- Department of Chemical and Biochemical Engineering, Technical University of Denmark, Kongens Lyngby, Denmark
The use of highly selective enzymes to catalyze value-added reactions outside the cell is commonly termed biocatalysis. In this brief perspective, some of the future opportunities for the application of biocatalysis are discussed. First, there are opportunities using multi-enzyme cascades where entirely new synthetic routes can be created independent of cellular constraints. Here the target is mostly high-priced products, such as pharmaceuticals. Secondly, there also exist opportunities for biocatalysis in the synthesis of low-priced products where the high productivities achievable make them eminently suited for drop-in solutions. Both options provide a wealth of interesting research and development possibilities, which are also discussed.
Introduction
Chemists have long recognized the importance of catalysis in the sustainable synthesis of important chemical products (Sheldon, 2016). Biocatalysis recruits nature’s catalysts, enzymes, in various formats for use as potential catalysts. Perhaps their use outside the cell is one of the most commonly used formats and will be the subject of this brief article. In particular, enzymes are very selective catalysts making them the method of choice in complex syntheses involving compounds with multiple chiral centers and/or multiple functional groups, overcoming the need for protection (and de-protection) steps. Unsurprisingly, therefore in the past few decades, many scientific publications have described the enormous potential of biocatalysis, not only in the pharmaceutical sector, but also in the industrial chemicals segment, with the promise of revolutionizing the current chemical industry (Sheldon and Woodley, 2018; Hauer, 2020). In particular in the last decade, enormous scientific progress has been made in the field driven especially by the developments in protein engineering to tune catalytic properties and even the discovery of entirely new enzyme activities, never before seen in Nature (Arnold, 2019; Chen and Arnold, 2020). Nevertheless, despite this extraordinary progress, translating these scientific discoveries into a new sustainable chemical industry has proved far harder to achieve (Woodley, 2019; Hauer, 2020). That is perhaps not so surprising given the exacting demands of the chemical industry (the need for safety, effective economy and sustainability). However, several motives for further implementation of bioprocesses are now becoming clear.
A first key driver to implement enzymatic catalysis in industry is the very high selectivity achievable, which is also tunable through protein engineering. Selectivity is of particular value in the pharmaceutical industry where conventionally long multi-step syntheses are required to build complex molecules with multiple functional groups (Devine et al., 2018; Adams et al., 2019; Romero et al., 2021). Using biocatalysis much shorter syntheses can be realized [e.g., Islatravir synthesis from 12 steps to 3 steps (Huffman et al., 2019) and Atorvastatin synthesis from 4 to 2 steps (Ma et al., 2010)]. Selectivity also has a role for lower-priced products where it is essential not only to convert all the substrate, but also to do so with a high yield.
A second driver to implement enzymatic catalysis in industry is sustainability, centered on using a renewable catalyst, which is both tunable (using protein engineering) and operates under mild conditions (around ambient temperature, neutral pH and atmospheric pressure) (Woodley, 2020; Dominguez de Maria, 2021; Woodley, 2022). In reality, this ensures an environmentally-friendly process, rather than a sustainable one, but it is an important development. However, unless the concentration of product achievable is high enough, such processes can involve the removal of a large amount of reaction solvent (usually water) downstream, which is neither sustainable nor cost effective (Ni et al., 2014; Woodley, 2022). Here too progress has been made in recent years with some excellent examples of high substrate and high product concentration (high intensity) reactions reported in the scientific literature (Hinzmann et al., 2019; Cui et al., 2020; Yang et al., 2020) and one supposes many others already operating in industry [e.g. Sitagliptin synthesis at 190 g/L using ω-transaminase (Savile et al., 2010) and a Lipitor intermediate at 390 g/L using nitrilase (De Santis et al., 2003)]. Nevertheless, at first sight many laboratory reactions are very far from looking attractive to implement in industry (other than the pharmaceutical sector), due to low titers and low specific yields. Especially for larger volume (lower-price) products (<5 USD/kg), it is clear that sustainability is an important goal, since the environmental impact of chemical processes producing over 100,000 t/year is considerable. However, for such products the economic pressure on production costs also requires very efficient processes, able to compete in economic terms with existing well-optimized catalytic routes, in terms of all the relevant performance metrics (Lange, 2021; Meissner and Woodley, 2022).
Thus, although biocatalysis has several excellent features, which should lead to sustainable and highly attractive processes for commercial exploitation in industry, the reality is that for industrial implementation the technology also needs to achieve adequate economic metrics in each individual case. The journey from laboratory scale reaction to industrial process is often long and therefore the greatest opportunities will arise in the development of particular modes of catalysis, in specific industrial sectors. In this brief article, I will outline some of the opportunities, as well as the research required into new development tools, new immobilization methods and reaction media that will further expand the field.
