- 1Department of Chemistry, Biochemistry and Pharmaceutical Sciences, University of Bern, Bern, Switzerland
- 2Novartis Institutes for Biomedical Research, Global Discovery Chemistry, Basel, Switzerland
The application of phenylalanine ammonia lyases (PALs) for the amination of a variety of cinnamic acids has been shown to be a cost-efficient method to produce a variety of phenylalanine analogues. Nonetheless, as many other biocatalytic tools, the process intensification, especially due to the high equivalents of ammonia needed, and the cost-efficiency of the catalyst production and use have been key points to further prove their usefulness. Here, we investigated the use of previously characterized PALs (AvPAL and PbPAL) for the amination of a series of substituted cinnamic acids. To enhance the process scalability and the reusability of the catalyst, we investigated the use of covalent immobilization onto commercially available supports, creating a heterogeneous catalyst with good recovered activity (50%) and excellent stability. The immobilized enzyme was also incorporated in continuous flow for the synthesis of 3-methoxy-phenyl alanine and 4-nitro-phenylalanine, which allowed for shorter reaction times (20 min of contact time) and excellent conversions (88% ± 4% and 89% ± 5%) respectively, which could be maintained over extended period of time, up to 24 h. This work exemplifies the advantages that the combination of enzyme catalysis with flow technologies can have not only in the reaction kinetics, but also in the productivity, catalyst reusability and downstream processing.
1 Introduction
Non-natural amino acids constitute an important group of building blocks for the drug, agrochemical, and protein therapeutics industry (Bommarius et al., 1998; Oliva et al., 2018; Narancic et al., 2019; Ding et al., 2020). Thus, their production as optically pure forms has been extensively studied and proved with multiple synthetic strategies. One of the most interesting and promising approach is the use of enzymes for their higher enantioselectivity and chemoselectivity. From the different strategies reported, the use of amine transferases, ammonia lyases and aminomutases has grown in attention mostly for the simplicity of the required starting materials and ammonia sources and their independence on addition of external cofactors, such as NAD(P)H, as in the case of oxidoreductases (Shin and Kim, 2009; Turner, 2011; Mathew and Yun, 2012; Nestl et al., 2014).
Among the different options, one of the most studied groups of enzymes are phenylalanine ammonia lyases and aminomutases. Both enzymes, have been primarily used for the production of phenylalanine analogues. Both classes, show very high homology in terms of sequence, folding and even catalysis (Heberling et al., 2013). First discovered in the biosynthesis of polyphenolic compounds in plants (Camm and Towers, 1973), phenylalanine ammonia lyases (PALs; EC 4.3.1.24) naturally catalyze the conversion of L-phenylalanine to trans-cinnamic acid, releasing ammonia. The reaction happens without need for external cofactors, only the 3,5-dihydro-5-methylidene-4H-imidazol-4-one (MIO) group, a prosthetic group formed spontaneously in the enzyme active site is needed for catalysis. More importantly, these enzymes show the capacity to catalyze the reverse reaction in high concentrations of ammonia, enabling the synthesis of substituted phenylalanine analogues from their corresponding cinnamic acids. Nonetheless, in the synthetic direction, even with high concentrations of ammonia in the reaction, the maximum reported conversions are around 80%–90% due to the unfavorable equilibria.
Another limitation is the narrow substrate scope and low specific activity that most of these enzymes exhibit. To enhance their activity and substrate scope, efforts have been centered in their engineering, towards the synthesis of products with various electronic characteristics with high similarity to phenylalanine (Lovelock and Turner, 2014; Weise et al., 2015; Bencze et al., 2017; Dreßen et al., 2017). In fact, these enzymes have even been combined in chemoenzymatic cascades for the synthesis of biaryl alanine (Ahmed et al., 2015; Weise et al., 2016; Ahmed et al., 2018; Nagy et al., 2019). In terms of operational stability in high concentrations of ammonia, these enzymes pose a challenge. However, their immobilization and therefore creation of heterogeneous catalysts, can greatly enhance their stability and reusability. Different techniques for the immobilization of PALs have been tested previously. Cross linked enzyme aggregates (CLEAs) showed increased thermal and operational stability, with good recovered activities (up to 45%) compared to the free enzyme (Cui et al., 2012). CLEAs have even been combined with magnetic particles to facilitate their separation from the reaction bulk (Sundlass et al., 2013; Cui et al., 2014). They have also been previously immobilized in carbon nanotubes (Jiang et al., 2004; Boros et al., 2021), even using site-specific chemistries for their implementation in continuous flow.
Here, we present the evaluation of two previously characterized PALs, AvPAL (Anabaena variabilis phenylalanine ammonia lyase) (Lovelock and Turner, 2014) and PbPAL (Planctomyces brasiliensis phenylalanine ammonia lyase) (Weise et al., 2017; Ahmed et al., 2018), for the synthesis of derivatives of phenylalanine. Through careful selection of the ammonia source, conversions of up to 85% in batch were achieved using the free enzyme. Moreover, through a screening of commercially available supports, we created an immobilized catalyst with up to 60% recovered activity and >90% immobilization yield, that could be used in a packed bed reactor in flow for the synthesis of two relevant cinnamic acid derivatives, with excellent productivities and high stability of the catalyst under those conditions. The combination of biocatalysis in continuous flow, also allowed for downstream extraction of the unreacted substrate and recovery through in-line liquid/liquid extraction.
2 Materials and methods
All reagents were purchased from Sigma Aldrich, Alfa Aesar, Apollo Scientific and used without further purification.
