- 1Department of Chemistry, University College London, London, United Kingdom
- 2Department of Drug Sciences, University of Pavia, Pavia, Italy
- 3FQPIMA Group Department of Chemical Engineering, Complutense University of Madrid, Madrid, Spain
- 4School of Biochemical Engineering, Pontificia Universidad Católica de Valparaíso, Valparaíso, Chile
- 5Department of Food, Environmental and Nutritional Sciences (DeFENS), University of Milan, Milan, Italy
Flow biocatalysis is a key enabling technology that is increasingly being applied to a wide array of reactions with the aim of achieving process intensification, better control of biotransformations, and minimization of waste stream. In this mini-review, selected applications of flow biocatalysis to the preparation of food ingredients, APIs and fat- and oil-derived commodity chemicals, covering the period 2020-2022, are described.
1 Introduction
The importance of flow chemistry, the transformation of iterative batch operations in continuous chemical processes as a component of the modern chemist toolbox, is reflected by the large number of publications and patents from both academia and industry over the past decade (Alper et al., 2019; Giroud et al., 2019; Kappe et al., 2019; Kinlen and Zweig, 2020; Griffiths and Ley, 2022; Polterauer et al., 2022; Gambacorta and Baxendale, 2022). Although flow chemistry was created for “conventional” organic chemistry reactions, this technique is being increasingly used also for biocatalytic processes due to the smaller equipment footprint, the increased safety for the operator, the better control of biotransformations while shortcutting reaction times and rendering scale-up more predictable (Tamborini et al., 2018; Benítez-Mateos et al., 2021).
Nowadays biocatalysis is recognized as an unparalleled tool to achieve reaction selectivity and sustainability, although low catalyst stability and productivity are still a sore point in many cases (Sheldon and Woodley, 2018; Hanefeld et al., 2022). Integration of enzymes within flow technology has been demonstrated to improve the biocatalyst performance and to generate highly productive biotransformations (Bolivar et al., 2019; Contente et al., 2019).
The use of enzymes in continuous reactors is associated to enzyme immobilization that streamlines both biocatalyst handling and product downstream. Suitable immobilization techniques generally enhance the stability of the biocatalyst under operational conditions, thus allowing for its repeated use and prolonging lifetime biocatalyst productivity to a high total turnover number (TTN) (Thompson et al., 2019; Bolivar and Lopez-Gallego, 2020; Bolivar et al., 2022). Downstream processing, which is generally recognized as a tricky step, is facilitated by reduced handling steps, reduced or negligible enzyme leaching (depending on the immobilization technique used), in situ product removal (that also reduces enzyme inhibition), and straightforward recovery and recycling of unreacted reagents. A key advantage of continuous flow is the overall alignment between analytical and preparative scale conditions, that shortens the optimization stage from discovery to process development. Last but not least, a number of smart solutions involving in-line devices based on phase separation, catch-and-release resins and scavengers, that are assisted by integrated real-time reaction monitoring, can be set-up.
On the other hand, the layout of flow systems overcomes some typical constraints of batch enzymatic reactions (long reaction times, catalyst concentration, scalability issues, risk of product variation from batch to batch). Reaction conversion can be increased by using several reactors in series, while process productivity can be enhanced by employing reactors in a parallel mode, or alternatively by simply allowing the system to work over time, without the need of proportionally increasing the reactor size and the biocatalyst amount (Britton et al., 2018; Tamborini et al., 2018; Benítez-Mateos et al., 2021; Meyer et al., 2022). Moreover, recent advancements include integration of flow systems with microwave (Martina et al., 2021), ultrasounds (Banakar et al., 2022), photocatalysis (Chanquia et al., 2022), and supported catalyst reactions (Colella et al., 2018).
The research and advances in continuous flow reactors are aiming at expanding the portfolio of reactions based on the aforementioned advantages, while tackling the challenges of these systems. Current challenges deal with the need of precise control and monitoring of some intensive variables (e.g., pH), fluid-dynamics for intensification of multi-phase reactions (e.g., gas-liquid-solid transformations), poorly soluble or insoluble substrates in the reaction medium, as well as the need of stabilized biocatalysts and a controlled operation under changes and perturbations that might occur in inflow streams. Biotransformations in continuous mode are developing rapidly: this mini-review aims at highlighting representative case-studies reported in 2020-2022 cutting across food, commodity and fine chemical sectors (Figure 1).
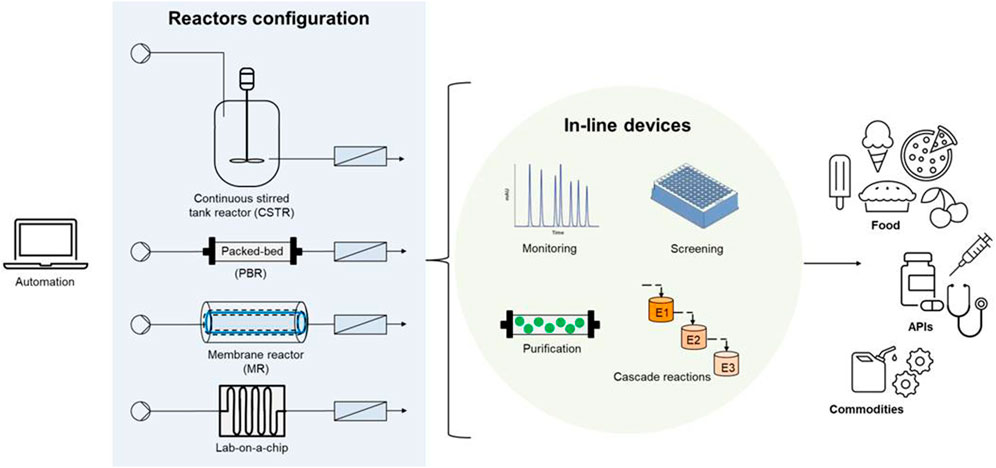
FIGURE 1. Continuous flow bioreactors at a glance: selected reactor layouts and applications discussed in the text.
2 Continuous reactors in a nutshell: Selected layouts and applications
Continuous or discontinuous operation is largely determined by the magnitude of the production task. For pharmaceutical and fine chemical production, batch is the customary mode of operation (Benítez-Mateos et al., 2021). In these cases, stirred tank reactors (STRs) are the most popular configuration at laboratory scale: their user-friendly design allows to quickly achieve the proof-of-concept of the reaction and enzyme characterization to appraise the potential for large-scale production. STRs can operate continuously in steady state (CSTRs); in this case the tank is instrumented with feed and exit pipes for reactant inlet and product outflow. They are generally considered versatile reactors in terms of operating conditions and ease of control of main operational variables. When (C)STRs are combined with immobilized biocatalysts, the mechanical stability of the enzyme carrier could be an issue since attrition can occur, thus resulting in biocatalyst leakage and/or low catalytic performance (Lutz, 2013).
