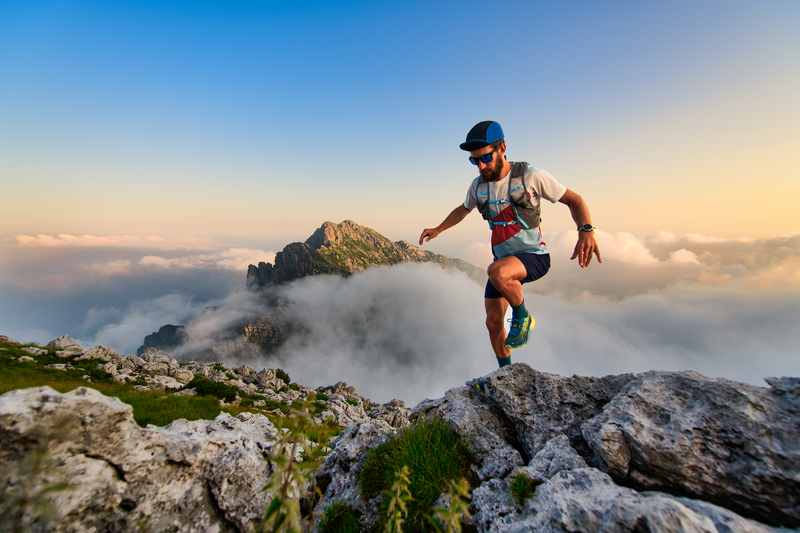
95% of researchers rate our articles as excellent or good
Learn more about the work of our research integrity team to safeguard the quality of each article we publish.
Find out more
REVIEW article
Front. Catal. , 16 February 2024
Sec. Biocatalysis
Volume 4 - 2024 | https://doi.org/10.3389/fctls.2024.1360702
This article is part of the Research Topic Thought Leaders in Catalytic Research View all 3 articles
Biodiesel is synthesized by the transesterification of triglycerides of oils with short-chain alcohols, such as methanol and ethanol. According to the Renewable Energy Directive guidelines (RED II 2018/2001/EU) the contribution of advanced biofuels, which do not include edible oils, towards the overall EU target, is at 1% in 2025 and at least 3.5% in 2030. Bioprocesses that valorize non-edible oils for the production of second-generation biodiesel could play a critical role in achieving this goal. Immobilized lipases, as well as other enzyme classes, such as cutinases and acyltransferases, are utilized as biocatalysts for this process. For the sustainability of the process, renewable materials can be used as immobilization matrices, or even enzymes anchored on the cells as whole-cell biocatalysts. Membrane reactors can also be employed to facilitate the enzymatic transesterification by conducting a continuous enzymatic reaction and simultaneously separate the products in a single operation. The advances on the aforementioned fast-pacing fields are presented in this work.
The obvious effects of global climate change and the ongoing petroleum depletion have intensified research efforts towards the development of new technologies for biofuel production. The 2030 objectives outlined in the Greek National Energy and Climate Plan (NECP) involve achieving a minimum 35% share of Renewable Energy Sources (RES) in overall energy consumption, attaining a RES share of 61%–64% in electricity consumption, and reaching a 19% RES share in the transport sector. Furthermore, within the total RES share in transport, there is a specific target of 8.2% for advanced biofuels (inclusive of multipliers as per RED 2018/2001) by 2030. Meeting these ambitious goals necessitates a more than threefold increase in the share of RES used in the transport sector in Greece. Notably, the majority of this increment must originate from technologies not presently deployed on a significant scale, including advanced biofuels and biogas (Paris et al., 2021). Until recently, the main contributors to fuel energy were coal, oil, and natural gas (Mandari and Devarai, 2022). However, fuels, such as bioethanol (Kim and Dale, 2004; Balat et al., 2008; Alvira et al., 2010; Sarkar et al., 2012), biogas (Holm-Nielsen et al., 2009; Weiland, 2010), biohydrogen (Levin et al., 2004; Kapdan and Kargi, 2006), and biodiesel (Ng et al., 2022), are enriching the arsenal. They are sustainable, since they are obtained from renewable resources, and their ecological footprint can be smaller.
Biodiesel is defined as a collection of fatty acid alkyl esters (FAAEs) (Teixeira et al., 2017). The fatty acids derive from the triglycerides (TAGs) of vegetable and animal fats and oils (Shahedi et al., 2019), while the alkyl group of the ester is usually a methyl or an ethyl group. Thus, biodiesel mainly consists of fatty acid methyl esters (FAMEs), fatty acid ethyl esters (FAEEs) or a mixture of them. In the selection of non-edible oils to produce second-generation biodiesel, various criteria are considered to ensure the efficiency and sustainability of the biodiesel production process. The choice of non-edible oils is driven by the need to avoid competition with food production and to utilize feedstocks that may be abundant, economically viable, and possess favorable characteristics for biodiesel synthesis (Luque and Melero, 2012). The key criteria employed in the selection process focus on feedstock availability, cultivation requirements, biodiesel yield and quality (Bart et al., 2010). Biodiesel has attracted significant attention since it can be used in combustion engines that are already in the market without significant modifications. Moreover, it possesses important advantages, such as biodegradability, low toxicity (due to low sulfur and aromatic content), and high oxygen concentration (which secures greater combustion efficiency of the engine), in comparison to petroleum-derived diesel (Parandi et al., 2022). In addition, the cetane number, i.e., another indicator of diesel quality, is larger in biodiesel (Giakoumis and Sarakatsanis, 2018). These characteristics of biodiesel leads to improvement of engine lubrication and its shelf-life. Since biodiesel has similar physicochemical properties to the petroleum-derived diesel (Harabi et al., 2019), it can be either blended with it or directly used.
Based on the feedstock used, biodiesel is categorized as first, second, third and fourth generation biodiesel (Aro, 2016), when produced from edible oils, non-edible oils (like waste cooking oils and fats), algal biomass, and bio-based oils (like engineered algal biomass), respectively (Vignesh et al., 2021). Second-generation biodiesel, which is the specific focus of this work, is produced from low quality oils, mainly industrial by-products, such as acid oils (Degfie et al., 2019) and urban waste oils, like cooking oils (Marchetti and Errazu, 2010). It should be mentioned that biodiesel should meet specific standards according to EN 14214:2003 to be marketable, such as viscosity of 3.5–5.0 mm2/s at 40°C, 96.5% wt minimum ester content, maximum acidity index 0.50 mg KOH/g, 0.8%, 0.2%, and 0.2% wt maximum monoacylglycerol, diacylglycerol, triacylglycerol contents, respectively, and 0.25% wt maximum glycerol value. The production of second-generation biodiesel from non-edible oils holds significant promise for enhancing environmental sustainability and reducing greenhouse gas emissions compared to first-generation biofuels. Non-edible oils, sourced from plants not intended for human consumption, provide a more sustainable feedstock, minimizing the potential conflict with food production. The cultivation of these crops often requires less intensive agricultural practices, leading to lower environmental impact. Additionally, second-generation biodiesel production predominantly utilizes waste materials, such as crop residues or dedicated energy crops, mitigating the competition for arable land (Alalwan et al., 2019). Furthermore, the transesterification process involved in second-generation biodiesel production, particularly with enzymes like lipases or acyltransferases, enhances efficiency and selectivity, resulting in a higher quality and more environmentally friendly fuel. Overall, the shift towards second-generation biodiesel contributes to a more ecologically sustainable and climate-friendly alternative to first-generation biofuels, aligning with the global pursuit of cleaner and more sustainable energy sources (Naik et al., 2010).
The conventional production of biodiesel employs direct use and blending, thermal cracking (pyrolysis), micro-emulsion, or transesterification of the feedstock (Esmi et al., 2021). Among these methods, transesterification is the most common, as it utilizes a wide variety of feedstocks, leads to higher conversions and improves the fuel properties (e.g., viscosity reduction); however, it is less cost-effective. In addition, transesterification is a reversible reaction, during which the alkoxy ester group is replaced by an alcohol, and vice versa (Talukder et al., 2010). The catalysts used in the transesterification reactions are either chemical (acidic or alkaline) (Thaiyasuit et al., 2012) or enzymatic (Hama and Kondo, 2013). The conventional chemical method, using alkaline homogeneous catalyst, offers high conversion rates and short reaction times. Nevertheless, it also comes with some drawbacks in the aspect of energy consumption and environmental concerns, such as difficulty in glycerol recovery, production of undesirable wastewater, and low recovery rate of the biocatalyst (Ma et al., 2018). The alcohol concentration should also be in excess to drive the transesterification reaction towards the desired products, e.g., FAME (Brahma et al., 2022). Moreover, vigorous stirring is required due the immiscibility of fats and oils with methanol, and when there is high content of free fatty acids (FFA) in the feedstock, soap is formed with the alkaline catalyst, hurdling downstream processing; thus, increasing the cost (Kumar et al., 2018). Similarly, the acidic catalysts (Vasić et al., 2020) yield low reaction conversions, the reaction time is longer, they only work under high temperatures (> 100°C), while they still require alcohol excess (Monteiro et al., 2021; Barbosa et al., 2022).
In comparison to the chemical methods, the enzymatic production of biodiesel is more environmentally friendly as the reaction takes place under mild conditions; thus, decreasing simultaneously the energy cost of the process (Cerioni Spiropulos Gonçalves et al., 2020). In addition, the ease of product recovery (Roume et al., 2016) and waste treatment (He et al., 2022) has established the biocatalytic biodiesel production a green approach in the fuel industry. Especially, when it comes to the utilization of feedstocks, such as, acid oils, enzymatic biodiesel production appears to be the most promising method, since the biocatalyst is not negatively affected by the high FFA content and the soap formation is avoided (Marchetti et al., 2011). All these points contribute to lowering the cost of enzymatic biodiesel production as it has been reported by Budžaki et al. (2018), who presented an overall cost analysis of biodiesel production by enzymatic transesterification from sunflower oil with methanol using immobilized Thermomyces lanuginosus lipase (TLL).
Lipases (triacyloglycerol hydrolases, EC 3.1.1.3) are the workhorse of biocatalysis for biodiesel production. Their application was reported for the first time in 1903 (Dakin, 1903), and they have gained special industrial attention since the 1980s, due to their high stability, biodegradability, efficiency and catalytic activity under a wide range of pH and temperature (Quayson et al., 2020a). They are a versatile group of enzymes that are broadly expressed in animals, plants, and microorganisms, like bacteria and fungi (Chandra et al., 2020).
Typically, they hydrolyze oils and fats, but in systems with low water availability, they can catalyze the reverse reactions, such as esterification, transesterification (alkoholysis or acidolysis) and interesterification (de Paula et al., 2021). Due to the interfacial activation mechanism (Maidana Serpa et al., 2022; Parandi et al., 2022), lipases exist in two forms, open (active) and closed (inactive). Most lipases possess an α-helixal oligopeptide structure called “lid”, which covers the active site of the lipases, consisting of a highly conserved catalytic triad (Ser, His, Asp/Glu). The lid isolates the hydrophobic active site from the solvent, attaining thus a closed form (inactive enzyme). When the enzyme is exposed to hydrophobic surfaces, such as oil droplets, hydrophobic immobilization supports, etc., attaches to them causing lid movement and active site exposure. Therefore, in contact with hydrophobic surfaces, the enzyme adopts its open form, and thus the enzyme activity increases (Maidana Serpa et al., 2022; Parandi et al., 2022).
Lipases also display high enantio- and/or regio-product selectivity and/or substrate specificity. Depending on their regioselectivity, lipases are grouped into sn-1,3 specific, sn-2 specific and non-specific lipases, as shown in Figure 1 (Abdulmalek et al., 2021). It should be mentioned that a strict sn-1,3 specific lipase cannot offer more than 66% biodiesel yield (Monteiro et al., 2021). To surpass this barrier, mixture of multiple lipases with different specificities, called combi-lipases (Rodriguez and Ayub, 2011), could be used in one-pot reaction, as acyl movement is avoided, intermediate products are eliminated, the reaction time is reduced and the biodiesel yield is enhanced (Abdulmalek et al., 2021; Monteiro et al., 2021). Combi-lipases act synergistically, targeting all TAG esters, increasing this way the biodiesel yield (Toro et al., 2019).
FIGURE 1. Transesterification reactions catalyzed by a sn-1,3 specific, a sn-2 specific and a non-specific lipase.
The main limitations in lipase-based biodiesel production are the high cost of the biocatalyst (Lv et al., 2021) along with the fact that native lipases are not optimized to function under harsh industrial conditions, e.g., high temperature, wide range of pH and presence of organic solvents (Ren et al., 2011; Zhong et al., 2021). To overcome these challenges, extensive studies are conducted on lipase immobilization, proving that immobilized lipases have great industrial potential as they overcome the free-lipases associated limitations and facilitate enzyme recovery (Venkatesagowda et al., 2018). Immobilization can increase the overall efficiency and selectivity of the enzymes by providing a controlled environment for the reaction. This results in improved substrate accessibility and facilitates better interaction between the enzyme and the reactants, leading to higher conversion rates. Upon interaction with interfaces of water and oil, lipases undergo interfacial activation, a phenomenon that involves the transition from their inactive (closed) form to their active (open) one, exposing thus hydrophobic areas of the protein to the surface. Thus, in the presence of hydrophobic materials like some immobilization carriers, lipases are usually hyperactivated when immobilized on hydrophobic carriers (Bastida et al., 1998). Strategies such as careful selection of immobilization methods, engineering of active sites, and the use of hybrid enzyme systems (Wang et al., 2023; Xu et al., 2020; dos Santos et al., 2014) can contribute to improved specificity and overall performance in the biodiesel synthesis process (Tan et al., 2023). Immobilized enzymes can be used for more than one reaction cycle with little loss in activity. Moreover, the immobilized catalyst can be easily separated from the reaction media (Marín-Suárez, et al., 2019). Immobilization processes are generally based on covalent bonding, physical adsorption, entrapment, and encapsulation (Filho et al., 2019), using an extended set of supports, varying from naturally occurring carriers (olive kernel, rice husk, diatomite, etc.) (Yücel, 2011; Meunier and Legge, 2012; Cespugli et al., 2018) to synthetic carriers (nanomaterials, alginate, activated carbon, etc.) (Pinto Brito et al., 2020). Herein, we review selected representative works on the utilization of immobilized lipases to produce biodiesel from low quality oils.
Wang et al. (2017) used MAS1 lipase from marine Streptomyces sp. strain W007 immobilized onto XAD1180 resin to convert waste cooking oil, achieving 95.45% yield in 24 h. The optimal reaction conditions were 30°C, methanol to oil ratio (MOR) 3:1 (with one-step addition of methanol), enzyme loading of 80 U/g substrate, and 200 rpm agitation. This biocatalyst lost 30% of its initial activity after four cycles. When compared with commercial immobilized enzymes, namely, Novozym 435, Lipozyme RM IM and Lipozyme TL IM, it performed significantly better, when all methanol was added in one step in the start of the process.