2.1 Protein expression and purification
Anabaena variabilis (AvPAL) and Plantomyces brasiliensis (PbPAL) phenylalanine ammonia lyases were expressed following previous protocols with minor modifications (Lovelock and Turner, 2014; Weise et al., 2017). For both PbPAL and AvPAL, after an overnight culture from a single colony, 300 mL of LB media were inoculated with 3 mL of the overnight culture. Once the OD600 reached 0.6–0.8 the media was supplemented with 1 mM IPTG (isopropylthio-β-galactoside) and expression was carried out at 18°C for 16 h. The cell pellet was collected by centrifugation (2,500 rpms, 30 min), resuspended in lysis buffer (100 mM Phosphate buffer, 0.3 M NaCl at pH 8) and sonicated for 10 min at 50% amplitude with a FisherBrand sonicator (40 cycles, 5″ON/10″OFF). The lysate was centrifuged at 14,500 rpms for 45 min to remove the insoluble part, and the supernatant filtered before purification with HisTrap Crude 1 mL columns loaded with Ni2+ with an ÄKTA pure FPLC system. Finally, the proteins were dyalized against 100 mM phosphate buffer pH 7.5 for 16 h and stored at 4°C. Protein concentration was measured by Abs280 using a BioTek Take3 plate in the BioTek EPOCH and an extinction coefficient estimated using ProtParam (https://web.expasy.org/protparam/).
2.2 Activity measurement
The activity of the two phenylalanine ammonia lyases used was measured identically and as reported previously (Lovelock and Turner, 2014; Weise et al., 2017), following the formation of cinnamic acid at 290 nm with a Cary60 UV-Vis Spectrophotometer. In a final volume of 1 mL, 790 µL of bicarbonate buffer pH 9 were mixed with 100 µL of a stock solution of 100 mM of L-Phenylalanine at pH 9. The solution was kept for 2 min at 37°C before the addition of 100 µL of the enzyme solution diluted to 0.1 mg/mL. The reaction was followed for 10 min using a Cary60 UV-Vis Spectrophotometer. One unit of enzymatic activity was defined as the amination of 1 nmol of cinnamic per minute. PbPAL showed an activity of 0.05 ± 0.01 U/mg protein and AvPAL showed an activity of 0.10 ± 0.02 U/mg protein.
2.3 Biotransformations
The biotransformations, either with the enzyme in its soluble or immobilized form, were performed in 2 mL Eppendorf tubes. Typically, unless stated otherwise, the biotransformations were performed in 1 mL of final volume with 2 mg/mL of free enzyme or 50 mg of immobilized form, 10 mM of the cinnamic acid derivative and 2 M of ammonium carbamate in bicarbonate buffer pH 10. The biotransformations were kept at 37°C in an incubator with 150 rpm mixing. Samples were taken at the desired times. For the immobilized enzyme, no more than 10% of the final volume was removed from the biotransformation during the reaction.
2.4 Enzyme immobilization
AvPAL and PbPAL immobilization was performed following previous reports (Mateo et al., 2007) with minor modifications. In short, 1 g of support was mixed with 4 mL of modification buffer [sodium borate (0.1 M) and iminodiacetic acid (2 M) in phosphate buffer (50 mM) pH 8.5], containing iminodiacetic acid and stirred for 2 h. After thorough washes with water, 10 mL of 0.1 M CoCl2 were added and incubated for 2 h before addition of the enzyme in 10 mL 50 mM phosphate buffer pH 8. The solution was left in contact with the resin for 16 h and the preparation was filtered, washed, and incubated for 16 h with blocking buffer at 25°C (4M glycine).
Immobilization yield is defined as the amount of protein bound to the support compared to the offered protein. The specific activity refers to the U/mgprotein once immobilized while the expressed activity refers to the U/gsupport.
2.5 Continuous flow
Flow reactions were performed with a Vapourtec R3+ system. In the different reactions, flow rates were adjusted from 20 to 200 μL/min to achieve the desired retention times or reaction times. Sampling was performed after the reactions and all results were analysed, at least, by the HPLC method detailed here. The column for the packed bed reactor was Omnifit glass column (6.6 mm i.d. X 100 mm length). In-line extraction was performed by mixing the reaction mixture after addition of H2SO4 with Isopropyl acetate (IpAc) at the same flow rate. The mixture was collected in a beaker and the organic phase containing the substrate pumped back to the original substrate mixture.
2.6 HPLC method
The samples were analyzed by HPLC (Dionex UltiMate 3000, Waters X-Bridge C18 (3.5 µm, 2.1 mm × 100 mm), measuring at 210, 250, and 265 nm), using a gradient method from 5:95 to 95:5 (H2O:MeCN 0.1%TFA) over 12 min with a flow rate of 0.8 mL/min. Conversions were assessed comparing the remaining substrate to a standard curve as well as the increase in the product concentration. Retention times were: o-methoxy cinnamic acid: 5.830 min; o-methoxy phenylalanine 4.617 min; p-methoxy cinnamic acid: 5,750 min; p-methoxy phenylalanine 3.730 min; o,p-dichloro cinnamic acid: 6.570 min; o,p-dichloro phenylalanine 4.980 min; p-NO2 cinnamic acid: 5.763 min; p- NO2 phenylalanine 4.590 min.
3 Results
3.1 Initial reaction characterization and batch biotransformations
First, to assess the reaction conditions necessary to prepare the desired L-phenylalanine derivatives, we set to explore the effect of the different ammonia sources in the reaction kinetics. These studies were performed with both AvPAL and PbPAL, in order to select the most suitable catalyst for the set of cinnamic acids under study. Other reports have shown the effect of the ammonia source can have on the final conversion depending on the enzyme used and the conditions (Weise et al., 2016; Boros et al., 2021). Thus, we selected m-MeO-cinnamic acid as the first test substrate and we investigated 4 different ammonia salts: ammonium carbonate, ammonium chloride, ammonium sulfate and ammonium carbamate.
