- Department of Chemistry, Biology and Biotechnology and CIRCC, University of Perugia, Perugia, Italy
Molecular hydrogen (H2) is considered an ideal energy vector and a clean fuel, due to its zero-carbon combustion. Nevertheless, despite hydrogen is the most and one of the most abundant elements in the universe and in earth crust, respectively, it is always combined with other elements in our planet and never appears in its elemental state. This means that H2 must be produced through, almost always, endergonic processes, whose sustainability depend not only on the starting material but also on the source of energy necessary for these processes to occur. Colors have been assigned to identify the level of sustainability of H2 production with the green one indicating H2 produced from water using a renewable source of energy, preferably sunlight. Redox water splitting (WS) into H2 (hydrogen evolution reaction, HER) and O2 (oxygen evolution reaction, OER) is, nevertheless, an extremely difficult process not only from the thermodynamic but also from the kinetic point of view. Relevant kinetic barriers are present in both sides of the redox process, especially in OER. For this reason, performing WS in an efficient manner requires the development of active and robust catalysts capable of offering alternative reaction pathways to WS, lowering down the unfavorable kinetic barriers and thus maximizing the energy conversion efficiency. Inspiration for developing efficient catalysts for HER and OER has traditionally derived from Nature, who, over the course of many billions of years, according to the evolutionary theory, has assembled two molecular catalytic pools, namely oxygen evolving complex and ferredoxin/ferredoxin NADP+ reductase, which offer viable kinetic pathways to both OER and reduction of NADP+ (the “biological form” of H2). In reality, after several attempts of mimicking natural catalysts, the efforts of the researchers have been addressed to different molecular systems, which exhibit best performances, unfortunately often based on noble-metal atoms, especially for OER. In this contribution we review the journey of the development of molecular catalysts for both HER and the OER, highlighting selected systems, which have brought us to the current level of knowledge.
Introduction
The current climate and energy crises urgently require the identification of a sustainable alternative to fossil-derived fuels. Molecular hydrogen is emerging as the leading candidate to fulfil this role, owing to its non-toxicity, high energy per unit mass (120 MJ kg−1) and zero combustion emissions (European Commission, 2020; IEA, 2020; Crabtree et al., 2004; Parra et al., 2019; Griffiths et al., 2021). The sustainable supply of H2 on a global scale, however, poses some serious technological challenges (Song et al., 2022). Both production and storage of H2 are, indeed, non-trivial. Even though it is one of the most abundant elements on Earth, hydrogen is not directly available as it is bound to other elements in natural organic and inorganic molecules, some of them being the feedstocks for a variety of industrial H2 production processes. To establish sustainability metrics for the latter processes, the scientific community has felt the need to attribute colors to hydrogen, which is, notoriously a colorless molecule (Figure 1) (Newborough and Cooley, 2020; Griffiths et al., 2021).
At present, the vast majority of commercial hydrogen is produced via steam reforming or partial oxidation of non-renewable fossil feedstocks, like coal (black hydrogen), natural gas (grey hydrogen) and lignite (brown hydrogen) (Griffiths et al., 2021). In a short-term perspective, many efforts are devoted to limit the carbon footprint of these production processes. Some examples include integrating them with carbon capture and utilization systems (CCSU; blue hydrogen), or opting for methane pyrolysis, which produces solid carbon residues as byproduct rather than gaseous CO2 (turquoise hydrogen) (Newborough and Cooley, 2020). Nevertheless, the transition from fossil to renewable raw materials is essential for long-term sustainable hydrogen production.
Water splitting (WS) appears to be the ideal approach in this respect, being based on a cheap, non-toxic and renewable starting material like H2O, and providing O2 as the only benign byproduct (Armaroli and Balzani, 2007):
The main limit of this approach lies in its unfavorable kinetics and thermodynamics. Using fossil-derived electricity to drive such energy demanding process is clearly unreasonable. Although relatively small amounts of “low-carbon hydrogen” are currently produced by water electrolysis using nuclear power (purple hydrogen) or grid electricity (yellow hydrogen) (Griffiths et al., 2021), the production of truly green hydrogen is necessarily bound to the combination of renewable feedstocks, like water, and energy sources, ideally sunlight (Lewis and Nocera, 2006; Armaroli and Balzani, 2007; Velazquez Abad and Dodds, 2020). This latter process would mimic natural photosynthesis, exploiting sunlight to drive the water oxidation (WO) half-reaction, and then using the resulting protons and electrons to reduce NADP+ to NADPH cofactor and, consequently, CO2 to carbohydrates (Kärkäs et al., 2014). In both cases, light is the primary energy source, while chemical bonds -of either H2 or NADPH- serve as a kind of accumulator to deliver the energy packages where needed.
To realize an artificial photosynthesis device two aspects are critical: 1) developing efficient systems for harvesting solar energy, and 2) optimizing its conversion into chemical energy in the form of H–H bonds. Two main types of devices are typically utilized: photovoltaic-coupled electrolyzers (PVE, Figure 2) and photoelectrochemical cells (PEC, Figure 2). In PVEs, a photovoltaic cell provides the necessary overpotential to drive WS in the electrolyzer; in PECs, sunlight is instead harvested by a photoanode having suitable valence band potentials to carry out the oxidative half-reaction, with the generated electrons being transferred to the photocathode for proton reduction (Ager et al., 2015). In both approaches, one of the main hurdles towards the development of efficient WS devices lies in the high overpotentials needed, especially for the WO half-reaction. The identification of highly active and robust catalysts is therefore essential for knocking down such unfavorable kinetic barriers.
Over the last few decades, research in catalyst discovery and optimization has sparked, providing a plethora of catalytic systems for both half-reactions. Despite significant progress has been made, the identification of high-performing catalysts for large scale applications remains an open challenge.
Along with heterogeneous systems, molecular catalysts based on coordination compounds have drawn significant attention (Esswein and Nocera, 2007; Wang et al., 2009, Wang et al., 2012 M.; Eckenhoff and Eisenberg, 2012; Berardi et al., 2014; Zhang and Sun, 2019a; Barrozo and Orio, 2019). Their well-defined molecular nature offer some advantages, primarily in terms of atom efficiency, as molecular catalysts typically guarantee nearly 100% active metal centers; this is especially important when noble metals are employed (Zhang and Sun, 2019a; Macchioni, 2019). Furthermore, their reactivity can be tuned by structural tailoring to enhance activity. In this respect, it is also important to note that, with respect to heterogeneous systems, the activity of molecular catalysts can be more easily described in terms of performance parameters like turnover number (TON) and turnover frequency (TOF), facilitating kinetic and benchmarking studies that are essential for rational catalyst development (Kondo et al., 2021). These are the reasons why many efforts are devoted towards the development of water splitting devices involving molecular catalysts, having in durability and recyclability their Achilles’ heel.
This Review highlights the role which molecular catalysts might have in the pursuing of sustainable global energy supply. The discussion will focus on both half-reactions of water splitting, namely the hydrogen (HER) and oxygen (OER) evolution reaction, summarizing selected milestones, persisting bottlenecks, and foreseeable prospects.
Hydrogen Evolving Reaction Catalysts
In nature, reversible H2 oxidation is catalyzed by [FeFe], [FeNi] or Fe hydrogenase enzymes (Zhang and Sun, 2019a) in combination with cofactors/coenzymes that contribute synergistically to reaction thermodynamics.
As outlined before, proton reduction is realized in the laboratory either electrochemically (Wang M. et al., 2012) or photochemically (Sakai and Ozawa, 2007; Stoll et al., 2015; Eckenhoff, 2018; Amaro-Gahete et al., 2021). With a few exceptions, most classes of catalysts are active in both processes. Herein we will focus on electrocatalysis, aiming at sketching out the main branches of research in homogeneous HER and setting future directions in catalyst design.
The obvious starting point has been to take inspiration from natural enzymes. Rauchfuss and coworkers demonstrated in 2001 the suitability of the structural hydrogenase model 1 (Figure 3) in electrocatalytic H2 generation (Gloaguen et al., 2001). Since then, the field has become very active (Capon et al., 2005, 2009), but hydrogenase models revealed to suffer from poor solubility in water and scarce resistance towards strong acids. Therefore, most studies are performed in organic media, which are not an ideal choice when designing a sustainable device for WS. Hydrophilic substitution (Na et al., 2006) or encapsulation in cyclodextrins (Singleton et al., 2010) can all be used to solubilize hydrogenase models in water, but applications are still very limited. [NiFe] models such as 2 also show moderate activity at 400–430 mV overpotential, with rate constants in the order of 50 s−1 in CH2Cl2 (Barton and Rauchfuss, 2010). Mn dimers 3 and 4, with structural features resembling hydrogenase cores, showed higher activities than [FeFe] and [FeNi] models. These species can reduce protons at moderate overpotentials (550-650 mV) in either DMF or MeCN, with TOF up to 44600 s−1 (Hou et al., 2014; Hou and Fan, 2014).
Overall, such catalytic performance is still not satisfactory enough to foresee applications of hydrogenase models in H2 generation technologies in the next future. In stark contrast, mononuclear complexes of earth-abundant metals offer a solid alternative, with some species showing activities comparable (or even higher) than that of natural enzymes (TOF or kcat in the order of 1–2 × 104 s−1) (Frey, 2002). It must be noted that a direct comparison between different systems is difficult, owing to the very different experimental conditions used in electrocatalysis. Particular attention must be paid to overpotentials, as highly active systems tend to work at high overpotentials. The ideal catalyst should show high activity at low overpotential and only very few electrocatalysts are able to do so, as yet.
Striving for the ideal HER electrocatalyst, a few families of compounds stood out during the past two decades. A first notable example is the class of polypyridyl (Zee et al., 2015) and pyridyl-amine complexes of Mo, Co. (Sun et al., 2011), Ni (Zhang et al., 2014c) and Cu. Long, Chang and co-workers reported that the Mo(II) species 5 activates water catalytically and, under controlled electrolysis conditions at pH = 7, produces H2 with TOF approaching 2.4 s−1 and TON values of 6.1 × 105 at an overpotential of 640 mV (Karunadasa et al., 2010). Complex 6, a soluble mimic of molybdenite (Karunadasa et al., 2012), a very efficient heterogeneous HER catalyst (Jaramillo et al., 2007), also catalyzes electrochemical proton reduction in both MeCN and water, with optimal activity observed at pH = 3. A TOF up to 280 s−1 was observed at 828 mV overpotential. Interestingly, both 5 and 6 showed catalytic activity for H2 production when tested with seawater. The Cu complex 7 is another remarkably active catalyst, evolving H2 with a kobs of over 10000 s−1 at pH = 2.5 and 420 mV overpotential. Mechanistically, the reaction is supposed to occur upon two consecutive PCET events, affording a Cu(II) hydride intermediate with a protonated ligand, which undergoes H2 elimination and regenerates the catalyst (Zhang et al., 2014b).
Cobalt glyoximate complexes are also excellent catalysts for H2 electroproduction, offering cheaper alternatives to Pt and showing high activities at reduced overpotentials (Artero et al., 2011). Co(III) complexes 8, which are active in proton reduction in DMF/[HNEt3][Cl] and, particularly at low acid loading, show higher activities when more electron-donating groups are introduced (TON of 100 over 2.5 h, when R = H, 8a). To improve water solubility, Zhang and co-workers functionalized the pyridine ring with a triazole-glucose pendant group and obtained complex 8d, an electrocatalyst able to work under neutral conditions at an overpotential of 341 mV (Yin et al., 2015). Co(II) glyoximates 9 are comparatively more active and reduce protons from a broad set of acids with modest to low pKa in MeCN at very low overpotentials. For example, HOAcF is reduced at less than 50 mV overpotential with an estimated kobs of 770 s−1 (Hu et al., 2005).
To overcome the instability of cobaloximes towards hydrolysis, imine/oxime catalysts have been developed. This class of catalysts is a notable step forward, as they retain catalytic activity in neat water. Complex 10 catalyzes proton reduction in water at pH = 2.2 with a rather low overpotential of 390 mV, affording 23 turnovers (McCrory et al., 2012). More recently, Zong, Lu and Sakai reported the Co macrocyclic complex 11, which represents a kind of evolution of the imine glyoxymate scaffold (Wang et al., 2019). 11 is an extremely efficient electrocatalyst and can reduce protons at pH = 7 with a kcat of 2210 s−1at 680 mV overpotential. On top of its good catalytic performance, 11 is tolerant to O2 and CO, making a good candidate for application in H2 generation devices and fuel cells.
