- 1Instituto Politécnico Nacional-Centro Interdisciplinario de Ciencias Marinas, Departamento de Oceanología, La Paz, Baja California Sur, Mexico
- 2Facultad de Ciencias Biologicas y Agropecuarias, Universidad Veracruzana, Región Poza Rica-Tuxpan, Veracruz, Mexico
- 3Instituto Politécnico Nacional-Centro Interdisciplinario de Ciencias Marinas, Departamento de Pesquerías y Biología Marina, La Paz, Baja California Sur, Mexico
- 4Universidad Nacional Autónoma de México, Instituto de Biología, Ciudad de México, Mexico
- 5Centro de Investigación en Alimentación y Desarrollo, A. C. Guaymas, Sonora, Mexico
- 6Centro de Investigación Científica y de Educación Superior de Ensenada (CICESE), Departamento de Oceanografía Física, Ensenada, Baja California, Mexico
The vaquita (Phocoena sinus) is an endemic species of the Upper Gulf of California (UGC), which is in a critical state of extinction. Bycatch has been considered the main factor leading to its potential extinction; however, the impact of the damming of the Colorado River on the species’ ecology has not been studied. Stable isotopes of carbon (δ13C) and oxygen (δ18O) from vaquita bones were analyzed as indicators of the carbon source of primary producers and habitat use in the UGC from 1982 to 1993. Based on the Colorado River’s flow into the UGC, two periods were markedly different: from 1982 to 1988, when freshwater arrived, and from 1989 to 1993, when the flow was null. Sea surface salinity (SSS) data showed the inverse of the river’s flow pattern, being significantly lower at the end of the 1980s than at the beginning of the 1990s. In agreement with the above, sea surface temperature (SST)/SSS diagrams showed the presence of two water masses inside a gradient from 33.8 to 35.2 psu. The δ13C was significantly different between both periods, with a mean value of -9.1‰ at the end of the 1980s and a mean value of -10.8‰ at the beginning of the 1990s. This means that, when the river flow was dammed, the carbon source of primary producers changed significantly in the UGC. However, the δ18O was not significantly different between both periods, with values of 30.4‰ and 30.5‰, respectively. Comparing the vaquita’s average values of δ18O from this study with those of other marine mammals obtained from previous studies revealed that the vaquita is the most enriched marine mammal species, which could be the result of the high evaporation and salinity that currently occur in the UGC, the vaquita’s habitat. A longer temporal series might show changes in δ18O, which have been detected in other species living in the UGC. From a conservation point of view, the results showed that the impact of environmental variability on the trophic ecology of the vaquita has potential effects on the species’ health.
1 Introduction
The management decisions and conservation actions focused on wild marine mammals should be supported by accurate ecological information about their population structure and function in the ecosystem (Franklin, 1993; Drago et al., 2020). Such knowledge could help us understand their habitat use and interactions with their prey and predators, as well as fisheries. Understanding the interactions of marine mammals with fisheries could avoid bycatch during competition for resources and habitat use (Reeves et al., 2005, 2013). Unfortunately, information about habitat use is often missing for many marine mammal species (Schipper et al., 2008). The study of wild marine mammals can be challenging, especially the study of free-swimming animals of turbid waters that surface for only brief periods (Moore et al., 2018), as is the case for the vaquita, Phocoena sinus, a small porpoise endemic to the Upper Gulf of California (UGC).
With a current population of only 10 animals (NOM-059-SEMARNAT-2010, Diario Oficial de la Federación (2010), https://iucn-csg.org/wp-content/uploads/2023/06/Vaquita-Survey-2023-Main-Report.pdf), the vaquita is on the verge of extinction. The leading cause of its potential extinction has been identified as bycatch in fishing nets (Vidal, 1995; Rojas-Bracho et al., 2006; CIRVA, 2019). In response to this issue, the UGC and Colorado River Delta Biosphere Reserve were officially designated as protected areas in 1993 (Diario Oficial de la Federación, 1993). Moreover, the Mexican government carried out essential steps to conserve the species, such as the establishment of a vaquita refuge in 2005 (Figure 1), which has an area of 1,245.85 km2 and covers the greatest concentration of the species (Diario Oficial de la Federación, 2005). Unfortunately, the refuge’s establishment has not prevented the vaquita population from declining (Jaramillo-Legorreta, 2008; Gerrodette and Rojas-Bracho, 2011). However, potential environmental and trophic changes in its habitat (the UGC) have not been studied to determine how vulnerable the vaquita is to other factors.
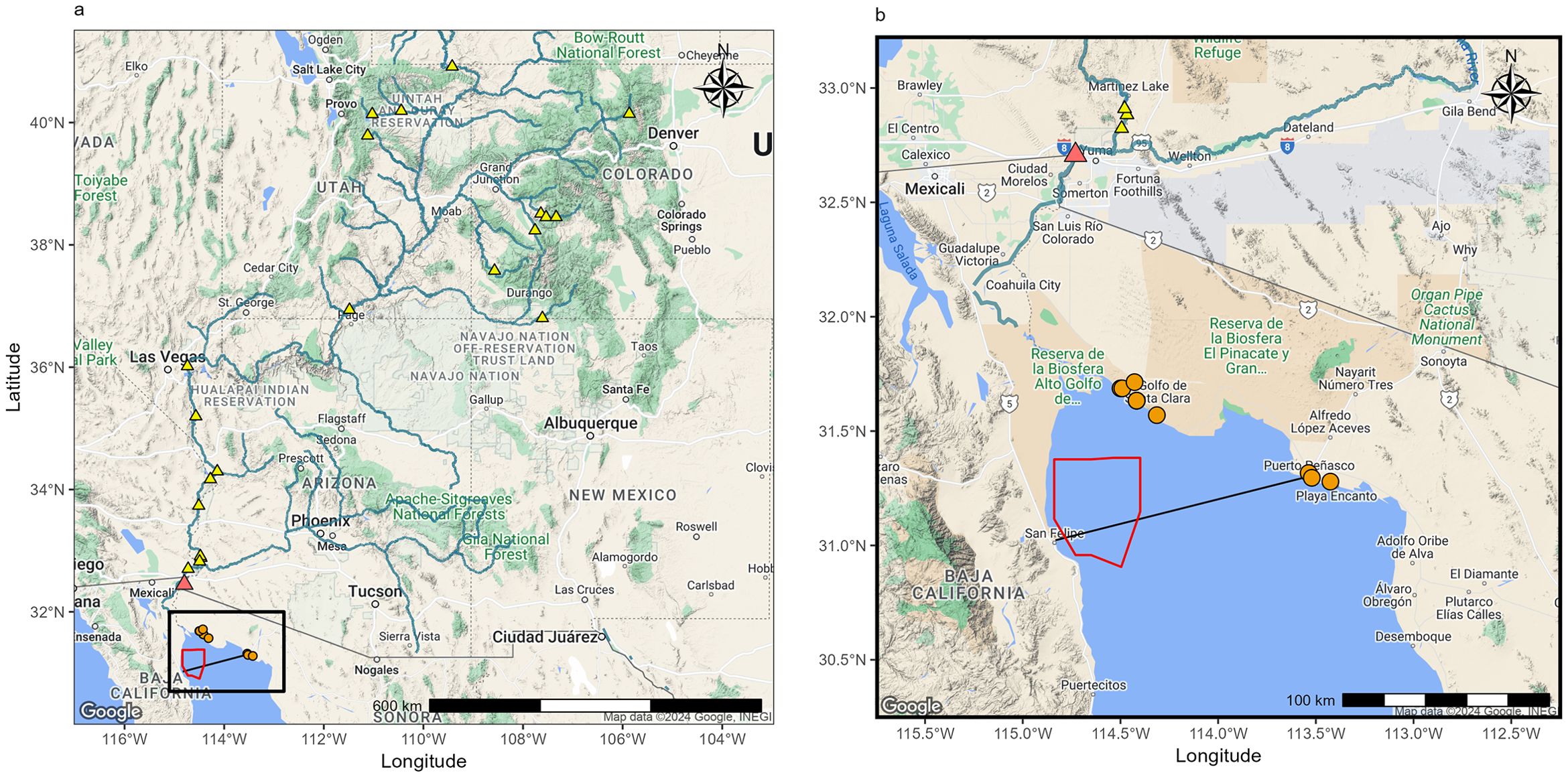
Figure 1. (A) Dams through the Colorado River in the United States (yellow triangles) and the Morelos Dam in Mexico (orange triangles). The black box indicates the location of the Upper Gulf of California; the red polygon indicates the vaquita’s refuge zone (Diario Oficial de la Federación, 2005). (B) Upper Gulf of California, indicating where the vaquita porpoises were recovered (orange circles). The black line indicates the southern limit of the Upper Gulf of California.
Over the past century, the UGC has undergone important environmental changes, mainly generated by the damming of the Colorado River along its course across the United States (20 dams; see Figure 1A) and by the Morelos Dam in the northernmost area of Mexico (Silber and Norris, 1991; Brusca et al., 2017). Between 1910 and 1920, the mean discharge was 21.4 × 109 m3/yr, but by 1952, the freshwater flow decreased to 0.8 × 109 m3/yr (4% of the original discharge; Carbajal et al., 1997). The UGC was transformed from an estuarine system with freshwater influence until San Felipe, B.C., and Puerto Peñasco, Son., to an inverse estuary with salinity values ≥40 psu and temperatures reaching up to ~32 °C in the summer (Álvarez-Borrego et al., 1975; Lavín et al., 1998; Lavín and Sánchez, 1999). After that, an influx of freshwater has occasionally been observed, serving as an approximate reference for the conditions that prevailed when the Colorado River entered the UGC without anthropogenic interruptions (Carbajal et al., 1997; Lavín et al., 1998). For example, between 1980 and 1987, a water release was necessary because of abnormal snow melts in the Upper Basin of the Colorado, with the dams reaching their maximum capacity (Lavín et al., 1998).
A crucial consequence of damming is an alteration in the input of nutrients by cutting the flow of sediments from the Colorado River, which could be reflected in decreases in the region’s turbidity and productivity (Carriquiry and Sánchez, 1999; Rodríguez-Pérez et al., 2018). Although there is little information about changes in the ecosystem, the abundance and distribution of many species could have been modified, including the distribution of the vaquita and its prey (Rodríguez-Pérez et al., 2021, 2023). In view of this, it is crucial to understand what the impact of these changes has been on the vaquita’s trophic relationships, such as the availability and quality of its prey, and the effects of these on its survival.
Considering that the vaquita is a difficult species to observe in the wild due to its small population size, its short and scarce emersions to the surface, and the UGC’s high turbidity (e.g., Rojas-Bracho et al., 2006; Rodriguez-Pérez et al., 2021), alternative research methods are crucial for understanding its habitat use and how this has changed over time. Analysis of stable isotopes of carbon (δ13C) and oxygen (δ18O) of bones emerges as a key tool in this context, enabling us to delve into productivity sources, the foraging habits of organisms, and the trophic interactions between organisms, as well as to quantify the habitat use of cetacean species and their populations over time (Matthews et al., 2016).
The δ13C in aquatic organisms primarily reflects differences in the carbon source of primary producers at the base of the food web (macrophytes, macroalgae, phytoplankton, and chemosynthetic sources) (Gearing et al., 1984; Cifuentes et al., 1988; Fry and Sherr, 1989; France, 1995; Fry and Wainwright, 1991). Thus, if the primary producers present apparent differences, then the δ13C of consumers can be used to infer the animals’ foraging zones, a practical application of stable isotopes in ecological research (Clementz and Koch, 2001). If only a small fractionation between prey and predator depends on the tissue turnover rate, it is possible to obtain information about the trophic route that a consumer follows and evaluate possible changes in it (Clementz and Koch, 2001). The δ18O is an indicator of the degree of utilization of aquatic habitats based on environmental gradients of temperature and salinity (transitions between freshwater, estuarine, coastal, and oceanic environments) (Clementz and Koch, 2001; Trueman et al., 2012; Trueman and Glew, 2019; Drago et al., 2020).
