromb Haemost (2017) 117:1379–90. doi:10.1160/TH16-10-0784
96. Merten M, Pakala R, Thiagarajan P, Benedict CR. Platelet microparticles promote platelet interaction with subendothelial matrix in a glycoprotein IIb/IIIa-dependent mechanism. Circulation (1999) 99:2577–82. doi:10.1161/01.CIR.99.19.2577
97. Suades R, Padro T, Vilahur G, Badimon L. Circulating and platelet-derived microparticles in human blood enhance thrombosis on atherosclerotic plaques. Thromb Haemost (2012) 108:1208–19. doi:10.1160/TH12-07-0486
98. Mause SF. Platelet microparticles: reinforcing the hegemony of platelets in atherothrombosis. Thromb Haemost (2013) 109:5–6. doi:10.1160/TH12-11-0817
99. Suades R, Padro T, Vilahur G, Martin-Yuste V, Sabate M, Sans-Rosello J, et al. Growing thrombi release increased levels of CD235a(+) microparticles and decreased levels of activated platelet-derived microparticles. Validation in ST-elevation myocardial infarction patients. J Thromb Haemost (2015) 13:1776–86. doi:10.1111/jth.13065
100. Berckmans RJ, Nieuwland R, Boing AN, Romijn FP, Hack CE, Sturk A. Cell-derived microparticles circulate in healthy humans and support low grade thrombin generation. Thromb Haemost (2001) 85(4):639–46.
101. Zwicker JI, Trenor CC III, Furie BC, Furie B. Tissue factor-bearing microparticles and thrombus formation. Arterioscler Thromb Vasc Biol (2011) 31:728–33. doi:10.1161/ATVBAHA.109.200964
102. Burnier L, Fontana P, Kwak BR, Angelillo-Scherrer A. Cell-derived microparticles in haemostasis and vascular medicine. Thromb Haemost (2009) 101(3):439–51. doi:10.1160/TH08-08-0521
103. Toth B, Nikolajek K, Rank A, Nieuwland R, Lohse P, Pihusch V, et al. Gender-specific and menstrual cycle dependent differences in circulating microparticles. Platelets (2007) 18:515–21. doi:10.1080/09537100701525843
104. Robert S, Poncelet P, Lacroix R, Arnaud L, Giraudo L, Hauchard A, et al. Standardization of platelet-derived microparticle counting using calibrated beads and a Cytomics FC500 routine flow cytometer: a first step towards multicenter studies? J Thromb Haemost (2009) 7:190–7. doi:10.1111/j.1538-7836.2008.03200.x
105. van der Zee PM, Biro E, Ko Y, De Winter RJ, Hack CE, Sturk A, et al. P-selectin- and CD63-exposing platelet microparticles reflect platelet activation in peripheral arterial disease and myocardial infarction. Clin Chem (2006) 52:657–64. doi:10.1373/clinchem.2005.057414
106. Bretelle F, Sabatier F, Desprez D, Camoin L, Grunebaum L, Combes V, et al. Circulating microparticles: a marker of procoagulant state in normal pregnancy and pregnancy complicated by preeclampsia or intrauterine growth restriction. Thromb Haemost (2003) 89(3):486–92.
107. Chaar V, Romana M, Tripette J, Broquere C, Huisse MG, Hue O, et al. Effect of strenuous physical exercise on circulating cell-derived microparticles. Clin Hemorheol Microcirc (2011) 47:15–25. doi:10.3233/CH-2010-1361
108. Sossdorf M, Otto GP, Claus RA, Gabriel HH, Lösche W. Cell-derived microparticles promote coagulation after moderate exercise. Med Sci Sports Exerc (2011) 43:1169–76. doi:10.1249/MSS.0b013e3182068645
109. Maruyama K, Kadono T, Morishita E. Plasma levels of platelet-derived microparticles are increased after anaerobic exercise in healthy subjects. J Atheroscler Thromb (2012) 19:585–7. doi:10.5551/jat.11791
110. Tushuizen ME, Nieuwland R, Scheffer PG, Sturk A, Heine RJ, Diamant M. Two consecutive high-fat meals affect endothelial-dependent vasodilation, oxidative stress and cellular microparticles in healthy men. J Thromb Haemost (2006) 4:1003–10. doi:10.1111/j.1538-7836.2006.01914.x
111. Enjeti AK, Ariyarajah A, D’Crus A, Seldon M, Lincz LF. Circulating microvesicle number, function and small RNA content vary with age, gender, smoking status, lipid and hormone profiles. Thromb Res (2017) 156:65–72. doi:10.1016/j.thromres.2017.04.019
112. Suades R, Padro T, Alonso R, Mata P, Badimon L. High levels of TSP1+/CD142+ platelet-derived microparticles characterise young patients with high cardiovascular risk and subclinical atherosclerosis. Thromb Haemost (2015) 114:1310–21. doi:10.1160/TH15-04-0325
113. Suades R, Padro T, Alonso R, Lopez-Miranda J, Mata P, Badimon L. Circulating CD45+/CD3+ lymphocyte-derived microparticles map lipid-rich atherosclerotic plaques in familial hypercholesterolaemia patients. Thromb Haemost (2014) 111:111–21. doi:10.1160/TH13-07-0612
114. Goichot B, Grunebaum L, Desprez D, Vinzio S, Meyer L, Schlienger JL, et al. Circulating procoagulant microparticles in obesity. Diabetes Metab (2006) 32:82–5. doi:10.1016/S1262-3636(07)70251-3
115. Heiss C, Amabile N, Lee AC, Real WM, Schick SF, Lao D, et al. Brief secondhand smoke exposure depresses endothelial progenitor cells activity and endothelial function: sustained vascular injury and blunted nitric oxide production. J Am Coll Cardiol (2008) 51:1760–71. doi:10.1016/j.jacc.2008.01.040
116. Gordon C, Gudi K, Krause A, Sackrowitz R, Harvey BG, Strulovici-Barel Y, et al. Circulating endothelial microparticles as a measure of early lung destruction in cigarette smokers. Am J Respir Crit Care Med (2011) 184(2):224–32. doi:10.1164/rccm.201012-2061OC
117. Li J, Zhang Y, Liu Y, Dai X, Li W, Cai X, et al. Microvesicle-mediated transfer of microRNA-150 from monocytes to endothelial cells promotes angiogenesis. J Biol Chem (2013) 288:23586–96. doi:10.1074/jbc.M113.489302
118. Jayachandran M, Litwiller RD, Lahr BD, Bailey KR, Owen WG, Mulvagh SL, et al. Alterations in platelet function and cell-derived microvesicles in recently menopausal women: relationship to metabolic syndrome and atherogenic risk. J Cardiovasc Transl Res (2011) 4:811–22. doi:10.1007/s12265-011-9296-9
119. Sabatier F, Darmon P, Hugel B, Combes V, Sanmarco M, Velut JG, et al. Type 1 and type 2 diabetic patients display different patterns of cellular microparticles. Diabetes (2002) 51:2840–5. doi:10.2337/diabetes.51.9.2840
120. Nomura S, Shouzu A, Omoto S, Nishikawa M, Iwasaka T, Fukuhara S. Activated platelet and oxidized LDL induce endothelial membrane vesiculation: clinical significance of endothelial cell-derived microparticles in patients with type 2 diabetes. Clin Appl Thromb Hemost (2004) 10:205–15. doi:10.1177/107602960401000302