Packed-bed reactors (PBRs) are probably the most used layouts for continuous processes since the biocatalyst is exposed to a lower shear stress. Moreover, PBRs offer clear advantages over CSTRs for enzymes following Michaelis-Menten or product-inhibition-type kinetics. This kinetic advantage applies both for mono-substrate and multi-substrate (e.g., synthesis reactions) conditions. Substrate enzyme inactivation or low solubility of the substrate can be overcome by multi-dose along the reactor (Gruber et al., 2017). The typical architecture of PBRs used for biocatalytic applications is such that a tube or channel contains the (bio)catalyst, and the solution of reactants flows through it, allowing the reaction to occur. In terms of reactor size, a significant part of the reactor volume (40%–60%) is occupied by the biocatalyst itself, while in CSTRs the biocatalyst usually accounts for less than 10% of the reactor volume. As a result, PBRs give higher conversion rates per reactor volume compared to other reactors (Lutz, 2013). PBRs are easily scalable and applied for large-scale continuous biotransformations in diverse industry sectors, from the well-known resolution of acyl- DL-amino acids employing the immobilized Aspergillus oryzae aminoacylase (Sato and Tosa, 2010), to the most recent production of biodiesel (Erdem and Woodley, 2022; Miotti et al., 2022) or fructose syrup (Neifar et al., 2020). Further recent applications include the continuous removal of urea in high polyphenol wines with an immobilized Lactobacillus fermentum acid urease to reduce ethyl carbamate formation (Fidaleo and Tavilli, 2021), and the hydrolysis of cellobiose with immobilized β-glucosidases (Alvarez-Gonzalez et al., 2022). Cellobiose, a β-1,4 glucose-based disaccharide, is used as a model-molecule in many studies aimed at finding solutions to the depolymerization of cellulose from renewable lignocellulosic biomass.
Photoreactor systems (PhRs) are another type of reactors which could be combined with biocatalysis under flow conditions (Chanquia et al., 2022; Masson et al., 2022). Many lab-scale PhRs are nowadays available from different suppliers; even though they are not explicitly meant for photobiocatalysis, they could be adapted. Reliable open-source systems (Winkler et al., 2021) as well as 3D-printed model rectors (Schiel et al., 2021) allowing for an easy exchange of reactor vessels and light sources are also available.
Membrane reactors (MRs) are also employed for continuous operations with enzymes. The integration of membranes in a biocatalytic reactor allows to keep the bulk of two reactants separated, thus preventing side reactions, or to selectively remove the products, circumventing thermodynamic equilibrium. MRs are an option for the conversion of large molecular size substrates; in this case, the membrane retains not only the enzyme, but also the unreacted starting material (Pottratz et al., 2022). Several factors are involved in the reactor selection and operation mode, being productivity (amount of product per unit of reactor volume and unit of time) and product-to-biocatalyst ratio (amount of product per unit of biocatalyst) the most important from an applicative standpoint (Grubecki and Kazimierska-Drobny, 2019; Carrazco-Escalante et al., 2021; Lindeque and Woodley, 2022).
The development of continuous flow reactors has been associated with the miniaturization and design of enzyme microreactors. Besides the advantages of reaction intensification, bioreactor downscaling into microfluidic devices (lab-on-a-chip) to perform chemical and biological transformations has the clear advantage of speeding up analysis and throughput, while reducing reagent and sample consumption. Enzymes can be, indeed, incorporated within a microchannel forming a microbioreactor (Brás et al., 2021; Cardoso Marques et al., 2021; Žnidaršič-Plazl, 2021).
Regardless of the reactor type, flow biocatalysis strongly relies on immobilized enzymes which are retained in the reactor while the substrate is continuously fed to be converted into product, thus allowing long-term or repeated use of the biocatalyst as a result of its higher operational stability and resistance to reaction changes. Moreover, the diversity of immobilization techniques gives rise to a vast number of bioreactor designs that can be customized and also connected together forming cascades (Benítez-Mateos et al., 2022b). In this context, implementation of cell-free multi-step reactions is a dream target in synthetic chemistry (Benítez-Mateos et al., 2022b). The space and time compartmentalization enabled by continuous flow reactors is a significant advantage for multi-enzymatic reactions. Aminations and redox reactions have received remarkable attention in this frame (Baumer et al., 2020; Menegatti and Žnidaršič-Plazl, 2021; Roura Padrosa et al., 2021).
Transaminases are a workhorse in the synthesis of enantiopure amines. The need for a continuous supply of the amino donor to shift the reaction equilibrium is routinely tackled by coupling an auxiliary reaction to the main biotransformation. In a recent work, an alanine dehydrogenase exhibiting excellent stability to different cosolvents has been combined with a formate dehydrogenase as L-alanine recycling system, in the amination of three model substrates with unfavorable equilibria. The whole biocatalytic system (transaminase and auxiliary enzymes) has been co-immobilized in a flow reactor (Roura Padrosa et al., 2021). In addition to the amino donor recycling, continuous flow reactors have been used for the retention of the pyridoxal phosphate cofactor by designing a porous copolymeric hydrogel matrix formed in a two-plate microreactor. Immobilization efficiency, productivity, and stability of the microreactor were evaluated (Menegatti and Žnidaršič-Plazl, 2021).
The need for cofactor regeneration in redox reactions has inspired different strategies for the “orchestration” of cascades involving the main enzyme(s), cofactors, and auxiliary proteins. In a recent work, a closed-loop recycling system for NADPH was developed. Although the strategy relies on the well-known in situ cofactor regeneration by the addition of a cosubstrate, the novelty stands in the recovery of aqueous outflow containing the cofactors and their recirculation into the system, allowing for self-sufficient bioreactors (Baumer et al., 2020).
Compartmentalization in continuous flow reactors needs also the development of new materials and immobilization strategies to “tailor” enzyme localization. For the trienzymatic synthesis of sialic acid, N-acyl-D-glucosamine 2-epimerase, N-acetylneuraminate lyase, and cytidine monophosphate (CMP)- sialic acid synthetase, were immobilized into bulk hydrogels and microstructured hydrogel-enzyme-dot arrays, which were then integrated into microfluidic devices. This study demonstrates how immobilizing enzymes in (compartmentalized) microfluidic devices can circumvent cross-inhibitions occurring under continuous conditions (Obst et al., 2021). Advances in the development of new controllable modular immobilization strategies were also reported. A modular cascade flow reactor using a generalizable solid-binding peptide-directed immobilization strategy was developed to allow the selective immobilization of fusion enzymes on anodic aluminum oxide monoliths with high positional precision (Yucesoy et al., 2021). Here, a lactate dehydrogenase and a formate dehydrogenase were fused with substrate-specific peptides to facilitate their self-immobilization through the membrane channels in a cascade geometry (Yucesoy et al., 2021). The development of multi-enzymatic systems also requires the fine tuning of the reaction conditions. Mathematical modeling was applied to meet this challenge by using both mechanistic and empirical tools to optimize a reaction involving a reductive aminase and a glucose dehydrogenase for continuous biocatalytic reductive amination in flow (Finnigan et al., 2020). Flow reactors offering the advantage of working in biphasic systems (e.g., water/organic solvents) are also a suitable venue for the implementation of chemoenzymatic cascades. A sequential as well as a tandem-type chemoenzymatic flow cascade combining an organocatalytic aldol reaction and a biocatalytic reduction to form stereoselectively a 1,3-diol with two stereogenic centers were developed (Schober et al., 2022).
3 Examples from literature (2020–2022)
An overview of the continuous systems for the biosynthesis of food-related compounds, active pharmaceutical ingredients (APIs) and their intermediates as well as fats and oil derivatives is reported in Table 1. Selected examples are described below in more detail.
