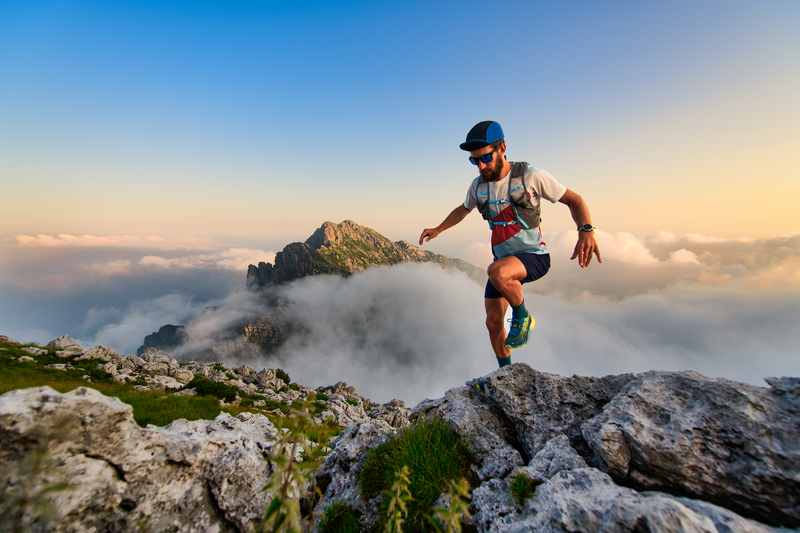
94% of researchers rate our articles as excellent or good
Learn more about the work of our research integrity team to safeguard the quality of each article we publish.
Find out more
ORIGINAL RESEARCH article
Front. Catal. , 16 February 2023
Sec. Biocatalysis
Volume 3 - 2023 | https://doi.org/10.3389/fctls.2023.1112154
Co-expression of enzymes allow to produce multiple enzymes in a single host, representing a cost-effective alternative in biocatalytic processes which can be used for pectin bioconversion. Pectin-rich biomass is an abundant by-product from the fruit and sugar industries that is usually disposed in landfill or sold as a low value feedstock. The aim of this work was to co-express a thermophilic pectin methyl esterase (PME) and exo-polygalacturonases (exo-PGs) in a single host for pectin bioconversion into D-galacturonic acid (GalA) using different pectic substrates such as apple, citrus and sugar beet pectin. To achieve this, a PME from Bacillus licheniformis (BLI09) with either an exo-PG from Thermotoga maritima (TMA01) or from Bacillus licheniformis (BLI04) were cloned in pETDuet-1 and co-expressed in E. coli BL21 (DE3). Four co-expression plasmids containing both pectinases were constructed and factors such as the effect of the genes’ cloning order and their expression were evaluated. Co-expression constructs 3 and 4 (pETDuet-TMA01-BLI09 and pETDuet-BLI04-BLI09, respectively) showed better expression of both pectinases compared to co-expression constructs 1 and 2 (pETDuet-BLI09-TMA01 and pETDuet-BLI09-BLI04, respectively). Co-expression constructs 3 and 4 were the most efficient for pectin bioconversion into GalA reaching 3 and 2.5 mM GalA, respectively from apple and citrus pectin after 4 h reaction. In conclusion, this work demonstrates that the co-expression of pectinases can potentially contribute to reduce the cost associated to their production and purification as well as to increase their applicability for exploiting pectin-rich biomass to obtain bio-based chemicals.
Pectin-rich biomass obtained mainly from agro-industrial residues is an important renewable feedstock to obtain valuable compounds within a biorefinery concept (Martins et al., 2020). Pectin is a heteropolymer composed by several substructures being the homogalacturonan (HG) the most abundant since accounts around 65% of the molecule. HG consists of α-(1→4)-linked D-galacturonic acid (GalA) that can be methylated at the carboxyl group of C6 and acetylated at O-2 or O-3 positions (Bonnin et al., 2014; Gawkowska et al., 2018; Dimopoulou et al., 2019). The bioconversion of pectin using pectinases has important economic and environmental advantages respect to the other treatments. Due to the heterogenicity of pectin structure, different kinds of pectinases are involved in its degradation, such as pectin methylesterases (PMEs) and polygalacturonases (PGs) (Voragen et al., 2009; Satapathy et al., 2020; Haile & Ayele, 2022). In addition, pectin acetylesterases (PAEs) are also relevant in highly acetylated pectin substrates (e.g., sugar beet pectin) (Bonnin et al., 2014; Leijdekkers et al., 2013; C Remoroza et al., 2014). These pectinases act in a synergistic and sequential manner to release GalA; the esterases first remove methyl and/or acetyl groups from the HG backbone, and subsequently exo-PGs catalyze monomeric GalA release by cleaving α-(1→4)-glycosidic bonds from the non-reducing ends (C Remoroza et al., 2014; Voragen et al., 2009).
Pectin-rich biomass has been hydrolyzed by synergistic pectinases cocktails to obtain pectic-derived oligosaccharides (POS) and monomerization of pectin. Some of these pectinases are commercially available as individual enzymes or as a mixture. Combo et al. (2013) used a commercial PME and endo-PG for sugar beet pectin hydrolysis to obtain POS with potential applications in medical and food industry; Leijdekkers et al. (2013) used a commercial pectinases cocktail for sugar beet pulp conversion into GalA and arabinose and; Leh et al. (2017) used a lyophilized fermented solid produced by Aspergillus oryzae with pectinolytic activity to the conversion of citrus pectin into GalA. Furthermore, pectinases have been mixed with cellulases, xylanases and cutinases for the hydrolysis of agricultural residues to improve processes in the textile and food industries (Xia et al., 2020; Degani, 2021; Olawuyi et al., 2022). Most of the commercial pectinases used for pectin hydrolysis has been produced by fungi (e.g., Aspergillus niger) showing their highest activity and stability at acidic conditions and temperatures below 50°C (Martens-Uzunova et al., 2006; Cerreti et al., 2017; Sudeep et al., 2020). TMA01 as well as BLI04 and BLI09 pectinases from Thermotoga maritima and Bacillus licheniformis, respectively are thermophilic enzymes which have been demonstrated to be active and stable in a wide range of pH and temperature (Flores-Fernández et al., 2022).
For multi-enzymatic reactions, including synergistic enzymatic reactions, the enzymes are usually cloned separately in an adequate plasmid and host cell. Then, they are mixed in different ratios for subsequent applications following their expression and purification; which demands extra costs and time (Roongsawang et al., 2010; Comlekcioglu et al., 2017). The co-expression of multiple enzymes in a single host is gaining more interest since it allows the cost-effective production of biocatalysts increasing their applicability in biocatalysis. Additional advantages of co-expression include the reduction of improper folding, ease of simultaneous purification and even the use of the clarified lysates as such (Dzivenu et al., 2004; Kumar et al., 2015). Some studies about enzymes co-expression have been carried out with different purposes. Among then, to reduce the production cost of carbohydrate degrading enzymes either to exploit sustainable biomass (Kumar et al., 2015; Comlekcioglu et al., 2017; Panpetch et al., 2018) or to be used as additives in animal feed (Roongsawang et al., 2010). Also, to develop whole cell biocatalysts through the co-expression of several degrading enzymes in bacteria or the construction of multi-enzymes synthetic pathways (Lan et al., 2006; Zhu et al., 2015). Finally, to enhance soluble and extracellular expression of enzymes by co-expressing them with chaperones or phospholipase C (Peng et al., 2016; Su et al., 2017).