121. Nomura S, Takahashi N, Inami N, Kajiura T, Yamada K, Nakamori H, et al. Probucol and ticlopidine: effect on platelet and monocyte activation markers in hyperlipidemic patients with and without type 2 diabetes. Atherosclerosis (2004) 174:329–35. doi:10.1016/j.atherosclerosis.2004.01.027
122. Shouzu A, Nomura S, Omoto S, Hayakawa T, Nishikawa M, Iwasaka T. Effect of ticlopidine on monocyte-derived microparticles and activated platelet markers in diabetes mellitus. Clin Appl Thromb Hemost (2004) 10:167–73. doi:10.1177/107602960401000207
123. Koga H, Sugiyama S, Kugiyama K, Watanabe K, Fukushima H, Tanaka T, et al. Elevated levels of VE-cadherin-positive endothelial microparticles in patients with type 2 diabetes mellitus and coronary artery disease. J Am Coll Cardiol (2005) 45:1622–30. doi:10.1016/j.jacc.2005.02.047
124. Omoto S, Nomura S, Shouzu A, Hayakawa T, Shimizu H, Miyake Y, et al. Significance of platelet-derived microparticles and activated platelets in diabetic nephropathy. Nephron (1999) 81:271–7. doi:10.1159/000045292
125. Diamant M, Nieuwland R, Pablo RF, Sturk A, Smit JW, Radder JK. Elevated numbers of tissue-factor exposing microparticles correlate with components of the metabolic syndrome in uncomplicated type 2 diabetes mellitus. Circulation (2002) 106:2442–7. doi:10.1161/01.CIR.0000036596.59665.C6
126. Koga H, Sugiyama S, Kugiyama K, Fukushima H, Watanabe K, Sakamoto T, et al. Elevated levels of remnant lipoproteins are associated with plasma platelet microparticles in patients with type-2 diabetes mellitus without obstructive coronary artery disease. Eur Heart J (2006) 27:817–23. doi:10.1093/eurheartj/ehi746
127. Bernard S, Loffroy R, Serusclat A, Boussel L, Bonnefoy E, Thevenon C, et al. Increased levels of endothelial microparticles CD144 (VE-Cadherin) positives in type 2 diabetic patients with coronary noncalcified plaques evaluated by multidetector computed tomography (MDCT). Atherosclerosis (2009) 203:429–35. doi:10.1016/j.atherosclerosis.2008.07.039
128. Ogata N, Nomura S, Shouzu A, Imaizumi M, Arichi M, Matsumura M. Elevation of monocyte-derived microparticles in patients with diabetic retinopathy. Diabetes Res Clin Pract (2006) 73:241–8. doi:10.1016/j.diabres.2006.01.014
129. Gunduz Z, Dursun I, Tulpar S, Bastug F, Baykan A, Yikilmaz A, et al. Increased endothelial microparticles in obese and overweight children. J Pediatr Endocrinol Metab (2012) 25:1111–7. doi:10.1515/jpem-2012-0194
130. Murakami T, Horigome H, Tanaka K, Nakata Y, Ohkawara K, Katayama Y, et al. Impact of weight reduction on production of platelet-derived microparticles and fibrinolytic parameters in obesity. Thromb Res (2007) 119:45–53. doi:10.1016/j.thromres.2005.12.013
131. Stepanian A, Bourguignat L, Hennou S, Coupaye M, Hajage D, Salomon L, et al. Microparticle increase in severe obesity: not related to metabolic syndrome and unchanged after massive weight loss. Obesity (Silver Spring) (2013) 21:2236–43. doi:10.1002/oby.20365
132. Cheng V, Kashyap SR, Schauer PR, Kirwan JP, Mccrae KR. Restoration of glycemic control in patients with type 2 diabetes mellitus after bariatric surgery is associated with reduction in microparticles. Surg Obes Relat Dis (2013) 9:207–12. doi:10.1016/j.soard.2011.09.026
133. Nomura S, Kanazawa S, Fukuhara S. Effects of efonidipine on platelet and monocyte activation markers in hypertensive patients with and without type 2 diabetes mellitus. J Hum Hypertens (2002) 16:539–47. doi:10.1038/sj.jhh.1001447
134. Preston RA, Jy W, Jimenez JJ, Mauro LM, Horstman LL, Valle M, et al. Effects of severe hypertension on endothelial and platelet microparticles. Hypertension (2003) 41:211–7. doi:10.1161/01.HYP.0000049760.15764.2D
135. Nomura S, Shouzu A, Omoto S, Nishikawa M, Iwasaka T. Effects of losartan and simvastatin on monocyte-derived microparticles in hypertensive patients with and without type 2 diabetes mellitus. Clin Appl Thromb Hemost (2004) 10:133–41. doi:10.1177/107602960401000203
136. Ferreira AC, Peter AA, Mendez AJ, Jimenez JJ, Mauro LM, Chirinos JA, et al. Postprandial hypertriglyceridemia increases circulating levels of endothelial cell microparticles. Circulation (2004) 110:3599–603. doi:10.1161/01.CIR.0000148820.55611.6B
137. Arteaga RB, Chirinos JA, Soriano AO, Jy W, Horstman L, Jimenez JJ, et al. Endothelial microparticles and platelet and leukocyte activation in patients with the metabolic syndrome. Am J Cardiol (2006) 98:70–4. doi:10.1016/j.amjcard.2006.01.054
138. Ueba T, Haze T, Sugiyama M, Higuchi M, Asayama H, Karitani Y, et al. Level, distribution and correlates of platelet-derived microparticles in healthy individuals with special reference to the metabolic syndrome. Thromb Haemost (2008) 100(2):280–5. doi:10.1160/TH07-11-0668
139. Helal O, Defoort C, Robert S, Marin C, Lesavre N, Lopez-Miranda J, et al. Increased levels of microparticles originating from endothelial cells, platelets and erythrocytes in subjects with metabolic syndrome: relationship with oxidative stress. Nutr Metab Cardiovasc Dis (2011) 21:665–71. doi:10.1016/j.numecd.2010.01.004
140. Amabile N, Cheng S, Renard JM, Larson MG, Ghorbani A, Mccabe E, et al. Association of circulating endothelial microparticles with cardiometabolic risk factors in the Framingham Heart Study. Eur Heart J (2014) 35:2972–9. doi:10.1093/eurheartj/ehu153
141. Ando M, Iwata A, Ozeki Y, Tsuchiya K, Akiba T, Nihei H. Circulating platelet-derived microparticles with procoagulant activity may be a potential cause of thrombosis in uremic patients. Kidney Int (2002) 62:1757–63. doi:10.1046/j.1523-1755.2002.00627.x
142. Nozaki T, Sugiyama S, Koga H, Sugamura K, Ohba K, Matsuzawa Y, et al. Significance of a multiple biomarkers strategy including endothelial dysfunction to improve risk stratification for cardiovascular events in patients at high risk for coronary heart disease. J Am Coll Cardiol (2009) 54:601–8. doi:10.1016/j.jacc.2009.05.022