The combination of multidentate azophosphine ligands and first row transition metals originates an outstanding class of electrocatalysts for H2 oxidation and production (Dubois and Dubois, 2009). Following up on previous work (Wilson et al., 2006), Bullock, DuBois and coworkers reported in 2011 that the dicationic Ni(II) complex 12 is a robust and highly efficient catalyst for proton reduction in MeCN, using [(DMF)H]OTf (pKa = 6.1) as proton source (Helm et al., 2011). Catalytic activity was observed at an overpotential of 625 mV and a remarkable TOF of 106000 s−1 was obtained in the presence of traces of H2O. The ability of the pendant–N group to act as a positioned proton relay is proposed to be the key mechanistic concept, mimicking somehow what is proposed to occur in natural [FeFe] hydrogenases (Léger and Bertrand, 2008). A similar concept was later explored by Masuda with the Ni(II) phosphinopyridyl complex 13, containing amino groups able to act as proton relay. 13 catalyzes HER in MeCN/[NBu4][ClO4] using acetic acid as proton source, with TOF values up to 8400 s−1at an overpotential of 590 mV (Tatematsu et al., 2016). To enhance water solubility of this class of compounds, DuBois and co-workers functionalized the–N groups of the [Ni(PR2NR2)]2+ motif with biomimetic aminoacidic fragments, such as glycine and arginine (Dutta et al., 2014). These functionalized complexes showed reversible catalytic activity, even at elevated temperatures, and could be used for both H2 oxidation and production. The arginine derivative 14 revealed to resist a broad set of pH conditions, ranging from 0.0 to 6.0, and showed high TOF values at 348K, comparable to those of [NiFe] hydrogenases. 14 tolerates exposure to CO, therefore showing great potential for working in fuel cells.
Another branch of HER electrocatalysts is constituted by homoleptic metal-dithiolenes, which emerged after the first reports by Holland/Eisenberg (McNamara et al., 2011) and Sarkar (Begum et al., 2010). In 2015, Jones and coworkers showed that also the heteroleptic diphosphine-dithiolene complex 15 catalyzes HER in THF, with TOF up to 1240 s−1 and overpotentials as low as 265 mV (Gan et al., 2015). Even though catalytic performance of 15 is promising, its poor solubility in water hampers any real-life application. In 2017, Yamauchi, Sakai and coworkers reported the anionic Ni complex 16, which is water soluble and catalyzes HER at relatively low overpotentials (330–400 mV at pH 4–6). Bulk electrolysis experiments reached a turnover number of 20000 over 24 h, indicating that this family of catalysts is highly durable (Koshiba et al., 2017). Mechanistically, a series of consecutive, ligand-based, PCET events is proposed to be the origin of the reduced overpotentials (Aimoto et al., 2018).
A last branch involves metal porphyrins, which have long been known to be active electrocatalysts for H2 production but did not attract much interest owing to poor activity and high overpotentials (Kellett and Spiro, 2002). However, proper tuning of functional group can increase the catalytic performance of these species and, at the same time, impart a high solubility in water. For example, the group of Bren reported in 2014 that acetylated cobalt mycroperoxidase-11 (17) is an active bioorganometallic catalyst for proton reduction. In aqueous solution at pH 7.0, 17 showed promising initial TOF (6.7 s−1 after 10 min) and TON values (2.4 × 104), at an overpotential of 852 mV. Such an enhancement of activity was ascribed to the presence of the axial imidazole group from the peptide unit. Interestingly, 17 tolerates exposure to oxygen and retains catalytic activity in air, which is an important feature for the ideal catalyst for hydrogen evolution. However, the activity of 17 decreases over time owing to degradation under electrolytic conditions (Kleingardner et al., 2014). To enhance catalyst’s durability, Hung and coworkers used the p-sulfonatophenyl complex 18 for electrocatalytic proton reduction in neutral water, obtaining good performance both in terms of TOF (up to 1.8 s−1) and TON (1.9 × 104) over a 73 h reaction time at 876 mV overpotential (Beyene et al., 2015).
Oxygen Evolution Reaction Catalysts
OER is the half-reaction of WS providing the electrons and protons necessary for hydrogen liberation. It requires a complex series of multielectron/multiproton transfers and represents the thermodynamic and kinetic bottleneck of the overall process:
To guarantee good activity and durability, efficient water oxidation catalysts (WOCs) must be able to easily accumulate multiple charges at the metal center without triggering degradation processes. The nature of the metal center is therefore crucial and evolution of ancillary ligands for WOCs has followed rather specific routes for each catalytically active transition element (vide infra).
The possibility of using sacrificial oxidants (SO), like cerium ammonium nitrate (CAN) and NaIO4, to drive OER has largely facilitated the screening of molecular WOCs, to the point that relatively few electrochemical studies have been reported. It should be noted here that comparing catalytic activities is a delicate task, since it strongly depends on experimental conditions, like the nature of the sacrificial oxidant (e.g., CAN vs. NaIO4) (Codolà et al., 2015; Pokhrel et al., 2015; Bucci et al., 2016; Mazloomi et al., 2020) or the electrochemical method used (Diaz-Morales et al., 2014; Kottrup et al., 2018; Olivares et al., 2020; Van Dijk et al., 2020).
Noble metals like ruthenium and iridium have shown the overall most promising performances (Blakemore et al., 2015; Matheu et al., 2019), and this is why many groups are trying to develop WOCs with a “noble metal atom economy” approach to minimize the use of such precious elements (Ledendecker et al., 2019; Macchioni, 2019). Starting from the Meyer’s “blue dimer” cis-[(H2O)RuIII(bpy)2 (μ-O)RuIII(bpy)2(OH2)]4+ (19, Figure 4), the first well-characterized molecular WOC ever reported (Gersten et al., 1982), research initially focused on developing dinuclear Ru-WOCs, designed to favor O–O bond formation via intramolecular interaction of two metal-oxo (I2M) fragments (Romain et al., 2009). Although this design principle has sometimes proven flawed, since some dinuclear catalysts actually lead to O–O bond formation via water nucleophilic attack (WNA) at a single Ru=O center (Cape and Hurst, 2008; Liu et al., 2008; Romain et al., 2009; Neudeck et al., 2014) or other more peculiar pathways (Ertem and Concepcion, 2020), it provided some interesting catalyst families like those featuring 3,5-bis(2-pyridyl)pyrazolate (Sens et al., 2004) and rigid polypyridyl (Zong and Thummel, 2005; Xu et al., 2010; Kagalwala et al., 2021) ligands. Complexes 20 and 21, reported by the groups of Llobet and Thummel respectively, are two notable examples of such a class of catalysts. 20 catalyses the oxidation of water to molecular oxygen driven by CAN with a TOF value of 0.014 s−1 and TON of 512, whereas 21 showed a higher TOF value of 0.046 s−1 and a TON of 538 under similar conditions.
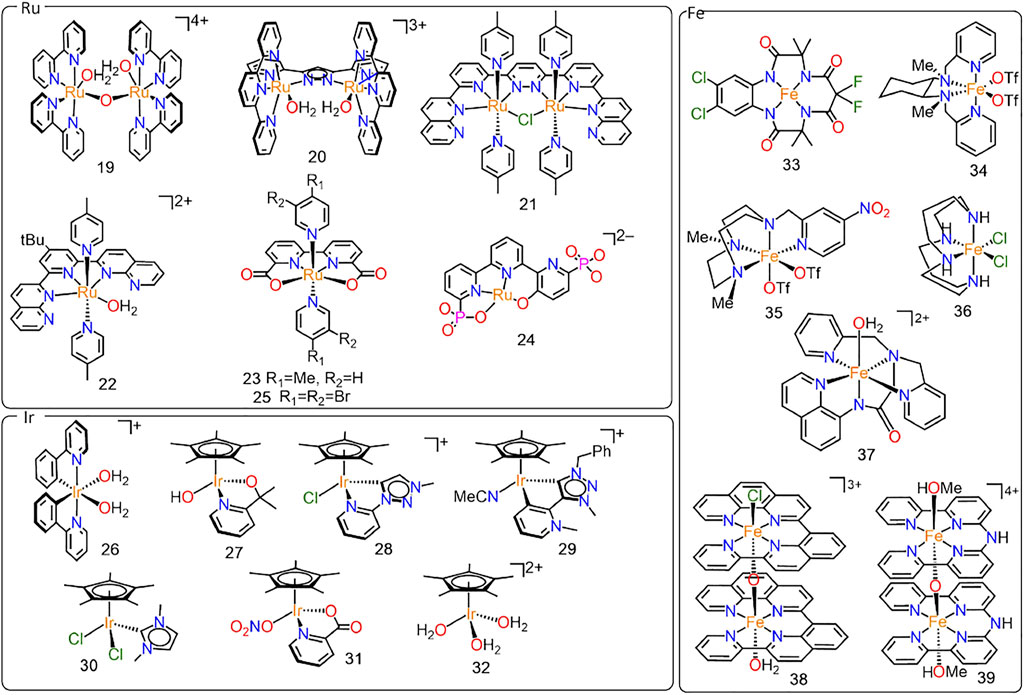
FIGURE 4. Structures of ruthenium (19–25), iridium (26–32) and iron (33–39) based water oxidation catalysts.
An important breakthrough in the field occurred in 2005, when Thummel and coworkers reported for the first time a series of mononuclear Ru complexes displaying water-oxidation activity (Zong and Thummel, 2005). In particular, the complex 22 with the methyl substituent showed the best performances reaching a TOF value of 0.014 s−1 and TON of 580 using CAN as sacrificial oxidant. Since then, the growing awareness that “one site is enough” (Concepcion et al., 2008) allowed to speed up Ru-catalyst development by focusing on simpler monomolecular species. Pyridyl based ligands were widely used (Wasylenko et al., 2010; Yagi et al., 2011; Kaveevivitchai et al., 2012; Kimoto et al., 2012; Tamaki et al., 2014). However, significant improvement in the catalytic activity for water oxidation was not achieved until the introduction of carboxylate-containing ligands into the catalyst structure, lowering the oxidation potential of Ru(II) to Ru(III). The family of Ru-bda catalysts, introduced by the group of Sun (Duan et al., 2009, 2012; Zhang and Sun, 2019b), is a most notable example (bda = 2,2′-bipyridine-6,6′-dicarboxylate). Complex 23 with the methyl substituent exhibits good catalytic performances in WO driven by CAN (Wang L. et al., 2012), and under electro- and photochemical conditions (Duan et al., 2010). The stabilizing effect of the introduced anionic carboxylate fragments appeared crucial to obtaining high activities (TON of 2000 and TOF of 41 s−1 using CAN as SO and 180 mV overpotential in electrocatalytic WO) (Duan et al., 2009; Wang et al., 2012b).
Several bda-ligand variations have been proposed to tune the redox properties of the metal center and/or the relay properties of the ancillary ligand (Matheu et al., 2015; Shaffer et al., 2017; Zhan et al., 2019; Vereshchuk et al., 2020; Yang et al., 2021). In particular, in complex 24 the use of phosphonate rather than carboxylate groups allowed to achieve a record TOF of 16000 s−1at pH = 7 with an overpotential of 530 mV (upon in situ ligand oxidation) (Vereshchuk et al., 2020). Also the axial ligands have drawn significant attention, due to their ability to facilitate Ru=O coupling by establishing intermolecular π-π stacking (Duan et al., 2013; Richmond et al., 2014; Wang et al., 2014; Creus et al., 2016; Fan et al., 2016; Xie et al., 2018) or, in some specific cases, electrostatic (Yi et al., 2021) interactions.
It should be noted, however, that such astonishingly high activities are often obtained at rather high catalyst concentrations, in the order of 10−3–10−5 M, to minimize the kinetic limitations of the bimolecular I2M mechanism that is characteristic of this catalyst class. Interestingly, Sun and coworkers have recently shown that the use of asymmetrically substituted pyridine axial ligands improves π-π stacking interactions, mitigating concentrations effects on activity; for instance, the TOF of the 3,4-dibromopyridine catalyst 25 drops from 460 s−1at [Ru] = 100 µM to a still noticeable TOF of 31 s−1at [Ru] = 1 µM in CAN-driven OER (Timmer et al., 2021). Alternative and more elaborate strategies have been proposed, for instance, by Reek and coworkers who exploited self-assembled nanospheres to increase local catalyst concentration (Yu et al., 2018). High activities at low concentrations can be achieved also with supramolecular Ru-bda macrocycles, following a WNA rather than I2M mechanism for reasons that remain to be fully understood and possibly related to the reduced mobility of the metal complexes in solution (Schulze et al., 2016).
