- Department Solar Materials, Helmholtz Centre for Environmental Research, Helmholtz Association of German Research Centres (HZ), Leipzig, Germany
Photosynthesis-driven whole-cell biocatalysis has great potential to contribute to a sustainable bio-economy since phototrophic cells use light as the only energy source. It has yet to be shown that phototrophic microorganisms, such as cyanobacteria, can combine the supply of high heterologous enzyme levels with allocation of sufficient reduction equivalents to enable efficient light-driven redox biocatalysis. Here, we demonstrated that the heterologous expression of an NADPH-dependent Baeyer–Villiger monooxygenase (BVMO) gene from Acidovorax sp. CHX100 turns Synechocystis sp. PCC6803 into an efficient oxyfunctionalization biocatalyst, deriving electrons and O2 from photosynthetic water oxidation. Several expression systems were systematically tested, and a PnrsB-(Ni2+)–controlled expression based on a replicative plasmid yielded the highest intracellular enzyme concentration and activities of up to 60.9 ± 1.0 U gCDW−1. Detailed analysis of reaction parameters, side reactions, and biocatalyst durability revealed—on the one hand—a high in vivo BVMO activity in the range of 6 ± 2 U mgBVMO−1 and—on the other hand—an impairment of biocatalyst performance by product toxicity and by-product inhibition. Scale-up of the reaction to 2-L fed-batch photo-bioreactors resulted in the stabilization of the bioconversion over several hours with a maximal specific activity of 30.0 ± 0.3 U g CDW−1, a maximal volumetric productivity of 0.21 ± 0.1 gL−1 h−1, and the formation of 1.3 ± 0.1 gL−1 of ε-caprolactone. Process simulations based on determined kinetic data revealed that photosynthesis-driven cyclohexanone oxidation on a 2-L scale under high-light conditions was kinetically controlled and not subject to a limitation by photosynthesis.
Introduction
Biotechnological processes have been developed in the past decades to produce materials, chemicals, and pharmaceuticals (Schmid et al., 2001). Compared to standard chemical processes, they, in general, require less energy due to milder reaction conditions, produce less toxic byproducts, and use less harmful reagents, giving them high potential to be environmentally friendly (Burk and Van Dien, 2016); (Bornscheuer et al., 2012). While there are numerous feasible processes using enzymes as catalysts or microbes as whole-cell factories, redox biocatalysis remains challenging (Schrewe et al., 2013). This is due to the dependence of redox enzymes on co-substrates such as electron carriers and O2 (Law et al., 2006). When using isolated enzymes as catalysts, process efficiencies suffer high enzyme and cofactor regeneration costs (Kadisch et al., 2017). Applying whole cells bears the advantage to employ their metabolism to balance redox demands, for example, by oxidizing readily available organic compounds to enable electron supply. However, respective costs and high O2 demands still often hinder efficient, stable, and cheap redox biocatalysis in heterotrophic cell factories (Baldwin and Woodley, 2006; Kadisch et al., 2017).
To overcome these issues, phototrophic microorganisms, such as the model cyanobacterium Synechocystis sp. PCC6803 (Syn6803 from here), are highly attractive host organisms for oxygenases (Lassen et al., 2014a; Lassen et al., 2014b; Böhmer et al., 2017; Hoschek et al., 2019b), reductases (Assil-Companioni et al., 2020), and hydrogenases (Appel et al., 2020). Their photosynthetic apparatus can be exploited to supply oxygenase reactions not only with reduction equivalents but also with O2 from water and sunlight, the most environmentally friendly co-substrates (Hoschek et al., 2017). As whole-cell biocatalysts, cyanobacteria combine this advantage with their self-(re)generation capacity, relying on light, water, and CO2 as main resources (Jodlbauer et al., 2021). Yet, phototrophs so far have gathered little attention as biocatalysts for regio-, chemo-, or stereoselective oxyfunctionalizations. Efforts to engineer cyanobacteria such as introducing a cytochrome P450 monooxygenase (CYP) (Berepiki et al., 2016), fusing a cytochrome P450 to a subunit of photosystem I (PS I) (Lassen et al., 2014a), or implementing CYP-dependent pathways (Wlodarczyk et al., 2015) remained on a proof-of-concept level.
Photosynthesis-driven oxyfunctionalization processes are hampered by a gap of knowledge regarding 1) sufficient gene expression (Lassen et al., 2014a), 2) protein stability and folding, and 3) cofactor supply. However, these aspects are of special importance to ensure that biocatalysis can be conducted in an efficient, stable, and host-compatible manner. Another hindrance for efficient photo-biotechnology are the high doubling times of cyanobacteria (8–12 h for Syn6803 (Heidorn et al., 2011)) and their light dependency, which limits high-density cultivation and scalability (Hoschek et al., 2019a).
High-level expression of heterologous genes in phototrophic organisms suffers a poorly developed molecular biology tool box compared to typical heterotrophic hosts (Berla et al., 2013). Specific characteristics such as the structure of the RNA polymerase (Imamura and Asayama, 2009), promoter types (Huang et al., 2010), and a circadian program (Johnson and Golden, 1999) are fundamental differences to well-studied heterotrophic hosts and often hamper transcriptional engineering (Camsund and Lindblad, 2014; Thiel et al., 2019). There are however pivotal recent developments such as the design of shuttle vectors (Huang et al., 2010) and expression studies focusing on promoters (Englund et al., 2016; Behle et al., 2020) and RBSs (Englund et al., 2016), utilized to increase product titers (Sebesta and Peebles, 2020). For detailed perspectives on regulatory systems and promoter types in cyanobacteria, the reader is referred to Gordon and Pfleger (2018); Immethun and Moon (2018); Gale et al. (2019); Till et al. (2020).
Recent studies employing oxygenases in Syn6803 already showed the accessibility of O2 and electrons derived from the light reaction (Hoschek et al., 2019b). The employment of strictly NADPH-dependent oxygenases, such as cyclohexanone monooxygenase from Acinetobacter calcoaceticus (AcCHMO), led to rather low activities in Syn6803 (Böhmer et al., 2017). This is unexpected as 1) sufficient NADPH supply can be assumed under light conditions, with NADPH as the main product of the photosynthetic light reaction and 2) high-level NADPH supply was shown recently for an NADPH-dependent reductase in Syn6803 (Assil-Companioni et al., 2020).
In this study, we aimed to establish and scale efficient NADPH-dependent BVMO biocatalysis driven by light. To this end, we chose a BVMO from Acidovorax sp. CHX100 for heterologous expression in Syn6803. This NADPH-dependent BVMO has been shown to exhibit high activity in Pseudomonas taiwanensis VLB120 and used for efficient cascade designs (Schäfer et al., 2020; Bretschneider et al., 2021b). We systematically investigated how expression levels of the respective gene in Syn6803 can be enhanced and investigated the resulting BVMO performance in Syn6803. Furthermore, we examined limitations regarding gene expression, reaction kinetics, and host metabolism. Respective reaction engineering and scaling are shown to enable BVMO catalysis with an efficiency auguring well for future applications of light-driven redox biocatalysis.
Materials and Methods
Strains, Chemicals, and Cultivation Methods
DNA oligonucleotides, plasmids, and bacterial strains used for cloning procedures are given in the Supporting Information (Supplementary Table S1). Synechocystis sp. PCC6803 (Stanier et al., 1971) was cultivated in 20 or 50 ml YBG11 medium (Shcolnick et al., 2007) with 50 mM 4-(2-hydroxyethyl)-1-piperazinethanesulfonic acid, pH 7.2, using 100 or 250 ml baffled shaking flasks with cotton stoppers, respectively. Cultivation conditions were 30°C, 150 rpm [2.5 cm amplitude], 50 µmolphotons m−2 s−1, ambient CO2 [0.04%], 75% humidity, and start OD750 = 0.06–0.08. Plate cultivation was conducted on BG11 agar plates with 1.5–2.0% agar at 30°C and 25 µmolphotons m−2 s−1. If required, kanamycin (Km) was used at a final concentration of 50 μg ml−1. Cyclohexanone, ≥ 99.5% purity, was purchased from Sigma-Aldrich (Steinheim, Germany). Cyclohexanol, ≥ 99% purity, was purchased from Merck (Darmstadt, Germany). All other chemicals were purchased from Carl Roth GmbH (Karlsruhe, Germany), Merck, or Sigma-Aldrich at the highest purity available.
Cloning and Transformation
Standard molecular biology procedures were applied as described by Sambrook and Russell (2001). For construction of pAH059, a fragment coding for the C-terminal Strep-tagged bvmo gene (Schäfer et al., 2020) was amplified from pCom10_Capro (Karande et al., 2018) via Phusion PCR using the primer pair PAH096/097 and cloned via Gibson assembly (Gibson et al., 2009) into pEERM3_Km, linearized by XbaI/PstI double digestion. For construction of pAH063, the expression cassette of PrnsB_bvmo_Strep-tag_terminator was amplified via Phusion PCR using the primer pair BVMO1/2 and cloned via Gibson assembly into pPMQAK1, linearized with EcoRI/PstI double digestion. Escherichia coli DH5α (Hanahan, 1983) was transformed with Gibson products via electroporation and correct assembly of plasmids was confirmed via colony PCR of transformants and subsequent sequencing. Commercial kits from Macherey-Nagel (Düren, Germany) were used for plasmid DNA extraction and DNA purification.
For electroporation of Syn6803, cells were grown under standard conditions to an OD750 = 0.5–0.7 determined with a LibraS11 spectrophotometer (Biochrom, Cambridge, United Kingdom), harvested by centrifugation at 5,000 g and 4 °C, washed and 50 x concentrated in 1 mM 4-(2-hydroxyethyl)-1-piperazinethanesulfonic acid, pH 7.5 [according to (Ludwig et al., 2008)]. In total, 60 µl of cell suspension was supplied with 0.5–1 µg of plasmid DNA and electroporated (2.5 kV; 5 ms). After 24 h recovery in YBG11 under standard conditions, selection was performed at 25 µmolphotons m−2 s−1 on BG11 agar plates containing Km. Transformants grown within 7–10 days were confirmed via colony PCR and sequencing. For chromosomal integration, complete segregation was achieved by iterative plating on selective agar plates with increasing Km concentration.
Protein Analysis
Exponentially growing Syn6803_Ni_cBVMO, Syn6803_Ni_pBVMO, and Syn6803_Cu_pBVMO cultures (see Supplementary Table S1 for strain designations) were harvested by centrifugation at 5,000 g and 4°C 24 h after induction with defined concentrations of NiSO4 and concentrated to an OD750 = 20 in 1 x TBS (1 mM phenyl-methyl-sulfonyl-fluoride, pH 7.5). Cells were disrupted in a Precellys® homogenizer for 3 cycles á 3 × 30″ with 30″ pause. After centrifugation at 13.300 g and 4°C for 15 min, the supernatant was used for protein concentration determination via the Bradford analysis (Bradford, 1976). Protein abundance was visualized using SDS-PAGE according to Laemmli (1970), with 3.6 and 12% acrylamide in the stacking and in the separation gels, respectively.