Teixeira et al. (2017) used two acidic oils of macauba (Acrocomia aculeata) as substrates to produce biodiesel with Lipozyme 435. The first acidic oil had 42.6% acidity and 5,000 ppm water content (WC), while the second oil had 36.9% acidity and 3,484 ppm WC. They reported that 5% w/w enzyme, MOR 2:1 and incubation time at 30°C lead to 91.7% yield in 4 h (Teixeira et al., 2017).
Lipase from Burkholderia cepacia immobilized onto mesoporous silica/iron oxide magnetic coreshell nanoparticles, using canola waste cooking oil, provided 85.2% yield in 25 h (Khoobbakht, et al., 2020). In this case, the optimal reaction conditions were 34°C, 36% biocatalyst, and MOR 3:1. The performance of the immobilized B. cepacia lipase was decreasing already from the third cycle, losing 11% of its initial activity. In another work, lipase from porcine pancreas immobilized on genipin cross-linked chitosan successfully converted waste cooking oil to biodiesel with 92.33% yield in 10 h, performing equally efficiently for six cycles (Khan et al., 2020). The optimal reaction conditions were, 40°C, 6% v/v WC, catalyst loading 7.5% wt immobilized enzyme, MOR 9:1, and 150 rpm agitation. Aspergillus niger lipase immobilized on mesoporous silica material SBA-15 was very recently investigated as biocatalyst for biodiesel production, using Calophyllum inophyllum oil as a non-edible renewable resource (Arumugam and Ponnusami, 2023). Its application at 30°C, MOR 6:1, 15% v/v WC (pH 7.0) and 500 rpm agitation led to 97% yield in 8 h.
In case of combi-enzymatic systems, Poppe et al. (2018a) suggested one with lipase B from Candida antarctica (CALB), lipase from Thermomyces lanuginosus (TLL), and RML. The substrates used for the transesterification reactions were waste oil and soybean oil. Using soybean oil as substrate, molar ratio of ethanol:soybean oil was 8.09:1 and combi-lipase composition of 22.5% TLL, 50% CALB, and 27.5% RML. Using waste oil as substrate, molar ratio of ethanol:waste oil was 9:1, and the combi-lipase composition was 40% TLL, 35% CALB, and 25% RML. The reactions were performed at 40°C under agitation for both substrates. Yield, was about 50%, with average productivity of 1.94 gethyl esters/gsubstrate/h, for both oils (Poppe et al., 2018a). The group evaluated the same system but after ultrasound-assisting the reactions (Poppe et al., 2018b). The reactions were carried out at 40°C for 18 h, with a 6:1 ethanol:oil molar ratio and 15% biocatalyst (by oil mass). The highest yield achieved was 90%, using soybean oil, and 70% when using the waste oil. Concerning the effect of the lipases in the reactions with waste oil, the greatest yield was obtained using pure CALB as biocatalyst, whereas for soybean oil, the highest yield was obtained with a combination 50% CALB and 50% RML.
Binhayeeding et al. (2020a), produced biodiesel (96.5% yield) from waste cooking oil in 24 h, by using Candida rugosa and Rhizomucor miehei lipase (CRL and RML, respectively) immobilized on poly (3-hydroxybutyrate) beads. The optimal reaction conditions were 45°C, 5% w/w WC, 1% of combi-lipase (ratio 1:1), MOR 6:1 and 250 rpm agitation. This combi-lipase system could be reused in six reaction cycles without losing activity (Binhayeeding et al., 2020b). The same combi-lipase system was used for biodiesel synthesis from by-products of chicken industry. Fats and skin of chicken could be converted into biodiesel (97.1% yield) in 12 h, by a mixture of CRL (1.5% wt) and RML (1% wt), immobilized on polyhydroxybutyrate (PHB) beads (Binhayeeding et al., 2020a). Here, the best conversion conditions were 40°C, 2.5% wt total enzyme loading, 5% w/w WC, MOR 6:1, and agitation 200 rpm. The combi-lipases could retain 50% of its initial activity for seven reaction cycles.
As stated above, lipases have great potential in industrial and biotechnological processes, like detergents (de Oliveira et al., 2020), flavour enchanters (Kendirci et al., 2020), oleochemicals (Abdelmoez et al., 2013), and pharmaceuticals (Contesini et al., 2020) manufacturing. However, the major drawback of the lipase-based processes is the high cost of the biocatalyst. Development of recovery and reuse technologies, mainly through their physical or chemical immobilization in suitable carriers, is mandatory to alleviate this downside. Apart from recovery and reuse, immobilization also facilitates the stabilization of enzymes under conditions that would not be tolerated by their free counterpart (Bilal et al., 2021). Innumerable materials categorized in organic, inorganic, hybrid, physical or artificial polymers and nanostructured matrices are under evaluation as potent immobilization materials (Pavlidis et al., 2014; Ismael and Baek, 2020). Various renewable materials are employed as immobilization matrices for enzymes, providing a supportive environment for their catalytic activity. Some common types include cellulose-based material, agarose (Arana-Peña et al., 2020), alginate, chitosan (de Oliveira et al., 2017), silica-based materials, polymeric materials, nanoparticles (de Oliveira et al., 2020) and magnetic nanoparticles (López et al., 2014; Gireli and Chiappini, 2023). Considerations such as biocompatibility, pore size, chemical stability, ease of preparation, mechanical strength, and recyclability (Mohidem et al., 2023), all contribute to the successful application of immobilized enzymes in various industrial processes (Mokhtar et al., 2020). This paragraph outlines indicative novel, eco-friendly, and low-cost materials as carrier matrices for lipase immobilization.
One of the major agricultural wastes is the rice husk produced during the milling of rice (Oryza sativa). According to Food and Agricultural Organization, the forecasted global production of rice in 2022/2023 would be 519.5 million metric tons, and for every 1 kg rice produced, approximately 0.28 kg rice husk is generated. Several groups have investigated its application as a matrix for enzyme immobilization. Costa-Silva et al. (2016) tested the production of biodiesel using a lipase from a fungal plant pathogen, named Cercospora kikuchii. The lipase was immobilized by covalent binding on rice husk, resulting in a loading of 5.5 mgprotein/gsupport (75%–80% immobilization efficiency). The residual lipase activity of the immobilizate was 75%, while in its free form was 12%, indicating a significant stabilization effect of the immobilization process to the enzyme. As for the reusability of the immobilized lipase, after five cycles of use the immobilizate maintained 87.5% of its initial activity. This preparation was used for the production of biodiesel from coconut oil, transesterified with anhydrous ethanol in a molar ratio of 1:12, using tert-butanol as a solvent. The reaction was performed at 50°C for 120 h under 150 rpm agitation. Results showed 97.1% FAEE yield after 72 h, meeting the requirements of ASTM D6751, and the potential to be used as alternative biofuel (Costa-Silva et al., 2016). In another work, Bonet-Ragel and coworkers (2018) investigated the application of rice husk ash in the immobilization of a recombinant Rhizopus oryzae lipase (rROL). The researchers compared the physical adsorption of the lipase on rice husk ash (a waste product of the use of rice husk for power generation via gasification) to a commercial hydrophobic support (RelOD). However, in this case, the lipolytic activity was found 50% lower in rice husk ash than in RelOD (Bonet-Ragel et al., 2018), showcasing that the effect of immobilization matrix on the enzyme should be studied case by case. Cespugli et al. (2018) also suggested high immobilization yields of lipase B from C. antarctica and two other commercial asparaginases. Rice husk was first oxidized and functionalized with di-amino spacer for covalent immobilization. After 48 h, the immobilization of CALB on the oxidized rice husk reached 72% compared to 95% immobilization on a commercial methacrylic resin after 24 h. The hydrolytic activity of CALB on the functionalized rice husk was 316 U/g. The immobilized enzyme was used in a solvent free poly-condensation reaction.
Another significant agricultural bio-waste is the olive pomace, a side-stream of the olive oil industry. The TLL was covalently immobilized onto olive pomace, leading to a maximum loading of TLL of 18.67 mgprotein/gsupport (Yücel, 2011). Using p-nitrophenyl palmitate (pNPP) as substrate, the specific hydrolytic activity of the immobilized enzyme reached 10.31 U/mgprotein and was retained for at least ten batch reactions. When olive pomace oil was used as a substrate for biodiesel production, and with a molar ratio of MOR 6:1, biodiesel yield was 93% at 25°C, 125 rpm agitation, in a 24 h reaction, almost achieving the required product purity (Yücel, 2011).
De Castro et al. (2022) suggested the immobilization through physical adsorption of the Botryosphaeria ribis EC-01 lipase on a low-cost and eco-friendly film, composed by cassava starch, polyvinyl alcohol, and sericin blend (CS–PVA–SS). Similarly, using a pNPP hydrolytic assay, they determined a 98.7% activity retention of the immobilized lipase. Their biocatalyst led to 95% esterification of oleic acid to ethyl oleate at 49°C in n-heptane, molar ratio ethanol:oleic acid of 3:1, 1.25 g lipase film after 30 h with 150 rpm agitation. However, the immobilization only slightly protected the protein, as after seven cycles of use the yield halved (de Castro et al., 2022).
Linsha et al. (2016), using pollen grains of Hibiscus rosa-sinensis to construct hierarchically porous alumino-siloxane aerogel, investigated the biocatalytic conversion of oleic acid to methyl oleate by immobilized steapsin lipase. The rare structural architecture was developed through a bio-templating method. The highest immobilization efficiency (∼95%) was obtained after the carrier was functionalized with amino propyl groups. This is the case with most biological materials, as specific functional groups are required for covalent immobilization, and their abundance is directly correlated with the maximum load. Concerning the potential application in biodiesel synthesis, methyl oleate yield reached 59% in a reaction that was held at 40°C, in n-hexane, with a molar ratio of alcohol:oleic acid was 6:1 (Linsha et al., 2016).
Tan et al. (2006) introduced the production of biodiesel using an immobilized Candida sp. lipase on a cheap cotton membrane at high WC. The biocatalyst performed at least six cycles at a WC of 15%–20%, yielding high conversion rates, up to 90%. The half-life of the immobilized lipase was more than 200 h. This immobilizate could be efficiently used for biodiesel production as after 30 h at 40°C, WC 15% (w/w), a MOR of 3:1, the conversion was up to 98% (Tan et al., 2006).
Khosla et al. (2017) suggested the immobilization of Pseudomonas sp. ISTPL3 lipase on biochar. Biochar is carbonized biomass produced from the pyrolysis of waste biomass, which due to the presence of surface functional groups, porosity, and moderate surface area, is an appealing material for enzyme immobilization (Pandey et al., 2020). The ISTPL3 lipase was immobilized through physical adsorption and covalent binding after biochar activation with phosphoric acid. Immobilization yield and efficiency were 83.04% and 62.86%, respectively, with 21.4% leaching in the first case (non-covalent binding) (Khosla et al., 2017). Reactions were performed with the lipids from Serratia ISTD04 with MOR 6:1, for 3 h at 300 rpm. The immobilized lipase gave the highest yield of FAMEs (92.23%) followed by non-immobilized lipase (87.81%), while the alkaline transesterification with NaOH yielded the lower amount of FAMEs (81.12%). The immobilized enzyme could be used for three subsequent biodiesel reaction cycles, retaining 75% of its initial activity (Khosla et al., 2017).
Besides lipases, cutinases appear very promising for biodiesel production (Serralha et al., 1998). Cutinases (EC 3.1.1.74) belong to the class of serine esterases, and to the superfamily of α/β hydrolases. They catalyze the hydrolysis, esterification, and transesterification of short and long chain esters, thus being very useful in the industry of detergents and biodiesel (Castro-Ochoa et al., 2012). The substrate of cutinases is a structural polymer lipid called cutin, which covers the plant epidermis and has a protective role. Cutin has a waxy texture and is made of long-chain fatty acids like palmitic acid (16:0) and oleic acid (18:1) joined by ester bonding, forming a steady three-dimensional structure (Bermúdez-García et al., 2017). Cutinases compete with lipases also for the enantioselective synthesis of chemicals and pharmaceuticals, as stated by Su et al. (2020). Lipases have been proven efficient for this task, given the resemblance of their active sites to tunnels, facilitating distinct orientation and binding of enantiomers. Unlike most lipases, cutinases have catalytic triads exposed to solvents, providing potential benefits in reactions by improving substrate accessibility and promoting product diffusion from active site. This characteristic might also enable catalytic reactions with sterically hindered substrates that struggle to reach confined active site clefts; thus, offering great potential in biodiesel industry.
Badenes et al. (2010) worked with the cutinase from the phytopathogenic fungus Fusarium solani pisi using TAGs as substrate. They reported as optimal conditions the 30°C, 600 rpm, 24 h reaction time and MOR of 3:1. In their case, methanolysis gave 75% conversion after 24 h. They could also show that at the same condition, the conversion could be increased, achieving 90% and 80% conversion, using 1-butanol or ethanol as alkyl donor, respectively. Bermúdez-García et al. (2017) observed similar behavior of a thermo-alkaline cutinase from Aspergillus nidulans when used to synthesize FAMEs from sesame oil. After optimizing the conditions (MOR 6:1, 2% w/v of lyophilized cutinase, 7% v/v WC, incubation at 60°C for 72 h at 250 rpm), and even adding methanol stepwise (every 24 h), they could only detect 2% conversion (Bermúdez-García et al., 2017).
The research team of Badenes and coworkers performed another work with a variant of F. solani pisi cutinase (mutant T179C), using a membrane reactor to produce biodiesel in organic media (Badenes et al., 2011a). They managed to produce up to 500 gproduct/day/genzyme from TAGs. After a 24 h reaction, with a 1.6 ratio of alcohol to fatty acid chains and enzyme concentration varying from 0.5 mg/L to 1 mg/mL they determined 90% conversion of TAGs to alkyl esters, using methanol, ethanol, and butanol (Badenes et al., 2011a). The same enzyme was also used in a reversed micellar system for alkyl esters production using acid oil (Badenes et al., 2011b). The optimum reaction conditions were 30°C, 600 rpm stirring and molar ratio of alcohol to fatty acids of 1.6. After 24 h reaction time, the methyl ester conversion achieved was 64%–78%. They observed a 45% loss of cutinase activity when incubated in the micellar system for 3 h. In addition, there was a 90% activity loss in the presence of methanol within 10 min of incubation. In contrast, an improvement of cutinase performance was achieved when incubated with ethanol or butanol, indicating that these alcohols act protectively. Mutant T179C displayed high stability in the presence of methanol, with an activity loss of only 16% (Badenes et al., 2011b).