The development of Ir-WOCs has been at least as prolific as that of Ru-based ones (Thomsen et al., 2015). Even though the first example, reported by Bernhard and coworkers in 2008, features two phenylpyridine ligands coordinated at an octahedral metal center (complex 26) (McDaniel et al., 2008), the evolution of molecular Ir-WOCs has mostly focused on pseudo-tetrahedral Cp∗-complexes, first reported by Crabtree and coworkers in 2009 (Hull et al., 2009). A plethora of Ir-Cp∗ complexes with (mostly bidentate) ligands has been proposed as water oxidation catalysts. To name but a few, these include complex 27 bearing the 2-(2′pyridyl)-2-propanolate (pyalc) ligand proposed by Crabtree and coworkers (Sharninghausen et al., 2016; Yang et al., 2016; Michaelos et al., 2017), complexes 28, 29 and 30 with different carbene ligands introduced by the groups of Albrecht, Bernhard and Hetterscheid (Lalrempuia et al., 2010; Hetterscheid and Reek, 2011; Venturini et al., 2014; Woods et al., 2014; Navarro et al., 2018; Vivancos et al., 2018), and complex 31 bearing the pyridine-carboxylate (pica) fragment introduced by Macchioni and coworkers (Bucci et al., 2012; Menendez Rodriguez et al., 2017a; Van Dijk et al., 2020; Rodriguez et al., 2021).
Comparing activity of these systems is difficult, as discussed above. Rather few electrochemical studies have been reported for molecular Ir-WOCs. The simple [Cp∗Ir(H2O)3]2+ (32) (Blakemore et al., 2011), as well as many other Cp∗ and non-Cp∗ catalysts (Van Dijk et al., 2020), easily undergo degradation to oxide nanoparticles under electrochemical OER conditions. Yet, 27, having a strongly electron donating and oxidation resistant pyridine-alkolate ligand, was found to remain homogenous under electrocatalytic WO conditions up to 680 mV overpotential (Schley et al., 2011), although some particle formation has been observed in chronoamperometric studies at higher potentials (670 mV) (Van Dijk et al., 2020). Also some pyridyl-triazolylidene Ir-WOCs remain molecular entities and deliver a stable 120 µA current at 1.8 V vs. RHE; only minor decomposition to catalytically inactive nanoparticles occurs (Olivares et al., 2020).
Catalytic performances in WO driven by sacrificial oxidants have been more widely explored, and some benchmarking studies are available with both CAN (Koelewijn et al., 2016) and NaIO4 (Menendez Rodriguez et al., 2017b). In particular, the most active molecular Ir-WOCs in combination with NaIO4 appear to be the simplest [Cp∗Ir(H2O)3]2+ (TOF = 7 s−1 in NaIO4-driven WO) and [Ir(OH)6]2- (TOF = 9 s−1), (Menendez Rodriguez et al., 2017b), even though the actual molecular vs. heterogeneous nature of the latter species has been debated (Savini et al., 2010; Hintermair et al., 2012; Menendez Rodriguez et al., 2017b).
This observation relates to a key issue in Ir-catalyzed WO, that is, the identification of the active species. Several spectroscopic studies revealed that catalyst activation involves in situ oxidative degradation of the Cp∗ (Savini et al., 2011; Wang et al., 2012a; Hintermair et al., 2013; Ingram et al., 2014; Zuccaccia et al., 2014; Sharninghausen et al., 2016; Yang et al., 2016). This leads to small molecules like acetic and formic acid, which are released in the reaction medium, and oxygenated fragments like substituted acetylacetonates, which likely remain coordinated at iridium. Strong experimental evidence supporting the latter hypothesis has been reported in a recent catalytic study on differently substituted Cp’-WOCs, demonstrating that intrinsic catalyst activity depends on the substituent on the Cp’ fragment (Gatto et al., 2021).
The fate of the non-Cp∗ ligands is far less explored. The above mentioned ligands are more oxidation resistant than Cp∗ and often assumed to remain coordinated at iridium during catalysis (Sharninghausen et al., 2016; Yang et al., 2016; Hu et al., 2021). However, as elegantly demonstrated by Fukuzumi and co-workers, even robust ligands like bipyridine can undergo decomposition if decorated with attackable substituents like–OH (Hong et al., 2012). Furthermore, albeit not degraded under OER conditions, differently substituted X-pica ligands likely detach from iridium upon catalyst activation (Rodriguez et al., 2021); this implies that [Cp∗Ir (X-pica)]+ complexes lead to the same active species formed with the simpler [Cp∗Ir(H2O)3]2+, with variations in catalytic activity being determined by the ease of ligand detachment. It remains to be proven whether this applies also to other types of ancillary ligands, but it is worth mentioning that this might explain the difficulties in developing Ir-WOCs more active than [Cp∗Ir(H2O)3]2+.
Along with Ru and Ir, several WOCs based on earth-abundant elements have been reported (Kärkäs and Åkermark, 2016; Lloret-Fillol and Costas, 2019; Kondo et al., 2021; Zhang et al., 2021). Initially, efforts focused on Mn (Najafpour et al., 2016), aiming at mimicking the Mn/Ca cubane active complex of natural Photosystem II (Kanaby et al., 2011; Kanady et al., 2014). Then, other elements have drawn significant attention, such as Co (Artero et al., 2011; Du and Eisenberg, 2012), Ni (Du and Eisenberg, 2012; Hessels et al., 2020a), Cu (Lee et al., 2020), and Fe (Du and Eisenberg, 2012; Casadevall et al., 2019; Liu et al., 2019). Herein, the most extensively studied metal, iron, is discussed as representative case.
Despite some early indications in the 1980s (Elizarova et al., 1981), the search for Fe-WOCs sparked in 2010 with the report on iron-centered tetraamido macrocyclic ligands (Fe-TAML) by Bernhard and Collins (Ellis et al., 2010). Among all, complex 33 exhibits the highest activity but very poor durability (TOF = 1.3 s−1; TON = 10), which could be somewhat improved using a Biuret-Modified ligand (TOF = 0.8 s−1; TON = 220 under photochemical conditions) (Panda et al., 2014).
The larger family of well-defined Fe-WOCs is based on non-heme, multidentate aminopyridine ligands (Codolà et al., 2013, 2019; Zhang B. et al., 2014; To et al., 2015; D’Agostini et al., 2021). For instance, complex 34 bearing the N,N′-bis(pyridin-2-ylmethyl)-cyclohexane-1,2-diamine (mcp) ligand have proved to be rather stable against decomposition in chemically driven WO (Fillol et al., 2011), but also under electrochemical conditions with an onset overpotential of 0.47 V (D’Agostini et al., 2021). 1-(2′ -pyridylmethyl)-1,4,7-triazacyclononane (pytacn) ligands have been widely studied showing how activity increases with electron withdrawing substituents in para position of the pyridine ring and non-hindering substituents in ortho (Codolà et al., 2013; D’Agostini et al., 2021). Complex 35 bearing the nitro substituted pyridine showed the best performances in this series with a TOF of 0.23 s-1 and TON of 180. Importantly, in order to be active, all these Fe(NNNN) complexes require the two labile ligands to be in cis-position with each other, as nicely demonstrated by Hetterscheid and co-workers by comparing cis-[Fe (cyclam)Cl2]Cl (cyclam = 1,4,8,11-tetraazacyclotetradecane; 36), which catalyzes WO at 0.47 V overpotential, and its trans-analogue, which is inactive in OER (Kottrup and Hetterscheid, 2016).
Aiming at improving the stability of high valent intermediates, Meyer and coworkers also proposed to use anionic rather than neutral tetradentate ligands, like bis(pyridine-2-ylmethyl)]amino-N-quinolin-8-yl-acetamido. The resulting complex 37 was found to be an effective electrocatalyst in propylene carbonate–water mixtures (Coggins et al., 2014), but not in aqueous solutions (Zhang B. et al., 2014).
Di- or even multi-metallic catalysts have been reported as well, aiming at facilitating the rate-limiting O–O bond formation as discussed above for Ru-WOCs (Panda et al., 2014; Das et al., 2016; Okamura et al., 2016; Kottrup et al., 2018). These include, for instance, the iron-tetrapyridyl electrocatalysts 38 and 39, first reported by the group of Tummel (Wickramasinghe et al., 2015), exhibiting some of the lowest overpotentials among Fe-WOCs of 300–400 mV (Wickramasinghe et al., 2015; Kottrup et al., 2018).
Concluding Remarks and Outlook
The versatility of molecular catalysts clearly emerges from the results summarized in the above sections. In particular, it appears evident that ancillary ligand modification represents an effective handle for properties tuning, which still offers large possibilities for further improvement. Establishing straightforward structure-properties correlations remains arduous at this stage, the main hurdle being the limited understanding of reaction mechanisms and (pre)catalyst speciation. Nevertheless, some successful examples of ligand tailoring might help orienting future research, like DuBois’ aza-diphosphino cyclic ligands with their proton relay properties (Helm et al., 2011) or the axial ligands establishing π-π stacking interactions in Ru-bda catalysts (Duan et al., 2013; Richmond et al., 2014; Wang et al., 2014; Creus et al., 2016; Fan et al., 2016; Xie et al., 2018). Increasing the currently very limited number of systematic catalyst screenings to buildup robust and consistent experimental databases is essential in this respect (Garrido-Barros et al., 2017; Craig et al., 2019; Craig and García-Melchor, 2021; Kondo et al., 2021). Improving activity/lowering the necessary overpotential is still an open challenge in HER and especially in OER. Nevertheless, it should be emphasized that rather high maximum TOFs are now accessible with the latest generation catalysts, and it is reasonable to expect that ligand design can quite easily lead to further improvement. Indeed, rather than catalytic activity, the biggest challenge for molecular catalysts regards stability (Limburg et al., 2012).
OER is more problematic than HER in this respect, due to the harsher reaction conditions needed in the former case. In situ ligands modifications are very common under the strongly oxidative reaction conditions necessary for both chemical and photo/electrochemical WO. Often, they are functional to the generation of the active species, as in the above discussed case of Ir-WOCs (Savini et al., 2011; Wang et al., 2012a; Hintermair et al., 2013; Ingram et al., 2014; Zuccaccia et al., 2014; Sharninghausen et al., 2016; Yang et al., 2016; Gatto et al., 2021), but also for ruthenium (Liu et al., 2014, 2018; Lòpez et al., 2014; Akhtar et al., 2016; Kagalwala et al., 2017; Vereshchuk et al., 2020) and earth-abundant catalysts (Hong et al., 2013; Kottrup and Hetterscheid, 2016; Codolà et al., 2019). In many other cases, however, they are strongly detrimental, typically leading to the formation of metal oxide nanoparticles. A relevant example is the pentanuclear iron complex reported by Masaoka and coworkers in 2016 (Okamura et al., 2016), whose catalytic activity was later attributed to iron oxide material deposited on the electrode surface (Pelosin et al., 2020). This is extremely problematic for earth-abundant WOCs (Chen et al., 2013; Parent et al., 2014; Hessels et al., 2020b), but certainly non-negligible also in the case of noble metals (Depasquale et al., 2013; Lewandowska-Andralojc et al., 2014; Van Dijk et al., 2020). The use of strongly electron donating, polydentate ligands has proven effective in mitigating this problem for Fe-WOCs (Casadevall et al., 2019), and similarly positive results have been obtained, for instance, with the pyalc ligand for Ir-WOCs (Schley et al., 2011); yet, catalyst stability remains difficult to optimize and still unsatisfactory for commercial applications.
It is worth emphasizing here that preventing and/or understanding catalyst in situ modifications would also facilitate the identification of rational design principles. The abovementioned results on Cp∗Ir-WOCs probably represent a most advanced, yet still intricate, case in this respect. By combining the information derived from several spectroscopic and kinetic studies (Savini et al., 2011; Wang et al., 2012a; Hong et al., 2012; Hintermair et al., 2013; Ingram et al., 2014; Zuccaccia et al., 2014; Sharninghausen et al., 2016; Yang et al., 2016; Gatto et al., 2021; Hu et al., 2021; Rodriguez et al., 2021), some important hints on the structure of the active species emerge: it is likely a multimetallic oxo-species containing fragments deriving from Cp∗ oxidative degradation and, at least in most cases, no other ancillary ligands of the parental precatalysts (Yang et al., 2016; Gatto et al., 2021; Rodriguez et al., 2021). This hypothesis of a multimetallic, possibly nanocluster, active species calls for a strong integration of homogenous and heterogeneous catalysis approaches (Li et al., 2021).
Regarding the applications of molecular catalysts, another important aspect to consider is the integration of these systems in photo/electrochemical devices for large scale hydrogen production. All the above discussed examples refer to molecular catalysts in homogenous phase in laboratory scale experiments, but these conditions can be hardly applied in industrial plants. First, the use of a molecular catalyst in electrolytic solution would be limited by only a small fraction of the catalyst being active in the diffusion layer around the electrode. Second, it would hamper the recovery and recycle of active metal, which is particularly important for noble metals.