The main factor influencing the isotopic enrichment between consumers’ bones and the source (prey) is associated with tissue turnover rates (Schoeninger and DeNiro, 1984). Bone has low turnover rates and represents a long-term isotopic bio-archive record of animals’ diet and habitat biochemistry (Newsome et al., 2010). However, Smith et al. (2020) found relatively slow tissue turnover in the intraskeletal bone tissue of porpoises, even in immature animals. In other groups, such as pinnipeds for instance, the skull bone contains information about the assimilated diet for a period of months in juveniles and for a period of up to five years in mature animals (Sealy et al., 1995; Newsome et al., 2006; Riofrío-Lazo et al., 2013). If, as Hohn et al. (1996) mentioned, the vaquita reaches maturity at three years of age, and the bone turnover rate is as low as in other porpoises, we would have information from several years of the animals analyzed.
As described above, variations in δ13C and δ18O occur for different productivity sources and for different ecosystems that present clear environmental gradients. Secondly, the Colorado River presented sporadic but important water releases to the UGC between 1983 and 1987, which offered a partial insight into the estuarine conditions before the damming. Based on these two facts, we expected to find significant differences in the isotopic signals between 1983 and 1987 relating to the increase in flow from the Colorado River and the consequential change in temperature and salinity in 1988–1993. Therefore, this study aimed to analyze the values of δ13C and δ18O in vaquita bones collected between 1983 and 1993 to find signals of changes in (i) the carbon source of primary producers and the trophic structure and (ii) the degree of habitat use based on salinity and temperature gradients. Both these aspects were examined in relation to the reduction in the flow of the Colorado River over time.
2 Method
2.1 Sampling
δ13C and δ18O values were obtained from vaquita bone samples from the inner dorsal section of the skull of 13 specimens from the Vertebrate Collection at the Centro de Investigación en Alimentación y Desarrollo (CIAD-Guaymas, Sonora) and the Mammal Collection at the Instituto de Biología-Universidad Nacional Autónoma de México (IB-UNAM). These specimens were collected from the Gulf of Santa Clara, Son., to Puerto Peñasco, Son., from 1983 to 1993 (Figure 1B; Table 1).
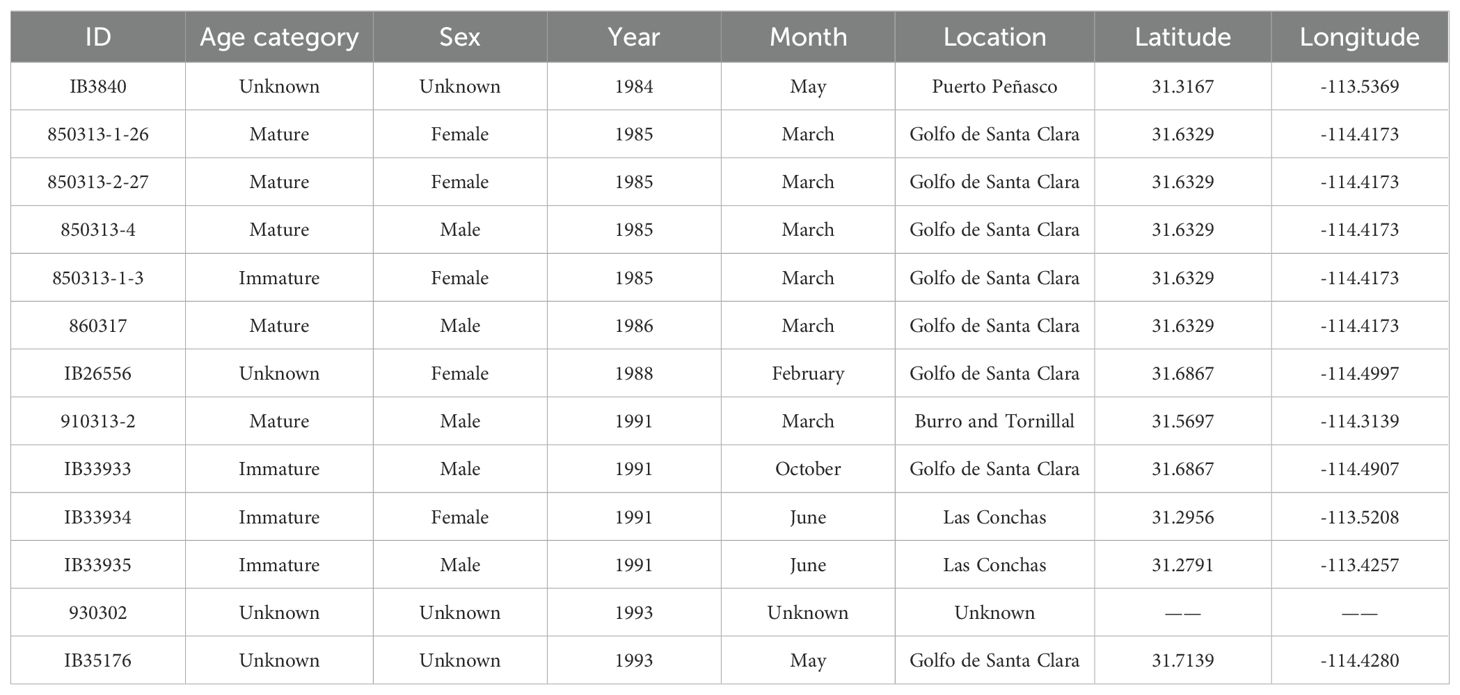
Table 1. Data of sampled specimens. The year and month refer to the date the vaquita remains were recovered.
The bone fragments were ground, and no pretreatment was applied because many studies show that pretreatment can affect δ18O values (Garvie-Lok et al., 2004; Pellegrini and Snoeck, 2016; Snoeck and Pellegrini, 2015). A minimum weight of 1 mg of each bone sample was collected using a high-precision drill Micro-Mill System at the Marine Mammals Laboratory, IPN-CICIMAR, La Paz, Baja California Sur, México.
2.2 Stable isotope analysis
The stable isotope analysis in bone was conducted at the University of California Davis Stable Isotope Laboratory (https://stableisotopefacility.ucdavis.edu/) using a Thermo Scientific GasBench II coupled to a Thermo Finnigan Delta Plus XL isotope-ratio mass spectrometer. These were equilibrated for 24 h at 30°C. The resulting data were normalized using commercially available laboratory reference materials (USGS-44, NBS-18, NBS-19, and LSVEC) calibrated to international standard calcium carbonate of Vienna Pee Dee Belemnite (V-PDB), with a standard deviation of ± 0.10‰ for δ13C and ± 0.15‰ for δ18O. Results are expressed as δ-values using the equation δ = [(18O/16O or 13C/12C) sample/(18O/16O or 13C/12C)standard) - 1] × 1000. Delta (δ) units are reported as parts per thousand (‰).
In marine mammal studies, the δ18O values are more commonly presented relative to the Vienna Standard Mean Oceanic Water (V-SMOW) index. In the present study, for comparative purposes, the δ18O values in vaquitas were converted from PDB to SMOW according to the following equation (Koch et al., 1997):
δ18O (SMOW) = [δ18O (PDB) × 1.03086] + 30.86
2.3 Environmental variables
In order to eliminate the seasonal signal in the variables, we estimated the monthly anomalies of the time-series data for sea surface temperature (SST) and sea surface salinity (SSS) utilizing daily composites from 1983 to 1993. We obtained SST and SSS measurements for the Upper Gulf of California (UGC), delineated to the south by San Felipe (Baja California) and Puerto Peñasco (Sonora) (Figure 1B). An anomaly was the deviation between the recorded environmental variable at a specific period and the long-term average of the same environmental variable for the same period and region. A high-resolution SST analysis was developed by NOAA using an optimal interpolation (OISST), with a spatial grid resolution of 0.25 degrees and a temporal resolution of 1 day (Huang et al., 2020). SST values were obtained from the Met Office Marine Data Bank (MDB), while SSS values were extracted from ESA MIRAS SMOS Level-2 swath Network Common Data Format (NetCDF) files (currently V6.62). These environmental data were obtained via ERDDAP (Environmental Research Division’s Data Access Program) (Simons, 2019) using the “rerddap” v1.0.4 and “rerddapXtracto” v1.1.7 packages in R (Chamberlain and Boettiger, 2017; R Core Team, 2023). Additionally, we obtained a record of the average monthly inflow of the Colorado River (FLX) from the International Boundary and Water Commission (http://www.cila.gob.mx/).
2.4 Data analysis
To evaluate possible changes in the carbon source of primary producers and the vaquita’s diet changes in relation to the variability in the flow of the Colorado River (Figure 2C), two periods were previously defined: (i) from 1982 to 1988, when freshwater arrived in the UGC and seven vaquita bone samples were collected, and (ii) from 1989 to 1993, when the river water stopped entering and six vaquita bone samples were collected.
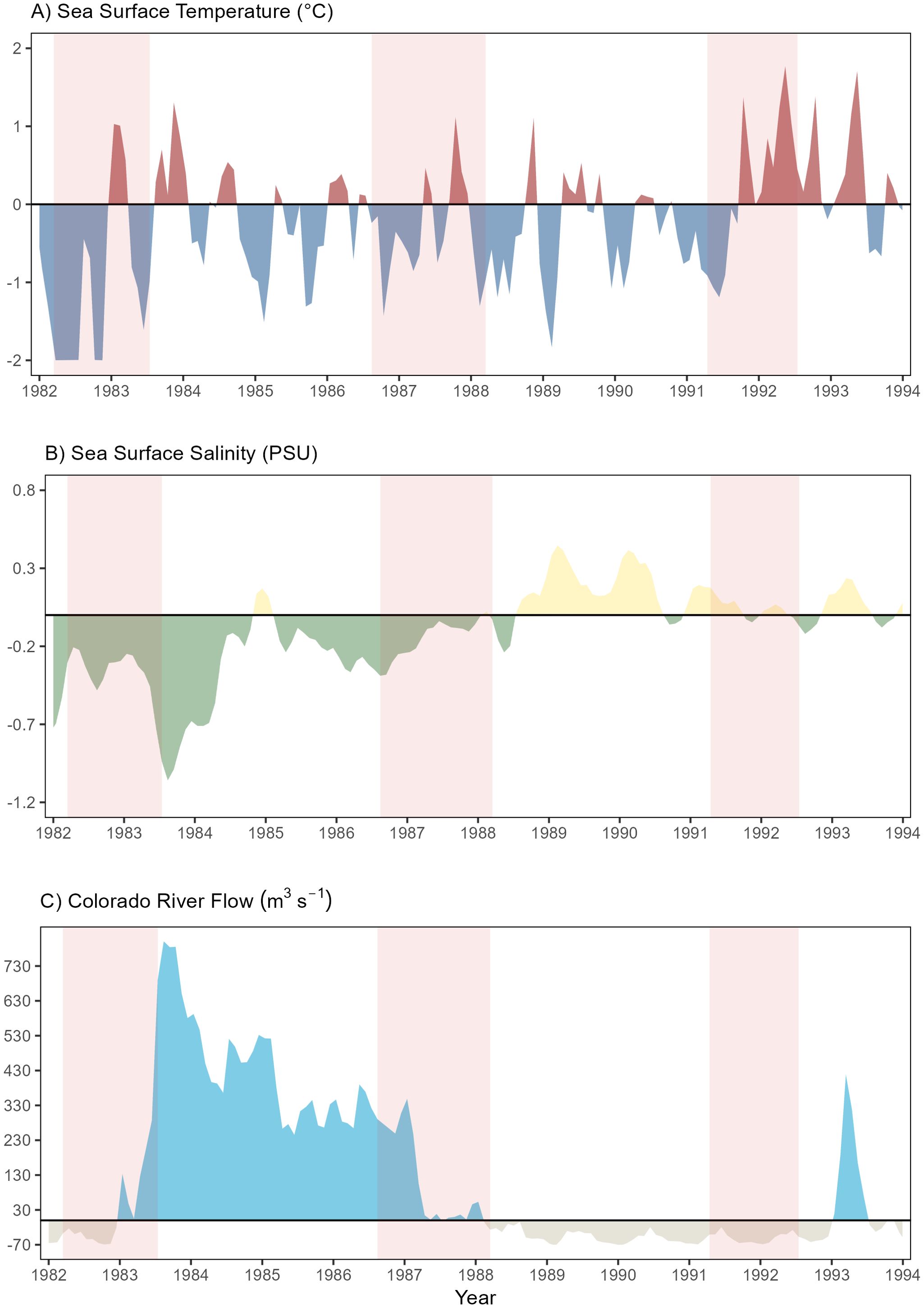
Figure 2. Annual average time-series anomalies in the Upper Gulf of California from 1983 to 1993. (A) Sea surface temperature (°C), (B) sea surface salinity (psu), (C) Colorado River flux (m3 s-1).
