- The Fire Centre, University of Tasmania, Hobart, TAS, Australia
Introduction: Pencil pine (Athrotaxis cupressoides) is an iconic, paleoendemic tree restricted to historic fire refugia in Tasmania’s western mountains. Anthropogenic climate change is increasingly exposing these areas to wildfire. Given that pencil pines have little capacity to recover from fire, and show scarce natural recruitment across their core range, they will be lost from many areas without interventions to restore population viability to burnt stands.
Methods: We conducted a large-scale field study targeting pencil pine stands burnt in recent (2016) and historic (1960) fires. Using small (0.5 m2) experimental plots distributed across a range of topography and vegetation, we trialled three interventions: i) protecting groups of naturally germinated seedlings from herbivores in situ (35 plots); ii) introducing seeds via multiple sowing methods (300 plots); and iii) transplanting tube stock propagated from seed or cutting material, with and without herbivore protection (1007 plots).
Results: We found that protecting natural germinants from herbivores did not prevent seedling mortality over 2.5 years, and sowing interventions largely failed. Most transplants exposed to herbivores failed to establish after 1.5 years, but establishment rates were high with herbivore exclusion, indicating strong predation by native macropod herbivores. Transplant establishment also varied with fine-scale topography, with the best outcomes in well-drained and Sphagnum dominated positions, and the worst outcomes in poorly-drained positions, suggesting young pencil pines are sensitive to waterlogging. Transplant establishment rates varied little between recently and historically burnt sites, and were insensitive to how plants were propagated.
Discussion: In summary, transplanting tube stock with herbivore protection is a promising method for restoring burnt pencil pine stands, and establishment rates can be improved by selecting favourable planting positions at fine scales. Our findings suggest pencil pine stands burnt decades previously are suitable for restoration. Managers seeking to conserve pencil pines may begin restoring both historically and recently burnt stands, alongside protecting unburned stands from fire. Interventions should be refined through adaptive management, including re-surveys of this long-term trial.
1 Introduction
Anthropogenic climate change is affecting species and ecosystems across the globe, including within the Earth’s most remote areas (Scheffers et al., 2016; Pecl et al., 2017). For many species and ecosystems, the establishment of protected areas alone is insufficient to mitigate human impacts (Hannah, 2008; Heywood, 2019; Gaitán-Espitia and Hobday, 2021). As a result, managers are increasingly challenged to consider more active interventions, both to restore ecosystems and prevent species declines in the face of ongoing environmental change (Bowman et al., 2017; Prober et al., 2019; Volis, 2019).
There is a range of options to mitigate human impacts on plant species. Sometimes plant populations, or whole ecosystems, can be restored by stimulating natural recruitment; for example by introducing disturbances (Klaus et al., 2018), manipulating hydrology (De Steven et al., 2006), changing fire regimes (Pyke et al., 2010) or reducing herbivore impacts (Ibanez and Hart, 2020). For example, vertebrate herbivory – a major ecological disturbance – can be reduced by controlling animal numbers (Beltran et al., 2014; Ibanez and Hart, 2020), or installing physical barriers (Nilar et al., 2019; Lorite et al., 2021). Such approaches are often inexpensive, and require no new genetic material to be introduced, hence minimising the legacy of human influence on the restored system (Holl and Aide, 2011). However, they are only possible where a natural seedbank or other regeneration source exists and may be slower than more active interventions (Zahawi et al., 2014).
Other interventions introduce propagules or plants (Dalrymple et al., 2012; Palma and Laurance, 2015; Grossnickle and Ivetić, 2017). Such introductions can augment wild plant populations (Jusaitis and Sorensen, 2007; Li et al., 2012), re-establish lost populations within a species historic range (Godefroid et al., 2011; Cogoni et al., 2013), or create new populations (Menges et al., 2016). Directly sowing seed into the field is a particularly convenient method – if an ex situ seed bank is available – because it can be applied quickly and cheaply. For example, aerial sowing was rapidly deployed in the Victorian Alps after repeat fires destroyed the natural seedbank of Eucalyptus delegatensis forests (Bassett et al., 2015). However, transplanting often has higher success rates, because transplant survivorship exceeds that of new germinants in the field (Palma and Laurance, 2015). Typically transplants are grown in a nursery from seed, though propagating from cuttings is also possible (Ray and Brown, 1995; Ashmore et al., 2011; Douterlungne et al., 2015). The source of germplasm can influence the effectiveness of transplanting and sowing, due to genetic variation across a species range. A ‘local is best’ ethos has traditionally prevailed (Mortlock, 2000; Broadhurst et al., 2008), but it is increasingly recognised that plantings may be more resilient to change if they leverage adaptive variation existing across a species’ range (Wang et al., 2010; Breed et al., 2013; Prober et al., 2015). Further, it has been suggested that restoration plantings should embed trials to assess the effects of provenance on short and long term success (Broadhurst et al., 2017; Breed et al., 2018).
Where interventions are situated can greatly influence their effectiveness in heterogenous environments. Optimal site choice depends on the ecophysiology of the target species. For example, introductions of shade-intolerant species may be more successful following disturbance (Ashton et al., 1997; Cox et al., 2004), while intact vegetation may advantage species requiring shading (Miandrimanana et al., 2019). Topography can also be important. For example, wetter or drier positions may be preferable depending on a species’ tolerance to drought and to waterlogging (Engelbrecht and Kursar, 2003; Raulings et al., 2007), and frost intolerant plants may fail in cold air drainage sinks (Marquis et al., 2021). Site choice can be aided by understanding the ecophysiology of the target species, and by empirical tests. However, the spatial scale of trials must be appropriate. For example, field experiments only considering environmental variables at large plot scales may miss important microsite effects (Douterlungne et al., 2015).
In summary, determining effective interventions to conserve imperilled plant species can be aided by understanding the reproductive biology, population genetics and disturbance ecology of the target species, and by undertaking fine-scale field surveys and population monitoring (Godefroid et al., 2011). These insights allow intervention approaches to be developed that adequately consider population genetics, the need for, or protection from disturbance, and the ecophysiological requirements of the target species.
One imperilled plant species is the slow growing Tasmanian paleoendemic (i.e. living fossil) conifer, the pencil pine (Athrotaxis cupressoides). This species is restricted to cold, wet areas in Tasmania’s western mountains, which have acted as historic fire refugia (Ogden, 1978), and most of its range is reserved within the UNESCO listed Tasmanian Wilderness World Heritage Area (TWWHA). Pencil pine stands are an iconic feature of the TWWHA, and differ markedly from surrounding forest types more typical of the Australian vegetation (Bowman et al., 2021). Pencil pines rarely exceed 20 m in height at maturity and are mostly much smaller. Dendrochronological studies show growth rates are very slow in natural settings (Dunwiddie and LaMarche, 1980), and that pencil pines are often very old despite their small size (Cullen and Kirkpatrick, 1988a). Indeed, adult pencil pines may reach ages of over 1000 years, and trees just 1 m tall have been reported to have an average age of 55 years old at a site in Tasmania’s south (Ogden, 1978). The species itself is considered a Gondwanan relict (Harris et al., 2018) because Athrotaxis is a ~145 million year old lineage that predates the breakup of the Gondwanan supercontinent (Jordan et al., 2016). Today, pencil pine stands are a hallmark example of a system vulnerable to collapse due to climate change (Harris et al., 2018; Bergstrom et al., 2021), due to both its direct effects (Mariani et al., 2019), and the associated increase in severe fire weather and dry lightning ignitions, which are increasingly exposing them to burning (Styger et al., 2018; Bowman et al., 2021).
Pencil pines are easily killed by fire (Prior et al., 2018), lack post-fire recruitment mechanisms (Bliss et al., 2021), and can be driven locally extinct from a single severe fire event (Bowman et al., 2019). Sexual reproduction is restricted to mast seeding events, whereby an abundance of large, wind-dispersed seeds are produced every few years (Cullen and Kirkpatrick, 1988a). These seeds are not fire-resistant and rarely travel long distances – germinants are mostly found within a few metres of adult trees (Cullen and Kirkpatrick, 1988a; Prior and Bowman, 2020). The species also reproduces by root suckering – particularly in wet areas, where dense clonal stands may occur (Cullen and Kirkpatrick, 1988a; Worth et al., 2016). However pencil pines don’t basally re-sprout after burning, and very rarely resprout from shoots (Bowman et al., 2019; Bliss et al., 2021).
Natural regeneration of killed stands has historically occurred through gradual re-colonisation from neighbouring areas, although the species’ short dispersal range and slow growth rates mean this process is very slow (Cullen and Kirkpatrick, 1988a; Fletcher et al., 2018; Mariani et al., 2019). Burnt stands are extremely unlikely to recover naturally in the modern climate (Fletcher et al., 2018), particularly given there has been widespread recruitment failure in recent decades in the Central Plateau – the core of the pencil pine’s range (Cullen and Kirkpatrick, 1988a; Holz et al., 2015; Bliss et al., 2021). The causes of this are not well understood (Bowman et al., 2019). One hypothesis is that vertebrate herbivores are responsible; an idea supported by the known palatability of young seedlings and adult foliage to browsers (Cullen and Kirkpatrick, 1988a; Holz et al., 2015). However, there is also evidence of other contributing factors. For example, a recent study reported pencil pine stands on small lake islands had fewer seedlings than adjacent mainland stands despite much lower herbivore presence on the islands; a finding that suggests bottom-up constraints may be also be an important filter on seedling recruitment (Bowman et al., 2019).
Pencil pines are listed as Vulnerable by the IUCN (The IUCN Red List of Threatened Species, 2021). They are a prominent aesthetic feature of the TWWHA, an iconic feature of Tasmanian flora, and a globally significant paleoendemic species; the threats to which have been highlighted in national and international media (Marris, 2016; Morton, 2016; Wahlquist, 2020). Accordingly, pencil pines are a conservation concern, and managers of the TWWHA have identified the need to develop techniques to restore burnt stands. We report on the first field trial of such interventions. The trial was conducted in an area where pencil pines were killed by two major fire events in 1960 and 2016, which occurred during periods of extreme drought. We focus on the establishment and first re-survey of this trial, but note that it is intended as a long-term experiment to be repeatedly surveyed.
Our overarching goal is to identify best-practice methods for restoring burnt pencil pine stands. We compared the effectiveness of three interventions: i) protecting naturally occurring germinants from herbivores; ii) direct sowing, and iii) transplanting. For transplanting interventions we also assessed: i) whether establishment is sensitive to the tube-stock transplanted (i.e., the propagation method, provenance and age); ii) whether herbivore protection was necessary; iii) whether establishment varied between planting positions at fine scales; and iv) whether establishment differed between stands burnt recently and stands burnt by historic fires.
2 Materials and methods
2.1 Study area
Trials were established at five sites (1150–1210 m.a.s.l.) within 2 km of Lake Mackenzie: a hydroelectric dam near the northwest tip of Tasmania’s Central Plateau (Figure 1B). Annual mean precipitation is 1985 mm; spread throughout the year (means of 316 mm in summer and 703 mm in winter) (data from Hydro Tasmania). Mean annual temperature is 6.9°C, ranging from a mean monthly minimum of −1.6°C in July to a mean monthly maximum of 19.1°C in January (Bureau of Meteorology, 2022). Frost and snowfalls may occur at any time of year. The terrain around Lake Mackenzie is gently undulating, with low peaks flanked by boulder fields, and shallow valleys containing wetlands and natural lakes. Bare rock is common; mostly Jurassic dolerite from a large sill exposed through Pleistocene glacial erosion (Banks, 1972). Alpine humus soils occur in well-drained positions, often as shallow pockets amongst rocks. Shallow organic soils dominate gentle slopes and drainage lines, with deep peats occurring in bogs. The organic substrates often form distinctive microtopographic features, including hummock-hollow terrain and tiered pool complexes (i.e., string bogs) (Whinam and Kirkpatrick, 1994). The vegetation is a fine-grained mosaic of shrubland, woodland, wetlands, grass and sedgelands, and remnant Gondwanan vegetation including pencil pine stands (Jackson, 1972), which are concentrated in fire refugia; areas that are very rocky or wet, or have sheltering topography (Bowman et al., 2019; Mariani et al., 2019).