3.1 Food-related compounds
In the last few years, flow biocatalysis has drawn interest in the preparation of food-related compounds such as additives, nutritional supplements and food preservatives. According to FDA and EMEA regulations, processing food ingredients via biocatalytic approaches let them to be commercialized as natural, thus improving their market value, while the use of flow facilities generally ensures a higher productivity with respect to conventional batch operations (Schrader et al., 2004; Gambacorta et al., 2021). Coupling biocatalysis with flow chemistry results in selective syntheses, enhancing the final product quality while reducing the process associated costs. For instance, the productivity of the enantiopure (R)-δ-decalactone via C-C double bond enzymatic reduction of the (R)-enantiomer of massoia lactone, drastically increased in flow. Under continuous mode, a 10 mM scale biotransformation was converted in 120 min residence time, while the same reaction in batch occurred with lower substrate loading and longer reaction time (3 mM, 4 h) (Szczepańska et al., 2021). Implementing flow biocatalytic systems often allows to improve the economic and environmental process efficiency by reducing enzyme inhibition, facilitating product isolation and enhancing biocatalyst stabilization and reuse (Bolivar and Lopez-Gallego, 2020; Cardoso Marques et al., 2021). As reported by Annunziata et al. (Annunziata et al., 2022), a panel of nature-inspired phenolic esters as antimicrobial food additives with enhanced lipophilicity was prepared by designing a flow process with the immobilized lipase Novozyme 435 in cyclopentyl methyl ether (CPME), a non-conventional green solvent. Similarly, a lipase from Candida rugosa (CrL) immobilized on silica packed microarrays, displayed 30 days of consistently high lipid-phytosterol ester production thanks to the improved biocatalyst stability (Xu et al., 2022). By exploiting a different immobilization strategy (cross-linked enzyme-adhered nanoparticles, CLEANs), the stability of the lipase B from Candida antarctica (CaLB) was maximized, thus allowing the process intensification for the production of terpene acetate fruit flavors and aromas (Nagy et al., 2022). Another continuous lipase-mediated process was developed for the interesterification of palm olein, an important modification for the triacylglycerol industrial usability (Zhang et al., 2022). A major breakthrough for the production of flavor-esters (20%–93% conversion) was obtained utilizing an acyltransferase from Mycobacterium smegmatis (MsAcT) in a biphasic medium with naturally occurring substrates in high concentration (125 mM) and 5 min residence time (Contente et al., 2020) (Supplementary Figure S1A). MsAcT was subsequently employed in pure toluene for the continuous preparation of vanillamides as new nature-inspired antimicrobials against foodborne pathogens (Pinna et al., 2022) (Supplementary Figure S1B). Several examples of food-applied flow biocatalysis regard the use of sugar modifying enzymes. Liu and coworkers co-immobilized the sugar nucleotide-dependent C-glycosyltransferase (CGT) with the sucrose synthase (SuSy) for the continuous production of the natural antioxidant C-glycoside nothofagin and the in situ regeneration of the expensive cosubstrate UDP-glucose (Liu and Nidetzky, 2021). A similar approach was employed to produce the non-reducing disaccharide trehalose by using monolithic microreactors (Kowalczykiewicz et al., 2022). A continuous bi-enzymatic cascade was set up by connecting two reactors in series, the UDP-glucose pyrophosphorylase (TaGalU) and the trehalose transferase (TuTreT), leading to a space-time-yield of 49.6 gproduct⋅L-1⋅h-1⋅mgprotein−1 and an excellent enzymatic operational stability (100 h). Bolivar and coworkers recently reported on a sustainable cellobiose hydrolysis. By designing a glucosidase-based reactor using glyoxyl-agarose for immobilization, the full conversion (34 g L-1) was achieved in 20 min residence time (Alvarez-Gonzalez et al., 2022). An engineered variant of the hydrolytic enzyme β-N-acetyl-hexosaminidase from Bifidobacterium bifidum was employed to produce the precious oligosaccharide lacto-N-triose II, a component of human milk oligosaccharides used as a synthon for nutritional supplements, providing a further example of the synergistic use of protein and reaction engineering for synthetic purposes (Ruzic et al., 2020).
3.2 Active pharmaceutical ingredients
Most of the literature covering 2020–2022 deal with the preparation of key building blocks (Al-Shameri et al., 2020; Böhmer et al., 2020; Hegarty and Paradisi, 2020; Roura Padrosa et al., 2020; Oeggl et al., 2021; Semproli et al., 2020; Teepakorn et al., 2021; Azevedo et al., 2022; Peng et al., 2022) for fine chemicals/APIs rather than with the total synthesis of drugs (Rinaldi et al., 2020; Tamborini et al., 2020; Wu et al., 2020; Kazan et al., 2021; Romero-Fernandez and Paradisi, 2021; Benítez-Mateos et al., 2022a; Benítez-Mateos and Paradisi, 2022). Interestingly, nucleoside analogues result the most reported examples in this framework (Rinaldi et al., 2020; Tamborini et al., 2020; Benítez-Mateos et al., 2022a; Benítez-Mateos and Paradisi, 2022) and the analysis of this trend was thus reported herein. Nucleoside analogues have been used since the 70s as anticancer and antiviral agents (De Clercq and Li, 2016). Owing to their chemical similarity with natural nucleosides, these molecules are able to impair DNA/RNA synthesis by inhibiting cellular or viral replication. Nucleoside-based drugs are strongly back in the game in the past 3 years; besides vaccine development (Szabó et al., 2022), the urgent need for quickly controlling the SARS-CoV-2 outbreak was tackled through repurposing already approved antiviral drugs (Kumar et al., 2021). Remdesivir (GS-5734), which was originally developed for the treatment of Ebola infection, and molnupiravir stand out in this scenario (Beigel et al., 2020; Bernal et al., 2022). Repurposing approaches of approved drugs were a need to speed up clinical translation of hopefully promising candidates (Chitalia and Munawar, 2020; Al-Karmalawy et al., 2021; De et al., 2021; Schultz et al., 2022), while novel technologies to access new drugs and to improve established drug processes were boosted at the same time.
The recent developments in biocatalytic routes to nucleoside analogues (Huffman et al., 2019; McIntosh et al., 2021; Burke et al., 2022), nicely reviewed by Cosgrove and Miller (Cosgrove and Miller, 2022) and by Westarp et al. (Westarp et al., 2022), and the demand for natural and modified nucleotides for mRNA vaccines have given a new impetus to nucleic-acid chemistry. Flow (bio)catalysis, which is being rapidly spreading for both bioprocess development and optimization in the pharma sector (Hughes, 2018), was indeed the next step. Four pharmaceutically relevant 5-halogenated-2’-deoxyuridine nucleoside analogues (fluoro-, chloro-, bromo-, and iodo-) were synthesized at 10 mM scale by a thymidine phosphorylase from Halomonas elongata (HeTP) immobilized on methacrylate microbeads achieving conversions >80% within 45 min residence time. The same reaction in batch gave 67%–84% conversions in 24 h (Supplementary Figure S2A). The synthesis of floxuridine (5-fluoro-2’-deoxyuridine) was further scaled-up to 20 mM obtaining the highest space-time yield (5.5 g/L/h) reported to date (Benítez-Mateos et al., 2022a). In the paper by Rinaldi et al., an immobilized enzyme reactor based on Lactobacillus reuteri 2’-deoxyribosyltransferase (LrNDT) was employed for the synthesis of 5-fluoro- and 5-iodo-2’-deoxyuridine at an analytical scale, affording 50%–59% conversion after 30 min residence time, respectively (Rinaldi et al., 2020) (Supplementary Figure S2B). The purine nucleoside phosphorylase from H. elongata (HePNP) immobilized on agarose microbeads was employed by Benitez-Mateos and Paradisi for the flow synthesis of a panel of nucleoside analogues with bulky nucleobases (6-O-methylguanine, 1,2,4-triazole-3-carboxamide, benzimidazole, aniline-purine, and benzamidepurine). A conversion >90% was obtained after 10 min residence time in the transglycosylation of inosine with 6-O-methylguanine, for the synthesis of a nucleoside analogue of the anticancer drug nelarabine, while modest conversions (<20%) were achieved with the other nucleobases tested (Benítez-Mateos and Paradisi, 2022). A uridine phosphorylase from Clostridium perfringens (CpUP) and a purine nucleoside phosphorylase from Aeromonas hydrophila (AhPNP) were co-immobilized in flow on glyoxyl-agarose (Supplementary Figure S2C) and used in a cascade mode for the preparative synthesis of vidarabine: about 1 g of the target product (55% isolated yield, >99% purity) was obtained after 1 week (Tamborini et al., 2020).
