- 1University of Chinese Academy of Sciences, Beijing, China
- 2Key Laboratory of Engineering Biology for Low-carbon Manufacturing, Tianjin Institute of Industrial Biotechnology, Chinese Academy of Sciences, Tianjin, China
- 3National Center of Technology Innovation for Synthetic Biology, Tianjin, China
In the past 20 years, unspecific peroxygenases (UPOs) have emerged as promising biocatalysts for various organic transformations. Particularly, we have witnessed great attention being paid to the screening of new enzymes and expansion of the substrates/products. However, challenges such as enzyme stability, low turnover numbers, and substrate specificity hinder their widespread utilization in practical organic synthesis. This review article provides a concrete and mini-overview of the challenges associated with using UPOs in organic synthesis and discusses strategies for enzyme engineering to overcome these limitations. The article highlights recent advancements in UPO research and presents potential solutions to enhance their catalytic efficiency, stability, substrate specificity, and regioselectivity. Additionally, the review outlines the current methodologies employed for directed evolution and protein engineering of UPOs, along with computational modeling approaches for rational enzyme design. By addressing the challenges and exploring avenues for enzyme engineering, this review aims to shed light on the prospects of UPOs in organic synthesis.
1 Introduction
Since its discovery in 2004, unspecific peroxygenases (UPOs, EC 1.11.2.1) have gained significant attention for their potential application in organic transformations. UPOs are a class of fungal enzymes capable of performing selective oxyfunctionalization reactions on a wide range of hydrocarbons, including aromatic and aliphatic compounds, in a nicotinamide cofactor-independent manner using hydrogen peroxide solely as the oxidant. These enzymes catalyze diverse oxygen transfer reactions, especially the hydroxylation of inactivated C-H bonds and epoxidation of C=C bonds (Peter et al., 2013). In some cases, O- and N-dealkylation, epoxide opening and deacylation may undergo spontaneously (Kinne et al., 2009; Kiebist et al., 2015; Hofrichter and Ullrich, 2014). In addition to the oxygenation of carbon atoms (C), UPOs also have the ability to oxygenate organic heteroatoms such as nitrogen atoms (N) and sulfur atoms (S) (Li et al., 2024). In the latter cases, extensive effort has been devoted to enhance the stereoselectivity and product titer via the protein engineering and reaction engineering. Moreover, UPOs exhibit peroxidase activity (one-electron oxidation) and halogenation activity as they are heme-thiolate proteins (Ullrich et al., 2004; Mate et al., 2017). According to different structural features and substrates preferences, UPOs are classified into long or short types (Figure 1), each exhibiting distinct catalytic performance (Hofrichter et al., 2020). Overall, long chian UPOs have a stronger preference for small aromatic compounds, whereas short chain UPOs are capable of transforming bulky compounds, including steroids and long aliphatic substrates (Beltrán-Nogal et al., 2022).
UPOs are considered promising for organic synthesis due to key features such as their typically high stability, regio- and enantioselectivity, and their ability to catalyze diverse transformations. Notably, UPOs share the same reactive intermediate, oxoferryl-heme (compound I), with P450 monooxygenases during the catalytic cycles. In principle, a very large scope of substrates and products is expected. Today, over thousands of substrates have been subjected to P450 monooxygenases leading to the synthesis of fine chemicals, agrochemicals, organic building blocks, and pharmaceuticals (Zhang et al., 2023). On the contrary, only a few hundred substrates have been documented with UPOs. Therefore, investigating the new substrates and establishing new reactions have become one of the frontiers for UPOs (see section 2 below). These reactions, along with the obtained products, in some cases are still not easy to achieve (if not impossible) with P450 monooxygenases or other oxidoreductases. Such examples are inspiring to continuously expand the applications of UPOs in organic synthesis.
Review articles on the current state-of-the-art of UPOs, including their availability, identification, expression, combination with in situ H2O2 generation, and screening approaches, have been widely discussed and will not be covered in this article (Kinner et al., 2021; Hofrichter et al., 2022; Martínez et al., 2017). Instead, we will focus on the expanding research on the application of UPOs in organic synthesis and examine the challenges associated with organic transformations. Additionally, we will discuss recent advancements in the protein engineering of UPOs, including how directed evolution methods can enhance their functional expression and catalytic properties, as well as research on structure-function relationships to further optimize their performance for specific synthetic applications. By concentrating on these advancements of the peroxygenases in organic synthesis, this review will also outline the scopes and limitations in using protein engineering to expand their applications in this field.
2 UPOs for organic synthesis
UPOs efficiently catalyze a wide range of oxidation reactions. Their broad substrate scope and mild reaction conditions make them indispensable tools in organic synthesis. In this context, we will present representative examples demonstrating the application of UPOs in chemical synthesis, highlighting both well-established reactions and novel reactions in the last 3 years.
The AaeUPO, derived from Agrocybe aegerita, is the most studied long chain UPO and has garnered significant attention since its discovery. Renowned for its wide substrate range, high catalytic activity, and stability, this enzyme has undergone several name changes, including unusual peroxidase, A. aegerita haloperoxidase (AaP), and aromatic peroxygenase (APO), before officially being classified as an unspecific peroxygenase in 2011 (Hofrichter and Ullrich, 2006; Pecyna et al., 2009; Peter et al., 2011). The UPO designation has not only provided clarity but has also deepened our understanding of its enzymatic characteristics and expanded our knowledge of its potential application in biotechnology and biocatalysis. Consequently, AaeUPO has emerged as a highly promising biocatalyst, showcasing versatile catalytic activities, such as hydroxylation on both aromatic and aliphatic substrates, as illustrated in Figure 2. In the established reactions with ethylbenzene along with H2O2 in-situ generation, the major reaction observed was benzylic hydroxylation, with a turnover number (TON) reaching up to hundreds of thousands, accompanied by a 25% overoxidation to acetophenone (Horst et al., 2016). However, when the substituent pattern is changed, e.g., from ethylbenzene to toluene, the reactivity and selectivity is significantly changed, resulting in the mixture of benzyl alcohol and cresols. To overcome this selectivity issue, optimization can be approached from both the substrate and enzyme perspectives. For instance, a substrate engineering-inspired strategy has been adopted, which has resulted in AaeUPO showing remarkable selectivity towards the toluene derivatives (Wang et al., 2023) (Figure 2A). Additionally, evaluating UPOs from diverse sources for benzylic substrate selectivity can reveal more effective enzymes, thereby enhancing catalytic efficiency and selectivity (Kluge et al., 2009; Yan et al., 2024).