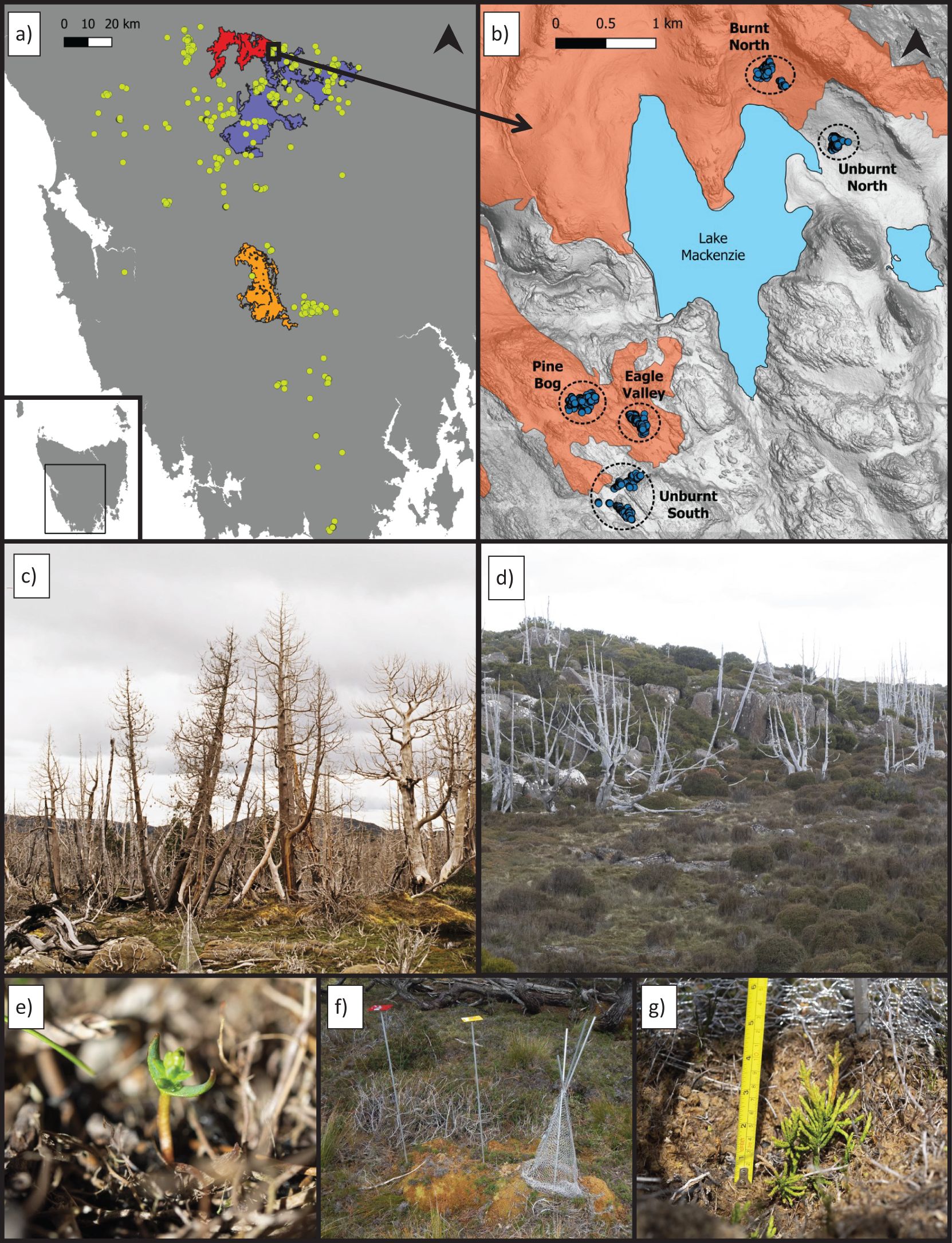
Figure 1. Geographic context of the trial, which explored restoration techniques for pencil pine stands damaged by fire in 2016 and 1960. (A) Map of Western Tasmania showing the global range of pencil pines (bright yellow points are observations from the Natural Values Atlas), footprints of fires which have burnt pencil pines since 1960 (purple=1960, red=2016, mustard=2018), and the location of the study area (black box towards top of panel). (B) Map of the study area showing the 2016 fire footprint (red) and locations of the plots (blue dots) within the five study sites. (C) Pencil pines at the Pine Bog site which were killed in the 2016 fires. (D) Pencil pines at the Unburnt South site which were killed in the 1960s fires (unburnt in 2016). (E) A naturally germinated pencil pine seedling found at one of the study sites. Tree guards were used to protect groups of natural germinants from herbivory in situ. (F) Pencil pine seeds were sown directly into the field, both with herbivore protection (plot to the right, with tree guard) and without (plot to the left). (G) At each site, juvenile pencil pines which had been propagated in a nursery were transplanted into the field (the individuals pictured are within a tree guard, protecting them from vertebrate herbivores).
2.1.1 Fire history
Burning is hampered in the study area by regular rainfall, slow fuel accumulation, and an abundance of water bodies and exposed rock. Nonetheless, palaeoecological records indicate regular fires over the last several hundred years, which were geographically limited and of low severity (Dodson, 2001; Holz et al., 2015). This likely reflects intentional burning to stimulate ‘green pick’ for herbivores, including Aboriginal use of patchy fires to aid macropod hunting (Holz et al., 2015), and later burning by European trappers and graziers (Cubit, 1996).
In the unusually dry summer of 1960–61, intentionally lit fires affected 60% of the Central Plateau, including part of the study area (Johnson and Marsden-Smedley, 2002). This single fire season is estimated to have reduced the global range of pencil pines by 10%, with negligible natural recovery (Holz et al., 2015).
After several fire-free decades, the study area burnt again in January 2016, when dry lightning ignitions followed an extremely dry winter and spring. The resulting fires destroyed 141 ha of pencil pine stands (Bowman et al., 2021), highlighting the threat that anthropogenic climate change – and associated drying trends and increased lighting ignitions – pose to these Gondwanan relicts (Harris et al., 2018; Styger et al., 2018). This threat was further highlighted by fires in 2018 that affected pencil pines in the south of the TWWHA (Figure 1A) (Bowman et al., 2022).
2.2 Study design
Interventions were trialled across five sites, in which numerous plots (262 to 290 per site) (Supplementary Table 1) were established (Figure 1B). Three sites were burnt by the 2016 fires (‘recently burnt’ hereafter) (Figure 1C), and two were outside the 2016 fire boundary but contained dead pencil pines from the 1960/61 fires (‘historically burnt’ hereafter) (Figure 1D). Natural germinant and sowing plots were established in March 2019, and transplant plots were established in March and April 2020. All plots were resurveyed in November–December 2021, concluding a 32 month trial of the natural germinant and sowing interventions, and a 20 month trial of the transplant interventions (Figure 2).
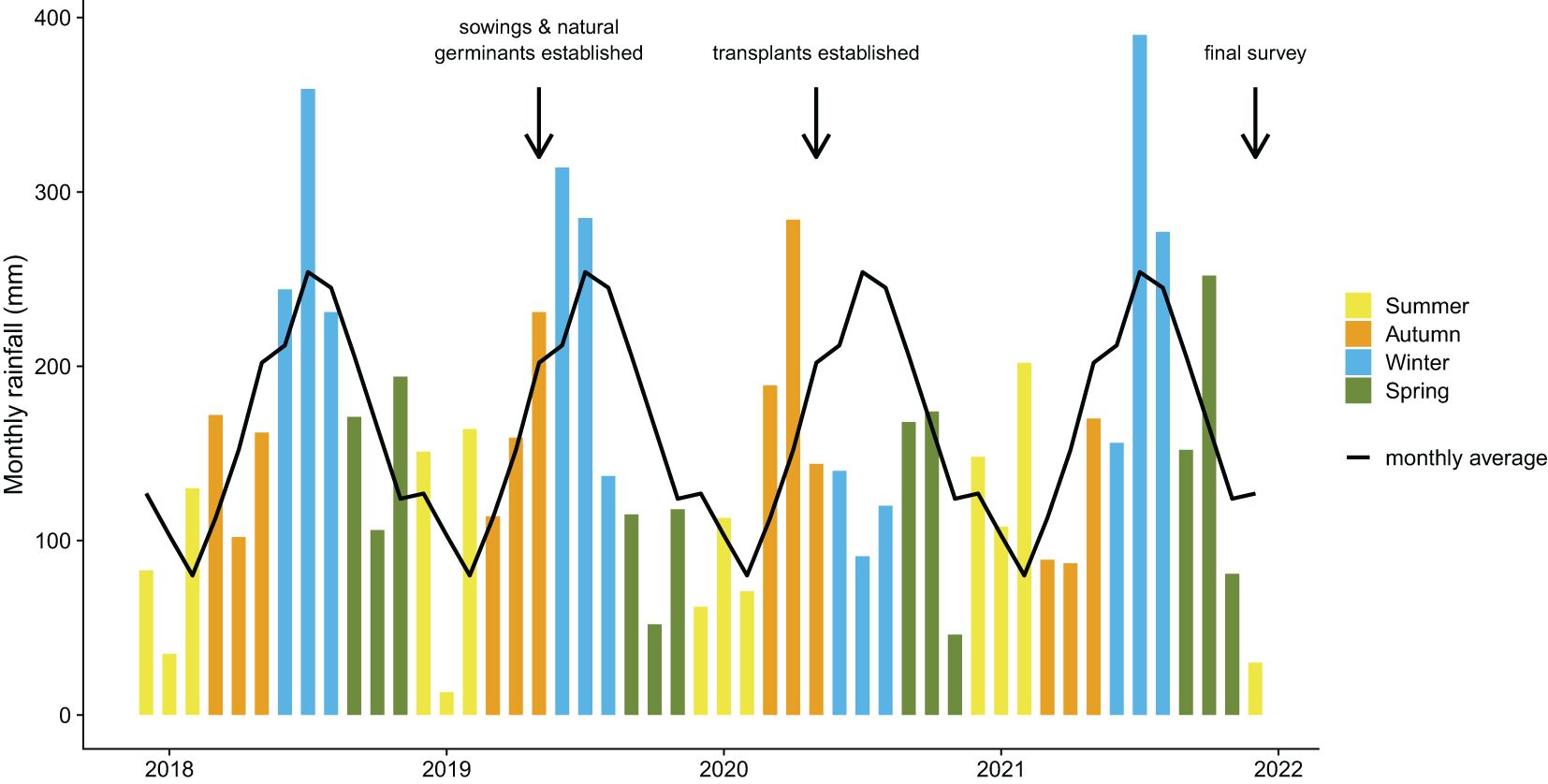
Figure 2. Timeline showing precipitation from one year before trial establishment (Dec 2017) until the final re-survey (Dec 2021). Coloured bars are monthly precipitation totals from the Lake Mackenzie gauge and the black line is the long-term monthly average (Data provided by Hydro Tasmania). Sowings occurred in March 2019, as did natural germinant interventions. Transplanting occurred in March and April 2020.
2.2.1 Protecting natural germinants from herbivores
Sites were searched for naturally germinated pencil pine seedlings (Figure 1E), and 35 plots (3–12 per site) were established to assess the practicality and effectiveness of protecting natural pencil pine germinants from herbivores. Natural germinants were only found near live trees; sometimes in groups of one to three, and often in dense clusters (i.e., > 100 per 1 m2). Plots were situated where germinants could be protected in situ by constructing a perimeter (≤1 m2) impervious to vertebrate herbivores using stainless steel mesh and aluminium posts. Such protection was installed for 18 plots (selected by coin toss) while 17 were left open (marked using posts without mesh). At both plot establishment and resurvey, germinants in each plot were counted. Where they were small (<2 cm) and numerous (>50), counts were to the nearest five.