In our case, a clear trend arose when comparing different ammonia sources (Figure 1). A large excess of ammonia is indeed needed to push the reaction toward the amination of cinnamic acid with these biocatalysts, in all cases. Thus, in our first screening, all ammonia sources were kept at a constant 5 M (500 equivalents) and 10 mM of the cinnamic acid derivative. While the pH did not change significantly during the reaction with any of the ammonia salts used, only with ammonium sulfate and ammonium carbamate conversion of >30% could be observed with PbPAL. PbPAL indeed reached >60% after 24 h with ammonium carbamate. For AvPAL a very similar trend appeared, but it showed consistently lower conversions. We then investigated the effect of the concentration of ammonium carbamate on the final conversion, and 2 M, yielded already a conversion superior to 70% after 24 h of reaction (Supplementary Table S2). Previous reports also indicated superior conversion rates with ammonium carbamate, explained by the lower ionic strength of this ammonium salt that could contribute to a higher enzyme stability, and therefore, higher final conversion of the initial substrate (Boros et al., 2021).
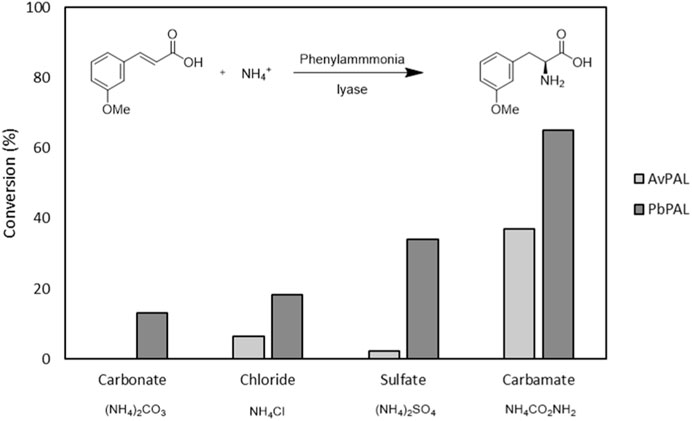
FIGURE 1. Ammonia sources tested for both AvPAL and PbPAL. The reaction was performed at 10 mM of the 3-methoxy cinnamic acid, 5M of ammonium salt and 0.5 mg/mL of the corresponding enzyme at 37°C and in a final volume of 1 mL. Samples were taken after 4 and 24 h. Conversions were measured by substrate depletion in the HPLC as detailed in the materials and methods. The results correspond to the average of two samples for each condition and enzyme.
We moved to explore a set of 4 different cinnamic acid derivatives with various substituents (Table 1). Three substrates showed significant conversion with both enzymes (m-methoxy cinnamic acid -1a-, o,p-dichloro cinnamic acid -2a- and p-NO2-cinnamic acid -4a-). It is noteworthy though, that 2a, showed very poor solubility even with the addition of 30% DMSO and therefore, could only be tested at 1 mM scale. These results are in accordance with previous reports with both AvPAL and PbPAL. Both enzymes’ favours cinnamic acids derivatives with electron withdrawing groups such as NO2 and halogens, rather than electron donating groups such as methoxy in para position. This preference, seems to be the result of a combination of factors: the electronics of the substrate and its interaction with the catalytic mechanism as well as steric effects and binding affinity (Ahmed et al., 2018). Previous reports also indicated the capacity of PbPAL to aminate m-methoxycinnamic acid in similar conditions, but significant engineering of the architecture of the active site was needed to achieve reasonable activity against the 4-methoxy version.
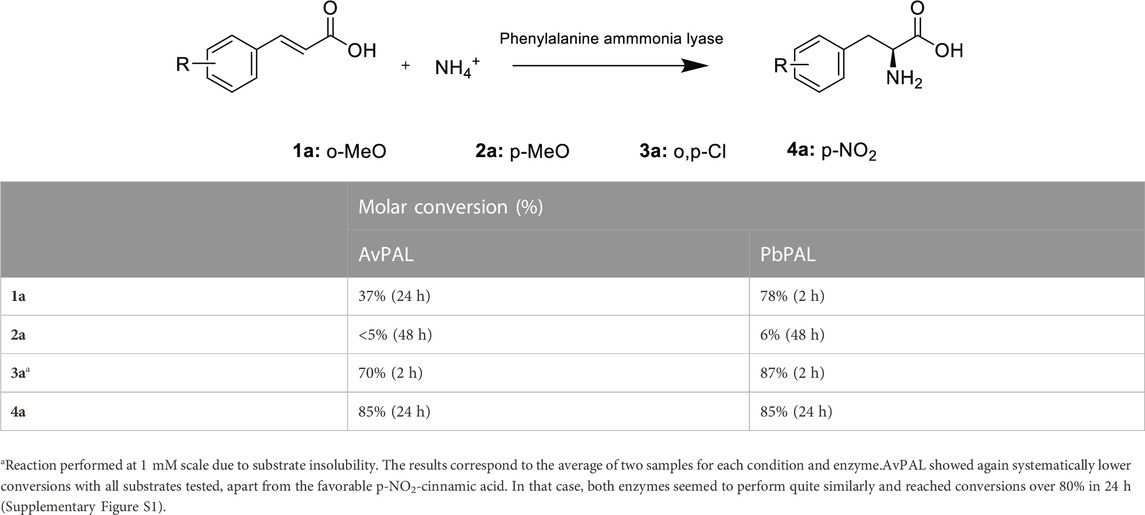
TABLE 1. Substrates tested for the amination with both AvPAL and PbPAL. The reaction was performed at 10 mM of the substrate, 5M of ammonium carbamate and 1 mg/mL of the corresponding enzyme at 37°C and in a final volume of 1 mL. Samples were taken after 4, 24 and 48 h. Conversions were measured by substrate depletion in the HPLC as detailed in the materials and methods.
With all substrates though, as reported before, the conversion was never complete. This issue, previously observed and discussed with phenylalanine ammonia lyase poses a limitation on the application of these enzymes. Efforts to push the equilibria further have been explored before through mostly, the addition of high equivalents of ammonia or aiding enzymes (Abu and Woodley, 2015; Weise et al., 2016; Hardegger et al., 2020). In our case, we wanted to apply this biotransformations in a continuous flow reactor to study the effect that a higher local concentration of catalyst might have on the biotransformation and to enable simpler and in-line separation of the product from the substrate and the recovery of the latter to enhance to cost-effectiveness of the process itself.