Whole-Cell Activity Assays and Determination of Reaction Kinetics
Syn6803_Cu_pBVMO, Syn6803_Ni_cBVMO, and 6803_Ni_pBVMO cultures (Supplementary Table S1) were harvested by centrifugation at 5,000 g and 4°C 24 h after induction with defined concentrations of NiSO4 and adjusted to a defined cell concentration by re-suspending in YBG 11 containing 50 mM 4-(2-hydroxyethyl)-1-piperazinethanesulfonic acid and 10 µM NiSO4, pH 7.2, if not stated otherwise. Cells were equilibrated for 10 min to assay conditions. Standard assay conditions were 30°C, 200 rpm, 150 µmolphotons m−2 s−1, ambient CO2 (0.04%). The conversion of cyclohexanone (C-one) to ε-caprolactone (ε-Cl) was started by adding C-one to a final concentration of 5 mM and was stopped after 30 min by either quenching with 1 vol diethylether containing 0.2 mM n-decane as an internal standard (for GC analysis) or by adding 0.1 vol of acetonitrile (for HPLC analysis).
For short-term assays (30 min), a cell concentration of 0.7–1 gCDW L−1 was used in a total volume of 1 ml in 10 ml Pyrex tubes. For the investigation of whole-cell kinetics, the cell concentration was reduced to 0.25–0.5 gCDW L−1 and the reaction time to 5 min to record initial activities.
Kinetic parameters (Vmax, KS and Ki) were obtained by fitting according to Michaelis–Menten kinetics with substrate inhibition using OriginPro2019 following Eq. 1, where V is the specific activity in U gCDW−1 and [S] is the substrate concentration.
For competitive and non-competitive inhibitors, Ks and Vmax in Eq. 1 were replaced by Ks’ according to Eq. 2 or Vmax’ according to Eq. 3, respectively, where [I] and Ki,I are the concentration and the inhibition constant of the respective inhibitor.
Production Process in a 2-L Stirred Tank Photo-Bioreactor
Whole-cell biotransformations were conducted in a stirred tank photo-bioreactor Labfors 5 Lux (Infors AG, Bottmingen, Switzerland) with 2 L culture volume. Cells were grown for 3–4 days at 30°C, 2 L min−1 aeration with (high carbon, HC, additional 20 ml min−1 CO2) or without (low carbon, LC, no additional CO2), 300 rpm stirrer speed, and specific light intensities (for details, see Table 1). Induction with 10 µM NiSO4 was performed 24 h prior to biotransformation start. During biotransformation, aeration was reduced to 0.2 L min−1 and light intensity was set to low light (LL, 150 µmolphotons m−2 s−1) or high light (HL, 700 µmolphotons m−2 s−1). After pre-conditioning for 30 min, biotransformation was initiated by starting a continuous feed of 0.25 M C-one in the YBG11 medium. Samples were taken at regular time intervals and quenched immediately by adding either 1 vol diethylether or 0.1 vol acetonitrile.
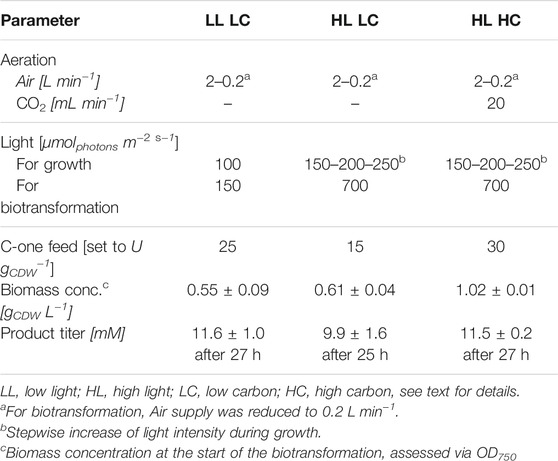
TABLE 1. Conditions and data for Syn6803_Ni_pBVMO-based C-one biotransformation in a 2-L photo STR (Infors AG, Bottmingen, Switzerland).
Analytics
The cell concentration was assessed by determining OD750 in a LibraS11 spectrophotometer (Biochrom, Cambridge, United Kingdom). The previously determined correlation factor of 0.225 gCDW L−1 per OD750 unit was used to calculate cell dry weight (CDW) concentrations (Hoschek et al., 2017). Chla concentration was determined as described by Grund et al. (2019). Concentrations of cyclohexanol (C-ol), C-one, and ε-Cl were determined by gas chromatography (GC) as described before (Schäfer et al., 2020). See Supplementary Figure S1 for exemplary calibration curves.
Concentrations of 6-hydroxyhexanoic acid (6-HA) were determined by HPLC on a Dionex Ultimate 3,000 system equipped with an Acclaim® OA column (both Thermo Fisher Scientific, Waltham, MA). The sample was acidified with 1 M HCl to pH 3.0. The mobile phase A consisted of 100 mM sodium sulfate, adjusted with methanesulfonic acid to pH 3. Acetonitrile (>99.95% purity, ProtoChem) was used as mobile phase B. Sample volumes of 10–20 μL were injected, while the flow and the column temperature were kept constant at 0.4 ml min−1 and 60°C, respectively. The flow profile was as follows: 5% B for 2 min, 5–30% B in 6 min, 30–80% B in 1 min, 80% B for 1 min, 80–5% B in 2 min, and 5% B for 5.5 min. Detection was accomplished via a UV detector at 210 nm.
Kinetic Bioprocess Modeling
For kinetic bioprocess modeling, the Berkeley Madonna software (version 8.3.18) was used. The biotransformation converting cyclohexanone (C-one) to ε-caprolactone (ε-Cl) as a product and cylohexanol (C-ol) as a by-product was described in a kinetic model. The concentration courses for substrate (S′, C-one), product (p′, ε-Cl), and by-product (B′, C-ol) were calculated using Eqs 4–6:
and
where the C-one feed rate F in mM min−1, the reaction rate of the keto reduction kB (C-ol formation), and of ε-Cl formation kP, described in Eqs 7, 8. The reaction rates kB and kP were calculated based on determined kinetic parameters (Ks and Vmax for keto reduction and Ks, Vmax, and Ki,C-one for ε-Cl formation); kP was extended by the competitive inhibition by C-ol and the non-competitive inhibition by ε-Cl as follows:
and
where the biomass concentration X is in gCDW L−1 and the inhibition constants Ki,C-ol, Ki,C-one, and Ki,ɛ-Cl for C-ol (B), C-one (S), and ɛ-Cl (p), respectively. See section 4.4 for the exact values chosen for the kinetic parameters.
S, P, and B were initially set to 0, and the parameters X (biomass concentration in gCDW L−1) and F (C-one feed rate in mM min−1) were fed into the model according to the respective experiment. The kinetic process model can be found in the supplemental material.
Results
Plasmid-Based Ni2+-Induced Gene Expression Leads to High Levels of Active BVMO
In order to systematically analyze different expression strategies in Syn6803, three different strains were constructed: Syn6803_Cu_pBVMO for plasmid-based BVMO gene expression under control of the Cu2+-inducible PpetE promoter and Syn6803_Ni_pBVMO and Syn6803_Ni_cBVMO for plasmid- and genome-based BVMO gene expression, respectively, under control of the Ni2+-inducible PnrsB promoter. All designed strains were compared in the detail regarding protein level and specific C-one to ε-CL conversion activity in short-term assays. Activities of the Ni2+-inducible strains Syn6803_Ni_pBVMO and Syn6803_Ni_cBVMO were found to increase with increasing Ni2+ concentration yielding maximum specific activities of 52.2 ± 0.3 (10 µM Ni2+) and 23.8 ± 4.0 U gCDW−1 (15 µM Ni2+), respectively (Figure 1A). The keto reduction of C-one to cyclohexanol (C-ol) was found to occur at a low rate of 0.5 ± 0.2 U gCDW−1 (for details, see Inhibitory Effects of Substrate and By-Product Necessitate Precise Reaction Control section). It is important to note that expression induced by 15 µM Ni2+ did not significantly affect growth of Syn6803_Ni_pBVMO, whereas this Ni2+ concentration slightly affected growth independently of BVMO gene expression (Supplementary Figure S2). Cu2+-induced Syn6803_Cu_pBVMO yielded a maximal specific activity of 29.7 ± 0.7 U gCDW−1 (with 0.5 µM Cu2+, Figure 1B). Ni2+-inducible systems resulted in tightly regulated expression, whereas the Cu2+-inducible system showed slight leakiness and poor titratability by the inducer concentration. Due to these reasons and the lower activity obtained upon plasmid-based expression, we focused on the Ni2+-inducible system and investigated plasmid- and genome-based expression in more detail. SDS-PAGE analyses of protein extracts of Syn6803_Ni_pBVMO and Syn6803_Ni_cBVMO showed a distinct band at ∼60 kDa, coinciding with the BVMO size (57.8 kDa) (Figures 1C,D). This band intensified with increasing Ni2+-concentration and was absent in un-induced cells and cells carrying an empty vector. Densitometric analysis indicated that BVMO accounted for 1.1 ± 0.2% of total soluble protein (TSP) in Syn6803_Ni_pBVMO induced with 10 µM Ni2+, a remarkably high value for heterologous expression in Syn6803. Assuming a total protein content of 0.32–0.57 g gCDW−1 (Zavrel et al., 2017), the measured specific activity of 52.2 ± 0.3 U gCDW−1 translates into an in vivo activity of 7–18 U mgBVMO−1 or a kcat of 6.9–17.6 s−1 as a rough estimation.