Effective strategies for the heterogenization are therefore needed for molecular catalysts to become suitable for commercial applications (Materna et al., 2017; Rosser and Reisner, 2017; Zhang and Sun, 2019a; Macchioni, 2019; Zahran et al., 2019; Xiao et al., 2021; Yi et al., 2021). They require elaborate optimization of the composition, morphology and particle size of the support, as well as engineering of the ancillary ligand for anchoring the organometallic complex; compatibilization with the electrode material is another critical aspect.
Several notable examples of heterogenized catalysts have been reported in both HER and OER. To name but a few, Artero and coworkers effectively grafted a diimine–dioxime cobalt complex onto the surface of a carbon nanotube electrode, catalyzing HER with a TON of 55000 at an overpotential of 350 mV in aqueous solutions (Andreiadis et al., 2013); even better performances were obtained with a nickel bisdiphosphine catalyst supported on multiwalled carbon nanotubes, reaching 100,000 turnovers at 20 mV overpotential in aqueous sulfuric acid solution (Alan et al., 2009). Alternative supports have also been reported, like metal organic surfaces (Clough et al., 2015). In OER, metal oxide supports are commonly used, and proved successful for instance in combination with a dimeric Ir-pyalc catalyst (TON >1 × 106at 250 mV overpotential) (Sheehan et al., 2015), Ir-bipyridine (TON >5 × 105 and TOF >6.7 s−1at 280 mV overpotential) (Joya et al., 2012) catalysts. Also IrCp∗-N-dimethylimidazolin-2-ylidene catalysts immobilized on a polycrystalline gold electrode exhibited good stability with a TOF of 2.9 s−1at an applied overpotential of 430 mV (Diaz-Morales et al., 2014). It is worth mentioning that molecular catalysts can be also used to enhance the performance of photoactive materials like BiVO4 (Wan et al., 2018) and WO3 (Klepser and Bartlett, 2014), or simply serve as well-defined precursors for the controlled deposition of metal atoms in the electrode surface.
These examples show how integrating molecular complexes with suitable supports represents a frontier in catalysis for water splitting. The close cooperation between material scientists and organometallic chemists will guarantee significant progress in this field.
Author Contributions
AM designed the review discussing it with all authors. AM and FZ wrote most of the paper. All authors contributed in finding relevant literature results to illustrate in the paper.
Conflict of Interest
The authors declare that the research was conducted in the absence of any commercial or financial relationships that could be construed as a potential conflict of interest.
Publisher’s Note
All claims expressed in this article are solely those of the authors and do not necessarily represent those of their affiliated organizations, or those of the publisher, the editors and the reviewers. Any product that may be evaluated in this article, or claim that may be made by its manufacturer, is not guaranteed or endorsed by the publisher.
Acknowledgments
The University of Perugia and MIUR (AMIS, “Dipartimenti di Eccellenza—2018–2022” programs) are gratefully acknowledged for financial support.
Abbreviations
WS, water splitting; OER, oxygen evolving reaction; HER, hydrogen evolving reaction; WO, water oxidation; WOC, water oxidation catalysts; TON, turnover number; TOF, turnover frequency; Cp∗, pentamethylcyclopentadienyl; NADPH, nicotinamide adenine dinucleotide phosphate; PEC, photoelectrochemical cell; PVE, photovoltaic-coupled electrolyzer; DMF, dimthylformamide; PCET, proton-coupled electron transfer; SO, sacrificial oxidant; CAN, cerium ammonium nitrate; bda, 2,2′-bipyridine-6,6′-dicarboxylate; pyalc, 2-(2′pyridyl)-2-propanolate.
References
Ager, J. W., Shaner, M. R., Walczak, K. A., Sharp, I. D., and Ardo, S. (2015). Experimental Demonstrations of Spontaneous, Solar-Driven Photoelectrochemical Water Splitting. Energy Environ. Sci. 8, 2811–2824. doi:10.1039/c5ee00457h
Aimoto, Y., Koshiba, K., Yamauchi, K., and Sakai, K. (2018). A Family of Molecular Nickel Hydrogen Evolution Catalysts Providing Tunable Overpotentials Using Ligand-Centered Proton-Coupled Electron Transfer Paths. Chem. Commun. 54, 12820–12823. doi:10.1039/C8CC07467D
Akhtar, U. S., Tae, E. L., Chun, Y. S., Hwang, I. C., and Yoon, K. B. (2016). Insights into Decomposition Pathways and Fate of Ru(bpy)32+ during Photocatalytic Water Oxidation with S2O82- as Sacrificial Electron Acceptor. ACS Catal. 6, 8361–8369. doi:10.1021/acscatal.6b02595
Amaro-Gahete, J., Pavliuk, M. V. M., Tian, H., Esquivel, D., Romero-Salguero, F. J., and Ott, S. (2021). Catalytic Systems Mimicking the [FeFe]-Hydrogenase Active Site for Visible-Light-Driven Hydrogen Production. Coord. Chem. Rev. 448, 214172. doi:10.1016/j.ccr.2021.214172
Andreiadis, E. S., Jacques, P.-A., Tran, P. D., Leyris, A., Chavarot-Kerlidou, M., Jousselme, B., et al. (2013). Molecular Engineering of a Cobalt-Based Electrocatalytic Nanomaterial for H2 Evolution under Fully Aqueous Conditions. Nat. Chem 5, 48–53. doi:10.1038/nchem.1481
Armaroli, N., and Balzani, V. (2007). The Future of Energy Supply: Challenges and Opportunities. Angew. Chem. Int. Ed. 46, 52–66. doi:10.1002/anie.200602373
Artero, V., Chavarot-Kerlidou, M., and Fontecave, M. (2011). Splitting Water with Cobalt. Angew. Chem. Int. Ed. 50, 7238–7266. doi:10.1002/anie.201007987
Barrozo, A., and Orio, M. (2019). Molecular Electrocatalysts for the Hydrogen Evolution Reaction: Input from Quantum Chemistry. ChemSusChem 12, 4905–4915. doi:10.1002/cssc.201901828
Barton, B. E., and Rauchfuss, T. B. (2010). Hydride-Containing Models for the Active Site of the Nickel−Iron Hydrogenases. J. Am. Chem. Soc. 132, 14877–14885. doi:10.1021/ja105312p
Begum, A., Moula, G., and Sarkar, S. (2010). A Nickel(II)-Sulfur‐Based Radical‐Ligand Complex as a Functional Model of Hydrogenase. Chem. Eur. J. 16, 12324–12327. doi:10.1002/CHEM.201001812
Berardi, S., Drouet, S., Francàs, L., Gimbert-Suriñach, C., Guttentag, M., Richmond, C., et al. (2014). Molecular Artificial Photosynthesis. Chem. Soc. Rev. 43, 7501–7519. doi:10.1039/c3cs60405e
Beyene, B. B., Mane, S. B., and Hung, C.-H. (2015). Highly Efficient Electrocatalytic Hydrogen Evolution from Neutral Aqueous Solution by a Water-Soluble Anionic Cobalt(II) Porphyrin. Chem. Commun. 51, 15067–15070. doi:10.1039/c5cc05582b
Blakemore, J. D., Crabtree, R. H., and Brudvig, G. W. (2015). Molecular Catalysts for Water Oxidation. Chem. Rev. 115, 12974–13005. doi:10.1021/acs.chemrev.5b00122
Blakemore, J. D., Schley, N. D., Olack, G. W., Incarvito, C. D., Brudvig, G. W., and Crabtree, R. H. (2011). Anodic Deposition of a Robust Iridium-Based Water-Oxidation Catalyst from Organometallic Precursors. Chem. Sci. 2, 94–98. doi:10.1039/C0SC00418A
Bucci, A., Menendez Rodriguez, G., Bellachioma, G., Zuccaccia, C., Poater, A., Cavallo, L., et al. (2016). An Alternative Reaction Pathway for Iridium-Catalyzed Water Oxidation Driven by Cerium Ammonium Nitrate (CAN). ACS Catal. 6, 4559–4563. doi:10.1021/acscatal.6b01325
Bucci, A., Savini, A., Rocchigiani, L., Zuccaccia, C., Rizzato, S., Albinati, A., et al. (2012). Organometallic Iridium Catalysts Based on Pyridinecarboxylate Ligands for the Oxidative Splitting of Water. Organometallics 31, 8071–8074. doi:10.1021/om301024s
Cape, J. L., and Hurst, J. K. (2008). Detection and Mechanistic Relevance of Transient Ligand Radicals Formed during [Ru(bpy)2(OH2)]2O4+-Catalyzed Water Oxidation. J. Am. Chem. Soc. 130, 827–829. doi:10.1021/ja077276s
Capon, J.-F., Gloaguen, F., Pétillon, F. Y., Schollhammer, P., and Talarmin, J. (2009). Electron and Proton Transfers at Diiron Dithiolate Sites Relevant to the Catalysis of Proton Reduction by the [FeFe]-Hydrogenases. Coord. Chem. Rev. 253, 1476–1494. doi:10.1016/J.CCR.2008.10.020
Capon, J., Gloaguen, F., Schollhammer, P., and Talarmin, J. (2005). Catalysis of the Electrochemical H Evolution by Di-iron Sub-site Models. Coord. Chem. Rev. 249, 1664–1676. doi:10.1016/J.CCR.2004.11.018
Casadevall, C., Bucci, A., Costas, M., and Lloret-Fillol, J. (2019). Water Oxidation Catalysis with Well-Defined Molecular Iron Complexes. Adv. Inorg. Chem. 74, 151–196. doi:10.1016/bs.adioch.2019.03.004
Chen, G., Chen, L., Ng, S.-M., Man, W.-L., and Lau, T.-C. (2013). Chemical and Visible-Light-Driven Water Oxidation by Iron Complexes at pH 7-9: Evidence for Dual-Active Intermediates in Iron-Catalyzed Water Oxidation. Angew. Chem. Int. Ed. 52, 1789–1791. doi:10.1002/anie.201209116
Clough, A. J., Yoo, J. W., Mecklenburg, M. H., and Marinescu, S. C. (2015). Two-Dimensional Metal-Organic Surfaces for Efficient Hydrogen Evolution from Water. J. Am. Chem. Soc. 137, 118–121. doi:10.1021/ja5116937
Codolà, Z., Gamba, I., Acuña-Parés, F., Casadevall, C., Clémancey, M., Latour, J.-M., et al. (2019). Design of Iron Coordination Complexes as Highly Active Homogenous Water Oxidation Catalysts by Deuteration of Oxidation-Sensitive Sites. J. Am. Chem. Soc. 141, 323–333. doi:10.1021/jacs.8b10211
Codolà, Z., Garcia-Bosch, I., Acuña-Parés, F., Prat, I., Luis, J. M., Costas, M., et al. (2013). Electronic Effects on Single-Site Iron Catalysts for Water Oxidation. Chem. Eur. J. 19, 8042–8047. doi:10.1002/chem.201301112
Codolà, Z., Gómez, L., Kleespies, S. T., Que Jr, L., Costas, M., and Lloret-Fillol, J. (2015). Evidence for an Oxygen Evolving Iron-Oxo-Cerium Intermediate in Iron-Catalysed Water Oxidation. Nat. Commun. 6, 5865. doi:10.1038/ncomms6865
Coggins, M. K., Zhang, M.-T., Vannucci, A. K., Dares, C. J., and Meyer, T. J. (2014). Electrocatalytic Water Oxidation by a Monomeric Amidate-Ligated Fe(III)-Aqua Complex. J. Am. Chem. Soc. 136, 5531–5534. doi:10.1021/ja412822u
Concepcion, J. J., Jurss, J. W., Templeton, J. L., and Meyer, T. J. (2008). One Site Is Enough. Catalytic Water Oxidation by [Ru(tpy)(bpm)(OH2)]2+ and [Ru(tpy)(bpz)(OH2)]2+. J. Am. Chem. Soc. 130, 16462–16463. doi:10.1021/ja8059649