Two main strategies for the co-expression of enzymes in a single host such as E. coli have been reported including the use of a single or multiple plasmids (Kumar et al., 2015). In the first strategy, the expression levels depend on the presence or absence of promoters and terminators as well as on the cloning order of the genes (Chen et al., 2017); here, two or more genes are cloned and expressed in a single plasmid and three options are possible. Firstly, all the genes could be under the control of a single promoter and terminator; secondly, each gene can be under the control of its own promoter and all share one terminator; and thirdly, each gene be under the control of its own promoter and its own terminator (Romier et al., 2006; Chen et al., 2017). pETDuet-1 is one of the most used plasmids that allows the co-expression of two target genes, it contains two multiple cloning sites (MCSs) and each cloned gene is under the control of its own T7 promoter, and both share one T7 terminator (D. Held et al., 2003; Lan et al., 2006). In the strategy using multiple plasmids, the genes are cloned and expressed in different plasmids with different origins of replication and resistance markers; one of its drawbacks is the maintenance of the plasmids by the host due to the high metabolic and bioenergetic burdens that it involves. Also, the copy number and expression levels of one plasmid are influenced by the presence of the others (Romier et al., 2006; Kumar et al., 2015). To the best of our knowledge, co-expression of pectinases has not been reported yet. The co-expression of PMEs and exo-PGs in a single plasmid and in E. coli as well as their synergistic with other pectinases including PAEs represent a promising alternative to improve GalA release from pectin rich-biomass.
This work aims to co-express thermophilic pectinases in a single plasmid and host for a cost-effective pectin bioconversion into GalA. To achieve this, four co-expression plasmids were constructed and the influence of the cloning order of the genes in enzymes expression was evaluated. Then, the synergistic action between the PME and exo-PG as co-expressed enzymes was tested in apple, citrus and sugar beet pectin, and compared with the synergistic action using the pectinases expressed separately. Also, the addition of a PAE into the synergistic reactions was evaluated to improve GalA release from sugar beet pectin. Product inhibition was also performed to determine if GalA release was limited by this effect.
All chemicals were purchased from Merck KGaA (Sigma-Aldrich) (Darmstadt, Germany) and molecular biology reagents were from New England Biolabs (Hitchin, United Kingdom) unless otherwise stated. The thermophilic pectinases used in this work include a PME from Bacillus licheniformis DSM13 (BLI09) and two exo-PGs from Thermotoga maritima DSM 3109 and Bacillus licheniformis DSM 13 coded as TMA01 and BLI04, respectively. The genomic DNA of the bacterial sources was extracted following the protocol described by Bahri & Ward (1990), and the genes encoding the pectinases were amplified using the primers described in Supplementary Table S1. Then, they were cloned in pET plasmids with a C-terminal His6-tag and successfully expressed individually in E. coli BL21(DE3).
Four co-expression plasmids containing a thermophilic PME and exo-PGs were constructed in pETDuet-1 (Merck-Novagen) (Supplementary Table S2). In co-expression constructs 1 and 2, BLI09 was cloned in the MCS-1 and either TMA01 or BLI04 in the MCS-2. In co-expression constructs 3 and 4, TMA01 or BLI04 were cloned in the MCS-1 and BLI09 in the MCS-2. The genes encoding BLI09, TMA01 and BLI04 were amplified using the primers described in Supplementary Table S2.
The genes were cloned in the MCS-1 using the restriction enzymes BamHI and NotI and in the MCS-2 using NdeI and XhoI. The flowchart of the construction of all co-expression plasmids containing both pectinases and detailed cloning schematic representations are shown in Supplementary Figures S1–S6. Briefly, in all cases the first gene was cloned in MCS-2 of the pETDuet-1 with a C-terminal S-tag. The resulting plasmids were transformed into E. coli NovaBlue(DE3) using 0.05 mg mL−1 ampicillin. Following the plasmids purification, the second gene was cloned in MCS-1 with a N-terminal His6-tag. All the co-expression plasmids containing both genes were transformed again into E. coli NovaBlue(DE3). Then, they were extracted and validated by sequencing. Finally, these plasmids were transformed into E. coli BL21(DE3) as expression host.
For proteins expression, E. coli BL21(DE3) cells harbouring the co-expression constructs were grown in 150 mL of LB broth containing 0.05 mg mL−1 ampicillin. Cultivation was carried out in 1 L baffled shake flasks at 37°C and 250 rpm in a shaker incubator (Climo-shaker ISF1-X, Kuhner, Switzerland) until the OD600 reached 0.45. At this point, the cells were induced with 0.5 mM isopropyl β-D-1-thiogalactopyranoside (IPTG) and incubated at 25°C and 250 rpm until 24 h. The cells were harvested by centrifugation (8,500 g at 4°C for 10 min), the cell pellets were re-suspended in lysis buffer and disrupted by sonication (MSE Soniprep 150 sonicator, Sanyo, Japan) with 20 cycles of 10 s ON and 15 s OFF at 12 μm amplitude. Then, the clarified cell lysates were recovered by centrifugation (18,000 g at 4°C for 15 min) and kept at 4°C until further analysis. Pectinases expression in the co-expression constructs was confirmed with SDS-PAGE analysis using Novex™ 10% Tris-Glycine Mini Gels, WedgeWell™ format and NuPAGE™ MOPS SDS running Buffer (Thermo Fisher Scientific Inc., United Kingdom).
Pectinases cloned in MCS-1 (with an N-terminal His6-tag) were purified using a His-Tag Ni-affinity resin (Ni-NTA Agarose, Thermo Fisher Scientific Inc., United Kingdom); 10, 50 and 500 mM imidazole (in buffer 20 mM sodium phosphate and 300 mM NaCl pH 7) were used for equilibration, washing and elution, respectively. The eluted proteins were precipitated with ammonium sulphate to a final saturation of 70% (w/v). Pectinases cloned in MCS-2 (with a C-terminal S-tag) were purified using a S-protein agarose (Merck-Novagen, Darmstadt, Germany), 20 mM sodium phosphate containing 150 mM NaCl and 0.1% Triton X-100 pH 7 was used as bind/wash buffer and the enzymes were eluted with 3 M MgCl2. The elution fractions were desalted and concentrated by using Amicon® Ultra-15 centrifugal filters (Merck, Carrigtwohill, Ireland). After the purification process, the clarified lysates and the collected fractions were stored at 4°C until further analysis. Purification was confirmed by SDS-PAGE gels and protein quantification was carried out using Quick Start™ Bradford Protein Assay (Bio-Rad Laboratories Inc., Hemel Hempstead, United Kingdom) and bovine serum albumin as standard (Bradford, 1976).
Synergistic activity of co-expressed BLI09 PME with either TMA01 or BLI04 exo-PGs was carried out by mixing 0.5 mL of 0.5% (w/v) apple pectin, citrus pectin, or sugar beet pectin (CP Kelco, New Jersey, USA) (in 20 mM sodium phosphate pH 7) and 0.5 mL of clarified lysate of each co-expression construct (enzymatic activity described in Supplementary Table S3). The mixture was incubated at 50°C and 300 rpm for 24 h. Control reactions contained only TMA01 or BLI04; and blank reactions were prepared without addition of the clarified lysates containing the co-expressed enzymes and with addition of a similar amount of buffer instead. Samples were taken periodically and used for methanol and GalA quantification according to the procedures described in Sections 2.8.2. For GalA yield (%) calculations, 74% GalA content was used for apple and citrus pectin (manufacturer information).