143. Jayachandran M, Litwiller RD, Owen WG, Heit JA, Behrenbeck T, Mulvagh SL, et al. Characterization of blood borne microparticles as markers of premature coronary calcification in newly menopausal women. Am J Physiol Heart Circ Physiol (2008) 295:H931–8. doi:10.1152/ajpheart.00193.2008
144. Zeiger F, Stephan S, Hoheisel G, Pfeiffer D, Ruehlmann C, Koksch M. P-Selectin expression, platelet aggregates, and platelet-derived microparticle formation are increased in peripheral arterial disease. Blood Coagul Fibrinolysis (2000) 11(8):723–8. doi:10.1097/00001721-200012000-00005
145. Chironi G, Simon A, Hugel B, Del Pino M, Gariepy J, Freyssinet JM, et al. Circulating leukocyte-derived microparticles predict subclinical atherosclerosis burden in asymptomatic subjects. Arterioscler Thromb Vasc Biol (2006) 26:2775–80. doi:10.1161/01.ATV.0000249639.36915.04
146. Bernal-Mizrachi L, Jy W, Jimenez JJ, Pastor J, Mauro LM, Horstman LL, et al. High levels of circulating endothelial microparticles in patients with acute coronary syndromes. Am Heart J (2003) 145:962–70. doi:10.1016/S0002-8703(03)00103-0
147. Sinning JM, Losch J, Walenta K, Bohm M, Nickenig G, Werner N. Circulating CD31+/Annexin V+ microparticles correlate with cardiovascular outcomes. Eur Heart J (2011) 32:2034–41. doi:10.1093/eurheartj/ehq478
148. Jansen F, Yang X, Proebsting S, Hoelscher M, Przybilla D, Baumann K, et al. MicroRNA expression in circulating microvesicles predicts cardiovascular events in patients with coronary artery disease. J Am Heart Assoc (2014) 3:e001249. doi:10.1161/JAHA.114.001249
149. Mallat Z, Benamer H, Hugel B, Benessiano J, Steg PG, Freyssinet JM, et al. Elevated levels of shed membrane microparticles with procoagulant potential in the peripheral circulating blood of patients with acute coronary syndromes. Circulation (2000) 101:841–3. doi:10.1161/01.CIR.101.8.841
150. Nomura S, Uehata S, Saito S, Osumi K, Ozeki Y, Kimura Y. Enzyme immunoassay detection of platelet-derived microparticles and RANTES in acute coronary syndrome. Thromb Haemost (2003) 89(3):506–12.
151. Bernal-Mizrachi L, Jy W, Fierro C, Macdonough R, Velazques HA, Purow J, et al. Endothelial microparticles correlate with high-risk angiographic lesions in acute coronary syndromes. Int J Cardiol (2004) 97:439–46. doi:10.1016/j.ijcard.2003.10.029
152. Li X, Cong H. Platelet-derived microparticles and the potential of glycoprotein IIb/IIIa antagonists in treating acute coronary syndrome. Tex Heart Inst J (2009) 36(2):134–9.
153. D’Alessandra Y, Devanna P, Limana F, Straino S, Di Carlo A, Brambilla PG, et al. Circulating microRNAs are new and sensitive biomarkers of myocardial infarction. Eur Heart J (2010) 31:2765–73. doi:10.1093/eurheartj/ehq167
154. Ueba T, Nomura S, Inami N, Nishikawa T, Kajiwara M, Iwata R, et al. Plasma level of platelet-derived microparticles is associated with coronary heart disease risk score in healthy men. J Atheroscler Thromb (2010) 17:342–9. doi:10.5551/jat.2964
155. Suades R, Padro T, Crespo J, Ramaiola I, Martin-Yuste V, Sabate M, et al. Circulating microparticle signature in coronary and peripheral blood of ST elevation myocardial infarction patients in relation to pain-to-PCI elapsed time. Int J Cardiol (2016) 202:378–87. doi:10.1016/j.ijcard.2015.09.011
156. Chiva-Blanch G, Laake K, Myhre P, Bratseth V, Arnesen H, Solheim S, et al. Platelet-, monocyte-derived and tissue factor-carrying circulating microparticles are related to acute myocardial infarction severity. PLoS One (2017) 12:e0172558. doi:10.1371/journal.pone.0172558
157. Lee YJ, Jy W, Horstman LL, Janania J, Reyes Y, Kelley RE, et al. Elevated platelet microparticles in transient ischemic attacks, lacunar infarcts, and multiinfarct dementias. Thromb Res (1993) 72:295–304. doi:10.1016/0049-3848(93)90138-E
158. Simak J, Gelderman MP, Yu H, Wright V, Baird AE. Circulating endothelial microparticles in acute ischemic stroke: a link to severity, lesion volume and outcome. J Thromb Haemost (2006) 4:1296–302. doi:10.1111/j.1538-7836.2006.01911.x
159. Jung KH, Chu K, Lee ST, Park HK, Bahn JJ, Kim DH, et al. Circulating endothelial microparticles as a marker of cerebrovascular disease. Ann Neurol (2009) 66:191–9. doi:10.1002/ana.21681
160. Lukasik M, Rozalski M, Luzak B, Michalak S, Kozubski W, Watala C. Platelet activation and reactivity in the convalescent phase of ischaemic stroke. Thromb Haemost (2010) 103:644–50. doi:10.1160/TH09-08-0599
161. Kim SJ, Moon GJ, Cho YH, Kang HY, Hyung NK, Kim D, et al. Circulating mesenchymal stem cells microparticles in patients with cerebrovascular disease. PLoS One (2012) 7:e37036. doi:10.1371/journal.pone.0037036
162. Chiva-Blanch G, Suades R, Crespo J, Pena E, Padro T, Jimenez-Xarrie E, et al. Microparticle shedding from neural progenitor cells and vascular compartment cells is increased in ischemic stroke. PLoS One (2016) 11:e0148176. doi:10.1371/journal.pone.0148176
163. Lackner P, Dietmann A, Beer R, Fischer M, Broessner G, Helbok R, et al. Cellular microparticles as a marker for cerebral vasospasm in spontaneous subarachnoid hemorrhage. Stroke (2010) 41:2353–7. doi:10.1161/STROKEAHA.110.584995
164. Michelsen AE, Notø AT, Brodin E, Mathiesen EB, Brosstad F, Hansen JB. Elevated levels of platelet microparticles in carotid atherosclerosis and during the postprandial state. Thromb Res (2009) 123:881–6. doi:10.1016/j.thromres.2008.10.016
165. Chiva-Blanch G, Suades R, Crespo J, Vilahur G, Arderiu G, Padro T, et al. CD3(+)/CD45(+) and SMA-alpha(+) circulating microparticles are increased in individuals at high cardiovascular risk who will develop a major cardiovascular event. Int J Cardiol (2016) 208:147–9. doi:10.1016/j.ijcard.2016.01.211
166. Chiva-Blanch G, Bratseth V, Ritschel V, Andersen GO, Halvorsen S, Eritsland J, et al. Monocyte-derived circulating microparticles (CD14+, CD14+/CD11b+ and CD14+/CD142+) are related to long-term prognosis for cardiovascular mortality in STEMI patients. Int J Cardiol (2017) 227:876–81. doi:10.1016/j.ijcard.2016.11.302
167. Walsh KB, Campos B, Hart K, Thakar C, Adeoye O. M2 monocyte microparticles are increased in intracerebral hemorrhage. J Stroke Cerebrovasc Dis (2017) 26(10):2369–75. doi:10.1016/j.jstrokecerebrovasdis.2017.05.027