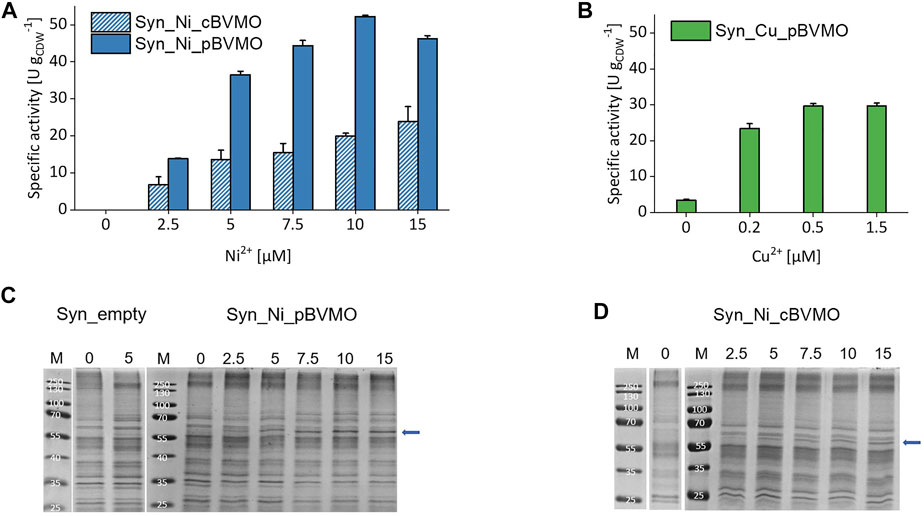
FIGURE 1. Systematic analysis of Ni2+- and Cu2+-dependent specific BVMO activities (A, B) and expression levels (C, D) in modified Syn6803 strains. BVMO activities of (A) Syn6803_Ni_pBVMO and Syn6803_Ni_cBVMO and (B) Syn6803_Cu_pBVMO determined in short-term assays are given in dependence of the inducer concentration. Depicted are means from ≥2 independent measurements. N.d. not detected; 1 U = 1 µmol product formed per min. (C,D) SDS-PAGE gels of protein extracts upon Ni2+-induced plasmid- and genome-based BVMO gene expression, respectively. Panel C also shows results for extracts of an empty vector control. Numbers above lanes indicate the Ni2+-concentrations applied in µM. Arrows indicate BVMO bands (57.8 kDa); M, marker (PageRuler™).
In summary, we were able to construct a highly active BVMO-containing Syn6803 strain. Our data show that the PnrsB-based expression system proved to be a useful tool allowing tight, tunable, and high-level expression. Syn6803_Ni_pBVMO was thus chosen for further characterization of photosynthesis-driven BVMO catalysis.
The BVMO Reaction in Synechocystis sp. PCC6803 Is Light Dependent
The BVMO reaction depends on NADPH as electron donor and thus is expected to constitute an artificial electron sink and thus to depend on the major electron source in phototrophs—the photosynthetic water oxidation. To test if and to what extent the BVMO reaction in Syn6803 depends on incident light, short-term activity assays at light intensities ranging from 0 to 250 µmolphotons m−2 s−1 were performed in medium deficient in organic compounds as potential electron donors. Clearly, specific activities increased with increasing light intensity with the maximal activity of 60.9 ± 1.0 U gCDW−1 measured at 150 µmolphotons m−2 s−1 (Figure 2A). With chlorophyll a content of 11.8 ± 0.8 mgChla gCDW−1, this activity translates into 5.2 ± 0.8 U mgChla−1. Higher light intensities (such as 250 µmolphotons m−2 s−1) led to a slight activity decrease, most likely due to photoinhibitory effects. When blocking electron transfer from PS II to the PQ pool by means of the respective inhibitor 3-(3,4-dichlorphenyl)-1,1-dimethylurea (DCMU) or upon incubation in the dark, residual activities of 13.6 ± 0.3 or 19.0 ± 0.8 U gCDW−1 were observed, respectively. These typical background/dark activities are most likely fueled by reducing equivalents derived from storage compounds. We could show that extended periods (up to 16 h) of incubation in the dark or with DCMU, which involves the consumption of storage compounds and thus a reduction of their availability, decreases the activities to 4.7 ± 0.1 and 1.3 ± 0.2 U gCDW−1, respectively (Figure 2B). These results clearly indicate a close coupling of the BVMO reaction to the photosynthetic electron transport chain.
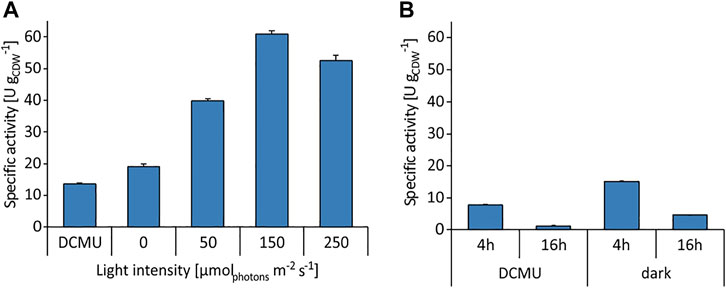
FIGURE 2. Specific BVMO activities of Syn6803_Ni_pBVMO (A) at different light intensities and (B) after extended pre-incubation in absence of photosynthetic water splitting. (A) Cells were grown under standard conditions and equilibrated at the given light intensities for 1 h before and during the assay. DCMU: Cells were treated with PS II inhibitor DCMU (20 µM final concentration) 1 h before and during the assay and irradiated with 150 µmolphotons m−2 s−1. Specific activities were measured in short-term assays applying a cell concentration of 0.7–1.0 gCDW L−1. (B) Cells were grown under standard conditions and then equilibrated with DCMU (at 150 µmolphotons m−2 s−1) or in the dark for the given incubation times followed by an activity assay under the same conditions. Depicted are means and standard deviation of ≥3 independent experiments.
Inhibitory Effects of Substrate and By-Product Necessitate Precise Reaction Control
To specify the optimal substrate concentration, the reaction kinetics were analyzed for Syn6803_Ni_pBVMO by varying the C-one concentration between 50 μM and 3 mM in 5 min assays. The cells showed Michaelis–Menten-type kinetics with slight substrate inhibition (Figure 3A). Fitting according to Michaelis–Menten kinetics with substrate inhibition gave a reasonable fit and the following kinetic parameters: KS = 79 ± 23 μM, Vmax = 83.7 ± 8.0 U gCDW−1, and Ki = 4.4 ± 1.5 mM. This indicates that the C-one concentration is a critical reaction parameter requiring careful control.
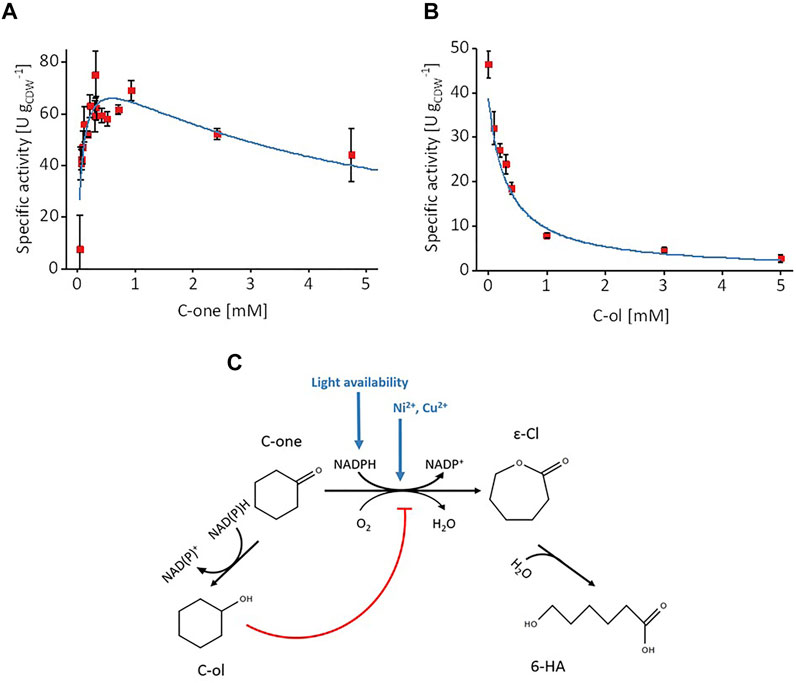
FIGURE 3. Kinetic characterization of C-one oxidation by Syn6803_Ni_pBVMO. (A) Kinetics for C-one oxidation. Kinetic experiments were performed with 1 ml induced cells (0.25–0.5 gCDW L−1) in 10 ml Pyrex tubes. BVMO reactions were started by adding varying amounts of C-one (50 μM–5 mM) to cells equilibrated at standard assay conditions for 10 min and was stopped after 5 min. (B) Effect of C-ol on C-one oxidation activity. Varying amounts of C-ol (0–5 mM) were added to 1 ml induced cells (0.7–1.0 gCDW L−1) in 10 ml Pyrex tubes. Also here, cells were equilibrated for 10 min, and the reaction was started by substrate addition. Reactions were stopped after 30 min. Depicted are mean values and standard deviations from ≥2 independent experiments. (C) Reactions and parameters influencing ɛ-Cl formation by Syn6803: BVMO-catalyzed conversion C-one into ε-Cl; intrinsic alcohol dehydrogenases catalyze C-one reduction to C-ol, which inhibits C-one oxidation. ε-Cl is hydrolyzed to 6-HA by intrinsic hydrolases.
C-one oxidation applying whole cells of both heterotrophic (Schäfer et al., 2020) and phototrophic hosts (Böhmer et al., 2017) has been reported to compete with keto reduction catalyzed by host-intrinsic enzymes, giving rise to C-ol (Figure 3C). This also was true for Syn6803_Ni_pBVMO, which was used for kinetic experiments investigating C-ol formation. Fitting according to Michaelis–Menten kinetics yielded a high KS of 7.4 ± 0.6 mM and a comparably low Vmax of 2.75 ± 0.15 U gCDW−1 (Supplementary Figure S3). Additionally, 6-hydroxyhexanoic acid (6-HA) was detected as by-product, which can be ascribed to abiotic hydrolysis of ε-CL (Supplementary Figure S4). Potential inhibition by these by-products also was tested in activity assays. Whereas 6-HA did not significantly affect C-one oxidation (Supplementary Figure S6), C-ol was found to be a strong inhibitor with a Ki of 0.03 mM, determined via kinetic fittings as described in Whole-cell activity assays and determination of reaction kinetics section (Figure 3B), corresponding to a Ki,C-ol/Ks,C-one ratio of 0.38. Figure 3C depicts a reaction scheme based on the findings described in this paragraph. Since intrinsic alcohol dehydrogenase activity can barely be avoided without drastically interfering with host metabolism, high BVMO levels can be considered pivotal to promote ε-CL formation. In this way, low yields due to by-product formation and—even more relevant—inhibition by C-ol can largely be avoided.
Gram Scale ɛ-Caprolactone Production in a 2-L Photo-Bioreactor
To test the long-term stability of C-one oxidation by Syn6803_Ni_pBVMO, we recorded the biotransformation time course in shaking flasks initially containing 5 mM C-one and fed by pulsing the same amount after 1 and 3 h. The 15 mM C-one supplied were completely converted to 14.2 ± 1.5 mM products (ε-Cl and 6-HA, Supplementary Figure S5). Thereby, the initial specific activity of 33 U gCDW−1 determined for the first hour was found to decrease continuously over time. This decrease can be attributed to C-ol accumulation, reaching a final titer of 0.51 ± 0.07 mM, and intermittently high C-one concentrations leading to enforced substrate inhibition.