Crabtree, G. W., Dresselhaus, M. S., and Buchanan, M. V. (2004). The Hydrogen Economy. Phys. Today 57, 39–44. doi:10.1063/1.1878333
Craig, M. J., Coulter, G., Dolan, E., Soriano-López, J., Mates-Torres, E., Schmitt, W., et al. (2019). Universal Scaling Relations for the Rational Design of Molecular Water Oxidation Catalysts with Near-Zero Overpotential. Nat. Commun. 10, 4993. doi:10.1038/s41467-019-12994-w
Craig, M. J., and García-Melchor, M. (2021). High-throughput Screening and Rational Design to Drive Discovery in Molecular Water Oxidation Catalysis. Cel Rep. Phys. Sci. 2, 100492. doi:10.1016/j.xcrp.2021.100492
Creus, J., Matheu, R., Peñafiel, I., Moonshiram, D., Blondeau, P., Benet-Buchholz, J., et al. (2016). A Million Turnover Molecular Anode for Catalytic Water Oxidation. Angew. Chem. Int. Ed. 55, 15382–15386. doi:10.1002/anie.201609167
D’Agostini, S., Kottrup, K. G., Casadevall, C., Gamba, I., Dantignana, V., Bucci, A., et al. (2021). Electrocatalytic Water Oxidation with α-[Fe(mcp)(OTf)2] and Analogues. ACS Catal. 11, 2583–2595. doi:10.1021/acscatal.0c05439
Das, D., Pattanayak, S., Singh, K. K., Garai, B., and Sen Gupta, S. (2016). Electrocatalytic Water Oxidation by a Molecular Cobalt Complex through a High Valent Cobalt Oxo Intermediate. Chem. Commun. 52, 11787–11790. doi:10.1039/C6CC05773J
Depasquale, J., Nieto, I., Reuther, L. E., Herbst-Gervasoni, C. J., Paul, J. J., Mochalin, V., et al. (2013). Iridium Dihydroxybipyridine Complexes Show that Ligand Deprotonation Dramatically Speeds Rates of Catalytic Water Oxidation. Inorg. Chem. 52, 9175–9183. doi:10.1021/ic302448d
Diaz-Morales, O., Hersbach, T. J. P., Hetterscheid, D. G. H., Reek, J. N. H., and Koper, M. T. M. (2014). Electrochemical and Spectroelectrochemical Characterization of an Iridium-Based Molecular Catalyst for Water Splitting: Turnover Frequencies, Stability, and Electrolyte Effects. J. Am. Chem. Soc. 136, 10432–10439. doi:10.1021/ja504460w
Du, P., and Eisenberg, R. (2012). Catalysts Made of Earth-Abundant Elements (Co, Ni, Fe) for Water Splitting: Recent Progress and Future Challenges. Energ. Environ. Sci. 5, 6012–6021. doi:10.1039/C2EE03250C
Duan, L., Bozoglian, F., Mandal, S., Stewart, B., Privalov, T., Llobet, A., et al. (2012). A Molecular Ruthenium Catalyst with Water-Oxidation Activity Comparable to that of Photosystem II. Nat. Chem 4, 418–423. doi:10.1038/nchem.1301
Duan, L., Fischer, A., Xu, Y., and Sun, L. (2009). Isolated Seven-Coordinate Ru(IV) Dimer Complex with [HOHOH]− Bridging Ligand as an Intermediate for Catalytic Water Oxidation. J. Am. Chem. Soc. 131, 10397–10399. doi:10.1021/ja9034686
Duan, L., Wang, L., Inge, A. K., Fischer, A., Zou, X., and Sun, L. (2013). Insights into Ru-Based Molecular Water Oxidation Catalysts: Electronic and Noncovalent-Interaction Effects on Their Catalytic Activities. Inorg. Chem. 52, 7844–7852. doi:10.1021/ic302687d
Duan, L., Xu, Y., Zhang, P., Wang, M., and Sun, L. (2010). Visible Light-Driven Water Oxidation by a Molecular Ruthenium Catalyst in Homogeneous System. Inorg. Chem. 49, 209–215. doi:10.1021/ic9017486
Dutta, A., DuBois, D. L., Roberts, J. A. S., and Shaw, W. J. (2014). Amino Acid Modified Ni Catalyst Exhibits Reversible H 2 Oxidation/production over a Broad pH Range at Elevated Temperatures. Proc. Natl. Acad. Sci. U.S.A. 111, 16286–16291. doi:10.1073/pnas.1416381111
Eckenhoff, W. T., and Eisenberg, R. (2012). Molecular Systems for Light Driven Hydrogen Production. Dalton Trans. 41, 13004–13021. doi:10.1039/c2dt30823a
Eckenhoff, W. T. (2018). Molecular Catalysts of Co, Ni, Fe, and Mo for Hydrogen Generation in Artificial Photosynthetic Systems. Coord. Chem. Rev. 373, 295–316. doi:10.1016/j.ccr.2017.11.002
Elizarova, G. L., Matvienko, L. G., Lozhkina, N. V., Maizlish, V. E., and Parmon, V. N. (1981). Homogeneous Catalysts for Dioxygen Evolution from Water. Oxidation of Water by Trisbipyridylruthenium(III) in the Presence of Metallophthalocyanines. React. Kinet. Catal. Lett. 16, 285–288. doi:10.1007/BF02065474
Ellis, W. C., McDaniel, N. D., Bernhard, S., and Collins, T. J. (2010). Fast Water Oxidation Using Iron. J. Am. Chem. Soc. 132, 10990–10991. doi:10.1021/ja104766z
Ertem, M. Z., and Concepcion, J. J. (2020). Oxygen Atom Transfer as an Alternative Pathway for Oxygen-Oxygen Bond Formation. Inorg. Chem. 59, 5966–5974. doi:10.1021/acs.inorgchem.9b03751
Esswein, A. J., and Nocera, D. G. (2007). Hydrogen Production by Molecular Photocatalysis. Chem. Rev. 107, 4022–4047. doi:10.1021/cr050193e
European Commission (2020). A Hydrogen Strategy for a Climate-Neutral Europe. Available at: https://ec.europa.eu/energy/sites/ener/files/hydrogen_strategy.pdf.
Fan, T., Zhan, S., and Ahlquist, M. S. G. (2016). Why Is There a Barrier in the Coupling of Two Radicals in the Water Oxidation Reaction? ACS Catal. 6, 8308–8312. doi:10.1021/acscatal.6b02697
Fillol, J. L., Codolà, Z., Garcia-Bosch, I., Gómez, L., Pla, J. J., and Costas, M. (2011). Efficient Water Oxidation Catalysts Based on Readily Available Iron Coordination Complexes. Nat. Chem 3, 807–813. doi:10.1038/nchem.1140
Frey, M. (2002). Hydrogenases: Hydrogen-Activating Enzymes. ChemBioChem 3, 153–160. doi:10.1002/1439-7633(20020301)3:2/3<153::AID-CBIC153>3.0.CO;2-B
Gan, L., Groy, T. L., Tarakeshwar, P., Mazinani, S. K. S., Shearer, J., Mujica, V., et al. (2015). A Nickel Phosphine Complex as a Fast and Efficient Hydrogen Production Catalyst. J. Am. Chem. Soc. 137, 1109–1115. doi:10.1021/ja509779q
Garrido-Barros, P., Gimbert-Suriñach, C., Matheu, R., Sala, X., and Llobet, A. (2017). How to Make an Efficient and Robust Molecular Catalyst for Water Oxidation. Chem. Soc. Rev. 46, 6088–6098. doi:10.1039/c7cs00248c
Gatto, G., De Palo, A., Carrasco, A. C., Pizarro, A. M., Zacchini, S., Pampaloni, G., et al. (2021). Modulating the Water Oxidation Catalytic Activity of Iridium Complexes by Functionalizing the Cp∗-Ancillary Ligand: Hints on the Nature of the Active Species. Catal. Sci. Technol. 11, 2885–2895. doi:10.1039/d0cy02306j
Gersten, S. W., Samuels, G. J., and Meyer, T. J. (1982). Catalytic Oxidation of Water by an Oxo-Bridged Ruthenium Dimer. J. Am. Chem. Soc. 104, 4029–4030. doi:10.1021/ja00378a053
Gloaguen, F., Lawrence, J. D., and Rauchfuss, T. B. (2001). Biomimetic Hydrogen Evolution Catalyzed by an Iron Carbonyl Thiolate. J. Am. Chem. Soc. 123, 9476–9477. doi:10.1021/ja016516f
Griffiths, S., Sovacool, B. K., Kim, J., Bazilian, M., and Uratani, J. M. (2021). Industrial Decarbonization via Hydrogen: A Critical and Systematic Review of Developments, Socio-Technical Systems and Policy Options. Energ. Res. Soc. Sci. 80, 102208. doi:10.1016/j.erss.2021.102208
Helm, M. L., Stewart, M. P., Bullock, R. M., DuBois, M. R., and DuBois, D. L. (2011). A Synthetic Nickel Electrocatalyst with a Turnover Frequency above 100,000 S −1 for H 2 Production. Science 333, 863–866. doi:10.1126/science.1205864
Hessels, J., Masferrer‐Rius, E., Yu, F., Detz, R. J., Klein Gebbink, R. J. M., and Reek, J. N. H. (2020a). Nickel Is a Different Pickle: Trends in Water Oxidation Catalysis for Molecular Nickel Complexes. ChemSusChem 13, 6629–6634. doi:10.1002/cssc.202002164
Hessels, J., Yu, F., Detz, R. J., and Reek, J. N. H. (2020b). Potential‐ and Buffer‐Dependent Catalyst Decomposition during Nickel‐Based Water Oxidation Catalysis. ChemSusChem 13, 5625–5631. doi:10.1002/cssc.202001428
Hetterscheid, D. G. H., and Reek, J. N. H. (2011). Me2-NHC Based Robust Ir Catalyst for Efficient Water Oxidation. Chem. Commun. 47, 2712–2714. doi:10.1039/c0cc05108j
Hintermair, U., Hashmi, S. M., Elimelech, M., and Crabtree, R. H. (2012). Particle Formation during Oxidation Catalysis with Cp∗ Iridium Complexes. J. Am. Chem. Soc. 134, 9785–9795. doi:10.1021/ja3033026
Hintermair, U., Sheehan, S. W., Parent, A. R., Ess, D. H., Richens, D. T., Vaccaro, P. H., et al. (2013). Precursor Transformation during Molecular Oxidation Catalysis with Organometallic Iridium Complexes. J. Am. Chem. Soc. 135, 10837–10851. doi:10.1021/ja4048762
Hong, D., Mandal, S., Yamada, Y., Lee, Y.-M., Nam, W., Llobet, A., et al. (2013). Water Oxidation Catalysis with Nonheme Iron Complexes under Acidic and Basic Conditions: Homogeneous or Heterogeneous? Inorg. Chem. 52, 9522–9531. doi:10.1021/ic401180r
Hong, D., Murakami, M., Yamada, Y., and Fukuzumi, S. (2012). Efficient Water Oxidation by Cerium Ammonium Nitrate with [IrIII(Cp∗)(4,4′-Bishydroxy-2,2′-bipyridine)(H2O)]2+as a Precatalyst. Energ. Environ. Sci. 5, 5708–5716. doi:10.1039/C2EE02964B
Hou, K., and Fan, W. Y. (2014). Electrocatalytic Proton Reduction Catalyzed by a Dimanganese Disulfide Carbonyl Complex Containing a Redox-Active Internal Disulfide Bond. Dalton Trans. 43, 16977–16980. doi:10.1039/c4dt02361g
Hou, K., Poh, H. T., and Fan, W. Y. (2014). Electrocatalytic Hydrogen Generation by a Trithiolato-Bridged Dimanganese Hexacarbonyl Anion with a Turnover Frequency Exceeding 40 000 S−1. Chem. Commun. 50, 6630–6632. doi:10.1039/C4CC02016B
Hu, G., Troiano, J. L., Tayvah, U. T., Sharninghausen, L. S., Sinha, S. B., Shopov, D. Y., et al. (2021). Accessing Molecular Dimeric Ir Water Oxidation Catalysts from Coordination Precursors. Inorg. Chem. 60, 14349–14356. doi:10.1021/acs.inorgchem.1c02025
Hu, X., Cossairt, B. M., Brunschwig, B. S., Lewis, N. S., and Peters, J. C. (2005). Electrocatalytic Hydrogen Evolution by Cobalt Difluoroboryl-Diglyoximate Complexes. Chem. Commun. 37, 4723–4725. doi:10.1039/b509188h
Hull, J. F., Balcells, D., Blakemore, J. D., Incarvito, C. D., Eisenstein, O., Brudvig, G. W., et al. (2009). Highly Active and Robust Cp∗ Iridium Complexes for Catalytic Water Oxidation. J. Am. Chem. Soc. 131, 8730–8731. doi:10.1021/ja901270f
IEA (2020). Energy Technology Perspectives. Paris: International Energy Agency. Available at: https://www.iea.org/reports/the-future-of-hydrogen.