3.2 Covalent immobilization of AvPAL and PbPAL
To incorporate the enzyme in continuous flow, we created a heterogeneous biocatalyst through enzyme immobilization. Several reports are available on the immobilization of PALs using carrier-free methods (Cui et al., 2012; Dong Cui et al., 2015) as well as entrapment or anchoring to a plethora of materials (Cui et al., 2014; Bartha-Vári et al., 2015; Dong Cui et al., 2015; Boros et al., 2021; Koplányi et al., 2021). In this study, we focused on the covalent immobilization of AvPAL and PbPAL in commercially available methacrylic supports and Agarose 6BCL using epoxy handles to create a stable, reusable and easy to prepare catalyst. Epoxy immobilization had been used previously for PALs in microreactors and magnetic nanoparticles with good results (Weiser et al., 2015; Sánta-Bell et al., 2019). As the enzymes possess a His-tag, we took advantage of this characteristic to direct the immobilization and promote the covalent binding, following an established protocol (Mateo et al., 2007). Initial analysis of the surfaces of both enzymes (Supplementary Figure S3), indicated that all of them possessed a significant amount of lysine’s on the surface (5% and 2%) but had a strong hydrophobic character with 18 and 14 clusters of hydrophobic residues (3 or more residues at less than 5Å distance). Our screening included EP400/SS, EP403/S (both methacrylic resins with different hydrophobicity), HFA403/S (a methacrylic resin with amino epoxy handles) and agarose 6BCL functionalized with epoxy groups (Supplementary Table S1).
With a loading of 5 mg protein/g support while the immobilization yield was over 70% in all cases (Supplementary Table S3), due to the low activity shown by both enzymes in the standard activity assay (0.1–0.05 U/mg protein), no activity could be detected using the spectrophotometric method in a reasonable time. Therefore, we moved away from this approach and directly applied the immobilized biocatalyst in biotransformations over longer periods of time. We tested the capacity of the different heterogeneous catalyst to aminate m-MeO cinnamic acid in identical conditions to the test performed with the free enzyme (Figure 2).
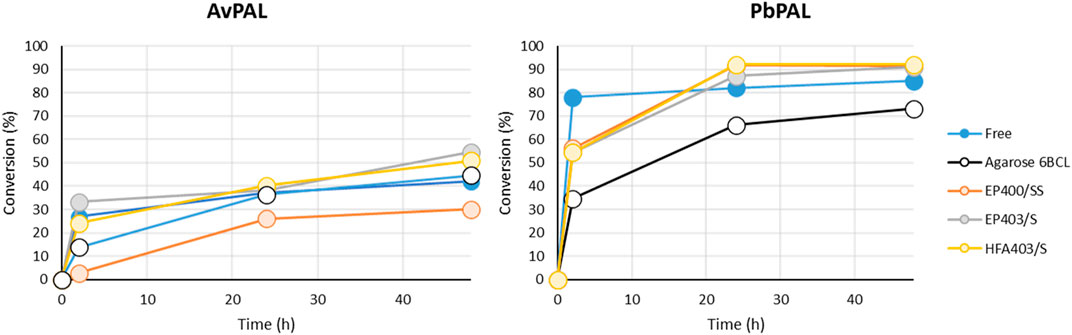
FIGURE 2. Time course of the biotransformation using immobilized enzyme for both AvPAL and PbPAL over 48 h with substrate 1a (m-MeO-cinnamic acid). 50 mg of the immobilized enzyme were mixed with 1 mL of 10 mM of 1a and 2 M of ammonium carbamate in 100 mM carbonate buffer pH10. Conversions were measured by HPLC using the method detailed in the materials and methods. The results correspond to a single measurement.
The biotransformation showed that in all cases the enzymes were indeed immobilized in their active form as activity could be detected with all supports. Interestingly, with both enzymes the biocatalyst prepared with agarose as the support, showed slower kinetics and lower final conversion, but all the methacrylic resins behaved very similarly. The immobilization, moreover, was confirmed to be exclusively covalent, as boiling of the resins did not show any protein leaching (Supplementary Figure S2). Even when immobilized, PbPAL clearly outperformed AvPAL.
Due to the higher catalytic efficiency obtained with the methacrylic resins EP400/SS and EP403/S, and the inert effect the amino group seemed to have on the immobilization, we selected both methacrylic resins for further studies with the better performing PbPAL. To enhance the expressed activity (U/g support) and maximize the catalytic efficiency of the heterogeneous catalyst we doubled the loading of the protein, reaching immobilized loadings of up to 9 mg protein/g support. With the higher loading used in these new preparations, the activity was measurable using the standard activity assay over extended reaction times (up to 20 min) and the recovered activities were up to 60% of the initial activity of the free enzyme (Table 2).

TABLE 2. Results of the immobilization of PbPAL in the two best supports tested. The immobilization was performed as detailed in the materials and methods. Activity was determined following the standard activity assay. The initial activity of the enzyme was 0.05 U/mg. The immobilization yield corresponds to the average of three measurements with the standard deviation, the expressed activity and recovered activity correspond to the average of duplicate measurements.
3.3 Application in continuous flow
Once the heterogeneous catalyst was optimized, we set to test its efficiency when used in a packed bed reactor (PBR) in continuous flow for the transformation of the two best substrates: m-MeO-cinnamic acid and p-NO2-cinnamic acid. Initial tests in flow, revealed that the substrate was sequestered by the methacrylate supports packed in the column with both EP403/S-PbPAL and EP400/S-PbPAL. When using the most hydrophilic resin (EP400/S) though, only 3 column volumes were needed to reach equilibration and detect both product and substrate in the outlet, and thus, all further tests were performed with EP400/SS-PbPAL 10 mg/g preparation with ammonium carbamate as the ammonia source.