Exploiting Biocatalysis in Industry
Cascade Biocatalysis
Today it is in the pharmaceutical sector that biocatalysis has flourished in particular, driven by the extraordinary vision of companies such as Merck and Co. Inc. (United States), Pfizer Inc. (United States), GlaxoSmithKline plc (United Kingdom) and Codexis Inc. (United States) amongst others, implementing cascade biocatalysis. Here it is not even selectivity which is the main driver, but rather the reduction in the number of reaction steps which becomes possible when synthesizing complex multi-functional small-molecule APIs using enzymatic cascades. Excellent examples such as the synthesis of Islatravir by Merck illustrate the point well (Huffman et al., 2019). A close to ‘ideal’ synthesis has even been reported using analogous technology by Merck for the synthesis of Molnupiravir, an antiviral treatment for COVID-19 (McIntosh et al., 2021). Most significant though, is that the possibilities are not limited to the modification of known pathways, but instead entirely new synthetic routes can be created, even driving thermodynamically unfavorable reactions in reverse (Abu and Woodley, 2015). Both the Islatravir and Molnupiravir examples illustrate running reactions ‘in reverse’, using the subsequent step in the cascade (or side reaction) to pull the equilibrium in the desired direction. Such new-to-nature syntheses offer enormous possibilities (Nazor et al., 2021) and provide an enormous opportunity over fermentation, even using metabolic engineering, where routes need to be based on modifications to existing pathways, and control becomes difficult.
Drop-In Biocatalysis
The second catalytic mode for exploiting enzymes is so-called ‘drop-in’ biocatalysis where an otherwise chemical synthetic sequence is 1) initiated by an enzyme step, 2) interrupted by a biocatalytic step, or else 3) concluded with an enzyme step. A common reason for implementing such single steps is the superb selectivity which can be attained, giving the necessary high yield (Heidlindemann et al., 2014; Rudroff et al., 2018). Since the biocatalytic step must be integrated with existing chemical catalytic steps, it is also essential that sufficient productivity (rate) is offered by the enzymatic reaction, otherwise the biocatalytic step becomes the rate-limiting step. Indeed, enzymatic catalysis can deliver high catalytic rates and productivities of 20–100 g/L.h are readily achievable. Some examples of the high productivity of biocatalysis have been collected in an excellent recent review (Wu et al., 2021). These high productivities have also been the driving force, amongst others, behind the implementation of huge volume biocatalytic processes such as the production of high-fructose corn syrup (HFCS) using glucose isomerase in the 1970s (Basso and Serban, 2019). These rates are many-fold those achievable by fermentation, limited to around 10 g/L.h and more usually less than 5 g/L.h, on account of oxygen limitations and requirements for cell growth. Two-stage fermentation and other well-known tricks in fermentation can also deliver higher productivity cell-based processes, but still not near the requirements for an effective drop-in (Burg et al., 2016). However, this does not mean that fermentation will not have a future role in the biological production of chemicals. For longer pathways where the entire synthesis from sustainable feedstock to product can be taken in the cell, based on the modification of existing pathways then fermentation may indeed prove beneficial (Becker and Wittmann, 2015; Lee et al., 2019). Likewise, in an entirely complementary way for low-priced products where a single step may benefit from high selectivity, then biocatalysis will be the choice. An excellent example is the synthesis of derivatives from 5-hydroxymethyl furfural (Dijkman et al., 2014; Carro et al., 2018; Yuan et al., 2020; Birmingham et al., 2021). Some drop-in reactions may even be integrated with the adjacent chemical step in a ‘one-pot’ reactor which can also bring advantages for the yield (Denard et al., 2013; Dumeignil et al., 2018; Gröger and Hummel, 2020).
Figure 1, schematically illustrates some of the opportunities for cascade and drop-in biocatalysis placed on a productivity/product value map. For low value products, drop-in biocatalysis gives an opportunity to add selectivity at high productivity, matching the rate required at such a scale to the other reactions. For intermediate value products, fermentation is both cheap enough but also at a rate appropriate to match the requirements at that scale. Finally, for the high value products the biocatalytic cascade provides the required selectivity. Rate is not as critical here due to the smaller scale of production.
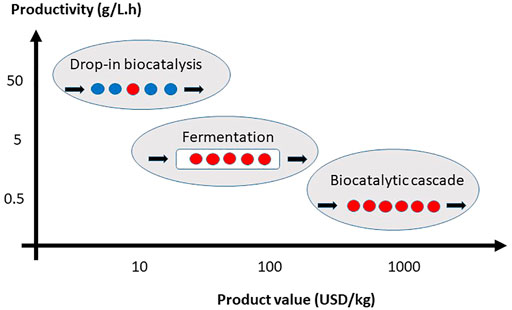
FIGURE 1. Options for implementation of drop-in and cascade biocatalysis, on the productivity/product value map. Red filled circles imply enzyme steps. Blue filled circles represent chemical steps.