168. Carmeliet P, Jain RK. Molecular mechanisms and clinical applications of angiogenesis. Nature (2011) 473:298–307. doi:10.1038/nature10144
169. Pircher A, Treps L, Bodrug N, Carmeliet P. Endothelial cell metabolism: a novel player in atherosclerosis? Basic principles and therapeutic opportunities. Atherosclerosis (2016) 253:247–57. doi:10.1016/j.atherosclerosis.2016.08.011
170. Juan-Babot JO, Martinez-Gonzalez J, Berrozpe M, Badimon L. [Neovascularization in human coronary arteries with lesions of different severity]. Rev Esp Cardiol (2003) 56:978–86. doi:10.1016/S0300-8932(03)76995-4
171. Belting M, Christianson HC. Role of exosomes and microvesicles in hypoxia-associated tumour development and cardiovascular disease. J Intern Med (2015) 278:251–63. doi:10.1111/joim.12393
172. Brill A, Dashevsky O, Rivo J, Gozal Y, Varon D. Platelet-derived microparticles induce angiogenesis and stimulate post-ischemic revascularization. Cardiovasc Res (2005) 67:30–8. doi:10.1016/j.cardiores.2005.04.007
173. Mezentsev A, Merks RM, O’Riordan E, Chen J, Mendelev N, Goligorsky MS, et al. Endothelial microparticles affect angiogenesis in vitro: role of oxidative stress. Am J Physiol Heart Circ Physiol (2005) 289:H1106–14. doi:10.1152/ajpheart.00265.2005
174. Soleti R, Benameur T, Porro C, Panaro MA, Andriantsitohaina R, Martinez MC. Microparticles harboring Sonic Hedgehog promote angiogenesis through the upregulation of adhesion proteins and proangiogenic factors. Carcinogenesis (2009) 30:580–8. doi:10.1093/carcin/bgp030
175. Benameur T, Soleti R, Porro C, Andriantsitohaina R, Martinez MC. Microparticles carrying Sonic hedgehog favor neovascularization through the activation of nitric oxide pathway in mice. PLoS One (2010) 5:e12688. doi:10.1371/journal.pone.0012688
176. Ou ZJ, Chang FJ, Luo D, Liao XL, Wang ZP, Zhang X, et al. Endothelium-derived microparticles inhibit angiogenesis in the heart and enhance the inhibitory effects of hypercholesterolemia on angiogenesis. Am J Physiol Endocrinol Metab (2011) 300:E661–8. doi:10.1152/ajpendo.00611.2010
177. Todorova D, Simoncini S, Lacroix R, Sabatier F, Dignat-George F. Extracellular vesicles in angiogenesis. Circ Res (2017) 120:1658–73. doi:10.1161/CIRCRESAHA.117.309681
178. Ohtsuka M, Sasaki K, Ueno T, Seki R, Nakayoshi T, Koiwaya H, et al. Platelet-derived microparticles augment the adhesion and neovascularization capacities of circulating angiogenic cells obtained from atherosclerotic patients. Atherosclerosis (2013) 227:275–82. doi:10.1016/j.atherosclerosis.2013.01.040
179. Hayon Y, Dashevsky O, Shai E, Brill A, Varon D, Leker RR. Platelet microparticles induce angiogenesis and neurogenesis after cerebral ischemia. Curr Neurovasc Res (2012) 9:185–92. doi:10.2174/156720212801619018
180. Shan LY, Li JZ, Zu LY, Niu CG, Ferro A, Zhang YD, et al. Platelet-derived microparticles are implicated in remote ischemia conditioning in a rat model of cerebral infarction. CNS Neurosci Ther (2013) 19:917–25. doi:10.1111/cns.12199
181. Kim HK, Song KS, Chung JH, Lee KR, Lee SN. Platelet microparticles induce angiogenesis in vitro. Br J Haematol (2004) 124:376–84. doi:10.1046/j.1365-2141.2003.04773.x
182. Mause SF, Ritzel E, Liehn EA, Hristov M, Bidzhekov K, Muller-Newen G, et al. Platelet microparticles enhance the vasoregenerative potential of angiogenic early outgrowth cells after vascular injury. Circulation (2010) 122:495–506. doi:10.1161/CIRCULATIONAHA.109.909473
183. Leroyer AS, Ebrahimian TG, Cochain C, Recalde A, Blanc-Brude O, Mees B, et al. Microparticles from ischemic muscle promotes postnatal vasculogenesis. Circulation (2009) 119:2808–17. doi:10.1161/CIRCULATIONAHA.108.816710
184. Lacroix R, Sabatier F, Mialhe A, Basire A, Pannell R, Borghi H, et al. Activation of plasminogen into plasmin at the surface of endothelial microparticles: a mechanism that modulates angiogenic properties of endothelial progenitor cells in vitro. Blood (2007) 110:2432–9. doi:10.1182/blood-2007-02-069997
185. Sun C, Feng SB, Cao ZW, Bei JJ, Chen Q, Zhao WB, et al. Up-regulated expression of matrix metalloproteinases in endothelial cells mediates platelet microvesicle-induced angiogenesis. Cell Physiol Biochem (2017) 41:2319–32. doi:10.1159/000475651
186. Leroyer AS, Rautou PE, Silvestre JS, Castier Y, Leseche G, Devue C, et al. CD40 ligand+ microparticles from human atherosclerotic plaques stimulate endothelial proliferation and angiogenesis a potential mechanism for intraplaque neovascularization. J Am Coll Cardiol (2008) 52:1302–11. doi:10.1016/j.jacc.2008.07.032
187. Merjaneh M, Langlois A, Larochelle S, Cloutier CB, Ricard-Blum S, Moulin VJ. Pro-angiogenic capacities of microvesicles produced by skin wound myofibroblasts. Angiogenesis (2017) 20:385–98. doi:10.1007/s10456-017-9554-9
188. Deregibus MC, Cantaluppi V, Calogero R, Lo Iacono M, Tetta C, Biancone L, et al. Endothelial progenitor cell derived microvesicles activate an angiogenic program in endothelial cells by a horizontal transfer of mRNA. Blood (2007) 110:2440–8. doi:10.1182/blood-2007-03-078709
189. Bian S, Zhang L, Duan L, Wang X, Min Y, Yu H. Extracellular vesicles derived from human bone marrow mesenchymal stem cells promote angiogenesis in a rat myocardial infarction model. J Mol Med (Berl) (2014) 92:387–97. doi:10.1007/s00109-013-1110-5
190. Doeppner TR, Herz J, Gorgens A, Schlechter J, Ludwig AK, Radtke S, et al. Extracellular vesicles improve post-stroke neuroregeneration and prevent postischemic immunosuppression. Stem Cells Transl Med (2015) 4:1131–43. doi:10.5966/sctm.2015-0078
191. Zhang HC, Liu XB, Huang S, Bi XY, Wang HX, Xie LX, et al. Microvesicles derived from human umbilical cord mesenchymal stem cells stimulated by hypoxia promote angiogenesis both in vitro and in vivo. Stem Cells Dev (2012) 21:3289–97. doi:10.1089/scd.2012.0095
192. Zou X, Gu D, Xing X, Cheng Z, Gong D, Zhang G, et al. Human mesenchymal stromal cell-derived extracellular vesicles alleviate renal ischemic reperfusion injury and enhance angiogenesis in rats. Am J Transl Res (2016) 8(10):4289–99.