2.2.2 Direct sowing
Direct sowing interventions were established across 300 plots (60 per site). Plot positions spanned the range of environmental variability at each site, provided that: i) a pencil pine stem (live or dead, including fallen) existed within 5 m; and ii) driving tree guard posts into the ground was feasible. Seeds were provided by the Royal Tasmanian Botanical Gardens (RTBG) seed bank, who collected them from across the species’ natural range during a 2015 mast seeding event. Each plot received fifteen viable seeds (17–20 total, depending on RTBG collection viability estimates). Plots were evenly split among three sowing methods: i) sowing onto the undisturbed ground surface; ii) sowing onto a disturbed area (~ 30 cm diameter) where vegetation was removed with a mattock; and iii) burying seeds in a 5 cm deep pit. Half the plots were protected from vertebrate herbivores (‘caged’) using tree guards (robust ‘tee-pees’ constructed from aluminium posts and stainless steel mesh, secured with mild steel U-pins), and half were left open (Figure 1F). Germinant presence (or absence) was recorded in the re-survey.
2.2.3 Transplanting
Transplanting interventions spanned 1007 plots (190 to 227 per site). Each plot received two tube stock; one grown from seed (seedling henceforth) and one from cutting material (cutting henceforth) (Figure 1G). Plot locations were selected (sampling site variability with the same restrictions as sowing plots) and prepared between February 3rd and March 23rd 2020, but planting was restricted to a later time window (between March 23rd and April 7th 2020) to minimise the variability in weather to which transplants were subjected. First a pair of plot positions with similar topography and vegetation were chosen, and a coin was flipped to determine which would receive herbivore protection. Over each position a quadrat (50 x 50 cm) was placed within which scat pellets were identified and counted, percentage cover of woody vegetation (as viewed from above) was recorded, and microtopography (0.5 m scale) was qualitatively described. Other environmental variables were also recorded which were later used to characterise the planting position (Supplementary Presentation 1). A mattock was then used to clear vegetation (~30 cm diameter area) and dig a rectangular hole 22 x 10 cm in footprint and 15 cm in depth.
At planting time, a cutting and seedling were randomly selected from tube stock trays preassembled with a mix of provenances. We measured the height of each individual plant before removing it from its tube. Seedlings were significantly shorter on average than cuttings (mean seedling height=123.3 mm, mean cutting height=131.6 mm, P(Kruskall-Wallis test)<0.001) but varied more in height (seedling height range 22–269 mm, cutting height range 36–220 mm). The seedling and cutting were planted in the same hole (20cm apart, bedded in with dislocated soil, mulched with cut vegetation, and hand watered if the soil was not already wet), and either protected using a tree guard (same design as for sowings, but triallling alternate materials for 88 of the 504 caged transplant plots: see Prior et al., 2023) or left open to herbivory (marked with a pair of aluminium posts).
At re-survey, on-ground observers classed each individual as: i) ‘dead’, if they were absent or had no live foliage; ii) ‘damaged’, if they had live foliage but were either broken-off near ground level or had ≥ 10% dead foliage, or; ‘healthy’, if they were intact with <10% dead foliage. Our field observations suggested transplants were healthy more often in well-drained areas and where Sphagnum predominated. To test this statistically a simple landscape position classification (i.e., at the 3 m scale) was developed and each plot assigned to one of four classes (Figure 3) (see Supplementary Presentation 1 for methodological details). We also tested whether microtopography (0.5 m scale) within the landscape position classes (3 m scale) was important. Each plot was assigned a microtopographic class; either i) ‘runoff’, where the original qualitative description of microtopography included the terms hummock, mound or slope; or ii) ‘runon’, where the description included the terms hollow, pan, depression or flat. The descriptions contained none of the above terms for twenty percent of the plots: these were excluded from the final analysis.
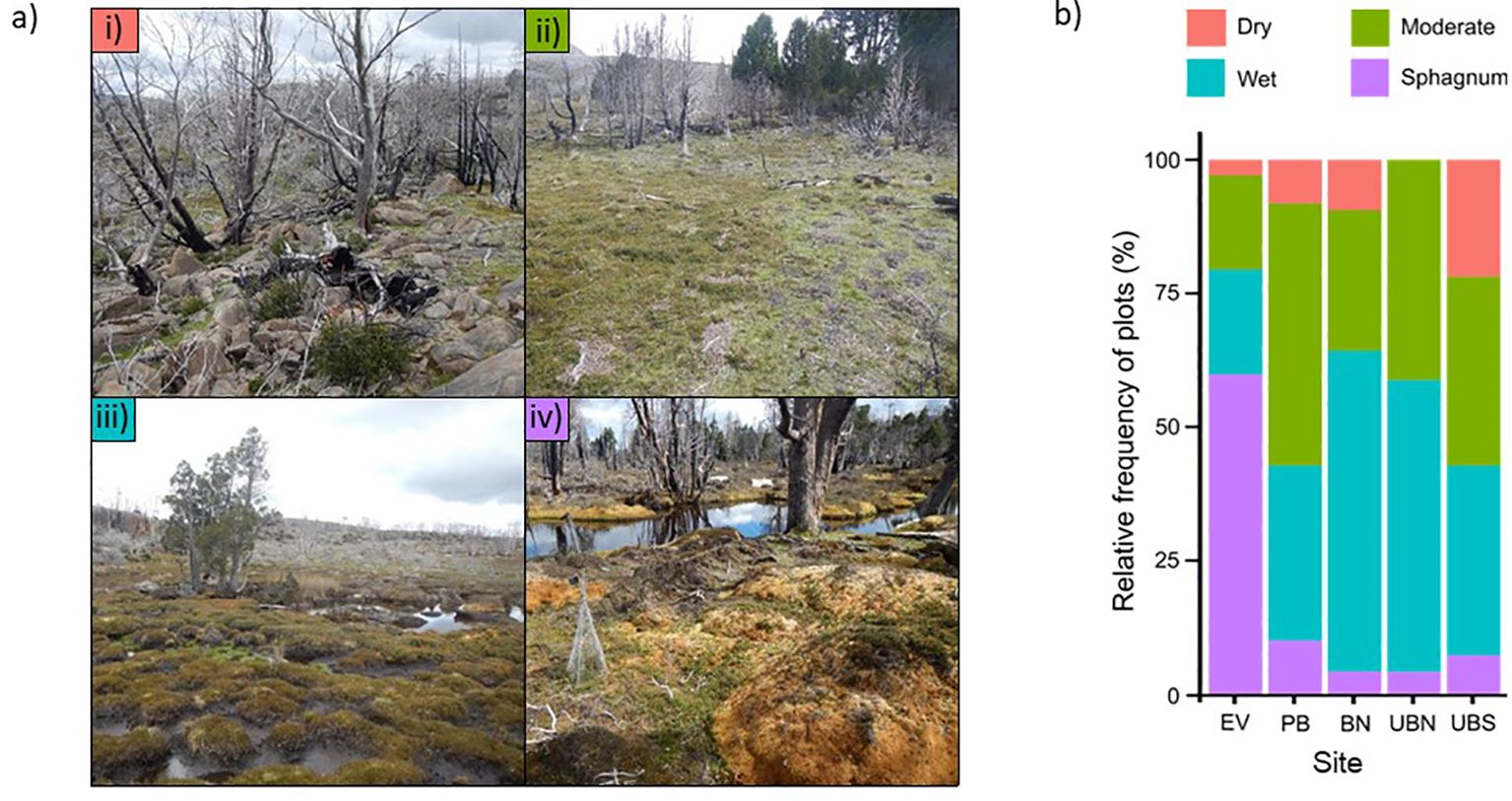
Figure 3. (A) Photographic examples of the four the landscape position classes to which plots were assigned (see Supplementary Presentation 1 for methodological details): (i) ‘dry’ positions are well drained, with ground cover ranging from grassy or shrubby vegetation to very rocky, and include raised areas, moderate to steep slopes and boulder fields; (ii) ‘moderate’ positions are of intermediate drainage, and include slopes, gently raised parts of flats and transition zones between dry and wet areas; (iii) ‘wet’ positions are poorly drained, are not dominated by Sphagnum (peat is typically the substrate), and include wet depressions, flats, gentle slopes, and other areas with standing water; (iv) in ‘Sphagnum’ positions live or dead Sphagnum moss dominates the ground surface or is the main substrate, and the cover of rock or bare soil is typically very low. (B) A stacked bar chart showing the proportion of plots in each landscape position class at the five study sites (EV, Eagle Valley; PB, Pine Bog; BN, Burnt North; UBN, Unburnt North; UBS, Unburnt South).
2.3 Data analyses
The three intervention types were assessed using different response variables and analysis pathways, which we describe in detail under the subheadings below. All analyses were undertaken using R statistical software (R Core Team, 2022).
2.3.1 Natural germinant interventions
Caged and uncaged natural germinant plots were compared by assessing change in seedling count (i.e., count at re-survey minus count at establishment). Data did not conform to any standard distribution, so caged and uncaged plots were compared using Kruskal-Wallis rank sum tests (a non-parametric comparison of means).
2.3.2 Direct sowing interventions
The direct sowing interventions were assessed using seedling presence-absence. The results were clear without formal statistical analysis.
2.3.3 Transplant interventions
Transplant establishment was assessed using two binary response variables – health and survival. These response variables were both constructed from the categorical re-survey data (dead, damaged and healthy), so they were related, but it was informative to include both (detailed below).
We assessed predictors of transplant health and survival using an information theoretic approach. We constructed generalised linear mixed effects models (lme4 package in R) with different combinations of predictors (site was always specified as a random effect to account for spatial and temporal clustering) and compared the models using Aikake information criterion (AIC) – which favours model fit and simplicity. We compared all possible models, and followed the approach of Richards (2008), whereby: i) only the lowest AIC (best) model and models with a difference in AIC (ΔAIC) of less than six were retained; and ii) models were rejected if there was a simpler nested model with a lower AIC. We used retained models to assess support for each predictor, whereby predictors have strong support if they are present in all retained models, moderate support if present in the lowest AIC model but absent from some others, weak support if absent from the lowest AIC model but present in some others, and no support if absent from all retained models. Lastly, the effect size of predictors in the lowest AIC (best) model was assessed by plotting their estimated coefficients and 95% confidence intervals.
2.3.3.1 Health
For this response variable, healthy transplants (i.e., intact with 90% living foliage) were considered successes, while damaged and dead transplants were considered failures. This highlighted the best outcome attainable in this short trial: living individuals showing no signs of likely failure. We assessed whether health was influenced by caging, fire history, propagation method, provenance, age, landscape position and microtopography. Data exploration revealed a striking tree guard effect (only 1.3% overall of open individuals were healthy) so we restricted analyses to caged individuals. To simplify the final analysis we excluded two predictors that had minimal effects: provenance and age (Supplementary Presentation 2, Supplementary Presentation 3 provide information on provenance and age effects respectively). Prior to constructing candidate models we tested for an interaction between landscape position and microtopography by comparing the AIC of the additive model and a model with the interaction term, but found no support.
2.3.3.2 Survival
For this response variable, all living transplants (both healthy and damaged) were considered successes and only dead individuals were considered failures. We included this variable to strengthen ecological insights from this study and aid comparison with other work. We used the same analysis pathway as for the health response variable, again focusing on caged individuals, excluding provenance and age as predictors, and testing for an interaction between microtopography and landscape position (for which there was no support).