Acyltransferases also emerged as promising candidates for biodiesel production. For instance, Rodrigues et al. (2016) investigated the biocatalytic production of biodiesel using a lipase/acyltransferase from Candida parapsilosis (CpLIP2). This biocatalyst preferably catalyses alcoholysis over hydrolysis when in aqueous or in biphasic (aqueous/organic) media. In this work, CpLIP2 was immobilized with physical adsorption and subsequent cross-linked with glutaraldehyde (GA) on two synthetic resins, polypropylene (Accurel MP 1000) and divinyl-benzene crosslinked methacrylate polymer (Lewatit VP OC 1600). The immobilization yield of CpLIP2 on Lewatit VP OC 1600 and Accurel MP 1000 was 77% and 80%, respectively. The substrate used for biodiesel production was jatropha oil in a lipid/aqueous system. The oil acidity was 3.7%. Transesterification reactions were held at 30°C, under agitation, using 10% w/w of immobilized enzyme in relation to oil. The molar ratio of methanol:TAG was 6:1. After 8 h reaction time FAME yield reached 80.5%, for CpLIP2 immobilized on Accurel MP 1000% and 93.8% for the enzyme immobilized on Lewatit VP OC 1600 (Rodrigues et al., 2016).
In another work, Mandal et al. (2020) induced the expression of acyltransferases in Graesiella emersonii NC-M1 and Chlorophyta sp. NC-M5 by the addition of phytohormones under nitrogen-limited (NL) conditions. More specifically, they used indole acetic acid and kinetin to enhance biomass and lipid production; nevertheless, this had also an effect on the expression of GPAT and DGAT (glycerol-3-phosphate acyltransferase and diacylglycerol acyltransferase) under NL conditions. The whole cells were used as biocatalysts for biodiesel production. The FAMEs profile of G. emersonii NC-M1 grown under NL and in the presence of phytohormones showed a 44.8% decline in saturated fatty acid (SFAs) content, whereas 179% increase in monounsaturated fatty acid (MUFAs), in comparison to the standard conditions. The FAMEs profile of Chlorophyta sp. NC-M5 showed 96.5% polyunsaturated fatty acid (PUFAs) contents respectively, while 52.2% drop in SFAs and MUFAs under the same conditions. Mandal and co-workers suggested that these acyltransferases could be potentially used for biodiesel production; however, until now, no follow-up work has been published.
Immobilized lipases and whole-cell lipases are the most studied biocatalysts for biodiesel production. However, when whole cells are employed, the indirect enzyme immobilization within or on the cells, bypasses the isolation, purification, and immobilization steps; thus, simplifying the time-consuming and material-intensive upstream processing. Moreover, whole-cell biocatalysis allows significantly better product recovery rates, simplifying also downstream processing, and further decreasing environmental and economic costs (de Carvalho, 2017; Lin and Tao, 2017). Two types of whole-cell catalysts are used for biodiesel production, i.e., whole cells producing cell-bound or intracellular lipases encoded by either native or heterologous genes (Hwang et al., 2014; Borrelli and Trono, 2015; Chandra et al., 2020) and recombinant cells displaying lipases on their surface (Smith et al., 2015; Tanaka and Kondo, 2015; Han et al., 2018; Kuroda and Ueda, 2022b; Lotti et al., 2018; Ye et al., 2021; Kuroda and Ueda, 2022a; Teymennet-Ramirez et al., 2022).
The most commonly used native lipase producers belong to the fungal species Mucor, Rhizopus (e.g., R. oryzae, R. chinensis, R. miehei), Geotrichum, Rhizomucor, Aspergillus (e.g., A. oryzae), Penicillium, Thermomyces, Fusarium. However, R. oryzae lipase (ROL) is in the spotlight, due to its remarkable 1,3-regiospecificity that yields 2-monoacylglycerol instead of glycerol, a compound that lubricates and upgrades biodiesel characteristics (López-Fernández et al., 2020). Both lipase producing R. oryzae cells and purified ROL are employed for biodiesel production usually after immobilization using various materials with biomass support particles (BSPs) being the most commonly used (Table 1). Other native lipase producers belong to the bacterial Pseudomonas, Bacillus sp., and yeast Yarrowia lipolytica, Candida antarctica and Rhodotorula sp. (Table 1). Heterologous lipase encoding genes most commonly are overexpressed in hosts such as Pichia pastoris, Saccharomyces cerevisiae, Aspergillus oryzae, and Escherichia coli (Table 2). In the case of cell-bound or intracellular lipases, low mass transfer rate of high molecular weight substrates from the solvent to the biocatalyst, cellular metabolism, protein synthesis, and also the toxicity of the alcohol used, can be the rate-limiting steps for the whole-cell bioconversion of the substrate (Schrewe et al., 2013; Aarthy et al., 2014; Wachtmeister and Rother, 2016). Permeabilization of the cells using chemical (detergents and solvents) or physical (e.g., air-drying, temperature shock) means is often applied to improve limited substrate transfer across cell walls and membranes (Ni and Chen, 2004; Aguieiras et al., 2015; Lin and Tao, 2017; Sakkow et al., 2019). While permeabilization via chemical or physical treatment of cell membrane is effective in small-scale process, large-scale implementation is problematic. Molecular engineering approaches to enhance mass transfer recently emerged as a much better alternative (Chen, 2007). The co-expression of membrane transporters can be such strategy, as demonstrated for the biocatalytic conversion of C7-C16 n-alkanes by Grant et al. (2014), who improved the specific yields of bioxidation of > C12 alkanes to fatty alcohols and acids by up to 100-fold, when co-expressed in E. coli the alkL gene from Pseudomonas putida, encoding an alkane import protein. Immobilizing the lipase on the cell surface by yeast cell surface display (YCSD) is another molecular engineering strategy that can be employed to overcome mass transfer related limitations (Smith et al., 2015; Tanaka and Kondo, 2015; Han et al., 2018; Kuroda and Ueda, 2022b; Ye et al., 2021; Kuroda and Ueda, 2022a; Teymennet-Ramirez et al., 2022). CSD has been one of the most valuable tools to not only study and comprehend protein functions, but also to bestow yeast cells with novel properties, such as novel catalytic functions, affinity binding to ligands, bioremediation or bio-monitoring properties, library screening purposes, whole-proteome studies, vaccine and antibiotics development, biosensors production, etc. (Shibasaki et al., 2009). With CSD, target peptides or proteins are displayed on the cell surface after fusion with an anchoring protein system, which in yeast typically comprises a cell wall protein (CWP) linked to glycosylphosphatidylinositol (GPI) (Tanaka and Kondo, 2015; Chen, 2017). Apart from the target enzyme and the anchor protein, the linker sequence and the host microbial cell are also structural units playing crucial role in CSD. However, the interplay between these units and lipase conformation and activity are not yet fully understood.
TABLE 1. Microbial strains producing native lipases used as whole-cell biocatalysts for biodiesel production.
TABLE 2. Microbial strains used as whole-cell biocatalysts for biodiesel production after heterologous expression of lipase genes.
“Arming” the yeast cell surface with enzymes, is nevertheless one of the most attractive applications of molecular display technology, given that yeast cells obtain novel potentials as whole-cell biocatalysts and innovative bioprocesses can be conceptualized (Shibasaki et al., 2009; Tabañag et al., 2018; Fan et al., 2020). The maturation of CSD technology occurred at the turn of the second (i.e., structural based biocatalyst engineering by rational design) and third (i.e., biocatalyst engineering by directed evolution) waves of biocatalysis evolution and enabled researchers to exploit its natural ability to link genotype and phenotype as powerful library screening tool for protein engineering (Bornscheuer et al., 2012; Smith et al., 2015). The construction of whole-cell biocatalysts using the YCSD technology has the benefits of combined fine-tuning of gene expression and “natural” enzyme immobilization; thus, generating renewable self-immobilized biocatalysts (Teymennet-Ramírez, 2022). Apart from mass transfer limitations, YCSD can overcome other serious drawbacks of the conventional immobilization techniques, such as structural and functional alterations, low enzyme loading, dissociation, costs of immobilization materials and process, etc. (Ueda and Tanaka, 2000). In addition, CSD enzyme allows whole cells to readily access soluble substrates while retaining the metabolic potential of their intracellular enzyme systems (e.g., extracellular degradation of cellulose and internalization of the major product, glucose, to produce ethanol) expanding the industrial applications of engineered biocatalysts in (Smith et al., 2015). CSD of Pichia pastoris, Yarrowia lipolytica, and Saccharomyces cerevisiae is widely used to construct whole-cell biocatalysts by expressing various heterologous lipase-encoding genes (Table 3). These systems display many advantages associated with safety, simplicity of genetic manipulation and rigidity of the cell-wall structure, standardized bioproduction of target proteins, use of the biocatalyst without purification and immobilization. Compared to S. cerevisiae, P. pastoris can achieve a much higher cell density in fermentation (Dong et al., 2020) and additionally, an indirect CSD method was developed that simply displays various enzymes with an average efficiency ten times higher than that of commonly used S. cerevisiae CSD methods (Li et al., 2019a). However, the exploitation of CSD lipases on yeast cells as whole-cell biocatalysts for biodiesel production is still very limited due to poor operational stability (Fu and Vasudevan, 2010; Liu et al., 2018). One of the main hurdles to increase the use of lipases in biodiesel industry is their low stability and tolerance under high methanol concentrations that are required. Since the stoichiometric ratio for the transesterification reaction requires 3 mol methanol and 1 mol TAGs to yield 3 mol FAMEs and 1 mol glycerol, the optimal methanol to oil ratios to promote the transesterification reaction should be higher than 3:1. A higher molar ratio though, can inactivate the enzyme, especially when the alcohol is insoluble in the reaction mixture (Huang et al., 2012; Lotti et al., 2018). Stepwise addition of methanol during the transesterification to keep its concentration at a relatively low level is generally applied to circumvent this limitation. Nonetheless, apart from the methanol to oil ratio, various other parameters have to be fine-tuned to make the transesterification reaction competitive. These parameters can be related to the overall process design (e.g., solvent quantity, solvent to oil ratio, temperature, biocatalyst concentration, WC to ensure active enzyme conformation), upstream strategies (e.g., type of raw materials used, pre-treatment, plant genetic engineering) and the biocatalyst performance (e.g., robustness, natural alcohol tolerance, protein engineering) (Hasheminejad et al., 2011; Lotti et al., 2018).
TABLE 3. Microbial strains displaying lipases on their cell surface used as whole-cell biocatalysts for biodiesel production.
Even though many obstacles remain to overcome, the elimination of separation and purification steps that are bypassed and the utilization of cheap waste materials for cell cultivation (thus, further reducing process costs), make whole-cell lipases very attractive catalysts for biodiesel production. In addition, both protein and metabolic engineering can facilitate the creation of tailor-made biocatalysts with desirable properties. Novel engineered lipases or even combination of lipases (compi-lipases) displaying different or complementary activities and enhanced methanol tolerance can be employed to optimize production yields, decrease unwanted side-products (unconverted intermediates such as monoglycerols, diacylglycerols, free fatty acids), and also improve biodiesel quality (Ogino and Amoah, 2019). The bioprospecting of novel native lipase producing microbial strains can also contribute to expand the spectrum of potential lipolytic enzymes and inspire novel synthetic processes (Escobar-Niño et al., 2014; Sahoo et al., 2017; Hama et al., 2018; Chow et al., 2021). On the other hand, metabolic engineering tools can be employed to design and develop biodiesel-producing microbial cell factories capable, not only of in vitro biotransformation of various common oil feedstocks to biodiesel, but also of de novo biosynthesis of biodiesel from glucose, glycerol or even cellulosic biomass (Yan et al., 2017).
Enzymatic transesterification processes face similar challenges to conventional transesterification processes, namely, mass transfer limitation due to the biphasic reaction mixture, thermodynamic limitations due to the reaction reversibility in the absence of product removal, scale-up and mixing issues, and technical constrains when operating at continuous mode (Athar and Zaidi, 2020). Membrane technology is a process that uses semi-permeable membranes to separate different substances in a mixture. Membrane performance is usually driven by selectivity and permeability parameters, while membrane acts as a physical barrier in liquid-liquid and solid-liquid systems (Hajilary et al., 2019). Membrane integration in enzymatic transesterification (Figure 2) may be a promising alternative to conventional processes, since they may overcome the aforementioned challenges given that they offer an unconventional immobilization option; they achieve simultaneous transesterification and biodiesel separation; and they facilitate downstream processing of enzymatically produced biodiesel and biocatalyst’s reuse.
FIGURE 2. Schematic representation of a membrane bioreactor employed for biodiesel production through enzymatic transesterification.
Immobilization of enzymes on membranes offers several advantages over other supports, such as high surface area, easy separation, low mass transfer resistance and good mechanical strength (Kujawa et al., 2021). Furthermore, the immobilization and/or encapsulation of free enzymes using novel or modified membrane modules could increase their stability and reduce down-stream separation/purification costs compared to conventional systems, making the reuse of these catalysts easier, simply by removing the enzyme-loaded membrane module from the reaction site. The enzyme retention can be achieved by physical or chemical interactions into or onto the membrane (Cen et al., 2019), including covalent bonding, encapsulation, entrapment and enzyme adsorption.
Li et al. (2019b) developed a hybrid hollow fiber membrane by modifying a polyacrilonitrile hollow fiber membrane, using the phase inversion method. Subsequently, CALB was successfully immobilized onto the modified membrane and was used for the transesterification of soybean oil. The biodiesel production yield was found to be 78.5%, which was comparable to the yield of commercial lipase Novozyme 435, under the same experimental conditions. In addition, the immobilized enzyme retained over 88% of its activity after 20 transesterification cycles, and the biodiesel yield decreased by only 11% at the 20th reaction time. Kuo et al. (2013) studied the biodiesel production from soybean oil using an immobilized lipase from C. rugosa on a pre-activated polyvinylidene fluoride (PVDF) membrane. The membrane-immobilized lipase yielded a 95.3% biodiesel production, which remained for more than five cycles. Sunflower seed oil was also successfully converted to biodiesel via Mucor miehei lipase (Handayani et al., 2016) immobilized, by physical adsorption, onto a synthesized aminated PES membrane (PES-NH2), without decrease of catalytic activity. In addition, it was found that the modified membrane attained higher enzyme loading compared to the commercial unmodified one, while the immobilized lipase showed approximately 10% higher transesterification activity than the free lipase. Machsun et al. (2010) immobilized a lipase from Pseudomonas fluorescens into an asymmetric polyethersulfone membrane of 300 kDa, reaching 80% triolein conversion after 19 min of reaction, while no enzyme activity decay was observed after 12 operational days. Notably, the enzyme activity increased after membrane-immobilization (3-fold) compared to the free lipase under the same reaction conditions.