In order to determine the possible correlation between the environmental variables, the normality of the data was assessed with a Shapiro test based on annual average values derived from monthly composites spanning from 1980 to 2010. Then we conducted a Spearman correlation test, with p-values below the significance threshold of 0.05, suggesting a non-normal distribution.
To test the existence of significant differences between the two periods and age categories, the datasets of δ13C and δ18O bone values were tested separately for normality using Kolmogorov–Smirnov (K–S) tests and assessed for homogeneity of variances with a Levene’s test. Because the datasets were not normally distributed, a Mann–Whitney U test was used. Statistical analyses were conducted using IBM SPSS Statistics v22.
To explore the pattern of δ13C and δ18O data and its relationship with the groups defined based on the flow of the Colorado River and the changes in salinity and temperature that they present, a principal component analysis (PCA) was carried out. Overall, to statistically confirm the link between the dependent variable (stable isotope) and the independent variables (SST, SST, FLX), a generalized linear model (GLM) was used. This model was the most robust for small sample sizes and non-normally distributed variables. To enhance the stability of the model results, we removed the random effect and employed a fixed-effects model. The FLX variable was standardized because it had a much higher significance value than the other variables. Also, we employed Akaike information criterion (AIC) weights to select the optimal model based on reduced AIC scores (Akaike, 1998; Burnham and Anderson, 2002). The delta-AIC (ΔAIC) and AIC weight (AICw) were used as evaluation metrics to rank the models. The AIC quantifies the difference in AIC scores between the best model and the model under assessment. On the other hand, the AIC weight, also known as the AICc weight, represents the proportion of predictive power that the evaluated model contributes to the complete collection of models (Akaike 1998). We generated the models using the glm function from the “Ime4” version 1.1-35 package (Bates et al., 2015) in the R programming language and evaluated the models using the “AICcmodavg” version 2.3-2 package in R (Mazerolle, 2023; R Core Team, 2023).
From satellite data, an SST/SSS diagram was built for each dataset (from 1982 to 1988 and from 1989 to 1993) to determine signs of change in the UGC’s water mass.
Finally, boxplots of the original values of each stable isotope (δ13C and δ18O) were built to compare the average values and ranges of bone (Drago et al., 2020) and tooth (Clementz and Koch, 2001) values of marine mammals from different ecosystems, compiled from the literature with respect to the vaquita as a hypersaline habitat species.
3 Results
3.1 Thermic, saline, and Colorado River inflow changes in the UGC
The Spearman correlation showed a non-normal distribution (p < 0.05). The p-values between SST and SSS were not significant (Appendix 1). The association between Colorado River flow and SSS was significant, although the correlation coefficient was quite low.
To determine whether the El Niño event influenced the pulses of water released into the UGC and, consequently, the environmental variability, a red polygon was incorporated into Figure 2 to denote the months with documented warm periods, with a threshold of ± 0.5°C for the Oceanic Nino Index (ONI; https://origin.cpc.ncep.noaa.gov/products/analysis_monitoring/ensostuff/ONI_v5.php). The ONI did not directly influence the environmental variables. The SST had negative anomalies during warm events, indicating a temperature decrease relative to previous years.
Temperature anomalies exhibited interannual variations during the study period (from 1983 to 1993). However, a range of negative anomalies (from -0.5 to -1.5°C) was observed between 1983 and 1991 (Figure 2A). During the later years of the time series (from 1992 to 1993), the highest positive anomalies (from 0.5 to 1.5°C) were observed.
Salinity anomalies showed a clear regime shift from negative to positive anomalies (Figure 2B). Negative salinity anomalies (from -0.1 to -1 psu) dominated from 1982 to 1988, with the highest negative values (-1 psu) in 1983. After 1988, a predominance of positive anomalies (from 0.1 to 0.5 psu) was observed until 1993, with a maximum value of +0.5 psu in 1989.
The Colorado River’s flux displayed the inverse of the salinity pattern (Figure 2C), with high positive anomalies (from 130 to 730 m3 s-1) between 1982 and 1988. The highest value (730 m3 s-1) was observed in 1983. From 1989, negative anomalies (< -70 m3 s-1) were observed until 1993. A positive anomaly peak (430 m3 s-1) was present in 1993.
The low salinity values (~< 34.5 psu) in the period from the end of 1983 to 1987 were aligned with high flow (~> 250 m3 s-1) from the Colorado River into the UGC. In contrast, the increase in salinity in the 1990s (~> 34.5 psu) corresponded with a decrease in the flow of the Colorado River (~< 250 m3 s-1), except in 1993, when an increment of water from the river was present (Figure 3).
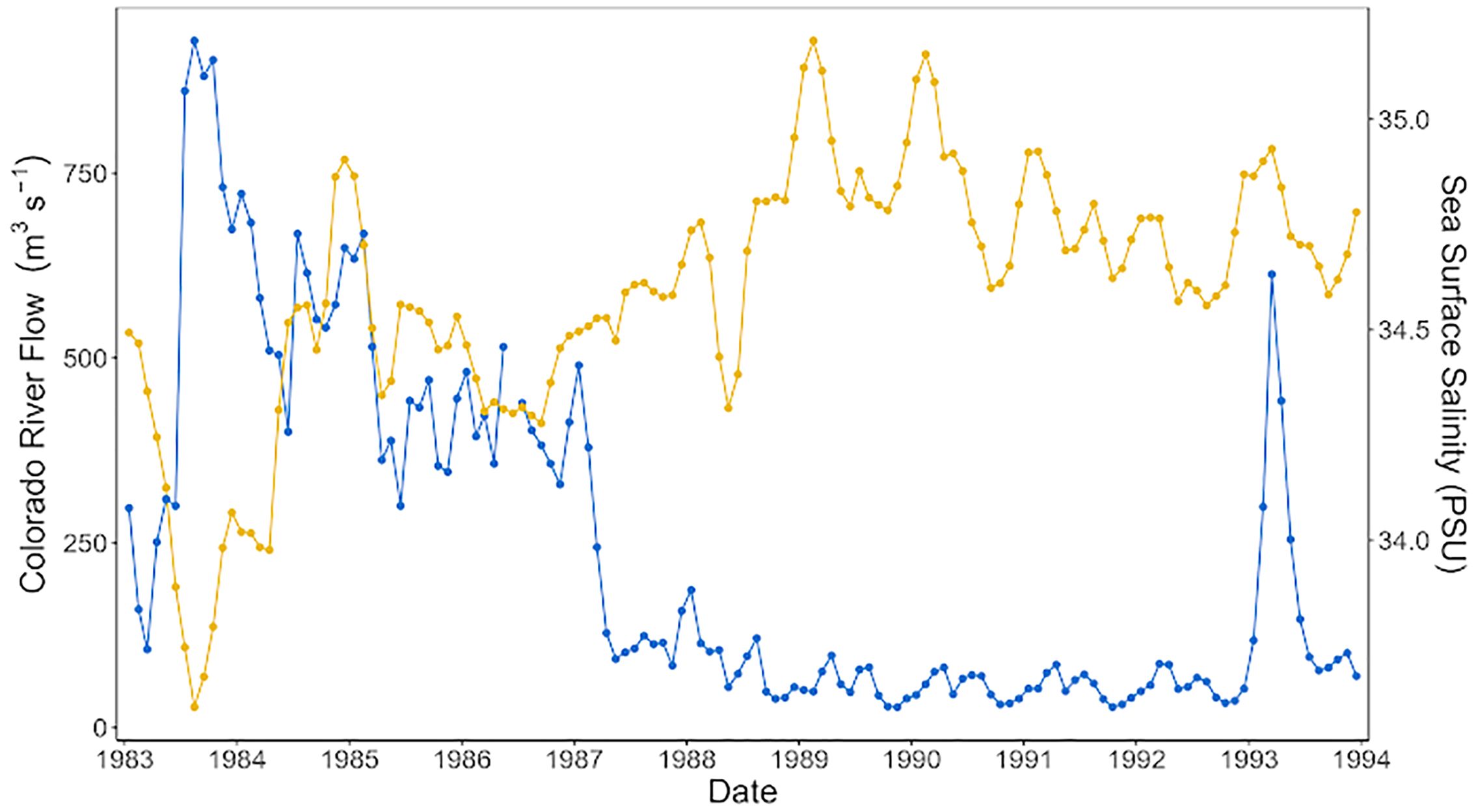
Figure 3. Annual average time-series comparison between sea surface salinity (orange line) and Colorado River flow (blue line) in the Upper Gulf of California from 1983 to 1993.
Based on the two datasets (from 1982 to 1988 and from 1989 to 1993), it was tested whether there were significant differences in each variable described above (Table 2). There were no significant differences between the two datasets for SST (t = -1.947, gl = 70, p = 0.056), but there were significant differences for SSS (t = -6.940, gl = 70, p < 0.05) and Colorado River inflow (U = 174.000, Z = -4.741, p < 0.05).

Table 2. Mean and standard deviation of isotopic data and anomalies of environmental values between the two datasets, from 1982 to 1988 and from 1989 to 1993.
Each dataset’s SST/SSS diagrams showed different water masses, based mainly on the salinity values (Figure 4). From 1982 to 1988, the water mass (blue area) displayed temperature values from ~16 to 24°C, with an average value of 19.4°C, and salinity values from 33.8 to 35 psu, with an average value of 34.4 psu. From 1989 to 1993, the water mass (yellow area) had shorter intervals than in the previous period. It showed temperature values between ~15 and 24°C, with an average of 19.7°C, and salinity values between 34.8 and 35.4 psu, with an average of 34.8 psu.