Initially, different retention times were evaluated to understand the reaction kinetics (Figure 3A). With the setup detailed, only 20 min were necessary to reach conversions of over 75% consistently over multiple cycles and a further increase in the retention time did not change significantly the yield. As for the temperature, while initially conversions seemed to be nearly identical at all tested temperatures (Supplementary Figure S4), at 40°C the conversion was slightly lower and at 45°C, over time, an inactivation of the catalyst was observed. Thus, the ideal conditions in flow were fixed to 20 min of retention time and 37°C. These conditions were tested with both substrates (Figure 3B) and the results indicated that the conversion could be maintained for at least 6 column volumes (2 h of reaction time, 3 h total) without apparent loss of activity or drop in conversion.
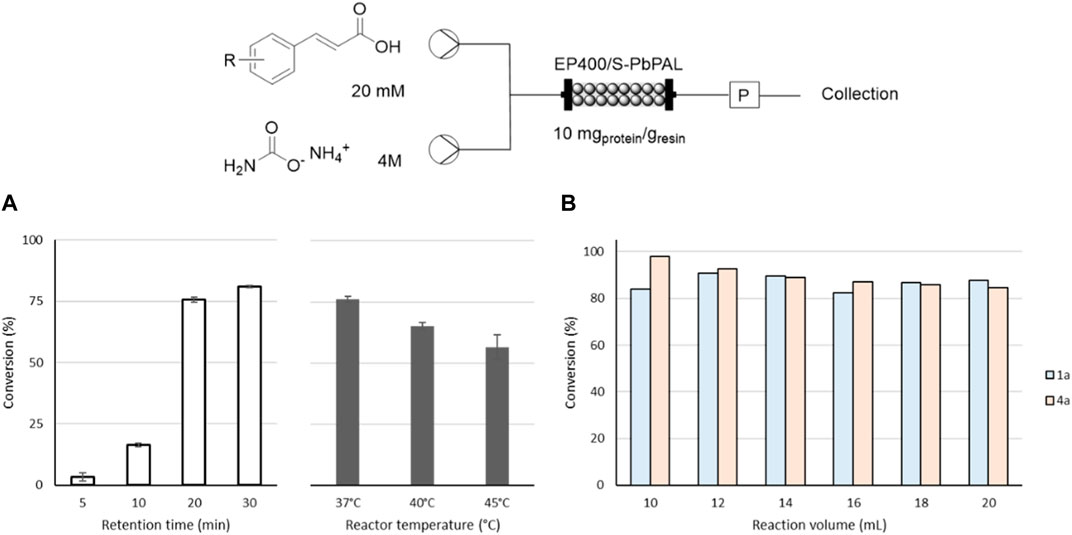
FIGURE 3. Continuous flow synthesis of 1a and 4a by immobilized PbPAL on methacrylate-based carriers (EP400/SS—10 mg/g). Conversions were always measured by HPLC. On top, the scheme of the flow setup is depicted. (A) Screening of the retention time and temperature needed to achieve maximal conversion of 1a. A column packed with 2.5 g of immobilized enzyme was used in all cases. Reactions were performed with 10 mM of 1a and 2 M ammonium carbamate at varying flow rates and reactor temperatures. The results correspond to the average conversion obtained after 5 column volumes, and the error bars correspond to the standard deviation. (B) Conversion profile over time of 1a and 4a processing a total volume of 20 mL. The reaction was performed with a retention time of 20 min at 37°C with 10 mM of 1a or 4a and 2 M ammonium carbamate in 100 mM bicarbonate buffer pH 10. The results correspond to single conversion values measured after the collection of 3 column volumes.
To further prove the stability of EP400/SS-PbPAL 10 mg/g, we performed 3 cycles processing 40 mL of volume in each using the same 2.5 g of immobilized enzyme. Each reaction was continued for a total time of 8 h. As the reaction is limited by the equilibrium (despite the excess of ammonia), here we introduced an in-line liquid-liquid extraction where the separation of the generated amine from the unreacted starting material could be performed (Figure 4). Through acidification to pH 5, the positively charged product stays in the water phase while the uncharged substrate can be recovered in the organic phase. Thus, a stream of concentrated H2SO4 was introduced after the PBR followed by the addition of isopropyl acetate (IPAc). Due to the high concentrations of ammonia, the amount of acid needed to bring the reaction mixture to a pH lesser than 5 was 1:1 ratio with the flow rate of the reaction. IPAc was added at half the flow rate to concentrate the substrate. With this setup, almost quantitative extraction of the substrate was achieved (Supplementary Figure S5) with no product detected in the organic phase. Over the accumulated 24 h, the catalyst did not show any significant loss of activity, indicating that it can be used up to 24 h without impacting the final yield. Here, washing the PBR with water between the different cycles was key to maintain the catalyst activity, as when stored for >6 h in contact with the high concentrations of ammonia used in the reaction resulted in a reduction of the expressed activity of the catalyst.
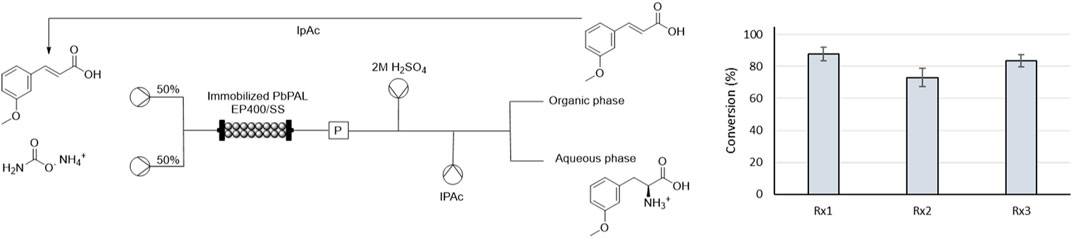
FIGURE 4. Results of the repeated use of 2 g of PbPAL immobilized in EP400/SS—10 mg/g with 1a and the scheme of the in-line separation of the unreacted substrate. The graph on the right shows the average conversions obtained in the continuous processing of 3 batches of 40 mL of reaction (6.7 h of reaction) of 10 mM of 1a, 2M ammonium carbamate in 100 mM bicarbonate buffer pH 10 with the same packed bed reactor and without changing the catalyst. The results correspond to the average conversion obtained after 3 measurements and the error bars correspond to the standard deviation.