To improve pectin bioconversion into GalA in sugar beet pectin, a PAE from Bacillus licheniformis (PAE21) was successfully cloned and expressed (Supplementary Section 1; Supplementary Figure S7). For he synergistic activity, the clarified lysate containing PAE21 (expressed separately) was mixed with the clarified lysates of the co-expressed pectinases. These synergistic reactions were carried out only with the co-expression constructs 3 and 4 and using 0.5% (w/v) sugar beet pectin (in 100 mM sodium phosphate pH 7). The reactions were performed as described above using 22 mU mL−1 of PAE21. Blank reactions were prepared without addition of enzymes solution and with addition of a similar amount of buffer instead. Samples were taken periodically and used for methanol, GalA and acetic acid quantification. For GalA yield (%) calculations, 65% GalA content was used for sugar beet pectin (manufacturer information).
The effect of methanol, GalA and acetic acid on BLI09, TMA01 and BLI04 was determined in co-expression constructs 3 and 4. Methanol and GalA were tested at final concentrations from 0.2 to 20 mM. Clarified lysates were pre-incubated for 10 min at room temperature in 20 mM sodium phosphate pH 7 containing the respective methanol or GalA concentration in a final volume of 500 μL. Pectinases (PME and exo-PG) activities were measured by adding the previous mixture to 500 μL of 0.5% (w/v) apple pectin. The reactions were carried out at 50°C and 300 rpm for 30 min. Methanol and GalA were quantified according to the procedures described in Section 2.8.2. The residual activity was expressed as percentage compared with a control assay without addition of methanol or GalA.
Similarly, acetic acid inhibition was tested from 0.2 to 10 mM final concentration. Clarified lysates were pre-incubated for 10 min at room temperature in 100 mM sodium phosphate pH 7 containing the respective acetic acid concentration in a final volume of 500 μL. Pectinases activities were measured under the same conditions mentioned above using apple pectin. Methanol and GalA were quantified following the procedures described in Section 2.8.2. The residual activity was calculated as the percentage of activity compared with a control assay without addition of acetic acid.
PME activity (∼0.3 μg mL−1) was carried out following the method reported by (M. T. Held et al., 2015). Briefly, 0.3 mL of 0.5% (w/v) apple pectin pH 7 were mixed with 0.2 mL of enzyme solution and incubated at 50°C for 15 min (Thermomixer™ C, Eppendorf, United Kingdom). Then, the reaction was cooled down on an ice bath and centrifuged at 10,000 g for 3 min. The colorimetric reaction was carried out in a 96-well plate where 25 μL of the supernatant, 175 μL of 50 mM HEPES buffer at pH 7, 10 μL of 36 mg mL−1 Fluoral-P and 10 μL of 20 U mL−1 alcohol oxidase (AO) were mixed. The mixture was incubated at room temperature for 30 min and the methanol was quantified at 410 nm in a plate reader (CLARIOstar Plus Microplate Reader, BMG LabTech, Germany). A calibration curve using formaldehyde as standard (ε = 0.0388 mM−1 cm−1) was used for calculations (Supplementary Figure S8). One unit (U) of PME activity was defined as the amount of enzyme that releases 1 μmol of methanol equivalent per min at pH 7°C and 50°C.
Exo-PG activity (∼2 μg mL−1) was performed following the method described by (Miller, 1959). Briefly, 0.5 mL of 0.5% (w/v) polyGalA pH 6.5 were mixed with 0.5 mL of enzyme solution and incubated at 50°C for 15 min. Then, 0.5 mL of 3,5-Dinitrosalicylic acid (DNS) were incorporated to the reaction, the mixture was heated at 100°C for 15 min and centrifuged at 10,000 g for 5 min. The reducing groups were quantified in the supernatant at 540 nm in 96-well plates. A calibration curve using GalA as standard (ε = 0.127 mM−1 cm−1) was used for calculations (Supplementary Figure S9). One unit (U) of exo-PG activity was defined as the amount of enzyme that releases 1 μmol of GalA equivalent per min at pH 6.5°C and 50°C.
PAE activity (∼0.6 mg mL−1) was measured according to the method reported by (C Remoroza et al., 2014) using 30 µL of 10 mM 4-nitrophenol acetate (pNPA) in DMSO as substrate, which was pre-incubated with 270 µL of 100 mM sodium phosphate pH 7 for 3 min at 50°C. Then, 0.2 mL of enzyme solution were added and the mixture was incubated at 50°C and 500 rpm for 5 min. Then, the reactions were cooled down on ice and centrifuged at 10,000 g at 4°C for 3 min. The released p-nitrophenol was quantified in the supernatant at 405 nm in 96-well plates. A calibration curve using p-nitrophenol as standard (ε = 8.3424 mM−1 cm−1) was used for calculations (Supplementary Figure S10). One unit (U) of PAE activity was defined as the amount of enzyme that releases 1 μmol of p-nitrophenol equivalent per min at pH 7°C and 50°C.
All activities assays were carried out in duplicates, and a blank reaction was also performed following the procedures described above without the addition of the enzyme solution and a similar amount of buffer was added instead.
For methanol quantification, the samples were taken periodically and then cooled down on an ice bath and centrifuged at 10,000 g for 3 min. Methanol was quantified in the recovered supernatants. Thus, 25 μL of the supernatant, 175 μL of 50 mM HEPES pH 7, 10 μL of 36 mg mL−1 Fluoral-P and 10 μL of 20 U mL−1 AO were mixed in a 96-well plate. The mixture was incubated at room temperature for 30 min and the absorbance was measured at 410 nm. The calibration curve using formaldehyde as standard was used for calculations (Supplementary Figure S8).
For GalA quantification, the samples from the synergistic reactions were stopped using 0.1% trifluoroacetic acid. The mixtures were centrifuged at 18,000 g for 15 min and the supernatants were recovered and used for the analysis. GalA was analyzed following the method reported by Ward et al. (2015) using Ion Chromatography System (ICS 5000+, Thermo Scientific, Hemel Hempstead, United Kingdom) equipped with a Dionex Aminopac™ PA1 anion exchange column 4 × 250 mm fitted with a Dionex Aminopac™ PA1 guard column 4 × 50 mm. The analysis was carried out using 5% (v/v) 1 M sodium acetate (electrochemical detection grade, Fisher Scientific, United Kingdom) as mobile phase and 95% (v/v) Milli Q water at 0.25 mL min−1 for 8 min at 30°C. An external standard calibration curve of GalA was used for quantitative analysis, which was performed by measuring the peak area. The retention time of GalA was 4 min.
For acetic acid quantification, the samples were taken periodically, then cooled down on an ice bath and centrifuged at 10,000 g for 3 min. Acetic acid was quantified in the recovered supernatants using the Megazyme acetic acid kit K-ACETRM 04/20 (Megazyme, Wicklow, Ireland). For this assay, the microplate procedure was followed using 210 μL samples. A calibration curve using acetic acid as standard (ε = 3.7541 mM−1 cm−1) was used for calculations (Supplementary Figure S11).