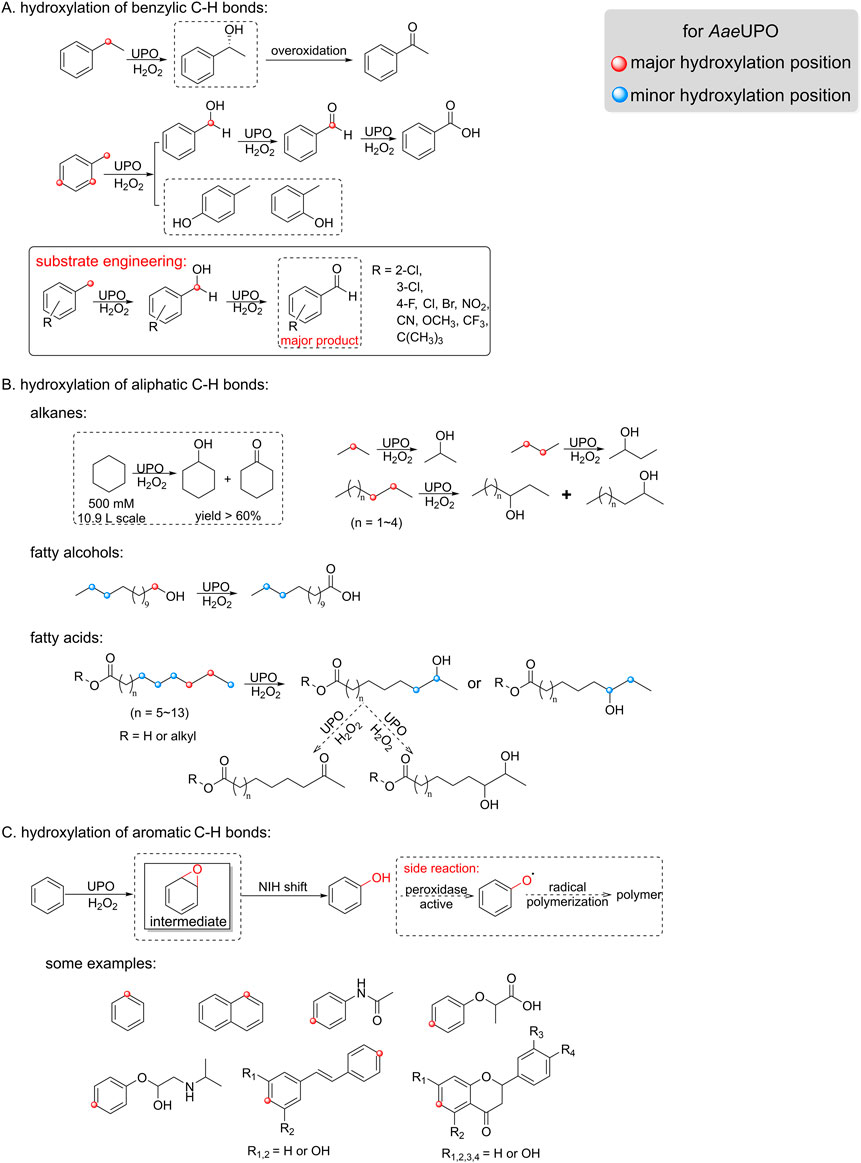
Figure 2. Established hydroxylation of hydrocarbons reactions by AaeUPO. (A) Hydroxylation of benzylic C-H bond (e.g., toluene and ethylbenzene). (B) Hydroxylation of aliphatic C-H bond, including (cyclo)alkanes, fatty alcohols and fatty acids. (C) Hydroxylation of aromatic C-H bond (e.g., benzene and naphthalene).
In addition to its capability for benzylic hydroxylation, AaeUPO also demonstrates broad applicability in the hydroxylation of aliphatic C-H bonds. Aliphatic hydrocarbons, such as cyclohexane, is a well-accepted substrate by peroxygenases. Recently, extremely high cyclohexanol concentration was achieved (yield >60%) using free AaeUPO (non-immobilized) in 50% acetonitrile with a high substrate loading (500 mM of cyclohexane) (Hilberath et al., 2023) (Figure 2B). This was believed only possible by using immobilized AaeUPO under neat reaction conditions, in which both the substrate and product titer could be improved. However, when linear alkanes of short-chain and medium-chain were used, AaeUPO exhibited poor regioselectivity, resulting in alcohols primarily at the 2-position and 3-position, with some degree of overoxidation to ketones. Unlike that observed for alkanes, hydroxylation of C12-C20 fatty acid occurs at the ω-1 or ω-2 position (Figure 2B). Different UPOs exhibit varying preferences for hydroxylation positions, for example, MroUPO preferentially hydroxylates terminal C-H bonds of linear saturated long chain alkanes, even converting them to fatty acids, whereas AaeUPO tends to hydroxylate at the ω-1 position and ω-2 position without generating acids (Olmedo et al., 2016). So in principle, the poor regioselectivity can be resolved by adjusting the size of heme cavity, as recently demonstrated by changing one single site to narrow the heme cavity (Gomez de Santos et al., 2023a).
AaeUPO’s hydroxylation of aromatic rings shows a more complex selectivity. Hydroxylating aromatic C-H bonds in benzene and naphthalene derivatives remains a big challenge due to low selectivity and reactivity. Specifically, AaeUPO can catalyze the aromatic C-H bond activation and leads to the formation of the so-called aromatic epoxide as an intermediate. This intermediate quickly transforms into phenol via a NIH shift mechanism (Karich et al., 2013) (Figure 2C). However, this process has two major drawbacks: poor selectivity and severe radical side reactions. To improve selectivity, different substitution groups can be introduced on benzene to anchor substrates in binding cavity, so that specific positions can be directed to Fe=O·+ of compound I. To prevent radical reaction, using radical scavengers such as vitamin C is a highly effective way (Kinne et al., 2008). Furthermore, protein engineering can reduce the peroxidative activity of UPOs, which is responsible for phenol polymerization (Molina-Espeja et al., 2016). Recently, Zhang and coworkers expanded the scope of aromatic hydroxylation by introducing azide as nucleophiles. This approach facilitates subsequent chemical methods, such as rearomatization, hydrogenation, and cycloaddition, enabling the synthesis of compounds with additional functional groups (Zhang et al., 2021).
In the very recent 3 years, due to in-depth understanding of the catalytic mechanism of heme-dependent enzymes (e.g., P450 monooxygenases, horseradish peroxidases and unspecific peroxygenases), the types and applications of reactions catalyzed by UPOs have been significantly expanded (Figures 3, 4).
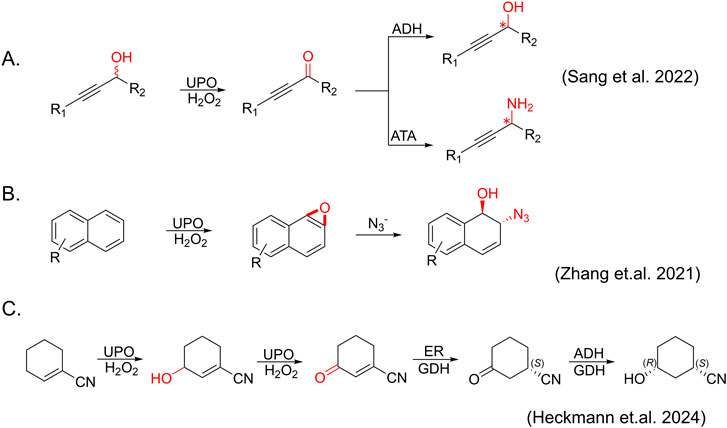
Figure 3. Newly expanded cascade reactions catalyzed by AaeUPO. (A) Dearomatization of naphthalene and its derivatives. (B) Synthesis of enantiopure propargylic alcohols and amines catalyzed by UPO, ADH (alcohol dehydrogenase) and ATA (amine transaminases) (C) Synthesis of (1S,3R)-3-Hydroxycyclohexanecarbonitrile catalyzed by UPO, ER (ene-reductase), ADH and using GDH (glucose dehydrogenase) as an NADH (nicotinamide adenine dinucleotide + hydrogen) recycling system.