4 Discussion
The application of PALs for the synthesis of amino acid derivatives has been vastly explored in the past, but their process intensification still remains one of the key objectives to move them towards powerful industrial synthetic tools. Through covalent immobilization in commercially available resins and the integration of the process in continuous flow, we greatly enhanced the process productivity and moreover, the catalyst productivity (Table 3). Compared to the batch reactions, either with free or immobilized enzyme, our continuous flow test constitutes further prove of the advantages the combination of biocatalysis and flow chemistry can bring to these syntheses. While the reaction in batch with immobilized enzyme had a decreased STY due to the longer periods of time needed to reach the full conversion, the short reaction times (20 min) necessary in flow give an increment of 3-fold compared to the productivity of the system in batch. Comparing this process to the benchmarks indicated by Meissner et al. (Meissner and Woodley, 2022), while the yield of the reaction is inferior to the desired 90%, the obtained STY rate is superior to the desired for high priced products (>100 $/kg) of 1–10 g/L/h or medium priced products (5–100 $/kg) of 10–100 g/L/h.

TABLE 3. Metrics of the reaction compared in batch with free enzyme, as an immobilized catalyst or in continuous flow. The calculation can be found in Supplementary Table S4. For the calculation of the catalyst productivity and E-factor, a volume of 100 mL was assumed to standardize the measures.
Moreover, due to the capacity to reuse the catalyst for long periods of time (up to 24 h), the catalyst productivity has been greatly enhanced (up to 10 times higher) taking into account the amount of enzyme needed to produce the same amount of product. While it is true that the productivity per total mass of catalyst still remains in the same figures as in batch, it is this capacity to recycle and reuse the catalyst when immobilized that pushes the cost-efficiency of the reaction even further. In this sense, comparing again with the benchmarks indicated by Meissner et al. in terms of specific yield, the enzyme productivity reported here is much closer to the desired catalyst productivity of 50 g product/g enzyme.
As for the sustainability of the system, amination of cinnamic acid with PALs still suffers from the need of high concentrations of ammonia and the high amount of water due to the low concentration of the substrate, which contribute the most the E-factor. While the reported E-factors are 7 times higher than the desired for pharmaceutical industry, we have been able to at least eliminate one of the produced wastes in the reaction: the catalyst. In addition and more importantly, the incorporation in flow of these biotransformations, allows for the recycling of the unused substrate by simple in-line liquid/liquid extraction, ensuring the minimum waste is produced and, possibly expensive substrates, can be recovered and reused in further reactions. Moreover, the water from these reactions could also be reused after treatment, reducing even more the environmental impact it could have.
In conclusion, this work highlights the advantages of the combination of flow technologies with biocatalysis, enabling the access in short reaction times and mild conditions to enantiopure analogues of phenylalanine, increasing the space time yield and catalyst productivity. This can be regarded as first step towards the application of this biotransformations in continuous flow and with in-line purification of the product, possible through liquid-liquid extractions as demonstrated here. The combination of biocatalysis and flow technologies, also opens the door toward diminishing the effect of the main solvent, water, towards the final reaction as it could also be recovered downstream to be recycled. On the other hand, the substrate concentration limitation could be surpassed by combining this technologies with novel approaches, such as deep eutectic solvents or alternative organic solvents to increase the substrate loading while maintaining the mild conditions reported here (Guajardo and Domínguez de María, 2019; van Schie et al., 2021; Holtmann and Hollmann, 2022).
Data availability statement
The original contributions presented in the study are included in the article/Supplementary Material, further inquiries can be directed to the corresponding author.
Author contributions
DRP performed the experiments and the data analysis. DRP, FP, HL and RS designed the project and discussed the results. DRP wrote the manuscript and all other authors reviewed and agreed to the final form of it.
Funding
We thank SNSF (200021_192274, FP) and BRIDGE Proof of Concept (40B1-0_213796, DRP) for financial support.
Acknowledgments
The authors wish to thank Prof. Turner group in Manchester University for sharing the plasmids of AvPAL and PbPAL used during this project.
Conflict of interest
Authors HL and RS are employed by Novartis.
The remaining authors declare that the research was conducted in the absence of any commercial or financial relationships that could be construed as a potential conflict of interest.
Publisher’s note
All claims expressed in this article are solely those of the authors and do not necessarily represent those of their affiliated organizations, or those of the publisher, the editors and the reviewers. Any product that may be evaluated in this article, or claim that may be made by its manufacturer, is not guaranteed or endorsed by the publisher.