In order to achieve a cost-effective pectin bioconversion into GalA, co-expression plasmids containing a thermophilic PME and exo-PGs were constructed in pETDuet-1 and the enzymes were expressed in E. coli BL21(DE3). Thus, four co-expression plasmids were constructed in which the effect of the cloning order of the genes in the pectinases expression was investigated. Co-expression constructs 1 and 2 were pETDuet-BLI09-TMA01 and pETDuet-BLI09-BLI04, respectively (Figures 1A1, A2), where BLI09 PME was cloned in MCS-1 and the exo-PGs in MCS-2. Co-expression constructs 3 and 4 were pETDuet-TMA01-BLI09 and pETDuet-BLI04-BLI09, respectively (Figures 1B1, B2), where the exo-PGs were cloned in MCS-1 and BLI09 PME in MCS-2. In all the co-expression constructs, each gene was under the control of its own T7 promoter, and both shared one T7 terminator.
FIGURE 1. Co-expression constructs containing BLI09 PME with either TMA01 or BLI04 exo-PGs in pETDuet-1. (A1) Co-expression construct 1: pETDuet-BLI09-TMA01, where BLI09 is in MCS1 (green) and TMA01 in MCS2 (pink); and (A2) co-expression construct 2: pETDuet-BLI09-BLI04, where BLI09 is in MCS1 (green) and BLI04 in MCS2 (light blue). (B1) Co-expression construct 3: pETDuet-TMA01-BLI09, where TMA01 is in MCS1 (pink) and BLI09 in MCS2 (green); and (B2) co-expression construct 4: pETDuet-BLI04-BLI09, where BLI04 is in MCS1 (light blue) and BLI09 in MCS2 (green). Co-expression constructs were plotted using SnapGene 4.2.11 software. PME: pectin methylesterase, exo-PG: exo-polygalacturonase, MCS: multiple cloning site.
BLI09, TMA01 and BLI04 with a molecular weight of 35, 50 and 48 kDa; respectively, were expressed in all the co-expression constructs and in their soluble form as shown in Figure 2. In co-expression constructs 1 and 2, TMA01 and BLI04 showed good expression levels, whilst BLI09 presented low expression. On the other hand, in the co-expression constructs 3 and 4 where the cloning order of the genes was inverted, both exo-PGs presented good expression and BLI09 cloned downstream the exo-PGs in MCS-2 with a T7 terminator behind, showed improved expression levels compared to co-expression constructs 1 and 2. Overall, the co-expression of the studied pectinases was better for the co-expression constructs 3 and 4.
FIGURE 2. SDS-PAGE showing the co-expression of BLI09 PME with either TMA01 or BLI04 exo-PGs in pETDuet-1 and E. coli BL21(DE3). Lanes: 1, co-expression construct 1 (pETDuet-BLI09-TMA01); 2, co-expression construct 2 (pETDuet-BLI09-BLI04); 3, co-expression construct 3 (pETDuet-TMA01-BLI09) and 4, co-expression construct 4 (pETDuet-BLI04-BLI09). MW, molecular weight marker (PageRuler™ Plus Prestained Protein Ladder, 10–250 kDa).
The co-expressed pectinases from all four co-expression constructs were purified by affinity chromatography (Supplementary Figures S12, S13). The purpose of this purification was to determine the enzymatic activity, protein concentration and specific activity of each purified co-expressed pectinase and compare these values between all the co-expression constructs (Table 1). BLI09 enzymatic activity was around 9-fold in co-expression constructs 3 and 4 compared to co-expression constructs 1 and 2. The highest BLI09 activity and protein concentration were 2556 U mL−1 and 0.142 mg mL−1, respectively and they were observed in the co-expression construct 3. Regarding TMA01 and BLI04, similar enzymatic activities and protein concentrations were observed in all the co-expression constructs ranging from 23 to 36 U mL−1 and 0.182–0.228 mg mL−1, respectively. Specific activities of BLI09, TMA01 and BLI04 were similar in all the co-expression constructs with values around 17,800, 166 and 118 U mg−1, respectively.
TABLE 1. Comparison of protein concentrations and enzymatic activities between purified pectinases (BLI09, TMA01 and BLI04) from the constructed co-expression constructs.
In addition, purification of co-expressed pectinases was carried out to compare the information from Table 1 with that from single expressed enzymes (Supplementary Table S4), both BLI09 enzymatic activity and protein concentration in single expression (9,000 U mL−1 and 0.5 mg mL−1) were 3.5 and 7.5-fold higher than in co-expression constructs 3 and 4, respectively. TMA01 and BLI04 enzymatic activities and protein concentrations in enzymes expressed separately (33 and 24 U mL−1, respectively and 0.2 mg mL−1) were similar to those in co-expressed enzymes. Specific activities of the three pectinases were similar in single expression and in the co-expression constructs.
The activity of the four co-expression constructs was tested using apple, citrus and sugar beet pectin with different degree of esterification and GalA content (Supplementary Table S5). The reactions were performed at compatible conditions for both enzymes (pH 7°C and 50°C) using the clarified lysates from the co-expression experiments. The pectinolytic activity of the co-expression constructs used for the synergistic reactions is presented in Supplementary Table S3. Based on our previous findings using individually expressed pectinases, we observed that the synergistic reactions for GalA release are more dependent on exo-PGs activity rather than PME (fast reaction) (Flores-Fernández et al., 2022). Thus, 0.5 and 2 U mL−1 of TMA01 and BLI04, respectively, were used in the synergistic reaction using co-expressed enzymes. The BLI09 activity was then determined as 4.5- (40.8 U mL−1) and 9.5-fold (86 U mL−1) higher in co-expression construct 3 and 4 compared to the individually expressed enzyme activity used for the synergistic reactions (9 U mL−1). Despite BLI09 enzymatic activity was higher in individual expressed enzymes, its levels in the co-expression constructs 3 and 4 were enough for the synergistic reactions.
Methanol release using the four co-expression constructs was similar for apple and citrus pectin (Figures 3A1, B1). Methanol levels were slightly higher using the co-expression constructs 3 and 4, reaching around 4 mM of methanol after 4 h of reaction. In sugar beet pectin, methanol release was also similar using all four co-expression constructs, but 50% less than in apple and citrus pectin (2 mM after 4 h of reaction) (Figure 3C1). Regarding GalA, similar concentrations were released using the four co-expression constructs for apple and citrus pectin (Figures 3A2, B2) at the end of the reaction (24 h). Remarkably, exo-PGs from co-expression constructs 3 and 4 showed faster reactions rates despite using the same activity units as the co-expression constructs 1 and 2; reaching concentrations around 3 and 2.5 mM GalA, respectively after 4 h of reaction. Therefore, assuming a 9 mM total GalA content in the reaction (based on 74% GalA in pectic), the synergistic reaction yields are around 35% and 29% GalA, respectively. For sugar beet pectin, GalA release was lower in comparison with apple and citrus pectin, around 0.1 and 0.2 mM were released after 24 h of reaction for all the co-expression constructs (Figure 3C2).
FIGURE 3. Synergistic activity between BLI09 PME with either TMA01 or BLI04 exo-PG as co-expressed enzymes using different pectic substrates. (A) Apple pectin, (B) citrus pectin and (C) sugar beet pectin. (A1,B1,C1) methanol release and (A2,B2,C2) GalA quantification. (
As low GalA concentrations were released using sugar beet pectin, it was hypothesized that its initial deacetylation by a PAE would improve GalA release. Thus, synergistic reactions between pectinases from co-expression constructs 3 and 4 along with PAE21 were carried out in a one-pot reaction (Figure 4). Acetic acid release was 0.6 mM after 4 h of reaction reaching a plateau at this point. Methanol levels were around 2 mM similar to those obtained using only co-expressed pectinases (Figure 3C1), indicating that the addition of PAE21 into the synergistic reactions did not affect BLI09 activity. Despite sugar beet pectin being demethylated and deacetylated, low GalA concentrations were released (around 0.7 mM) in comparison with apple and citrus pectin. However, these low concentrations were a 7- and 3.5-fold increase compared to co-expression constructs 3 and 4 with no PAE21, respectively. Thus, it can be inferred that the addition of PAE21 does not enhance GalA release which might be related to other issues such as substrate or product inhibition, or the unknown chemical features of the commercial substrate.