Ingram, A. J., Wolk, A. B., Flender, C., Zhang, J., Johnson, C. J., Hintermair, U., et al. (2014). Modes of Activation of Organometallic Iridium Complexes for Catalytic Water and C-H Oxidation. Inorg. Chem. 53, 423–433. doi:10.1021/ic402390t
Jaramillo, T. F., Jørgensen, K. P., Bonde, J., Nielsen, J. H., Horch, S., and Chorkendorff, I. (2007). Identification of Active Edge Sites for Electrochemical H 2 Evolution from MoS 2 Nanocatalysts. Science 317, 100–102. doi:10.1126/science.1141483
Joya, K. S., Subbaiyan, N. K., D'Souza, F., and de Groot, H. J. M. (2012). Surface-immobilized Single-Site Iridium Complexes for Electrocatalytic Water Splitting. Angew. Chem. Int. Ed. 51, 9601–9605. doi:10.1002/anie.201203560
Kagalwala, H. N., Deshmukh, M. S., Ramasamy, E., Nair, N., Zhou, R., Zong, R., et al. (2021). Revisiting Dinuclear Ruthenium Water Oxidation Catalysts: Effect of Bridging Ligand Architecture on Catalytic Activity. Inorg. Chem. 60, 1806–1813. doi:10.1021/acs.inorgchem.0c03281
Kagalwala, H. N., Tong, L., Zong, R., Kohler, L., Ahlquist, M. S. G., Fan, T., et al. (2017). Evidence for Oxidative Decay of a Ru-Bound Ligand during Catalyzed Water Oxidation. ACS Catal. 7, 2607–2615. doi:10.1021/acscatal.6b03278
Kanady, J. S., Lin, P.-H., Carsch, K. M., Nielsen, R. J., Takase, M. K., Goddard, W. A., et al. (2014). Toward Models for the Full Oxygen-Evolving Complex of Photosystem II by Ligand Coordination to Lower the Symmetry of the Mn3CaO4 Cubane: Demonstration that Electronic Effects Facilitate Binding of a Fifth Metal. J. Am. Chem. Soc. 136, 14373–14376. doi:10.1021/ja508160x
Kanady, J. S., Tsui, E. Y., Day, M. W., and Agapie, T. (2011). A Synthetic Model of the Mn 3 Ca Subsite of the Oxygen-Evolving Complex in Photosystem II. Science 333, 733–736. doi:10.1126/science.1206036
Kärkäs, M. D., and Åkermark, B. (2016). Water Oxidation Using Earth-Abundant Transition Metal Catalysts: Opportunities and Challenges. Dalton Trans. 45, 14421–14461. doi:10.1039/c6dt00809g
Kärkäs, M. D., Verho, O., Johnston, E. V., and Åkermark, B. (2014). Artificial Photosynthesis: Molecular Systems for Catalytic Water Oxidation. Chem. Rev. 114, 11863–12001. doi:10.1021/cr400572f
Karunadasa, H. I., Chang, C. J., and Long, J. R. (2010). A Molecular Molybdenum-Oxo Catalyst for Generating Hydrogen from Water. Nature 464, 1329–1333. doi:10.1038/nature08969
Karunadasa, H. I., Montalvo, E., Sun, Y., Majda, M., Long, J. R., and Chang, C. J. (2012). A Molecular MoS 2 Edge Site Mimic for Catalytic Hydrogen Generation. Science 335, 698–702. doi:10.1126/science.1215868
Kaveevivitchai, N., Zong, R., Tseng, H.-W., Chitta, R., and Thummel, R. P. (2012). Further Observations on Water Oxidation Catalyzed by Mononuclear Ru(II) Complexes. Inorg. Chem. 51, 2930–2939. doi:10.1021/ic202174j
Kellett, R. M., and Spiro, T. G. (2002). Cobalt(I) Porphyrin Catalysts of Hydrogen Production from Water. Inorg. Chem. 24, 2373–2377. doi:10.1021/IC00209A011
Kimoto, A., Yamauchi, K., Yoshida, M., Masaoka, S., and Sakai, K. (2012). Kinetics and DFT Studies on Wateroxidation by Ce4+ Catalyzed by [Ru(terpy)(bpy)(OH2)]2+. Chem. Commun. 48, 239–241. doi:10.1039/c1cc15109f
Kleingardner, J. G., Kandemir, B., and Bren, K. L. (2014). Hydrogen Evolution from Neutral Water under Aerobic Conditions Catalyzed by Cobalt Microperoxidase-11. J. Am. Chem. Soc. 136, 4–7. doi:10.1021/ja406818h
Klepser, B. M., and Bartlett, B. M. (2014). Anchoring a Molecular Iron Catalyst to Solar-Responsive WO3 Improves the Rate and Selectivity of Photoelectrochemical Water Oxidation. J. Am. Chem. Soc. 136, 1694–1697. doi:10.1021/ja4086808
Koelewijn, J. M., Lutz, M., Dzik, W. I., Detz, R. J., and Reek, J. N. H. (2016). Reaction Progress Kinetic Analysis as a Tool to Reveal Ligand Effects in Ce(IV)-Driven IrCp∗-Catalyzed Water Oxidation. ACS Catal. 6, 3418–3427. doi:10.1021/acscatal.6b00297
Kondo, M., Tatewaki, H., and Masaoka, S. (2021). Design of Molecular Water Oxidation Catalysts with Earth-Abundant Metal Ions. Chem. Soc. Rev. 50, 6790–6831. doi:10.1039/D0CS01442G
Koshiba, K., Yamauchi, K., and Sakai, K. (2017). A Nickel Dithiolate Water Reduction Catalyst Providing Ligand-Based Proton-Coupled Electron-Transfer Pathways. Angew. Chem. Int. Ed. 56, 4247–4251. doi:10.1002/ange.20170092710.1002/anie.201700927
Kottrup, K. G., D’Agostini, S., van Langevelde, P. H., Siegler, M. A., and Hetterscheid, D. G. H. (2018). Catalytic Activity of an Iron-Based Water Oxidation Catalyst: Substrate Effects of Graphitic Electrodes. ACS Catal. 8, 1052–1061. doi:10.1021/acscatal.7b03284
Kottrup, K. G., and Hetterscheid, D. G. H. (2016). Evaluation of Iron-Based Electrocatalysts for Water Oxidation - an On-Line Mass Spectrometry Approach. Chem. Commun. 52, 2643–2646. doi:10.1039/C5CC10092E
Lalrempuia, R., McDaniel, N. D., Müller-Bunz, H., Bernhard, S., and Albrecht, M. (2010). Water Oxidation Catalyzed by strong Carbene-type Donor-Ligand Complexes of Iridium. Angew. Chem. Int. Ed. 49, 9765–9768. doi:10.1002/anie.201005260
Le Goff, A., Artero, V., Jousselme, B., Tran, P. D., Guillet, N., Métayé, R., et al. (2009). From Hydrogenases to Noble Metal-free Catalytic Nanomaterials for H 2 Production and Uptake. Science 326, 1384–1387. doi:10.1126/science.1179773
Ledendecker, M., Geiger, S., Hengge, K., Lim, J., Cherevko, S., Mingers, A. M., et al. (2019). Towards Maximized Utilization of Iridium for the Acidic Oxygen Evolution Reaction. Nano Res. 12, 2275–2280. doi:10.1007/s12274-019-2383-y
Lee, H., Wu, X., and Sun, L. (2020). Copper-based Homogeneous and Heterogeneous Catalysts for Electrochemical Water Oxidation. Nanoscale 12, 4187–4218. doi:10.1039/C9NR10437B
Léger, C., and Bertrand, P. (2008). Direct Electrochemistry of Redox Enzymes as a Tool for Mechanistic Studies. Chem. Rev. 108, 2379–2438. doi:10.1021/cr0680742
Lewandowska-Andralojc, A., Polyansky, D. E., Wang, C.-H., Wang, W.-H., Himeda, Y., and Fujita, E. (2014). Efficient Water Oxidation with Organometallic Iridium Complexes as Precatalysts. Phys. Chem. Chem. Phys. 16, 11976–11987. doi:10.1039/c3cp55101f
Lewis, N. S., and Nocera, D. G. (2006). Powering the Planet: Chemical Challenges in Solar Energy Utilization. Proc. Natl. Acad. Sci. U.S.A. 103, 15729–15735. doi:10.1073/pnas.0603395103
Li, J., Triana, C. A., Wan, W., Adiyeri Saseendran, D. P., Zhao, Y., Balaghi, S. E., et al. (2021). Molecular and Heterogeneous Water Oxidation Catalysts: Recent Progress and Joint Perspectives. Chem. Soc. Rev. 50, 2444–2485. doi:10.1039/D0CS00978D
Limburg, B., Bouwman, E., and Bonnet, S. (2012). Molecular Water Oxidation Catalysts Based on Transition Metals and Their Decomposition Pathways. Coord. Chem. Rev. 256, 1451–1467. doi:10.1016/j.ccr.2012.02.021
Liu, F., Concepcion, J. J., Jurss, J. W., Cardolaccia, T., Templeton, J. L., and Meyer, T. J. (2008). Mechanisms of Water Oxidation from the Blue Dimer to Photosystem II. Inorg. Chem. 47, 1727–1752. doi:10.1021/ic701249s
Liu, T., Zhang, B., and Sun, L. (2019). Iron-Based Molecular Water Oxidation Catalysts: Abundant, Cheap, and Promising. Chem. Asian J. 14, 31–43. doi:10.1002/asia.201801253
Liu, Y., Chen, G., Yiu, S.-M., Wong, C.-Y., and Lau, T.-C. (2018). Intermediates in the Oxidative Degradation of a Ruthenium-Bound 2,2′-Bipyridyl-Phenoxy Ligand during Catalytic Water Oxidation. ChemCatChem 10, 501–504. doi:10.1002/cctc.201701319
Liu, Y., Ng, S.-M., Yiu, S.-M., Lam, W. W. Y., Wei, X.-G., Lau, K.-C., et al. (2014). Catalytic Water Oxidation by Ruthenium(II) Quaterpyridine (Qpy) Complexes: Evidence for Ruthenium(III) qpy-N,N′′′-dioxide as the Real Catalysts. Angew. Chem. Int. Ed. 53, 14468–14471. doi:10.1002/anie.201408795
Lloret-Fillol, J., and Costas, M. (2019). Water Oxidation at Base Metal Molecular Catalysts. Adv. Organomet. Chem. 71, 1–52. doi:10.1016/bs.adomc.2019.02.003
López, I., Ertem, M. Z., Maji, S., Benet-Buchholz, J., Keidel, A., Kuhlmann, U., et al. (2014). A Self-Improved Water-Oxidation Catalyst: Is One Site Really Enough? Angew. Chem. Int. Ed. 53, 205–209. doi:10.1002/anie.201307509
Macchioni, A. (2019). The Middle‐Earth between Homogeneous and Heterogeneous Catalysis in Water Oxidation with Iridium. Eur. J. Inorg. Chem. 2019, 7–17. doi:10.1002/ejic.20180151410.1002/ejic.201800798
Materna, K. L., Crabtree, R. H., and Brudvig, G. W. (2017). Anchoring Groups for Photocatalytic Water Oxidation on Metal Oxide Surfaces. Chem. Soc. Rev. 46, 6099–6110. doi:10.1039/c7cs00314e
Matheu, R., Ertem, M. Z., Benet-Buchholz, J., Coronado, E., Batista, V. S., Sala, X., et al. (2015). Intramolecular Proton Transfer Boosts Water Oxidation Catalyzed by a Ru Complex. J. Am. Chem. Soc. 137, 10786–10795. doi:10.1021/jacs.5b06541
Matheu, R., Garrido-Barros, P., Gil-Sepulcre, M., Ertem, M. Z., Sala, X., Gimbert-Suriñach, C., et al. (2019). The Development of Molecular Water Oxidation Catalysts. Nat. Rev. Chem. 3, 331–341. doi:10.1038/s41570-019-0096-0
Mazloomi, Z., Margalef, J., Gil-Sepulcre, M., Romero, N., Albrecht, M., Llobet, A., et al. (2020). Effect of Ligand Chelation and Sacrificial Oxidant on the Integrity of Triazole-Based Carbene Iridium Water Oxidation Catalysts. Inorg. Chem. 59, 12337–12347. doi:10.1021/acs.inorgchem.0c01439
McCrory, C. C. L., Uyeda, C., and Peters, J. C. (2012). Electrocatalytic Hydrogen Evolution in Acidic Water with Molecular Cobalt Tetraazamacrocycles. J. Am. Chem. Soc. 134, 3164–3170. doi:10.1021/ja210661k
McDaniel, N. D., Coughlin, F. J., Tinker, L. L., and Bernhard, S. (2008). Cyclometalated Iridium(III) Aquo Complexes: Efficient and Tunable Catalysts for the Homogeneous Oxidation of Water. J. Am. Chem. Soc. 130, 210–217. doi:10.1021/ja074478f