3.3 Commodity chemicals from fats and oils
Lipids are a valuable renewable source for the sustainable manufacturing of multiple chemicals such as biolubricants, biosurfactants, and biofuels. Hydrolysis, esterification, interesterification, and decarboxylation are the main target reactions involved. Continuous bioreactors are a useful tool both for the study and the intensification of biotransformations. Lipases are the “first in class” enzymes in this frame and are generally used as immobilized biocatalysts in PBRs. A recent report described the synthesis of a biolubricant from isoamyl alcohol, contained in fusel oil (>60% wt), and oleic acid (1: 1.5 M ratio) catalyzed by Rhizopus oryzae lipase and performed in a two-stage PBR coupled to a water extraction column filled with molecular sieves. Under optimized conditions, ester productivity was 292.20 μmol g−1 min−1 and biocatalyst stability (t1/2) was 179.6 h (Vilas Bôas et al., 2021) (Supplementary Figure S3). In another report, palm oil was interesterified in a fixed-bed reactor: flow rate and temperature were studied as key parameters controlling the interesterification degree (Zhang et al., 2022). Decarboxylation of fatty acids to the corresponding hydrocarbons (Cn-1) was carried out to produce biofuels. Compared to lipase-catalyzed reactions, photodecarboxylase-based reactions are in an early phase. Continuous flow reactors have been used for the study of the reactions and assessment of photostability and suitable light penetration (Benincá et al., 2022; Simić et al., 2022). The full conversion of palmitic acid in the photodecarboxylation catalyzed by Chlorella variabilis fatty acid photodecarboxylase was obtained in a rapid residence time (15 min) by using a high power blue LED source (300 W). Furthermore, the use of less expensive and sustainable light sources such as common white LED source (300 W) or even sunlight were evaluated, achieving full conversions after 1 h.
4 Conclusion and outlook
Continuous processes merging the advantages of flow reactors, the inherent selectivity of enzymes, and the access to heterogeneous and stable biocatalysts as a result of their compartmentalization by immobilization complement nowadays the classical round-bottom flask batch chemistry. Furthermore, automation, less space and easy scale-up, better mixing, more efficient heat transfer are only a few of the technical strength points of continuous processes which translate in better control over reaction conditions by real-time monitoring as well as of product variability, less energy consumption, higher safety and productivity. Thus, it is not surprising that this enabling technology answers all the Green Chemistry principles. In this context, flow biocatalysis has proved to tackle some of the main challenges of start-and-stop batch biotransformations. Scalability of biotransformations is still far from the industrial requirements and is rarely coupled with industrially feasible and straightforward downstream processes (that are high product concentration and product recovery by precipitation/crystallization over extraction or chromatographic steps). Biocatalyst turnover and reusability are further issues that have to be assessed when developing bioprocesses. Therefore, biocatalyst stabilization by immobilization plays a key role in this regard. When approaching flow mode operations with equilibrium-controlled reactions, the use of an excess of reactants is generally avoided (to minimize waste formation and to assist the purification step), while taking advantage of the progressive removal of the substrate/product. For cofactor-dependent enzymes, recycling systems in flow (e.g., enzyme and cofactor co-immobilization strategies) need to be designed for efficient processes: this is an issue that it is expected to catalyze much effort in the next future.
The numerous advantages of continuous flow processes have favored the application of this technology, initially confined to continuous bulk production, also to the fine chemicals sector. Taking into account the increasing demand for a safer, less-energy demanding, and sustainable chemistry, it is foreseen that flow biocatalysis will play a major role in this frame. Nevertheless, the higher complexity of flow compared to batch processes as well as time and cost investments required to switch from batch to flow are the other side of the coin. Continuous set-ups do not outperform traditional batch techniques by definition. Hence, a batch versus flow assessment and a bioprocess design should be done first to figure out whether a “traditional” biotransformation can really benefit from continuous technology. Systematic analysis and comparison of reactor formats should be accompanied with standardized metrics of both catalyst and reactor performance (Thompson et al., 2019; Bolivar and Lopez-Gallego, 2020). Metrics assessing reaction performance should be ideally accompanied with the analysis of product quality, process cost-effectiveness and energy efficiency to assist optimal process design and reactor implementation (Cardoso Marques et al., 2021).
Author contributions
Conceptualization: MLC, JMB, and DU; Writing—original draft: MC and MSR; Writing—Review and Editing: all authors; Supervision and Data curation: MLC. All authors contributed to the article and approved the submitted version.
Acknowledgments
MSR and DU thank Cariplo Foundation (Italy) (call: “Circular Economy for a sustainable future 2020, BioSurf, ID 2020-1094”). JMB acknowledges funding from the Government of Community of Madrid, Spain (2018-T1/BIO-10200). JMB and LW acknowledge funding from “Attraction of Advanced Human from Foreigners” Project MEC 80180020- PCI-ANID (Chile). MLC acknowledges funding from University of Milan (Piano di Sostegno UNIMI Linea 2–2021) (Italy) through the project W-BioFlow “From agro-food waste to valuable bioactives through flow biocatalysis”.
Conflict of interest
The authors declare that the research was conducted in the absence of any commercial or financial relationships that could be construed as a potential conflict of interest.
Publisher’s note
All claims expressed in this article are solely those of the authors and do not necessarily represent those of their affiliated organizations, or those of the publisher, the editors and the reviewers. Any product that may be evaluated in this article, or claim that may be made by its manufacturer, is not guaranteed or endorsed by the publisher.