FIGURE 4. Synergistic activity of pectinases from co-expression constructs 3 and 4 along with PAE21 individually expressed using sugar beet pectin where methanol, acetic acid and GalA were quantified. The reactions were carried out at 50°C using 0.5% (w/v) substrate (in 100 mM phosphate pH 7), and 0.5 and 2 U mL−1 of TMA01 and BLI04, respectively. The BLI09 activity was calculated in the volumes containing the U mL−1 of exo-PGs mentioned before (Supplementary Table S3). The results shown correspond to a reaction time of 24 h. Error bars represent one standard deviation from the mean (n = 2). CexCons: co-expression construct, PAE: pectin acetylesterase.
The effect of product inhibition using methanol, GalA and acetic acid was tested on the co-expressed BLI09 with either TMA01 or BLI04 of co-expression constructs 3 and 4, since they were the most efficient for GalA release.
Methanol had an inhibitory effect on BLI09 from 6 mM and caused a complete inhibition from 14 mM (Figure 5A). Our results also showed a slight inhibitory effect of methanol on both exo-PGs from around 7 mM (Figures 5B, C). Regarding GalA, high concentrations (>7 mM) inhibited BLI09 (Figure 6A). Meanwhile, inhibition of TMA01 and BLI04 by this product occurred from 3 to 2.5 mM, respectively (Figures 6B, C).
FIGURE 5. Product inhibition effect of methanol on pectinases from co-expression constructs 3 and 4. (A) Methanol effect on BLI09, (B) TMA01 and (C) BLI04. The reactions were carried out at 50°C and 300 rpm for 30 min using 0.5% (w/v) apple pectin (in 20 mM phosphate pH 7), and 0.5 and 2 U mL−1 of TMA01 and BLI04, respectively. The BLI09 activity was calculated in the volume containing the U mL−1 of TMA01 mentioned before (Supplementary Table S3). Error bars represent one standard deviation from the mean (n = 2). PME: pectin methylesterase, exo-PG: exo-polygalacturonase.
FIGURE 6. Product inhibition effect of GalA on pectinases from co-expression constructs 3 and 4. (A) GalA effect on BLI09, (B) TMA01 and (C) BLI04. The reactions were carried out at 50°C and 300 rpm for 30 min using 0.5% (w/v) apple pectin (in 20 mM phosphate pH 7), and 0.5 and 2 U mL−1 of TMA01 and BLI04, respectively. The BLI09 activity was calculated in the volume containing the U mL−1 of TMA01 mentioned before (Supplementary Table S3). Error bars represent one standard deviation from the mean (n = 2). PME: pectin methylesterase, exo-PG: exo-polygalacturonase, GalA: galacturonic acid.
Low GalA concentrations were released in the synergistic reactions between co-expressed pectinases along with PAE21 using sugar beet pectin as substrate, which might be due to a potential pectinases inhibition by the released acetic acid. However, all pectinases (BLI09, TMA01 and BLI04) maintained more than 90% of their activity up to 10 mM acetic acid concentration (Supplementary Figure 14), showing good stability even at high concentrations of this compound.
Pectin has a very complex chemical structure and its biodegradation involves the synergistic action of pectinases mainly PMEs and PGs. This synergistic action allows accessing to bio-based chemicals such as GalA which is found in the highest concentration in pectin from different sources. Moreover, co-expression of pectinases with synergistic activities in a single culture represent a cost-effective alternative for pectin bioconversion. Co-expression plasmid systems such as pETDuet-1, pACYCDuet-1, pCDFDuet-1, pCOLADuet-1 and pRSFDuet-1 allow the cloning of up to two target genes. In all these plasmids, the genes can be cloned in the MCS-1 with a N-terminal His6-tag and in the MCS-2 with a C-terminal S-tag; however, they contain different antibiotic resistance genes and plasmid replicons as well as variable relative copy numbers which influence in the expression levels of the target proteins. pETDuet-1 has ampicillin resistance marker, ColE1 (pBR322) replicon and presents one of the highest copy numbers (∼40) (D. Held et al., 2003). Furthermore, in all the plasmids mentioned above, each gene is under the control of its own T7 promoter which ensures that the mRNA be transcribed for every gene independently of the read-through transcript. In addition, studies have suggested that the presence of a T7 promoter for each gene could increase the expression of the genes (Chen et al., 2017). Likewise, the sharing of a single T7 terminator by the two genes in all these plasmids suggests an influence from the first gene in the expression of the second one (Romier et al., 2006). In this work, pETDuet-1 was used to clone the pectinases BLI09, TMA01 and BLI04 which allowed to obtain four co-expression constructs.
Our results showed that the co-expression constructs 3 and 4 (with the PME in MCS-2) showed better expression levels of both pectinases based on SDS-PAGE analysis in comparison with the co-expression constructs 1 and 2. The expression of both exo-PGs was similar in all co-expression constructs regardless of their cloning position. However, BLI09 expression levels were higher in co-expression constructs 3 and 4 compared with those in co-expression constructs 1 and 2, which might be related to the cloning position as also reported by Chen et al. (2017). Expression and activity of BLI09 were significantly improved when cloned in MCS-2, this might be either associated to the presence of T7 terminator behind, since it has been reported that its presence can enhance the expression level of some genes (Liu et al., 2013; Chen et al., 2017); or to the S-tag in the C-terminus since studies have described that some fusion tags (such as S-Tag) can improve protein solubility in recombinant expression due to the presence of polar and charged amino acid residues (Costa et al., 2014; Paraskevopoulou & Falcone, 2018). In addition, protein expression can be improved by selecting a more appropriate expression host or optimizing the growth conditions such as: IPTG concentration, induction time, post-induction temperature and medium composition (Lan et al., 2006; Liu et al., 2013). These factors were not explored in this work, as the levels of the co-expressed pectinases (especially BLI09) were enough for subsequent experiments.
From the synergistic activity of the co-expressed pectinases, similar methanol concentrations were released from apple and citrus pectin (4 mM) since both substrates used in this work presented similar degree of methylation (Supplementary Table S5). However, low methanol concentrations were released from sugar beet pectin, which could be explained by the lower methylation of this substrate in comparison with apple and citrus pectin (35% in comparison with 58% and 55%, respectively). Regarding GalA, 3 and 2.5 mM were released in the synergistic reactions using the co-expression constructs 3 and 4, respectively after 4 h of reaction. However, using the co-expression constructs 1 and 2, similar concentrations were released after 24 h of reaction. These findings demonstrated that the co-expression constructs 3 and 4 were the most efficient for GalA release, despite same exo-PGs activities were used for all co-expression constructs. This could be due to the very high BLI09 activities in the co-expression constructs 3 and 4 (around 9-fold higher compared to co-expression constructs 1 and 2) causing a quick demethylation and therefore a faster depolymerization of the GalA backbone by the exo-PGs. In addition, GalA concentrations released using the clarified lysates of co-expressed pectinases were comparable to those using a cocktail of purified pectinases expressed separately (Flores-Fernández et al., 2022). These findings confirm that co-expression of enzymes is more efficient in terms of bioprocess performance and cost.