In order to test the feasibility of Syn6803_Ni_pBVMO to produce ε-Cl in the gram scale, this strain was applied in a 2-L stirred-tank photo-bioreactor (photo-STR) providing an efficient O2 and CO2 mass transfer and a controlled environment. The latter included the application of a limiting continuous substrate feed to minimize both substrate and by-product inhibition. To establish different source/sink conditions, light intensity (low light, LL: 150 µmolphotons m−2 s−1 or high light, HL: 700 µmolphotons m−2 s−1) and CO2 supply via aeration (low CO2, LC: ambient CO2 or high CO2, HC, 1 and 10% CO2 during growth and biotransformation, respectively) were varied to establish the three source/sink regimes LLLC, HLLC, and HLHC (for details, see Table 1).
After a growth phase of 3–4 days, including induction by adding 10 µM NiSO4 24 h before biotransformation start, C-one feeding was initiated at a fixed rate matching a specifically chosen activity. The first reactor was run with 0.55 ± 0.09 gCDW L−1 under LLLC and a feed rate set to 25 U gCDW−1. A final product titer (sum of ε-Cl and 6-HA) of 11.6 ± 1.0 mM was reached after 28 h (see Figure 4A). However, 5.4 ± 0.1 mM C-one remained unconverted, as the specific activity strongly decreased within the first hours. A second reactor was set up for HLLC conditions to rule out light limitation as a reason for the decreasing activity. To avoid substrate accumulation, the initial substrate feed rate was adjusted to match an activity of 15 U gCDW−1 using 0.61 ± 0.04 gCDW L−1. This lead to a prolonged product formation phase, yielding however a slightly lower final product titer of 9.8 ± 1.6 mM after 25 h (Figure 4B). To test, if product formation benefits from higher biomass concentrations, the third photo-STR was conducted using HLHC conditions. These conditions allowed for a 70% higher initial biomass concentration (1.02 ± 0.01 gCDW L−1, see Table 1). To increase the C-one conversion rate, substrate supply was set to match 30 U gCDW−1. Comparable to the previous reactor runs, this preset activity of 30 U gCDW−1 only was met initially and was decreasing in time, resulting in C-one accumulation, requiring adjustment of the C-one feed rate (decrease to 70% after 4 h and stop of C-one feed after 8 h, Figure 4C). The final product titer was 11.5 ± 0.2 mM after 27 h, corresponding to a volumetric productivity of 48.6 ± 0.8 mg L−1 h−1 and a product yield of 0.88 (molε-Cl+6-HA molC-one−1). Thus, all experiments led to similar product titers (9.8–11.6 mM corresponding to 1.12–1.32 g L−1).
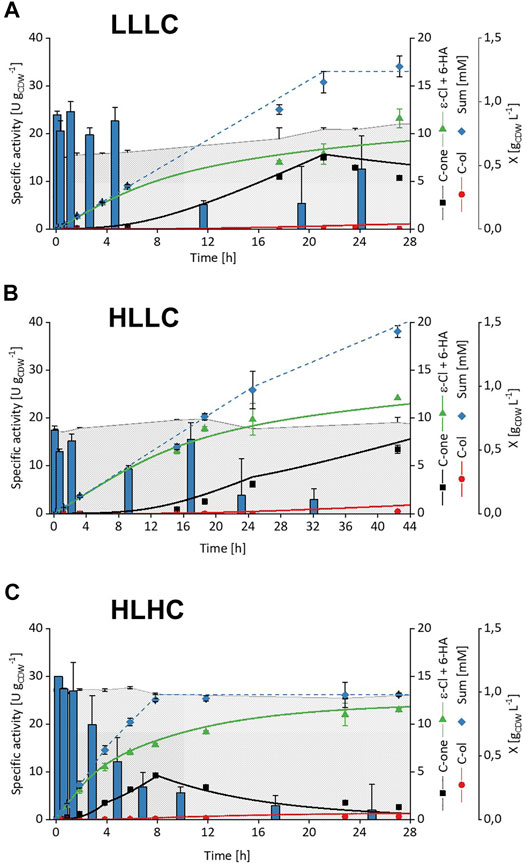
FIGURE 4. Photosynthesis-driven C-one oxidation in a 2-L stirred tank photo-bioreactor under different light and CO2 regimes. Syn6803_Ni_pBVMO was grown for 3–4 days and induced with 10 µM NiSO4. Biotransformations were started 24 h after induction by feeding C-one in a substrate-limited regime, for detailed parameters see Table 1. (A) Low light/low carbon (LLLC): C-one was fed at a rate matching a specific activity of 25 U gCDW−1 for 21.5 h with an initial biomass concentration of 0.55 ± 0.09 gCDW L−1. (B) High light/low carbon (HLLC): C-one was fed at a rate matching 15 U gCDW−1 for 24.5 h and then at a rate matching 10 U gCDW−1; the initial biomass concentration was 0.61 ± 0.04 gCDW L−1. (C) High light/high carbon (HLHC): C-one was fed at a rate matching 30 U gCDW−1 for 4 h, which then was decreased by 30% until 8 h; the initial biomass concentration was of 1.02 ± 0.01 gCDW L−1. Experimental data are given as blue bars: specific activity in U [= µmol (ε-Cl + 6-HA) min−1] gCDW −1; black squares: C-one in mM; green triangles: total product in mM; red circles: C-ol in mM; and blue circles: sum of all analytes in mM. Depicted are mean values and standard deviations from ≥2 technical replicates. Dashed line (blue): Total C-one fed in mM. Straight lines depict simulated courses of C-one (black), ε-Cl (green), and C-ol (red) concentrations obtained via Berkeley Madonna software using a model based on determined and fitted kinetic parameters (see text for details).
Irrespective of the applied source/sink conditions, the specific activity decreased in all setups at substrate (C-one) and by-product (C-ol) levels not expected to lead to significant inhibition (Figures 4A–C). The independency of initial specific activities on the applied biomass concentration (varied between 0.55 and 1.02 gCDW L−1) indicates that light availability did not limit the specific activity (Table 1 and Figures 4A–C). This was further confirmed by light intensity variation (250 µmolphotons m−2 s−1 vs. 700 µ µmolphotons m−2 s−1) under otherwise identical conditions as in the HLHC experiment (Figure 4C). This low-light high-carbon (LLHC) experiment resulted in a similar initial volumetric productivity as in the HLHC experiment corroborating that light did not limit the biocatalytic activity (Supplementary Table S2). These results indicate that neither inhibition by C-one or C-ol nor photosynthesis limited the biocatalytic activity. Instead, product inhibition may have prevented a more effective and stable conversion. Indeed, ε-Cl was found to compromise BVMO activity in short term assays (Supplementary Figure S6). As no product inhibition has been observed on the enzyme level (Schäfer et al., 2020), ε-Cl rather inhibits on a physiological level, which can best be described as a non-competitive type of inhibition. With the respective fit (Eqs 1, 3), the inhibitory studies yielded a Ki,ε-Cl of 0.83 mM.
These findings prompted us to describe the biotransformations by means of a kinetic bioprocess model using Berkeley Madonna software. The model was parametrized using the kinetic data reported in the Potentials and Limitations of Photosynthesis-Driven Redox Biocatalysis section, which were derived from fitting according to Michaelis–Menten kinetics with substrate inhibition (Eq. 1). Taking into account that this approach neglects by-product and product inhibition, which occur, but were not considered for the determination of Vmax and Ki,C-one, these parameters were set to the upper boundaries of the fittings, namely, 91 U gCDW−1 and 5.9 mM. The inhibition by ε-Cl observed under assay conditions (Supplementary Figure S6, Ki,ε-Cl = 0.83 mM) appeared to be less pronounced in bioreactor experiments. Thus, Ki,ε-Cl was fitted to bioreactor data resulting in a value of 2.0 mM. This allowed to simulate concentration courses for substrate (C-one), product (ε-Cl + 6-HA), and by-product (C-ol) (depicted as lines in Figures 4A–C) with striking accuracy. This high simulation accuracy obtained with a mere kinetic model, not considering possible influences of light intensity and CO2 supply variation in the experiments, indicates that C-one conversion was kinetically controlled under the conditions applied, including a physiological effect of ε-Cl, and was not influenced by the tested variation in source/sink regime.
Furthermore, the process model was used to identify optimal process parameters to handle the complex reaction kinetics, namely, the feed regime and the biocatalyst concentration. For this purpose, only reaction kinetics were considered, and avoidance of a possible light limitation was assumed. To explore the sensitivity regarding the biocatalyst concentration, values of 0.5, 1, 2, and 5 gCDW L−1 were chosen, being aware that high cell concentrations such as 5 gCDW L−1 would involve light limitation issues in the experimental setup (as further discussed in the Potentials and Limitations of Photosynthesis-Driven Redox Biocatalysis section). Thereby, the feed regime applied in the HLHC experiment (Figure 4C) was scaled to different biocatalyst concentrations (Figure 5A). The highest biomass concentration tested (5 gCDW L−1) indeed yielded the highest product titer (17.0 mM) but accumulated C-one (36.9 mM) and C-ol (11.4 mM) in high amounts. To avoid extreme substrate and by-product accumulation and thereby optimize product titer and yield, a more conservative C-one feed was targeted. To this end, an ideal C-one conversion rate was calculated by considering product inhibition only and neglecting substrate and by-product inhibition, reflecting the upper limit of C-one supply which can be handled by the catalyst. This continuously decreasing rate was translated into a feed rate followed by simulation using the kinetic model, again testing different biocatalyst concentrations. Irrespective of the initial feed rate (30, 20, or 10 U gCDW L−1, Figures 5B–D), the overall picture remained the same: Higher biocatalyst concentrations (2 and 5 gCDW L−1) heavily accumulated C-one and—to a minor extent—C-ol, limiting product formation. Although lower biocatalyst concentrations (0.75 and 1 gCDW L−1) suffered less C-one accumulation and consequential C-ol formation, the final product titer did not exceed 10.7 mM. To conclude, the “optimized” C-one feed regime led to only a moderate increase in final product titer compared to the HLHC experiment (15.5 vs. 11.5 mM), but only with 5 times higher biocatalyst concentration and thus on the cost of low biocatalyst-based yield (0.4 vs. 1.3 g ε-Cl+6-HA gCDW−1) and product yield (0.15 vs. 0.88 molε-Cl+6-HA molC-one−1). With the same biocatalyst concentration as in the HLHC experiment, the achieved product titer was even lower (10.7 vs. 11.5 mM), which emphasizes the strong impact of the by-product C-ol demanding a more aggressive feed rate decrease. Overall, the reaction system remains intricate, as product titer and yield are not only restricted by product inhibition but also by the difficile interplay between competitive by-product inhibition and the formation of this by-product: A high substrate concentration can counteract competitive inhibition but simultaneously increases inhibitor formation. Importantly, assuming the absence of light limitation for higher cell densities did not enable significantly higher product titers than experimentally obtained, reemphasizing that kinetic restrictions are the primary factors limiting the performance of the investigated reaction system.