3 Results
3.1 Protecting natural germinants from herbivores
Protecting naturally germinated seedlings from herbivores had no statistical effect on the change in seedling count through time (Supplementary Figure 1). Between establishment and re-survey, seedling counts decreased in both caged (from 29.8 to 19.5 on average) and open (from 24.9 to 15.9 on average) plots.
3.2 Direct sowing
Almost all sowings failed, regardless of sowing method or caging treatment (Supplementary Table 2). Of the 300 sowing plots, only 10 (3.3%) contained seedlings in the 2021 re-survey. Further, it seems likely many of these seedlings originated from naturally occurring seed rather than our sowings because: i) they were only found on plots close to live adult pencil pines; ii) they were few in number (average of 2 per plot); and iii) where they were present in sowing plots, seedlings of similar height were also noted in the surrounding environment.
3.3 Transplanting
3.3.1 Herbivore protection
Tree guards had a striking positive effect on transplants (Figure 4A). Of the 1006 individuals transplanted in open plots, just 90 were found alive (8.9%), and only 13 were healthy (1.3%). In contrast, of 1008 caged individuals, 747 were alive (74.1%) and 559 were healthy (55.5%). Among open individuals, more individuals survived in historically burnt sites, in which plots had higher woody plant cover (Figure 4B), and lower macropod scat counts (Figure 4C) at the time of establishment. Scat counts suggest macropods are by far the dominant herbivore. Across all plots combined, 8144 macropod scats were recorded compared to only 93 possum scats, which were the next most common. Only 24 wombat and 30 lagomorph scats were recorded.
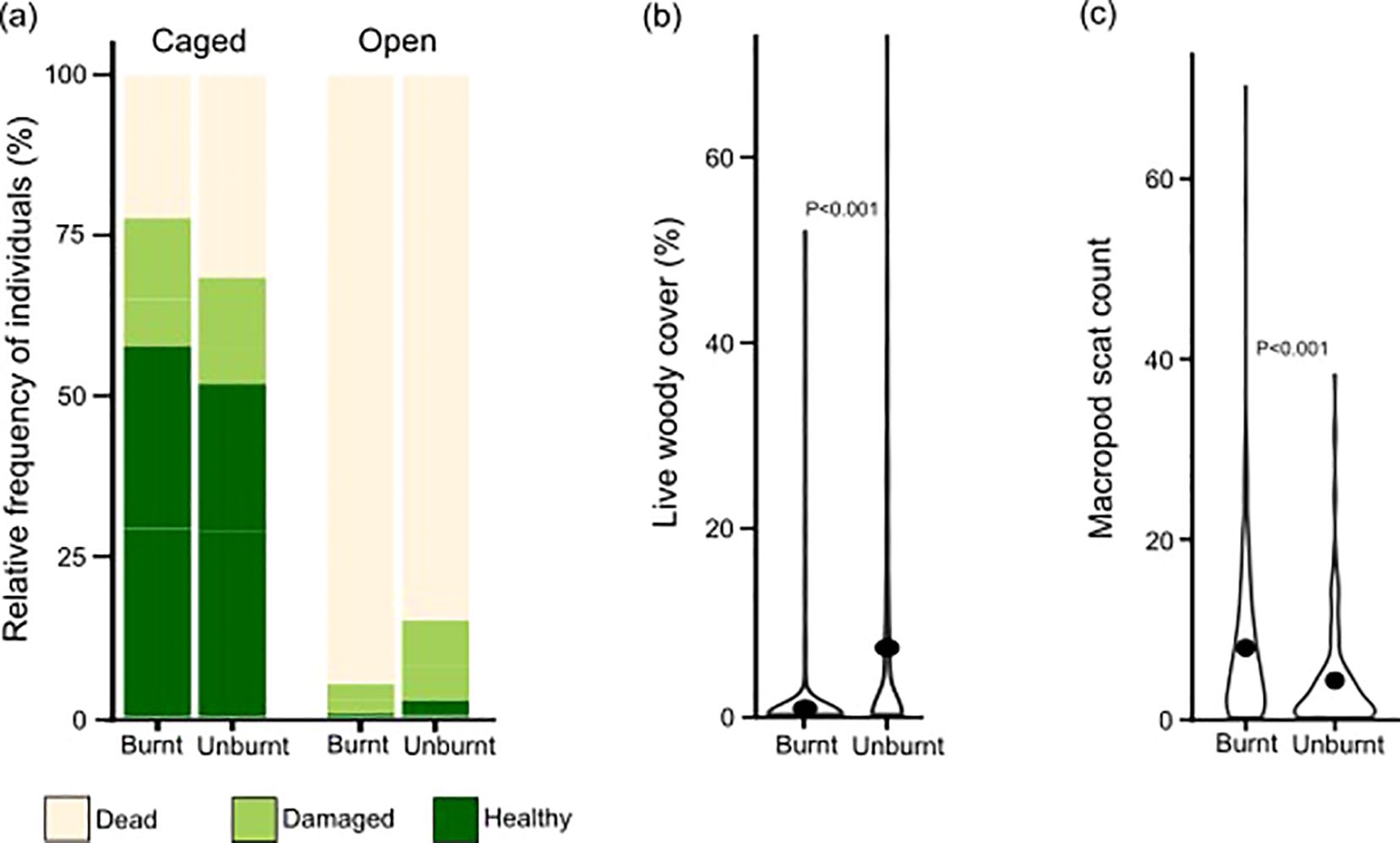
Figure 4. (A) Stacked bar chart showing how the status of individual transplants at the end of the trial in caged and open plots varied in relation to fire history. Transplants survived far more frequently in caged plots, and survival in open plots was more frequent in historically burnt areas (unburnt in 2016) than recently burnt areas (burnt in 2016). (B) Violin plot showing that historically burnt plots had higher woody vegetation cover. (C) Historically burnt plots had lower macropod scat counts, at the time of trial establishment.
3.3.2 Health and survival of caged transplants
3.3.2.1 Landscape position
Landscape position was strongly supported as a predictor of both survival and health (Supplementary Table 3). This was driven by a higher likelihood of survival and health in Sphagnum and dry plots than in moderate and wet plots, where individuals were more likely to be dead or damaged (Figure 5). Outcomes in dry and Sphagnum plots were similar for both response variables; in dry plots 85.5% of individuals survived and 77.4% were healthy, while in Sphagnum plots 90.5% of individuals survived and 76.4% were healthy. Survival was similar between wet (67.1% survival) and moderate (71.9% survival) plots, however individuals were more likely to be healthy in moderate plots, where 54.2% of individuals were healthy, than in wet plots, which had the lowest likelihood of health at 43.5% (Figure 6).
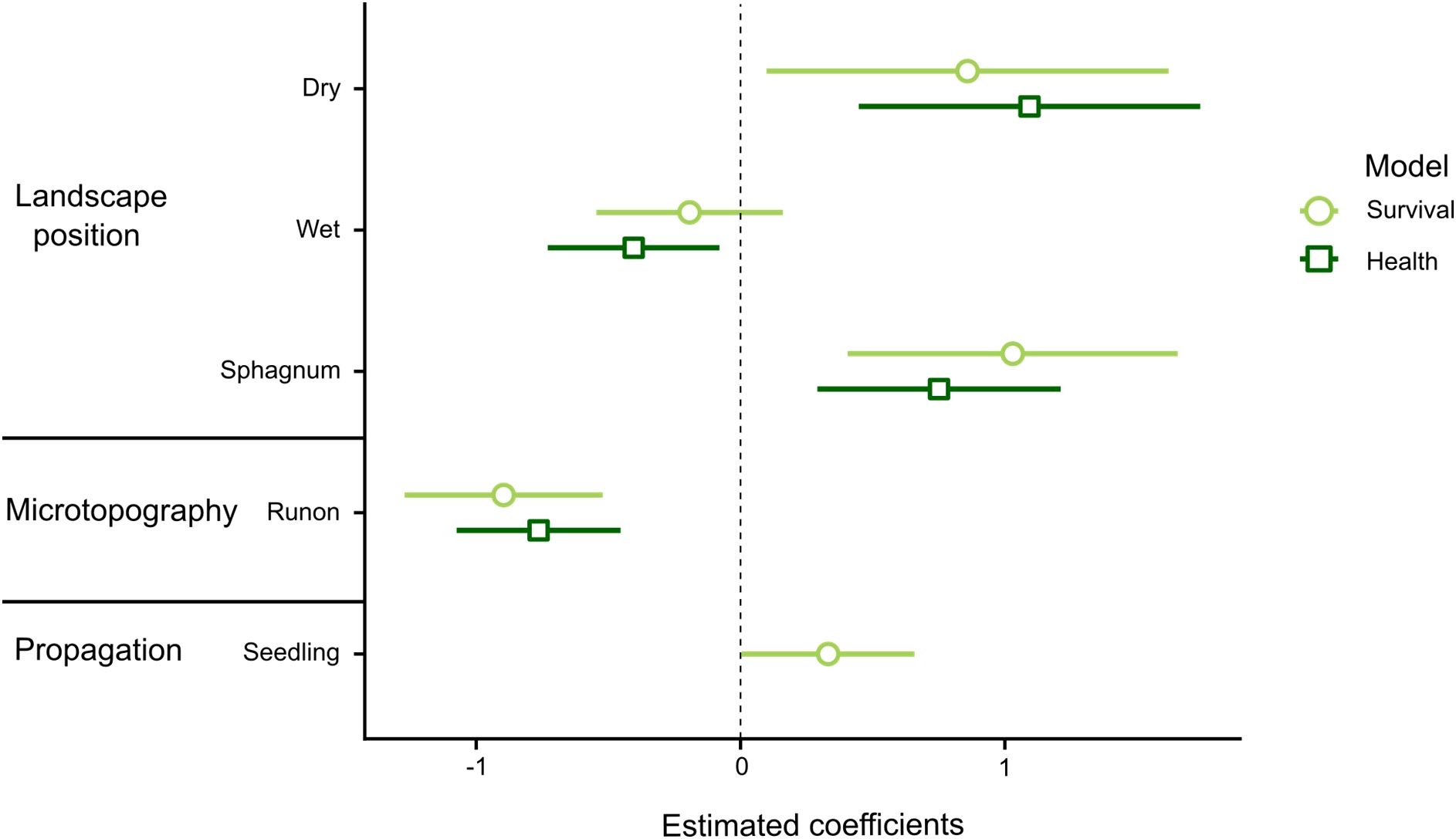
Figure 5. Effect size plot for supported predictors of survival and health of transplanted pencil pines. Coefficients and 95% CIs for predictors in the best (lowest AIC) models are shown. Effect size (difference from 0) is relative to the base model of moderate landscape position, runoff microtopography and cutting propagation, and where 95% CIs do not overlap zero a statistically supported effect can be inferred.