Apart from polymeric membranes, composite, as well as ceramic membranes have been used for the immobilization of lipases. Jafarian et al. (2020) incorporated graphene oxide nanosheets (GON) into polyethersulfone (PES) membrane for the immobilization of CRL, and used enzymatic hybrid PES-GON membrane, where the flow is perpendicular to the membrane surface, for the hydrolysis of p-nitrophenyl palmitate (p-NPP) to p-nitrophenol (p-NP). Membrane catalytic activity and p-NP release were subsequently evaluated. The results indicated that the composite enzymatic membrane could be used for biodiesel production, preventing enzyme wash-out, while p-NP rejection rate was approx. 30%. A lipase from C. rugosa was immobilized on an alumina hollow fiber membrane surface by covalent binding, as described by Ranieri et al. (2016). The specific activities of both free and immobilized enzymes were evaluated during triglycerides hydrolysis in a continuous stirred tank reactor, indicating that the immobilized lipase maintains an observed specific activity of approximately 93% compared to the free enzyme. The enzymatic membrane was also tested for olive oil hydrolysis, and after six reaction cycles, no significant decrease of the immobilized enzyme specific activity was observed. Therefore, enzyme immobilization on membranes is a promising technique for biodiesel production from vegetable oils by transesterification, since it can improve the stability, reusability and activity of lipases (Zhang et al., 2012). However, current studies present results from laboratory-scale tests and challenges still remain regarding the overall cost and the robustness of the developed materials under real large-scale operating conditions.
Membrane reactors for biodiesel production combine transesterification process and product/s separation in one process step. Figure 3 illustrates the concept of membrane reactors in biodiesel production. The reaction takes place at the retentate side of membrane module. The resulting products (FAME and glycerol) and the catalyst dissolve in the methanol phase selectively permeates through the membrane into the permeate side, while unreacted oil retained due to its larger molecular size. The continuous permeation of biodiesel and glycerol, from the reactive system, promotes transesterification reaction in the direction of product formation and thus catalytic efficiency is enhanced (Ding et al., 2020). Membrane reactor technologies play a crucial role in enhancing industrial processes by selectively removing or transporting reactants and products, thereby improving reaction efficiency, selectivity, and overall performance (Basile, 2013). In the treatment of industrial wastewater, membrane reactors can be employed for processes such as the removal of specific ions or the degradation of pollutants through catalytic reactions, electrochemical applications, such as electrolysis and enhancing ammonia production by selectively removing hydrogen, demonstrating the broad impact of membrane reactors across various industrial sectors (Sirkar et al., 1999; Stephenson et al., 2000; Basile, 2013). Many studies assess different membrane reactor configurations for biodiesel production (Atadashi et al., 2011; Bhatia et al., 2021; Emmanouilidou and Kokkinos, 2022) employing chemically catalyzed transesterification; among them, few examine the integration of membrane separation with enzymatic transesterification.
FIGURE 3. Schematic representation of a single membrane module combining reaction and separation for biodiesel production through enzymatic transesterification.
Badenes et al. (2011a) studied the transesterification of triolein employing a recombinant cutinase of Fusarium solanipisi microencapsulated in reverse micelles. The enzymatic transesterification process was combined with an ultrafiltration ceramic membrane with approx. 15 kDa pore size to retain the enzyme while permitting permeation of transesterification products. The enzymatic membrane reactor operated both in batch and continuous operation mode, achieving almost complete retention of the enzyme and its partial adsorption on the membrane surface. The enzymatic membrane reactor operated continuously for more than 28 days, resulting in high productivity (>500 g/day/genzyme) and satisfactory enzyme stability. Ko et al. (2012) developed a membrane reactor system, for the transesterification of soybean oil employing CALB, with continuous removal of glycerol and methanol through a regenerated cellulose membrane module (10 kDa molecular weight cut-off, MWCO). Methanol and glycerol were withdrawn, while unreacted oil and FAME were recirculated back to the bioreactor tank. Authors assessed both batch and continuous operation and concluded that in batch experiments the conversion reached 82.4%, whereas the conversion rate slightly decreased, after each MeOH addition step. On the contrary, in continuous mode operation, the removal of glycerol from the reaction improved the conversion rate and the biodiesel conversion found to be more than 99%. To study the effect of membrane pore size on the final conversion of biodiesel, as well as on the conversion rate, they conducted additional experiments using 25 and 50 kDa MWCO membrane. By increasing the pore size they increased conversion rate, achieving approx. 75, 50% and 40% FAME conversion in 5 h reaction for 50, 25, and 10 kDa membranes, respectively; however, the final conversion of biodiesel decreased, from 99% to 78.5%. This was attributed to the faster permeation of methanol at 50 and 25 kDa MWCO membranes, respectively, resulting in enzyme deactivation. Furthermore, when using the membrane with the larger pore size (50 kDa), oil and FAME were observed in permeate, denoting that the membranes separation was insufficient. Aghababaie et al. (2019) developed a transesterification process with a two-phase enzymatic membrane reactor to produce biodiesel from raw oil of Eruca sativa. They used a hydrophilic membrane (30 and 100 kDa) made of polyacrylonitrile (PAN) to increase the biodiesel yield by separating the organic and the polar reaction phases. The enzyme (C. rugosa lipase) was added to the organic phase (oil and FAME), which was separated by the polar phase (MeOH and glycerol) through the membrane. The two phases were recirculated in counterflow mode and the reaction took place on the membrane’s surface. Therefore, the enzyme contact with methanol and glycerol was minimum and their negative effect on the enzyme activity significantly decreased. The biodiesel yield of the two-phase bioreactor system with PAN 100 membrane and a 40 mL/min flow rate of the organic phase reached almost 100%. Moreover, glycerol was successfully removed from the organic phase while the enzyme was entrapped in the organic phase.
Even though enzymatic transesterification has great potential for biodiesel production, the downstream processing has many challenges to overcome, which are similar to the conventional transesterification processes, such as: 1) the large amount of unreacted alcohol, mainly methanol, that is used to achieve high transesterification efficiency, 2) the recovery of the lipases (especially when used as free enzymes) to be re-used for further transesterification cycles, and 3) the separation of glycerol, which is the main by-product of the transesterification process. There are several separation/purification technologies that are currently used for biodiesel post-treatment, such as filtration, decantation, wet or dry washing, distillation, adsorption and others (Bhatia et al., 2021). However, there are problems associated with these conventional methods, including large amount of water, excessive heat demand and high energy consumption that have resulted in the development of competitive novel technologies, such as membrane separation. The interest on membrane separation in enzymatically produced biodiesel arises from the fact that these methods require lower energy consumption and time scales, and is also safe, easy and environmental-friendly (Ding et al., 2020).
Sokač et al. (2020) recently evaluated the purification of biodiesel produced by lipase catalyzed transesterification from edible sunflower oil carried out by decantation, followed by ultrafiltration membrane technology. Four different ultrafiltration membranes, polypropylene, polyethersulfone, polyacrylonitrile and regenerated cellulose, were tested for the elimination of the remaining glycerol, after removing the bulk of the glycerol through centrifugation. No additional water was added in the enzymatically produced biodiesel prior to ultrafiltration at 4 bar trans-membrane pressure. Their experiments were conducted at room temperature (25°C) and the authors found that polyacrylonitrile membrane was the most efficient for glycerol removal, compared to the other three membranes tested. More specifically, polyacrylonitrile membrane successfully removed approx. 91.5% of free glycerol content, while polyethersulfone, and regenerated cellulose membranes exhibited a glycerol removal efficiency of approx. 83.8% and 83.2%, respectively. In addition, the membrane fouling was investigated, using the Hermia’s model, and the results showed that in discontinuous filtration mode, intermediate blocking appeared after the first filtration cycle, while complete cake layer appeared at the following filtration cycles. Gojun et al. (2021) investigated the impact of polyethersulfone membrane at a cross-flow separation process of glycerol, which was produced as a by-product of TLL catalyzed transesterification of sunflower oil. The membrane used in their system had 10 kDa MWCO and significantly removed glycerol from biodiesel, achieving a glycerol-content below 0.02% (w/w) in the permeate stream.
Romero et al. (2022) recently evaluated the performance of different pore sizes (MWCO) flat sheet polymeric membranes (0.2 μm–90 kDa), in the purification of fatty acid methyl esters (FAME) concerning soap and moisture content, acidity and color. The authors used crude industrial FAME that exceed the maximum amount of glycerol according to EN 14214:2013 (Committee for Standardization, 2003). A cross flow system was used at a constant trans-membrane pressure of 3 bars. It was shown that both hydrophobic and hydrophilic NF membranes were unsatisfactory in terms of permeation of biodiesel, suggesting fouling to be the main cause. Unsatisfactory results were also achieved with the 0.2 μm MF membrane. However, the industrial FAME was successfully purified using a UF membrane (10 kDa); lower amount of soap content (undetected amount), 13% less moisture and phosphorous reduction of approx. 97% was achieved, while the color of the FAME was also significantly reduced (10 on the Gardner color scale) and meet the requirements established by ASTM. Kumar and Pal (2021) developed an integrated experimental process for the downstream separation and purification of biodiesel, produced by lipase catalyzed transesterification. They combined effectively two membrane-based applications: a solar-driven direct contact membrane distillation (DCMD), followed by a cross-flow membrane process. At the first step of distillation, a separation of the excess ethanol was aimed, so as to be reused at the transesterification process, while at the second step, the removal of glycerol was evaluated using a flat sheet polyethersulfone membrane employing a typical cross-flow membrane module. Regarding the novel designed DCMD module system, of the two hydrophobic membranes tested, it was shown that the PTFE/PET (polyethylene terephthalate) was more efficient compared to the PP (polypropylene). More specific, at the optimum experimental conditions, the PTFE/PET membrane resulted in a maximum flux of 41 kg EtOH/m2/24 h, at temperatures of 60°C and 20°C in the feed and distillate stream, respectively. At the same conditions, the PP membrane reached a maximum of 28 kg EtOH/m2/24 h. One remarkable result is that the membrane performance and their characteristics was not affected after the distillation experiments. In addition, concerning cross flow experiments using a PES membrane at an operating transmembrane pressure of 2.5 bars, 77% separation of glycerol (concentration of 0.02% w/w in the permeate) was accomplished after 150 min. The biodiesel at the permeate was within the limits of the ASTM and EN standards.
An alternative use of membrane separation process was investigated by Andrade et al. (2019), focusing on the recovery of liquid enzymes, specifically Eversa Transform and Resinase HT, from the reaction mixture after transesterification. The authors investigated a water diafiltration process for the removal of methanol and glycerol from the reaction mixture, using ultrafiltration membrane to avoid the deactivation of the enzyme. Next, the condensation of the remaining solution (after the diafiltration process) was attempted using tubular ceramic ultrafiltration membranes with MWCO of 15 and 25 kDa. This separation procedure resulted in the reduction of glycerol and methanol content to less than 1% w/w at the Eversa Transform and Resinase HT solution. Additionally, they concluded that both enzyme-rich solutions can be efficiently reused (achieving FAME yields of around 80%–83%), and that the ceramic membranes tested did not exhibit any deterioration concerning their performance behavior. Similarly, Wijaya et al. (2020) investigated the use of a sulfonated polyethersulfone nanofiltration membrane (3 kDa MWCO) for the condensation of an extracellular lipase expressed from A. oryzae. After nanofiltration, the concentrate stream presented an 83% increased lipase activity against the initial (unconcentrated) lipase solution. In addition, the biodiesel production of unrefined palm oil, using the concentrated lipase, resulted in relatively high yield of FAME (around 97%) compared to the FAME yield produced from the corresponding commercially available lipase Callera Trans L (CalT). Therefore, it is obvious that membrane technologies can provide significant advantages in enzymatic transesterification as low-cost and environmental-friendly downstream processes, both for biodiesel purification and for the recovery and reuse of biocatalysts resulting in a more sustainable biodiesel production technology (Kim et al., 2018).
The biocatalytic approaches in biodiesel synthesis have been proven, in many cases, advantageous to the alkaline or acidic synthesis, as the reaction can be performed in low quality, non-edible oils, with high acidity levels, resulting in reduced process cost (Sales et al., 2022). Biocatalytic esterification/transesterification can even take place in the presence of water if, for instance, acyltransferases are employed. However, the methanol-induced enzymatic inhibition is one of the most challenging bottlenecks of the field so far. To overcome that, different bioreactor setups and processing modes, or even longer alcohols, such as ethanol or 1-butanol, have been evaluated. Protein engineering of lipases or other enzymes of interest, like cutinases, which seem to be affected the most, could provide variants that are more stable and do not suffer from methanol inhibition. The combination of these variants with whole-cell systems is expected to provide sustainable processes for the production of second-generation biodiesel.
Concerning the bioreactor setup, membrane reactors membranes may play several roles during biodiesel production and purification. First, membranes may act as a selective barrier for biodiesel impurities, such as soap, catalysts, and other contaminants. Also, the removal of glycerol, which is a by-product of transesterification process, either operating in batch or in continuous mode, leads in higher reaction and conversion rates of the biodiesel produced. UF membranes showed the best results for refining biodiesel, using mostly polymeric materials such as PES, PAN and regenerated cellulose. Another key role that the membranes can achieve is the concentration of liquid lipase mixtures after transesterification for their subsequent reuse, making lipase catalyzed production of biodiesel more economical. It should be noted also that bioreactors are often integrated with automation systems, allowing for the control and optimization of important parameters such as temperature, agitation, and pH. Thus, the application of such systems in biodiesel production is expected to increase significantly in the coming years.
Furthermore, in the last few years, researchers focused on the lipase immobilization on commercial or modified membranes. This procedure shows promising results even after multiple transesterification cycles due to enhanced stability of the immobilized enzyme. Membrane immobilization could be an effective way of enzyme’s activity protection during biodiesel production. This direction of the formulation of biocatalyst could be especially beneficial for the simultaneous transesterification reaction and separation of by-products, as well as for the scale-up of the biodiesel production process. However, in order to enhance the use of immobilized enzymes in industrial processes, more attention is required to the immobilization techniques and operational conditions depending on the specific characteristics of each transesterification process case.
AS: Writing–original draft, Writing–review and editing. AM: Writing–original draft, Writing–review and editing. ET: Writing–original draft, Writing–review and editing, Conceptualization, Supervision. SP: Conceptualization, Supervision, Writing–original draft, Writing–review and editing. IP: Supervision, Writing–original draft, Writing–review and editing, Conceptualization.
The author(s) declare financial support was received for the research, authorship, and/or publication of this article. This research has been co-financed by the European Regional Development Fund of the European Union and Greek national funds through the Operational Program Competitiveness, Entrepreneurship and Innovation, under the call RESEARCH—CREATE—INNOVATE (project code: Τ2ΕDΚ-00573). The Research implemented at the University of Crete was supported by the University of Crete Research Committee funds (Project Code Number 11512).
The authors declare that the research was conducted in the absence of any commercial or financial relationships that could be construed as a potential conflict of interest.
The author(s) declared that they were an editorial board member of Frontiers, at the time of submission. This had no impact on the peer review process and the final decision.
All claims expressed in this article are solely those of the authors and do not necessarily represent those of their affiliated organizations, or those of the publisher, the editors and the reviewers. Any product that may be evaluated in this article, or claim that may be made by its manufacturer, is not guaranteed or endorsed by the publisher.