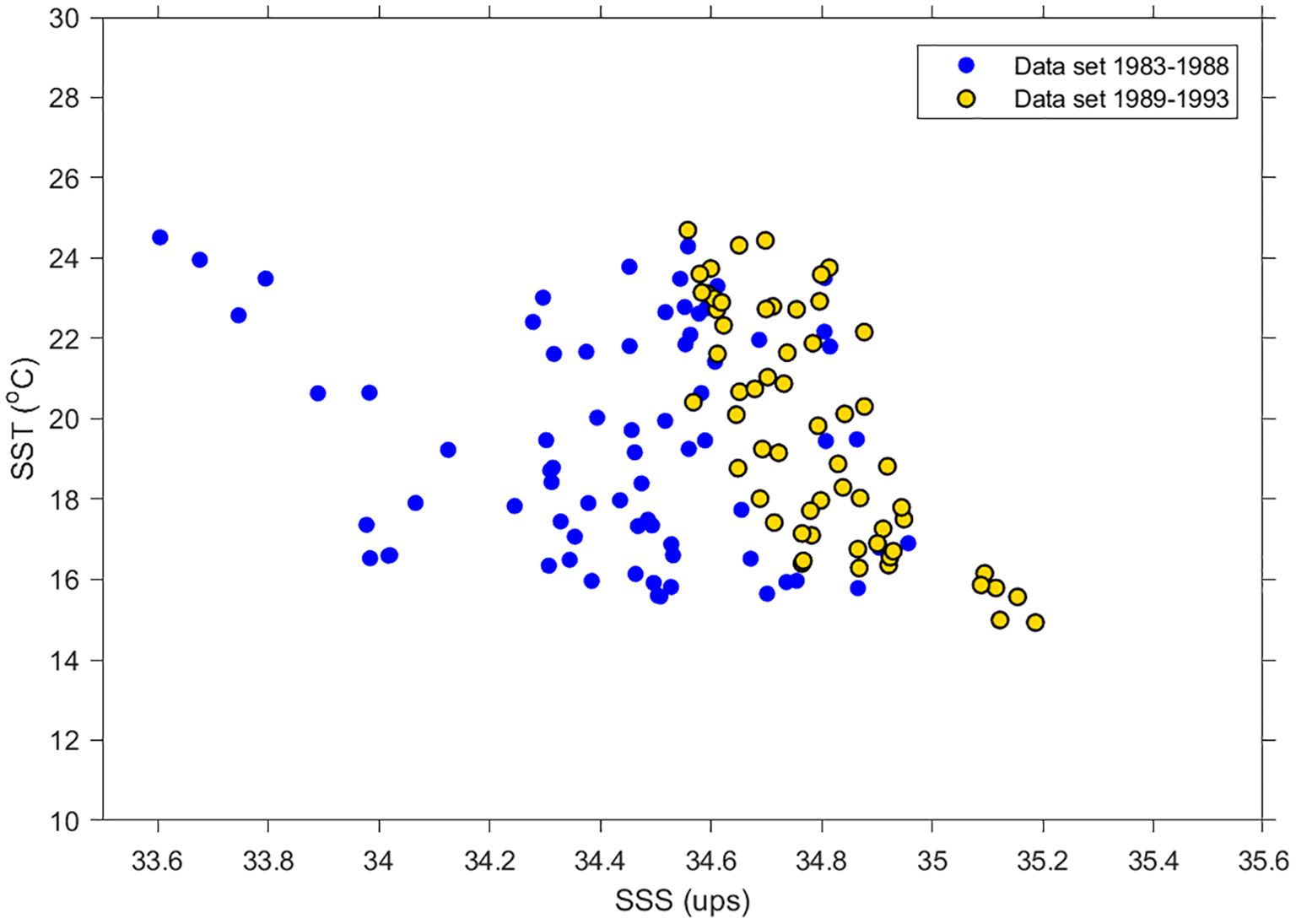
Figure 4. SST/SSS diagrams of the Upper Gulf of California during two periods: from 1982 to 1988 (blue) and from 1989 to 1993 (orange).
3.2 Isotopes (δ13C, δ18O) from vaquita bone and environmental variables
The hypothesis test showed significant differences for δ13C (U = 144.000, Z = -5.233, p < 0.05) but not for δ18O (U = 432.000, Z = -1.744, p = 0.081) between the two datasets (1983–1988 vs. 1989–1993). Richer δ13C values were found for the earlier period (δ13C = -9.1 ± 1.3‰) compared with the later period (δ13C = -10.8 ± 2.1‰). In the case of oxygen, the values of the two groups were very similar, from 1983 to 1988 (δ18O = 30.5 ± 0.4‰) and from 1989 to 1993 (δ18O = 30.4 ± 0.6‰) (Table 2).
Also, there were significant differences for δ13C (U = 1.000, Z = -2.205, p < 0.032) but not for δ18O (U = 8.000, Z = -0.490, p = 0.730) between the two age categories (mature and immature). Richer δ13C values were found for mature animals (δ13C = -8.7 ± 0.8‰) and lower values for immature animals (δ13C = -11.7 ± 2.0‰). In the case of oxygen, the values of the two groups were also similar, but the richest values were found in immature animals (δ18O = 30.6 ± 0.7‰) compared with mature animals (δ18O = 30.3 ± 0.2‰).
The PCA based on the average values of each variable per dataset showed that the determinate values (0.047) had no collinearity among the variables, reinforcing the accuracy of our analysis.
According to the PCA, the first two components explained 81% of the variance (Table 3). The two datasets are superimposed over the PCA biplot (Figure 5). The dataset from 1982–1988 (blue circles and ellipse) is located over the right quadrants (superior and inferior), but most of the data are close to the X-axis, as the centromere indicates (the largest circle of the group). This dataset is associated with the Colorado River inflow and δ13C vectors and has most values in the right quadrants.
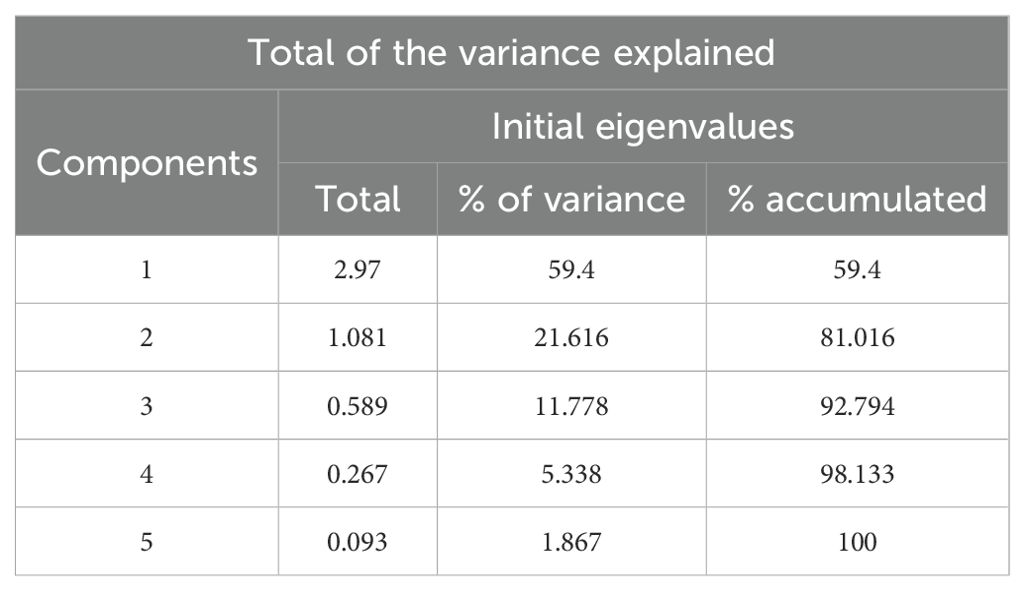
Table 3. Correlation matrix and eigenvalues and variance explained by the principal component analysis of the stable oxygen and carbon isotopes of vaquita skull bones and environmental variables.
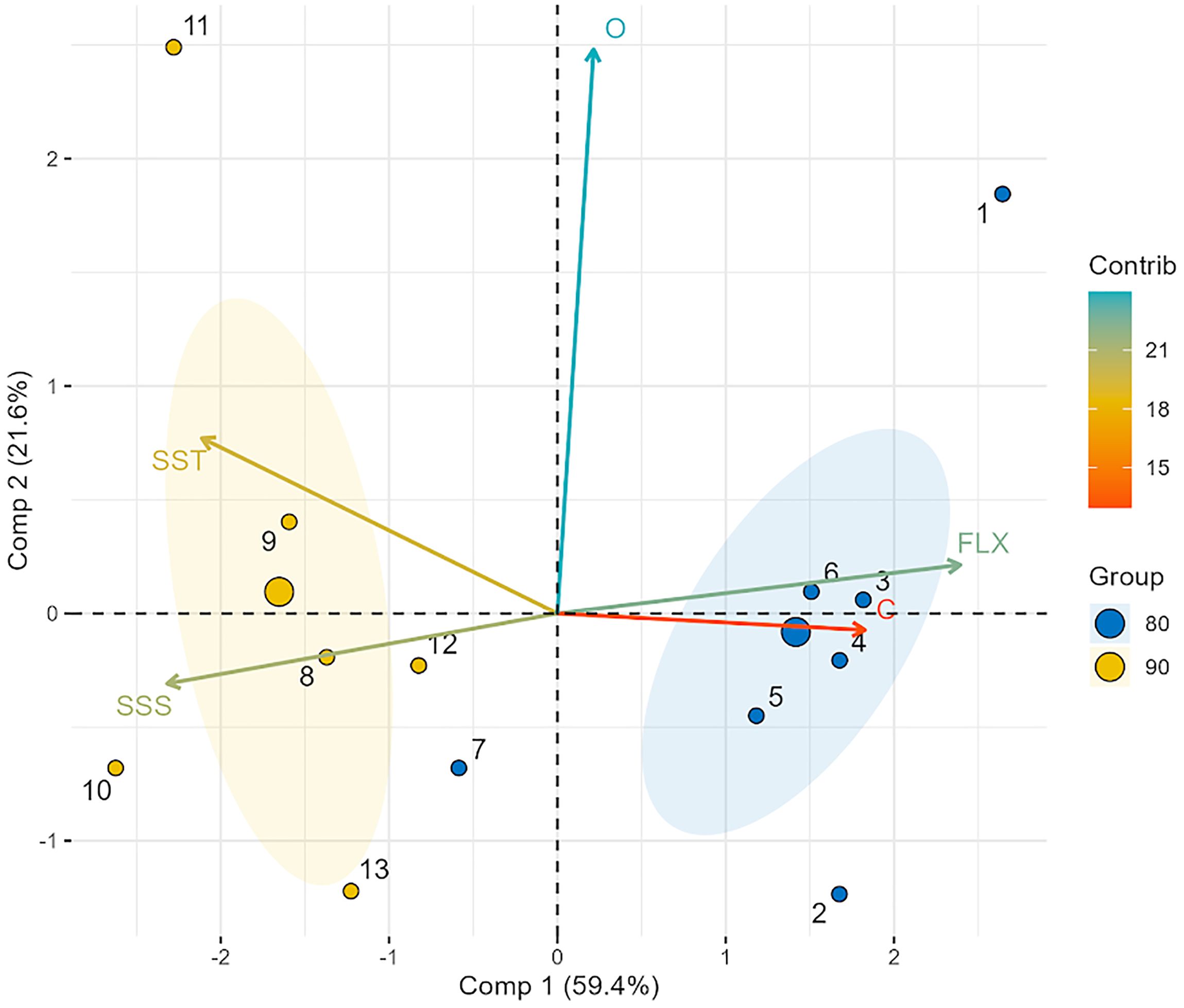
Figure 5. Principal component analysis between stable oxygen and carbon isotopes of vaquita skull bones and environment variables. Sea surface temperature (SST), sea surface salinity (SSS), and Colorado River inflow. The blue circles and ellipse represent the dataset from 1982 to 1988. The orange circles and ellipse represent the dataset from 1989 to 1993. For both datasets, the largest circles represent the centromere. The length of the vectors is the relative importance of each variable in the analysis.
The dataset from 1989–1993 (yellow ellipse and circles) is located in the left quadrants, and most of the data are close to the X-axis, as indicated by the centromere (the largest circle of the group). This dataset is related to the SST and SSS vectors and has most values in the left quadrants.