In sugar beet pectin, using all the co-expression constructs, low amounts of GalA were released (below 0.25 mM). It could be due to the presence of a high acetylation levels (20%) in comparison with apple and citrus pectin (∼1.5%) (Ma et al., 2020). The presence of acetyl groups hinders the exo-PGs activity (Bonnin et al., 2014). In this study, PAE21 improved GalA release but the concentrations obtained were lower than for apple and citrus pectin; this might be related to PAE21 mechanism of action and the position of acetyl groups in the GalA residues. It has been described that in sugar beet pectin, GalA is acetylated at the O-2 and O-3 positions. In addition, PAE21 deacetylates sugar beet pectin specifically at the O-3 positions in non-methylated GalA residues, leaving O-2 still acetylated (C Remoroza et al., 2014). This O-2 acetylation may still hinder exo-PGs activity and therefore GalA release. Another possible explanation might be the distribution of acetyl groups in the substrate associated to the deacetylation pattern of PAEs. A blockwise distribution of acetyl groups in HG from commercial sugar beet pectin has been reported (Ralet et al., 2008). Moreover, PAEs similar to PMEs, catalyze pectin deacetylation either in a random or blockwise pattern (Limberg et al., 2000; Remoroza et al., 2015; Kent et al., 2016). Therefore, if acetyl groups in sugar beet pectin are blockwise distributed and assuming that PAE21 deacetylates in a random manner, blocks of deacetylated pectin will not be produced. Thus, the presence of acetyl groups will obstruct exo-PGs activity and GalA release (Figure 7).
FIGURE 7. Schematic representation of the synergistic action between BLI09 PME with either TMA01 or BLI09 exo-PGs along with PAE21 on the HG backbone in sugar beet pectin. The blockwise demethylation pattern of BLI09, the blockwise distribution of acetyl groups and the deacetylation of PAE21 at O-3 positions of non-methylated GalA suggesting a random deacetylation pattern are illustrated. In addition, the exo-PGs action in the non-reducing end of demethylated and deacetylated pectin leading to monomeric GalA release is presented. No major increase in GalA release was observed with the addition of PAE21 into the synergistic reactions. GalA monomers highlighted in red (from 1 to 4) can be released by BLI09 with either TMA01 or BLI04. While the additional GalA monomer highlighted in purple (number 5) can be released with the addition of PAE21. (
Product inhibition assays revealed that BLI09 inhibition by methanol occurred from 6 mM, whilst TMA01 and BLI04 were not inhibited even at high methanol concentrations. These findings indicated that BLI09, TMA01 and BLI04 were not inhibited by methanol product during the synergistic reactions where up to 4 mM methanol was released. In situ removal of methanol may also improve the synergistic reaction performance, this could be achieved by increasing reaction temperature to the methanol boiling point (65°C) which would not affect thermophilic pectinases activity used in this work as these enzymes are highly thermostable at this temperature (Flores-Fernández et al., 2022).
Regarding pectinase inhibition by GalA, concentrations higher than 7 mM inhibited BLI09 indicating that this enzyme was not under GalA inhibition in the synergistic reactions where around 3 mM was obtained. GalA concentrations higher than 3 and 2.5 mM GalA inhibited TMA01 and BLI04, respectively. Thus, product inhibition might explain why only GalA below of 3 and 2.5 mM were achieved in the synergistic reactions with TMA01 and BLI04, respectively. Competitive inhibition by GalA has been reported for some exo-PGs (Kester et al., 1996; Baciu & Jördening, 2004; Bélafi-Bakó et al., 2007), where GalA binds to the active site of the enzyme by competing with the substrate and the inhibition effect can be reduced by increasing substrate concentration (Baciu & Jördening, 2004). From the bioprocesses perspective, product inhibition could be tackled through a simultaneous reaction (Bechtold & Panke, 2009), or in situ recovery using electrodialysis (Molnár et al., 2009) or bioreactors incorporating ultrafiltration membranes (Bélafi-Bakó et al., 2007; Kiss et al., 2009). Hence, although synergistic action between PMEs and exo-PGs is a promising strategy to release monomeric GalA from pectin, factors such as product inhibition limit the efficiency of the process. Respect to PAE21, this enzyme released low acetic acid levels (0.6 mM) from sugar beet pectin, which demonstrates that did not inhibit BLI09, TMA01 and BLI04 in the synergistic reactions. Finally, sugar beet pectin is highly branched (arabinans and galactans) compared to other pectic sources (Cárdenas-Fernández et al., 2017), which can also hinder the activity of the pectinases used in this work.
This work allowed co-expressing a thermophilic PME and exo-PGs which act synergistically to obtain GalA from pectin-rich sources. The co-expression constructs obtained here will ease the production of the two pectinases in a single culture. Likewise, the synergistic activity of the pectinases as co-expressed enzymes using the clarified lysates was efficient for pectin bioconversion into GalA. These together are very advantageous as it reduces the bioprocess cost associated to enzyme production and purification. Finally, this study contributes to a better understanding of pectin composition in different substrates as well as the mechanisms of action of pectinases; providing further insights for the applicability of pectinases and for improving pectin rich-biomass bioconversion into valuable compounds.
The original contributions presented in the study are included in the article/Supplementary Materials, further inquiries can be directed to the corresponding authors.
All authors contributed to the conception and the main idea of the work. CNF-F carried out the experiments, wrote the manuscript and elaborate figures and tables. MC-F supervised the work, provided feedback and additional scientific information, and revised the manuscript. JMW also supervised the work, reviewed and revised the manuscript. GJL reviewed and revised the manuscript. All authors read and approved the final version of the work.
This work was supported by the National Program of Scholarships and Student Loans (PRONABEC), Minister of Education, Peru (Law No 29837, Supreme Decree 013-2012-ED, Supreme Decree 008-2013-ED, Supreme Decree 001–2015-ED, RJ No 4264-2018- MINEDU/VMGI-PRONABEC-OBE). Funding was also received from the BBSRC BB/R021627/1.
The authors declare that the research was conducted in the absence of any commercial or financial relationships that could be construed as a potential conflict of interest.
All claims expressed in this article are solely those of the authors and do not necessarily represent those of their affiliated organizations, or those of the publisher, the editors and the reviewers. Any product that may be evaluated in this article, or claim that may be made by its manufacturer, is not guaranteed or endorsed by the publisher.