Supplementary material
The Supplementary Material for this article can be found online at: https://www.frontiersin.org/articles/10.3389/fctls.2023.1154452/full#supplementary-material
References
Al-Karmalawy, A. A., Soltane, R., Elmaaty, A. A., Tantawy, M. A., Antar, S. A., Yahya, G., et al. (2021). Coronavirus disease (COVID-19) control between drug repurposing and vaccination: A comprehensive overview. Vaccines 9, 1317. doi:10.3390/vaccines9111317
Al-Shameri, A., Petrich, M.-C., Puring, K. U. P., Nestl, B. M., and Lauterbach, L. (2020). Powering artificial enzymatic cascades with electrical energy. Angew. Chem. Int. Ed. 59, 10929–10933. doi:10.1002/anie.202001302
Alper, P., Deane, J., Jiang, S., Jiang, T., Knoepfel, T., Michellys, P. Y., et al. (2019). Compounds and compositions as inhibitors of endosomal toll-like receptors. U.S. Patent Application, US20190211009A1, Novartis Institutes for Biomedical Research Inc, Cambridge, MA 02139, USA
Alvarez-Gonzalez, C., Santos, V. E., Ladero, M., and Bolivar, J. M. (2022). Immobilization-stabilization of β-glucosidase for implementation of intensified hydrolysis of cellobiose in continuous flow reactors. Catalysts 12, 80. doi:10.3390/catal12010080
Annunziata, F., Contente, M. L., Anzi, V., Donzella, S., Conti, P., Molinari, F., et al. (2022). Enzymatic continuous-flow preparation of nature-inspired phenolic esters as antiradical and antimicrobial agents. Food Chem. 390, 133195. doi:10.1016/j.foodchem.2022.133195
Annunziata, F., Contente, M. L., Betti, D., Pinna, C., Molinari, F., Tamborini, L., et al. (2020). Efficient chemo-enzymatic flow synthesis of high value amides and esters. Catalysts 10, 939. doi:10.3390/catal10080939
Azevedo, L. S. D., Aguillon, A. R., Lima, M. T., Leão, R. A. C., and de Souza, R. O. M. A. (2022). Continuous flow synthesis of the lamivudine precursor L-menthyl glyoxylate. J. Flow. Chem. 12, 59–69. doi:10.1007/s41981-021-00189-8
Banakar, V. V., Sabnis, S. S., Gogate, P. R., Raha, A., and Saurabh, (2022). Ultrasound assisted continuous processing in microreactors with focus on crystallization and chemical synthesis-A critical review. Chem. Eng. Res. Des. 182, 273–289. doi:10.1016/j.cherd.2022.03.049
Baumer, B., Classen, T., Pohl, M., and Pietruszka, J. (2020). Efficient nicotinamide adenine dinucleotide phosphate [NADP(H)] recycling in closed-loop continuous flow biocatalysis. Adv. Synth. Catal. 362, 2894–2901. doi:10.1002/adsc.202000058
Beigel, J. H., Tomashek, K. M., Dodd, L. E., Mehta, A. K., Zingman, B. S., Kalil, A. C., et al. (2020). Remdesivir for the treatment of covid-19 — final report. N. Engl. J. Med. 383, 1813–1826. doi:10.1056/nejmoa2007764
Benincá, L. A. D., França, A. S., Breda, G. C., Leao, R. A. C., Almeida, R. V., Hollmann, F., et al. (2022). Continuous-flow CvFAP photodecarboxylation of palmitic acid under environmentally friendly conditions. Mol. Catal. 528, 112469. doi:10.1016/j.mcat.2022.112469
Benítez-Mateos, A. I., Contente, M. L., Roura Padrosa, D., and Paradisi, F. (2021). Flow biocatalysis 101: Design, development and applications. React. Chem. Eng. 6, 599–611. doi:10.1039/d0re00483a
Benítez-Mateos, A. I., Klein, C., Roura Padrosa, D., and Paradisi, F. (2022a). A novel thymidine phosphorylase to synthesize (halogenated) anticancer and antiviral nucleoside drugs in continuous flow. Catal. Sci. Technol. 12, 6231–6238. doi:10.1039/d2cy00751g
Benítez-Mateos, A. I., and Paradisi, F. (2022). Sustainable flow-synthesis of (bulky) nucleoside drugs by a novel and highly stable nucleoside phosphorylase immobilized on reusable supports. ChemSusChem 15, e202102030. doi:10.1002/cssc.202102030
Benítez-Mateos, A. I., Roura Padrosa, D., and Paradisi, F. (2022b). Multistep enzyme cascades as a route towards green and sustainable pharmaceutical syntheses. Nat. Chem. 14, 489–499. doi:10.1038/s41557-022-00931-2
Bernal, A. J., Gomes da Silva, M. M., Musungaie, D. B., Kovalchuk, E., Gonzalez, A., Delos Reyes, V., et al. (2022). Molnupiravir for oral treatment of Covid-19 in nonhospitalized patients. N. Engl. J. Med. 386, 509–520. doi:10.1056/nejmoa2116044
Böhmer, W., Volkov, A., Cassimjee, K. E., and Mutti, F. G. (2020). Continuous flow bioamination of ketones in organic solvents at controlled water activity using immobilized ω-transaminases. Adv. Synth. Catal. 362, 1858–1867. doi:10.1002/adsc.201901274
Bolivar, J. M., and Lopez-Gallego, F. (2020). Characterization and evaluation of immobilized enzymes for applications in flow reactors. Curr. Opin. Green Sustain. Chem. 25, 100349. doi:10.1016/j.cogsc.2020.04.010
Bolivar, J. M., Valikhani, D., and Nidetzky, B. (2019). Demystifying the flow: Biocatalytic reaction intensification in microstructured enzyme reactors. Biotechnol. J. 14, 1800244. doi:10.1002/biot.201800244
Bolivar, J. M., Woodley, J. M., and Fernandez-Lafuente, R. (2022). Is enzyme immobilization a mature discipline? Some critical considerations to capitalize on the benefits of immobilization. Chem. Soc. Rev. 51, 6251–6290. doi:10.1039/d2cs00083k
Brás, E. J. S., Chu, V., Conde, J. P., and Fernandes, P. (2021). Recent developments in microreactor technology for biocatalysis applications. React. Chem. Eng. 6, 815–827. doi:10.1039/d1re00024a
Britton, J., Majumdar, S., and Weiss, G. A. (2018). Continuous flow biocatalysis. Chem. Soc. Rev. 47, 5891–5918. doi:10.1039/c7cs00906b
Burke, A. J., Birmingham, W. R., Zhuo, Y., Thorpe, T. W., Zucoloto da Costa, B., Crawshaw, R., et al. (2022). An engineered cytidine deaminase for biocatalytic production of a key intermediate of the Covid-19 antiviral Molnupiravir. J. Am. Chem. Soc. 144, 3761–3765. doi:10.1021/jacs.1c11048
Cardoso Marques, M. P., Lorente-Arevalo, A., Bolivar, J. M., Bahnemann, J., and Grünberger, A. (2021). “Biocatalysis in continuous-flow microfluidic reactors,” in Microfuidics in biotechnology advances in biochemical engineering/biotechnology (Berlin, Germany: Springer).
Carrazco-Escalante, M., Hernandez-Calderon, O., Iribe-Salazar, R., Vasquez-Lopez, Y., Rios-Iribe, E., Alarid-Garcia, C., et al. (2021). Modeling glucose isomerization in a packed bed reactor using a new approach to the Briggs-Haldane mechanism. Rev. Mex. Ing. Quím. 20, 1–30. doi:10.24275/rmiq/cat2474
Chanquia, S. N., Valotta, A., Gruber-Woel, H., and Kara, S. (2022). Photobiocatalysis in continuous flow. Front. Catal. 1, 816538.
Chitalia, V. C., and Munawar, A. H. (2020). A painful lesson from the COVID-19 pandemic: The need for broad-spectrum, host-directed antivirals. J. Transl. Med. 18, 390. doi:10.1186/s12967-020-02476-9
Colella, M., Carlucci, C., and Luisi, R. (2018). Supported catalysts for continuous flow synthesis. Top. Curr. Chem. 376, 46. doi:10.1007/s41061-018-0225-0
Contente, M. L., Farris, S., Tamborini, L., Molinari, F., and Paradisi, F. (2019). Flow-based enzymatic synthesis of melatonin and other high value tryptamine derivatives: A five-minute intensified process. Green Chem. 21, 3263–3266. doi:10.1039/c9gc01374a
Contente, M. L., Tamborini, L., Molinari, F., and Paradisi, F. (2020). Aromas flow: Eco-friendly, continuous, and scalable preparation of flavour esters. J. Flow. Chem. 10, 235–240. doi:10.1007/s41981-019-00063-8
Cosgrove, S. C., and Miller, G. J. (2022). Advances in biocatalytic and chemoenzymatic synthesis of nucleoside analogues. Expert Opin. Drug Discov. 17, 355–364. doi:10.1080/17460441.2022.2039620
De Clercq, E., and Li, G. (2016). Approved antiviral drugs over the past 50 years. Clin. Microbiol. Rev. 29, 695–747. doi:10.1128/cmr.00102-15
De, P., Chakraborty, I., Karna, B., and Mazumder, N. (2021). Brief review on repurposed drugs and vaccines for possible treatment of COVID-19. Eur. J. Pharmacol. 898, 173977. doi:10.1016/j.ejphar.2021.173977
Erdem, E., and Woodley, J. M. (2022). Industrially useful enzymology: Translating biocatalysis from laboratory to process. Chem. Catal. 2, 2499–2505. doi:10.1016/j.checat.2022.09.037
do Nascimento, M. A., Vargas, J. P. C., Rodrigues, J. G. A., Leao, R. A. C., de Moura, P. H. B., et al. (2022). Lipase-catalyzed acylation of levoglucosan in continuous flow: Antibacterial and biosurfactant studies. RSC Adv. 12, 3027–3035. doi:10.1039/d1ra08111j
Fidaleo, M., and Tavilli, E. (2021). Urea removal in rosé and red wines by immobilised acid urease in a packed bed reactor. Food Bioprod. process. 126, 42–50. doi:10.1016/j.fbp.2020.12.008
Finnigan, W., Citoler, J., Cosgrove, S. C., and Turner, N. J. (2020). Rapid model-based optimization of a two-enzyme system for continuous reductive amination in flow. Org. Process Res. Dev. 24, 1969–1977. doi:10.1021/acs.oprd.0c00075
Gambacorta, G., and Baxendale, I. R. (2022). Continuous-flow Hofmann rearrangement using trichloroisocyanuric acid for the preparation of 2-benzoxazolinone. Org. Process Res. Dev. 26 (2), 422–430. doi:10.1021/acs.oprd.1c00440
Gambacorta, G., Sharley, J. S., and Baxendale, I. R. (2021). A comprehensive review of flow chemistry techniques tailored to the flavours and fragrances industries. Beilstein J. Org. Chem. 17, 1181–1312. doi:10.3762/bjoc.17.90
Giroud, M., Haap, W., Kuhn, B., and Martin, R. E. (2019). Trypanosomes inhibitors. U.S. Patent Application US10377719 B2. Hoffmann-La Roche Inc.