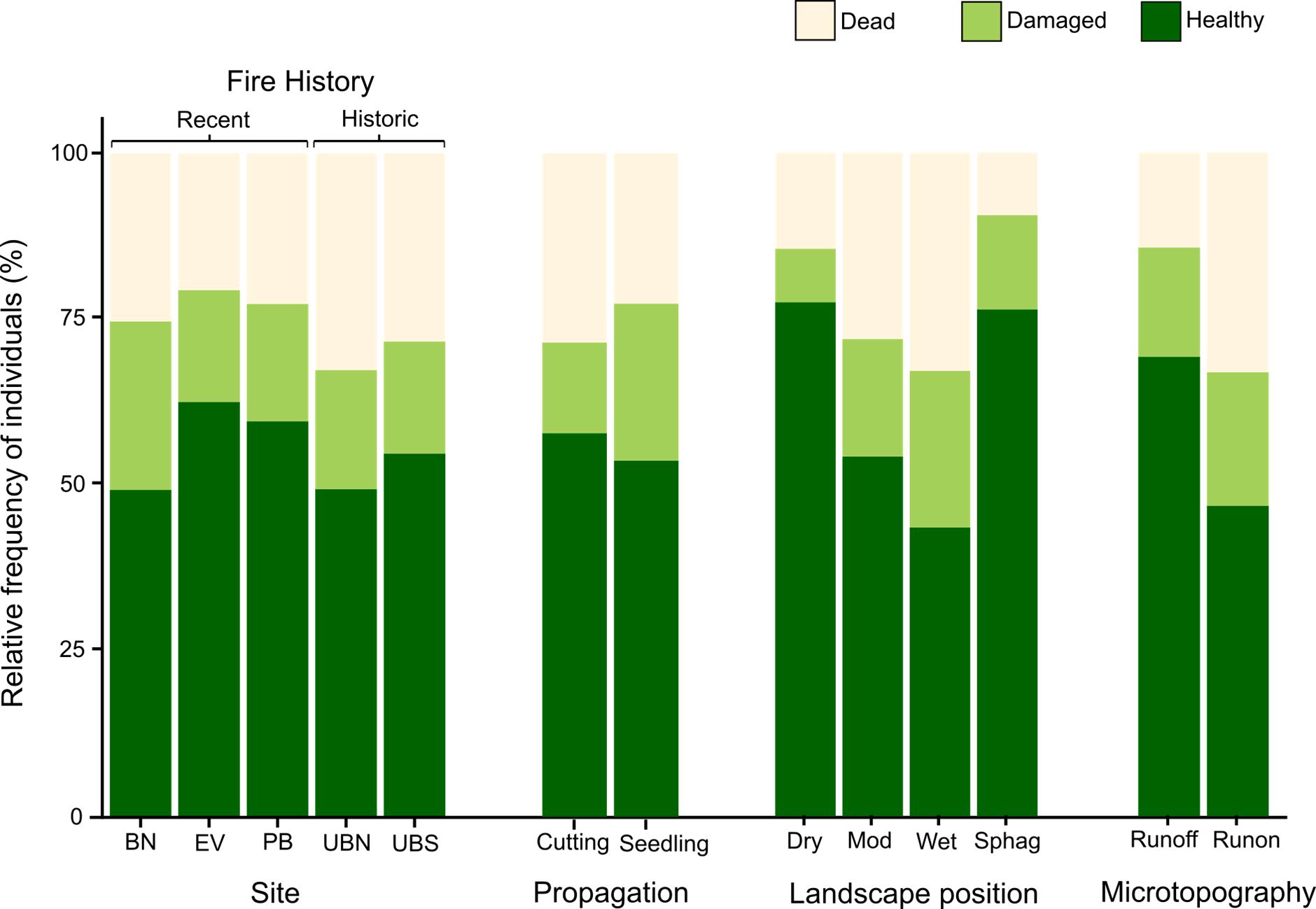
Figure 6. Stacked bar charts showing the relative frequency of transplanted individuals that were dead (absent or without live foliage), damaged (broken off and/or >10% dead foliage), and healthy (intact and >=90% live foliage) in the 2021 re-survey, with regard to predictor variables included in the AIC analyses.
3.3.2.2 Microtopography
There was strong support for microtopography as a predictor of transplant establishment (Supplementary Table 3), with both survival and health being higher in runoff than runon positions (Figure 6). This result reinforces drainage as a key influence on transplants. Surprisingly the positive effect of runoff microtopography held true in all landscape positions (no AIC support found for the macrotopography x landscape position interaction term). Hence, even in dry positions, there was some disadvantage in planting in poorly drained (runon) microtopography.
3.3.2.3 Propagation method
The most notable difference in results between the two establishment response variables was that propagation method was included in the best model of survival but received no support as a predictor of health (Supplementary Table 3). Seedlings were more likely to survive than cuttings, but they were also more likely to be damaged, with the net result being no difference in the overall likelihood of a cutting or a seedling being healthy.
3.3.2.4 Fire history
Fire history received no AIC support as a predictor of either health or survival in the AIC analyses (Supplementary Table 3).
4 Discussion
This study provides insights for conserving a paleoendemic tree species that is threatened by climate change and wildfire, within a globally significant protected area (Bergstrom et al., 2021; Bowman et al., 2021). Of the three interventions trialled for restoring fire-damaged pencil pine stands, transplanting was the most promising: direct sowing largely failed, and protecting natural germinants from herbivores did not prevent a decline in seedling numbers. Transplant establishment rates were drastically improved by herbivore protection, and also varied with fine-scale topography. However, establishment rates were similar between recently and historically burnt sites, and were little influenced by variables relating to the tube stock used (i.e., propagation method, provenance and age). In this section we begin by discussing the three interventions we trialled, then explore the transplanting results in detail. We conclude with practical recommendations for conserving pencil pines in situ.
4.1 Protecting natural germinants from herbivores
The decline of natural germinants in both caged and open plots suggests burnt pencil pine stands are unlikely to be restored using herbivore protection alone. Previous work has indicated high juvenile attrition rates in the Lake Mackenzie area (Bliss et al., 2021). However, it is surprising that caging didn’t mitigate this decline given that we detected strong herbivore effects on transplants at the same sites (discussed below). Further, a similar field experiment in the Central Plateau found natural germinant counts increased by 3% in caged quadrats while decreasing by 56% in adjacent open quadrats over 18 months (Cullen and Kirkpatrick, 1988a). While design differences between the two experiments may have contributed to these contrasting results, it seems unlikely that our simpler study would have failed to detect a herbivore effect of the magnitude reported in the earlier study. We conclude that natural germinant attrition during our trial was driven by factors other than vertebrate herbivory.
The effectiveness of this intervention type is limited by the fact natural germinants only occur near live adult pencil pines (Cullen and Kirkpatrick, 1988a; Bliss et al., 2021), where conditions may be suboptimal for their survival. Pencil pine seedlings are subject to several bottom-up filters. For example, field studies suggest pencil pines have limited shade tolerance (Cullen and Kirkpatrick, 1988a) – a likely constraint on the germinants we targeted given most were beneath live trees (i.e. were shaded). Additionally, laboratory experiments have shown young seedlings are susceptible to both drought and waterlogging (Sakai et al., 1981; Cullen and Kirkpatrick, 1988b). Though it is not possible to determine the causes of natural germinant attrition in our study, it is clear that protecting natural germinants from herbivores is not a reliable standalone approach for restoring burnt stands.
4.2 Direct sowing
We found no evidence that introducing seed – with or without soil disturbance or recent fire, and in a range of microsites – can effectively restore pencil pine stands. Both abiotic and biotic factors may cause direct sowing interventions to fail. Seed predation is a common impediment (Overdyck et al., 2013; Palma and Laurance, 2015; Linabury et al., 2019); for example removal of seeds by ants has been documented in the Tasmanian Midlands (Bailey et al., 2021b). Additonally, unsuitable weather may cause sowings to fail to germinate or persist (Hallett et al., 2014; Muñoz-Rojas et al., 2016). This may be particularly relevant to our sowings, because all were implemented within a relatively short temporal window. Though we sowed in March – within the timeframe of natural seed fall (Ogden, 1978) – unusually high precipitation in the following weeks (Figure 2) may have affected germination or washed seeds away from the plots. Further trials of direct sowing are warranted given the convenience and low cost of this intervention (relative to transplanting), and because a collection of pencil pine seed for conservation purposes already exists (RTBG). Such trials could account for weather by sowing at several time points (both within and between years) and investigate seed predation by applying insecticides to some sowings, as has been done in the Tasmanian Midlands (Bailey et al., 2021b).
The failure of our sowings is also notable because germination failure has not been considered a constraint on pencil pine recruitment. Pencil pine seeds have high viability rates and germinate under various temperature and light conditions in laboratory tests (Read, 1989). Frequent observations of pencil pine seedlings after mast seeding events suggest germination also readily occurs in the field (Cullen and Kirkpatrick, 1988a; Holz et al., 2015; Bowman et al., 2019). Several recruitment constraints relating to the spatial and temporal occurrence of pencil pine seed have been identified; specifically that it does not persist in the soil or the canopy, is episodically produced and disperses over only short distances (Cullen, 1987; Cullen and Kirkpatrick, 1988a). Thus some work indicates it is seed supply – rather than germination and early seedling survival – which limits sexual recruitment of pencil pine. However this inference was not supported by our study, in which direct sowing failed across a range of treatments, fire histories and topographic positions.
It has been speculated that low severity disturbance (e.g., animal digging) may promote pencil pine germination in natural settings (Bowman et al., 2019). However our study – which incorporated three levels of mechanical disturbance – provides no evidence to support this claim.
4.3 Transplanting
Our findings indicate transplanting is a promising intervention to restore pencil pines. This aligns with restoration trials using other species, which have also found transplanting to be an effective approach (Palma and Laurance, 2015).
For some species, transplanting effectiveness is strongly influenced by tube stock type; for example, the method of propagation (Ray and Brown, 1995; Zahawi and Holl, 2009), genetic provenance (Bailey et al., 2021a), and age at the time of planting (Siles et al., 2010). In our study these variables were unimportant compared to the use of herbivore protection and choice of planting location, suggesting the outcomes of pencil pine transplanting are not highly sensitive to the tube stock used. However, these results are based only on transplant establishment and should be re-visited following future re-surveys.
4.3.1 Herbivores
Our results demonstrate that herbivore protection is necessary for transplants to establish, regardless of their position in the landscape. Although we found increased herbivore pressure in recently burnt sites – indicated by elevated herbivore scat counts and unprotected transplant failure rates – both of these variables were also high in sites not burnt for decades. Thus, our findings suggest herbivores are effectively ubiquitous, and reinforce herbivory as a key top-down filter on juvenile pencil pines in the Central Plateau (Cullen and Kirkpatrick, 1988a).
Macropods were clearly the dominant herbivore in our study area, evidenced by their scats being more abundant than possum scats (the next most common) by almost two orders of magnitude. Following the extinction of the Thylacine (Thylacinus cynocephalus), population decline of the Tasmanian Devil (Sarcophilus harrisii) and cessation of hunting by Aboriginal people and later European trappers, there is little predation to control macropods in the Central Plateau (Holz et al., 2015). Our findings add to existing evidence that macropods constrain pencil pine recruitment and growth, including the dearth of adult foliage below wallaby (Macropus rufogriseus) browse height, and direct observations of macropods consuming pencil pine foliage (Cullen and Kirkpatrick, 1988a; Holz et al., 2015).
4.3.2 Fire history
The pencil pines we transplanted established equally well in both recently and historically burnt sites. For some species, the time between a fire and a restoration intervention is a crucial. For instance, restoring Eucalytpus delegatensis forest requires sowing seeds in the first winter after a fire, while a suitable ash bed still exists (Bassett et al., 2015). Conversely, other plants cannot establish immediately, and need pioneer species to create suitable conditions. Our findings suggest that fire history is not a key factor for restoring pencil pines, as transplanting soon after fire or decades later yields similar results. We note shrub cover was higher in historically burnt than recently burnt sites, which we discuss in a separate section below.
4.3.3 Landscape position and microtopography
We demonstrate that fine-scale choice of planting position influences pencil pine transplant establishment rates. This aligns with a growing body of work showing that restoration plantings benefit from selecting appropriate microsites based on indicator species (Dunwiddie and Martin, 2016), proximity to shelter (Bailey et al., 2012), substrate characteristics (Douterlungne et al., 2015), or topographic variables (Larkin et al., 2006). For example, fine-scale topography influences transplant survival in Pacific North West prairies (Dunwiddie and Martin, 2016), dune systems in Michigan (Halsey et al., 2017) and southeast Australian wetlands (Raulings et al., 2007). We used our simple topographic classification system to demonstrate microsite effects in our study system, at both 3 m (i.e., landscape position) and 0.5 m (i.e., microtopography) scales. Though we intended our system to be a practical tool, we did not field-test it and it may need refinement for on-ground use. However, it is clear that fine-scale choice of favourable planting positions can enhance the effectiveness of pencil pine transplanting, and should be considered.