Aarthy, M., Saravanan, P., Gowthaman, M. K., Rose, C., and Kamini, N. R. (2014). Enzymatic transesterification for production of biodiesel using yeast lipases: an overview. Chem. Eng. Res. Des. 92 (8), 1591–1601. doi:10.1016/j.cherd.2014.04.008
Abdelmoez, W., Mostafa, N. A., and Mustafa, A. (2013). Utilization of oleochemical industry residues as substrates for lipase production for enzymatic sunflower oil hydrolysis. J. Clean. Prod. 59, 290–297. doi:10.1016/j.jclepro.2013.06.032
Abdulmalek, S. A., Li, K., Wang, J., Ghide, M. K., and Yan, Y. (2021). Co-immobilization of Rhizopus oryzae and Candida rugosa lipases onto mMWCNTs@4-arm-PEG-NH2 —a novel magnetic nanotube–polyethylene glycol amine composite—and its applications for biodiesel production. Int. J. Mol. Sci. 22 (21), 11956. doi:10.3390/ijms222111956
Aghababaie, M., Beheshti, M., Razmjou, A., and Bordbar, A. K. (2019). Two phase enzymatic membrane reactor for the production of biodiesel from crude Eruca sativa oil. Renew. Energy 140, 104–110. doi:10.1016/j.renene.2019.03.069
Aguieiras, E. C. G., Cavalcanti-Oliveira, E. D., and Freire, D. M. G. (2015). Current status and new developments of biodiesel production using fungal lipases. Fuel 159, 52–67. doi:10.1016/j.fuel.2015.06.064
Alalwan, H. A., Alminshid, A. H., and Aljaafari, H. A. S. (2019). Promising evolution of biofuel generations. Subject review. Renew. Energy Focus 28, 127–139. doi:10.1016/j.ref.2018.12.006
Alvira, P., Tomas-Pejo, E., Ballesteros, M., and Negro, M. J. (2010). Pretreatment technologies for an efficient bioethanol production process based on enzymatic hydrolysis: a review. Biores. Technol. 101, 4851–4861. doi:10.1016/j.biortech.2009.11.093
Amoah, J., Ho, S.-H., Hama, S., Yoshida, A., Nakanishi, A., Hasunuma, T., et al. (2016). Converting oils high in phospholipids to biodiesel using immobilized Aspergillus oryzae whole-cell biocatalysts expressing Fusarium heterosporum lipase. Biochem. Eng. J. 105, 10–15. doi:10.1016/j.bej.2015.08.007
Amoah, J., Quayson, E., Hama, S., Yoshida, A., Hasunuma, T., Ogino, C., et al. (2017). Simultaneous conversion of free fatty acids and triglycerides to biodiesel by immobilized Aspergillus oryzae expressing Fusarium heterosporum lipase. Biotechnol. J. 12 (3), 1600400. doi:10.1002/biot.201600400
Andrade, T. A., Errico, M., and Christensen, K. V. (2019). Investigation of the use of ceramic membranes in recovering liquid enzymes for castor oil transesterification. Chem. Eng. Trans. 74, 769–774. doi:10.3303/cet1974129
Arana-Peña, S., Rios, N. S., Carballares, D., Mendez-Sanchez, C., Lokha, Y., Gonçalves, L. R. B., et al. (2020). Effects of enzyme loading and immobilization conditions on the catalytic features of lipase from Pseudomonas fluorescens immobilized on octyl-agarose beads. Front. Bioeng. Biotechnol. 8, 36. doi:10.3389/fbioe.2020.00036
Aro, E. M. (2016). From first generation biofuels to advanced solar biofuels. Ambio 45 (1), 24–31. doi:10.1007/s13280-015-0730-0
Arumugam, A., and Ponnusami, V. (2023). Synthesis of SBA-15 from low cost silica precursor obtained from sugarcane leaf ash and its application as a support matrix for lipase in biodiesel production. Renew. Energy 6, 244–250. doi:10.1007/s10971-013-3070-1
Atadashi, I. M., Aroua, M. K., Abdul Aziz, A. R., and Sulaiman, N. M. N. (2011). Membrane biodiesel production and refining technology: a critical review. J. Sol-Gel Sci. Techn. 15, 5051–5062. doi:10.1016/j.rser.2011.07.051
Athar, M., and Zaidi, S. (2020). A review of the feedstocks, catalysts, and intensification techniques for sustainable biodiesel production. J. Env. Chem. Eng. 8 (6), 104523. doi:10.1016/j.jece.2020.104523
Badenes, S. M., Lemos, F., and Cabral, J. M. S. (2010). Assessing the use of cutinase reversed micellar catalytic system for the production of biodiesel from triglycerides. J. Chem. Technol. Biot. 85 (7), 993–998. doi:10.1002/jctb.2393
Badenes, S. M., Lemos, F., and Cabral, J. M. S. (2011a). Performance of a cutinase membrane reactor for the production of biodiesel in organic media. Biotechnol. Bioeng. 108 (6), 1279–1289. doi:10.1002/bit.23054
Badenes, S. M., Lemos, F., and Cabral, J. M. S. (2011b). Stability of cutinase, wild type and mutants, in AOT reversed micellar system-effect of mixture components of alkyl esters production. J. Chem. Technol. Biot. 86 (1), 34–41. doi:10.1002/jctb.2505
Balasubramaniam, B., Sudalaiyadum Perumal, A., Jayaraman, J., Mani, J., and Ramanujam, P. (2012). Comparative analysis for the production of fatty acid alkyl esterase using whole cell biocatalyst and purified enzyme from Rhizopus oryzae on waste cooking oil (sunflower oil). Waste Manage 32 (8), 1539–1547. doi:10.1016/j.wasman.2012.03.011
Balat, M., Balat, H., and Öz, C. (2008). Progress in bioethanol processing. Prog. Energy Combust. 34, 551–573. doi:10.1016/j.pecs.2007.11.001
Ban, K., Hama, S., Nishizuka, K., Kaieda, M., Matsumoto, T., Kondo, A., et al. (2002). Repeated use of whole-cell biocatalysts immobilized within biomass support particles for biodiesel fuel production. J. Mol. Catal. B Enzym. 17 (3–5), 157–165. doi:10.1016/s1381-1177(02)00023-1
Ban, K., Kaieda, M., Matsumoto, T., Kondo, A., and Fukuda, H. (2001). Whole cell biocatalyst for biodiesel fuel production utilizing Rhizopus oryzae cells immobilized within biomass support particles. Biochem. Eng. J. 8, 39–43. doi:10.1016/s1369-703x(00)00133-9
Barbosa, S. L., Rocha, A. C. P., Nelson, D. L., de Freitas, M. S., Mestre, A. A. P. F., Klein, S. I., et al. (2022). Catalytic transformation of triglycerides to biodiesel with SiO2-SO3H and quaternary ammonium salts in toluene or DMSO. Molecules 27 (3), 953. doi:10.3390/molecules27030953
Bart, J. C. J., Palmeri, N., and Cavallaro, S. (2010). in 5-Feedstocks for biodiesel production in Biodiesel, Science and Technology. Editors J. C. J. Bart, N. Palmeri, and S. Cavallaro (China: Woodhead publishing), 130–225. doi:10.1533/9781845697761.130
Basile, A. (2013). Handbook of membrane reactors: reactor types and industrial applications (Germany: Elsevier).
Bastida, A., Sabuquillo, P., Armisen, P., Fernández-Lafuente, R., Huguet, J., and Guisán, J. M. (1998). A single step purification, immobilization, and hyperactivation of lipases via interfacial adsorption on strongly hydrophobic supports. Biotechnol. Bioeng. 58, 486–493. doi:10.1002/(SICI)1097-0290(19980605)58:5<486::AID-BIT4>3.0.CO;2-9
Bermúdez-García, E., Peña-Montes, C., Castro-Rodríguez, J. A., González-Canto, A., Navarro-Ocaña, A., and Farrés, A. (2017). ANCUT2, a Thermo-alkaline cutinase from Aspergillus nidulans and its potential applications. Appl. Biochem. Biotech. 182 (3), 1014–1036. doi:10.1007/s12010-016-2378-z
Bhatia, S. K., Bhatia, R. K., Jeon, J. M., Pugazhendhi, A., Awasthi, M. K., Kumar, D., et al. (2021). An overview on advancements in biobased transesterification methods for biodiesel production: oil resources, extraction, biocatalysts, and process intensification technologies. Fuel 285, 119117. doi:10.1016/j.fuel.2020.119117
Bilal, M., Fernandes, C. D., Mehmood, T., Nadeem, F., Tabassam, Q., and Ferreira, L. F. R. (2021). Immobilized lipases-based nano-biocatalytic systems — a versatile platform with incredible biotechnological potential. Int. J. Biol. Macromol. 175, 108–122. doi:10.1016/j.ijbiomac.2021.02.010
Binhayeeding, N., Klomklao, S., Prasertsan, P., and Sangkharak, K. (2020b). Improvement of biodiesel production using waste cooking oil and applying single and mixed immobilised lipases on polyhydroxyalkanoate. Renew. Energy 162, 1819–1827. doi:10.1016/j.renene.2020.10.009
Binhayeeding, N., Yunu, T., Pichid, N., Klomklao, S., and Sangkharak, K. (2020a). Immobilisation of Candida rugosa lipase on polyhydroxybutyrate via a combination of adsorption and cross-linking agents to enhance acylglycerol production. Process Biochem. 95, 174–185. doi:10.1016/j.procbio.2020.02.007
Bonet-Ragel, K., López-Pou, L., Tutusaus, G., Benaiges, M. D., and Valero, F. (2018). Rice husk ash as a potential carrier for the immobilization of lipases applied in the enzymatic production of biodiesel. Biocatal. Biotransfor. 36 (2), 151–158. doi:10.1080/10242422.2017.1308498
Bornscheuer, U. T., Huisman, G. W., Kazlauskas, R. J., Lutz, S., Moore, J. C., and Robins, K. (2012). Engineering the third wave of biocatalysis. Nature 485 (7397), 185–194. doi:10.1038/nature11117
Borrelli, G. M., and Trono, D. (2015). Recombinant lipases and phospholipases and their use as biocatalysts for industrial applications. Int. J. Mol. Sci. 16 (9), 20774–20840. doi:10.3390/ijms160920774
Brahma, S., Nath, B., Basumatary, B., Das, B., Saikia, P., Patir, K., et al. (2022). Biodiesel production from mixed oils: a sustainable approach towards industrial biofuel production. Chem. Eng. J. Adv. 10, 100284. doi:10.1016/j.ceja.2022.100284
Budžaki, S., Miljić, G., Sundaram, S., Tišma, M., and Hessel, V. (2018). Cost analysis of enzymatic biodiesel production in small-scaled packed-bed reactors. Appl. Energy 210, 268–278. doi:10.1016/j.apenergy.2017.11.026
Castro-Ochoa, D., Peña-Montes, C., González-Canto, A., Alva-Gasca, A., Esquivel-Bautista, R., Navarro-Ocaña, A., et al. (2012). ANCUT2, an extracellular cutinase from Aspergillus nidulans induced by olive oil. Appl. Biochem. Biotech. 166 (5), 1275–1290. doi:10.1007/s12010-011-9513-7
Cen, Y. K., Liu, Y. X., Xue, Y. P., and Zheng, Y. G. (2019). Immobilization of enzymes in/on membranes and their applications. Adv. Synth. Catal. 361 (24), 5500–5515. doi:10.1002/adsc.201900439
Cerioni Spiropulos Gonçalves, E., Martínez Pérez, M., Vici, A. C., Santos Salgado, J. C., de Souza Rocha, M., Zaghetto de Almeida, P., et al. (2020). Potential biodiesel production from Brazilian plant oils and spent coffee grounds by Beauveria bassiana lipase 1 expressed in Aspergillus nidulans A773 using different agroindustry inputs. J. Clean. Prod. 256, 120513. doi:10.1016/j.jclepro.2020.120513
Cespugli, M., Lotteria, S., Navarini, L., Lonzarich, V., Del Terra, L., Vita, F., et al. (2018). Rice husk as an inexpensive renewable immobilization carrier for biocatalysts employed in the food, cosmetic and polymer sectors. Catalysts 8 (10), 471. doi:10.3390/catal8100471
Chandra, P., Enespa Singh, R., and Arora, P. K. (2020). Microbial lipases and their industrial applications: a comprehensive review. Microb. Cell. Fact. 19, 169. doi:10.1186/S12934-020-01428-8
Chen, G., Liu, J., Qi, Y., Yao, J., and Yan, B. (2016). Biodiesel production using magnetic whole-cell biocatalysts by immobilization of Pseudomonas mendocina on Fe3O4-chitosan microspheres. Biochem. Eng. J. 113, 86–92. doi:10.1016/j.bej.2016.06.003
Chen, G., Liu, J., Yao, J., Qi, Y., and Yan, B. (2017). Biodiesel production from waste cooking oil in a magnetically fluidized bed reactor using whole-cell biocatalysts. Energy Convers. manage. 138, 556–564. doi:10.1016/j.enconman.2017.02.036
Chen, J. P., and Lin, G. H. (2010). Optimization of biodiesel production catalyzed by fungus cells immobilized in fibrous supports. Appl. Biochem. Biotech. 161 (1–8), 181–194. doi:10.1007/S12010-009-8776-8
Chen, R. R. (2007). Permeability issues in whole-cell bioprocesses and cellular membrane engineering. Appl. Microbiol. Biot. 74 (4), 730–738. doi:10.1007/S00253-006-0811-X
Chen, X. (2017). Yeast cell surface display: an efficient strategy for improvement of bioethanol fermentation performance. Bioengineered 8 (2), 115–119. doi:10.1080/21655979.2016.1212135
Chow, J. Y., Choo, K. L. S., Lim, Y. P., Ling, L. H., Nguyen, G. K. T., Xue, B., et al. (2021). Scalable workflow for green manufacturing: discovery of bacterial lipases for biodiesel production. ACS Sustain. Chem. Eng. 9 (40), 13450–13459. doi:10.1021/acssuschemeng.1C03721
Committee for Standardization (2003). Automotive Fuels 2013 Fatty acid methyl esters (FAME) for diesel engines requirements and test methods; Standard EN 14214. Brussels, Belgium: European Committee for Standardization.