Gkantzou, E., Govatsi, K., Chatzikonstantinou, A. V., Yannopoulos, S. N., and Stamatis, H. (2021). Development of a ZnO nanowire continuous flow microreactor with β-glucosidase activity: Characterization and application for the glycosylation of natural products. ACS Sustain. Chem. Eng. 9, 7658–7667. doi:10.1021/acssuschemeng.1c02557
Griffiths, O. M., and Ley, S. V. (2022). Multicomponent direct assembly of N-heterospirocycles facilitated by visible-light-driven photocatalysis. J. Org. Chem. 87, 13204–13223. doi:10.1021/acs.joc.2c01684
Grubecki, I., and Kazimierska-Drobny, K. (2019). Prediction of the fixed-bed reactor behavior for biotransformation with parallel enzyme deactivation using dispersion model: A case study on hydrogen peroxide decomposition by commercial catalase. Pol. J. Chem. Technol. 21, 106–115. doi:10.2478/pjct-2019-0046
Gruber, P., Marques, M. P. C., O’Sullivan, B., Baganz, F., Wohlgemuth, R., and Szita, N. (2017). Conscious coupling: The challenges and opportunities of cascading enzymatic microreactors. Biotechnol. J. 12, 1700030. doi:10.1002/biot.201700030
Hanefeld, U., Hollmann, F., and Paul, C. E. (2022). Biocatalysis making waves in organic chemistry. Chem. Soc. Rev. 51, 594–627. doi:10.1039/d1cs00100k
Hegarty, E., and Paradisi, F. (2020). Implementation of biocatalysis in continuous flow for the synthesis of small cyclic amines. Chimia 74, 890–894. doi:10.2533/chimia.2020.890
Huffman, M. A., Fryszkowska, A., Alvizo, O., Borra-Garske, M., Campos, K. R., Canada, K. A., et al. (2019). Design of an in vitro biocatalytic cascade for the manufacture of Islatravir. Science 366, 1255–1259. doi:10.1126/science.aay8484
Hughes, D. L. (2018). Applications of flow chemistry in drug development: Highlights of recent patent literature. Org. Process Res. Dev. 22, 13–20. doi:10.1021/acs.oprd.7b00363
Jorge, J. M., de, M., Silva, M. V., Brêda, G. C., de Souza, C. P., Leão, R. A. C., et al. (2022). α-Ketobutyrate production under continuous-flow conditions catalyzed by immobilized L-methionine γ-lyase. Eur. J. Org. Chem., e202200579.
Kappe, C. O., Gutmann, B., Weigl, U., Egli, P., Cox, D. P., and Cantillo, D. (2019). Processes and oxazolidine-containing intermediates for the preparation of morphine analogs and derivatives. US20190127389A, Noramco Inc, Athens, GA, USA
Kazan, A., Hu, X., Stahl, A., Frerichs, H., Smirnova, I., and Yesil-Celiktas, O. (2021). An enzyme immobilized microreactor for continuous-flow biocatalysis of ginsenoside Rb1. J. Chem. Technol. Biotechnol. 96, 3349–3357. doi:10.1002/jctb.6887
Kinlen, P. J., and Zweig, A. M. (2020). Flow reactor synthesis of polymers. Progress in Polymer Science, 107, doi:10.1016/j.progpolymsci.2020.101256
Klein, E., Weiler, J., Wagner, M., Čelikić, M., Niemeyer, C. M., Horn, H., et al. (2022). Enrichment of phosphate-accumulating organisms (PAOs) in a microfluidic model biofilm system by mimicking a typical aerobic granular sludge feast/famine regime. Appl. Microbiol. Biotechnol. 106, 1313–1324. doi:10.1007/s00253-022-11759-8
Kowalczykiewicz, D., Przypis, M., Mestrom, L., Kumpf, A., Tischler, D., Hagedoorn, P.-L., et al. (2022). Engineering of continuous bienzymatic cascade process using monolithic microreactors – in flow synthesis of trehalose. Chem. Eng. J. 427, 131439. doi:10.1016/j.cej.2021.131439
Kruschitz, A., Peinsipp, L., Pfeiffer, M., and Nidetzky, B. (2021). Continuous process technology for glucoside production from sucrose using a whole cell-derived solid catalyst of sucrose phosphorylase. Appl. Microbiol. Biotechnol. 105, 5383–5394. doi:10.1007/s00253-021-11411-x
Kumar, S., Caliskan, D. M., Janowski, J., Faist, A., Gisela Conrad, B. C., Lange, J., et al. (2021). Beyond vaccines: Clinical status of prospective COVID-19 therapeutics. Front. Immunol. 12, 752227. doi:10.3389/fimmu.2021.752227
Lindeque, R. M., and Woodley, J. M. (2022). Modeling and experimental validation of continuous biocatalytic oxidation in two continuous stirred tank reactors in series. Org. Process Res. Dev. 26, 2030–2037. doi:10.1021/acs.oprd.1c00429
Liu, H., and Nidetzky, B. (2021). Leloir glycosyltransferases enabled to flow synthesis: Continuous production of the natural C-glycoside nothofagin. Biotechnol. Bioeng. 118, 4402–4413. doi:10.1002/bit.27908
Maheswari, R. U., Thirugnanasambantham, K., Mondal, A., Halder, G., and Sikder, J. (2022). Enzymatic hydrolysis of structurally upgraded lignocellulosic biomass with the aid of humic acid: A case study in a membrane integrated bioreactor. Environ. Dev. Sustain.doi:10.1007/s10668-022-02233-6
Martina, K., Cravotto, G., and Varma, R. S. (2021). Impact of microwaves on organic synthesis and strategies toward flow processes and scaling up. J. Org. Chem. 86, 13857–13872. doi:10.1021/acs.joc.1c00865
Masson, T. M., Zondag, S. D. A., Debije, M. G., and Noël, T. (2022). Rapid and replaceable luminescent coating for silicon-based microreactors enabling energy-efficient solar photochemistry. ACS Sustain. Chem. Eng. 10, 10712–10717. doi:10.1021/acssuschemeng.2c03390
McIntosh, J. A., Benkovics, T., Silverman, S. M., Huffman, M. A., Kong, J., Maligres, P. E., et al. (2021). Engineered ribosyl-1-kinase enables concise synthesis of Molnupiravir, an antiviral for COVID-19. ACS Cent. Sci. 7, 1980–1985. doi:10.1021/acscentsci.1c00608
Menegatti, T., and Žnidaršič-Plazl, P. (2021). Hydrogel-based enzyme and cofactor co-immobilization for efficient continuous transamination in a microbioreactor. Front. Bioeng. Biotechnol. 9, 752064. doi:10.3389/fbioe.2021.752064