McNamara, W. R., Han, Z., Alperin, P. J., Brennessel, W. W., Holland, P. L., and Eisenberg, R. (2011). A Cobalt-Dithiolene Complex for the Photocatalytic and Electrocatalytic Reduction of Protons. J. Am. Chem. Soc. 133, 15368–15371. doi:10.1021/ja207842r
Menendez Rodriguez, G., Bucci, A., Hutchinson, R., Bellachioma, G., Zuccaccia, C., Giovagnoli, S., et al. (2017a). Extremely Active, Tunable, and pH-Responsive Iridium Water Oxidation Catalysts. ACS Energ. Lett. 2, 105–110. doi:10.1021/acsenergylett.6b00606
Menendez Rodriguez, G., Gatto, G., Zuccaccia, C., and Macchioni, A. (2017b). Benchmarking Water Oxidation Catalysts Based on Iridium Complexes: Clues and Doubts on the Nature of Active Species. ChemSusChem 10, 4503–4509. doi:10.1002/cssc.201701818
Michaelos, T. K., Shopov, D. Y., Sinha, S. B., Sharninghausen, L. S., Fisher, K. J., Lant, H. M. C., et al. (2017). A Pyridine Alkoxide Chelate Ligand that Promotes Both Unusually High Oxidation States and Water-Oxidation Catalysis. Acc. Chem. Res. 50, 952–959. doi:10.1021/acs.accounts.6b00652
Na, Y., Wang, M., Jin, K., Zhang, R., and Sun, L. (2006). An Approach to Water-Soluble Hydrogenase Active Site Models: Synthesis and Electrochemistry of Diiron Dithiolate Complexes with 3,7-diacetyl-1,3,7-triaza-5-phosphabicyclo[3.3.1]nonane Ligand(s). J. Organomet. Chem. 691, 5045–5051. doi:10.1016/j.jorganchem.2006.08.082
Najafpour, M. M., Renger, G., Hołyńska, M., Moghaddam, A. N., Aro, E.-M., Carpentier, R., et al. (2016). Manganese Compounds as Water-Oxidizing Catalysts: From the Natural Water-Oxidizing Complex to Nanosized Manganese Oxide Structures. Chem. Rev. 116, 2886–2936. doi:10.1021/acs.chemrev.5b00340
Navarro, M., Smith, C. A., Li, M., Bernhard, S., and Albrecht, M. (2018). Optimization of Synthetically Versatile Pyridylidene Amide Ligands for Efficient Iridium‐Catalyzed Water Oxidation. Chem. Eur. J. 24, 6386–6398. doi:10.1002/chem.201705619
Neudeck, S., Maji, S., López, I., Meyer, S., Meyer, F., and Llobet, A. (2014). New Powerful and Oxidatively Rugged Dinuclear Ru Water Oxidation Catalyst: Control of Mechanistic Pathways by Tailored Ligand Design. J. Am. Chem. Soc. 136, 24–27. doi:10.1021/ja409974b
Newborough, M., and Cooley, G. (20202020). Developments in the Global Hydrogen Market: The Spectrum of Hydrogen Colours. Fuel Cell Bull. 2020, 16–22. doi:10.1016/S1464-2859(20)30546-0
Okamura, M., Kondo, M., Kuga, R., Kurashige, Y., Yanai, T., Hayami, S., et al. (2016). A Pentanuclear Iron Catalyst Designed for Water Oxidation. Nature 530, 465–468. doi:10.1038/nature16529
Olivares, M., van der Ham, C. J. M., Mdluli, V., Schmidtendorf, M., Müller‐Bunz, H., Verhoeven, T. W. G. M., et al. (2020). Relevance of Chemical vs. Electrochemical Oxidation of Tunable Carbene Iridium Complexes for Catalytic Water Oxidation. Eur. J. Inorg. Chem. 2020, 801–812. doi:10.1002/ejic.202000090
Panda, C., Debgupta, J., Díaz Díaz, D., Singh, K. K., Sen Gupta, S., and Dhar, B. B. (2014). Homogeneous Photochemical Water Oxidation by Biuret-Modified Fe-TAML: Evidence of FeV(O) Intermediate. J. Am. Chem. Soc. 136, 12273–12282. doi:10.1021/ja503753k
Parent, A. R., Nakazono, T., Lin, S., Utsunomiya, S., and Sakai, K. (2014). Mechanism of Water Oxidation by Non-heme Iron Catalysts when Driven with Sodium Periodate. Dalton Trans. 43, 12501–12513. doi:10.1039/C4DT01188K
Parra, D., Valverde, L., Pino, F. J., and Patel, M. K. (2019). A Review on the Role, Cost and Value of Hydrogen Energy Systems for Deep Decarbonisation. Renew. Sust. Energ. Rev. 101, 279–294. doi:10.1016/j.rser.2018.11.010
Pelosin, P., Gil-Sepulcre, M., Garrido-Barros, P., Moonshiram, D., Benet-Buchholz, J., Gimbert-Suriñach, C., et al. (2020). Analysis of the Active Species Responsible for Water Oxidation Using a Pentanuclear Fe Complex. iScience 23, 101378. doi:10.1016/j.isci.2020.101378
Pokhrel, R., Goetz, M. K., Shaner, S. E., Wu, X., and Stahl, S. S. (2015). The “Best Catalyst” for Water Oxidation Depends on the Oxidation Method Employed: A Case Study of Manganese Oxides. J. Am. Chem. Soc. 137, 8384–8387. doi:10.1021/jacs.5b05093
Rakowski Dubois, M., and Dubois, D. L. (2009). Development of Molecular Electrocatalysts for CO2 Reduction and H2 Production/Oxidation. Acc. Chem. Res. 42, 1974–1982. doi:10.1021/AR900110C
Richmond, C. J., Matheu, R., Poater, A., Falivene, L., Benet-Buchholz, J., Sala, X., et al. (2014). Supramolecular Water Oxidation with Ru-Bda-Based Catalysts. Chem. Eur. J. 20, 17282–17286. doi:10.1002/chem.201405144
Rodriguez, G. M., Zaccaria, F., Van Dijk, S., Zuccaccia, C., and Macchioni, A. (2021). Substituent Effects on the Activity of Cp∗Ir(pyridine-Carboxylate) Water Oxidation Catalysts: Which Ligand Fragments Remain Coordinated to the Active Ir Centers? Organometallics 40, 3445–3453. doi:10.1021/acs.organomet.1c00464
Romain, S., Vigara, L., and Llobet, A. (2009). Oxygen−Oxygen Bond Formation Pathways Promoted by Ruthenium Complexes. Acc. Chem. Res. 42, 1944–1953. doi:10.1021/ar900240w
Rosser, T. E., and Reisner, E. (2017). Understanding Immobilized Molecular Catalysts for Fuel-Forming Reactions through UV/Vis Spectroelectrochemistry. ACS Catal. 7, 3131–3141. doi:10.1021/acscatal.7b00326
Sakai, K., and Ozawa, H. (2007). Homogeneous Catalysis of Platinum(II) Complexes in Photochemical Hydrogen Production from Water. Coord. Chem. Rev. 251, 2753–2766. doi:10.1016/j.ccr.2007.08.014
Savini, A., Belanzoni, P., Bellachioma, G., Zuccaccia, C., Zuccaccia, D., and Macchioni, A. (2011). Activity and Degradation Pathways of Pentamethyl-Cyclopentadienyl-Iridium Catalysts for Water Oxidation. Green. Chem. 13, 3360–3374. doi:10.1039/c1gc15899f
Savini, A., Bellachioma, G., Ciancaleoni, G., Zuccaccia, C., Zuccaccia, D., and MacChioni, A. (2010). Iridium(iii) Molecular Catalysts for Water Oxidation: The Simpler the Faster. Chem. Commun. 46, 9218–9219. doi:10.1039/c0cc03801f
Schley, N. D., Blakemore, J. D., Subbaiyan, N. K., Incarvito, C. D., D’Souza, F., Crabtree, R. H., et al. (2011). Distinguishing Homogeneous from Heterogeneous Catalysis in Electrode-Driven Water Oxidation with Molecular Iridium Complexes. J. Am. Chem. Soc. 133, 10473–10481. doi:10.1021/ja2004522
Schulze, M., Kunz, V., Frischmann, P. D., and Würthner, F. (2016). A Supramolecular Ruthenium Macrocycle with High Catalytic Activity for Water Oxidation that Mechanistically Mimics Photosystem II. Nat. Chem 8, 576–583. doi:10.1038/nchem.2503
Sens, C., Romero, I., Rodríguez, M., Llobet, A., Parella, T., and Benet-Buchholz, J. (2004). A New Ru Complex Capable of Catalytically Oxidizing Water to Molecular Dioxygen. J. Am. Chem. Soc. 126, 7798–7799. doi:10.1021/ja0486824
Shaffer, D. W., Xie, Y., Szalda, D. J., and Concepcion, J. J. (2017). Lability and Basicity of Bipyridine-Carboxylate-Phosphonate Ligand Accelerate Single-Site Water Oxidation by Ruthenium-Based Molecular Catalysts. J. Am. Chem. Soc. 139, 15347–15355. doi:10.1021/jacs.7b06096
Sharninghausen, L. S., Sinha, S. B., Shopov, D. Y., Choi, B., Mercado, B. Q., Roy, X., et al. (2016). High Oxidation State Iridium Mono-μ-Oxo Dimers Related to Water Oxidation Catalysis. J. Am. Chem. Soc. 138, 15917–15926. doi:10.1021/jacs.6b07716
Sheehan, S. W., Thomsen, J. M., Hintermair, U., Crabtree, R. H., Brudvig, G. W., and Schmuttenmaer, C. A. (2015). A Molecular Catalyst for Water Oxidation that Binds to Metal Oxide Surfaces. Nat. Commun. 6, 1–9. doi:10.1038/ncomms7469
Singleton, M. L., Reibenspies, J. H., and Darensbourg, M. Y. (2010). A Cyclodextrin Host/guest Approach to a Hydrogenase Active Site Biomimetic Cavity. J. Am. Chem. Soc. 132, 8870–8871. doi:10.1021/ja103774j
Song, H., Luo, S., Huang, H., Deng, B., and Ye, J. (2022). Solar-Driven Hydrogen Production: Recent Advances, Challenges, and Future Perspectives. ACS Energ. Lett. 7, 1043–1065. doi:10.1021/acsenergylett.1c02591
Stoll, T., Castillo, C. E., Kayanuma, M., Sandroni, M., Daniel, C., Odobel, F., et al. (2015). Photo-induced Redox Catalysis for Proton Reduction to Hydrogen with Homogeneous Molecular Systems Using Rhodium-Based Catalysts. Coord. Chem. Rev. 304-305, 20–37. doi:10.1016/j.ccr.2015.02.002
Sun, Y., Bigi, J. P., Piro, N. A., Tang, M. L., Long, J. R., and Chang, C. J. (2011). Molecular Cobalt Pentapyridine Catalysts for Generating Hydrogen from Water. J. Am. Chem. Soc. 133, 9212–9215. doi:10.1021/ja202743r
Tamaki, Y., Vannucci, A. K., Dares, C. J., Binstead, R. A., and Meyer, T. J. (2014). One-Electron Activation of Water Oxidation Catalysis. J. Am. Chem. Soc. 136, 6854–6857. doi:10.1021/ja502637s
Tatematsu, R., Inomata, T., Ozawa, T., and Masuda, H. (2016). Electrocatalytic Hydrogen Production by a Nickel(II) Complex with a Phosphinopyridyl Ligand. Angew. Chem. 128, 5333–5336. doi:10.1002/ANGE.201511621
Thomsen, J. M., Huang, D. L., Crabtree, R. H., and Brudvig, G. W. (2015). Iridium-based Complexes for Water Oxidation. Dalton Trans. 44, 12452–12472. doi:10.1039/c5dt00863h
Timmer, B. J. J., Kravchenko, O., Liu, T., Zhang, B., and Sun, L. (2021). Off‐Set Interactions of Ruthenium-Bda Type Catalysts for Promoting Water‐Splitting Performance. Angew. Chem. Int. Ed. 60, 14504–14511. doi:10.1002/anie.202101931
To, W.-P., Wai-Shan Chow, T., Tse, C.-W., Guan, X., Huang, J.-S., and Che, C.-M. (2015). Water Oxidation Catalysed by Iron Complex of N,N′-dimethyl-2,11-diaza[3,3](2,6)pyridinophane. Spectroscopy of Iron-Oxo Intermediates and Density Functional Theory Calculations. Chem. Sci. 6, 5891–5903. doi:10.1039/C5SC01680K