Supplementary material
The Supplementary Material for this article can be found online at: https://www.frontiersin.org/articles/10.3389/fctls.2023.1147205/full#supplementary-material
References
Abu, R., and Woodley, J. M. (2015). Application of enzyme coupling reactions to shift thermodynamically limited biocatalytic reactions. ChemCatChem 7 (19), 3094–3105. doi:10.1002/cctc.201500603
Ahmed, S. T., Parmeggiani, F., Weise, N. J., Flitsch, S. L., and Turner, N. J. (2015). Chemoenzymatic synthesis of optically pure L- and d-biarylalanines through biocatalytic asymmetric amination and palladium-catalyzed arylation. ACS Catal. 5 (9), 5410–5413. doi:10.1021/acscatal.5b01132
Ahmed, S. T., Parmeggiani, F., Weise, N. J., Flitsch, S. L., and Turner, N. J. (2018). Engineered ammonia lyases for the production of challenging electron-rich l -phenylalanines. ACS Catal. 8 (4), 3129–3132. doi:10.1021/acscatal.8b00496
Bartha-Vári, J. H., Toşa, M. I., Irimie, F. D., Weiser, D., Boros, Z., Vértessy, B. G., et al. (2015). Immobilization of phenylalanine ammonia-lyase on single-walled carbon nanotubes for stereoselective biotransformations in batch and continuous-flow modes. ChemCatChem 7 (7), 1122–1128. doi:10.1002/cctc.201402894
Bencze, L. C., Filip, A., Bánóczi, G., Toşa, M. I., Irimie, F. D., Gellért, Á., et al. (2017). Expanding the substrate scope of phenylalanine ammonia-lyase from: Petroselinum crispum towards styrylalanines. Org. Biomol. Chem. 15 (17), 3717–3727. doi:10.1039/c7ob00562h
Bommarius, A. S., Schwarm, M., and Drauz, K. (1998). Biocatalysis to amino acid-based chiral pharmaceuticals - examples and perspectives. J. Mol. Catal. - B Enzym. 5 (1–4), 1–11. doi:10.1016/S1381-1177(98)00009-5
Boros, K., Moisǎ, M. E., Nagy, C. L., Paizs, C., Toşa, M. I., and Bencze, L. C. (2021). Robust, site-specifically immobilized phenylalanine ammonia-lyases for the enantioselective ammonia addition of cinnamic acids. Catal. Sci. Technol. 11 (16), 5553–5563. doi:10.1039/d1cy00195g
Camm, E. L., and Towers, G. H. N. (1973). Phenylalanine ammonia lyase. Phytochemistry 12 (5), 961–973. doi:10.1016/0031-9422(73)85001-0
Cui, J. D., Cui, L. L., Zhang, S. P., Zhang, Y. F., Su, Z. G., and Ma, G. H. (2014). Hybrid magnetic cross-linked enzyme aggregates of phenylalanine ammonia lyase from rhodotorula glutinis. PLoS One 9 (5), e97221–e97228. doi:10.1371/journal.pone.0097221
Cui, J. D., Zhang, S., and Sun, L. M. (2012). Cross-linked enzyme aggregates of phenylalanine ammonia lyase: Novel biocatalysts for synthesis of L-phenylalanine. Appl. Biochem. Biotechnol. 167 (4), 835–844. doi:10.1007/s12010-012-9738-0
Ding, Y., Ting, J. P., Liu, J., Al-Azzam, S., Pandya, P., and Afshar, S. (2020). Impact of non-proteinogenic amino acids in the Discovery and development of peptide therapeutics. Amino Acids 52 (9), 1207–1226. doi:10.1007/s00726-020-02890-9
Dong Cui, J., Lin Liu, R., and Lian Li, L. (2015). “Imprinted cross-linked enzyme aggregate (iclea) of phenylalanine ammonia lyase: A new stable biocatalyst,” in Lecture notes in electrical engineering T.-C. Zhang, and M. Nakajima (Berlin, Heidelberg: Springer Berlin Heidelberg), 332. doi:10.1007/978-3-662-45657-6
Dreßen, A., Hilberath, T., Mackfeld, U., Rudat, J., and Pohl, M. (2017). Phenylalanine ammonia lyase from arabidopsis thaliana (AtPAL2): A potent MIO-enzyme for the synthesis of non-canonical aromatic alpha-amino acids.: Part II: Application in different reactor concepts for the production of (S)-2-Chloro-Phenylalanine. J. Biotechnol. 258, 158–166. doi:10.1016/j.jbiotec.2017.04.035
Guajardo, N., and Domínguez de María, P. (2019). Continuous biocatalysis in environmentally-friendly media: A triple synergy for future sustainable processes. ChemCatChem 11 (14), 3128–3137. doi:10.1002/cctc.201900773
Hardegger, L. A., Beney, P., Bixel, D., Fleury, C., Gao, F., Perrenoud, A. G. G., et al. (2020). Toward a scalable synthesis and process for EMA401, Part III: Using an engineered phenylalanine ammonia lyase enzyme to synthesize a non-natural phenylalanine derivative. Org. Process Res. Dev. 24 (9), 1763–1771. doi:10.1021/acs.oprd.0c00217
Heberling, M. M., Wu, B., Bartsch, S., and Janssen, D. B. (2013). Priming ammonia lyases and aminomutases for industrial and therapeutic applications. Curr. Opin. Chem. Biol. 17 (2), 250–260. doi:10.1016/j.cbpa.2013.02.013
Holtmann, D., and Hollmann, F. (2022). Is water the best solvent for biocatalysis? Mol. Catal. 517, 112035. doi:10.1016/j.mcat.2021.112035
Jiang, K., Schadler, L. S., Siegel, R. W., Zhang, X., Zhang, H., and Terrones, M. (2004). Protein immobilization on carbon nanotubes via a two-step process of diimide-activated amidation. J. Mat. Chem. 14 (1), 37–39. doi:10.1039/b310359e
Koplányi, G., Sánta-Bell, E., Molnár, Z., Tóth, G. D., Józó, M., Szilágyi, A., et al. (2021). Entrapment of phenylalanine ammonia-lyase in nanofibrous polylactic acid matrices by emulsion electrospinning. Catalysts 11 (10), 1149. doi:10.3390/catal11101149