Meyer, L.-E., Hobisch, M., and Kara, S. (2022). Process intensification in continuous flow biocatalysis by up and downstream processing strategies. Curr. Opin. Biotechnol. 78, 102835. doi:10.1016/j.copbio.2022.102835
Miotti, R. H. J., Cortez, D. V., and de Castro, H. F. (2022). Transesterification of palm kernel oil with ethanol catalyzed by a combination of immobilized lipases with different specificities in continuous two-stage packed-bed reactor. Fuel 310, 122343. doi:10.1016/j.fuel.2021.122343
Nagy, F., Sánta-Bell, E., Jipa, M., Hornyánszky, G., Szilágyi, A., László, K., et al. (2022). Cross-linked enzyme-adhered nanoparticles (CLEANs) for continuous-flow bioproduction. ChemSusChem 15, e202102284. doi:10.1002/cssc.202102284
Neifar, S., Cervantes, F. V., Bouanane-Darenfed, A., BenHlima, H., Ballesteros, A. O., Plou, F. J., et al. (2020). Immobilization of the glucose isomerase from Caldicoprobacter algeriensis on Sepabeads EC-HA and its efficient application in continuous high fructose syrup production using packed bed reactor. Food Chem. 309, 125710. doi:10.1016/j.foodchem.2019.125710
Obst, F., Mertz, M., Mehner, P. J., Beck, A., Castiglione, K., Richter, A., et al. (2021). Enzymatic synthesis of sialic acids in microfluidics to overcome cross-inhibitions and substrate supply limitations. ACS Appl. Mat. Interfaces 13, 49433–49444. doi:10.1021/acsami.1c12307
Oeggl, R., Glaser, J., von Lieres, E., and Rother, D. (2021). Continuous enzymatic stirred tank reactor cascade with unconventional medium yielding high concentrations of (S)-2-hydroxyphenyl propanone and its derivatives. Catal. Sci. Technol. 11, 7886–7897. doi:10.1039/d0cy01666g
Palma, B. G., Leao, R. A. C., de Souza, R. O. M. A., and Pandoli, O. G. (2021). Immobilization of lipases on lignocellulosic bamboo powder for biocatalytic transformations in batch and continuous flow. Catal. Today 381, 280–287. doi:10.1016/j.cattod.2020.04.041
Peng, M., Siebert, D. L., Engqvist, M. K. M., Niemeyer, C. M., and Rabe, K. S. (2022). Modeling-assisted design of thermostable benzaldehyde lyases from Rhodococcus erythropolis for continuous production of α-hydroxy ketones. ChemBioChem 23, e202100468. doi:10.1002/cbic.202100468
Pinna, C., Martino, P. A., Meroni, G., Sora, V. M., Tamborini, L., Dallavalle, S., et al. (2022). Biocatalyzed synthesis of vanillamides and evaluation of their antimicrobial activity. J. Agric. Food Chem. 70, 223–228. doi:10.1021/acs.jafc.1c06213
Polterauer, D., Roberge, D. M., Hanselmann, P., Littich, R., Hone, C. A., and Kappe, C. O. (2022). A continuous flow investigation of sulfonyl chloride synthesis using N-chloroamides: Optimization, kinetics and mechanism. React. Chem. Eng. 7, 2582–2592. doi:10.1039/d2re00280a
Pottratz, I., Müller, I., and Hamel, C. (2022). Potential and scale-up of pore-through-flow membrane reactors for the production of prebiotic galacto-oligosaccharides with immobilized β-galactosidase. Catalysts 12, 7. doi:10.3390/catal12010007
Rinaldi, F., Fernandez-Lucas, J., de la Fuente, D., Zheng, C., Bavaro, T., Peters, B., et al. (2020). Immobilized enzyme reactors based on nucleoside phosphorylases and 2′-deoxyribosyltransferase for the in-flow synthesis of pharmaceutically relevant nucleoside analogues. Bioresour. Technol. 307, 123258. doi:10.1016/j.biortech.2020.123258
Robescu, M. S., Annunziata, F., Somma, V., Calvio, C., Morelli, C. F., Speranza, G., et al. (2022). From batch to continuous flow bioprocessing: Use of an immobilized γ-glutamyl transferase from B. subtilis for the synthesis of biologically active peptide derivatives. J. Agric. Food Chem. 70, 13692–13699. doi:10.1021/acs.jafc.2c03702
Romero-Fernandez, M., Heckmann, C. M., and Paradisi, F. (2022). Biocatalytic production of a Nylon 6 precursor from caprolactone in continuous flow. ChemSusChem 15, e202200811. doi:10.1002/cssc.202200811
Romero-Fernandez, M., and Paradisi, F. (2021). Biocatalytic access to betazole using a one-pot multienzymatic system in continuous flow. Green Chem. 23, 4594–4603. doi:10.1039/d1gc01095f
Roura Padrosa, D., Benítez-Mateos, A. I., Calvey, L., and Paradisi, F. (2020). Cell-free biocatalytic syntheses of l-pipecolic acid: A dual strategy approach and process intensification in flow. Green Chem. 22, 5310–5316. doi:10.1039/d0gc01817a
Roura Padrosa, D., and Contente, M. L. (2021). Multi-gram preparation of cinnamoyl tryptamines as skin whitening agents through a chemo-enzymatic flow process. Tetrahedron Lett. 86, 153453. doi:10.1016/j.tetlet.2021.153453
Roura Padrosa, D., Nissar, Z., and Paradisi, F. (2021). Efficient amino donor recycling in amination reactions: Development of a new alanine dehydrogenase in continuous flow and dialysis membrane reactors. Catalysts 11, 520. doi:10.3390/catal11040520
Ruzic, L., Bolivar, J. M., and Nidetzky, B. (2020). Glycosynthase reaction meets the flow: Continuous synthesis of lacto-N-triose II by engineered β-hexosaminidase immobilized on solid support. Biotechnol. Bioeng. 117, 1597–1602. doi:10.1002/bit.27293
Ryazantseva, K. A., Agarkova, E. Y., and Fedotova, O. B. (2021). Continuous hydrolysis of milk proteins in membrane reactors of various configurations. Foods Raw Mater 9, 271–281. doi:10.21603/2308-4057-2021-2-271-281
Santana, J. L., Oliveira, J. M., Nascimento, J. S., Mattedi, S., Krause, L. C., Freitas, L. S., et al. (2020). Continuous flow reactor based with an immobilized biocatalyst for the continuous enzymatic transesterification of crude coconut oil. Biotechnol. Appl. Biochem. 67, 404–413. doi:10.1002/bab.1885
Sato, T., and Tosa, T. (2010). L-Amino acids production by aminoacylase in Encyclopedia of Industrial Biotechnology. Bioprocess Biosep. Cell. Technol.