Future Scientific Challenges
New Enzymes and Biocatalytic Retro-Synthesis
In the examples discussed here and elsewhere, entirely new synthetic routes can be conceived, limited solely by known enzyme activities. Hence, one very interesting scientific challenge for the future is to develop entirely new enzyme activities, catalyzing new-to-nature reactions. This is an enormously exciting field for academic chemists, discovering new enzymes as well as new synthetic pathways. Pioneering work in this direction has already been started, and holds great promise for the future (Kan et al., 2016; Chen and Arnold, 2020). However, availability of enzymes to catalyze all sorts of new reactions is not in itself sufficient. It is also necessary to understand how to link the enzymes together to create new pathways. This is the growing field of biocatalytic retro-synthesis (Turner and O’Reilly, 2013; De Souza et al., 2017; Hönig et al., 2017). Here the approach has been based on the ground-breaking work on retro-synthesis for conventional chemical reactions (Corey, 1991). Nevertheless, there are around 50 enzyme classes, which is far fewer than offered by conventional catalysts. The key again will likely prove to be protein engineering, enabling tuning and modification of enzyme activities against specific targets, as well as finding entirely new activities to expand the number of enzyme classes. As methods for protein engineering use computational power to an ever-increasing extent (Li et al., 2019; Wu et al., 2019; Yang et al., 2019), and this is integrated with high-throughput automation (Dör et al., 2016) together with computer-assisted retro-synthesis (Finnigan et al., 2021) one can envisage great promise for the start of automated route generation, and subsequent screening and evaluation. In this way, entirely new syntheses will be created (either wholly enzyme-based for cascades or partly enzyme–based for drop-in). Matching reaction conditions, kinetics, thermodynamics and (bio)catalyst stability will all prove fascinating challenges.
Biocatalyst Stability
When considering a single-step biocatalytic reaction other considerations will prove important, especially with a view to drop-in solutions in the chemical industry. While enzymatic synthesis can often deliver the required productivity (volumetric rate or space-time yield, g product/L reactor.h), the challenge here is how much enzyme is required to achieve this productivity (biocatalyst productivity or specific yield, g product/g biocatalyst). This is a dual problem of ensuring adequate specific activity (through protein engineering) as well as operational stability (maintenance of the activity over operating time). Storage stability is also of importance and needs to be considered particularly at larger scale. Methods to stabilize enzymes during operation include protein engineering (Meng et al., 2020) and immobilization (Basso and Serban, 2019; Boudrant et al., 2020; Sheldon et al., 2021). Such technologies continue to develop, but still there is much to understand about the operational stability of enzymes (Polozzi et al., 2007; Bommarius, 2015). Specifically, it is a challenge to understand enzyme stability under industrial conditions, which are far away from conditions investigated in conventional enzymology (e.g., at high concentrations of substrate and/or product, in multi-phase mixtures, and with fluctuating pH values). Here new tools will be required to assess stability in a rapid and more practical way. A particular problem is that those enzymes most attractive for implementation, and therefore highly stable, present a practical problem of measurement within a reasonable timeframe. Approaches using high temperature ramps are one possibility, but other tools will also be required.
Enzyme Immobilization and Reaction Media
A related scientific challenge here, when the enzymes of interest have already been stabilized, is how to make the best use of this activity. Options include operating in continuous flow reactors or via enzyme recycle and reuse. Both options require retention of the enzyme prior to downstream processing, via a second phase, use of membranes or else via immobilization. This will likely be the third area of scientific interest and growth. Here the issue is less about the development of new tools and more about new methodologies for presenting the reaction components to each other. A first target is the interaction of enzymes with new materials for immobilization (Dos Santos et al., 2015). Modification of activity, stability and selectivity also become possible via the correct selection of immobilization support material (Sheldon, 2019). Opportunities are also possible via the use of magnetic support materials for immobilization where there has been considerable progress recently (Zhang et al., 2020; Matveeva and Bronstein, 2021; Zhou et al., 2021). As material science develops further, many more opportunities will be forthcoming to use immobilization to exploit biocatalysis to the fullest possible extent. The cost of immobilization will always need to be considered but is often offset by the benefits for retention of enzyme in continuous reactors or separation and reuse of the enzymes in multiple batches (Thompson et al., 2019). Recently, co-immobilization of multiple enzymes provide entirely new opportunities for managing enzymatic cascades via spatial as well as temporal control. A second target is the reaction medium. In the 1970s and 1980s significant progress was made in the study of enzymes in non-aqueous environments, usually either anhydrous solvents or aqueous-organic liquid-liquid mixtures. The former enables reactions to be carried out which would be thermodynamically impossible in an aqueous environment (such as esterification), and the latter enable reactions with poorly water-soluble inhibitory substrates or products to be run at high overall loading. In the subsequent decades, a range of other non-conventional media have been tested with enzymes,* (Kourist and Gonzalez-Sabin, 2020) including deep eutectic solvents (Pätzold et al., 2019; Tan and Dou, 2020) and ionic liquids (Cantone et al., 2007; Sheldon, 2021). New ionic liquids, based on renewable materials, giving improvements for sustainability are also being developed (Seitkalieva et al., 2021). Of particular interest is that ionic liquids can be tuned to have particular properties and in this way match not only the requirements of the enzyme and reaction, but also the reactor.