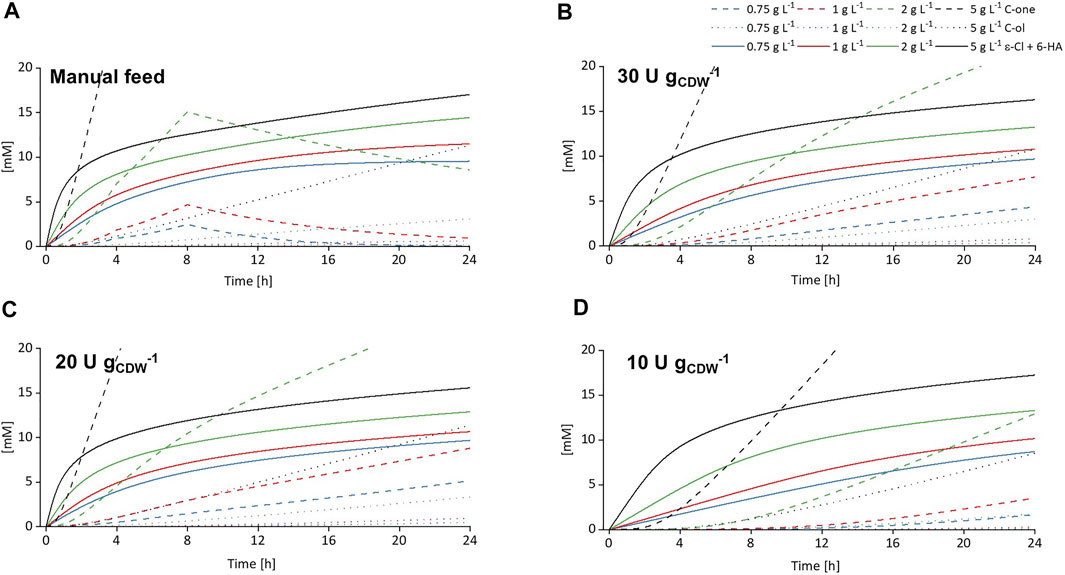
FIGURE 5. Simulation and sensitivity analysis of the BVMO process applying the kinetic process model with (A) manual and (B, C), and (D) optimized feeding regimes, with 30 U gCDW−1, 20 U gCDW−1, and 10 U gCDW−1, respectively. (A) The feed regime applied for the HLHC reactor (see Figure 4) was translated to 0.75, 1.0, 2.0, and 5.0 gCDW L−1 for a sensitivity analysis regarding the biocatalyst concentration (B/C/D). An ideal C-one conversion rate based on initial rates of 30/20/10 U gCDW−1, respectively, was calculated by considering product inhibition only and neglecting substrate and by-product inhibition. This reflects the upper limit of C-one supply which can be handled by the catalyst. The conversion rate was translated into a feed rate, followed by simulations using the kinetic process model with cell concentrations of 0.75, 1.0, 2.0, and 5.0 gCDW L−1 (blue, red, green, and black lines), to test the system’s sensitivity towards the biocatalyst concentration. Depicted are concentrations of C-one, C-ol, and ɛ-Cl in dashed, dotted, and straight lines, respectively.
Discussion
Photosynthesis is the central biochemical transformation process converting light energy into chemical energy and therefore a highly attractive module for environmentally friendly industrial processes. It can be exploited for biotechnology by making use of photosynthetically active microorganisms such as cyanobacteria (Barber, 2009); (Angermayr et al., 2009). Their capability to fix CO2 gives rise to the conversion of CO2 into organic chemicals such as biofuels (Gao et al., 2016). Inter alia, the production of ethanol (Gao et al., 2012), 2,3-butanediol (Oliver et al., 2013), glycerol (Savakis et al., 2015), and 1-butanol (Atsumi et al., 2009) has been investigated, typically achieving rather low productivities, not exceeding 10 mg L−1 h−1. These processes mainly suffer low energy efficiency as respective metabolic pathways are linked to carbon fixation and central carbon metabolism, which are evolutionary optimized for biomass formation under given conditions and not explicitly with respect to energy efficiency. One option to increase light-to-product energy efficiency is to link production processes closer to the photosynthetic electron transport chain and thus avoid downstream energy loss (Barber, 2009).
Following this idea, recent studies focused on the utilization of photosynthesis-derived electrons for redox biocatalysis. The coupling is realized either by directly linking electron consuming enzymes to PS I (Lassen et al., 2014a; Appel et al., 2020) or via reduced cofactors produced by the light reaction (Hoschek et al., 2019b; Assil-Companioni et al., 2020), for example, NADPH as in the presented study. This approach is particularly elegant for oxygenation reactions as not only electron supply but also O2 supply—often a limiting factor in aerobic large-scale bioprocesses—is realized in situ via photosynthetic water oxidation (Hoschek et al., 2018). In this study, the use of a 2-L stirred tank photo-bioreactor setup enabled light-driven ε-Cl formation in the gram scale within 1 day exemplifying the potential of this approach.
Enhanced Gene Expression Makes Synechocystis sp. PCC6803 an Attractive Biocatalyst
The development and application of photosynthetically active microorganisms as microbial hosts is still restricted by the limited availability of molecular biology tools and the comparably poor knowledge on cell metabolism and physiology. Here, we investigated different expression systems regarding expression levels and specific activities of BVMO from Acidovorax sp. CHX100 in Syn6803. The Ni2+-inducible PnrsB promoter system was found to enable high BVMO expression levels and be superior to the Cu2+-inducible PpetE promoter system in terms of inducibility and expression level, in accordance with previous studies (Englund et al., 2016; Liang et al., 2018). It is important to note that the expression level achieved with a promoter depends on the genetic context (Englund et al., 2016). The expression levels of up to 1.1 ± 0.2% of TSP reached in this study are to our best knowledge unprecedented with the PnrsB system in Syn6803. Numerous studies have characterized different controllable promoter systems in Syn6803: non-native ones such as the LacI-repressible Ptrc1O system (Huang et al., 2010), the TetR-regulated L03 promoter (Huang and Lindblad, 2013), and other Plac variants (Thiel et al., 2018) as well as native ones like Cu2+-inducible PpetE (Briggs et al., 1990) and Ni2+-inducible PnrsB promoters (Englund et al., 2016). However, most studies focused on the optimization of expression systems rather than on designing suitable biocatalysts and use fluorescent proteins as read-out for expression strength. Thus, not much is known about the cellular concentrations of heterologous protein. For catalytically active enzymes, such as dehydrogenases or monooxygenases (Lassen et al., 2014a), constitutive systems, such as the discovered “super strong” promoter Pcpc560 (Zhou et al., 2014) or the light-inducible promoter PpsbA, have been applied (Wang et al., 2018). They yield high expression levels up to 15% (Zhou et al., 2014) and 12.6% of TSP (Wang et al., 2018). However, for oxygenase biocatalysis, well-steerable promoter systems are more desirable to control metabolic burden such as ROS formation. They yield lower but still remarkable expression levels of 0.4% (Sebesta and Peebles, 2020) and 1.1% of TSP (Savakis et al., 2015), of which the latter was met by Syn6803_Ni_pBVMO generated in this study.
With PnrsB, plasmid-based expression yielded a 2.6 times higher BVMO level and activity than chromosome-based expression (Figure 1). This difference may be due to a higher copy number of the plasmid than the chromosome (Huang et al., 2010). It however has been reported that the copy number of pPMQAK1 is comparable to that of the chromosome (Jin et al., 2018). Such contradiction has been explained by the observation that both plasmid and chromosome copy numbers vary strongly in Syn6803 (Heidorn et al., 2011). This makes chromosomal integration the more laborious method due to the necessity for segregation, whereas the plasmid-based approach can suffer instability and the necessity for constant antibiotic selection (Kadisch et al., 2017).
The strong correlation of specific BVMO activity and the expression level (Figure 1) suggests that the achieved BVMO level still limits the biocatalyst activity. Future advances regarding molecular biology tools for cyanobacteria can be expected to enable even higher biocatalyst activities. Promising candidate expression systems include the recently characterized rhamnose-inducible promoter PrhaBAD (Kelly et al., 2018) or the vannilate-inducible promoter PvanCC (Behle et al., 2020).
Photosynthesis-Driven BVMO Catalysis Is Hampered by Reactant Inhibition Rather Than Host Metabolism
BVMO containing Syn6803 was found to feature a 3.7 times lower apparent uptake constant KS for C-one (80 ± 23 µM) than Pseudomonas taiwanensis VLB 120 containing the same enzyme (316 ± 21 µM) (Bretschneider et al., 2021a). This indicates a rather efficient substrate mass transfer into the cell. Furthermore, we observed moderate substrate inhibition, an often encountered effect with BVMOs (Scherkus et al., 2017; Fürst et al., 2019; Schmidt and Bornscheuer, 2020). The host metabolic background interferes with the biotransformation in terms of C-one reduction to C-ol as reported before (Böhmer et al., 2017), but with a rather low Vmax (2.75 ± 0.15 mM) and a rather high KS (7.4 ± 0.6 mM). More important was the inhibition of BVMO activity by C-ol already at low concentrations (Ki = 0.03 mM). This is in agreement with published data on heterotrophs containing the same enzyme (Schäfer et al., 2020), indicating an inhibitory effect on the enzyme level. To reduce formation of the (inhibiting) by-product, the introduction of an enzyme for re-conversion of C-ol to C-one could be an interesting solution (Bayer et al., 2017). Deletion of intrinsic alcohol dehydrogenases constitute another option but may harm cell viability and thus be tedious. Taken together, these data show that controlling substrate concentration is key for efficient photosynthesis-driven BVMO catalysis.