Schiel, F., Peinsipp, C., Kornigg, S., and Böse, D. (2021). A 3D-printed open access photoreactor designed for versatile applications in photoredox- and photoelectrochemical synthesis. ChemPhotoChem 5, 431–437. doi:10.1002/cptc.202000291
Schober, L., Tonin, F., Hanefeld, U., and Gröger, H. (2022). Combination of asymmetric organo- and biocatalysis in flow processes and comparison with their analogous batch syntheses. Eur. J. Org. Chem. 2022, e202101035. doi:10.1002/ejoc.202101035
Schrader, J., Etschmann, M. M. W., Sell, D., Hilmer, J. M., and Rabenhorst, J. (2004). Applied biocatalysis for the synthesis of natural flavour compounds – current industrial processes and future prospects. Biotechnol. Lett. 26, 463–472. doi:10.1023/b:bile.0000019576.80594.0e
Schultz, D. C., Johnson, R. M., Ayyanathan, K., Miller, J., Whig, K., Kamalia, B., et al. (2022). Pyrimidine inhibitors synergize with nucleoside analogues to block SARS-CoV-2. Nature 604, 134–140. doi:10.1038/s41586-022-04482-x
Semproli, R., Vaccaro, G., Ferrandi, E. E., Vanoni, M., Bavaro, T., Marrubini, G., Annunziata, F., et al. (2020). Use of immobilized amine transaminase from Vibrio fluvialis under flow conditions for the synthesis of (S)-1-(5-fluoropyrimidin-2-yl)-ethanamine. ChemCatChem 12, 1359–1367. doi:10.1002/cctc.201902080
Sheldon, R. A., and Woodley, J. M. (2018). Role of biocatalysis in sustainable chemistry. Chem. Rev. 118, 801–838. doi:10.1021/acs.chemrev.7b00203
Simić, S., Jakštaitė, M., Huck, W. T. S., Winkler, C. K., and Kroutil, W. (2022). Strategies for transferring photobiocatalysis to continuous flow exemplified by photodecarboxylation of fatty acids. ACS Catalalysis 12, 14040–14049. doi:10.1021/acscatal.2c04444
Szabó, G. T., Mahiny, A. J., and Vlatkovic, I. (2022). COVID-19 mRNA vaccines: Platforms and current developments. Mol. Ther. 30, 1850–1868. doi:10.1016/j.ymthe.2022.02.016
Szczepańska, E., Colombo, D., Tentori, F., Olejniczak, T., Brenna, E., Monti, D., et al. (2021). Ene-reductase transformation of massoia lactone to δ-decalactone in a continuous-flow reactor. Sci. Rep. 11, 18794. doi:10.1038/s41598-021-97585-w
Tamborini, L., Fernandez, P., Paradisi, F., and Molinari, F. (2018). Flow bioreactors as complementary tools for biocatalytic process intensification. Trends Biotechnol. 36, 73–88. doi:10.1016/j.tibtech.2017.09.005
Tamborini, L., Previtali, C., Annunziata, F., Bavaro, T., Terreni, M., Calleri, E., et al. (2020). An enzymatic flow-based preparative route to Vidarabine. Molecules 25, 1223. doi:10.3390/molecules25051223
Teepakorn, C., Zajkoska, P., Cwicklinski, G., De, V., Zaparucha, A., Nonglaton, G., et al. (2021). Nitrilase immobilization and transposition from a micro-scale batch to a continuous process increase the nicotinic acid productivity. Biotechnol. J. 16, 2100010. doi:10.1002/biot.202100010
Tentori, F., Brenna, E., Crotti, M., Pedrocchi-Fantoni, G., Ghezzi, M. C., and Tessaro, D. (2020). Continuous-flow biocatalytic process for the synthesis of the best stereoisomers of the commercial fragrances leather cyclohexanol (4-isopropylcyclohexanol) and woody acetate (4-(tert-butyl)cyclohexyl acetate). Catalysts 10, 102. doi:10.3390/catal10010102
Thompson, M. P., Peñafiel, I., Cosgrove, S. C., and Turner, N. J. (2019). Biocatalysis using immobilized enzymes in continuous flow for the synthesis of fine chemicals. Org. Process Res. Dev. 23, 9–18. doi:10.1021/acs.oprd.8b00305
Vilas Bôas, R. N., de Lima, R., Mendes, A. A., Freitas, L., Bento, H. B. S., de Carvalho, A. K. F., et al. (2021). Batch and continuous production of biolubricant from fusel oil and oleic acid: Lipase screening, reactor system development, and reaction optimization. Chem. Eng. Process. Process Intensif. 168, 108568. doi:10.1016/j.cep.2021.108568
Westarp, S., Kaspar, F., Neubauer, P., and Kurreck, A. (2022). Industrial potential of the enzymatic synthesis of nucleoside analogs: Existing challenges and perspectives. Curr. Opin. Biotechnol. 78, 102829. doi:10.1016/j.copbio.2022.102829
Winkler, C. K., Simić, S., Jurkaš, V., Bierbaumer, S., Schmermund, L., Poschenrieder, S., et al. (2021). Accelerated reaction engineering of photo(bio)catalytic reactions through parallelization with an open-source photoreactor. ChemPhotoChem 5, 957–965. doi:10.1002/cptc.202100109
Wu, B., Zhang, S., Hong, T., Zhou, Y., Wang, H., Shi, M., et al. (2020). Merging biocatalysis, flow, and surfactant chemistry: Innovative synthesis of an FXI (Factor XI) inhibitor. Org. Process Res. Dev. 24, 2780–2788. doi:10.1021/acs.oprd.0c00412
Xu, L., Wang, J., Huang, F., and Zheng, M. (2022). An efficient and robust continuous-flow bioreactor for the enzymatic preparation of phytosterol esters based on hollow lipase microarray. Food Chem. 372, 131256. doi:10.1016/j.foodchem.2021.131256
Yucesoy, D. T., Akkineni, S., Tamerler, C., Hinds, B. J., and Sarikaya, M. (2021). Solid-binding peptide-guided spatially directed immobilization of kinetically matched enzyme cascades in membrane nanoreactors. ACS Omega 6, 27129–27139. doi:10.1021/acsomega.1c03774
Zhang, Z., Lee, W. J., Sun, X., and Wang, Y. (2022). Enzymatic interesterification of palm olein in a continuous packed bed reactor: Effect of process parameters on the properties of fats and immobilized Thermomyces lanuginosus lipase. LWT- Food Sci. Technol. 162, 113459. doi:10.1016/j.lwt.2022.113459
Keywords: flow biocatalysis, enzymes, enzyme immobilization, active pharmaceutical ingredients, fats and oils, food ingredients
Citation: Crotti M, Robescu MS, Bolivar JM, Ubiali D, Wilson L and Contente ML (2023) What’s new in flow biocatalysis? A snapshot of 2020–2022. Front. Catal. 3:1154452. doi: 10.3389/fctls.2023.1154452
Received: 30 January 2023; Accepted: 03 April 2023;
Published: 21 April 2023.
Edited by:
Gonzalo De Gonzalo, Sevilla University, SpainReviewed by:
Ana I. Benítez-Mateos, University of Bern, SwitzerlandNadia Guajardo, Metropolitan University of Technology, Chile
Copyright © 2023 Crotti, Robescu, Bolivar, Ubiali, Wilson and Contente. This is an open-access article distributed under the terms of the Creative Commons Attribution License (CC BY). The use, distribution or reproduction in other forums is permitted, provided the original author(s) and the copyright owner(s) are credited and that the original publication in this journal is cited, in accordance with accepted academic practice. No use, distribution or reproduction is permitted which does not comply with these terms.
*Correspondence: Martina L. Contente, bWFydGluYS5jb250ZW50ZUB1bmltaS5pdA==
†These authors have contributed equally to this work