Outlook
The vision presented here of cascade biocatalysis and drop-in biocatalysis is already starting to be implemented (Heath et al., 2022). Its further expansion will be dependent upon the continued development of new tools to address some of the remaining scientific challenges. Computer-assisted approaches will speed up this development. It remains difficult to control each individual reaction step in a cell and precisely control protein expression, aside from limitations to the total protein load in the cell, as well as effective transport of substrate into the cell and product back out of the cell (Sperl and Sieber, 2018). For the medium-term therefore the two modes of cascade biocatalysis and drop-in biocatalysis seem likely to prevail. This will be made simpler by another change which is already starting to impact the industry, and that concerns the lower and lower cost of enzyme manufacture. As the cost of enzyme production is reduced, through the use of more productive hosts, better methods of protein expression and improved downstream enzyme recovery, new opportunities will open up in biocatalysis and other industries (Arbige et al., 2019). In particular, options for lower-priced products will become increasingly attractive. Interestingly this will also present new challenges for biocatalytic science caused by much larger scale reactor operation where gradients of pH, oxygen and substrate concentration can affect enzyme stability and thereby biocatalytic process performance. Much can be learnt from previous work on fermentation at the 100–500 m3 scale, although the rate of enzyme-catalyzed reactions is sufficiently fast to exacerbate the effects of such gradients.
Developments in biocatalytic retro-synthesis linked with protein engineering and automated route selection will be of great importance in the implementation of new cascades. For drop-in biocatalysis developments in understanding the stability of enzymes under industrial conditions linked with protein engineering will be important to enable implementation in lower-priced chemical markets. Finally, the development of new materials for enzyme immobilization, as well as new media in which to carry out reactions will be key areas of scientific endeavor in the coming decade.
In summary, as we learn more and more about how to manipulate enzymes to be able to carry out industrial chemistry, many more opportunities will be forthcoming. At the same time, new challenges for biocatalytic science will present themselves, giving interesting and exciting work for those in academia and industry alike. Much of the most exciting developments will take place at the interface of protein science, bioprocess engineering and synthetic chemistry.
Data Availability Statement
The original contributions presented in the study are included in the article/Supplementary Material, further inquiries can be directed to the corresponding author.
Author Contributions
The author confirms being the sole contributor of this work and has approved it for publication.
Funding
JW acknowledges that this is part of a project that has received funding from the European Research Council (ERC) under the European Union’s Horizon 2020 research and innovation programme (Grant agreement No. 101021024).
Conflict of Interest
The author declares that the research was conducted in the absence of any commercial or financial relationships that could be construed as a potential conflict of interest.
Publisher’s Note
All claims expressed in this article are solely those of the authors and do not necessarily represent those of their affiliated organizations, or those of the publisher, the editors and the reviewers. Any product that may be evaluated in this article, or claim that may be made by its manufacturer, is not guaranteed or endorsed by the publisher.