The optimized strain Syn6803_Ni_pBVMO reached a maximal specific activity of 60.9 ± 1.0 U gCDW−1. This is around 4 to 5 times lower than published for the heterotrophic host Pseudomonas taiwanensis VLB120 (Schäfer et al., 2020), which showed significantly higher BVMO expression levels (Bretschneider et al., 2021a) and features a highly active energy and redox metabolism based on organic acids and sugars (Volmer et al., 2014). It is however noteworthy that the BVMO exhibited a much higher in vivo activity in Syn6803 than in P. taiwanensis, that is, a 4.6 to 11 times higher kcat (6.9–17.6 s−1 vs. 1.5 s−1 (Bretschneider et al., 2021a)). It should be noted that BVMO catalysis in Syn6803 appeared not to be limited by the metabolic capacity to supply reduction equivalents as the photoproduction of NADPH in Syn6803 is in the range of 9–18 U mg Chla (Kauny and Setif, 2014), which translates to 106–212 U gCDW−1. Presumably, this limitation was decisive for BVMO catalysis in P. taiwanensis. The activities achieved already indicate the great potential of Syn6803 as host for energy-dependent biotransfomations. Future research efforts to fill knowledge gaps regarding expression systems, other molecular biology tools, and cofactor regeneration can be expected to further improve photosynthesis-driven biotransformation efficiency (Hitchcock et al., 2020).
Despite keeping by-product and substrate strictly below inhibiting values, the biocatalyst performance was found to be compromised in the long term. Neither increased light nor CO2 supply had a positive effect (Figure 4B). Instead, the product ɛ-Cl itself was found to affect biocatalyst performance (Supplementary Figure S6). Such product inhibition also was the decisive limitation in simulations based on kinetic data, which overall fit well the bioreactor data. Interestingly, product inhibition was not detected with heterotrophic hosts (Schäfer et al., 2020) and seems to be Syn6803 specific. Effects of ɛ-Cl on Syn6803 physiology may involve inhibition of metabolism leading to NADPH limitation as discussed below. To address product inhibition, in situ product removal technologies can be applied (Dafoe and Daugulis, 2014). Whereas two liquid-phase approaches are compromised by the intermediary polarity of the product, solid-phase extraction may be a promising but costly solution. Alternatively, BVMO catalysis can be amended by a lipase (Scherkus et al., 2017) or lactonase (Schäfer et al., 2020) reaction to obtain 6-HA which is less problematic in terms of inhibition but still feasible as a polymer precursor.
Potentials and Limitations of Photosynthesis-Driven Redox Biocatalysis
The biocatalyst-based yield (gProduct gCDW−1) has been proposed as an important measure for catalyst performance (Fürst et al., 2019). Syn6803_Ni_pBVMO reached a yield of 1.3 and 1.6 gProduct gCDW−1 in a 2-L photo-bioreactor and in shaking flasks, respectively. A sensitivity analysis based on the kinetic model affirmed a yield of 1.5 gProduct gCDW−1 to be realistic for the used bioreactor setup. This is on the same level as an industry-relevant BVMO-based process with Escherichia coli featuring a yield of 1.6 gProduct gCDW−1 for a however more complex product (Woo et al., 2018). However, phototrophic processes are not on the same readiness level as heterotrophic biocatalytic processes: It should be noted that the abovementioned metric neglects the immense differences in cell densities applied. The mentioned E. coli–based process uses around 25-times higher biomass concentrations than those applied in this study and thus features a higher volumetric productivity. On the other hand, Syn6803-based biocatalyst production, regeneration, and operation are based on CO2 as carbon and water as electron source, making the addition of an organic carbon and energy source such as glucose dispensable. Future improvements of reactor design and cultivation strategies such as the use of capillary biofilm reactor concepts (Karande et al., 2014; Hoschek et al., 2019a) or immobilized cells (Vajravel et al., 2020) and internal illumination (Hobisch et al., 2021) are expected to push the boundaries of phototrophic cultivation/process operation. Already noteworthy are the initial and 24 h-average productivity (187 ± 1 mg L−1 h−1 and 59 ± 1 mg L−1 h−1, respectively), ranging among the highest productivities for biotransformations using cyanobacteria. Additionally, the product yield of 0.88 (molε-Cl+6-HA molC-one−1) prefigures the high potential of phototrophic hosts for biocatalysis.
The comparably high in vivo BVMO activity obtained in this study indicates that—sufficient light supply provided—NADPH can efficiently be withdrawn from phototrophic metabolism to fuel productive reactions. As confirmed by process simulation merely based on whole-cell reaction kinetics, the photosynthesis-based NADPH supply capacity did not limit the biotransformation, with efficient illumination as a prerequisite. The product itself might interfere with NADPH supply at higher concentrations by affecting membrane integrity (Sikkema et al., 1995) and thus photosynthetic electron transfer. In contrast, electron consuming redox biotransformations catalyzed by the ene-reductase Yqjm were found to be limited by NADPH availability at activities above 150 U gCDW−1 (Assil-Companioni et al., 2020). By inactivating the proteins Flv1/Flv3, an approach known to reduce electron loss to unproductive O2 reductions (Thiel et al., 2019), Syn6803 reached specific activities up to 170 U gCDW−1. It has to be investigated though, if this elevated electron supply can be sustained in the long term.
As oxygenases are prone to uncouple, that is, reduce O2 without concomitant substrate oxidation, ROS formation represents another electron sink increasing the electron demand. Furthermore, ROS formation possibly affects photosynthetic metabolism (Nishiyama et al., 2005). Cyanobacteria have evolved different mechanisms to cope with and detoxify ROS (Latifi et al., 2009), which however involves additional electron/energy investment further increasing the electron demand.
Today, the disposability of electrons from water oxidation, for example, by water electrolysis, is a field of particular interest, especially for H2 production. In this context, envisaged light-to-H2 solutions using cyanobacterial photosynthesis as a transformation module are highly attractive (Appel et al., 2020). Success towards this goal relies, beside O2 tolerance of biocatalysts and high cell-density cultivation concepts, on the electron supply capacity of photoautotrophic microbes. It is still unclear, if a high electron demand for technically feasible H2 production can be met by cells still able to sustain themselves. Interestingly, additional electron sinks were shown to increase photosynthetic efficiency and thereby electron fluxes through the photosynthetic apparatus (Zhou et al., 2016; Grund et al., 2019). This observation reinforces the sink-limitation hypothesis, which states that photosynthesis is rather limited by the availability of electron sinks than by electron (energy) sources (Berepiki et al., 2016). This gives the possibility to tap unused electron supply potential for electron consuming reactions. Limits of and factors influencing electron supply via the photosynthetic light reaction for biotechnological application remain to be investigated. Reductases, such as Yqjm (Assil-Companioni et al., 2020), and oxygenases, such as the cycloalkane hydroxylating cytochrome P450 monoxygenase (Hoschek et al., 2019b) and the BVMO investigated in this study, have now been established as prominent electron sinks in cyanobacteria and constitute excellent blueprints to decipher the potential of the photosynthetic light reaction as electron source for biotechnological application.
Data Availability Statement
The original contributions presented in the study are included in the article/Supplementary Materials; further inquiries can be directed to the corresponding authors.
Author Contributions
AT, AS, BB, and JT were involved in the conception and design of the study. AT, MU, and FN performed experimental work and collected data. AT, BB, and JT did data curation and interpretation. AT wrote the original draft of the manuscript. BB and JT contributed in terms of article structuring and editing. All authors were involved in editing and approved the submitted version.
Funding
AT is funded by the German National Academic Foundation.
Conflict of Interest
The authors declare that the research was conducted in the absence of any commercial or financial relationships that could be construed as a potential conflict of interest.
Publisher’s Note
All claims expressed in this article are solely those of the authors and do not necessarily represent those of their affiliated organizations, or those of the publisher, the editors, and the reviewers. Any product that may be evaluated in this article, or claim that may be made by its manufacturer, is not guaranteed or endorsed by the publisher.
Acknowledgments
We acknowledge the use of the facilities of the Centre for Biocatalysis (MiKat) at the Helmholtz Centre for Environmental Research, which is supported by European Regional Development Funds (EFRE, Europe funds Saxony) and the Helmholtz Association.