It is unintuitive that waterlogging would impede young pencil pines given the densest pencil pine stands are in wet areas. However poor drainage reduced establishment rates at both scales we considered (3 m and 0.5 m). Topography influences vegetation by modulating drought stress (Tongway and Ludwig, 1994) and frost occurrence (Marquis et al., 2021), in additon to waterlogging (Raulings et al., 2007). Whilst both frost and waterlogging impacts are strongest in drainage hollows, frost seems an unlikely driver of the topographic effects we observed because laboratory studies show juvenile and adult pencil pines are remarkably frost tolerant (Sakai et al., 1981; Cullen and Kirkpatrick, 1988b). In contrast, waterlogging is a known constraint on pencil pine seedlings (Cullen and Kirkpatrick, 1988b). We suggest pencil pine transplanting should target well-drained microsites, but note that the influence of topography might differ in dry periods, when hollows are not inundated, and plants in well-drained positions may be more prone to drought stress and fire.
The successful establishment of transplants in Sphagnum moss, which often growns in inundated environments, may seem to contradict the idea of negative waterlogging effects. However, when planting in Sphagnum we did not dig down to the underlying soil (which was often beneath >1 m of Sphagnum). Rather, we planted into the surface of the moss itself, where free water (i.e. puddling) was less common than in wet and moderate positions (especially after rain). Therefore, the high rate of transplant establishment in Sphagnum positions further highlights that waterlogging and its effects can vary at very fine scales. Though transplants in Sphagnum and dry positions showed similar establishment rates, we hypothesise that transplants in dry positions will exhibit better long-term survival and growth, due to more nutrients being available in soil compared to Sphagnum. These ideas are speculative, but future re-surveys could explore them in detail.
4.4 Effects of shrub cover on pencil pines
Shrubs – a major vegetation component where pencil pines occur – are an important consideration for restoring burnt stands, because they may influence pencil pine recruitment and survival via several mechanisms.
Shrubs may promote natural pencil pine recruitment by providing structural protection from herbivores; acting as ‘nurse’ plants (Padilla and Pugnaire, 2006). Our finding that transplants without tree guards survived more often in shrubbier sites (i.e. burnt historically rather than recently) is consistent with this, suggesting naturally occurring pencil pine seedlings are more vulnerable to herbivory in areas with low shrub cover.
Shrubs compete with pencil pine seedlings for light and other resources. For example, shading by the shrub layer likely limits seedling establishment, given pencil pine recruitment in mature stands is restricted to light gaps (Cullen and Kirkpatrick, 1988a). We found no evidence of such effects for our transplants, around which a small area of vegetation was removed, reducing shading and possibly below ground competition. Re-growth of this cleared vegetation was minimal over the course of our study. Future plantings are also unlikely to be affected by high shrub cover if they follow the same protocol. However, the extent of shrub competition effects in the absence of such treatment is unknown.
High shrub cover increases the flammability of pencil pine stands, reducing the likelihood of both juvenile and adult pencil pines surviving in the long term. Further, shrub-fire feedbacks have been demonstrated – whereby shrubs proliferate after pencil pine stands burn, increasing tree mortality rates in subsequent fires (Holz et al., 2015; Bowman et al., 2019). Such feedbacks may jeopardise burnt stand restoration if the increased fire risk is not managed.
4.5 Management recommendations
We recommend transplanting tube stock, with herbivore protection, as an intervention approach to conserve pencil pines. Tube stock may be propagated from seed or from cuttings – whichever is most convenient – and tube stock 1.5 years old at the time of transplanting are sufficient for establishment. We found no evidence that tube stock provenance is important. Transplants must be protected from vertebrate herbivores, particularly macropods, and in the rugged terrain where pencil pines occur individual tree guards are an effective way of achieving this. Stands burnt recently, and in historic fires, are equally suitable targets for restoration, however establishment rates are influenced by the fine-scale choice of planting position. Well-drained landscape positions are best, followed by Sphagnum dominated areas. At the fine scale, runoff microtopography (hummocks, mounds and slopes) is preferable to run-on microtopography (hollows, pans, depressions, flats), particularly in wet areas.
We stress that our recommendations – which are based on pencil pine establishment patterns in the first trial of conservation interventions for the species – must be adapted as new information is gathered. More work exploring the patterns of longer-term transplant survival and growth is required. Additionally, the topography effects we outline (i.e. well-drained positions are favourable) could reverse in drought conditions, particularly since transplants were resurveyed after a single summer that was unusually wet. Future resurveys of this trial are required, particularly following drought years. The potential of direct sowing and natural germinant interventions also warrants further attention.
The abundant historically burnt stands in the Central Plateau provide many restoration opportunities. Fires since European invasion of Tasmania have removed pencil pines from approximately one third of their global range (Bowman et al., 2019); a continuing trend (Harris et al., 2018; Mariani et al., 2019) which only active intervention will mitigate. Rather than wait to respond to new fires, it would be prudent to initiate a planting program promptly, given pencil pines grow very slowly, and that lead time is required (i.e., to source funding, obtain permits and propagate tube stock). Such elective restoration efforts could be re-routed if higher priority (e.g., more frequently visited) stands burn in the meantime.
Where interventions are located at the landscape scale should be carefully considered. A useful tool to inform such decisions is bioclimatic modelling, which allows populations to be assessed for their resilience to climate change. Bioclimatic models can also highlight refugia – areas that can facilitate a species persistence through adverse conditions (Keppel et al., 2012). Athrotaxis species have already been used in bioclimatic models, providing clues for decision makers (Porfirio et al., 2014; Keppel et al., 2015; Mariani et al., 2019). For example, one study found that many montane forest stands, containing Athrotaxis, are near the climatic margins for that forest type, and suggested conservation efforts should focus on stands in the most climatically suitable areas (Mariani et al., 2019). That study also highlighted many unoccupied refugia in Tasmania into which pencil pines could be translocated. This could increase the spatial redundancy of pencil pines, and hence reduce the species vulnerability to disturbances.
If restoring burnt pencil pine stands proves possible it will be slow, expensive, and will produce stands of a different character to natural ones (e.g., more uniform age structure, different spatial patterning and a lack of clonal clusters). Thus, managers should continue to prioritise protecting existing stands. Targeted fire suppression has often been used to protect Gondwanan vegetation remnants, including the use of temporary sprinklers to save an Athrotaxis stand at Lake Rhona from wildfire in 2018. Mechanical fuel management – such as removing shrubs to decrease pencil pine mortality rates during fire (Bowman et al., 2019) – could also be an effective means of protection. Our results highlight a potential trade-off for shrub removal, as this may make wild pencil pine seedlings more vulnerable to herbivory. Nonetheless, it is likely that proactively managing fuels would prove cheaper and more effective than solely relying on wildfire suppression.
4.6 Conclusion
Climate change is increasing the exposure of pencil pines to fire, and burnt stands are very unlikely to recover naturally given recruitment is absent across much of the species range (Holz et al., 2015; Harris et al., 2018; Bergstrom et al., 2021; Bliss et al., 2021). Therefore, interventions to retain this relictual Gondwanan species in the landscape are justified. Restoration of burnt pencil pine stands is most likely to succeed if methods are based on robust field data and refined through adaptive management; the highly replicated field trial we have established provides a strong starting point for this. Transplanting is a more promising intervention than either direct sowing or protecting naturally occurring germinants from herbivores, though further testing of these approaches is warranted. Transplanting will only succeed if plants are protected from macropod herbivores, which are a strong top-down filter. Transplant establishment can be further improved by choosing favourable planting positions at fine scales, because bottom-up effects, such as waterlogging, also constrain young pencil pines. The many burnt stands that already exist are suitable for restoration, and hence a planting program targeting these stands could start now. The guidelines presented must be adapted following future research, including re-surveys of this trial, in which longer-term patterns of survival and growth can be assessed. Other interventions to conserve pencil pines should also be considered, such as establishing populations in unoccupied fire refugia, and using fine-scale fuel management to protect existing stands from fire.
Data availability statement
The raw data supporting the conclusions of this article will be made available by the authors, without undue reservation.
Author contributions
BF: Conceptualization, Data curation, Formal analysis, Funding acquisition, Investigation, Methodology, Project administration, Visualization, Writing – original draft, Writing – review & editing. LP: Conceptualization, Formal analysis, Investigation, Methodology, Supervision, Validation, Visualization, Writing – review & editing. DB: Conceptualization, Funding acquisition, Investigation, Methodology, Project administration, Supervision, Writing – review & editing.
Funding
The author(s) declare financial support was received for the research, authorship, and/or publication of this article. This project was funded by the Department of Natural Resources and Environment Tasmania, as part of the Lake Mackenzie Alpine Restoration Project. BF was supported by a Research Training Program (RTP) scholarship from the Australian Government and a Future Leaders Scholarship from the Westpac Scholars Trust.
Acknowledgments
We pay our respects to the palawa peoples of lutrawita/Tasmania, who are the traditional custodians of the lands on which this work was undertaken. Chris Johnson and Steven Leonard significantly contributed to interpreting the results. Participants in the on-site planning event in 2018 contributed to the project, including Micah Visoiu, Kath Storey, Chris Emms and Rob Buck. Darren Turner undertook the UAV surveys, and he and Grant Williamson provided GIS support. We thank Herb and Sally Staubman (Habitat Plants Tasmania), who propagated the transplants and provided advice on the transplanting and sowing methods. The Royal Tasmanian Botanical Gardens donated seeds from their collection; James Wood facilitated this. In kind support was provided by the Tasmanian Parks and Wildlife Service Great Western Tiers Field Centre. Dale Crosswell helped design the tree guards. Cam Geeves assisted in the field. Expert advice was provided by Tanya Bailey, Greg Jordan, Jamie Kirkpatrick and Tim Brodribb. We thank the following field volunteers for their substantial contributions: Tony French, Morgan Harding, Barb French, Claire Butler, Adrien Butler, Bill Butler, Kate Brown, Harrison Raine, Elise Ringwaldt, Loic Auderset, Julie Hunt, Steph Gebbie, Henry Pörner, Ryan King, Benjamin Cendrie, Thalia Goode, Andy Szollosi, Sinéad Nicholson, Sharna Turner, Jordie Reinets, Alyssa Hawley, Maddy King, Lisa Shiu Fen, Toby Travers, Owen Cameron, Cam Geeves, Charlotte Blake, Jill Vaughan and Liisa Syndänmestä.
Conflict of interest
The authors declare that the research was conducted in the absence of any commercial or financial relationships that could be construed as a potential conflict of interest.
The reviewer MM declared a past co-authorship with the author DB to the handling editor.
Publisher’s note
All claims expressed in this article are solely those of the authors and do not necessarily represent those of their affiliated organizations, or those of the publisher, the editors and the reviewers. Any product that may be evaluated in this article, or claim that may be made by its manufacturer, is not guaranteed or endorsed by the publisher.