References
Abu, R., and Woodley, J. M. (2015). Application of Enzyme Coupling Reactions to Shift Thermodynamically Limited Biocatalytic Reactions. ChemCatChem 7, 3094–3105. doi:10.1002/cctc.201500603
Adams, J. P., Brown, M. J. B., Diaz-Rodriguez, A., Lloyd, R. C., and Roiban, G.-D. (2019). Biocatalysis: A Pharma Perspective. Adv. Synth. Catal. 361, 2421–2432. doi:10.1002/adsc.201900424
Arbige, M. V., Shetty, J. K., and Chotani, G. K. (2019). Industrial Enzymology: The Next Chapter. Trends Biotechnol. 37, 1355–1366. doi:10.1016/j.tibtech.2019.09.010
Arnold, F. H. (2019). Innovation by Evolution: Bringing New Chemistry to Life (Nobel Lecture). Angew. Chem. Int. Ed. 58, 14420–14426. doi:10.1002/anie.201907729
Basso, A., and Serban, S. (2019). Industrial Applications of Immobilized Enzymes-A Review. Mol. Catal. 479, 110607. doi:10.1016/j.mcat.2019.110607
Becker, J., and Wittmann, C. (2015). Advanced Biotechnology: Metabolically Engineered Cells for the Bio-Based Production of Chemicals and Fuels, Materials, and Health-Care Products. Angew. Chem. Int. Ed. 54, 3328–3350. doi:10.1002/anie.201409033
Birmingham, W. R., Toftgaard Pedersen, A., Dias Gomes, M., Bøje Madsen, M., Breuer, M., Woodley, J. M., et al. (2021). Toward Scalable Biocatalytic Conversion of 5-hydroxymethylfurfural by Galactose Oxidase Using Coordinated Reaction and Enzyme Engineering. Nat. Commun. 12, 4946. doi:10.1038/s41467-021-25034-3
Bommarius, A. S. (2015). Biocatalysis: A Status Report. Annu. Rev. Chem. Biomol. Eng. 6, 319–345. doi:10.1146/annurev-chembioeng-061114-123415
Boudrant, J., Woodley, J. M., and Fernandez-Lafuente, R. (2020). Parameters Necessary to Define an Immobilized Enzyme Preparation. Process Biochem. 90, 66–80. doi:10.1016/j.procbio.2019.11.026
Burg, J. M., Cooper, C. B., Ye, Z., Reed, B. R., Moreb, E. A., and Lynch, M. D. (2016). Large-scale Bioprocess Competitiveness: The Potential of Dynamic Metabolic Control in Two-Stage Fermentations. Curr. Opin. Chem. Eng. 14, 121–136. doi:10.1016/j.coche.2016.09.008
Cantone, S., Hanefeld, U., and Basso, A. (2007). Biocatalysis in Non-conventional Media-Ionic Liquids, Supercritical Fluids and the Gas Phase. Green Chem. 9, 954–971. doi:10.1039/b618893a
Carro, J., Fernández-Fueyo, E., Fernández-Alonso, C., Cañada, J., Ullrich, R., Hofrichter, M., et al. (2018). Self-sustained Enzymatic Cascade for the Production of 2,5-furandicarboxylic Acid from 5-methoxymethylfurfural. Biotechnol. Biofuels 11, 86. doi:10.1186/s13068-018-1091-2
Chen, K., and Arnold, F. H. (2020). Engineering New Catalytic Activities in Enzymes. Nat. Catal. 3, 203–213. doi:10.1038/s41929-019-0385-5
Corey, E. J. (1991). The Logic of Chemical Synthesis: Multistep Synthesis of Complex Carbogenic Molecules(Nobel Lecture). Angew. Chem. Int. Ed. Engl. 30, 455–465. doi:10.1002/anie.199104553
Cui, Z., Mao, Y., Zhao, Y., Zheng, M., Wang, Z., Ma, H., et al. (2020). One-pot Efficient Biosynthesis of (3R)-Acetoin from Pyruvate by a Two-Enzyme Cascade. Catal. Sci. Technol. 10, 7734–7744. doi:10.1039/d0cy01332c
De Santis, G., Wong, K., Farwell, B., Chatman, K., Zhu, Z., Tomlinson, G., et al. (2003). Creation of a Productive, Highly Enantioselective Nitrilase through Gene Site Saturation Mutagenesis (GSSM). J. Am. Chem. Soc. 125, 11476–11477. doi:10.1021/ja035742h
De Souza, R. O. M. A., Miranda, L. S. M., and Bornscheuer, U. T. (2017). A Retrosynthesis Approach for Biocatalysis in Organic Synthesis. Chemistry 23, 12040–12063. doi:10.1002/chem.201702235
Denard, C. A., Hartwig, J. F., and Zhao, H. (2013). Multistep One-Pot Reactions Combining Biocatalysts and Chemical Catalysts for Asymmetric Synthesis. ACS Catal. 3, 2856–2864. doi:10.1021/cs400633a