Supplementary Material
The Supplementary Material for this article can be found online at: https://www.frontiersin.org/articles/10.3389/fctls.2021.780474/full#supplementary-material
References
Angermayr, S. A., Hellingwerf, K. J., Lindblad, P., and Teixeira de Mattos, M. J. (2009). Energy Biotechnology with Cyanobacteria. Curr. Opin. Biotechnol. 20 (3), 257–263. doi:10.1016/j.copbio.2009.05.011
Appel, J., Hueren, V., Boehm, M., and Gutekunst, K. (2020). Cyanobacterial In Vivo Solar Hydrogen Production Using a Photosystem I-Hydrogenase (PsaD-HoxYH) Fusion Complex. Nat. Energ. 5, 458–467. doi:10.1038/s41560-020-0609-6
Assil-Companioni, L., Büchsenschütz, H. C., Solymosi, D., Dyczmons-Nowaczyk, N. G., Bauer, K. K. F., Wallner, S., et al. (2020). Engineering of NADPH Supply Boosts Photosynthesis-Driven Biotransformations. ACS Catal. 10 (20), 11864–11877. doi:10.1021/acscatal.0c02601
Atsumi, S., Higashide, W., and Liao, J. C. (2009). Direct Photosynthetic Recycling of Carbon Dioxide to Isobutyraldehyde. Nat. Biotechnol. 27 (12), 1177–1180. doi:10.1038/nbt.1586
Baldwin, C. V. F., and Woodley, J. M. (2006). On Oxygen Limitation in a Whole Cell Biocatalytic Baeyer-Villiger Oxidation Process. Biotechnol. Bioeng. 95 (3), 362–369. doi:10.1002/bit.20869
Barber, J. (2009). Photosynthetic Energy Conversion: Natural and Artificial. Chem. Soc. Rev. 38 (1), 185–196. doi:10.1039/b802262n
Bayer, T., Milker, S., Wiesinger, T., Winkler, M., Mihovilovic, M. D., and Rudroff, F. (2017). In Vivo Synthesis of Polyhydroxylated Compounds from a “Hidden Reservoir” of Toxic Aldehyde Species. ChemCatChem 9 (15), 2919–2923. doi:10.1002/cctc.201700469
Behle, A., Saake, P., Germann, A. T., Dienst, D., and Axmann, I. M. (2020). Comparative Dose-Response Analysis of Inducible Promoters in Cyanobacteria. ACS Synth. Biol. 9, 843–855. doi:10.1021/acssynbio.9b00505
Berepiki, A., Hitchcock, A., Moore, C. M., and Bibby, T. S. (2016). Tapping the Unused Potential of Photosynthesis with a Heterologous Electron Sink. ACS Synth. Biol. 5 (12), 1369–1375. doi:10.1021/acssynbio.6b00100
Berla, B. M., Saha, R., Immethun, C. M., Maranas, C. D., Moon, T. S., and Pakrasi, H. B. (2013). Synthetic Biology of Cyanobacteria: Unique Challenges and Opportunities. Front. Microbiol. 4, 246. doi:10.3389/fmicb.2013.00246
Böhmer, S., Köninger, K., Gómez-Baraibar, Á., Bojarra, S., Mügge, C., Schmidt, S., et al. (2017). Enzymatic Oxyfunctionalization Driven by Photosynthetic Water-Splitting in the Cyanobacterium Synechocystis Sp. PCC 6803. Catalysts 7 (8), 240. doi:10.3390/catal7080240
Bornscheuer, U. T., Huisman, G. W., Kazlauskas, R. J., Lutz, S., Moore, J. C., and Robins, K. (2012). Engineering the Third Wave of Biocatalysis. Nature 485 (7397), 185–194. doi:10.1038/nature11117
Bradford, M. M. (1976). A Rapid and Sensitive Method for the Quantitation of Microgram Quantities of Protein Utilizing the Principle of Protein-Dye Binding. Anal. Biochem. 72, 248–254. doi:10.1016/0003-2697(76)90527-3
Bretschneider, L., Heuschkel, I., Ahmed, A., Bühler, K., Karande, R., and Bühler, B. (2021a). Characterization of Different Biocatalyst Formats for BVMO‐catalyzed Cyclohexanone Oxidation. Biotechnol. Bioeng. 118, 2719–2733. doi:10.1002/bit.27791
Bretschneider, L., Wegner, M., Bühler, K., Bühler, B., and Karande, R. (2021b). One‐pot Synthesis of 6‐aminohexanoic Acid from Cyclohexane Using Mixed‐species Cultures. Microb. Biotechnol. 14 (3), 1011–1025. doi:10.1111/1751-7915.13744
Briggs, L. M., Pecoraro, V. L., and McIntosh, L. (1990). Copper-induced Expression, Cloning, and Regulatory Studies of the Plastocyanin Gene from the Cyanobacterium Synechocystis Sp. PCC 6803. Plant Mol. Biol. 15 (4), 633–642. doi:10.1007/BF00017837
Burk, M. J., and Van Dien, S. (2016). Biotechnology for Chemical Production: Challenges and Opportunities. Trends Biotechnol. 34 (3), 187–190. doi:10.1016/j.tibtech.2015.10.007
Camsund, D., and Lindblad, P. (2014). Engineered Transcriptional Systems for Cyanobacterial Biotechnology. Front. Bioeng. Biotechnol. 2, 40. doi:10.3389/fbioe.2014.00040
Dafoe, J. T., and Daugulis, A. J. (2014). In Situ product Removal in Fermentation Systems: Improved Process Performance and Rational Extractant Selection. Biotechnol. Lett. 36 (3), 443–460. doi:10.1007/s10529-013-1380-6
Englund, E., Liang, F., and Lindberg, P. (2016). Evaluation of Promoters and Ribosome Binding Sites for Biotechnological Applications in the Unicellular Cyanobacterium Synechocystis Sp. PCC 6803. Sci. Rep. 6, 36640. doi:10.1038/srep36640
Fürst, M. J. L. J., Gran-Scheuch, A., Aalbers, F. S., and Fraaje, M. W. (2019). Baeyer-Villiger Monooxygenases: Tunable Oxidative Biocatalysts. ACS Catal. 9, 11207–11241. doi:10.10.21/acscatal.9b03396
Gale, G. A. R., Schiavon Osorio, A. A., Mills, L. A., Wang, B., Lea-Smith, D. J., and McCormick, A. J. (2019). Emerging Species and Genome Editing Tools: Future Prospects in Cyanobacterial Synthetic Biology. Microorganisms 7 (10), 409. doi:10.3390/microorganisms7100409
Gao, X., Sun, T., Pei, G., Chen, L., and Zhang, W. (2016). Cyanobacterial Chassis Engineering for Enhancing Production of Biofuels and Chemicals. Appl. Microbiol. Biotechnol. 100 (8), 3401–3413. doi:10.1007/s00253-016-7374-2
Gao, Z., Zhao, H., Li, Z., Tan, X., and Lu, X. (2012). Photosynthetic Production of Ethanol from Carbon Dioxide in Genetically Engineered Cyanobacteria. Energy Environ. Sci. 5 (12), 9857–9865. doi:10.1039/C2EE22675H
Gibson, D. G., Young, L., Chuang, R.-Y., Venter, J. C., Hutchison, C. A., and Smith, H. O. (2009). Enzymatic Assembly of DNA Molecules up to Several Hundred Kilobases. Nat. Methods 6 (5), 343–345. doi:10.1038/nmeth.1318
Gordon, G. C., and Pfleger, B. F. (2018). Regulatory Tools for Controlling Gene Expression in Cyanobacteria. Adv. Exp. Med. Biol. 1080, 281–315. doi:10.1007/978-981-13-0854-3_12
Grund, M., Jakob, T., Wilhelm, C., Bühler, B., and Schmid, A. (2019). Electron Balancing under Different Sink Conditions Reveals Positive Effects on Photon Efficiency and Metabolic Activity of Synechocystis Sp. PCC 6803. Biotechnol. Biofuels 12, 43. doi:10.1186/s13068-019-1378-y
Hanahan, D. (1983). Studies on Transformation of Escherichia coli with Plasmids. J. Mol. Biol. 166 (4), 557–580. doi:10.1016/s0022-2836(83)80284-8
Heidorn, T., Camsund, D., Huang, H.-H., Lindberg, P., Oliveira, P., Stensjö, K., et al. (2011). Synthetic Biology in Cyanobacteria. Methods Enzymol. 497, 539–579. doi:10.1016/B978-0-12-385075-1.00024-X
Hitchcock, A., Hunter, C. N., and Canniffe, D. P. (2020). Progress and Challenges in Engineering Cyanobacteria as Chassis for Light‐driven Biotechnology. Microb. Biotechnol. 13 (2), 363–367. doi:10.1111/1751-7915.13526
Hobisch, M., Spasic, J., Malihan‐Yap, L., Barone, G. D., Castiglione, K., Tamagnini, P., et al. (2021). Internal Illumination to Overcome the Cell Density Limitation in the Scale‐up of Whole‐Cell Photobiocatalysis. ChemSusChem 14 (15), 3219–3225. doi:10.1002/cssc.202100832
Hoschek, A., Bühler, B., and Schmid, A. (2017). Overcoming the Gas-Liquid Mass Transfer of Oxygen by Coupling Photosynthetic Water Oxidation with Biocatalytic Oxyfunctionalization. Angew. Chem. Int. Ed. 56 (47), 15146–15149. doi:10.1002/anie.201706886
Hoschek, A., Heuschkel, I., Schmid, A., Bühler, B., Karande, R., and Bühler, K. (2019a). Mixed-species Biofilms for High-Cell-Density Application of Synechocystis Sp. PCC 6803 in Capillary Reactors for Continuous Cyclohexane Oxidation to Cyclohexanol. Bioresour. Technol. 282, 171–178. doi:10.1016/j.biortech.2019.02.093
Hoschek, A., Schmid, A., and Bühler, B. (2018). In Situ O2Generation for Biocatalytic Oxyfunctionalization Reactions. ChemCatChem 10 (23), 5366–5371. doi:10.1002/cctc.201801262
Hoschek, A., Toepel, J., Hochkeppel, A., Karande, R., Bühler, B., and Schmid, A. (2019b). Light‐Dependent and Aeration‐Independent Gram‐Scale Hydroxylation of Cyclohexane to Cyclohexanol by CYP450 Harboring Synechocystis Sp. PCC 6803. Biotechnol. J. 14 (8), 1800724. doi:10.1002/biot.201800724
Huang, H.-H., Camsund, D., Lindblad, P., and Heidorn, T. (2010). Design and Characterization of Molecular Tools for a Synthetic Biology Approach towards Developing Cyanobacterial Biotechnology. Nucleic Acids Res. 38 (8), 2577–2593. doi:10.1093/nar/gkq164
Huang, H.-H., and Lindblad, P. (2013). Wide-dynamic-range Promoters Engineered for Cyanobacteria. J. Biol. Eng. 7 (1), 10. doi:10.1186/1754-1611-7-10
Imamura, S., and Asayama, M. (2009). Sigma Factors for Cyanobacterial Transcription. Gene Regul Syst. Bio 3, 65–87. doi:10.4137/grsb.s2090
Immethun, C. M., and Moon, T. S. (2018). “Synthetic Gene Regulation in Cyanobacteria,” in Synthetic Biology of Cyanobacteria. Editors W. Zhang, and X. Song ((Singapore: Springer), 317–355. doi:10.1007/978-981-13-0854-3_13
Jin, H., Wang, Y., Idoine, A., and Bhaya, D. (2018). Construction of a Shuttle Vector Using an Endogenous Plasmid from the Cyanobacterium Synechocystis Sp. PCC6803. Front. Microbiol. 9, 1662. doi:10.3389/fmicb.2018.01662
Jodlbauer, J., Rohr, T., Spadiut, O., Mihovilovic, M. D., and Rudroff, F. (2021). Biocatalysis in Green and Blue: Cyanobacteria. Trends Biotechnol. 39, 875–889. doi:10.1016/j.tibtech.2020.12.009
Johnson, C. H., and Golden, S. S. (1999). Circadian Programs in Cyanobacteria: Adaptiveness and Mechanism. Annu. Rev. Microbiol. 53, 389–409. doi:10.1146/annurev.micro.53.1.389
Kadisch, M., Willrodt, C., Hillen, M., Bühler, B., and Schmid, A. (2017). Maximizing the Stability of Metabolic Engineering-Derived Whole-Cell Biocatalysts. Biotechnol. J. 12 (8), 1600170. doi:10.1002/biot.201600170
Karande, R., Halan, B., Schmid, A., and Buehler, K. (2014). Segmented Flow Is Controlling Growth of Catalytic Biofilms in Continuous Multiphase Microreactors. Biotechnol. Bioeng. 111 (9), 1831–1840. doi:10.1002/bit.25256
Karande, R., Salamanca, D., Schmid, A., and Buehler, K. (2018). Biocatalytic Conversion of Cycloalkanes to Lactones Using an In-Vivo cascade in Pseudomonas Taiwanensis VLB120. Biotechnol. Bioeng. 115 (2), 312–320. doi:10.1002/bit.26469
Kauny, J., and Sétif, P. (2014). NADPH Fluorescence in the Cyanobacterium Synechocystis Sp. PCC 6803: a Versatile Probe for In Vivo Measurements of Rates, Yields and Pools. Biochim. Biophys. Acta (Bba) - Bioenerg. 1837 (6), 792–801. doi:10.1016/j.bbabio.2014.01.009
Kelly, C. L., Taylor, G. M., Hitchcock, A., Torres-Méndez, A., and Heap, J. T. (2018). A Rhamnose-Inducible System for Precise and Temporal Control of Gene Expression in Cyanobacteria. ACS Synth. Biol. 7 (4), 1056–1066. doi:10.1021/acssynbio.7b00435
Laemmli, U. K. (1970). Cleavage of Structural Proteins during the Assembly of the Head of Bacteriophage T4. Nature 227 (5259), 680–685. doi:10.1038/227680a0
Lassen, L. M., Nielsen, A. Z., Olsen, C. E., Bialek, W., Jensen, K., Møller, B. L., et al. (2014a). Anchoring a Plant Cytochrome P450 via PsaM to the Thylakoids in Synechococcus Sp. PCC 7002: Evidence for Light-Driven Biosynthesis. PLoS One 9 (7), e102184. doi:10.1371/journal.pone.0102184
Lassen, L. M., Nielsen, A. Z., Ziersen, B., Gnanasekaran, T., Møller, B. L., and Jensen, P. E. (2014b). Redirecting Photosynthetic Electron Flow into Light-Driven Synthesis of Alternative Products Including High-Value Bioactive Natural Compounds. ACS Synth. Biol. 3 (1), 1–12. doi:10.1021/sb400136f
Latifi, A., Ruiz, M., and Zhang, C.-C. (2009). Oxidative Stress in Cyanobacteria. FEMS Microbiol. Rev. 33 (2), 258–278. doi:10.1111/j.1574-6976.2008.00134.x
Law, H. E. M., Baldwin, C. V. F., Chen, B. H., and Woodley, J. M. (2006). Process Limitations in a Whole-Cell Catalysed Oxidation: Sensitivity Analysis. Chem. Eng. Sci. 61, 6646–6652. doi:10.1016/j.ces.2006.06.007
Liang, F., Englund, E., Lindberg, P., and Lindblad, P. (2018). Engineered Cyanobacteria with Enhanced Growth Show Increased Ethanol Production and Higher Biofuel to Biomass Ratio. Metab. Eng. 46, 51–59. doi:10.1016/j.ymben.2018.02.006
Ludwig, A., Heimbucher, T., Gregor, W., Czerny, T., and Schmetterer, G. (2008). Transformation and Gene Replacement in the Facultatively Chemoheterotrophic, Unicellular Cyanobacterium Synechocystis Sp. PCC6714 by Electroporation. Appl. Microbiol. Biotechnol. 78 (4), 729–735. doi:10.1007/s00253-008-1356-y
Nishiyama, Y., Allakhverdiev, S. I., and Murata, N. (2005). Inhibition of the Repair of Photosystem II by Oxidative Stress in Cyanobacteria. Photosynth Res. 84 (1-3), 1–7. doi:10.1007/s11120-004-6434-0
Oliver, J. W. K., Machado, I. M. P., Yoneda, H., and Atsumi, S. (2013). Cyanobacterial Conversion of Carbon Dioxide to 2,3-butanediol. Proc. Natl. Acad. Sci. USA 110 (4), 1249–1254. doi:10.1073/pnas.1213024110
Sambrook, J., and Russell, D. W. (2001). Molecular Cloning : A Laboratory Manual. Cold Spring Harbor. N.Y.: Cold Spring Harbor Laboratory Press.