Supplementary material
The Supplementary Material for this article can be found online at: https://www.frontiersin.org/articles/10.3389/fcosc.2024.1491062/full#supplementary-material
References
Ashmore S. E., Hamilton K. N., Offord C. A. (2011). Conservation technologies for safeguarding and restoring threatened flora: case studies from eastern Australia. In Vitro Cell. Dev. Biol. - Plant 47, 99–109. doi: 10.1007/s11627-010-9320-9
Ashton P., Gamage S., Gunatilleke I., Gunatilleke C. (1997). Restoration of a Sri Lankan rainforest: using Caribbean pine (Pinus caribaea) as a nurse for establishing late-successional tree species. J. Appl. Ecol. 34, 915–925. doi: 10.2307/2405282
Bailey T. G., Davidson N. J., Close D. C. (2012). Understanding the regeneration niche: microsite attributes and recruitment of eucalypts in dry forests. For. Ecol. Manage. 269, 229–238. doi: 10.1016/j.foreco.2011.12.021
Bailey T. G., Harrison P. A., Davidson N. J., Weller-Wong A., Tilyard P., Steane D. A., et al. (2021a). Embedding genetics experiments in restoration to guide plant choice for a degraded landscape with a changing climate. Ecol. Manage. Restor. 22, 92–105. doi: 10.1111/emr.v22.s2
Bailey T. G., Harrison P. A., Hanusch Y., Ranyard C., Hooghkirk C., J. Davidson N., et al. (2021b). Investigating constraints on direct seeding for native revegetation in the Tasmanian Midlands. Ecol. Manage. Restor. 22, 106–117. doi: 10.1111/emr.v22.s2
Banks M. (1972). “Geomorphology,” in Papers and Proceedings of the Royal Society of Tasmania (Hobart: The Lake Country of Tasmania), 55–60. Available at: https://rst.org.au/papers-and-proceedings/.
Bassett O. D., Prior L. D., Slijkerman C. M., Jamieson D., Bowman D. M. (2015). Aerial sowing stopped the loss of alpine ash (Eucalyptus delegatensis) forests burnt by three short-interval fires in the Alpine National Park, Victoria, Australia. For. Ecol. Manage. 342, 39–48. doi: 10.1016/j.foreco.2015.01.008
Beltran R. S., Kreidler N., Van Vuren D. H., Morrison S. A., Zavaleta E. S., Newton K., et al. (2014). Passive recovery of vegetation after herbivore eradication on Santa Cruz Island, California. Restor. Ecol. 22, 790–797. doi: 10.1111/rec.2014.22.issue-6
Bergstrom D. M., Wienecke B. C., van den Hoff J., Hughes L., Lindenmayer D. B., Ainsworth T. D., et al. (2021). Combating ecosystem collapse from the tropics to the Antarctic. Global Change Biol. 27, 1692–1703. doi: 10.1111/gcb.15539
Bliss A., Prior L. D., Bowman D. M. (2021). Lack of reliable post-fire recovery mechanisms makes the iconic Tasmanian conifer Athrotaxis cupressoides susceptible to population decline. Aust. J. Bot. 69, 162–173. doi: 10.1071/BT20117
Bowman D. M. J. S., Bliss A., Bowman C. J. W., Prior L. D. (2019). Fire caused demographic attrition of the Tasmanian palaeoendemic conifer Athrotaxis cupressoides. Austral Ecol. 44, 1322–1339. doi: 10.1111/aec.12789
Bowman D. M. J. S., Garnett S. T., Barlow S., Bekessy S. A., Bellairs S. M., Bishop M. J., et al. (2017). Renewal ecology: conservation for the Anthropocene. Restor. Ecol. 25, 674–680. doi: 10.1111/rec.2017.25.issue-5
Bowman D. M., Kolden C. A., Williamson G. J. (2022). Bushfires in Tasmania, Australia: an introduction. Fire 5, 33. doi: 10.3390/fire5020033
Bowman D. M., Rodriguez-Cubillo D., Prior L. D. (2021). “The 2016 Tasmanian wilderness fires: fire regime shifts and climate change in a Gondwanan biogeographic refugium,” in Ecosystem Collapse and Climate Change (Springer), 133–153.
Breed M. F., Harrison P. A., Bischoff A., Durruty P., Gellie N. J., Gonzales E. K., et al. (2018). Priority actions to improve provenance decision-making. BioScience 68, 510–516. doi: 10.1093/biosci/biy050
Breed M. F., Stead M. G., Ottewell K. M., Gardner M. G., Lowe A. J. (2013). Which provenance and where? Seed sourcing strategies for revegetation in a changing environment. Conserv. Genet. 14, 1–10. doi: 10.1007/s10592-012-0425-z
Broadhurst L. M., Lowe A., Coates D. J., Cunningham S. A., McDonald M., Vesk P. A., et al. (2008). Seed supply for broadscale restoration: maximizing evolutionary potential. Evolution. Appl. 1, 587–597. doi: 10.1111/j.1752-4571.2008.00045.x
Broadhurst L., Prober S., Dickson F., Bush D. (2017). Using restoration as an experimental framework to test provenancing strategies and climate adaptability. Ecol. Manage. Restor. 18, 205–208. doi: 10.1111/emr.2017.18.issue-3
Bureau of Meteorology. (2022). Climate data online. Available online at: http://www.bom.gov.au/climate/data/ (accessed July 15, 2022).
Cogoni D., Fenu G., Concas E., Bacchetta G. (2013). The effectiveness of plant conservation measures: the Dianthus morisianus reintroduction. Oryx 47, 203–206. doi: 10.1017/S003060531200169X
Cox A. C., Gordon D. R., Slapcinsky J. L., Seamon G. S. (2004). Understory restoration in longleaf pine sandhills. Natural Areas J. 24, 4–14.
Cubit S. (1996). [amp]]lsquo;Burning back with the snow’: traditional approaches to grassland management in Tasmania. Aust. Geogr. Stud. 34, 216–224. doi: 10.1111/j.1467-8470.1996.tb00117.x
Cullen P. (1987). Regeneration patterns in populations of Athrotaxis selaginoides D. Don. from Tasmania. J. Biogeogr., 39–51.
Cullen P., Kirkpatrick J. (1988a). The ecology of Athrotaxis D. Don (Taxodiaceae). I. Stand structure and regeneration of A. cupressoides. Aust. J. Bot. 36, 547–560. doi: 10.1071/BT9880547
Cullen P., Kirkpatrick J. (1988b). The ecology of Athrotaxis D. Don (Taxodiaceae). II. The distributions and ecological differentiation of A. cupressoides and A. selaginoides. Aust. J. Bot. 36, 561–573. doi: 10.1071/BT9880561
Dalrymple S. E., Banks E., Stewart G. B., Pullin A. S. (2012). “A meta-analysis of threatened plant reintroductions from across the globe,” in Plant reintroduction in a changing climate. Eds. Maschinski J., Hawkins K. (Springer), 31–50.
De Steven D., Sharitz R. R., Singer J. H., Barton C. D. (2006). Testing a passive revegetation approach for restoring coastal plain depression wetlands. Restor. Ecol. 14, 452–460. doi: 10.1111/j.1526-100X.2006.00153.x
Dodson J. (2001). A vegetation and fire history in a subalpine woodland and rain-forest region, Solomons Jewel Lake, Tasmania. Holocene 11, 111–116. doi: 10.1191/095968301670253295
Douterlungne D., Ferguson B. G., Siddique I., Soto-Pinto L., Jímenez-Ferrer G., Gavito M. E. (2015). Microsite determinants of variability in seedling and cutting establishment in tropical forest restoration plantations. Restor. Ecol. 23, 861–871. doi: 10.1111/rec.2015.23.issue-6
Dunwiddie P., LaMarche V. Jr. (1980). Dendrochronological characteristics of some native Australian trees. Aust. Forest. 43, 124–135. doi: 10.1080/00049158.1980.10674257
Dunwiddie P. W., Martin R. A. (2016). Microsites matter: improving the success of rare species reintroductions. PloS One 11, e0150417. doi: 10.1371/journal.pone.0150417
Engelbrecht B. M., Kursar T. A. (2003). Comparative drought-resistance of seedlings of 28 species of co-occurring tropical woody plants. Oecologia 136, 383–393. doi: 10.1007/s00442-003-1290-8
Fletcher M. S., Bowman D. M. J. S., Whitlock C., Mariani M., Stahle L. (2018). The changing role of fire in conifer-dominated temperate rainforest through the last 14,000 years. Quater. Sci. Rev. 182, 37–47. doi: 10.1016/j.quascirev.2017.12.023
Gaitán-Espitia J. D., Hobday A. J. (2021). Evolutionary principles and genetic considerations for guiding conservation interventions under climate change. Global Change Biol. 27, 475–488. doi: 10.1111/gcb.15359
Godefroid S., Piazza C., Rossi G., Buord S., Stevens A.-D., Aguraiuja R., et al. (2011). How successful are plant species reintroductions? Biol. Conserv. 144, 672–682. doi: 10.1016/j.biocon.2010.10.003
Grossnickle S. C., Ivetić V. (2017). Direct seeding in reforestation–a field performance review. Reforesta 4, 94–142. doi: 10.21750/REFOR.4.07.46
Hallett L. M., Standish R. J., Jonson J., Hobbs R. J. (2014). Seedling emergence and summer survival after direct seeding for woodland restoration on old fields in south-western Australia. Ecol. Manage. Restor. 15, 140–146. doi: 10.1111/emr.2014.15.issue-2
Halsey S. J., Bell T. J., Bowles M. (2017). Initial transplant size and microsite influence transplant survivorship and growth of a threatened dune thistle. Ecol. Restor. 35, 52–59. doi: 10.3368/er.35.1.52
Hannah L. (2008). Protected areas and climate change. Ann. New York Acad. Sci. 1134, 201–212. doi: 10.1196/nyas.2008.1134.issue-1
Harris R. M., Beaumont L. J., Vance T. R., Tozer C. R., Remenyi T. A., Perkins-Kirkpatrick S. E., et al. (2018). Biological responses to the press and pulse of climate trends and extreme events. Nat. Climate Change 8, 579–587. doi: 10.1038/s41558-018-0187-9
Heywood V. H. (2019). Conserving plants within and beyond protected areas – still problematic and future uncertain. Plant Diversity 41, 36–49. doi: 10.1016/j.pld.2018.10.001
Holl K. D., Aide T. M. (2011). When and where to actively restore ecosystems? For. Ecol. Manage. 261, 1558–1563. doi: 10.1016/j.foreco.2010.07.004
Holz A., Wood S. W., Veblen T. T., Bowman D. M. (2015). Effects of high-severity fire drove the population collapse of the subalpine Tasmanian endemic conifer Athrotaxis cupressoides. Global Change Biol. 21, 445–458. doi: 10.1111/gcb.2014.21.issue-1
Ibanez T., Hart P. J. (2020). Spatial patterns of tree recruitment in a montane Hawaiian wet forest after cattle removal and pig population control. Appl. Veget. Sci. 23, 197–209. doi: 10.1111/avsc.12478
Jackson W. (1972). Vegetation of the central plateau. Papers Proc. R. Soc. Tasmania, 61–86. doi: 10.26749/rstpp.106.1.61
Johnson K., Marsden-Smedley J. (2002). Fire history of the northern part of the Tasmanian Wilderness World Heritage Area and its associated regions. Papers Proc. R. Soc. Tasmania 136, 145–152. doi: 10.26749/SFCE4919
Jordan G. J., Harrison P. A., Worth J. R., Williamson G. J., Kirkpatrick J. B. (2016). Palaeoendemic plants provide evidence for persistence of open, well-watered vegetation since the Cretaceous. Global Ecol. Biogeogr. 25, 127–140. doi: 10.1111/geb.2016.25.issue-2
Jusaitis M., Sorensen B. (2007). Successful augmentation of an Acacia whibleyana (Whibley Wattle) population by translocation. Australasian Plant Conservation: Journal of the Australian Network for Plant Conserv. 16, 23–24.
Keppel G., Mokany K., Wardell-Johnson G. W., Phillips B. L., Welbergen J. A., Reside A. E. (2015). The capacity of refugia for conservation planning under climate change. Front. Ecol. Environ. 13, 106–112. doi: 10.1890/140055
Keppel G., Van Niel K. P., Wardell-Johnson G. W., Yates C. J., Byrne M., Mucina L., et al. (2012). Refugia: identifying and understanding safe havens for biodiversity under climate change. Global Ecol. Biogeogr. 21, 393–404. doi: 10.1111/j.1466-8238.2011.00686.x
Klaus V. H., Hoever C. J., Fischer M., Hamer U., Kleinebecker T., Mertens D., et al. (2018). Contribution of the soil seed bank to the restoration of temperate grasslands by mechanical sward disturbance. Restor. Ecol. 26, S114–S122. doi: 10.1111/rec.2018.26.issue-S2
Larkin D., Vivian-Smith G., Zedler J. B. (2006). Topographic heterogeneity theory and ecological restoration,” in Found. Restor. Ecol. ed. Falk D. A., Palmer M. A., Zedler J. B. (Washinton: Island Press), 142–164.