The Supplementary Material for this article can be found online at: https://www.frontiersin.org/articles/10.3389/fctls.2023.1112154/full#supplementary-material
Baciu, I. E., and Jördening, H. J. (2004). Kinetics of galacturonic acid release from sugar-beet pulp. Enzyme Microb. Technol. 34, 505–512. doi:10.1016/j.enzmictec.2003.12.008
Bahri, S. M., and Ward, J. M. (1990). Cloning and expression of an α-amylase gene from Streptomyces thermoviolaceus CUB74 in Escherichia coli JM107 and S. lividans TK24. J. Gen. Microbiol. 136, 811–818. doi:10.1099/00221287-136-5-811
Bechtold, M., and Panke, S. (2009). In situ product recovery integrated with biotransformations. Chim. (Aarau) 63, 345–348. doi:10.2533/chimia.2009.345
Bélafi-Bakó, K., Eszterle, M., Kiss, K., Nemestóthy, N., and Gubicza, L. (2007). Hydrolysis of pectin by Aspergillus Niger polygalacturonase in a membrane bioreactor. J. Food Eng. 78, 438–442. doi:10.1016/j.jfoodeng.2005.10.012
Bonnin, E., Garnier, C., and Ralet, M. C. (2014). Pectin-modifying enzymes and pectin-derived materials: Applications and impacts. Appl. Microbiol. Biotechnol. 98, 519–532. doi:10.1007/s00253-013-5388-6
Bradford, M. M. (1976). A rapid and sensitive method for the quantitation of microgram quantities of protein utilizing the principle of protein-dye binding. Anal. Biochem. 72, 248–254. doi:10.1016/0003-2697(76)90527-3
Cárdenas-Fernández, M., Bawn, M., Hamley-Bennett, C., Bharat, P. K. V., Subrizi, F., Suhaili, N., et al. (2017). An integrated biorefinery concept for conversion of sugar beet pulp into value-added chemicals and pharmaceutical intermediates. Faraday Discuss. 202, 415–431. doi:10.1039/c7fd00094d
Cerreti, M., Markošová, K., Esti, M., Rosenberg, M., and Rebroš, M. (2017). Immobilisation of pectinases into PVA gel for fruit juice application. Int. J. Food Sci. Technol. 52, 531–539. doi:10.1111/ijfs.13309
Chen, H., Huang, R., and Zhang, Y. H. P. (2017). Systematic comparison of co-expression of multiple recombinant thermophilic enzymes in Escherichia coli BL21(DE3). Appl. Microbiol. Biotechnol. 101, 4481–4493. doi:10.1007/s00253-017-8206-8
Combo, A. M. M., Aguedo, M., Quiévy, N., Danthine, S., Goffin, D., Jacquet, N., et al. (2013). Characterization of sugar beet pectic-derived oligosaccharides obtained by enzymatic hydrolysis. Int. J. Biol. Macromol. 52, 148–156. doi:10.1016/j.ijbiomac.2012.09.006
Comlekcioglu, U., Gunes, M., Altun, H., Ekiz, D. O., and Aygan, A. (2017). Coexpression of rumen fungal xylanase and bifunctional cellulase genes in Escherichia coli. Braz. Arch. Biol. Technol. 60, 1. doi:10.1590/1678-4324-2017160462
Costa, S., Almeida, A., Castro, A., and Domingues, L. (2014). Fusion tags for protein solubility, purification, and immunogenicity in Escherichia coli: The novel Fh8 system. Front. Microbiol. 5, 63–20. doi:10.3389/fmicb.2014.00063
Degani, O. (2021). Synergism between cutinase and pectinase in the hydrolysis of cotton fibers’ cuticle. Catalysts 11, 84–18. doi:10.3390/catal11010084
Dimopoulou, M., Alba, K., Campbell, G., and Kontogiorgos, V. (2019). Pectin recovery and characterization from lemon juice waste streams. J. Sci. Food Agric. 99, 6191–6198. doi:10.1002/jsfa.9891
Dzivenu, O. K., Park, H. H., and Wu, H. (2004). General co-expression vectors for the overexpression of heterodimeric protein complexes in Escherichia coli. Protein Expr. Purif. 38, 1–8. doi:10.1016/j.pep.2004.07.016
Flores-Fernández, C. N., Cárdenas-Fernández, M., Lye, G. J., and Ward, J. M. (2022). Synergistic action of thermophilic pectinases for pectin bioconversion into D-galacturonic acid. Enzyme Microb. Technol. 160, 110071. doi:10.1016/j.enzmictec.2022.110071
Gawkowska, D., Cybulska, J., and Zdunek, A. (2018). Structure-related gelling of pectins and linking with other natural compounds: A review. Polym. (Basel) 10, 762. doi:10.3390/polym10070762
Haile, S., and Ayele, A. (2022). Pectinase from microorganisms and its industrial applications. Sci. World J. 2022, 1–15. doi:10.1155/2022/1881305
Held, D., Yaeger, K., and Novy, R. (2003). New coexpression vectors for expanded compatibilities in E. coli. Innovations 4–6.
Held, M. T., Anthon, G. E., and Barrett, D. M. (2015). The effects of bruising and temperature on enzyme activity and textural qualities of tomato juice. J. Sci. Food Agric. 95, 1598–1604. doi:10.1002/jsfa.6990
Kent, L. M., Loo, T. S., Melton, L. D., Mercadante, D., Williams, M. A. K., and Jameson, G. B. (2016). Structure and properties of a non-processive, salt-requiring, and acidophilic pectin methylesterase from aspergillus Niger provide insights into the key determinants of processivity control. J. Biol. Chem. 291, 1289–1306. doi:10.1074/jbc.M115.673152
Kester, H. C. M., Kusters-Van Someren, M. A., Müller, Y., and Visser, J. (1996). Primary structure and characterization of an exopolygalacturonase from Aspergillus tubingensis. Eur. J. Biochem. 240, 738–746. doi:10.1111/j.1432-1033.1996.0738h.x
Kiss, K., Nemestóthy, N., Gubicza, L., and Bélafi-Bakó, K. (2009). Vacuum assisted membrane bioreactor for enzymatic hydrolysis of pectin from various agro-wastes. Desalination 241, 29–33. doi:10.1016/j.desal.2008.01.055
Kumar, S., Jain, K. K., Bhardwaj, K. N., Chakraborty, S., and Kuhad, R. C. (2015). Multiple genes in a single host: Cost-effective production of bacterial laccase (cotA), pectate lyase (pel), and endoxylanase (xyl) by simultaneous expression and cloning in single vector in E. Coli. PLoS One 10, 1–15. doi:10.1371/journal.pone.0144379
Lan, W. S., Gu, J. D., Zhang, J. L., Shen, B. C., Jiang, H., Mulchandani, A., et al. (2006). Coexpression of two detoxifying pesticide-degrading enzymes in a genetically engineered bacterium. Int. Biodeterior. Biodegr. 58, 70–76. doi:10.1016/j.ibiod.2006.07.008
Leh, D. S., Biz, A., de Paula, D. H. F., Richard, P., Gonçalves, A. G., Noseda, M. D., et al. (2017). Conversion of citric pectin into D-galacturonic acid with high substrate loading using a fermented solid with pectinolytic activity. Biocatal. Agric. Biotechnol. 11, 214–219. doi:10.1016/j.bcab.2017.07.003
Leijdekkers, A. G. M., Bink, J. P. M., Geutjes, S., Schols, H. A., and Gruppen, H. (2013). Enzymatic saccharification of sugar beet pulp for the production of galacturonic acid and arabinose; a study on the impact of the formation of recalcitrant oligosaccharides. Bioresour. Technol. 128, 518–525. doi:10.1016/j.biortech.2012.10.126
Limberg, G., Körner, R., Buchholt, H. C., Christensen, T. M. I. E., Roepstorff, P., and Mikkelsen, J. D. (2000). Analysis of different de-esterification mechanisms for pectin by enzymatic fingerprinting using endopectin lyase and endopolygalacturonase II from A. Niger. Carbohydr. Res. 327, 293–307. doi:10.1016/S0008-6215(00)00067-7