Lovelock, S. L., and Turner, N. J. (2014). Bacterial anabaena variabilis phenylalanine ammonia lyase: A biocatalyst with broad substrate specificity. Bioorg. Med. Chem. 22 (20), 5555–5557. doi:10.1016/j.bmc.2014.06.035
Mateo, C., Grazu, V., Palomo, J. M., Lopez-Gallego, F., Fernandez-Lafuente, R., and Guisan, J. M. (2007). Immobilization of enzymes on heterofunctional epoxy supports. Nat. Protoc. 2 (5), 1022–1033. doi:10.1038/nprot.2007.133
Mathew, S., and Yun, H. (2012). ω-Transaminases for the production of optically pure amines and unnatural amino acids. ACS Catal. 2 (6), 993–1001. doi:10.1021/cs300116n
Meissner, M. P., and Woodley, J. M. (2022). Mass-based biocatalyst metrics to guide protein engineering and bioprocess development. Nat. Catal. 5 (1), 2–4. doi:10.1038/s41929-021-00728-5
Nagy, E. Z. A., Tork, S. D., Lang, P. A., Filip, A., Irimie, F. D., Poppe, L., et al. (2019). Mapping the hydrophobic substrate binding site of phenylalanine ammonia-lyase from petroselinum crispum. ACS Catal. 9 (9), 8825–8834. doi:10.1021/acscatal.9b02108
Narancic, T., Almahboub, S. A., and O’Connor, K. E. (2019). Unnatural amino acids: Production and biotechnological potential. World J. Microbiol. Biotechnol. 35 (4), 67. doi:10.1007/s11274-019-2642-9
Nestl, B. M., Hammer, S. C., Nebel, B. A., and Hauer, B. (2014). New generation of biocatalysts for organic synthesis. Angew. Chem. - Int. Ed. 53 (12), 3070–3095. doi:10.1002/anie.201302195
Oliva, R., Chino, M., Pane, K., Pistorio, V., De Santis, A., Pizzo, E., et al. (2018). Exploring the role of unnatural amino acids in antimicrobial peptides. Sci. Rep. 8 (1), 8888–8916. doi:10.1038/s41598-018-27231-5
Sánta-Bell, E, Molnár, Z., Varga, A., Nagy, F., Hornyánszky, G., Paizs, C., et al. (2019). “Fishing and hunting”—selective immobilization of a recombinant phenylalanine ammonia-lyase from fermentation media. Molecules 24 (22), 4146. doi:10.3390/molecules24224146
Shin, J. S., and Kim, B. G. (2009). Transaminase-catalyzed asymmetric synthesis of L-2-aminobutyric acid from achiral reactants. Biotechnol. Lett. 31 (10), 1595–1599. doi:10.1007/s10529-009-0057-7
Sundlass, N. K., Eller, C. H., Cui, Q., and Raines, R. T. (2013). Contribution of electrostatics to the binding of pancreatic-type ribonucleases to membranes. Biochemistry 52 (37), 6304–6312. doi:10.1021/bi400619m
Turner, N. J. (2011). Ammonia lyases and aminomutases as biocatalysts for the synthesis of α-amino and β-amino acids. Curr. Opin. Chem. Biol. 15 (2), 234–240. doi:10.1016/j.cbpa.2010.11.009
van Schie, M. M. C. H., Spöring, J.-D., Bocola, M., Domínguez de María, P., and Rother, D. (2021). Applied biocatalysis beyond just buffers – from aqueous to unconventional media. Options and guidelines. Green Chem. 23 (9), 3191–3206. doi:10.1039/D1GC00561H
Weise, N. J., Ahmed, S. T., Parmeggiani, F., Galman, J. L., Dunstan, M. S., Charnock, S. J., et al. (2017). Zymophore identification enables the Discovery of novel phenylalanine ammonia lyase enzymes. Sci. Rep. 7 (1), 13691–13699. doi:10.1038/s41598-017-13990-0
Weise, N. J., Ahmed, S. T., Parmeggiani, F., Siirola, E., Pushpanath, A., Schell, U., et al. (2016). Intensified biocatalytic production of enantiomerically pure halophenylalanines from acrylic acids using ammonium carbamate as the ammonia source. Catal. Sci. Technol. 6 (12), 4086–4089. doi:10.1039/c6cy00855k
Weise, N. J., Parmeggiani, F., Ahmed, S. T., and Turner, N. J. (2015). The bacterial ammonia lyase EncP: A tunable biocatalyst for the synthesis of unnatural amino acids. J. Am. Chem. Soc. 137 (40), 12977–12983. doi:10.1021/jacs.5b07326
Weiser, D., Bencze, L. C., Bánõczi, G., Ender, F., Kiss, R., Kõkai, E., et al. (2015). Phenylalanine ammonia-lyase-catalyzed deamination of an acyclic amino acid: Enzyme mechanistic studies aided by a novel microreactor filled with magnetic nanoparticles. ChemBioChem 16 (16), 2283–2288. doi:10.1002/cbic.201500444
Keywords: biocatalysis, phenylalanine ammonia-lyase (PAL), enzyme immobilization, flow chemistry, process intensification
Citation: Roura Padrosa D, Lehmann H, Snajdrova R and Paradisi F (2023) Sustainable synthesis of L-phenylalanine derivatives in continuous flow by immobilized phenylalanine ammonia lyase. Front. Catal. 3:1147205. doi: 10.3389/fctls.2023.1147205
Received: 18 January 2023; Accepted: 19 April 2023;
Published: 04 May 2023.
Edited by:
Juan M. Bolivar, Complutense University of Madrid, SpainReviewed by:
Fernando López Gallego, CIC biomaGUNE, SpainSusana Velasco-Lozano, University of Zaragoza, Spain
Marco P.C. Marques, University College London, United Kingdom
Copyright © 2023 Roura Padrosa, Lehmann, Snajdrova and Paradisi. This is an open-access article distributed under the terms of the Creative Commons Attribution License (CC BY). The use, distribution or reproduction in other forums is permitted, provided the original author(s) and the copyright owner(s) are credited and that the original publication in this journal is cited, in accordance with accepted academic practice. No use, distribution or reproduction is permitted which does not comply with these terms.
*Correspondence: Francesca Paradisi, ZnJhbmNlc2NhLnBhcmFkaXNpQHVuaWJlLmNo