Contesini, F. J., Davanço, M. G., Borin, G. P., Vanegas, K. G., Cirino, J. P. G., de Melo, R. R., et al. (2020). Advances in recombinant lipases: production, engineering, immobilization and application in the pharmaceutical industry. Catalysts 10 (9), 1032. doi:10.3390/catal10091032
Costa-Silva, T. A., Carvalho, A. K. F., Souza, C. R. F., De Castro, H. F., Said, S., and Oliveira, W. P. (2016). Enzymatic synthesis of biodiesel using immobilized lipase on a non-commercial support. Energy fuels. 30 (6), 4820–4824. doi:10.1021/acs.energyfuels.6b00208
Dakin, H. D. (1903). The hydrolysis of optically inactive esters by means of enzymes. J. Physiol. 30, 253–263. doi:10.1113/jphysiol.1903.sp000992
de Carvalho, C. C. C. R. (2017). Whole cell biocatalysts: essential workers from Nature to the industry. Microb. Biotechnol. 10 (2), 250–263. doi:10.1111/1751-7915.12363
de Castro, M. da C., Garcia, P. S., Andrade, M. M., Grossmann, M. V. E., Simões, B. M., Samulewski, R. B., et al. (2022). Lipase immobilization on biodegradable film with sericin. Biotechnol. Appl. Bioc. 69 (2), 660–667. doi:10.1002/bab.2141
Degfie, T. A., Mamo, T. T., and Mekonnen, Y. S. (2019). Optimized biodiesel production from waste cooking oil (WCO) using calcium oxide (CaO) nano-catalyst. Sci. Rep. 9, 18982. doi:10.1038/s41598-019-55403-4
de Oliveira, U. M. F., Lima de Matos, L. J. B., de Souza, M. C. M., Pinheiro, B. B., dos Santos, J. C. S., and Gonçalves, L. R. B. (2017). Immobilization conditions on catalysts’ properties of Rhizomucor miehei lipase onto chitosan. Appl. Biochem. Biotechnol. 184, 1263–1285. doi:10.1007/s12010-017-2622-1
de Oliveira, B. H., Coradi, G. V., de Oliva-Neto, P., and do Nascimento, V. M. G. (2020). Biocatalytic benefits of immobilized Fusarium sp. (GFC) lipase from solid state fermentation on free lipase from submerged fermentation. Ind. Crops Prod. 147, 112235. doi:10.1016/j.indcrop.2020.112235
de Paula, M. S., de Oliveira, L. F. C., Cunha, F. T., Gomes, T. P. C., Pereira, P. I. N. M., and Langone, M. A. P. (2021). Evaluation of continuous ethyl ester synthesis from acid soybean oil using a dual immobilized enzyme system. Biomass Bioenerg. 144, 105898. doi:10.1016/j.biombioe.2020.105898
Ding, J., Qu, S., Lv, E., Lu, J., and Yi, W. (2020). Mini review of biodiesel by integrated membrane separation technologies that enhanced esterification/transesterification. Energy and Fuels 34 (12), 15614–15633. doi:10.1021/acs.energyfuels.0c03307
Dong, C., Qiao, J., Wang, X., Sun, W., Chen, L., Li, S., et al. (2020). Engineering Pichia pastoris with surface-display minicellulosomes for carboxymethyl cellulose hydrolysis and ethanol production. Biotechnol. Biofuels Bioprod. 13, 108. doi:10.1186/S13068-020-01749-1
dos Santos, J. C. S., Garcia-Galan, C., Rodrigues, R. C., de Sant’ Ana, H. B., Gonçalves, L. R. B., and Fernandez-Lafuente, R. (2014). Improving the catalytic properties of immobilized Lecitase via physical coating with ionic polymers. Enz. Microb. Technol. 60, 1–8. doi:10.1016/j.enzmictec.2014.03.001
Emmanouilidou, E., and Kokkinos, N. C. (2022). Membrane performance on biodiesel production and purification: a review. Int. J. Membr. Sci. Techno. 9, 1–13. doi:10.15379/2410-1869.2022.01
Escobar-Niño, A., Luna, C., Luna, D., Marcos, A. T., Cánovas, D., and Mellado, E. (2014). Selection and characterization of biofuel-producing environmental bacteria isolated from vegetable oil-rich wastes. PLoS One 9 (8), e104063. doi:10.1371/journal.pone.0104063
Esmi, F., Nematian, T., Salehi, Z., Khodadadi, A. A., and Dalai, A. K. (2021). Amine and aldehyde functionalized mesoporous silica on magnetic nanoparticles for enhanced lipase immobilization, biodiesel production, and facile separation. Fuel 291, 120126. doi:10.1016/j.fuel.2021.120126
Fan, S., Liang, B., Xiao, X., Bai, L., Tang, X., Lojou, E., et al. (2020). Controllable display of sequential enzymes on yeast surface with enhanced biocatalytic activity toward efficient enzymatic biofuel cells. J. Am. Chem. Soc. 142 (6), 3222–3230. doi:10.1021/JACS.9B13289
Filho, D. G., Silva, A. G., and Guidini, C. Z. (2019). Lipases: sources, immobilization methods, and industrial applications. Appl. Microbiol. Biot. 103 (18), 7399–7423. doi:10.1007/s00253-019-10027-6
Fu, B., and Vasudevan, P. T. (2010). Effect of solvent-co-solvent mixtures on lipase-catalyzed transesterification of canola oil. Energy fuels. 24 (9), 4646–4651. doi:10.1021/ef901176h
Giakoumis, E. G., and Sarakatsanis, C. K. (2018). Estimation of biodiesel cetane number, density, kinematic viscosity and heating values from its fatty acid weight composition. Fuel 222, 574–585. doi:10.1016/j.fuel.2018.02.187
Girelli, A. M., and Chiappini, V. (2023). Renewable, sustainable, and natural lignocellulosic carriers for lipase immobilization: a review. J. Biotechnol. 365, 29–47. doi:10.1016/j.jbiotec.2023.02.003
Gojun, M., Šalić, A., and Zelić, B. (2021). Integrated microsystems for lipase-catalyzed biodiesel production and glycerol removal by extraction or ultrafiltration. Renew. Energy 180, 213–221. doi:10.1016/j.renene.2021.08.064
Grant, C., Deszcz, D., Wei, Y.-C., Martínez-Torres, R. J., Morris, P., Folliard, T., et al. (2014). Identification and use of an alkane transporter plug-in for applications in biocatalysis and whole-cell biosensing of alkanes. Sci. Rep. 4, 5844. doi:10.1038/srep05844
Guldhe, A., Singh, P., Kumari, S., Rawat, I., Permaul, K., and Bux, F. (2016). Biodiesel synthesis from microalgae using immobilized Aspergillus niger whole cell lipase biocatalyst. Renew. Energy 85, 1002–1010. doi:10.1016/j.renene.2015.07.059
Hajilary, N., Rezakazemi, M., and Shirazian, S. (2019). Biofuel types and membrane separation. Env. Chem. Lett. 17 (1), 1–18. doi:10.1007/s10311-018-0777-9
Hama, S., and Kondo, A. (2013). Enzymatic biodiesel production: an overview of potential feedstocks and process development. Biores. Technol. 135, 386–395. doi:10.1016/j.biortech.2012.08.014
Hama, S., Noda, H., and Kondo, A. (2018). How lipase technology contributes to evolution of biodiesel production using multiple feedstocks. Curr. Opin. Biotechnol. 50, 57–64. doi:10.1016/J.COPBIO.2017.11.001
Hama, S., Tamalampudi, S., Suzuki, Y., Yoshida, A., Fukuda, H., and Kondo, A. (2008). Preparation and comparative characterization of immobilized Aspergillus oryzae expressing Fusarium heterosporum lipase for enzymatic biodiesel production. Appl. Microbiol. Biot. 81 (4), 637–645. doi:10.1007/S00253-008-1689-6
Hama, S., Yamaji, H., Fukumizu, T., Numata, T., Tamalampudi, S., Kondo, A., et al. (2007). Biodiesel-fuel production in a packed-bed reactor using lipase-producing Rhizopus oryzae cells immobilized within biomass support particles. Biochem. Eng. J. 34 (3), 273–278. doi:10.1016/j.bej.2006.12.013
Han, L., Zhao, Y., Cui, S., and Liang, B. (2018). Redesigning of microbial cell surface and its application to whole-cell biocatalysis and biosensors. Appl. Biochem. Biotech. 185 (2), 396–418. doi:10.1007/S12010-017-2662-6
Han, Z. L., Han, S. Y., Zheng, S. P., and Lin, Y. (2009). Enhancing thermostability of a Rhizomucor miehei lipase by engineering a disulfide bond and displaying on the yeast cell surface. Appl. Microbiol. Biot. 85, 117–126. doi:10.1007/S00253-009-2067-8
Handayani, N., Wahyuningrum, D., Zulfikar, M. A., Nurbaiti, S., Radiman, C. L., and Buchari, H. (2016). The synthesis of biodiesel catalyzed by Mucor miehei lipase immobilized onto aminated polyethersulfone membranes. Biores. Bioprocess. 3 (1), 22. doi:10.1186/s40643-016-0098-4
Harabi, M., Bouguerra, S. N., Marrakchi, F., Chrysikou, L. P., Bezergianni, S., and Bouaziz, M. (2019). Biodiesel and crude glycerol from waste frying oil: production, characterization and evaluation of biodiesel oxidative stability with diesel blends. Sustainability 11 (7), 1937. doi:10.3390/su11071937
Hasheminejad, M., Tabatabaei, M., Mansourpanah, Y., far, M. K., and Javani, A. (2011). Upstream and downstream strategies to economize biodiesel production. Biores. Technol. 102 (2), 461–468. doi:10.1016/j.biortech.2010.09.094
He, Q., Xia, Q., Wang, Y., Li, X., Zhang, Y., Hu, B., et al. (2016). Biodiesel production: utilization of loofah sponge to immobilize Rhizopus chinensis CGMCC #3.0232 cells as a whole-cell biocatalyst. J. Microbiol. Biotechn. 26 (7), 1278–1284. doi:10.4014/jmb.1601.01075
He, Y., Li, K., Bo, G., Wang, J., Xu, L., Yan, J., et al. (2022). Enhancing biodiesel production via liquid Yarrowia lipolytica lipase 2 in deep eutectic solvents. Fuel 316, 123342. doi:10.1016/j.fuel.2022.123342
Holm-Nielsen, J. B., Al Seadi, T., and Oleskowicz-Popiel, P. (2009). The future of anaerobic digestion and biogas utilization. Biores. Technol. 100 (22), 5478–5484. doi:10.1016/j.biortech.2008.12.046
Huang, D., Han, S., Han, Z., and Lin, Y. (2012). Biodiesel production catalyzed by Rhizomucor miehei lipase-displaying Pichia pastoris whole cells in an isooctane system. Biochem. Eng. J. 63, 10–14. doi:10.1016/J.BEJ.2010.08.009
Hwang, H. T., Qi, F., Yuan, C., Zhao, X., Ramkrishna, D., Liu, D., et al. (2014). Lipase-catalyzed process for biodiesel production: protein engineering and lipase production. Biotechnol. Bioeng. 111, 639–653. doi:10.1002/bit.25162
Ismail, A. R., and Baek, K. H. (2020). Lipase immobilization with support materials, preparation techniques, and applications: present and future aspects. Int. J. Biol. Macromol. 163, 1624–1639. doi:10.1016/j.ijbiomac.2020.09.021
Iyyappan, J., Jayamuthunagai, J., Bharathiraja, B., Saravanaraj, A., Praveen Kumar, R., Balraj, S., et al. (2022). Production of biodiesel from Caulerpa racemosa oil using recombinant Pichia pastoris whole cell biocatalyst with double displayed over expression of Candida antartica lipase. Biores. Technol. 363, 127893. doi:10.1016/j.biortech.2022.127893
Jafarian, F., Bordbar, A. K., Razmjou, A., and Zare, A. (2020). The fabrication of a high performance enzymatic hybrid membrane reactor (EHMR) containing immobilized Candida rugosa lipase (CRL) onto graphene oxide nanosheets-blended polyethersulfone membrane. J. Membr. Sci. 613, 118435. doi:10.1016/j.memsci.2020.118435
Jin, Z., Han, S. Y., Zhang, L., Zheng, S. P., Wang, Y., and Lin, Y. (2013). Combined utilization of lipase-displaying Pichia pastoris whole-cell biocatalysts to improve biodiesel production in co-solvent media. Biores. Technol. 130, 102–109. doi:10.1016/j.biortech.2012.12.020
Kapdan, I. K., and Kargi, F. (2006). Bio-hydrogen production from waste materials. Enz. Microb. Technol. 38 (5), 569–582. doi:10.1016/j.enzmictec.2005.09.015
Kendirci, P., Salum, P., Bas, D., and Erbay, Z. (2020). Production of enzyme-modified cheese (EMC) with ripened white cheese flavour: II- effects of lipases. Food Bioprod. process. 122, 230–244. doi:10.1016/j.fbp.2020.05.010
Khan, N., Maseet, M., and Basir, S. F. (2020). Synthesis and characterization of biodiesel from waste cooking oil by lipase immobilized on genipin cross-linked chitosan beads: a green approach. Int. J. Green Energy 17 (1), 84–93. doi:10.1080/15435075.2019.1700122
Khoobbakht, G., Kheiralipour, K., Yuan, W., Seifi, M. R., and Karimi, M. (2020). Desirability function approach for optimization of enzymatic transesterification catalyzed by lipase immobilized on mesoporous magnetic nanoparticles. Renew. Energy 158, 253–262. doi:10.1016/j.renene.2020.05.087
Khosla, K., Rathour, R., Maurya, R., Maheshwari, N., Gnansounou, E., Larroche, C., et al. (2017). Biodiesel production from lipid of carbon dioxide sequestrating bacterium and lipase of psychrotolerant Pseudomonas sp. ISTPL3 immobilized on biochar. Biores. Technol. 245, 743–750. doi:10.1016/j.biortech.2017.08.194
Kim, K. H., Lee, O. K., and Lee, E. Y. (2018). Nano-immobilized biocatalysts for biodiesel production from renewable and sustainable resources. Catalysts 8 (2), 68. doi:10.3390/catal8020068
Kim, S., and Dale, B. E. (2004). Global potential bioethanol production from wasted crops and crop residues. Biomass Bioenerg. 26, 361–375. doi:10.1016/j.biombioe.2003.08.002
Kim, S. J., Song, J. K., and Kim, H. K. (2013). Cell surface display of Staphylococcus haemolyticus L62 lipase in Escherichia coli and its application as a whole cell biocatalyst for biodiesel production. J. Mol. Catal. B Enzym. 97, 54–61. doi:10.1016/j.molcatb.2013.07.017
Ko, M. J., Park, H. J., Hong, S. Y., and Yoo, Y. J. (2012). Continuous biodiesel production using in situ glycerol separation by membrane bioreactor system. Bioprocess Biosyst. Eng. 35 (1), 69–75. doi:10.1007/s00449-011-0604-1
Kujawa, J., Glodek, M., Li, G., Al-Gharabli, S., Knozowska, K., and Kujawski, W. (2021). Highly effective enzymes immobilization on ceramics: requirements for supports and enzymes. Sci. Total Environ. 801, 149647. doi:10.1016/j.scitotenv.2021.149647
Kumar, D., Das, T., Giri, B. S., and Verma, B. (2018). Characterization and compositional analysis of highly acidic karanja oil and its potential feedstock for enzymatic synthesis of biodiesel. New J. Chem. 42 (19), 15593–15602. doi:10.1039/C8NJ03084G