193. Prokopi M, Pula G, Mayr U, Devue C, Gallagher J, Xiao Q, et al. Proteomic analysis reveals presence of platelet microparticles in endothelial progenitor cell cultures. Blood (2009) 114:723–32. doi:10.1182/blood-2009-02-205930
194. Varon D, Hayon Y, Dashevsky O, Shai E. Involvement of platelet derived microparticles in tumor metastasis and tissue regeneration. Thromb Res (2012) 130(Suppl 1):S98–9. doi:10.1016/j.thromres.2012.08.289
195. Agouni A, Mostefai HA, Porro C, Carusio N, Favre J, Richard V, et al. Sonic hedgehog carried by microparticles corrects endothelial injury through nitric oxide release. FASEB J (2007) 21:2735–41. doi:10.1096/fj.07-8079com
196. Guller S, Tang Z, Ma YY, Di Santo S, Sager R, Schneider H. Protein composition of microparticles shed from human placenta during placental perfusion: potential role in angiogenesis and fibrinolysis in preeclampsia. Placenta (2011) 32:63–9. doi:10.1016/j.placenta.2010.10.011
197. Yang C, Mwaikambo BR, Zhu T, Gagnon C, Lafleur J, Seshadri S, et al. Lymphocytic microparticles inhibit angiogenesis by stimulating oxidative stress and negatively regulating VEGF-induced pathways. Am J Physiol Regul Integr Comp Physiol (2008) 294:R467–76. doi:10.1152/ajpregu.00432.2007
198. Ramakrishnan DP, Hajj-Ali RA, Chen Y, Silverstein RL. Extracellular vesicles activate a CD36-dependent signaling pathway to inhibit microvascular endothelial cell migration and tube formation. Arterioscler Thromb Vasc Biol (2016) 36:534–44. doi:10.1161/ATVBAHA.115.307085
199. Lopatina T, Bruno S, Tetta C, Kalinina N, Porta M, Camussi G. Platelet-derived growth factor regulates the secretion of extracellular vesicles by adipose mesenchymal stem cells and enhances their angiogenic potential. Cell Commun Signal (2014) 12:26. doi:10.1186/1478-811X-12-26
200. Boopathy GTK, Kulkarni M, Ho SY, Boey A, Chua EWM, Barathi VA, et al. Cavin-2 regulates the activity and stability of endothelial nitric oxide synthase (eNOS) in angiogenesis. J Biol Chem (2017) 292(43):17760–76. doi:10.1074/jbc.M117.794743
201. Turu MM, Slevin M, Matou S, West D, Rodriguez C, Luque A, et al. C-reactive protein exerts angiogenic effects on vascular endothelial cells and modulates associated signalling pathways and gene expression. BMC Cell Biol (2008) 9:47. doi:10.1186/1471-2121-9-47
202. Habersberger J, Strang F, Scheichl A, Htun N, Bassler N, Merivirta RM, et al. Circulating microparticles generate and transport monomeric C-reactive protein in patients with myocardial infarction. Cardiovasc Res (2012) 96:64–72. doi:10.1093/cvr/cvs237
203. Belik D, Tsang H, Wharton J, Howard L, Bernabeu C, Wojciak-Stothard B. Endothelium-derived microparticles from chronically thromboembolic pulmonary hypertensive patients facilitate endothelial angiogenesis. J Biomed Sci (2016) 23:4. doi:10.1186/s12929-016-0224-9
204. Wilhelm EN, Gonzalez-Alonso J, Parris C, Rakobowchuk M. Exercise intensity modulates the appearance of circulating microvesicles with proangiogenic potential upon endothelial cells. Am J Physiol Heart Circ Physiol (2016) 311:H1297–310. doi:10.1152/ajpheart.00516.2016
205. Jain M, Bhosale V, Tripathi D, Singh H, Pal N, Hanif K, et al. Antihypertensive drugs aliskiren, nebivolol, and olmesartan reduce hypertension by reducing endothelial microparticles and regulating angiogenesis. J Cardiovasc Pharmacol (2017) 70:176–83. doi:10.1097/FJC.0000000000000503
206. Kang T, Jones TM, Naddell C, Bacanamwo M, Calvert JW, Thompson WE, et al. Adipose-derived stem cells induce angiogenesis via microvesicle transport of miRNA-31. Stem Cells Transl Med (2016) 5:440–50. doi:10.5966/sctm.2015-0177
207. Ranghino A, Cantaluppi V, Grange C, Vitillo L, Fop F, Biancone L, et al. Endothelial progenitor cell-derived microvesicles improve neovascularization in a murine model of hindlimb ischemia. Int J Immunopathol Pharmacol (2012) 25:75–85. doi:10.1177/039463201202500110
208. Cantaluppi V, Biancone L, Figliolini F, Beltramo S, Medica D, Deregibus MC, et al. Microvesicles derived from endothelial progenitor cells enhance neoangiogenesis of human pancreatic islets. Cell Transplant (2012) 21:1305–20. doi:10.3727/096368911X627534
209. Lombardo G, Dentelli P, Togliatto G, Rosso A, Gili M, Gallo S, et al. Activated Stat5 trafficking via endothelial cell-derived extracellular vesicles controls IL-3 pro-angiogenic paracrine action. Sci Rep (2016) 6:25689. doi:10.1038/srep25689
210. Zernecke A, Bidzhekov K, Noels H, Shagdarsuren E, Gan L, Denecke B, et al. Delivery of microRNA-126 by apoptotic bodies induces CXCL12-dependent vascular protection. Sci Signal (2009) 2:ra81. doi:10.1126/scisignal.2000610
211. Rak J, Milsom C, May L, Klement P, Yu J. Tissue factor in cancer and angiogenesis: the molecular link between genetic tumor progression, tumor neovascularization, and cancer coagulopathy. Semin Thromb Hemost (2006) 32:54–70. doi:10.1055/s-2006-933341