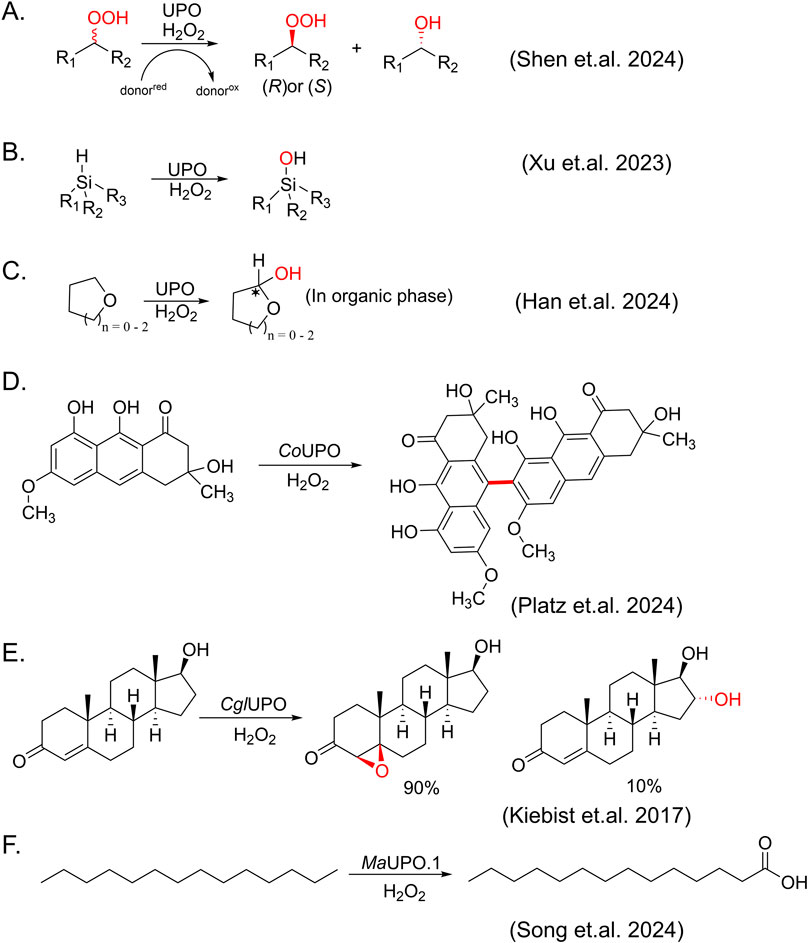
Figure 4. Newly expanded reactions catalyzed by UPOs. (A) Kinetic resolution of organoperoxides. (B) Oxidation of silanes to silanols. (C) Synthesis of chiral cyclic hemiacetals. (D) Oxidative phenol coupling catalyzed by Cortinarius odorifer unspecific peroxygenase (CoUPO). (E) Selective oxygenation of testosterone by Chaetomium globosum unspecific peroxygenase (CglUPO). (F) Terminal carboxylation of tetradecane catalyzed by Moesziomyces aphidis XM01 unspecific peroxygenase (MaUPO.1).
Notably, combining UPO-mediated oxidation reactions with subsequent catalytic transformations represents a more efficient and atom-economical approach, offering an attractive alternative for producing complex molecules, including pharmaceuticals, agrochemicals, and fine chemicals (Zhang et al., 2021). For example, Sang et al. demonstrated a straightforward enzymatic cascade strategy for synthesizing optically pure propargylic alcohols and amines from racemic propargylic alcohols using AaeUPO in combination with enantioselective alcohol dehydrogenase (ADH) or amine transaminases (ATA) (Sang et al., 2022) (Figure 3A). Zhang et al. showed a chemo-enzymatic cascade for the synthesis of trans-disubstituted cyclohexadiene derivates through AaeUPO-catalyzed aromatic epoxidation and nucleophilic ring opening as already mentioned (Zhang et al., 2021) (Figure 3B). Very recently, Heckmann et al. demonstrated a telescoped synthesis of (1S,3R)-3 hydroxycyclohexanecarbonitrile, the active pharmaceutical ingredient (API) building block of LPA1-antagonist BMS-986278, using a three-step, one-pot UPO - ene-reductase (ER) - ADH cascade (Heckmann et al., 2024) (Figure 3C). These cases of UPO cascade provide high yields and enantioselectivity, showcasing their potential for efficient and sustainable synthesis of chiral molecules potentially used in pharmaceuticals and fine chemicals.
Furthermore, building on the advancements in understanding the catalytic mechanisms of heme-dependent enzymes, recent reports have also described some reactions that were not known before. For example, UPOs are typically known as oxidases that use hydrogen peroxide to oxidize substrates and reduce hydrogen peroxide to water. However, Shen and co-workers have demonstrated the first example of AaeUPO-catalyzed reductive kinetic resolution of racemic organoperoxides through asymmetric reduction, which is a departure from its usual role as an oxidase (Shen et al., 2024) (Figure 4A). In this study, organoperoxides serve as a substitute for hydrogen peroxide, leading to the formation of Compound I. Using only (R)-organoperoxides and guaiacol as a sacrificial electron donor, the model reaction shows a high turnover number up to 60,000 with excellent enantioselectivity (e.g., up to 99% ee for (S)-organoperoxide product). 18 substrates and some commercial mutants of AaeUPO with chirality inversion are also demonstrated with this reduction reaction, showing a robust and selective approach to obtain optically pure organoperoxides. Another interesting reaction is the selective oxidation of silanes to silanols catalyzed by peroxygenase. It is well established that UPOs catalyze C-H activation. Given the physiochemical similarity between Si and C, and the lower bond dissociation energy of Si-H bonds (approximately 10 kcal/mol lower than C-H bonds), activation of Si-H bonds is also possible with UPO catalysis. Xu et al. represented hydroxylation of organosilanes by AaeUPO, showing a rather broad range of silanes subjected to oxidation for silanol synthesis (Xu et al., 2023) (Figure 4B). Interestingly, for such insoluble compounds, increasing dosage of both enzyme and hydrogen peroxide, even beyond conventional limits, can significantly enhance product concentrations. This underscores the remarkable stability of AaeUPO, highlighting its potential for the conversion of insoluble substrates. Very recently, Han et al. demonstrated an enzymatic synthesis method for producing chiral cyclic hemiacetals through reaction engineering, successfully surpassing the instability issues of hemiacetal compounds in aqueous environments (Han et al., 2024) (Figure 4C). Starting from C-H bond oxyfunctionalization, AaeUPO directly yielded chiral hemiacetal products, which is difficult to achieve with either chemical or enzymatic methods. Moreover, Platz et al. revealed an unprecedented UPO-mediated asymmetric oxidative phenol coupling (OPC) reaction by heterologous expression and feeding experiments (Platz et al., 2024) (Figure 4D). This is a pioneer report of UPO-catalyzed C–C bond formation to produce natural products. Prior to this, OPC reactions generating bioactive dimeric (pre-)anthraquinones had only been observed in cytochrome P450 enzymes, peroxidases, or laccases (Fürtges et al., 2019; Thiele et al., 2020; Zetzsche and Narayan, 2020; Zhao et al., 2022).
With the continuous discovery of new enzymes, an increasing number of new reactions are also being expected. Steroids are an important class of drug molecules, widely used in the treatment of inflammation, immune system disorders and cancer. Hydroxylation of steroid drugs is crucial for enhancing their bioactivity and metabolic stability. However, early research found that AaeUPO failed to oxidase substrates like Reichstein substance S and 17α-ethinylestradiol, instead favoring steroids with a C17 alkyl side chain and predominantly producing 25-hydroxy derivative (Poraj-Kobielska et al., 2011). In 2017, Kiebist et al. showed an example of UPO-catalyzed oxygenation of testosterone (Kiebist et al., 2017) (Figure 4E). CglUPO from Chaetomium globosum accepted testosterone as substrate and converted it into two oxygenated products: the 4,5-epoxide of testosterone in β-configuration and 16α-hydroxytestosterone, indicating a broader or more flexible substrate channel. Furthermore, recent advancements have highlighted the versatility of UPO enzymes. As mentioned above, linear alkanes cannot be transformed to corresponding acids by AaeUPO. Recently, MaUP O .1 from a marine Basidiomycetous yeast, Moesziomyces aphidis XM01, was found to exhibit the ability to carboxylate n-tetradecane to tetradecanoic acid as MroUPO did (Song et al., 2024) (Figure 4F). These new enzymes not only expand the scope and selectivity of reactions but also offer the possibility of conducting complex chemical synthesis under mild conditions.