Kumar, R., and Pal, P. (2021). Lipase immobilized graphene oxide biocatalyst assisted enzymatic transesterification of Pongamia pinnata (Karanja) oil and downstream enrichment of biodiesel by solar-driven direct contact membrane distillation followed by ultrafiltration. Fuel Process. Technol. 211, 106577. doi:10.1016/j.fuproc.2020.106577
Kuo, C. H., Peng, L. T., Kan, S. C., Liu, Y. C., and Shieh, C. J. (2013). Lipase-immobilized biocatalytic membranes for biodiesel production. Biores. Technol. 145, 229–232. doi:10.1016/j.biortech.2012.12.054
Kuroda, K., and Ueda, M. (2022a). Simultaneous display of multiple kinds of enzymes on the yeast cell surface for multistep reactions. Methods Mol. Biol. 2491, 627–641. doi:10.1007/978-1-0716-2285-8_26
Kuroda, K., and Ueda, M. (2022b). Generation of arming yeasts with active proteins and peptides via cell surface display system: cell surface engineering, bio-arming technology. Methods Mol. Biol. 2513, 59–77. doi:10.1007/978-1-0716-2399-2_5
Kyeong, J. S., and Yeom, S. H. (2014). Preparation of immobilized whole cell biocatalyst and biodiesel production using a packed-bed bioreactor. Bioprocess Biosyst. Eng. 37 (11), 2189–2198. doi:10.1007/S00449-014-1196-3
Levin, D. B., Pitt, L., and Love, M. (2004). Biohydrogen production: prospects and limitations to practical application. Int. J. Hydrog. Energy 29 (2), 173–185. doi:10.1016/S0360-3199(03)00094-6
Li, S., Qiao, J., Lin, S., Liu, Y., and Ma, L. (2019a). A highly efficient indirect P. pastoris surface display method based on the CL7/Im7 ultra-high-affinity system. Molecules 24 (8), 1483. doi:10.3390/molecules24081483
Li, Y., Wang, H., Lu, J., Chu, A., Zhang, L., Ding, Z., et al. (2019b). Preparation of immobilized lipase by modified polyacrylonitrile hollow membrane using nitrile-click chemistry. Biores. Technol. 274, 9–17. doi:10.1016/j.biortech.2018.11.075
Lin, B., and Tao, Y. (2017). Whole-cell biocatalysts by design. Microb. Cell. Fact. 16, 106. doi:10.1186/s12934-017-0724-7
Linsha, V., Aboo Shuhailath, K., Mahesh, K. V., Mohamed, A. A. P., and Ananthakumar, S. (2016). Biocatalytic conversion efficiency of steapsin lipase immobilized on hierarchically porous biomorphic aerogel supports. ACS Sus. Chem. Eng. 4 (9), 4692–4703. doi:10.1021/acssuschemeng.6b00821
Liu, J., Chen, G., Yan, B., Yi, W., and Yao, J. (2022). Biodiesel production in a magnetically fluidized bed reactor using whole-cell biocatalysts immobilized within ferroferric oxide-polyvinyl alcohol composite beads. Bioresour. Technol. 355, 127253. doi:10.1016/j.biortech.2022.127253
Liu, Y., Huang, L., Zheng, D., Fu, Y., Shan, M., Xu, Z., et al. (2018). Development of a Pichia pastoris whole-cell biocatalyst with overexpression of mutant lipase PCLG47I from Penicillium cyclopium for biodiesel production. RSC Adv. 8 (46), 26161–26168. doi:10.1039/c8ra04462g
López, C., Cruz-Izquierdo, Á., Picó, E. A., Garcia-Bárcena, T., Villarroel, N., Llama, M. J., et al. (2014). Magnetic biocatalysts and their uses to obtain biodiesel and biosurfactants. Front. Chem. 2, 72. doi:10.3389/fchem.2014.00072
López-Fernández, J., Benaiges, M. D., and Valero, F. (2020). Rhizopus oryzae lipase, a promising industrial enzyme: biochemical characteristics, production and biocatalytic applications. Catalysts 10 (11), 1277. doi:10.3390/catal10111277
Lotti, M., Pleiss, J., Valero, F., and Ferrer, P. (2018). Enzymatic production of biodiesel: strategies to overcome methanol inactivation. Biotechnol. J. 13 (5), 1700155. doi:10.1002/biot.201700155
Lugue, R., and Melero, J. A. (2012). “1-Introduction to advanced biodiesel production,” in Advanced biodiesel production. Editors R. Lugue,, and J. A. Melero (China: Woodhead Publishing), 1–9. doi:10.1533/9780857095862.1
Lv, L., Dai, L., Du, W., and Liu, D. (2021). Progress in enzymatic biodiesel production and commercialization. Processes 9 (2), 355. doi:10.3390/pr9020355
Ma, G., Dai, L., Liu, D., and Du, W. (2018). A robust two-step process for the efficient conversion of acidic soybean oil for biodiesel production. Catalysts 8 (11), 527. doi:10.3390/catal8110527
Machsun, A. L., Gozan, M., Nasikin, M., Setyahadi, S., and Yoo, Y. J. (2010). Membrane microreactor in biocatalytic transesterification of triolein for biodiesel production. Biotechnol. Bioproc. Eng. 15, 911–916. doi:10.1007/s12257-010-0151-7
Maidana Serpa, J. D., Cavalieri de Alencar Guimarães, N., Kioshi Yonekawa, M. A., Pereira de Almeida, A., Ruller, R., Augusto dos Santos Jaques, J., et al. (2022). Sarocladium strictum lipase (LipSs) produced using crude glycerol as sole carbon source: a promising enzyme for biodiesel production. Biocatal. Agric. Biotechnol. 40, 102299. doi:10.1016/j.bcab.2022.102299
Mandal, M. K., Chanu, N. K., and Chaurasia, N. (2020). Exogenous addition of indole acetic acid and kinetin under nitrogen-limited medium enhances lipid yield and expression of glycerol-3-phosphate acyltransferase and diacylglycerol acyltransferase genes in indigenous microalgae: a potential approach for biodiesel production. Biores. Technol. 297, 122439. doi:10.1016/j.biortech.2019.122439
Mandari, V., and Devarai, S. K. (2022). Biodiesel production using homogeneous, heterogeneous, and enzyme catalysts via transesterification and esterification reactions: a critical review. BioEnergy Res. 15, 935–961. doi:10.1007/s12155-021-10333-w
Marchetti, J. M., and Errazu, A. F. (2010). Biodiesel production from acid oils and ethanol using a solid basic resin as catalyst. Biomass Bioenergy 34 (3), 272–277. doi:10.1016/j.biombioe.2009.10.016
Marchetti, J. M., Pedernera, M. N., and Schbib, N. S. (2011). Production of biodiesel from acid oil using sulfuric acid as catalyst: kinetics study. Int. J. Low. Carbon Technol. 6 (1), 38–43. doi:10.1093/ijlct/ctq040
Marín-Suárez, M., Méndez-Mateos, D., Guadix, A., and Guadix, E. M. (2019). Reuse of immobilized lipases in the transesterification of waste fish oil for the production of biodiesel. Renew. Energy 140, 1–8. doi:10.1016/j.renene.2019.03.035
Matsumoto, T., Fukuda, H., Ueda, M., Tanaka, A., and Kondo, A. (2002). Construction of yeast strains with high cell surface lipase activity by using novel display systems based on the Flo1p flocculation functional domain. Appl. Environ. Microbiol. 68 (9), 4517–4522. doi:10.1128/aem.68.9.4517-4522.2002
Matsumoto, T., Takahashi, S., Kaieda, M., Ueda, M., Tanaka, A., Fukuda, H., et al. (2001). Yeast whole-cell biocatalyst constructed by intracellular overproduction of Rhizopus oryzae lipase is applicable to biodiesel fuel production. Appl. Microbiol. Biot. 57 (4), 515–520. doi:10.1007/S002530100733
Meunier, S. M., and Legge, R. L. (2012). Evaluation of diatomaceous earth supported lipase sol-gels as a medium for enzymatic transesterification of biodiesel. J. Mol. Catal. B Enzym. 77, 92–97. doi:10.1016/j.molcatb.2012.01.014
Mohimed, N. A., Mohamad, M., Rashid, M. U., Norizan, M. N., Hamzah, F., and Mat, H. b. (2023). Recent advances in enzyme immobilisation strategies: an overview of techniques and composite carriers. J. Compos. Sci. 7 (12), 488. doi:10.3390/jcs7120488
Mokhtar, N. F., Rahman, R. N. Z. R. A., Noor, N. D. M., Shariff, F. M., and Ali, M. S. M. (2020). The immobilization of lipases on porous support by adsorption and hydrophobic interaction method. Catalysts 10 (7), 744. doi:10.3390/catal10070744
Monteiro, R. R. C., Arana-Peña, S., da Rocha, T. N., Miranda, L. P., Berenguer-Murcia, Á., Tardioli, P. W., et al. (2021). Liquid lipase preparations designed for industrial production of biodiesel. Is it really an optimal solution? Renew. Energy 164, 1566–1587. doi:10.1016/j.renene.2020.10.071
Naik, S. N., Goud, V. V., Rout, P. K., and Dalai, A. K. (2010). Production of first and second generation biofuels: a comprehensive review. Renew. Sust. Energy Rev. 14 (2), 578–597. doi:10.1016/j.rser.2009.10.003
Ng, W. Z., Obon, A. A., Lee, C. L., Ong, Y. H., Gourich, W., Maran, K., et al. (2022). Techno-economic analysis of enzymatic biodiesel co-produced in palm oil mills from sludge palm oil for improving renewable energy access in rural areas. Energy 243, 122745. doi:10.1016/j.energy.2021.122745
Ni, Y., and Chen, R. R. (2004). Accelerating whole-cell biocatalysis by reducing outer membrane permeability barrier. Biotechnol. Bioeng. 87 (6), 804–811. doi:10.1002/bit.20202
Ogino, C., and Amoah, J. (2019). Energy production: biodiesel. Yeast Cell. Surf. Eng., 43–61. doi:10.1007/978-981-13-5868-5_4
Pandey, D., Daverey, A., and Arunachalam, K. (2020). Biochar: production, properties and emerging role as a support for enzyme immobilization. J. Clean. Prod. 255, 120267. doi:10.1016/j.jclepro.2020.120267
Parandi, E., Safaripour, M., Abdellattif, M. H., Saidi, M., Bozorgian, A., Rashidi Nodeh, H., et al. (2022). Biodiesel production from waste cooking oil using a novel biocatalyst of lipase enzyme immobilized magnetic nanocomposite. Fuel 313, 123057. doi:10.1016/j.fuel.2021.123057
Paris, B., Papadakis, G., Janssen, R., and Rutz, D. (2021). Economic analysis of advanced biofuels, renewable gases, electrofuels and recycled carbon fuels for the Greek transport sector until 2050. Renew. Sustain. Energy Rev. 144, 111038. doi:10.1016/j.rser.2021.111038
Pavlidis, I. V., Patila, M., Bornscheuer, U. T., Gournis, D., and Stamatis, H. (2014). Graphene-based nanobiocatalytic systems: recent advances and future prospects. Trends Biotechnol. 32 (6), 312–320. doi:10.1016/j.tibtech.2014.04.004
Pinto Brito, M. J., Bauer, L. C., Flores Santos, M. P., Santos, L. S., Ferreira Bonomo, R. C., da Costa Ilhéu Fontan, R., et al. (2020). Lipase immobilization on activated and functionalized carbon for the aroma ester synthesis. Micropor. Mesopor. Mat. 309, 110576. doi:10.1016/j.micromeso.2020.110576
Poppe, J. K., Matte, C. R., de Freitas, V. O., Fernandez-Lafuente, R., Rodrigues, R. C., and Záchia Ayub, M. A. (2018a). Enzymatic synthesis of ethyl esters from waste oil using mixtures of lipases in a plug-flow packed-bed continuous reactor. Biotechnol. Prog. 34 (4), 952–959. doi:10.1002/btpr.2650
Poppe, J. K., Matte, C. R., Fernandez-Lafuente, R., Rodrigues, R. C., and Ayub, M. A. Z. (2018b). Transesterification of waste frying oil and soybean oil by combi-lipases under ultrasound-assisted reactions. Appl. Biochem. Biotech. 186 (3), 576–589. doi:10.1007/s12010-018-2763-x
Quayson, E., Amoah, J., Rachmadona, N., Hama, S., Yoshida, A., Kondo, A., et al. (2020a). Biodiesel-mediated biodiesel production: a recombinant Fusarium heterosporum lipase-catalyzed transesterification of crude plant oils. Fuel Process. Technol. 199, 106278. doi:10.1016/j.fuproc.2019.106278
Quayson, E., Amoah, J., Rachmadona, N., Morita, K., Darkwah, L., Hama, S., et al. (2020b). Valorization of palm biomass waste into carbon matrices for the immobilization of recombinant Fusarium heterosporum lipase towards palm biodiesel synthesis. Biomass Bioenerg. 142, 105768. doi:10.1016/j.biombioe.2020.105768
Rana, Q. U. A., Irfan, M., Ahmed, S., Hasan, F., Shah, A. A., Khan, S., et al. (2022). Bio-catalytic transesterification of mustard oil for biodiesel production. Biofuels 13 (1), 69–76. doi:10.1080/17597269.2019.1655907
Ranieri, G., Mazzei, R., Wu, Z., Li, K., and Giorno, L. (2016). Use of a ceramic membrane to improve the performance of two-separate-phase biocatalytic membrane reactor. Molecules 21 (3), 345. doi:10.3390/molecules21030345
Raoufi, Z., and Mousavi Gargari, S. L. (2018). Biodiesel production from microalgae oil by lipase from Pseudomonas aeruginosa displayed on yeast cell surface. Biochem. Eng. J. 140, 1–8. doi:10.1016/j.bej.2018.09.008
Ren, Y., Rivera, J. G., He, L., Kulkarni, H., Lee, D. K., and Messersmith, P. B. (2011). Facile, high efficiency immobilization of lipase enzyme on magnetic iron oxide nanoparticles via a biomimetic coating. BMC Biotechnol. 11, 63. doi:10.1186/1472-6750-11-63
Rodrigues, J., Perrier, V., Lecomte, J., Dubreucq, E., and Ferreira-Dias, S. (2016). Biodiesel production from crude jatropha oil catalyzed by immobilized lipase/acyltransferase from Candida parapsilosis in aqueous medium. Biores. Technol. 218, 1224–1229. doi:10.1016/j.biortech.2016.07.090
Rodrigues, R. C., and Ayub, M. A. Z. (2011). Effects of the combined use of Thermomyces lanuginosus and Rhizomucor miehei lipases for the transesterification and hydrolysis of soybean oil. Process Biochem. 46 (3), 682–688. doi:10.1016/j.procbio.2010.11.013
Romero, A. S., de Mello Innocentini, M. D., Hotza, D., and Oliveira, J. V. (2022). Dry polishing of enzymatically produced fatty acid methyl esters through polymeric membranes. Chem. Eng. Res. Des. 186, 64–72. doi:10.1016/j.cherd.2022.07.040
Roume, H., Arends, J. B. A., Ameril, C. P., Patil, S. A., and Rabaey, K. (2016). Enhanced product recovery from glycerol fermentation into 3-carbon compounds in a bioelectrochemical system combined with in situ extraction. Front. Bioeng. Biotechnol. 4, 73. doi:10.3389/fbioe.2016.00073