One data point from each set is associated with the δ18O vector. These two data points are located outside the ellipses in the upper part of the quadrants.
The GLM results broadly support the PCA predictions. The best model produced by the GLM to explain the behavior of δ13C was the Colorado River flow, with an intercept value of 1.17e-14 and a p-value of 0.0209. The best model to explain the behavior of δ18O was SSS, with an intercept value of 2.0e-16 and a p-value of 0.593 (Table 4).
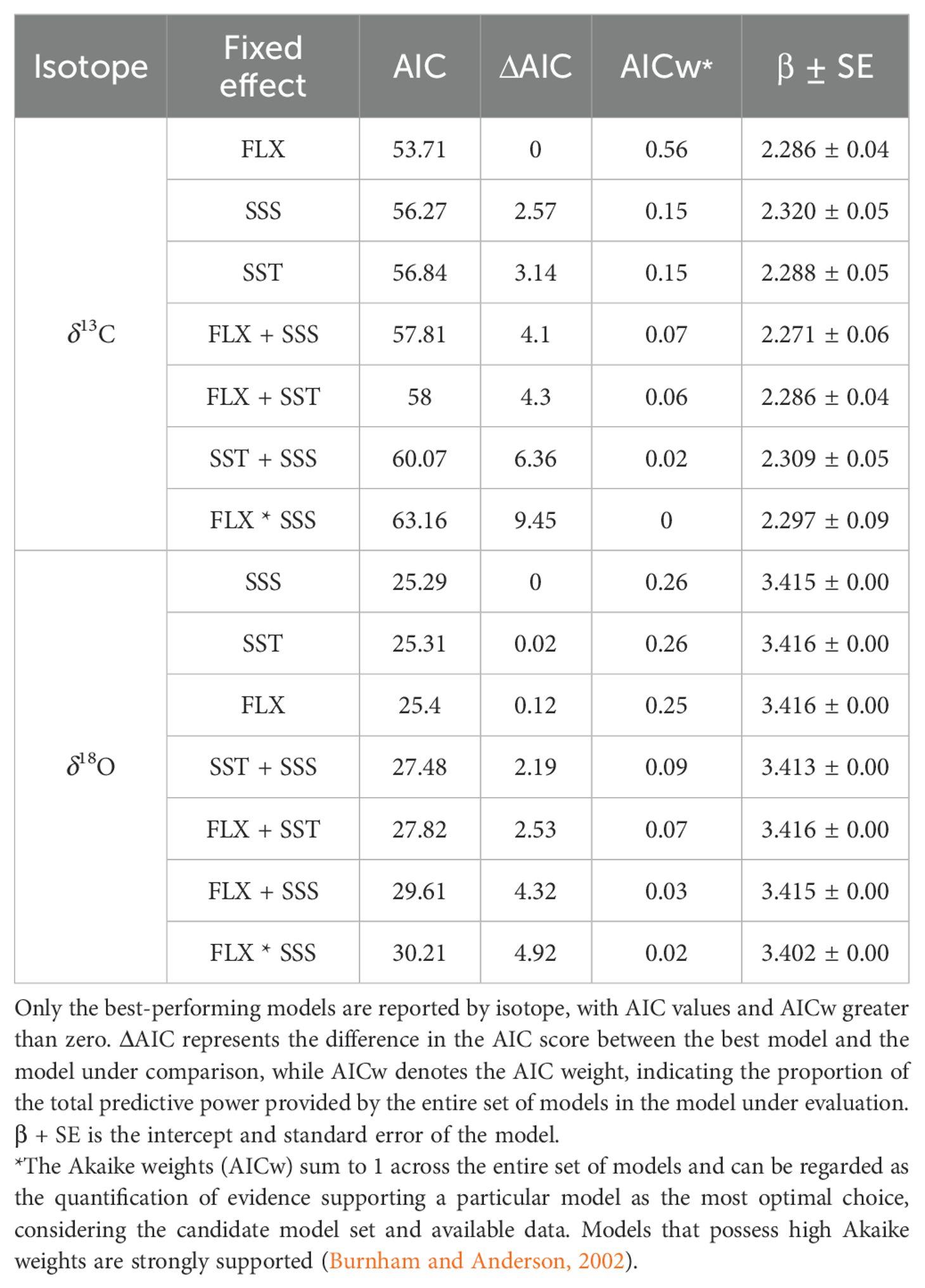
Table 4. Ranking of GLMs using AIC values for stable isotopes (family Gamma) and environmental variables (SST, sea surface temperature; SSS, sea surface salinity; FLX, Colorado River flow).
3.3 Comparison with other marine mammals
The bone and tooth δ13C and δ18O values of marine mammals in different habitats, as obtained from the literature, were compared with those obtained from the vaquita in this study (Table 5). The δ13C values in coastal mammal species ranged from ~-9 to -11‰. The vaquita presented a mean value of δ13C = -9.1 ± 1.3‰ at the end of the 1980s and a mean value of -10.8 ± 2.1‰ at the beginning of the 1990s. Both values are inside the range of the other coastal mammals. Extreme values were observed in otter species: Enhydra lutris, an otter species that inhabits kelp fields, showed a clear enrichment (-6.1 ± 0.9‰), and Lontra canadensis, an otter species of rivers, displayed an impoverishment (–17.3 ± 4.3‰) (Clementz and Koch, 2001; Drago et al., 2020).
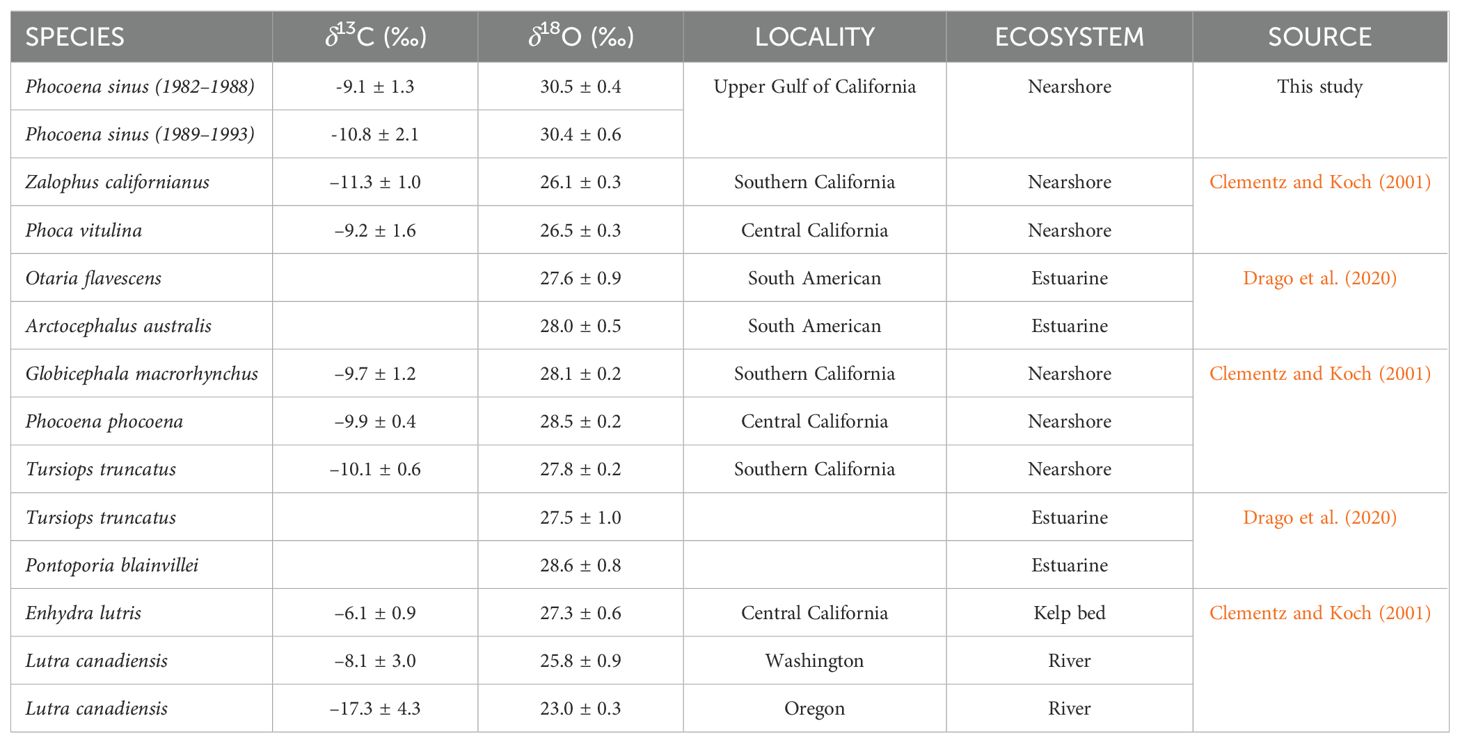
Table 5. Mean and standard deviation of enamel (Clementz and Koch, 2001) and bone (Drago et al., 2020) δ13C and δ18O stable isotope values from different aquatic mammals; and the skull bone of vaquitas.
In the case of δ18O, the most enriched values were found in estuarine species (~27–28‰), followed by coastal species (~26–28‰), and the most impoverished values were detected in otter species that inhabit rivers (~23–26‰). The average values of δ18O in vaquitas were the most enriched, with mean values of 30.5 ± 0.4‰ and 30.4 ± 0.6‰, respectively, for each period defined here To complement the analysis above, the vaquita isotopic values and the values of the coastal and estuarine species considered in Table 4 were compared in a plot (Figure 6). It shows the distance between the vaquita δ13C values and those of the other species in both periods (1.7‰), which was higher than that between species such as Phocoena phocoena (white square), Tursiops truncatus (black circle), and Globicephala macrorhynchus (black circle) (< 0.03‰). On the other hand, even though there was no difference in vaquita δ18O values between the two periods, the distance from the values of the rest of the plotted species was > 2‰.