3 Challenges associated with the organic transformations
Despite demonstrating excellent capabilities in organic synthesis, UPOs indeed need to address some issues in catalyzing organic transformation reactions. These included comparably low substrate concentration, poor selectivity for specific substrates and feasibility issues in scaling up. Apart from protein engineering, alternative approaches such as substrate engineering, reaction engineering and process optimization can address these issues. Additionally, developing robust bioprocesses suitable for industrial scale-up and production is crucial. In this context, we will not delve into the specifics of every individual study employing the aforementioned strategies. Instead, we will highlight a few selected examples that effectively showcase the concept of enhancing the robustness of peroxygenase catalysis (Figure 5).
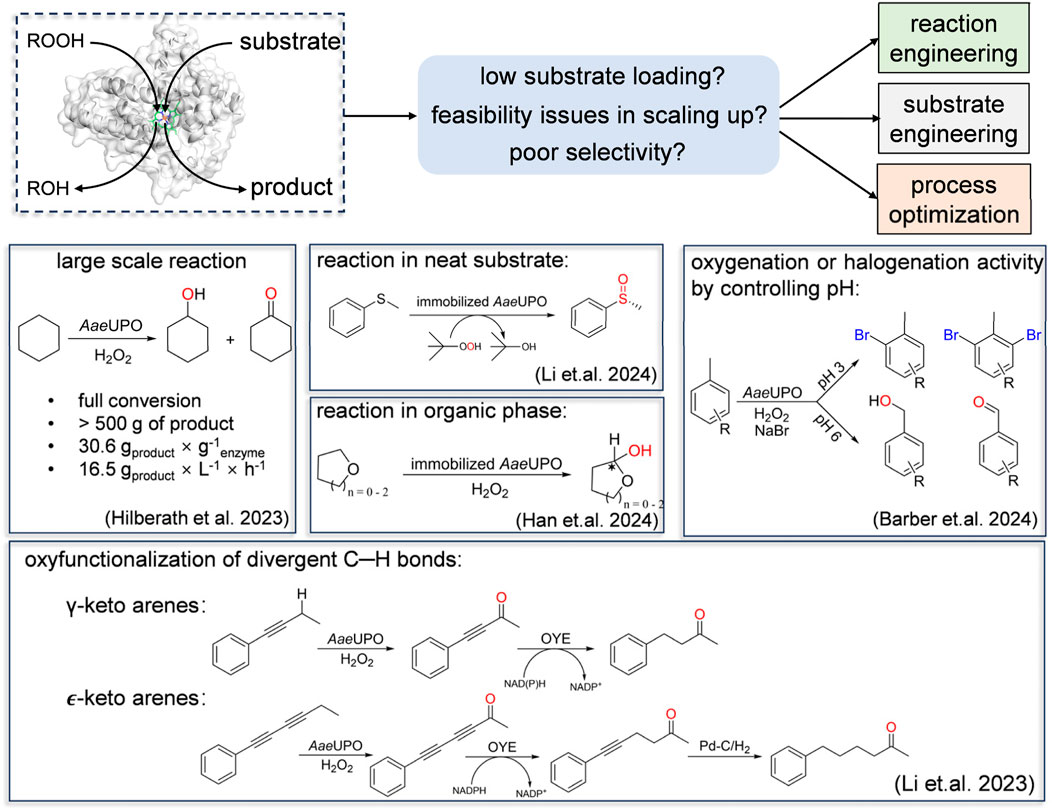
Figure 5. Selected examples that effectively showcase the concept of enhancing the robustness of peroxygenase catalysis. Including large scale reaction, reaction in neat substrate, reaction in organic phase, shift of oxygenation and halogenation activity by controlling pH and oxyfunctionalization of divergent C-H bonds.
Improving the substrate loading product titer is key to steer the application of peroxygenase. In 2011, Hollmann’s group reported a selective, peroxygenase-catalyzed oxidation of cyclohexane, however, with very low substrate loading and consequently the product concentrations. Given the extraordinary stability and activity of AaeUPO, recently they evaluated the ability towards kilogram-scale in synthesis of ketone-alcohol oil (KA-oil). Increasing the enzyme concentration led to a 2.7-fold improvement in the turnover number (TON), which rose from 7,000 to 19,500. This enhancement enabled respectable productivities, achieving a rate of 157 mM h⁻1 in the mol-scale oxyfunctionalization of cyclohexane to cyclohexanol/cyclohexanone (KA-oil). Suitable immobilization strategies will further improve the economic attractiveness. Since UPOs’ substrates are typically hydrophobic, high concentrations of cosolvents are often required, which can induce conformational changes and potentially disrupt enzyme activity. Immobilized peroxygenases are known for their extended stability and enhanced resilience to reaction conditions. For instance, Li et al. showed a peroxygenase-catalyzed sulfoxidation in neat substrate using immobilized enzymes (Li et al., 2024). However, it should be noted that a solvent-free reaction (which on the other means incomplete conversion) is not as advantageous as one using a cosolvent that enables full conversion. This underscores the importance of process optimization.
Reaction engineering plays a crucial role in enhancing selectivity and efficiency in chemical reactions. By optimizing reactor design and reaction conditions, selectivity for desired products can be significantly enhanced. Barber et al. demonstrated that AaeUPO can undergo a transformation from hydroxylation to halogenation, highlighting their interconversion depending on pH adjustment (Barber et al., 2024). Acidic conditions facilitate halogen ionization in the reaction medium, enhancing their reactivity with substrates and promoting the formation of halogenation products. By understanding and controlling the properties and reaction kinetics of transient intermediates, it is possible to manipulate product selectivity in chemical reactions. For example, Zhang et al. shifted the reactivity of aromatic C-H hydroxylation towards a dearomatization reaction by capturing the aromatic oxide, an instantaneous intermediate for the NIH shift to phenols (Zhang et al., 2021) (Figure 3B). In established reactions catalyzed by UPOs, the demethylation process involves a hemiacetal intermediate that is unstable in aqueous solutions. As a result, this intermediate has not been well-regarded in previous enzyme catalysis studies. Han et al. utilized principles of reaction engineering to hinder the hydrolysis of hemiacetals, enabling AaeUPO to synthesize chiral cyclic hemiacetals (Han et al., 2024). Subsequently, they further transformed the hemiacetals into lactones by adjusting pH. This study demonstrates the practical application of reaction engineering and further proves that precise control of reaction conditions and catalytic systems opens new potentials of peroxygenases as versatile biocatalysts in synthetic chemistry.
Enhancing catalytic selectivity can be achieved not only by modifying the enzyme but also by altering the substrate. Substrate engineering maximizes enzyme-substrate interactions, improving efficiency, specificity, and expanding reaction possibilities. For example, ethyl benzene and it homologue toluene showed different selectivity in AaeUPO–mediated reaction, highlighting the influence of aromatic ring substitution on substrate binding to Cpd I. Inspired by the high selectivity with ethyl benzene, Wang et al. introduced functional groups onto the benzene ring to optimize the interaction between the substrate and the enzyme, improving reaction efficiency and selectivity (Wang et al., 2023) (Figure 2A). Similarly, Li et al. extended the advantageous activity and selectivity at the propargylic position by introducing a rigid alkynyl group. This strategy, coupled with an ene-reductase (an old yellow enzyme (OYE)), presents a simple access to γ- and ɛ-keto arenes (Li et al., 2023).
4 Protein engineering of UPOs
Though unspecific peroxygenases have been emerging as a highly promising catalyst, the often-observed low regioselectivity and catalytic activity is particularly considered as flaw in the pharmaceutical application. This sector will thus focus on reviewing the inherent issues of UPO that can be addressed by protein engineering. More specifically, the chapter will summarize current methods (Table 1) and efforts in protein engineering to enhance UPO’s catalytic performance (Figure 6).