212. Arderiu G, Pena E, Aledo R, Badimon L. Tissue factor-Akt signaling triggers microvessel formation. J Thromb Haemost (2012) 10:1895–905. doi:10.1111/j.1538-7836.2012.04848.x
213. Arderiu G, Espinosa S, Pena E, Crespo J, Aledo R, Bogdanov VY, et al. Tissue factor variants induce monocyte transformation and transdifferentiation into endothelial cell-like cells. J Thromb Haemost (2017) 15(8):1689–703. doi:10.1111/jth.13751
214. Svensson KJ, Kucharzewska P, Christianson HC, Skold S, Lofstedt T, Johansson MC, et al. Hypoxia triggers a proangiogenic pathway involving cancer cell microvesicles and PAR-2-mediated heparin-binding EGF signaling in endothelial cells. Proc Natl Acad Sci U S A (2011) 108:13147–52. doi:10.1073/pnas.1104261108
215. Collier ME, Ettelaie C. Induction of endothelial cell proliferation by recombinant and microparticle-tissue factor involves beta1-integrin and extracellular signal regulated kinase activation. Arterioscler Thromb Vasc Biol (2010) 30:1810–7. doi:10.1161/ATVBAHA.110.211854
216. Arderiu G, Pena E, Badimon L. Angiogenic microvascular endothelial cells release microparticles rich in tissue factor that promotes postischemic collateral vessel formation. Arterioscler Thromb Vasc Biol (2015) 35:348–57. doi:10.1161/ATVBAHA.114.303927
217. Martinez MC, Andriantsitohaina R. Microparticles in angiogenesis: therapeutic potential. Circ Res (2011) 109:110–9. doi:10.1161/CIRCRESAHA.110.233049
218. Graves LE, Ariztia EV, Navari JR, Matzel HJ, Stack MS, Fishman DA. Proinvasive properties of ovarian cancer ascites-derived membrane vesicles. Cancer Res (2004) 64:7045–9. doi:10.1158/0008-5472.CAN-04-1800
219. Janowska-Wieczorek A, Wysoczynski M, Kijowski J, Marquez-Curtis L, Machalinski B, Ratajczak J, et al. Microvesicles derived from activated platelets induce metastasis and angiogenesis in lung cancer. Int J Cancer (2005) 113:752–60. doi:10.1002/ijc.20657
220. Taraboletti G, D’Ascenzo S, Giusti I, Marchetti D, Borsotti P, Millimaggi D, et al. Bioavailability of VEGF in tumor-shed vesicles depends on vesicle burst induced by acidic pH. Neoplasia (2006) 8:96–103. doi:10.1593/neo.05583
221. Al-Nedawi K, Meehan B, Kerbel RS, Allison AC, Rak J. Endothelial expression of autocrine VEGF upon the uptake of tumor-derived microvesicles containing oncogenic EGFR. Proc Natl Acad Sci U S A (2009) 106:3794–9. doi:10.1073/pnas.0804543106
222. Yang C, Gagnon C, Hou X, Hardy P. Low density lipoprotein receptor mediates anti-VEGF effect of lymphocyte T-derived microparticles in Lewis lung carcinoma cells. Cancer Biol Ther (2010) 10:448–56. doi:10.4161/cbt.10.5.12533
223. Yang C, Xiong W, Qiu Q, Shao Z, Hamel D, Tahiri H, et al. Role of receptor-mediated endocytosis in the antiangiogenic effects of human T lymphoblastic cell-derived microparticles. Am J Physiol Regul Integr Comp Physiol (2012) 302:R941–9. doi:10.1152/ajpregu.00527.2011
224. Zhuang G, Wu X, Jiang Z, Kasman I, Yao J, Guan Y, et al. Tumour-secreted miR-9 promotes endothelial cell migration and angiogenesis by activating the JAK-STAT pathway. EMBO J (2012) 31:3513–23. doi:10.1038/emboj.2012.183
225. Zhang H, Bai M, Deng T, Liu R, Wang X, Qu Y, et al. Cell-derived microvesicles mediate the delivery of miR-29a/c to suppress angiogenesis in gastric carcinoma. Cancer Lett (2016) 375:331–9. doi:10.1016/j.canlet.2016.03.026
226. Feng Q, Zhang C, Lum D, Druso JE, Blank B, Wilson KF, et al. A class of extracellular vesicles from breast cancer cells activates VEGF receptors and tumour angiogenesis. Nat Commun (2017) 8:14450. doi:10.1038/ncomms14450
227. Huaitong X, Yuanyong F, Yueqin T, Peng Z, Wei S, Kai S. Microvesicles releasing by oral cancer cells enhance endothelial cell angiogenesis via Shh/RhoA signaling pathway. Cancer Biol Ther (2017) 18(10):783–91. doi:10.1080/15384047.2017.1373213
228. Treps L, Perret R, Edmond S, Ricard D, Gavard J. Glioblastoma stem-like cells secrete the pro-angiogenic VEGF-A factor in extracellular vesicles. J Extracell Vesicles (2017) 6:1359479. doi:10.1080/20013078.2017.1359479
229. Estruch R, Ros E, Salas-Salvado J, Covas MI, Corella D, Aros F, et al. Primary prevention of cardiovascular disease with a Mediterranean diet. N Engl J Med (2013) 368:1279–90. doi:10.1056/NEJMoa1200303
230. Chiva-Blanch G, Crespo J, Suades R, Arderiu G, Padro T, Vilahur G, et al. CD142+/CD61+, CD146+ and CD45+ microparticles predict cardiovascular events in high risk patients following a Mediterranean diet supplemented with nuts. Thromb Haemost (2016) 116:103–14. doi:10.1160/TH16-02-0130
231. Nomura S, Shouzu A, Omoto S, Hayakawa T, Kagawa H, Nishikawa M, et al. Effect of cilostazol on soluble adhesion molecules and platelet-derived microparticles in patients with diabetes. Thromb Haemost (1998) 80:388–92.