Devine, P. N., Howard, R. M., Kumar, R., Thompson, M. P., Truppo, M. D., and Turner, N. J. (2018). Extending the Application of Biocatalysis to Meet the Challenges of Drug Development. Nat. Rev. Chem. 2, 409–421. doi:10.1038/s41570-018-0055-1
Dijkman, W. P., Groothuis, D. E., and Fraaije, M. W. (2014). Enzyme‐Catalyzed Oxidation of 5‐Hydroxymethylfurfural to Furan‐2,5‐dicarboxylic Acid. Angew. Chem. Int. Ed. 53, 6515–6518. doi:10.1002/anie.201402904
Domínguez de María, P. (2021). Biocatalysis, Sustainability, and Industrial Applications: Show Me the Metrics. Curr. Opin. Green Sustain. Chem. 31, 100514. doi:10.1016/j.cogsc.2021.100514
Dör, M., Fibinger, M. P. C., Last, D., Schmidt, S., Santos-Aberturas, J., Böttcher, D., et al. (2016). Fully Automatized High-Throughput Enzyme Library Screening Using a Robotic Platform. Biotech. Bioeng. 113, 1421–1432. doi:10.1002/bit.25925
Dumeignil, F., Guehl, M., Gimbernat, A., Capron, M., Ferreira, N. L., Froidevaux, R., et al. (2018). From Sequential Chemoenzymatic Synthesis to Integrated Hybrid Catalysis: Taking the Best of Both Worlds to Open up the Scope of Possibilities for a Sustainable Future. Catal. Sci. Technol. 8, 5708–5734. doi:10.1039/c8cy01190g
Finnigan, W., Hepworth, L. J., Flitsch, S. L., and Turner, N. J. (2021). RetroBioCat as a Computer-Aided Synthesis Planning Tool for Biocatalytic Reactions and Cascades. Nat. Catal. 4, 98–104. doi:10.1038/s41929-020-00556-z
Gröger, H., and Hummel, W. (2020). Combining the ‘two Worlds’ of Chemocatalysis and Biocatalysis towards Multi-step One-Pot Processes in Aqueous Media. Curr. Opin. Chem. Biol. 19, 171–179. doi:10.1016/j.cbpa.2014.03.002
Hauer, B. (2020). Embracing Nature's Catalysts: A Viewpoint on the Future of Biocatalysis. ACS Catal. 10, 8418–8427. doi:10.1021/acscatal.0c01708
Heath, R. S., Ruscoe, R. E., and Turner, N. J. (2022). The Beauty of Biocatalysis: Sustainable Synthesis of Ingredients in Cosmetics. Nat. Prod. Rep. 39 (2), 335–388. doi:10.1039/d1np00027f
Heidlindemann, M., Rulli, G., Berkessel, A., Hummel, W., and Gröger, H. (2014). Combination of Asymmetric Organo- and Biocatalytic Reactions in Organic Media Using Immobilized Catalysts in Different Compartments. ACS Catal. 4, 1099–1103. doi:10.1021/cs4010387
Hinzmann, A., Glinski, S., Worm, M., and Gröger, H. (2019). Enzymatic Synthesis of Aliphatic Nitriles at a Substrate Loading of up to 1.4 kg/L: A Biocatalytic Record Achieved with a Heme Protein. J. Org. Chem. 84, 4867–4872. doi:10.1021/acs.joc.9b00184
Hönig, M., Sondermann, P., Turner, N. J., and Carreira, E. M. (2017). Enantioselective Chemo- and Biocatalysis: Partners in Retrosynthesis. Angew. Chem. Int. Ed. Engl. 56, 8942–8973. doi:10.1002/anie.201612462
Huffman, M. A., Fryszkowska, A., Alvizo, O., Borra-Garske, M., Campos, K. R., Canada, K. A., et al. (2019). Design of an In Vitro Biocatalytic Cascade for the Manufacture of Islatravir. Science 366, 1255–1259. doi:10.1126/science.aay8484
Kan, S. B. J., Lewis, R. D., Chen, K., and Arnold, F. H. (2016). Directed Evolution of Cytochrome C for Carbon-Silicon Bond Formation: Bringing Silicon to Life. Science 354, 1048–1051. doi:10.1126/science.aah6219
Kourist, R., and Gozalez-Sabin, J. (2020). Non-conventional Media as Strategy to Overcome the Solvent Dilemma in Chemoenzymatic Tandem Catalysis. ChemCatChem 12, 1–11. doi:10.1002/cctc.201902192
Lange, J.-P. (2021). Performance Metrics for Sustainable Catalysis in Industry. Nat. Catal. 4, 186–192. doi:10.1038/s41929-021-00585-2
Lee, S. Y., Kim, H. U., Chae, T. U., Cho, J. S., Kim, J. W., Shin, J. H., et al. (2019). A Comprehensive Metabolic Map for Production of Bio-Based Chemicals. Nat. Catal. 2, 18–33. doi:10.1038/s41929-018-0212-4
Li, G., Dong, Y., and Reetz, M. T. (2019). Can Machine Learning Revolutionize Directed Evolution of Selective Enzymes? Adv. Synth. Catal. 361, 2377–2386. doi:10.1002/adsc.201901429