Liu, L., Yang, H., Shin, H. D., Chen, R. R., Li, J., Du, G., et al. (2013). How to achieve high-level expression of microbial enzymes: Strategies and perspectives. Bioengineered 4, 212–223. doi:10.4161/bioe.24761
Ma, X., Chen, W., Yan, T., Wang, D., Hou, F., Miao, S., et al. (2020). Comparison of citrus pectin and apple pectin in conjugation with soy protein isolate (SPI) under controlled dry-heating conditions. Food Chem. 309, 125501. doi:10.1016/j.foodchem.2019.125501
Martens-Uzunova, E. S., Zandleven, J. S., Benen, J. A. E., Awad, H., Kools, H. J., Beldman, G., et al. (2006). A new group of exo-acting family 28 glycoside hydrolases of Aspergillus Niger that are involved in pectin degradation. Biochem. J. 400, 43–52. doi:10.1042/bj20060703
Martins, L. C., Monteiro, C. C., Semedo, P. M., and Sá-Correia, I. (2020). Valorisation of pectin-rich agro-industrial residues by yeasts: Potential and challenges. Appl. Microbiol. Biotechnol. 104, 6527–6547. doi:10.1007/s00253-020-10697-7
Miller, G. L. (1959). Use of dinitrosalicylic acid reagent for determination of reducing sugar. Anal. Chem. 31, 426–428. doi:10.1021/ac60147a030
Molnár, E., Eszterle, M., Kiss, K., Nemestóthy, N., Fekete, J., and Bélafi-Bakó, K. (2009). Utilization of electrodialysis for galacturonic acid recovery. Desalination 241, 81–85. doi:10.1016/j.desal.2008.01.059
Olawuyi, I. F., Park, J. J., Park, G. D., and Lee, W. Y. (2022). Enzymatic hydrolysis modifies emulsifying properties of okra pectin. Foods 11, 1497. doi:10.3390/foods11101497
Panpetch, P., Field, R. A., and Limpaseni, T. (2018). Heterologous co-expression in E. coli of isoamylase genes from cassava Manihot esculenta Crantz ‘KU50’ achieves enzyme-active heteromeric complex formation. Plant Mol. Biol. 96, 417–427. doi:10.1007/s11103-018-0707-z
Paraskevopoulou, V., and Falcone, F. H. (2018). Polyionic tags as enhancers of protein solubility in recombinant protein expression. Microorganisms 6, 47. doi:10.3390/microorganisms6020047
Peng, S., Chu, Z., Lu, J., Li, D., Wang, Y., Yang, S., et al. (2016). Co-expression of chaperones from P. furiosus enhanced the soluble expression of the recombinant hyperthermophilic α-amylase in E. coli. Cell Stress Chaperones 21, 477–484. doi:10.1007/s12192-016-0675-7
Ralet, M. C., Crépeau, M. J., and Bonnin, E. (2008). Evidence for a blockwise distribution of acetyl groups onto homogalacturonans from a commercial sugar beet (Beta vulgaris) pectin. Phytochemistry 69, 1903–1909. doi:10.1016/j.phytochem.2008.03.008
Remoroza, C., Wagenknecht, M., Gu, F., Buchholt, H. C., Moerschbacher, B. M., Schols, H. A., et al. (2014). A Bacillus licheniformis pectin acetylesterase is specific for homogalacturonans acetylated at O-3. Carbohydr. Polym. 107, 85–93. doi:10.1016/j.carbpol.2014.02.006
Remoroza, Connie, Wagenknecht, M., Buchholt, H. C., Moerschbacher, B. M., Gruppen, H., and Schols, H. A. (2015). Mode of action of Bacillus licheniformis pectin methylesterase on highly methylesterified and acetylated pectins. Carbohydr. Polym. 115, 540–550. doi:10.1016/j.carbpol.2014.09.016
Romier, C., Ben Jelloul, M., Albeck, S., Buchwald, G., Busso, D., Celie, P. H. N., et al. (2006). Co-expression of protein complexes in prokaryotic and eukaryotic hosts: Experimental procedures, database tracking and case studies. Acta Crystallogr. Sect. D. Biol. Crystallogr. 62, 1232–1242. doi:10.1107/S0907444906031003
Roongsawang, N., Promdonkoy, P., Wongwanichpokhin, M., Sornlake, W., Puseenam, A., Eurwilaichitr, L., et al. (2010). Coexpression of fungal phytase and xylanase utilizing the cis-acting hydrolase element in Pichia pastoris. FEMS Yeast Res. 10, 909–916. doi:10.1111/j.1567-1364.2010.00669.x
Satapathy, S., Rout, J. R., Kerry, R. G., Thatoi, H., and Sahoo, S. L. (2020). Biochemical prospects of various microbial pectinase and pectin: An approachable concept in pharmaceutical bioprocessing. Front. Nutr. 7, 117–17. doi:10.3389/fnut.2020.00117
Su, L., Jiang, Q., Yu, L., and Wu, J. (2017). Enhanced extracellular production of recombinant proteins in Escherichia coli by co-expression with Bacillus cereus phospholipase C. Microb. Cell Fact. 16, 24–11. doi:10.1186/s12934-017-0639-3
Sudeep, K. C., Upadhyaya, J., Joshi, D. R., Lekhak, B., Chaudhary, D. K., Pant, B. R., et al. (2020). Production, characterization, and industrial application of pectinase enzyme isolated from fungal strains. Fermentation 6, 59. doi:10.3390/fermentation6020059
Voragen, A. G. J., Coenen, G. J., Verhoef, R. P., and Schols, H. A. (2009). Pectin, a versatile polysaccharide present in plant cell walls. Struct. Chem. 20, 263–275. doi:10.1007/s11224-009-9442-z
Xia, J., Shu, J., Yao, K., Xu, J., Yu, X., Xue, X., et al. (2020). Synergism of cellulase, pectinase and xylanase on hydrolyzing differently pretreated sweet potato residues. Prep. Biochem. Biotechnol. 50, 181–190. doi:10.1080/10826068.2019.1680390
Keywords: enzymes co-expression, thermophilic pectinases, pectin, D-galacturonic acid, pectin methyl esterases, exo-polygalacturonases
Citation: Flores-Fernández CN, Cárdenas-Fernández M, Lye GJ and Ward JM (2023) Co-expression of thermophilic pectinases in a single host for cost-effective pectin bioconversion into D-galacturonic acid. Front. Catal. 3:1112154. doi: 10.3389/fctls.2023.1112154
Received: 30 November 2022; Accepted: 03 February 2023;
Published: 16 February 2023.
Edited by:
Cesar Mateo, Spanish National Research Council (CSIC), SpainReviewed by:
Jan Muschiol, GEOMAR Helmholtz Center for Ocean Research Kiel and Helmholtz Association of German Research Centres (HZ), GermanyCopyright © 2023 Flores-Fernández, Cárdenas-Fernández, Lye and Ward. This is an open-access article distributed under the terms of the Creative Commons Attribution License (CC BY). The use, distribution or reproduction in other forums is permitted, provided the original author(s) and the copyright owner(s) are credited and that the original publication in this journal is cited, in accordance with accepted academic practice. No use, distribution or reproduction is permitted which does not comply with these terms.
*Correspondence: Max Cárdenas-Fernández, bWMyMDQwQGtlbnQuYWMudWs=; John M. Ward, ai53YXJkQHVjbC5hYy51aw==
Disclaimer: All claims expressed in this article are solely those of the authors and do not necessarily represent those of their affiliated organizations, or those of the publisher, the editors and the reviewers. Any product that may be evaluated in this article or claim that may be made by its manufacturer is not guaranteed or endorsed by the publisher.
Research integrity at Frontiers
Learn more about the work of our research integrity team to safeguard the quality of each article we publish.