232. Nomura S, Omoto S, Yokoi T, Fujita S, Ozasa R, Eguchi N, et al. Effects of miglitol in platelet-derived microparticle, adiponectin, and selectin level in patients with type 2 diabetes mellitus. Int J Gen Med (2011) 4:539–45. doi:10.2147/IJGM.S22115
233. Mobarrez F, He S, Broijersen A, Wiklund B, Antovic A, Antovic J, et al. Atorvastatin reduces thrombin generation and expression of tissue factor, P-selectin and GPIIIa on platelet-derived microparticles in patients with peripheral arterial occlusive disease. Thromb Haemost (2011) 106:344–52. doi:10.1160/TH10-12-0810
234. Wang JM, Yang Z, Xu MG, Chen L, Wang Y, Su C, et al. Berberine-induced decline in circulating CD31+/CD42- microparticles is associated with improvement of endothelial function in humans. Eur J Pharmacol (2009) 614:77–83. doi:10.1016/j.ejphar.2009.04.037
235. Cheng F, Wang Y, Li J, Su C, Wu F, Xia WH, et al. Berberine improves endothelial function by reducing endothelial microparticles-mediated oxidative stress in humans. Int J Cardiol (2013) 167:936–42. doi:10.1016/j.ijcard.2012.03.090
236. Zu L, Ren C, Pan B, Zhou B, Zhou E, Niu C, et al. Endothelial microparticles after antihypertensive and lipid-lowering therapy inhibit the adhesion of monocytes to endothelial cells. Int J Cardiol (2016) 202:756–9. doi:10.1016/j.ijcard.2015.10.035
237. Goto S, Tamura N, Li M, Handa M, Ikeda Y, Handa S, et al. Different effects of various anti-GPIIb-IIIa agents on shear-induced platelet activation and expression of procoagulant activity. J Thromb Haemost (2003) 1:2022–30. doi:10.1046/j.1538-7836.2003.00349.x
238. Morel O, Hugel B, Jesel L, Mallat Z, Lanza F, Douchet MP, et al. Circulating procoagulant microparticles and soluble GPV in myocardial infarction treated by primary percutaneous transluminal coronary angioplasty. A possible role for GPIIb-IIIa antagonists. J Thromb Haemost (2004) 2:1118–26. doi:10.1111/j.1538-7836.2004.00805.x
239. Judge HM, Buckland RJ, Sugidachi A, Jakubowski JA, Storey RF. Relationship between degree of P2Y12 receptor blockade and inhibition of P2Y12-mediated platelet function. Thromb Haemost (2010) 103:1210–7. doi:10.1160/TH09-11-0770
240. Franca CN, Pinheiro LF, Izar MC, Brunialti MK, Salomao R, Bianco HT, et al. Endothelial progenitor cell mobilization and platelet microparticle release are influenced by clopidogrel plasma levels in stable coronary artery disease. Circ J (2012) 76:729–36. doi:10.1253/circj.CJ-11-1145
241. Bulut D, Becker V, Mugge A. Acetylsalicylate reduces endothelial and platelet-derived microparticles in patients with coronary artery disease. Can J Physiol Pharmacol (2011) 89:239–44. doi:10.1139/y11-013
242. Skeppholm M, Mobarrez F, Malmqvist K, Wallen H. Platelet-derived microparticles during and after acute coronary syndrome. Thromb Haemost (2012) 107:1122–9. doi:10.1160/TH11-11-0779
243. Lubsczyk B, Kollars M, Hron G, Kyrle PA, Weltermann A, Gartner V. Low dose acetylsalicylic acid and shedding of microparticles in vivo in humans. Eur J Clin Invest (2010) 40:477–82. doi:10.1111/j.1365-2362.2010.02299.x
244. Chiva-Blanch G, Suades R, Padro T, Vilahur G, Pena E, Ybarra J, et al. Microparticle shedding by erythrocytes, monocytes and vascular smooth muscular cells is reduced by aspirin in diabetic patients. Rev Esp Cardiol (Engl Ed) (2016) 69(7):672–80. doi:10.1016/j.rec.2015.12.033
245. Labios M, Martinez M, Gabriel F, Guiral V, Munoz A, Aznar J. Effect of eprosartan on cytoplasmic free calcium mobilization, platelet activation, and microparticle formation in hypertension. Am J Hypertens (2004) 17:757–63. doi:10.1016/j.amjhyper.2004.05.010
246. Nomura S, Inami N, Kimura Y, Omoto S, Shouzu A, Nishikawa M, et al. Effect of nifedipine on adiponectin in hypertensive patients with type 2 diabetes mellitus. J Hum Hypertens (2007) 21:38–44. doi:10.1038/sj.jhh.1002100
247. Morel O, Jesel L, Hugel B, Douchet MP, Zupan M, Chauvin M, et al. Protective effects of vitamin C on endothelium damage and platelet activation during myocardial infarction in patients with sustained generation of circulating microparticles. J Thromb Haemost (2003) 1:171–7. doi:10.1046/j.1538-7836.2003.00010.x
248. Kagawa H, Nomura S, Nagahama M, Ozaki Y, Fukuhara S. Effect of bezafibrate on soluble adhesion molecules and platelet activation markers in patients with connective tissue diseases and secondary hyperlipidemia. Clin Appl Thromb Hemost (2001) 7:153–7. doi:10.1177/107602960100700213
249. Esposito K, Ciotola M, Giugliano D. Pioglitazone reduces endothelial microparticles in the metabolic syndrome. Arterioscler Thromb Vasc Biol (2006) 26:1926. doi:10.1161/01.ATV.0000231512.15115.25
250. Nebor D, Romana M, Santiago R, Vachiery N, Picot J, Broquere C, et al. Fetal hemoglobin and hydroxycarbamide modulate both plasma concentration and cellular origin of circulating microparticles in sickle cell anemia children. Haematologica (2013) 98(6):862–7. doi:10.3324/haematol.2012.073619
251. Weitz IC, Razavi P, Rochanda L, Zwicker J, Furie B, Manly D, et al. Eculizumab therapy results in rapid and sustained decreases in markers of thrombin generation and inflammation in patients with PNH independent of its effects on hemolysis and microparticle formation. Thromb Res (2012) 130:361–8. doi:10.1016/j.thromres.2012.04.001
252. Diamant M, Tushuizen ME, Abid-Hussein MN, Hau CM, Boing AN, Sturk A, et al. Simvastatin-induced endothelial cell detachment and microparticle release are prenylation dependent. Thromb Haemost (2008) 100(3):489–97. doi:10.1160/TH07-12-0760
253. Mobarrez F, Egberg N, Antovic J, Broijersen A, Jorneskog G, Wallen H. Release of endothelial microparticles in vivo during atorvastatin treatment; a randomized double-blind placebo-controlled study. Thromb Res (2012) 129:95–7. doi:10.1016/j.thromres.2011.09.027