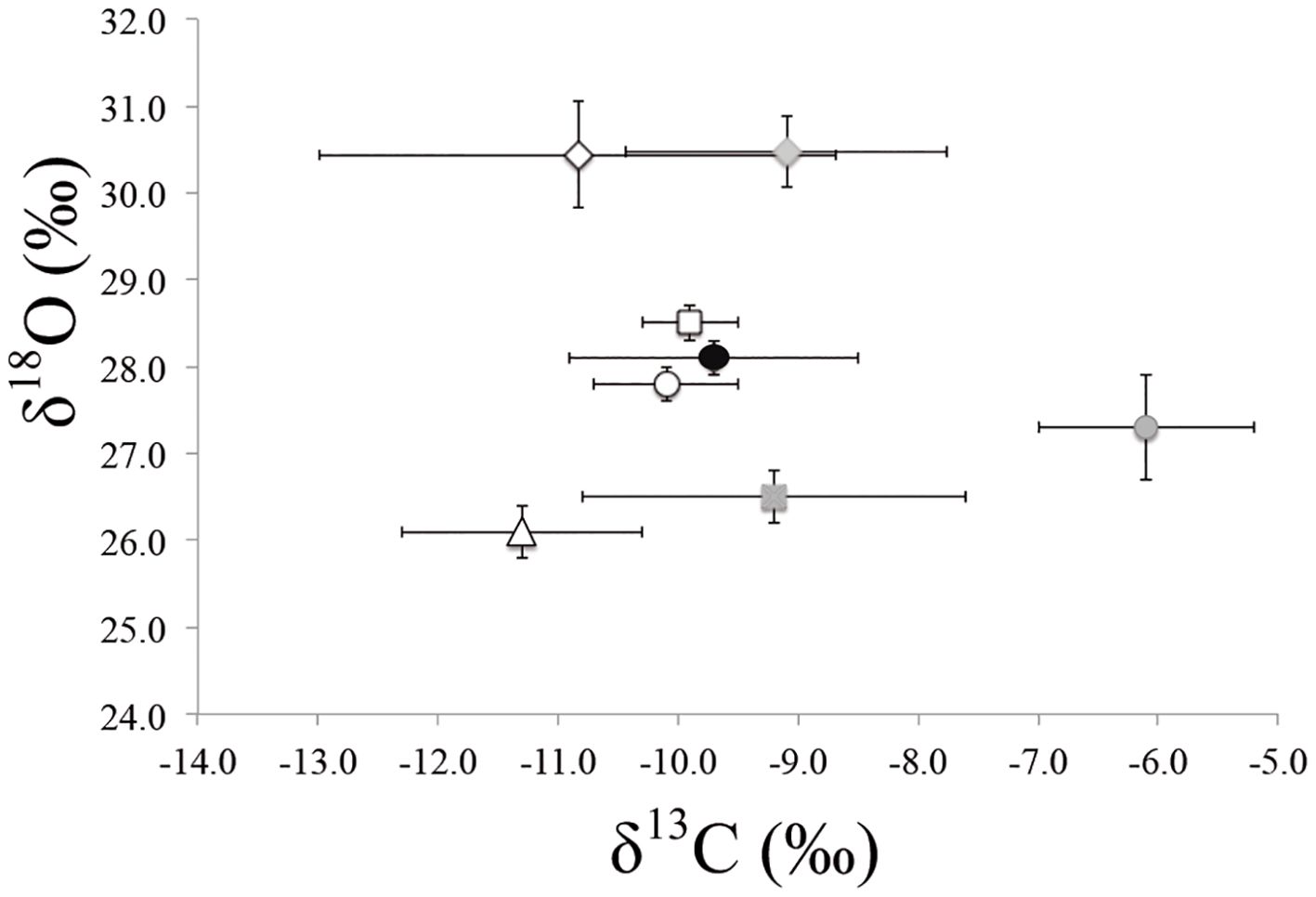
Figure 6. Mean and standard deviation of δ13C and δ18O of different aquatic mammal species. Grey (1982–1988) and white (1989–1993) diamonds: vaquita; white square: Phocoena phocoena; grey square: Phoca vitulina; white triangle: Zalophus californianus; black circle: Globicephala macrorhynchus; white circle: Tursiops truncatus; grey circle: Enhydra lutris.
4 Discussion
4.1 Thermic, saline, and Colorado River inflow changes in the UGC
Considering that the vaquita, P. sinus, an endemic species of the UGC, is classified as critically endangered by the Mexican government and is on the IUCN Red List (NOM-059-SEMARNAT-2010, Diario Oficial de la Federación (2010), https://iucn-csg.org/wp-content/uploads/2023/06/Vaquita-Survey-2023-Main-Report.pdf), this study analyzed signals of the damming of the Colorado River across U.S. territory and the Morelos Dam in Mexico in the vaquita’s habitat, using satellite data and stable isotopes of carbon (δ13C) and oxygen (δ18O) from vaquita bones.
In the study period (from 1983 to 1993), in the context of the evolution of the UGC from an estuarine system to an inverse estuary (Álvarez-Borrego et al., 1975; Lavín et al., 1998; Lavín and Sánchez, 1999), the UGC was in the latter condition. However, a big influx of freshwater arrived in the UGC between ~1980 and 1988 because of abnormal snow melts in the Upper Colorado Basin, causing the dams to reach their maximum capacity (Lavín et al., 1998) and allowing us to compare two different conditions.
Based on the drastic change in the Colorado River’s flow toward the UGC, both periods were statistically identified using SSS and SST variation. These results were supported by SST/SSS diagrams showing two water masses inside a salinity gradient from 33.8 to 35.2 psu (Figure 4). The water mass from the end of the 1980s had an average salinity of 34.4 psu, while the water mass detected at the beginning of the 1990s had an average salinity of 34.8 psu. Although the diagrams were built with satellite data, and without in situ data, a temporal change was observed in the water mass, demonstrating that variations in the Colorado River’s flow could have modified the vaquita’s habitat. According to Wright and Colling (1995), an average difference of 0.4 psu in salinity is a significative change in water mass properties.
Sometimes, marked hypersaline conditions develop in estuaries, exposing the primary producers to high osmotic stress (Kinne 1964), and the change in productivity can reduce an ecosystem’s diversity and alter trophic levels (Reist et al., 2006; Sora et al., 2024). Thus, when hypersalinity increases, the diversity decreases, sometimes resulting in mass mortalities and even the local extinction of flora and fauna (Molony and Parry, 2006; Kim et al., 2013; Tweedley et al., 2019). In the case of the UGC, it has been proven in various faunal groups such as fish, shrimp, and clams that the flow of the Colorado River modulates both their breeding areas and their life cycles, negatively impacting the species as salinity and temperature increase (Kowalewski et al., 2000; Rodriguez et al., 2001; Rowell et al., 2005; Rowell et al., 2008; Lozano-Montes et al., 2008; Galindo-Bect et al., 2021). Even a decrease in biomass and diversity of fish larvae has been observed in the most saline larval habitats within the UGC (Sánchez-Velasco et al., 2012). In this context, we could assume that the vaquita has been affected by changes in environmental conditions caused by fluctuations in river flow, at least indirectly through a decrease in prey availability.
4.2 δ13C from vaquita bones
Carbon isotope values (δ13C) significantly differed between periods, with a mean value of -9.1‰ at the end of the 1980s and a mean value of -10.8‰ at the beginning of the 1990s (Table 2). These were interpreted as indicators of variable carbon sources between both periods. When the river’s flow is blocked, the nutrient source also decreases, giving rise to primary producers with depleted 13C values. This is likely because the low growth rate of phytoplankton allows greater discrimination of the heavy isotope as they do not reproduce fast enough to exhaust the 12C in the medium. In contrast, when the flow toward the delta region is active, organic matter would be expected to enter from the marshes, leading to higher concentrations of nutrients and giving rise to rapid phytoplanktonic reproduction in a short period of time (phytoplanktonic bloom). Due to its high reproductive rate and different carbon sources, the phytoplankton would be enriched in 13C (Fry and Sherr, 1989), leading to bioaccumulation along the trophic web (Miller et al., 2008). This agrees with the results obtained by the PCA (Figure 5), in which a positive association was observed between the δ13C isotopes and the flow of the Colorado River during the first period analyzed.
On the other hand, it has been observed that isotopic values in primary producers in estuarine environments are influenced by saline concentration, with higher values in areas of lower salinity (Fogel et al., 1992; Brutemark et al., 2009), as reported in this study (Table 2). In addition to salinity differences, this is probably related to the entry of freshwater that carries organic matter enriched in 13C from primary producers with higher isotopic values, such as macrophytes in the marsh area. This phenomenon has been observed in sedimentary organic carbon in nearshore environments with inputs of organic matter from macrophytes to the system and values between -9.8‰ and -14.0‰ (Fry et al., 1977; France, 1995). Another explanation for the differences in the δ13C in both periods may be a change in the available prey. Suppose the δ13C isotopic values show only a small difference between diet and consumer due to isotopic fractionation (~1‰ enrichment with each trophic step) (Clementz and Koch, 2001). In that case, it is reasonable to assume that during the period of greater input from the Colorado River, the vaquita had access to prey at a higher trophic level than during the period of no input from the Colorado River.
It is essential to mention that differences between age categories may affect δ13C isotopes since a difference of 3.0‰ was found between both categories. Previous studies have reported differences, although not significant, in the prey captured by adult animals (predominance of demersal and benthic organisms) and immature animals, which tend to feed on smaller pelagic prey (Findley et al., 1994). In addition to a greater niche breadth, a greater variability assessed by δ15N is observed in immature animals than in mature animals, suggesting that mature animals had a more selective feeding spectrum with probably higher trophic levels (Rodríguez-Pérez et al., 2021). Given that most mature specimens belonged to the 1980s, it is also essential to consider that the differences in isotopic signals between periods were possibly due to this factor.
4.3 δ18O in vaquitas and other aquatic mammals
The oxygen (δ18O) values were not significantly different between periods or age categories. This similarity may be due to the turnover rate of bone tissue, which stores information from long periods (Hedges et al., 2004), possibly making it impossible to separate the periods in the case of δ18O.
The comparisons of marine mammals were made in different tissues. Those taken from Clementz and Koch (2001) came from dental enamel, which includes the isotopic composition of the mother’s diet and body water, plus any fractionations associated with diffusion across the placenta, nursing, and the rapid growth of the young animal, and bone, which also offers a long-term record of the life history of an animal and includes the last years or probably months in immature specimens. Despite the above, since both tissues come from apatite, the isotope fractionations for water to carbonate are roughly equal for the different materials (Clementz and Koch, 2001), so we consider the presented comparison viable.
Conversely, the δ18O values in vaquitas differed from those of other aquatic mammals (Figure 6) in previous studies (Clementz and Koch, 2001; Drago et al., 2020). It is important to note that our study focused on coastal/estuarine or freshwater specimens (Table 4). These high values are in line with Drago et al. (2020), who found that isotopic values increase in environments where aquatic mammals live in more saline conditions. As a result, freshwater or estuarine organisms record lower values (Table 4) than oceanic organisms (~> 30.0‰; Drago et al., 2020). The δ18O isotope was not associated with any of the environmental variables analyzed in this study, as was observed in the PCA.
Although it was not possible to demonstrate changes in the vaquita’s habitat use by δ18O values, changes in the environment and in the trophic source of the primary producers were evident. This is significant because our hypothesis is that the vaquita’s enrichment, which is similar to that of other groups in the same habitat referred to as the VPDB, may result from the high evaporation rate and salinity in the UGC. This enrichment in clams and fish has been related to a population decline in species such as Mulinia coloradoensis, Cynoscion othonopterus, and Totoaba macdonaldi and has been attributed to high temperatures due to environmental change caused by the blocking of the flow of the Colorado River toward the UGC (Rodriguez et al., 2001; Dettman et al., 2004; Rowell et al., 2005, 2008). However, in the vaquita, an endothermic organism, temperature is not a primary determining factor (Clementz and Koch, 2001), as the impact on its diet may be due to an increase in salinity and temperature. Studies focusing on vaquita structures with longer temporal records, such as teeth and bullae, will help answer whether the damming of the Colorado River affects the vaquita’s habitat use.
5 Conclusion
The present study reveals for the first time the isotopic signals of δ13C and δ18O during two decades for the vaquita’s apatite bone, showing other possible pressures than bycatch in fishing nets that influence the habitat use and prey selection of the vaquita. The study suggests changes in primary productivity in the UGC or prey consumption during the period studied, with higher δ13C values for 1982–1988 than for 1989–1993. This was related to the presence or lack of Colorado River inflow (with the higher entry of freshwater in the first period), which influenced the environmental characteristics of the vaquita’s habitat, showing lower salinities and temperatures in minor hypersaline conditions. Although δ18O could not define the apparent differences between both decades, it allowed us to evaluate differences concerning other marine mammals from different habitats, finding characteristically high values in the vaquita relative to other estuarine or coastal aquatic mammals.
The findings on the change in productivity and the structure of the food web that were recorded in the vaquita’s bone tissue help us understand the relationship between the vaquita and the Colorado River, at least partially. Therefore, the present study underscores the importance of taking measures in the UGC and the delta between Mexico and the United States that, together with government actions of both countries, reinforce the delivery of water to the UGC and implement infrastructure that allows the restoration of the delta’s wetlands and the flow of water and organic matter to the UGC. We call for increasing conservation efforts for the UGC and the last survivors of the vaquita.