Savakis, P., Tan, X., Du, W., Branco dos Santos, F., Lu, X., and Hellingwerf, K. J. (2015). Photosynthetic Production of Glycerol by a Recombinant Cyanobacterium. J. Biotechnol. 195, 46–51. doi:10.1016/j.jbiotec.2014.12.015
Schäfer, L., Bühler, K., Karande, R., and Bühler, B. (2020). Rational Engineering of a Multi‐Step Biocatalytic Cascade for the Conversion of Cyclohexane to Polycaprolactone Monomers in Pseudomonas Taiwanensis. Biotechnol. J. 15, 2000091. doi:10.1002/biot.202000091
Scherkus, C., Schmidt, S., Bornscheuer, U. T., Gröger, H., Kara, S., and Liese, A. (2017). Kinetic Insights into ϵ-caprolactone Synthesis: Improvement of an Enzymatic cascade Reaction. Biotechnol. Bioeng. 114 (6), 1215–1221. doi:10.1002/bit.26258
Schmid, A., Dordick, J. S., Hauer, B., Kiener, A., Wubbolts, M., and Witholt, B. (2001). Industrial Biocatalysis Today and Tomorrow. Nature 409 (6817), 258–268. doi:10.1038/35051736
Schmidt, S., and Bornscheuer, U. T. (2020). Baeyer-Villiger Monooxygenases: From Protein Engineering to Biocatalytic Applications. Enzymes 47, 231–281. doi:10.1016/bs.enz.2020.05.007
Schrewe, M., Julsing, M. K., Bühler, B., and Schmid, A. (2013). Whole-cell Biocatalysis for Selective and Productive C-O Functional Group Introduction and Modification. Chem. Soc. Rev. 42 (15), 6346–6377. doi:10.1039/c3cs60011d
Sebesta, J., and Peebles, C. A. (2020). Improving Heterologous Protein Expression in Synechocystis Sp. PCC 6803 for Alpha-Bisabolene Production. Metab. Eng. Commun. 10, e00117. doi:10.1016/j.mec.2019.e00117
Shcolnick, S., Shaked, Y., and Keren, N. (2007). A Role for mrgA, a DPS Family Protein, in the Internal Transport of Fe in the Cyanobacterium Synechocystis Sp. PCC6803. Biochim. Biophys. Acta (Bba) - Bioenerg. 1767 (6), 814–819. doi:10.1016/j.bbabio.2006.11.015
Sikkema, J., de Bont, J. A., and Poolman, B. (1995). Mechanisms of Membrane Toxicity of Hydrocarbons. Microbiol. Rev. 59 (2), 201–222. doi:10.1128/mr.59.2.201-222.1995
Stanier, R. Y., Kunisawa, R., Mandel, M., and Cohen-Bazire, G. (1971). Purification and Properties of Unicellular Blue-green Algae (Order Chroococcales). Bacteriol. Rev. 35 (2), 171–205. doi:10.1128/br.35.2.171-205.1971
Thiel, K., Mulaku, E., Dandapani, H., Nagy, C., Aro, E.-M., and Kallio, P. (2018). Translation Efficiency of Heterologous Proteins Is Significantly Affected by the Genetic Context of RBS Sequences in Engineered Cyanobacterium Synechocystis Sp. PCC 6803. Microb. Cell Fact 17 (1), 34. doi:10.1186/s12934-018-0882-2
Thiel, K., Patrikainen, P., Nagy, C., Fitzpatrick, D., Pope, N., Aro, E.-M., et al. (2019). Redirecting Photosynthetic Electron Flux in the Cyanobacterium Synechocystis Sp. PCC 6803 by the Deletion of Flavodiiron Protein Flv3. Microb. Cell Fact 18 (1), 189. doi:10.1186/s12934-019-1238-2
Till, P., Toepel, J., Bühler, B., Mach, R. L., and Mach-Aigner, A. R. (2020). Regulatory Systems for Gene Expression Control in Cyanobacteria. Appl. Microbiol. Biotechnol. 104 (5), 1977–1991. doi:10.1007/s00253-019-10344-w
Vajravel, S., Sirin, S., Kosourov, S., and Allahverdiyeva, Y. (2020). Towards Sustainable Ethylene Production with Cyanobacterial Artificial Biofilms. Green. Chem. 22 (19), 6404–6414. doi:10.1039/D0GC01830A
Volmer, J., Neumann, C., Bühler, B., and Schmid, A. (2014). Engineering of Pseudomonas Taiwanensis VLB120 for Constitutive Solvent Tolerance and Increased Specific Styrene Epoxidation Activity. Appl. Environ. Microbiol. 80 (20), 6539–6548. doi:10.1128/AEM.01940-14
Wang, B., Eckert, C., Maness, P.-C., and Yu, J. (2018). A Genetic Toolbox for Modulating the Expression of Heterologous Genes in the Cyanobacterium Synechocystis Sp. PCC 6803. ACS Synth. Biol. 7 (1), 276–286. doi:10.1021/acssynbio.7b00297
Wlodarczyk, A., Gnanasekaran, T., Nielsen, A. Z., Zulu, N. N., Mellor, S. B., Thøfner, J. F. B., et al. (2016). Metabolic Engineering of Light-Driven Cytochrome P450 Dependent Pathways into Synechocystis Sp. PCC 6803. Metab. Eng. 33, 1–11. doi:10.1016/j.ymben.2015.10.009
Woo, J.-M., Jeon, E.-Y., Seo, E.-J., Seo, J.-H., Lee, D.-Y., Yeon, Y. J., et al. (2018). Improving Catalytic Activity of the Baeyer-Villiger Monooxygenase-Based Escherichia coli Biocatalysts for the Overproduction of (Z)-11-(heptanoyloxy)undec-9-enoic Acid from Ricinoleic Acid. Sci. Rep. 8 (1), 10280. doi:10.1038/s41598-018-28575-8
Zavřel, T., Očenášová, P., and Červený, J. (2017). Phenotypic Characterization of Synechocystis Sp. PCC 6803 Substrains Reveals Differences in Sensitivity to Abiotic Stress. PLoS One 12 (12), e0189130. doi:10.1371/journal.pone.0189130
Zhou, J., Zhang, F., Meng, H., Zhang, Y., and Li, Y. (2016). Introducing Extra NADPH Consumption Ability Significantly Increases the Photosynthetic Efficiency and Biomass Production of Cyanobacteria. Metab. Eng. 38, 217–227. doi:10.1016/j.ymben.2016.08.002
Keywords: whole-cell biocatalysis, photo-biotechnology, heterologous expression, scale-up, cyanobacteria, Baeyer–Villiger monooxygenase
Citation: Tüllinghoff A, Uhl MB, Nintzel FEH, Schmid A, Bühler B and Toepel J (2022) Maximizing Photosynthesis-Driven Baeyer–Villiger Oxidation Efficiency in Recombinant Synechocystis sp. PCC6803. Front. Catal. 1:780474. doi: 10.3389/fctls.2021.780474
Received: 21 September 2021; Accepted: 14 December 2021;
Published: 21 January 2022.
Edited by:
Florian Rudroff, Vienna University of Technology, AustriaReviewed by:
Shuke Wu, Huazhong Agricultural University, ChinaOliver Spadiut, Vienna University of Technology, Austria
Copyright © 2022 Tüllinghoff, Uhl, Nintzel, Schmid, Bühler and Toepel. This is an open-access article distributed under the terms of the Creative Commons Attribution License (CC BY). The use, distribution or reproduction in other forums is permitted, provided the original author(s) and the copyright owner(s) are credited and that the original publication in this journal is cited, in accordance with accepted academic practice. No use, distribution or reproduction is permitted which does not comply with these terms.
*Correspondence: Bruno Bühler, bruno.bühler@ufz.de; Jörg Toepel, joerg.toepel@ufz.de