Ma, S. K., Gruber, J., Davis, C., Newman, L., Gray, D., Wang, A., et al. (2010). A Green-By-Design Biocatalytic Process for Atorvastatin Intermediate. Green Chem. 12, 81–86. doi:10.1039/b919115c
Matveeva, V. G., and Bronstein, L. M. (2021). Magnetic Nanoparticle-Containing Supports as Carriers of Immobilized Enzymes: Key Factors Influencing the Biocatalyst Performance. Nanomaterials 11, 2257. doi:10.3390/nano11092257
McIntosh, J. A., Benkovics, T., Silverman, S. M., Huffman, M. A., Kong, J., Maligres, P. E., et al. (2021). Engineered Ribosyl-1-Kinase Enables Concise Synthesis of Molnupiravir, an Antiviral for COVID-19. ACS Cent. Sci. 7, 1980–1985. doi:10.1021/acscentsci.1c00608
Meissner, M. P., and Woodley, J. M. (2022). Mass-based Biocatalyst Metrics to Guide Protein Engineering and Bioprocess Development. Nat. Catal. 5, 2–4. doi:10.1038/s41929-021-00728-5
Meng, Q., Capra, N., Palacio, C. M., Lanfranchi, E., Otzen, M., van Schie, L. Z., et al. (2020). Robust ω-Transaminases by Computational Stabilization of the Subunit Interface. ACS Catal. 10, 2915–2928. doi:10.1021/acscatal.9b05223
Nazor, J., Liu, J., and Huisman, G. (2021). Enzyme Evolution for Industrial Biocatalytic Cascades. Curr. Opin. Biotechnol. 69, 182–190. doi:10.1016/j.copbio.2020.12.013
Ni, Y., Holtmann, D., Holtmann, D., and Hollmann, F. (2014). How Green Is Biocatalysis? to Calculate Is to Know. ChemCatChem 6, 930–943. doi:10.1002/cctc.201300976
Pätzold, M., Siebenhaller, S., Kara, S., Liese, A., Syldatk, C., and Holtmann, D. (2019). Deep Eutectic Solvents as Efficient Solvents in Biocatalysis. Trends Biotechnol. 37, 943–959. doi:10.1016/j.tibtech.2019.03.007
Polizzi, K. M., Bommarius, A. S., Broering, J. M., and Chaparro-Riggers, J. F. (2007). Stability of Biocatalysts. Curr. Opin. Chem. Biol. 11, 220–225. doi:10.1016/j.cbpa.2007.01.685
Romero, E., Jones, B. S., Hogg, B. N., Rué Casamajo, A., Hayes, M. A., Flitsch, S. L., et al. (2021). Enzymatic Late‐Stage Modifications: Better Late Than Never. Angew. Chem. Int. Ed. 60, 16824–16855. doi:10.1002/anie.202014931
Rudroff, F., Mihovilovic, M. D., Gröger, H., Snajdrova, R., Iding, H., and Bornscheuer, U. T. (2018). Opportunities and Challenges for Combining Chemo- and Biocatalysis. Nat. Catal. 1, 12–22. doi:10.1038/s41929-017-0010-4
Santos, J. C. S. d., Barbosa, O., Ortiz, C., Berenguer-Murcia, A., Rodrigues, R. C., and Fernandez-Lafuente, R. (2015). Importance of the Support Properties for Immobilization or Purification of Enzymes. ChemCatChem 7, 2413–2432. doi:10.1002/cctc.201500310
Seitkalieva, M. M., Vavina, A. V., Posvyatenko, A. V., Egorova, K. S., Kashin, A. S., Gordeev, E. G., et al. (2021). Biomass-Derived Ionic Liquids Based on a 5-HMF Platform Chemical: Synthesis, Characterization, Biological Activity, and Tunable Interactions at the Molecular Level. ACS Sustain. Chem. Eng. 9, 3552–3570. doi:10.1021/acssuschemeng.0c08790
Sheldon, R. A., Basso, A., and Brady, D. (2021). New Frontiers in Enzyme Immobilisation: Robust Biocatalysts for a Circular Bio-Based Economy. Chem. Soc. Rev. 50, 5850–5862. doi:10.1039/d1cs00015b
Sheldon, R. A. (2021). Biocatalysis in Ionic Liquids: State-Of-The-Union. Green Chem. 23, 8406–8427. doi:10.1039/d1gc03145g
Sheldon, R. A. (2016). Engineering a More Sustainable World through Catalysis and Green Chemistry. J. R. Soc. Interface. 13, 20160087. doi:10.1098/rsif.2016.0087
Sheldon, R. A., and Woodley, J. M. (2018). Role of Biocatalysis in Sustainable Chemistry. Chem. Rev. 118, 801–838. doi:10.1021/acs.chemrev.7b00203
Sheldon, R. (2019). CLEAs, Combi-CLEAs and 'Smart' Magnetic CLEAs: Biocatalysis in a Bio-Based Economy. Catalysts 9, 261. doi:10.3390/catal9030261
Sperl, J. M., and Sieber, V. (2018). Multienzyme Cascade Reactions-Status and Recent Advances. ACS Catal. 8, 2385–2396. doi:10.1021/acscatal.7b03440