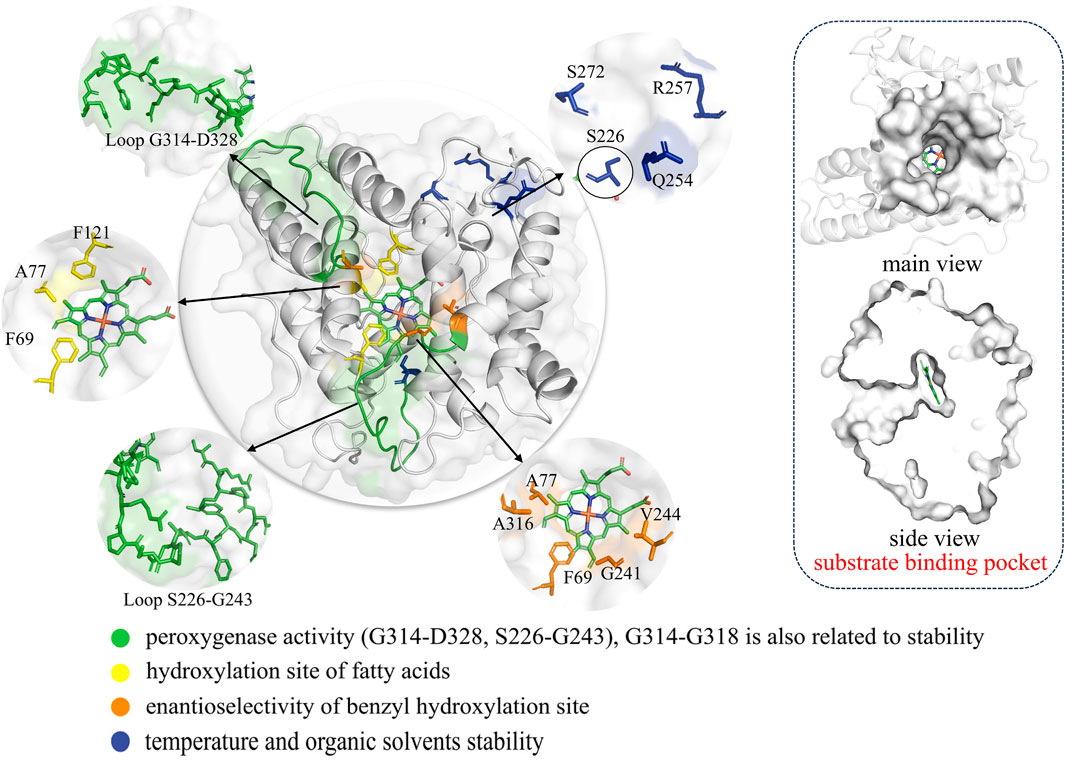
Figure 6. The structure of AaeUPO (PDB ID 5OXU) including complete structure, substrate binding pocket and the key mutation sites associated with enzymatic functions are highlighted in different colors. In this image, S226 is distinguished from the other blue sites by the addition of a black border, because they are far apart in space.
The first challenge in the research and application of UPOs has been their extremely low levels of heterologous production. This limitation hinders their practical use in research settings. According to incomplete statistics, from 2004 to 2014, only 40 papers related to UPO (using keywords such as A. aegerita haloperoxidase, aromatic peroxygenase and unspecific peroxygenase) were published on PubMed. However, following 2014 there has been more than 150 related papers up to now, and the number of publications continues to rise. This significant shift is primarily attributed to the groundbreaking research by Alcalde and coworkers. In 2014, a variant (PaDa-I) containing nine mutations was obtained through five rounds of directed evolution, four of nine were in signal peptide. This mutant had an expression level of 7.8 mg/L in Saccharomyces cerevisiae, which was 1,114 times higher than the wild type, and its total enzyme activity increased by 3,250 times. And signal peptides are considered important factors affecting UPO expression. The success of the PaDa-I mutant highlighted the potential of directed evolution in enhancing protein function and yield. Subsequently, the group further increased the expression level to 217 mg/L in Pichia pastoris, demonstrating that optimizing the expression system can significantly improve UPO production efficiency (Molina-Espeja et al., 2015).
The successful expression of PaDa-I undoubtedly provides confidence and valuable experience for subsequent UPO expression efforts. Later, heterologous expression levels of various fungal peroxygenases were enhanced through redesigning their promoters and signal peptides, as well as by employing structural and sequence alignment for site-directed mutagenesis (Gomez de Santos et al., 2021; Pullmann and Weissenborn, 2021), Recently, with the advancement of AI technology, success rates have been enhancing through structural modeling and computational prediction, thereby reducing the need for manual workload (Munch et al., 2024).
As UPOs exist peroxygenative (O-transferring two-electron oxidations) and peroxidative activities (one-electron oxidation reactions) in the same biocatalyst, the latter activity, however, often leads to significant side reactions (e.g., the well-known of benzene oxidation to phenol followed by polymerization), reducing the yield of the desired products. Introduction of free radical scavengers (such as vitamin C) into the reaction can mitigate these side reactions (Gomez de Santos et al., 2018). However, the introduction of new cosubstrates complicates the reaction system. Alcalde’s group engineered PaDa-I through random mutagenesis and semi-rational design to obtain two mutants, JaWa and SoLo, which exhibit high activity and regioselectivity for aromatic compounds.
By combining computational simulations with saturation mutagenesis, Molina-Espeja et al. identified the origins of UPO peroxygenase activity, emphasizing two flexible loops (S226-G243 and G314-D328) on the heme tunnel as the primary regions responsible for this activity (Molina-Espeja et al., 2021) (Figure 6). These studies can provide valuable guidance and inspiration for addressing specific issues related to different substrates and reactions. However, achieving optimal selectivity and efficiency in UPO-mediated reactions remains a significant challenge.
Although UPOs exhibit a broad substrate scope, their selectivity—chemo-, regio-, and enantio-selectivity—varies with specific substrates. Therefore, screening natural enzymes to identify more suitable parent enzymes, which possess different active site conformations, is an important initial step. Undoubtedly, enzyme engineering aimed at altering selectivity often focuses on the active site pocket, establishing small but smart mutant libraries through methods such as saturation mutagenesis, CAST/ISM, and focused rational iterative site-specific mutagenesis (FRISM).
The hydroxylation of fatty acids is an attractive reaction in synthetic chemistry; however, its industrial application is hindered by the lack of selective catalysts. For example, PaDa-I exhibits hydroxylation activity at the ω-1 and ω-2 positions and is prone to overoxidation, forming fatty ketonic acids. Therefore, refining PaDa-I through enzyme engineering is crucial. Based on structure-guided active site modification, A77 is prominently located in the same plane as the Phe tripod, approximately 4.4–5.0 Å away from Phe121 and Phe69 (Figure 6). It directs towards the inner heme channel, influencing its diameter. This suggests that even subtle modifications at this position could affect substrate diffusion and positioning during catalysis. Indeed, through saturation mutagenesis at position 77, a mutant named Fett (A77L) was obtained that exclusively hydroxylates at the ω-1 position (Gomez de Santos et al., 2023a). Short-chain UPOs exhibit higher catalytic efficiency and selectivity towards aliphatic substrates compared to PaDa-I, possibly due to the number of phenylalanine residues in their heme pocket and channel (Linde et al., 2020). However, aliphatic compounds exhibit greater flexibility, leading to insufficient selectivity in catalysis by short-chain UPOs. In order to significantly enhance the regional and chemical selectivity of UPOs towards unsaturated fatty acids, Carro et al. used a rational design strategy combined with gas chromatography-mass spectrometry and computer-assisted methods. They identified the I153 position in MroUPO as a key site. As a result, the I153T variant exhibited enhanced epoxidation capability, while the I153F/S156F mutations completely eliminated epoxidation activity (Carro et al., 2019). It is evident that determining key sites based on structural or computational assistance indeed results in higher success rates compared to random mutagenesis.