Data availability statement
The raw data supporting the conclusions of this article will be made available by the authors, without undue reservation.
Ethics statement
The manuscript presents research on animals that do not require ethical approval for their study.
Author contributions
M-YR-P: Conceptualization, Data curation, Formal analysis, Investigation, Methodology, Resources, Supervision, Validation, Visualization, Writing – original draft, Writing – review & editing. LS-V: Conceptualization, Funding acquisition, Investigation, Project administration, Resources, Supervision, Writing – original draft, Writing – review & editing. M-PR-H: Data curation, Formal analysis, Methodology, Software, Writing – review & editing. CH-C: Investigation, Methodology, Resources, Visualization, Writing – review & editing. FC-R: Data curation, Resources, Writing – review & editing. J-PG-R: Data curation, Resources, Writing – review & editing. FA-S: Funding acquisition, Project administration, Resources, Writing – review & editing. VG: Software, Visualization, Writing – review & editing.
Funding
The author(s) declare that financial support was received for the research, authorship, and/or publication of this article. The research is funded by the projects of the Instituto Politécnico Nacional SIP- 2023-RE/060, “Cambio en el hábitat de la vaquita marina”, and SIP-20240892, “Influencia del ambiente en los gradientes de distribución del zooplancton con énfasis en larvas de peces”. The first and third authors’ research are funded by postdoctoral grant CONAHCYT.
Acknowledgments
Thanks to Dr. Salvador Galindo Bect and Oceanologist David Aguilar for the logistical and coordinating support to carry out this project. Thanks to Mastozoological Collection at the Instituto de Biología UNAM and to Osteological Vertebrate Collection at the Centro de Investigación en Alimentación y Desarrollo (CIAD-Guaymas, Sonora), also thanks to M. C. Yolanda Hortelano Moncada, M. C. Julieta Vargas Cuenca and Isai Barba Acuña for the help in obtaining vaquita’s bone. The authors are very grateful for the suggestions of the reviewers of this manuscript.
Conflict of interest
The authors declare that the research was conducted in the absence of any commercial or financial relationships that could be construed as a potential conflict of interest.
Publisher’s note
All claims expressed in this article are solely those of the authors and do not necessarily represent those of their affiliated organizations, or those of the publisher, the editors and the reviewers. Any product that may be evaluated in this article, or claim that may be made by its manufacturer, is not guaranteed or endorsed by the publisher.
Supplementary material
The Supplementary Material for this article can be found online at: https://www.frontiersin.org/articles/10.3389/fcosc.2024.1490262/full#supplementary-material
References
Akaike H. (1998). “Information theory and an extension of the maximum likelihood principle,” in Selected papers of hirotugu akaike. springer series in statistics. Eds. Parzen E., Tanabe K., Kitagawa G. (New York, NY: Springer). doi: 10.1007/978-1-4612-1694-0_15
Álvarez-Borrego S., Flores-Báez B. P., Galindo-Bect L. A. (1975). Hidrología del Alto Golfo de California II. Condiciones durante invierno, primavera y verano. Cienc Mar 2, 21–36. doi: 10.7773/cm.v2i1.275
Bates D., Mächler M., Bolker B., Walker S. (2015). Fitting linear mixed-effects models using lme4. J. Stat. Software 67 (1), 1–48. doi: 10.18637/jss.v067.i01
Brusca R. C., Álvarez-Borrego S., Hastings P. A., Findley L. T. (2017). Colorado River flow and biological productivity in the Northern Gulf of California, Mexico. Earth-Sci. Rev 164, 1–30. doi: 10.1016/j.earscirev.2016.10.012
Brutemark A., Lindehoff E., Granéli E., Granéli W. (2009). Carbon isotope signature variability among cultured microalgae: Influence of species, nutrients and growth. J. Exp. Mar. Biol. Ecology 372, 98–105. doi: 10.1016/j.jembe.2009.02.013
Burnham K. P., Anderson D. R. (2002). Model selection and inference: A practical information-theoretic approach. 2nd Edition (New York: Springer-Verlag). doi: 10.1007/b97636
Carbajal N., Sousa A., Durazo R. (1977). A numerical model of the ex-ROFI of the Colorado River. J. Mar. Syst 12, 17–33. doi: 10.1016/S0924-7963(96)00086-3
Carriquiry J. D., Sánchez A. (1999). Sedimentation in the Colorado River delta and Upper Gulf of California after nearly a century of discharge loss. Mar. Geol 158, 125–145. doi: 10.1016/S0025-3227(98)00189-3
Chamberlain S. A., Boettiger C. (2017). R Python, and Ruby clients for GBIF species occurrence data. PeerJ Prepr 5, e3304v1. doi: 10.7287/peerj.preprints.3304v1
Cifuentes L. A., Sharp J. H., Fogel M. L. (1988). Stable carbon and nitrogen isotope biogeochemistry in the Delaware estuary. Limnol. Oceanogr 33, 1102–1115. doi: 10.4319/lo.1988.33.5.1102
Clementz M. T., Koch P. L. (2001). Differentiating aquatic mammal habitat and foraging ecology with stable isotopes in tooth enamel. Oecologia 129, 461–472. doi: 10.1007/s004420100745
Comisión Internacional para la Recuperación de la Vaquita (CIRVA) (2019). (La Jolla CA, USA). Available online at: https://iucn-csg.org/wp-content/uploads/2024/04/CIRVA-11-Final-Report-6-March.pdf. (accessed April 6, 2024).
Dettman D. L., Flessa K. W., Roopnarine P. D., Schöne B. R., Goodwin D. H. (2004). The use of oxygen isotope variation in shells of estuarine mollusks as a quantitative record of seasonal and annual colorado river discharge 1 1Associate. editor Falkner K. K. Geochim. Cosmochim. Acta 68, 1253–1263. doi: 10.1016/j.gca.2003.09.008
Diario Oficial de la Federación (1993). Decreto por el que se declara área natural protegida con el carácter de Reserva de la Biosfera, la región conocida como Alto Golfo de California y Delta del Río Colorado, ubicada en aguas del Golfo de California y los municipios de Mexicali, Baja California. Mexico City, Mexico.
Diario Oficial de la Federación (2005). Acuerdo mediante el cual se establece el área de refugio para la protección de la vaquita (Phocoena sinus). Mexico City: Secretaría de Medio Ambiente y Recursos Naturales. Mexico City, Mexico.
Diario Oficial de la Federación (2010). Norma Oficial Mexicana NOM-059-SEMARNAT-2010. Protección ambiental-Especies nativas de México de flora y fauna silvestres-Categorías de riesgo y especificaciones para su inclusión, exclusión o cambio- Lista de especies en riesgo. (Mexican Official Standard NOM-059-SEMARNAT-2010. Environmental protection-Native species of Mexico of wild flora and fauna-Risk categories and specifications for inclusion, exclusion or change-List of species at risk). SEMARNAT (Secretaría de Medio Ambiente y Recursos Naturales), Thursday December 30, 2010. Available online at: http://www.profepa.gob.mx/innovaportal/file/435/1/NOM_059_SEMARNAT_2010.pdf (accessed March 5, 2022).
Drago M., Valdivia M., Bragg D., González E. M., Aguilar A., Cardona L. (2020). Stable oxygen isotopes reveal habitat use by marine mammals in the Río de la Plata estuary and adjoining Atlantic Ocean. Estuar. Coast. Shelf Sci. 238, 106708. doi: 10.1016/j.ecss.2020.106708
Findley L., Nava J., Torre J. (1994). “Food habits of. phocoena sinus. (Cetacea: Phocoeanidae),” in Final Proc. Bienal Mar. Mamm. Biol. Conf. XI, USA. 1–37.
Fogel M. L., Cifuentes L. A., Velinsky D. J., Sharp J. H. (1992). Relationship of carbon availability in estuarine phytoplankton to isotopic composition. Mar. Ecol. Prog. Ser. 82, 291–300. doi: 10.3354/meps082291
France R. L. (1995). Differentiation between littoral and pelagic food webs in lakes using carbon isotopes. Limnol. Oceanogr 40, 1310–1313. doi: 10.4319/lo.1995.40.7.1310
Franklin J. F. (1993). Preserving biodiversity: species, ecosystems, or landscapes? Ecol. Appl 3, 202–205. doi: 10.2307/1941820
Fry B., Scalan R. S., Parker P. L. (1977). Stable carbon isotope evidence for two sources of organic matter in coastal sediments: seagrasses and plankton. Geochim. Cosmochim. Acta 41, 1875–1877. doi: 10.1016/0016-7037(77)90218-6
Fry B., Sherr E. B. (1989). “δ13C measurements as indicators of carbon flow in marine and freshwater ecosystems BT,” in Stable isotopes in ecological research. Eds. Rundel P. W., Ehleringer J. R., Nagy K. A. (Springer New York, New York, NY), 196–229.
Fry B., Wainright S. C. (1991). Diatom sources of 13 C-rich carbon in marine food webs. Mar. Ecol. Prog. Ser 76, 149–157. doi: 10.3354/MEPS076149
Galindo-Bect M. S., Vázquez-León C. I., Aguilar-Montero D. (2021). The colorado river damming impact on the shrimp fishery in the upper gulf of california, mexico. Front. Norte 33 (9), 23. doi: 10.33679/rfn.v1i1.2137p
Garvie-Lok S. J., Varney T. L., Katzenberg M. A. (2004). Preparation of bone carbonate for stable isotope analysis: the effects of treatment time and acid concentration. J. Archaeol Sci. 31, 763–776. doi: 10.1016/j.jas.2003.10.014
Gearing J. N., Gearing P. J., Rudnick D. T., Requejo A. G., Hutchins M. J. (1984). Isotopic variability of organic carbon in a phytoplankton- based estuary. Geochim Cosmochim Acta 48, 1089–1098. doi: 10.1016/0016-7037(84)90199-6
Gerrodette T., Rojas-Bracho L. (2011). Estimating the success of protected areas for the vaquita, Phocoena sinus. Mari. Mamm. Sci. 27, E101–E125. doi: 10.1111/j.1748-7692.2010.00449.x
Hedges R. E., Stevens R. E., Richards M. P. (2004). Bone as a stable isotope archive for local climatic information. Quat. Sci. Rev 23, 959–965. doi: 10.1016/j.quascirev.2003.06.022
Hohn A. A., Read A. J., Fernandez S., Vidal O., Findley L. T. (1996). Life history of the vaquita, phocoena sinus (Phocoenidae, cetacea). J. Zool. 239 (2), 235–251. doi: 10.1111/j.1469-7998.1996.tb05450.x
Huang B., Chunying L., Banzon V. F., Freeman E., Graham G., Hankins W., et al. (2020). NOAA 0.25-degree daily optimum interpolation sea surface temperature (OISST), version 2.1. NOAA Natl. Centers Environ. Information. doi: 10.25921/RE9P-PT57
Jaramillo-Legorreta A. M. (2008). Estatus actual de una especie en peligro de extincíon, la vaquita (Phocoena sinus): Una aproximacíon poblacional con métodos acústicos y bayesianos. PhD Thesis. Centro de Investigacíon Científica y de educacíon Superior de Ensenada (CICESE). PhD Thesis (Ensenada, Baja California Norte, México), 1–108.
Kim D. H., Aldridge K. T., Brookes J. D., Ganf G. G. (2013). The effect of salinity on the germination of ruppia tuberosa and ruppia megacarpa and implications for the coorong: A coastal lagoon of southern australia. Aquat. Bot. 111, 81–88. doi: 10.1016/j.aquabot.2013.06.008
Kinne O. (1964). The effects of temperature and Salinity on marine and brackish water animals. II. Salinity and temperature. Salinity combinations. Oceanogr. Mar. Biol. Ann. Rev. 2, 281–339.