Li Y.-Y., Tsang E. P. K., Cui M.-Y., Chen X.-Y. (2012). Too early to call it success: an evaluation of the natural regeneration of the endangered Metasequoia glyptostroboides. Biol. Conserv. 150, 1–4. doi: 10.1016/j.biocon.2012.02.020
Linabury M. C., Turley N. E., Brudvig L. A. (2019). Insects remove more seeds than mammals in first-year prairie restorations. Restor. Ecol. 27, 1300–1306. doi: 10.1111/rec.13004
Lorite J., Salazar-Mendías C., Pawlak R., Cañadas E. M. (2021). Assessing effectiveness of exclusion fences in protecting threatened plants. Sci. Rep. 11, 1–8. doi: 10.1038/s41598-021-95739-4
Mariani M., Fletcher M. S., Haberle S., Chin H., Zawadzki A., Jacobsen G. (2019). Climate change reduces resilience to fire in subalpine rainforests. Global Change Biol. 25, 2030–2042. doi: 10.1111/gcb.2019.25.issue-6
Marquis B., Bergeron Y., Simard M., Tremblay F. (2021). Disentangling the effect of topography and microtopography on near-ground growing-season frosts at the boreal-temperate forest ecotone (Québec, Canada). New Forests 52, 1079–1098. doi: 10.1007/s11056-021-09840-7
Marris E. (2016). Tasmanian bushfires threaten iconic ancient forests. Nature 530, 137–138. doi: 10.1038/nature.2016.19308
Menges E. S., Smith S. A., Weekley C. W. (2016). Adaptive introductions: how multiple experiments and comparisons to wild populations provide insights into requirements for long-term introduction success of an endangered shrub. Plant Divers. 38, 238–246. doi: 10.1016/j.pld.2016.09.004
Miandrimanana C., Reid J. L., Rivoharison T., Birkinshaw C. (2019). Planting position and shade enhance native seedling performance in forest restoration for an endangered malagasy plant. Plant Divers. 41, 118–123. doi: 10.1016/j.pld.2018.09.005
Mortlock B. W. (2000). Local seed for revegetation: where will all that seed come from? Ecol. Manage. Restor. 1, 93–101. doi: 10.1046/j.1442-8903.2000.00029.x
Morton A.. (2016). ‘Like losing the thylacine’: fire burns Tasmanian wilderness world heritage area. Available online at: https://www.smh.com.au/environment/like-losing-the-thylacine-fire-burns-tasmanian-wilderness-world-heritage-area-20160131-gmi2re.html.
Muñoz-Rojas M., Erickson T. E., Martini D. C., Dixon K. W., Merritt D. J. (2016). Climate and soil factors influencing seedling recruitment of plant species used for dryland restoration. Soil 2, 287–298. doi: 10.5194/soil-2-287-2016
Nilar H., Maute K., Dawson M. J., Scarborough R., Hudson J., Reay J., et al. (2019). Effectiveness of different herbivore exclusion strategies for restoration of an endangered rainforest community. For. Ecol. Manage. 435, 18–26. doi: 10.1016/j.foreco.2018.12.041
Ogden J. (1978). Investigations of the dendrochronology of the genus Athrotaxis D. Don (Taxodiaceae) in Tasmania. Tree Ring Bull. 38, 1–13.
Overdyck E., Clarkson B. D., Laughlin D. C., Gemmill C. E. (2013). Testing broadcast seeding methods to restore urban forests in the presence of seed predators. Restor. Ecol. 21, 763–769. doi: 10.1111/j.1526-100X.2012.00933.x
Padilla F. M., Pugnaire F. I. (2006). The role of nurse plants in the restoration of degraded environments. Front. Ecol. Environ. 4, 196–202. doi: 10.1890/1540-9295(2006)004[0196:TRONPI]2.0.CO;2
Palma A. C., Laurance S. G. (2015). A review of the use of direct seeding and seedling plantings in restoration: what do we know and where should we go? Appl. Veget. Sci. 18, 561–568. doi: 10.1111/avsc.2015.18.issue-4
Pecl G. T., Araújo M. B., Bell J. D., Blanchard J., Bonebrake T. C., Chen I.-C., et al. (2017). Biodiversity redistribution under climate change: impacts on ecosystems and human well-being. Science 355, 9214. doi: 10.1126/science.aai9214
Porfirio L. L., Harris R. M., Lefroy E. C., Hugh S., Gould S. F., Lee G., et al. (2014). Improving the use of species distribution models in conservation planning and management under climate change. PloS One 9, e113749. doi: 10.1371/journal.pone.0113749
Prior L. D., Bowman D. M. (2020). Classification of post-fire responses of woody plants to include pyrophobic communities. Fire 3, 15. doi: 10.3390/fire3020015
Prior L. D., French B. J., Bowman D. M. (2018). Effect of experimental fire on seedlings of Australian and Gondwanan trees species from a Tasmanian montane vegetation mosaic. Aust. J. Bot. 66, 511–517. doi: 10.1071/BT18124
Prior L., French B., Williamson G., Nichols S., Bliss A., Porter M., et al. (2023). TWWHA Bushfire Recovery Lake Mackenzie Sphagnum and Pencil Pine Rehabilitation Trials Final Report (NRE Tas, Hobart). Available at: https://nre.tas.gov.au/Documents/TWWHA%20Bushfire%20Recovery%20-%20Lake%20Mackenzie%20Sphagnum%20and%20Pencil%20Pine%20Rehabilitation%20Trials.pdf.
Prober S. M., Byrne M., McLean E. H., Steane D. A., Potts B. M., Vaillancourt R. E., et al. (2015). Climate-adjusted provenancing: a strategy for climate-resilient ecological restoration. Front. Ecol. Evol. 3, 65. doi: 10.3389/fevo.2015.00065
Prober S. M., Doerr V. A., Broadhurst L. M., Williams K. J., Dickson F. (2019). Shifting the conservation paradigm: a synthesis of options for renovating nature under climate change. Ecol. Monogr. 89, e01333. doi: 10.1002/ecm.2019.89.issue-1
Pyke D. A., Brooks M. L., D’Antonio C. (2010). Fire as a restoration Tool: a decision framework for predicting the control or enhancement of plants using fire. Restor. Ecol. 18, 274–284. doi: 10.1111/j.1526-100X.2010.00658.x
Raulings E. J., Boon P. I., Bailey P. C., Roache M. C., Morris K., Robinson R. (2007). Rehabilitation of swamp paperbark (Melaleuca ericifolia) wetlands in south-eastern Australia: effects of hydrology, microtopography, plant age and planting technique on the success of community-based revegetation trials. Wetlands Ecol. Manage. 15, 175–188. doi: 10.1007/s11273-006-9022-6
Ray G. J., Brown B. J. (1995). Restoring Caribbean dry forests: evaluation of tree propagation techniques. Restor. Ecol. 3, 86–94. doi: 10.1111/j.1526-100X.1995.tb00081.x
R Core Team (2022). R: A language and environment for statistical computing (Vienna, Austria: R Foundation for Statistical Computing).
Read J. (1989). Phenology and germination in some rainforest canopy species at Mt Field National Park, Tasmania. Papers Proc. R. Soc. Tasmania 123, 211–221. doi: 10.26749/rstpp.123.211
Richards S. A. (2008). Dealing with overdispersed count data in applied ecology. J. Appl. Ecol. 45, 218–227. doi: 10.1111/j.1365-2664.2007.01377.x
Sakai A., M. P. D., Wardle P. (1981). Freezing resistance of trees of the South Temperate Zone, especially subalpine species of Australasia. Ecology 62, 563–570. doi: 10.2307/1937722
Scheffers B. R., De Meester L., Bridge T. C., Hoffmann A. A., Pandolfi J. M., Corlett R. T., et al. (2016). The broad footprint of climate change from genes to biomes to people. Science 354, aaf7671. doi: 10.1126/science.aaf7671
Siles G., Rey P. J., Alcántara J. M., Bastida J. M., Herreros J. L. (2010). Effects of soil enrichment, watering and seedling age on establishment of Mediterranean woody species. Acta Oecol. 36, 357–364. doi: 10.1016/j.actao.2010.03.002
Styger J., Marsden-Smedley J., Kirkpatrick J. (2018). Changes in lightning fire incidence in the Tasmanian wilderness world heritage area 1980–2016. Fire 1, 38. doi: 10.3390/fire1030038
The IUCN Red List of Threatened Species. (2023). Pencil Pine. Available online at: https://www.iucnredlist.org/en (accessed November 23, 2024).
Tongway D. J., Ludwig J. A. (1994). Small-scale resource heterogeneity in semi-arid landscapes. Pacific Conserv. Biol. 1, 201–208. doi: 10.1071/PC940201
Volis S. (2019). Conservation-oriented restoration – a two for one method to restore both threatened species and their habitats. Plant Diversity 41, 50–58. doi: 10.1016/j.pld.2019.01.002
Wahlquist C. (2020). “Seeds of hope: on a mission to protect Tasmania’s ancient pencil pines,” in The Guardian. Available at: https://www.theguardian.com/environment/2020/may/14/seeds-of-hope-on-a-mission-to-protect-tasmanias-ancient-pencil-pines.
Wang T., O’Neill G. A., Aitken S. N. (2010). Integrating environmental and genetic effects to predict responses of tree populations to climate. Ecol. Appl. 20, 153–163. doi: 10.1890/08-2257.1
Whinam J., Kirkpatrick J. (1994). The Mount Wellington string bog, Tasmania. Papers Proc. R. Soc. Tasmania 128, 63–68. doi: 10.26749/rstpp.128.63
Worth J. R., Sakaguchi S., Rann K. D., Bowman C. J., Ito M., Jordan G. J., et al. (2016). Gondwanan conifer clones imperilled by bushfire. Sci. Rep. 6, 1–6. doi: 10.1038/srep33930
Zahawi R. A., Holl K. D. (2009). Comparing the performance of tree stakes and seedlings to restore abandoned tropical pastures. Restor. Ecol. 17, 854–864. doi: 10.1111/j.1526-100X.2008.00423.x
Keywords: Athrotaxis, paleoendemic species, Gondwanan relict, climate change adaptation, active restoration, Tasmanian Central Plateau
Citation: French BJ, Prior LD and Bowman DMJS (2024) Transplanting interventions could help conserve the living fossil Athrotaxis cupressoides under fire regimes induced by climate change. Front. Conserv. Sci. 5:1491062. doi: 10.3389/fcosc.2024.1491062
Received: 04 September 2024; Accepted: 21 October 2024;
Published: 28 November 2024.
Edited by:
Richard T. Corlett, Chinese Academy of Sciences (CAS), ChinaReviewed by:
Michela Mariani, University of Nottingham, United KingdomMatt Sean McGlone, Manaaki Whenua Landcare Research, New Zealand
Copyright © 2024 French, Prior and Bowman. This is an open-access article distributed under the terms of the Creative Commons Attribution License (CC BY). The use, distribution or reproduction in other forums is permitted, provided the original author(s) and the copyright owner(s) are credited and that the original publication in this journal is cited, in accordance with accepted academic practice. No use, distribution or reproduction is permitted which does not comply with these terms.
*Correspondence: Ben J. French, QmVuLkZyZW5jaEB1dGFzLmVkdS5hdQ==