254. Tramontano AF, O’Leary J, Black AD, Muniyappa R, Cutaia MV, El-Sherif N. Statin decreases endothelial microparticle release from human coronary artery endothelial cells: implication for the Rho-kinase pathway. Biochem Biophys Res Commun (2004) 320:34–8. doi:10.1016/j.bbrc.2004.05.127
255. Suades R, Padro T, Alonso R, Mata P, Badimon L. Lipid-lowering therapy with statins reduces microparticle shedding from endothelium, platelets and inflammatory cells. Thromb Haemost (2013) 110:366–77. doi:10.1160/TH13-03-0238
256. Pawelczyk M, Chmielewski H, Kaczorowska B, Przybyla M, Baj Z. The influence of statin therapy on platelet activity markers in hyperlipidemic patients after ischemic stroke. Arch Med Sci (2015) 11:115–21. doi:10.5114/aoms.2015.49216
257. Nomura S, Shouzu A, Omoto S, Nishikawa M, Fukuhara S, Iwasaka T. Losartan and simvastatin inhibit platelet activation in hypertensive patients. J Thromb Thrombolysis (2004) 18:177–85. doi:10.1007/s11239-005-0343-8
258. Tehrani S, Mobarrez F, Antovic A, Santesson P, Lins PE, Adamson U, et al. Atorvastatin has antithrombotic effects in patients with type 1 diabetes and dyslipidemia. Thromb Res (2010) 126:e225–31. doi:10.1016/j.thromres.2010.05.023
259. Lins LC, Franca CN, Fonseca FA, Barbosa SP, Matos LN, Aguirre AC, et al. Effects of Ezetimibe on endothelial progenitor cells and microparticles in high-risk patients. Cell Biochem Biophys (2014) 70(1):687–96. doi:10.1007/s12013-014-9973-9
260. Ferreira CE, Franca CN, Izar MC, Camargo LM, Roman RM, Fonseca FA. High-intensity statin monotherapy versus moderate-intensity statin plus ezetimibe therapy: effects on vascular biomarkers. Int J Cardiol (2014) 180:78–9. doi:10.1016/j.ijcard.2014.11.177
261. van Dommelen SM, Vader P, Lakhal S, Kooijmans SA, Van Solinge WW, Wood MJ, et al. Microvesicles and exosomes: opportunities for cell-derived membrane vesicles in drug delivery. J Control Release (2011) 161:635–44. doi:10.1016/j.jconrel.2011.11.021
262. Timmers L, Lim SK, Arslan F, Armstrong JS, Hoefer IE, Doevendans PA, et al. Reduction of myocardial infarct size by human mesenchymal stem cell conditioned medium. Stem Cell Res (2007) 1:129–37. doi:10.1016/j.scr.2008.02.002
263. Timmers L, Lim SK, Hoefer IE, Arslan F, Lai RC, Van Oorschot AA, et al. Human mesenchymal stem cell-conditioned medium improves cardiac function following myocardial infarction. Stem Cell Res (2011) 6:206–14. doi:10.1016/j.scr.2011.01.001
264. Kervadec A, Bellamy V, El Harane N, Arakelian L, Vanneaux V, Cacciapuoti I, et al. Cardiovascular progenitor-derived extracellular vesicles recapitulate the beneficial effects of their parent cells in the treatment of chronic heart failure. J Heart Lung Transplant (2016) 35:795–807. doi:10.1016/j.healun.2016.01.013
265. Ratajczak MZ, Ratajczak J. Horizontal transfer of RNA and proteins between cells by extracellular microvesicles: 14 years later. Clin Transl Med (2016) 5:7. doi:10.1186/s40169-016-0087-4
266. Gatti S, Bruno S, Deregibus MC, Sordi A, Cantaluppi V, Tetta C, et al. Microvesicles derived from human adult mesenchymal stem cells protect against ischaemia-reperfusion-induced acute and chronic kidney injury. Nephrol Dial Transplant (2011) 26:1474–83. doi:10.1093/ndt/gfr015
267. Wang Y, Wei S, Wang YL, Liu M, Shang M, Zhang Q, et al. Protective effects of circulating microvesicles derived from myocardial ischemic rats on apoptosis of cardiomyocytes in myocardial ischemia/reperfusion injury. Oncotarget (2017) 8(33):54572–82. doi:10.18632/oncotarget.17424
268. Malliaras K, Makkar RR, Smith RR, Cheng K, Wu E, Bonow RO, et al. Intracoronary cardiosphere-derived cells after myocardial infarction: evidence of therapeutic regeneration in the final 1-year results of the CADUCEUS trial (CArdiosphere-Derived aUtologous stem CElls to reverse ventricUlar dySfunction). J Am Coll Cardiol (2014) 63:110–22. doi:10.1016/j.jacc.2013.08.724
269. Gallet R, Dawkins J, Valle J, Simsolo E, De Couto G, Middleton R, et al. Exosomes secreted by cardiosphere-derived cells reduce scarring, attenuate adverse remodelling, and improve function in acute and chronic porcine myocardial infarction. Eur Heart J (2017) 38:201–11. doi:10.1093/eurheartj/ehw240
270. Getts DR, Terry RL, Getts MT, Deffrasnes C, Muller M, Van Vreden C, et al. Therapeutic inflammatory monocyte modulation using immune-modifying microparticles. Sci Transl Med (2014) 6:219ra217. doi:10.1126/scitranslmed.3007563
Keywords: angiogenesis, atherosclerosis, cardiovascular diseases, cell-derived microvesicles, endothelial dysfunction, inflammation, neovascularization, thrombosis
Citation: Badimon L, Suades R, Arderiu G, Peña E, Chiva-Blanch G and Padró T (2017) Microvesicles in Atherosclerosis and Angiogenesis: From Bench to Bedside and Reverse. Front. Cardiovasc. Med. 4:77. doi: 10.3389/fcvm.2017.00077
Received: 22 August 2017; Accepted: 22 November 2017;
Published: 18 December 2017
Edited by:
Elena Aikawa, Brigham and Women’s Hospital, United StatesReviewed by:
Carlos Alonso Escudero, University of the Bío Bío, ChileAlbert Rizvanov, Kazan Federal University, Russia
Copyright: © 2017 Badimon, Suades, Arderiu, Peña, Chiva-Blanch and Padró. This is an open-access article distributed under the terms of the Creative Commons Attribution License (CC BY). The use, distribution or reproduction in other forums is permitted, provided the original author(s) or licensor are credited and that the original publication in this journal is cited, in accordance with accepted academic practice. No use, distribution or reproduction is permitted which does not comply with these terms.
*Correspondence: Lina Badimon, bGJhZGltb25AY3NpYy1pY2NjLm9yZw==