If the implementation of the above methods still involves complexity and technical difficulty, such as requiring a deep understanding of structural research or highly specialized software and algorithmic support, then the use of AI to automatically design efficient and functionally diverse enzyme libraries are more appealing. Recently, Gomez de Santos et al. utilized FuncLib to modify the stereoselectivity of PaDa-I (Gomez de Santos et al., 2023b). FuncLib integrates Rosetta atomic modeling and evolutionary conservation principles to automate the analysis and design of multi-point mutations in enzyme active sites. It successfully designed 30 mutants, with 24 expressing effectively, each carrying 4-5 amino acid mutations in the catalytic center. This approach achieved unprecedented chiral inversion and improved catalytic efficiency by 10-fold, with a total turnover number 15 times higher than that of the parental enzyme.
5 Conclusion remarks
UPOs have gained significant attention in the field of biocatalysis due to their high catalytic activity, broad substrate specificity, and extracellular stability. However, during the catalytic transformations their chemo-, regio- and enantio-selectivity varies with specific substrates, thus limiting their applications in organic synthesis. In recent years, progress has been made through substrate, reaction and enzyme engineering to enhance the robustness of UPOs. However, challenges still remain in using UPOs in organic synthesis. The primary challenges involve discovering new enzymes for specific reactions and exploring novel reactions for the discovered enzymes. Although databases contain over 7,000 entries labeled as UPO-like enzymes, understanding protein functions and leveraging them in bioengineering have greatly advanced in catalysis. These advancements are all reliant on the development of ultra-high-throughput screening methods, including analytical detection technique and virtual screening approaches (Romero et al., 2022; Huang et al., 2023). Continued innovation in these areas will be crucial for overcoming existing challenges and unlocking the full potential of UPOs in industrial applications.
The demand for biocatalysis and its applications, in turn, drives the development of enzyme engineering technologies. This motivates researchers to design and modify more optimized biocatalysts to meet market and environmental needs. One promising future direction for UPOs is the exploration of new reactions and expansion of substrate/product scopes to unlock their full potential in organic synthesis. Also, the selective oxidation of complex natural products, particularly in the synthesis of chiral pharmaceuticals, are appealing. With the power of protein engineering, it is anticipated that the identified weaknesses of UPOs can be effectively addressed on a case-by-case basis. However, it is essential to acknowledge that challenges associated with protein engineering, including the establishment of high throughput screening methods, reduction of mutant libraries, and the development of efficient engineering techniques, represent significant tasks that need to be tackled.
Author contributions
YH: Writing–original draft, Writing–review and editing. JS: Writing–original draft, Writing–review and editing. JZ: Writing–original draft, Writing–review and editing. WZ: Conceptualization, Funding acquisition, Writing–original draft, Writing–review and editing.
Funding
The author(s) declare that financial support was received for the research, authorship, and/or publication of this article. The work was financially supported by the National Natural Science Foundation of China (No. 32171253), and the Tianjin Synthetic Biotechnology Innovation Capacity Improvement Project (No. TSBI-CIP-CXRC-032).
Acknowledgments
We acknowledge the use of ChatGPT (version GPT-4, developed by OpenAI) for language refinement in this manuscript.
Conflict of interest
The authors declare that the research was conducted in the absence of any commercial or financial relationships that could be construed as a potential conflict of interest.
The author(s) declared that they were an editorial board member of Frontiers, at the time of submission. This had no impact on the peer review process and the final decision.
Publisher’s note
All claims expressed in this article are solely those of the authors and do not necessarily represent those of their affiliated organizations, or those of the publisher, the editors and the reviewers. Any product that may be evaluated in this article, or claim that may be made by its manufacturer, is not guaranteed or endorsed by the publisher.
References
Barber, V., Mielke, T., Cartwright, J., Díaz-Rodríguez, A., Unsworth, W. P., and Grogan, G. (2024). Unspecific peroxygenase (UPO) can be tuned for oxygenation or halogenation activity by controlling the reaction pH. Chemistry 30 (40), e202401706. doi:10.1002/chem.202401706
Beltrán-Nogal, A., Sánchez-Moreno, I., Méndez-Sánchez, D., Gómez de Santos, P., Hollmann, F., and Alcalde, M. (2022). Surfing the wave of oxyfunctionalization chemistry by engineering fungal unspecific peroxygenases. Curr Opin Struct Biol 73, 102342. doi:10.1016/j.sbi.2022.102342
Carro, J., González-Benjumea, A., Fernández-Fueyo, E., Aranda, C., Guallar, V., Gutiérrez, A., et al. (2019). Modulating fatty acid epoxidation vs hydroxylation in a fungal peroxygenase. ACS Catal. 9 (7), 6234–6242. doi:10.1021/acscatal.9b01454
Fürtges, L., Obermaier, S., Thiele, W., Foegen, S., and Müller, M. (2019). Diversity in fungal intermolecular phenol coupling of polyketides: regioselective laccase-based systems. ChemBioChem 20 (15), 1928–1932. doi:10.1002/cbic.201900041
Gomez de Santos, P., Canellas, M., Tieves, F., Younes, S. H., Molina-Espeja, P., Hofrichter, M., et al. (2018). Selective synthesis of the human drug metabolite 5'-hydroxypropranolol by an evolved self-sufficient peroxygenase. ACS Catal. 8 (6), 4789–4799. doi:10.1021/acscatal.8b01004
Gomez de Santos, P., González-Benjumea, A., Fernandez-Garcia, A., Aranda, C., Wu, Y., But, A., et al. (2023a). Engineering a highly regioselective fungal peroxygenase for the synthesis of hydroxy fatty acids. Angew. Chem. Int. Ed. Engl. 62 (9), e202217372. doi:10.1002/anie.202217372
Gomez de Santos, P., Hoang, M. D., Kiebist, J., Kellner, H., Ullrich, R., Scheibner, K., et al. (2021). Functional expression of two unusual acidic peroxygenases from Candolleomyces aberdarensis in yeasts by adopting evolved secretion mutations. Appl. Environ. Microbiol. 87 (19), e0087821. doi:10.1128/AEM.00878-21