Van Dijk, B., Rodriguez, G. M., Wu, L., Hofmann, J. P., Macchioni, A., and Hetterscheid, D. G. H. (2020). The Influence of the Ligand in the Iridium Mediated Electrocatalyic Water Oxidation. ACS Catal. 10, 4398–4410. doi:10.1021/acscatal.0c00531
Velazquez Abad, A., and Dodds, P. E. (2020). Green Hydrogen Characterisation Initiatives: Definitions, Standards, Guarantees of Origin, and Challenges. Energy Policy 138, 111300. doi:10.1016/j.enpol.2020.111300
Venturini, A., Barbieri, A., Reek, J. N. H., and Hetterscheid, D. G. H. (2014). Catalytic Water Splitting with an Iridium Carbene Complex: A Theoretical Study. Chem. Eur. J. 20, 5358–5368. doi:10.1002/chem.201303796
Vereshchuk, N., Matheu, R., Benet-Buchholz, J., Pipelier, M., Lebreton, J., Dubreuil, D., et al. (2020). Second Coordination Sphere Effects in an Evolved Ru Complex Based on Highly Adaptable Ligand Results in Rapid Water Oxidation Catalysis. J. Am. Chem. Soc. 142, 5068–5077. doi:10.1021/jacs.9b11935
Vivancos, Á., Segarra, C., and Albrecht, M. (2018). Mesoionic and Related Less Heteroatom-Stabilized N-Heterocyclic Carbene Complexes: Synthesis, Catalysis, and Other Applications. Chem. Rev. 118, 9493–9586. doi:10.1021/acs.chemrev.8b00148
Wan, X., Wang, L., Dong, C.-L., Menendez Rodriguez, G., Huang, Y.-C., Macchioni, A., et al. (2018). Activating Kläui-type Organometallic Precursors at Metal Oxide Surfaces for Enhanced Solar Water Oxidation. ACS Energ. Lett. 3, 1613–1619. doi:10.1021/acsenergylett.8b00847
Wang, C., Wang, J.-L., and Lin, W. (2012a). Elucidating Molecular Iridium Water Oxidation Catalysts Using Metal-Organic Frameworks: A Comprehensive Structural, Catalytic, Spectroscopic, and Kinetic Study. J. Am. Chem. Soc. 134, 19895–19908. doi:10.1021/ja310074j
Wang, J. W., Yamauchi, K., Huang, H. H., Sun, J. K., Luo, Z. M., Zhong, D. C., et al. (2019). A Molecular Cobalt Hydrogen Evolution Catalyst Showing High Activity and Outstanding Tolerance to CO and O 2. Angew. Chem. 131, 11039–11043. doi:10.1002/ANGE.201904578
Wang, L., Duan, L., Stewart, B., Pu, M., Liu, J., Privalov, T., et al. (2012b). Toward Controlling Water Oxidation Catalysis: Tunable Activity of Ruthenium Complexes with Axial Imidazole/DMSO Ligands. J. Am. Chem. Soc. 134, 18868–18880. doi:10.1021/ja309805m
Wang, L., Duan, L., Wang, Y., Ahlquist, M. S. G., and Sun, L. (2014). Highly Efficient and Robust Molecular Water Oxidation Catalysts Based on Ruthenium Complexes. Chem. Commun. 50, 12947–12950. doi:10.1039/C4CC05069J
Wang, M., Chen, L., and Sun, L. (2012c). Recent Progress in Electrochemical Hydrogen Production with Earth-Abundant Metal Complexes as Catalysts. Energ. Environ. Sci. 5, 6763–6778. doi:10.1039/c2ee03309g
Wang, M., Na, Y., Gorlov, M., and Sun, L. (2009). Light-driven Hydrogen Production Catalysed by Transition Metal Complexes in Homogeneous Systems. Dalton Trans. 1, 6458. doi:10.1039/b903809d
Wasylenko, D. J., Ganesamoorthy, C., Henderson, M. A., Koivisto, B. D., Osthoff, H. D., and Berlinguette, C. P. (2010). Electronic Modification of the [RuII(tpy)(bpy)(OH2)]2+ Scaffold: Effects on Catalytic Water Oxidation. J. Am. Chem. Soc. 132, 16094–16106. doi:10.1021/ja106108y
Wickramasinghe, L. D., Zhou, R., Zong, R., Vo, P., Gagnon, K. J., and Thummel, R. P. (2015). Iron Complexes of Square Planar Tetradentate Polypyridyl-type Ligands as Catalysts for Water Oxidation. J. Am. Chem. Soc. 137, 13260–13263. doi:10.1021/jacs.5b08856
Wilson, A. D., Newell, R. H., McNevin, M. J., Muckerman, J. T., Rakowski DuBois, M., and DuBois, D. L. (2006). Hydrogen Oxidation and Production Using Nickel-Based Molecular Catalysts with Positioned Proton Relays. J. Am. Chem. Soc. 128, 358–366. doi:10.1021/ja056442y
Woods, J. A., Lalrempuia, R., Petronilho, A., McDaniel, N. D., Müller-Bunz, H., Albrecht, M., et al. (2014). Carbene Iridium Complexes for Efficient Water Oxidation: Scope and Mechanistic Insights. Energ. Environ. Sci. 7, 2316–2328. doi:10.1039/c4ee00971a
Xiao, M., Wu, Q., Li, L., Mu, S., Sørensen, M. N., Wang, W., et al. (2021). Regenerable Catalyst for Highly Alkaline Water Oxidation. ACS Energ. Lett. 6, 1677–1683. doi:10.1021/acsenergylett.1c00127
Xie, Y., Shaffer, D. W., and Concepcion, J. J. (2018). O-O Radical Coupling: From Detailed Mechanistic Understanding to Enhanced Water Oxidation Catalysis. Inorg. Chem. 57, 10533–10542. doi:10.1021/acs.inorgchem.8b00329
Xu, Y., Fischer, A., Duan, L., Tong, L., Gabrielsson, E., Åkermark, B., et al. (2010). Chemical and Light-Driven Oxidation of Water Catalyzed by an Efficient Dinuclear Ruthenium Complex. Angew. Chem. Int. Edition 49, 8934–8937. doi:10.1002/anie.201004278
Yagi, M., Tajima, S., Komi, M., and Yamazaki, H. (2011). Highly Active and Tunable Catalysts for O2 Evolution from Water Based on Mononuclear Ruthenium(ii) Monoaquo Complexes. Dalton Trans. 40, 3802–3804. doi:10.1039/C0DT01826K
Yang, J., Wang, L., Zhan, S., Zou, H., Chen, H., Ahlquist, M. S. G., et al. (2021). From Ru-Bda to Ru-Bds: a Step Forward to Highly Efficient Molecular Water Oxidation Electrocatalysts under Acidic and Neutral Conditions. Nat. Commun. 12, 373. doi:10.1038/s41467-020-20637-8
Yang, K. R., Matula, A. J., Kwon, G., Hong, J., Sheehan, S. W., Thomsen, J. M., et al. (2016). Solution Structures of Highly Active Molecular Ir Water-Oxidation Catalysts from Density Functional Theory Combined with High-Energy X-ray Scattering and EXAFS Spectroscopy. J. Am. Chem. Soc. 138, 5511–5514. doi:10.1021/jacs.6b01750
Yi, J., Zhan, S., Chen, L., Tian, Q., Wang, N., Li, J., et al. (2021). Electrostatic Interactions Accelerating Water Oxidation Catalysis via Intercatalyst O-O Coupling. J. Am. Chem. Soc. 143, 2484–2490. doi:10.1021/jacs.0c07103
Yin, X., Liu, C., Zhuo, S., Xu, Y., and Zhang, B. (2015). A Water-Soluble Glucose-Functionalized Cobalt(III) Complex as an Efficient Electrocatalyst for Hydrogen Evolution under Neutral Conditions. Dalton Trans. 44, 1526–1529. doi:10.1039/c4dt02951h
Yu, F., Poole, D., Mathew, S., Yan, N., Hessels, J., Orth, N., et al. (2018). Control over Electrochemical Water Oxidation Catalysis by Preorganization of Molecular Ruthenium Catalysts in Self-Assembled Nanospheres. Angew. Chem. Int. Ed. 57, 11247–11251. doi:10.1002/anie.201805244
Zahran, Z. N., Tsubonouchi, Y., Mohamed, E. A., and Yagi, M. (2019). Recent Advances in the Development of Molecular Catalyst‐Based Anodes for Water Oxidation toward Artificial Photosynthesis. ChemSusChem 12, 1775–1793. doi:10.1002/cssc.201802795
Zee, D. Z., Chantarojsiri, T., Long, J. R., and Chang, C. J. (2015). Metal-Polypyridyl Catalysts for Electro- and Photochemical Reduction of Water to Hydrogen. Acc. Chem. Res. 48, 2027–2036. doi:10.1021/ACS.ACCOUNTS.5B00082
Zhan, S., De Gracia Triviño, J. A., and Ahlquist, M. S. G. (2019). The Carboxylate Ligand as an Oxide Relay in Catalytic Water Oxidation. J. Am. Chem. Soc. 141, 10247–10252. doi:10.1021/jacs.9b02585
Zhang, B., Li, F., Yu, F., Cui, H., Zhou, X., Li, H., et al. (2014a). Homogeneous Oxidation of Water by Iron Complexes with Macrocyclic Ligands. Chem. Asian J. 9, 1515–1518. doi:10.1002/asia.201400066
Zhang, B., and Sun, L. (2019a). Artificial Photosynthesis: Opportunities and Challenges of Molecular Catalysts. Chem. Soc. Rev. 48, 2216–2264. doi:10.1039/c8cs00897c
Zhang, B., and Sun, L. (2019b). Ru-bda: Unique Molecular Water-Oxidation Catalysts with Distortion Induced Open Site and Negatively Charged Ligands. J. Am. Chem. Soc. 141, 5565–5580. doi:10.1021/jacs.8b12862
Zhang, L. H., Mathew, S., Hessels, J., Reek, J. N. H., and Yu, F. (2021). Homogeneous Catalysts Based on First‐Row Transition‐Metals for Electrochemical Water Oxidation. ChemSusChem 14, 234–250. doi:10.1002/cssc.202001876
Zhang, P., Wang, M., Yang, Y., Yao, T., and Sun, L. (2014b). A Molecular Copper Catalyst for Electrochemical Water Reduction with a Large Hydrogen-Generation Rate Constant in Aqueous Solution. Angew. Chem. Int. Ed. 53, 13803–13807. doi:10.1002/ANIE.201408266
Zhang, P., Wang, M., Yang, Y., Zheng, D., Han, K., and Sun, L. (2014c). Highly Efficient Molecular Nickel Catalysts for Electrochemical Hydrogen Production from Neutral Water. Chem. Commun. 50, 14153–14156. doi:10.1039/C4CC05511J
Zong, R., and Thummel, R. P. (2005). A New Family of Ru Complexes for Water Oxidation. J. Am. Chem. Soc. 127, 12802–12803. doi:10.1021/ja054791m
Keywords: green hydrogen, water oxidation, hydrogen evolution, homogeneous catalysis, organometallics
Citation: Zaccaria F, Menendez Rodriguez G, Rocchigiani L and Macchioni A (2022) Molecular Catalysis in “Green” Hydrogen Production. Front. Catal. 2:892183. doi: 10.3389/fctls.2022.892183
Received: 08 March 2022; Accepted: 07 April 2022;
Published: 10 May 2022.
Edited by:
Roberto Gobetto, University of Turin, ItalyReviewed by:
Biswarup Chakraborty, Indian Institute of Technology Delhi, IndiaEdmund Chun Ming Tse, The University of Hong Kong, Hong Kong SAR, China
Copyright © 2022 Zaccaria, Menendez Rodriguez, Rocchigiani and Macchioni. This is an open-access article distributed under the terms of the Creative Commons Attribution License (CC BY). The use, distribution or reproduction in other forums is permitted, provided the original author(s) and the copyright owner(s) are credited and that the original publication in this journal is cited, in accordance with accepted academic practice. No use, distribution or reproduction is permitted which does not comply with these terms.
*Correspondence: Alceo Macchioni, YWxjZW8ubWFjY2hpb25pQHVuaXBnLml0; Francesco Zaccaria, ZnJhbmNlc2NvLnphY2NhcmlhQHVuaXBnLml0