Sahoo, R. K., Kumar, M., Sukla, L. B., and Subudhi, E. (2017). Bioprospecting hot spring metagenome: lipase for the production of biodiesel. Environ. Sci. Pollut. Res. 24 (4), 3802–3809. doi:10.1007/S11356-016-8118-7
Sakkos, J. K., Wackett, L. P., and Aksan, A. (2019). Enhancement of biocatalyst activity and protection against stressors using a microbial exoskeleton. Sci. Rep. 9, 3158. doi:10.1038/S41598-019-40113-8
Sales, M. B., Borges, P. T., Ribeiro Filho, M. N., Miranda da Silva, L. R., Castro, A. P., Sanders Lopes, A. A., et al. (2022). Sustainable feedstocks and challenges in biodiesel production: an advanced bibliometric analysis. Bioengineering 9 (10), 539. doi:10.3390/bioengineering9100539
Sarkar, N., Ghosh, S. K., Bannerjee, S., and Aikat, K. (2012). Bioethanol production from agricultural wastes: an overview. Renew. Energy 37, 19–27. doi:10.1016/j.renene.2011.06.045
Schrewe, M., Julsing, M. K., Bühler, B., and Schmid, A. (2013). Whole-cell biocatalysis for selective and productive C–O functional group introduction and modification. Chem. Soc. Rev. 42 (15), 6346–6377. doi:10.1039/c3cs60011d
Sena, R. O., Carneiro, C., Holanda Moura, M. V., Brêda, G. C., Pinto, M. C. C., Gomes Moura Fé, L. X. S., et al. (2021) Application of Rhizomucor miehei lipase-displaying Pichia pastoris whole cell for biodiesel production using agro-industrial residuals as substrate. Int. J. Biol. Macromol., 189, 734–743. doi:10.1016/j.ijbiomac.2021.08.173
Serralha, F. N., Lopes, J. M., Lemos, F., Prazeres, D. M. F., Aires-Barros, M. R., Cabral, J. M. S., et al. (1998). Zeolites as supports for an enzymatic alcoholysis reaction. J. Mol. Catal. B Enz. 4 (5–6), 303–311. doi:10.1016/S1381-1177(98)00069-1
Shahedi, M., Yousefi, M., Habibi, Z., Mohammadi, M., and As’habi, M. A. (2019). Co-immobilization of Rhizomucor miehei lipase and Candida antarctica lipase B and optimization of biocatalytic biodiesel production from palm oil using response surface methodology. Renew. Energy 141, 847–857. doi:10.1016/j.renene.2019.04.042
Shibasaki, S., Maeda, H., and Ueda, M. (2009). Molecular display technology using yeast-arming technology. Anal. Sci. 25, 41–49. doi:10.2116/analsci.25.41
Sirkar, K. K., Shanbhag, P. V., and Kovvali, A. S. (1999). Membrane in a reactor: a functional perspective. Ind. Eng. Chem. Res. 38 (10), 3715–3737. doi:10.1021/ie990069j
Smith, M. R., Khera, E., and Wen, F. (2015). Engineering novel and improved biocatalysts by cell surface display. Ind. Eng. Chem. Res. 54 (16), 4021–4032. doi:10.1021/ie504071f
Sokač, T., Gojun, M., Tušek, A. J., Šalić, A., and Zelić, B. (2020). Purification of biodiesel produced by lipase catalysed transesterification by ultrafiltration: selection of membranes and analysis of membrane blocking mechanisms. Renew. Energy 159, 642–651. doi:10.1016/j.renene.2020.05.132
Stephenson, T., Brindle, K., Judd, S., and Jefferson, B. (2000). Membrane bioreactors for wastewater treatment. USA: IWA publishing. doi:10.2166/9781780402147
Su, A., Kiokekli, S., Naviwala, M., Shirke, A. N., Pavlidis, I. V., and Gross, R. A. (2020). Cutinases as stereoselective catalysts: specific activity and enantioselectivity of cutinases and lipases for menthol and its analogs. Enz. Microb. Technol. 133, 109467. doi:10.1016/j.enzmictec.2019.109467
Sun, T., Du, W., and Liu, D. (2011). Comparative study on stability of whole cells during biodiesel production in solvent-free system. Process Biochem. 46 (3), 661–664. doi:10.1016/j.procbio.2010.11.006
Surendhiran, D., Vijay, M., and Sirajunnisa, A. R. (2014). Biodiesel production from marine microalga Chlorella salina using whole cell yeast immobilized on sugarcane bagasse. J. Environ. Chem. Eng. 2 (3), 1294–1300. doi:10.1016/j.jece.2014.05.004
Tabañag, I. D. F., Chu, I. M., Wei, Y. H., and Tsai, S. L. (2018). The role of yeast-surface-display techniques in creating biocatalysts for consolidated bioprocessing. Catalysts 8 (3), 94. doi:10.3390/catal8030094
Talukder, M. M. R., Wu, J. C., and Chua, L. P. L. (2010). Conversion of waste cooking oil to biodiesel via enzymatic hydrolysis followed by chemical esterification. Energy fuels. 24 (3), 2016–2019. doi:10.1021/ef9011824
Tamalampudi, S., Talukder, M. R., Hama, S., Numata, T., Kondo, A., and Fukuda, H. (2008). Enzymatic production of biodiesel from Jatropha oil: a comparative study of immobilized-whole cell and commercial lipases as a biocatalyst. Biochem. Eng. J. 39, 185–189. doi:10.1016/j.bej.2007.09.002
Tan, T., Nie, K., and Wang, F. (2006). Production of biodiesel by immobilized Candida sp. lipase at high water content. Appl. Biochem. Biotech. 128 (2), 109–116. doi:10.1385/abab:128:2:109
Tan, Z., Chen, G., Chen, S., Zhang, J., Liu, J., Ma, X., et al. (2023). Engineering lipase at the molecular scale for cleaner biodiesel production – a review. Mol. Catal. 546, 113271. doi:10.1016/j.mcat.2023.113271
Tanaka, T., and Kondo, A. (2015). Cell surface engineering of industrial microorganisms for biorefining applications. Biotechnol. Adv. 33 (7), 1403–1411. doi:10.1016/j.biotechadv.2015.06.002
Teixeira, D. A., da Motta, C. R., Ribeiro, C. M. S., and de Castro, A. M. (2017). A rapid enzyme-catalyzed pretreatment of the acidic oil of macauba (Acrocomia aculeata) for chemoenzymatic biodiesel production. Process Biochem. 53, 188–193. doi:10.1016/j.procbio.2016.12.011
Teymennet-Ramírez, K. V., Martínez-Morales, F., and Trejo-Hernández, M. R. (2022). Yeast surface display system: strategies for improvement and biotechnological applications. Front. Bioeng. Biotechnol. 9, 794742. doi:10.3389/fbioe.2021.794742
Thaiyasuit, P., Pianthong, K., and Worapun, I. (2012). Acid esterification-alkaline transesterification process for methyl ester production from crude rubber seed oil. J. Oleo Sci. 61 (2), 81–88. doi:10.5650/jos.61.81
Toro, E. C., Rodríguez, D. F., Morales, N., García, L. M., and Godoy, C. A. (2019). Novel combi-lipase systems for fatty acid ethyl esters production. Catalysts 9 (6), 546. doi:10.3390/catal9060546
Ueda, M., and Tanaka, A. (2000). Genetic immobilization of proteins on the yeast cell surface. Biotechnol. Adv. 18 (2), 121–140. doi:10.1016/S0734-9750(00)00031-8
Vasić, K., Podrepšek, G. H., Knez, Ž., and Leitgeb, M. (2020). Biodiesel production using solid acid catalysts based on metal oxides. Catalysts 10 (2), 237. doi:10.3390/catal10020237
Venkatesagowda, B., Ponugupaty, E., Barbosa-Dekker, A. M., and Dekker, R. F. H. (2018). The purification and characterization of lipases from Lasiodiplodia theobromae, and their immobilization and use for biodiesel production from coconut oil. Appl. Biochem. Biotech. 185 (3), 619–640. doi:10.1007/s12010-017-2670-6
Vignesh, P., Pradeep Kumar, A. R., Ganesh, N. S., Jayaseelan, V., and Sudhakar, K. (2021). Biodiesel and green diesel generation: an overview. Oil Gas. Sci. Technol. 76, 6. doi:10.2516/ogst/2020088
Wachtmeister, J., and Rother, D. (2016). Recent advances in whole cell biocatalysis techniques bridging from investigative to industrial scale. Curr. Opin. Biotechnol. 42, 169–177. doi:10.1016/j.copbio.2016.05.005
Wang, Q., Liu, M., Ma, L., and Zhang, W. (2023). Co-immobilization of lipases with different specificities for efficient and recyclable biodiesel production from waste oils: optimization using response surface methodology. Int. J. Mol. Sci. 24 (5), 4726. doi:10.3390/ijms24054726
Wang, X., Qin, X., Li, D., Yang, B., and Wang, Y. (2017). One-step synthesis of high-yield biodiesel from waste cooking oils by a novel and highly methanol-tolerant immobilized lipase. Biores. Technol. 235, 18–24. doi:10.1016/j.biortech.2017.03.086
Weiland, P. (2010). Biogas production: current state and perspectives. Appl. Microbiol. Biot. 85 (4), 849–860. doi:10.1007/s00253-009-2246-7
Wijaya, H., Sasaki, K., Kahar, P., Quayson, E., Rachmadona, N., Amoah, J., et al. (2020). Concentration of lipase from Aspergillus oryzae expressing Fusarium heterosporum by nanofiltration to enhance transesterification. Processes 8 (4), 450. doi:10.3390/pr8040450
Xu, K., Chen, X., Zheng, R., and Zheng, Y. (2020). Immobilization of multi-enzymes on support materials for efficient biocatalysis. Front. Bioeng. Biotechnol. 8, 660. doi:10.3389/fbioe.2020.00660
Yan, J., Li, A., Xu, Y., Ngo, T. P. N., Phua, S., and Li, Z. (2012). Efficient production of biodiesel from waste grease: one-pot esterification and transesterification with tandem lipases. Biores. Technol. 123, 332–337. doi:10.1016/j.biortech.2012.07.103
Yan, J., Yan, Y., Madzak, C., and Han, B. (2017). Harnessing biodiesel-producing microbes: from genetic engineering of lipase to metabolic engineering of fatty acid biosynthetic pathway. Crit. Rev. Biotechnol. 37 (1), 26–36. doi:10.3109/07388551.2015.1104531
Yan, J., Zheng, X., Du, L., and Li, S. (2014). Integrated lipase production and in situ biodiesel synthesis in a recombinant Pichia pastoris yeast: an efficient dual biocatalytic system composed of cell free enzymes and whole cell catalysts. Biotechnol. Biofuels 7, 55. doi:10.1186/1754-6834-7-55
Yan, J., Zheng, X., and Li, S. (2014). A novel and robust recombinant Pichia pastoris yeast whole cell biocatalyst with intracellular overexpression of a Thermomyces lanuginosus lipase: preparation, characterization and application in biodiesel production. Biores. Technol. 151, 43–48. doi:10.1016/J.BIORTECH.2013.10.037
Yan, Y., Xu, L., and Dai, M. (2012). A synergetic whole-cell biocatalyst for biodiesel production. RSC Adv. 2 (15), 6170–6173. doi:10.1039/C2RA20974H
Yang, J., Huang, K., Xu, X., Miao, Y., Lin, Y., and Han, S. (2020). Cell surface display of Thermomyces lanuginosus lipase in Pichia pastoris. Front. Bioeng. Biotechnol. 8, 544058. doi:10.3389/FBIOE.2020.544058
Yang, X., Zhang, Y., Pang, H., Yuan, S., Wang, X., Hu, Z., et al. (2021). Codisplay of Rhizopus oryzae and Candida rugosa lipases for biodiesel production. Catalysts 11 (4), 421. doi:10.3390/catal11040421
Ye, M., Ye, Y., Du, Z., and Chen, G. (2021). Cell-surface engineering of yeasts for whole-cell biocatalysts. Bioprocess Biosyst. Eng. 44 (6), 1003–1019. doi:10.1007/S00449-020-02484-5
Yeom, S. H. (2016). Enhancing biodiesel production by immobilized whole cells by optimizing reaction conditions and adding glycerol and water. Biotechnol. Bioprocess Eng. 21 (2), 274–282. doi:10.1007/S12257-016-0046-3
Yücel, Y. (2011). Biodiesel production from pomace oil by using lipase immobilized onto olive pomace. Biores. Technol. 102 (4), 3977–3980. doi:10.1016/j.biortech.2010.12.001
Zhang, B., Weng, Y., Xu, H., and Mao, Z. (2012). Enzyme immobilization for biodiesel production. Appl. Microbiol. Biot. 93, 61–70. doi:10.1007/s00253-011-3672-x
Zhang, K., Jin, Z., Wang, P., Zheng, S. P., Han, S. Y., and Lin, Y. (2017). Improving the catalytic characteristics of lipase-displaying yeast cells by hydrophobic modification. Bioprocess Biosyst. Eng. 40 (11), 1689–1699. doi:10.1007/S00449-017-1824-9
Zhong, L., Feng, Y., Hu, H., Xu, J., Wang, Z., Du, Y., et al. (2021). Enhanced enzymatic performance of immobilized lipase on metal organic frameworks with superhydrophobic coating for biodiesel production. J. Colloid Interf. Sci. 602, 426–436. doi:10.1016/j.jcis.2021.06.017
Keywords: biodiesel, lipase, biocatalysis, whole-cell biocatalysts, membrane bioreactors, transesterification
Citation: Spanou A, Moschona A, Theodosiou E, Patsios SI and Pavlidis IV (2024) Novel concepts for the biocatalytic synthesis of second-generation biodiesel. Front. Catal. 4:1360702. doi: 10.3389/fctls.2024.1360702
Received: 23 December 2023; Accepted: 01 February 2024;
Published: 16 February 2024.
Edited by:
Frank Hollmann, Delft University of Technology, NetherlandsReviewed by:
José Cleiton Sousa dos Santos, University of International Integration of Afro-Brazilian Lusophony, BrazilCopyright © 2024 Spanou, Moschona, Theodosiou, Patsios and Pavlidis. This is an open-access article distributed under the terms of the Creative Commons Attribution License (CC BY). The use, distribution or reproduction in other forums is permitted, provided the original author(s) and the copyright owner(s) are credited and that the original publication in this journal is cited, in accordance with accepted academic practice. No use, distribution or reproduction is permitted which does not comply with these terms.
*Correspondence: Ioannis V. Pavlidis, aXBhdmxpZGlzQHVvYy5ncg==
Disclaimer: All claims expressed in this article are solely those of the authors and do not necessarily represent those of their affiliated organizations, or those of the publisher, the editors and the reviewers. Any product that may be evaluated in this article or claim that may be made by its manufacturer is not guaranteed or endorsed by the publisher.
Research integrity at Frontiers
Learn more about the work of our research integrity team to safeguard the quality of each article we publish.