Gomez de Santos, P., Mateljak, I., Hoang, M. D., Fleishman, S. J., Hollmann, F., and Alcalde, M. (2023b). Repertoire of computationally designed peroxygenases for enantiodivergent C–H oxyfunctionalization reactions. J. Am. Chem. Soc. 145 (6), 3443–3453. doi:10.1021/jacs.2c11118
Han, X., Chen, F., Li, H., Ge, R., Shen, Q., Duan, P., et al. (2024). Reaction engineering blocks ether cleavage for synthesizing chiral cyclic hemiacetals catalyzed by unspecific peroxygenase. Nat. Commun. 15 (1), 1235. doi:10.1038/s41467-024-45545-z
Heckmann, C. M., Bürgler, M., and Paul, C. E. (2024). Peroxygenase-catalyzed allylic oxidation unlocks telescoped synthesis of (1S,3R)-3-hydroxycyclohexanecarbonitrile. ACS Catal. 14 (5), 2985–2991. doi:10.1021/acscatal.4c00177
Hilberath, T., Van Oosten, R., Victoria, J., Brasselet, H., Alcalde, M., Woodley, J. M., et al. (2023). Toward kilogram-scale peroxygenase-catalyzed oxyfunctionalization of cyclohexane. Org. Process Res. Dev. 27 (7), 1384–1389. doi:10.1021/acs.oprd.3c00135
Hofrichter, M., Kellner, H., Herzog, R., Karich, A., Kiebist, J., Scheibner, K., et al. (2022). Peroxide-mediated oxygenation of organic compounds by fungal peroxygenases. Antioxidants (Basel) 11 (1), 163. doi:10.3390/antiox11010163
Hofrichter, M., Kellner, H., Herzog, R., Karich, A., Liers, C., Scheibner, K., et al. (2020) “Fungal peroxygenases: a phylogenetically old superfamily of heme enzymes with promiscuity for oxygen transfer reactions,” in Grand challenges in fungal biotechnology, 369–403. doi:10.1007/978-3-030-29541-7_14
Hofrichter, M., and Ullrich, R. (2006). Heme-thiolate haloperoxidases: versatile biocatalysts with biotechnological and environmental significance. Appl. Microbiol. Biotechnol. 71 (3), 276–288. doi:10.1007/s00253-006-0417-3
Hofrichter, M., and Ullrich, R. (2014). Oxidations catalyzed by fungal peroxygenases. Curr. Opin. Chem. Biol. 19, 116–125. doi:10.1016/j.cbpa.2014.01.015
Horst, A., Bormann, S., Meyer, J., Steinhagen, M., Ludwig, R., Drews, A., et al. (2016). Electro-enzymatic hydroxylation of ethylbenzene by the evolved unspecific peroxygenase of Agrocybe aegerita. J. Mol. Catal. B-Enzym 133, S137–S142. doi:10.1016/j.molcatb.2016.12.008
Huang, J., Lin, Q., Fei, H., He, Z., Xu, H., Li, Y., et al. (2023). Discovery of deaminase functions by structure-based protein clustering. Cell S0092-8674 (24), 3182–3195.e14. doi:10.1016/j.cell.2023.05.041
Karich, A., Kluge, M., Ullrich, R., and Hofrichter, M. (2013). Benzene oxygenation and oxidation by the peroxygenase of Agrocybe aegerita. Amb. Express 3 (1), 5. doi:10.1186/2191-0855-3-5
Kiebist, J., Holla, W., Heidrich, J., Poraj-Kobielska, M., Sandvoss, M., Simonis, R., et al. (2015). One-pot synthesis of human metabolites of SAR548304 by fungal peroxygenases. Bioorg Med. Chem. 23 (15), 4324–4332. doi:10.1016/j.bmc.2015.06.035
Kiebist, J., Schmidtke, K. U., Zimmermann, J., Kellner, H., Jehmlich, N., Ullrich, R., et al. (2017). A peroxygenase from Chaetomium globosum catalyzes the selective oxygenation of testosterone. ChemBioChem 18 (6), 563–569. doi:10.1002/cbic.201600677
Kinne, M., Poraj-Kobielska, M., Ralph, S. A., Ullrich, R., Hofrichter, M., and Hammel, K. E. (2009). Oxidative cleavage of diverse ethers by an extracellular fungal peroxygenase. J. Biol. Chem. 284 (43), 29343–29349. doi:10.1074/jbc.M109.040857
Kinne, M., Ullrich, R., Hammel, K. E., Scheibner, K., and Hofrichter, M. (2008). Regioselective preparation of (R)-2-(4-hydroxyphenoxy) propionic acid with a fungal peroxygenase. Tetrahedron Lett. 49 (41), 5950–5953. doi:10.1016/j.tetlet.2008.07.152
Kinner, A., Rosenthal, K., and Lütz, S. (2021). Identification and expression of new unspecific peroxygenases–recent advances, challenges and opportunities. Fron Bioeng Biotechnol 9, 705630. doi:10.3389/fbioe.2021.705630
Kluge, M., Ullrich, R., Dolge, C., Scheibner, K., and Hofrichter, M. (2009). Hydroxylation of naphthalene by aromatic peroxygenase from Agrocybe aegerita proceeds via oxygen transfer from H2O2 and intermediary epoxidation. Appl. Microbiol. Biotechnol. 81 (6), 1071–1076. doi:10.1007/s00253-008-1704-y
Knorrscheidt, A., Soler, J., Hünecke, N., Püllmann, P., Garcia-Borràs, M., and Weissenborn, M. J. (2021a). Accessing chemo- and regioselective benzylic and aromatic oxidations by protein engineering of an unspecific peroxygenase. ACS Catal. 11 (12), 7327–7338. doi:10.1021/acscatal.1c00847
Knorrscheidt, A., Soler, J., Hünecke, N., Püllmann, P., Garcia-Borràs, M., and Weissenborn, M. J. (2021b). Simultaneous screening of multiple substrates with an unspecific peroxygenase enabled modified alkane and alkene oxyfunctionalisations. Catal. Sci. Technol. 11 (18), 6058–6064. doi:10.1039/d0cy02457k
Li, H., Shen, Q., Zhou, X., Duan, P., Hollmann, F., Huang, Y., et al. (2024). Peroxygenase-catalysed sulfoxidations in non-aqueous media. ChemSusChem 17 (6), e202301321. doi:10.1002/cssc.202301321
Li, H., Zhang, Y., Huang, Y., Duan, P., Ge, R., Han, X., et al. (2023). A simple access to γ-and ε-keto arenes via enzymatic divergent C-H bond oxyfunctionalization. Adv. Sci. (Weinh) 10 (34), 2304605. doi:10.1002/advs.202304605
Linde, D., Olmedo, A., González-Benjumea, A., Estévez, M., Renau-Mínguez, C., Carro, J., et al. (2020). Two new unspecific peroxygenases from heterologous expression of fungal genes in Escherichia coli. Appl Environ Microbiol. Appl. Environ. Microbiol. 86 (7), e02899–e02819. doi:10.1128/AEM.02899-19
Martin-Diaz, J., Paret, C., García-Ruiz, E., Molina-Espeja, P., and Alcalde, M. (2018). Shuffling the neutral drift of unspecific peroxygenase in Saccharomyces cerevisiae. Appl. Environ. Microbiol. 84 (15), e00808-18–e00818. doi:10.1128/AEM.00808-18
Martínez, A. T., Ruiz-Dueñas, F. J., Camarero, S., Serrano, A., Linde, D., Lund, H., et al. (2017). Oxidoreductases on their way to industrial biotransformations. Biotechnol. Adv. 35 (6), 815–831. doi:10.1016/j.biotechadv.2017.06.003
Mate, D. M., Palomino, M. A., Molina-Espeja, P., Martin-Diaz, J., and Alcalde, M. (2017). Modification of the peroxygenative: peroxidative activity ratio in the unspecific peroxygenase from Agrocybe aegerita by structure-guided evolution. Protein Eng. Des. Sel. 30 (3), 189–196. doi:10.1093/protein/gzw073
Molina-Espeja, P., Beltran-Nogal, A., Alfuzzi, M. A., Guallar, V., and Alcalde, M. (2021). Mapping potential determinants of peroxidative activity in an evolved fungal peroxygenase from Agrocybe aegerita. Front. Bioeng. Biotechnol. 9, 741282. doi:10.3389/fbioe.2021.741282
Molina-Espeja, P., Cañellas, M., Plou, F. J., Hofrichter, M., Lucas, F., Guallar, V., et al. (2016). Synthesis of 1-naphthol by a natural peroxygenase engineered by directed evolution. ChemBioChem 17 (4), 341–349. doi:10.1002/cbic.201500493