Tan, J.-N., and Dou, Y. (2020). Deep Eutectic Solvents for Biocatalytic Transformations: Focused Lipase-Catalyzed Organic Reactions. Appl. Microbiol. Biotechnol. 104, 1481–1496. doi:10.1007/s00253-019-10342-y
Thompson, M. P., Peñafiel, I., Cosgrove, S. C., and Turner, N. J. (2019). Biocatalysis Using Immobilized Enzymes in Continuous Flow for the Synthesis of Fine Chemicals. Org. Process Res. Dev. 23, 9–18. doi:10.1021/acs.oprd.8b00305
Turner, N. J., and O'Reilly, E. (2013). Biocatalytic Retrosynthesis. Nat. Chem. Biol. 9, 285–288. doi:10.1038/nchembio.1235
Woodley, J. M. (2019). Accelerating the Implementation of Biocatalysis in Industry. Appl. Microbiol. Biotechnol. 103, 4733–4739. doi:10.1007/s00253-019-09796-x
Woodley, J. M. (2022). Ensuring the Sustainability of Biocatalysis. ChemSusChem 15, e202102683. doi:10.1002/cssc.202102683
Woodley, J. M. (2020). New Frontiers in Biocatalysis for Sustainable Synthesis. Curr. Opin. Green Sustain. Chem. 21, 22–26. doi:10.1016/j.cogsc.2019.08.006
Wu, S., Snajdrova, R., Moore, J. C., Baldenius, K., and Bornscheuer, U. T. (2021). Biocatalysis: Enzymatic Synthesis for Industrial Applications. Angew. Chem. Int. Ed. 60, 88–119. doi:10.1002/anie.202006648
Wu, Z., Kan, S. B. J., Lewis, R. D., Wittmann, B. J., and Arnold, F. H. (2019). Machine Learning-Assisted Directed Protein Evolution with Combinatorial Libraries. Proc. Natl. Acad. Sci. U.S.A. 116, 8852–8858. doi:10.1073/pnas.1901979116
Yang, K. K., Wu, Z., and Arnold, F. H. (2019). Machine-learning-guided Directed Evolution for Protein Engineering. Nat. Methods 16, 687–694. doi:10.1038/s41592-019-0496-6
Yang, Z., Ye, W., Xie, Y., Liu, Q., Chen, R., Wang, H., et al. (2020). Efficient Asymmetric Synthesis of Ethyl (S)-4-chloro-3-hydroxybutyrate Using Alcohol Dehydrogenase SmADH31 with High Tolerance of Substrate and Product in a Monophasic Aqueous System. Org. Process Res. Dev. 24, 1068–1076. doi:10.1021/acs.oprd.0c00088
Yuan, H., Liu, H., Du, J., Liu, K., Wang, T., and Liu, L. (2020). Biocatalytic Production of 2,5-furandicarboxylic Acid: Recent Advances and Future Perspectives. Appl. Microbiol. Biotechnol. 104, 527–543. doi:10.1007/s00253-019-10272-9
Zhang, Q., Yu, L., Liu, B., Li, F., and Tang, B. (2020). Reduction of Nitroarenes by Magnetically Recoverable Nitroreductase Immobilized on Fe3O4 Nanoparticles. Sci. Rep. 10, 2810. doi:10.1038/s41598-020-59754-1
Zhou, Z.-W., Cai, C.-X., Xing, X., Li, J., Hu, Z.-E., Xie, Z.-B., et al. (2021). Magnetic COFs as Satisfactory Support for Lipase Immobilization and Recovery to Effectively Achieve the Production of Biodiesel by Maintenance of Enzyme Activity. Biotechnol. Biofuels 14, 156. doi:10.1186/s13068-021-02001-0
Keywords: biocatalysis, biocatalytic cascade, drop-in biocatalysis, enzyme stability, biocatalytic retro-synthesis
Citation: Woodley JM (2022) New Horizons for Biocatalytic Science. Front. Catal. 2:883161. doi: 10.3389/fctls.2022.883161
Received: 24 February 2022; Accepted: 27 April 2022;
Published: 17 May 2022.
Edited by:
Frank Hollmann, Delft University of Technology, NetherlandsReviewed by:
Roland Wohlgemuth, Lodz University of Technology, PolandRoger Arthur Sheldon, University of the Witwatersrand, South Africa
Copyright © 2022 Woodley. This is an open-access article distributed under the terms of the Creative Commons Attribution License (CC BY). The use, distribution or reproduction in other forums is permitted, provided the original author(s) and the copyright owner(s) are credited and that the original publication in this journal is cited, in accordance with accepted academic practice. No use, distribution or reproduction is permitted which does not comply with these terms.
*Correspondence: John M. Woodley, andAa3QuZHR1LmRr