Koch P. L., Tuross N., Fogel M. L. (1997). The effects of sample treatment and diagenesis on the isotopic integrity of carbonate in biogenic hydroxylapatite. J. Archaeol. Sci. 24, 417–429. doi: 10.1006/jasc.1996.0126
Kowalewski M., Avila Serrano G. E., Flessa K. W., Goodfriend G. A. (2000). Dead delta’s former productivity: Two trillion shells at the mouth of the colorado river. Geol. 28, 1059–1062.
Lavı́n M. F., Godı́nez V. M., Álvarez L. G. (1998). Inverse-estuarine features of the upper gulf of california. Estuar. Coast. Shelf. Sci. 47, 769–795. doi: 10.1006/ecss.1998.0387
Lavı́n M. F., Sánchez S. (1999). On how the colorado river affected the hydrography of the upper Gulf of California. Cont. Shelf. Res 19, 1545–1560. doi: 10.1016/S0278-4343(99)00030-8
Lozano-Montes H. M., Pitcher T. J., Haggan N. (2008). Shifting environmental and cognitive baselines in the upper gulf of california. Front. Ecol. Environ. 6 (2), 1–7. doi: 10.1890/070056
Matthews C. J. D., Longstaffe F. J., Ferguson S. H. (2016). Dentine oxygen isotopes (δ18O) as a proxy for odontocete distributions and movements. Ecol. Evol 6, 4643–4653. doi: 10.1002/ece3.2238
Mazerolle M. J. (2023). AICcmodavg: Model selection and multimodel inference based on (Q)AIC(c). R package version 2.3.3. Available online at: https://cran.r-project.org/package=AICcmodavg.
Miller T. W., Brodeur R. D., Rau G. H. (2008). Carbon stable isotopes reveal relative contribution of shelf-slope production to the Northern California Current pelagic community. Limnol Oceanogr 53, 1493–1503. doi: 10.4319/lo.2008.53.4.1493
Molony B. W., Parry G. O. (2006). Predicting and managing the effects of hypersalinity on the fish community in solar salt fields in north-western australia. J. Appl. Ichthyol. 22 (2), 109–118. doi: 10.1111/j.1439-0426.2006.00714.x
Moore K. M., Simeone C. A., Brownell R. L. (2018). “Strandings,” in Encyclopedia of marine mammals (USA: Academic Press), 945–951.
Newsome S. D., Clementz M. T., Koch P. L. (2010). Using stable isotope biogeochemistry to study marine mammal ecology. Mar. Mamm Sci. 26, 509–572. doi: 10.2112/JCOASTRES-D-14-00192.1
Newsome S. D., Koch P. L., Etnier M. A., Aurioles-Gamboa D. (2006). Using carbon and nitrogen isotope values to investigate maternal strategies in northeast pacific otariids. Mar. Mamm. Sci. 22 (3), 556–572. doi: 10.1111/j.1748-7692.2006.00043.x
Pellegrini M., Snoeck C. (2016). Comparing bioapatite carbonate pre-treatments for isotopic measurements: Part 2—Impact on carbon and oxygen isotopic compositions. Chem. Geol 420, 88–96. doi: 10.1016/j.chemgeo.2015.10.004
R CoreTeam (2023). R: A language and environment for statistical computing (Vienna, Austria: R Foundation for Statistical Computing). Available at: https://www.R-project.org (accessed August 12, 2024).
Reeves R. R., Berggren P., Crespo E. A., Gales N., Northridge S. P., di Sciara G. N., et al. (2005). Global priorities for reduction of cetacean bycatch. World Wildlife Fund. Available online at: https://www.bmisbycatch.org/system/files/zotero_attachments/library_1/AGGBMN8I%20-%20Reeves%20et%20al.%20%20Global%20Priorities%20for%20Reduction%20of%20Cetacean%20Bycatc.pdf.
Reeves R. R., McClellan K., Werner T. B. (2013). Marine mammal bycatch in gillnet and other entangling net fisherie 1990 to 2011. Endanger. Species Res 20, 71–97. doi: 10.3354/esr00481
Reist J. D., Wrona F. J., Prowse T. D., Power M., Dempson J. B., King J. R., et al. (2006). An overview of effects of climate change on selected arctic freshwater and anadromous fishes. AMBIO: J. Hum. Environ. 35 (7), 381–387. doi: 10.1579/0044-7447(2006)35[381:aooeoc]2.0.co;2
Riofrío-Lazo M., Arreguín-Sánchez F., Zetina-Rejón M., Escobar-Toledo F. (2013). The ecological role of the Vaquita, Phocoena sinus, in the ecosystem of the Northern Gulf of California. Ecosystems 16, 416–433. doi: 10.1007/s10021-012-9618-z
Rodríguez-Pérez M. Y., Aurioles-Gamboa D., Sánchez-Velásco L., Lavı́n M. F., Newsome S. D. (2018). Identifying critical habitat of the endangered vaquita (Phocoena sinus) with regional δ13C and δ15N isoscapes of the Upper Gulf of California, Mexico. Mar. Mamm Sci. 34, 790–805. doi: 10.1111/mms.12483
Rodríguez-Pérez M. Y., Ruiz-Cooley R. I., Aurioles-Gamboa D., Sánchez-Velasco L., Lavín M. F., Gallo-Reynoso J. P. (2021). Deciphering the trophic niche of the nearly extinct vaquita (Phocoena sinus) and its variability through time. Prog. Oceanogr 199, 102694. doi: 10.1016/j.pocean.2021.102694
Rodríguez-Pérez M. Y., Sánchez-Velasco L., Godínez V. M., Galindo-Bect M. S., Martínez-Rincón R. O. (2023). Vaquita’s habitat suitability in the Upper Gulf of California between two contrasting environmental years: 1997 and 2008. Reg. Stud. Mar. Sci. 62, 102907. doi: 10.1016/j.rsma.2023.102907
Rojas-Bracho L., Reeves R. R., Jaramillo-Legorreta A. (2006). Conservation of the vaquita Phocoena sinus. Mamm Rev 36, 179–216. doi: 10.1111/j.1365-2907.2006.00088.x
Rowell K., Flessa K. W., Dettman D. L., Román M. (2005). The importance of colorado river flow to nursery habitats of the gulf corvina (Cynoscion othonopterus). Can. J. Fish. Aquat. Sci. 62, 2874–2885. doi: 10.1139/f05-193
Rowell K., Flessa K. W., Dettman D. L., Román M. J., Gerber L. R., Findley L. T. (2008). Diverting the colorado river leads to a dramatic life history shift in an endangered marine fish. Biol. Conserv. 141, 1138–1148. doi: 10.1016/j.biocon.2008.02.013
Sánchez-Velasco L., Lavín M. F., Jiménez-Rosenberg S. P. A., Montes J. M., Turk- Boyer P. J. (2012). Larval fish habitats and hydrography in the biosphere reserve of the upper Gulf of California (2008). Contin. Shelf Res. 33, 89–99. doi: 10.1016/j.csr.2011.11.009
Schipper J., Chanson J. S., Chiozza F., Cox N. A., Hoffmann M., Katariya V., et al. (2008). The status of the world's land and marine mammals: diversity, threat, and knowledge. Science 322, 225–230. doi: 10.1126/science.1165115
Schoeninger M. J., DeNiro M. J. (1984). Nitrogen and carbon isotopic composition of bone collagen from marine and terrestrial animals. Geochim. Cosmochim. Acta 48, 625–639. doi: 10.1016/0016-7037(84)90091-7
Sealy J., Armstrong R., Schrire C. (1995). Beyond lifetime averages: Tracing life histories through isotopic analysis of different calcified tissues from archaeological human skeletons. Antiquity 69, 290–300. doi: 10.1017/S0003598X00064693
Silber G. K., Norris K. S. (1991). The geographic and seasonal distribution of the vaquita, Phocoena sinus. Ann. Insit. Biol., Universidad Nacional Autónoma de México. Ser. Zool 62, 263–268.
Simons R. A. (2019). ERDDAP, NOAA southwest fisheries science center monterey, CA: NOAA. NMFS/SWFSC/ERD. Available online at: http://coastwatch.pfeg.noaa.gov/erddap (accessed August 12, 2024).
Smith K. J., Sparks J. P., Timmons Z. L., Peterson M. J. (2020). Cetacean skeletons demonstrate ecologically relevant variation in intraskeletal stable isotopic values. Front. Mar. Sci. 7 (388), 1–9. doi: 10.3389/fmars.2020.00388
Snoeck C., Pellegrini M. (2015). Comparing bioapatite carbonate pre-treatments for isotopic measurements: Part 1—Impact on structure and chemical composition. Chem. Geol 417, 394–403. doi: 10.1016/j.chemgeo.2015.10.004
Sora K. J., Wabnitz C. C. C., Steiner N. S., Sumaila U. R., Hoover C., Niemi A., et al. (2024). Historical climate drivers and species’ ecological niche in the beaufort sea food web. ICES J. Mar. Sci., fsae062. doi: 10.1093/icesjms/fsae062
Trueman C. N., MacKenzie K. M., Palmer M. R. (2012). Identifying migrations in marine fishes through stable-isotope analysis. J. Fish Biol. 81, 826–847. doi: 10.1111/j.1095-8649.2012.03361.x
Trueman C. N., St John Glew K., Hobson K. A., Wassenaar LIBT-TAM with SI (2019). “Isotopic tracking of marine animal movement,” in Tracking animal migration with stable isotopes, 2nd ed. (United Kingdom: Elsevier), 137–172.
Tweedley J. R., Dittmann S. R., Whitfield A. K., Withers K., Hoeksema S. D., Potter I. C. (2019). Hypersalinity: Global distribution, causes, and present and future effects on the biota of estuaries and lagoons. Coasts Estuaries. 30, 523–546. doi: 10.1016/B978-0-12-814003-1.00030-7
Vidal O. (1995). Population biology and incidental mortality of the vaquita, phocoena sinus. Oceanographic Literature Review 10, 1042.
Keywords: marine mammals, endemic, Colorado River, Gulf of California, stable isotopes, δ18O
Citation: Rodríguez-Pérez M-Y, Sánchez-Velasco L, Rosas-Hernández M-P, Hernández-Camacho CJ, Cervantes FA, Gallo-Reynoso JP, Arreguín-Sánchez F and Godínez VM (2024) Stable isotopes of carbon (δ13C) and oxygen (δ18O) from vaquita (Phocoena sinus) bones as indicators of habitat use in the Upper Gulf of California. Front. Conserv. Sci. 5:1490262. doi: 10.3389/fcosc.2024.1490262
Received: 02 September 2024; Accepted: 26 November 2024;
Published: 18 December 2024.
Edited by:
Biao Yang, China West Normal University, ChinaReviewed by:
Ichiro Tayasu, Research Institute for Humanity and Nature, JapanJuan Pablo Torres-Florez, Fujairah Research Centre, United Arab Emirates
Copyright © 2024 Rodríguez-Pérez, Sánchez-Velasco, Rosas-Hernández, Hernández-Camacho, Cervantes, Gallo-Reynoso, Arreguín-Sánchez and Godínez. This is an open-access article distributed under the terms of the Creative Commons Attribution License (CC BY). The use, distribution or reproduction in other forums is permitted, provided the original author(s) and the copyright owner(s) are credited and that the original publication in this journal is cited, in accordance with accepted academic practice. No use, distribution or reproduction is permitted which does not comply with these terms.
*Correspondence: Mónica-Yanira Rodríguez-Pérez, eWFuaXJhcnBlcmV6QGdtYWlsLmNvbQ==; Laura Sánchez-Velasco, bHN2ZWxhc2NAaXBuLm14