Molina-Espeja, P., Garcia-Ruiz, E., Gonzalez-Perez, D., Ullrich, R., Hofrichter, M., Alcalde, M., et al. (2014). Directed evolution of unspecific peroxygenase from Agrocybe aegerita. Appl. Environ. Microbiol. 80 (11), 3496–3507. doi:10.1128/aem.00490-14
Molina-Espeja, P., Ma, S., Mate, D. M., Ludwig, R., and Alcalde, M. (2015). Tandem-yeast expression system for engineering and producing unspecific peroxygenase. ChemBioChem 73, 29–33. doi:10.1016/j.enzmictec.2015.03.004
Munch, J., Dietz, N., Barber-Zucker, S., Seifert, F., Matschi, S., Pullmann, P., et al. (2024). Functionally diverse peroxygenases by Alphafold2, design, and signal peptide shuffling. ACS Catal. 14 (7), 4738–4748. doi:10.1021/acscatal.4c00883
Munch, J., Soler, J., Hunecke, N., Homann, D., Garcia-Borras, M., and Weissenborn, M. J. (2023). Computational-aided engineering of a selective unspecific peroxygenase toward enantiodivergent β-ionone hydroxylation. ACS Catal. 13 (13), 8963–8972. doi:10.1021/acscatal.3c00702
Olmedo, A., Aranda, C., Del Río, J. C., Kiebist, J., Scheibner, K., Martínez, A. T., et al. (2016). From alkanes to carboxylic acids: terminal oxygenation by a fungal peroxygenase. Angew. Chem. Int. Ed. Engl. 128 (40), 12436–12439. doi:10.1002/ange.201605430
Pecyna, M. J., Ullrich, R., Bittner, B., Clemens, A., Scheibner, K., Schubert, R., et al. (2009). Molecular characterization of aromatic peroxygenase from Agrocybe aegerita. Appl. Microbiol. Biotechnol. 84 (5), 885–897. doi:10.1007/s00253-009-2000-1
Peter, S., Kinne, M., Ullrich, R., Kayser, G., and Hofrichter, M. (2013). Epoxidation of linear, branched and cyclic alkenes catalyzed by unspecific peroxygenase. Enzyme Microb Technol 52 (6), 370–376. doi:10.1016/j.enzmictec.2013.02.013
Peter, S., Kinne, M., Wang, X., Ullrich, R., Kayser, G., Groves, J. T., et al. (2011). Selective hydroxylation of alkanes by an extracellular fungal peroxygenase. FEBS J. 278 (19), 3667–3675. doi:10.1111/j.1742-4658.2011.08285.x
Platz, L., Löhr, N. A., Girkens, M. P., Eisen, F., Bär, C., Braun, K., et al. (2024). Regioselective oxidative phenol coupling by a mushroom unspecific peroxygenase. Angew. Chem. Int. Ed. Engl., e202407425. doi:10.1002/anie.202407425
Poraj-Kobielska, M., Kinne, M., Ullrich, R., Scheibner, K., Kayser, G., Hammel, K. E., et al. (2011). Preparation of human drug metabolites using fungal peroxygenases. Biochem. Pharmacol. 82 (7), 789–796. doi:10.1016/j.bcp.2011.06.020
Pullmann, P., and Weissenborn, M. J. (2021). Improving the heterologous production of fungal peroxygenases through an episomal Pichia pastoris promoter and signal peptide shuffling system. ACS Catal. 10 (6), 1360–1372. doi:10.1021/acssynbio.0c00641
Romero, E., Johansson, M. J., Cartwright, J., Grogan, G., and Hayes, M. A. J. A. C. (2022). Oxalate oxidase for in situ H2O2-generation in unspecific peroxygenase-catalysed drug oxyfunctionalisations. Angew. Chem. Int. Ed. Engl. 134 (39), e202207831. doi:10.1002/anie.202207831
Sang, X., Tong, F., Zeng, Z., Wu, M., Yuan, B., Sun, Z., et al. (2022). A biocatalytic platform for the synthesis of enantiopure propargylic alcohols and amines. Org. Lett. 24 (23), 4252–4257. doi:10.1021/acs.orglett.2c01547
Shen, Q., Yan, J., Han, Y., Zhang, Z., Li, H., Kong, D., et al. (2024). Peroxygenase-enabled reductive kinetic resolution for the enantioenrichment of organoperoxides. Angew. Chem. Int. Ed. Engl. 136 (21), e202401590. doi:10.1002/anie.202401590
Song, J.-Z., Wang, C.-Q., Yu, G.-S., Sun, Z., Wu, A.-H., Chi, Z.-M., et al. (2024). Simultaneous production of biosurfactant and extracellular unspecific peroxygenases by Moesziomyces aphidis XM01 enables an efficient strategy for crude oil degradation. J. Hazard Mater 471, 134437. doi:10.1016/j.jhazmat.2024.134437
Thiele, W., Froede, R., Steglich, W., and Müller, M. (2020). Enzymatic Formation of rufoschweinitzin, a binaphthalene from the basidiomycete Cortinarius rufoolivaceus. ChemBioChem 21 (10), 1423–1427. doi:10.1002/cbic.201900742
Ullrich, R., Nuske, J., Scheibner, K., Spantzel, J., and Hofrichter, M. (2004). Novel haloperoxidase from the agaric basidiomycete Agrocybe aegerita oxidizes aryl alcohols and aldehydes. Appl. Environ. Microbiol. 70 (8), 4575–4581. doi:10.1128/aem.70.8.4575-4581.2004
Wang, Y., Teetz, N., Holtmann, D., Alcalde, M., van Hengst, J. M., Liu, X., et al. (2023). Selective peroxygenase-catalysed oxidation of toluene derivates to benzaldehydes. ChemCatChem 15 (13), e202300645. doi:10.1002/cctc.202300645
Xu, X., van Hengst, J. M., Mao, Y., Martinez, M., Roda, S., Floor, M., et al. (2023). Peroxygenase-catalysed selective oxidation of silanes to silanols. Angew. Chem. Int. Ed. Engl. 135 (24), e202302844. doi:10.1002/anie.202302844
Yan, X., Zhang, X., Li, H., Deng, D., Guo, Z., Kang, L., et al. (2024). Engineering of unspecific peroxygenases using a superfolder-green-fluorescent-protein-mediated secretion system in Escherichia coli. JACS Au 4, 1654–1663. doi:10.1021/jacsau.4c00129
Zetzsche, L. E., and Narayan, A. R. H. (2020). Broadening the scope of biocatalytic C–C bond formation. Nat. Rev. Chem. 4 (7), 334–346. doi:10.1038/s41570-020-0191-2
Zhang, W., Li, H., Younes, S. H., Gómez de Santos, P., Tieves, F., Grogan, G., et al. (2021). Biocatalytic aromaticity-breaking epoxidation of naphthalene and nucleophilic ring-opening reactions. ACS Catal. 11 (5), 2644–2649. doi:10.1021/acscatal.0c05588
Zhang, Y., Pan, X., Shi, T., Gu, Z., Yang, Z., Liu, M., et al. (2023). P450Rdb: a manually curated database of reactions catalyzed by cytochrome P450 enzymes. J. Adv. Res. S2090-1232 (23), 35–42. doi:10.1016/j.jare.2023.10.012
Keywords: unspecific peroxygenases, biocatalysis, protein engineering, oxygenation, oxidoreductases, directed evolution
Citation: Huang Y, Sha J, Zhang J and Zhang W (2024) Challenges and perspectives in using unspecific peroxygenases for organic synthesis. Front. Catal. 4:1470616. doi: 10.3389/fctls.2024.1470616
Received: 25 July 2024; Accepted: 30 September 2024;
Published: 15 October 2024.
Edited by:
Frank Hollmann, Delft University of Technology, NetherlandsReviewed by:
Marco Girhard, Heinrich Heine University of Düsseldorf, GermanyDavid González Pérez, Spanish National Research Council (CSIC), Spain
Copyright © 2024 Huang, Sha, Zhang and Zhang. This is an open-access article distributed under the terms of the Creative Commons Attribution License (CC BY). The use, distribution or reproduction in other forums is permitted, provided the original author(s) and the copyright owner(s) are credited and that the original publication in this journal is cited, in accordance with accepted academic practice. No use, distribution or reproduction is permitted which does not comply with these terms.
*Correspondence: Wuyuan Zhang, emhhbmd3eUB0aWIuY2FzLmNu
†These authors have contributed equally to this work