- 1Independent Researcher, Jonesboro, ME, United States
- 2Norwegian Polar Institute, Fram Center, Tromsø, Norway
Arctic ecosystems are especially vulnerable to the impacts of climate change because of the limit to possible northward shifts for species dependent on land or continental shelf and because the rate of warming of the region has been 2-4 x the global average in recent decades. The decline in sea ice in the Arctic has both direct and indirect impacts on the species that live in association with ice, breeding on it, traveling over it, feeding on other ice-dependent species or avoiding competition with subarctic species that cannot exploit resources in ice-covered areas. Herein, we present a metamodel of a top-level predator, the polar bear (Ursus maritimus), and two of its key prey species, ringed seals (Pusa hispida) and bearded seals (Erignathus barbatus), which are important in maintaining current polar bear densities and in turn are strongly influenced by bear predation. We used a metamodel that links Population Viability Analyses of the three species in order to examine how the impacts of declining spring land-fast sea ice on the fjords of Svalbard (Norway) and Frans Josef Land (Russia) can cascade through this predator-prey system. As the ice conditions that allow ringed seals to raise pups in snow-covered lairs on the frozen fjords diminish, or even disappear, ringed seal populations using the land-fast sea ice will collapse due to lack of successful recruitment. Consequently, the polar bear population, which relies heavily on hunting ringed seals in the land-fast sea ice to be able to raise their own offspring is also likely to decline. Our models suggest time-lags of decades, with the polar bear population not entering into decline until the lack of recruitment of ringed seals results in the depletion of breeding age ringed seals – starting in the third decade from the start point of the model and dropping below the initial population size only some decades later. Although lags between climate change and impacts on the ice-associated fauna are expected, the sea ice conditions have already changed dramatically in the northern Barents Sea region, including the Svalbard Archipelago, and the collapse of this Arctic species assemblage might already be underway.
1 Introduction
Global warming is impacting species across the globe (Pacifici et al., 2015; Stanton et al., 2015; Scheffers et al., 2016; IPCC, 2023), but Arctic endemic species are especially vulnerable to the ongoing warming, because of: 1) the limit to possible northward expansion for species dependent on land or continental shelf waters, 2) their inherent physiological adaptations to cold temperatures, 3) their conservative life-history strategies (long life-times and low reproductive rates), designed to buffer interannual variation and 4) their ecological dependencies on snow/ice, and 5) because the rate of warming in this region is two to four times the rate of the planet as a whole (Gilg et al., 2012; Previdi et al., 2021; Rantanen et al., 2022; Shu et al., 2022; Taylor et al., 2022).
The ongoing declines in sea ice in the Arctic are a particularly visible sign of global warming, which has dramatic implications for sympagic (ice-dependent) communities. IPCC’s 6th assessment suggested that the Arctic could experience ice-free conditions in September prior to 2050 (IPCC, 2023). Other recent projections suggest that it is likely that these conditions will be experienced in the next decade or two (Kim et al., 2023; Jahn et al., 2024). Arctic marine mammals use sea ice in diverse ways that include seeking refuge from open water predators, in order to travel over long distances (without swimming in the case of polar bears), for feeding on other ice-dependent species (invertebrates, fish, marine mammals), to reproduce, molt, rest and also to avoid competition with subarctic species that cannot exploit resources that occur in ice-covered areas (ACIA, 2005; CAFF, 2013, 2017; Meredith et al., 2019; AMAP, 2021; Kovacs et al., 2021a).
All Arctic endemic marine mammals are strongly ice-affiliated and hence are threatened by both the direct effects of habitat loss and the indirect effects of sea ice losses on Arctic marine food webs and concomitant human activity increases in areas previously protected by ice cover (Tynan and DeMaster, 1997; Regehr et al., 2007; Laidre et al., 2008, 2015; Kovacs et al., 2011, 2012, 2021a; Reeves et al., 2014). Populations of some currently abundant species are likely to decline (or even be regionally extirpated), and because marine mammals are key species in Arctic food webs, there will likely be secondary impacts on species that prey upon them, are preyed on by them, or compete with them (Blanchet et al., 2019; Kiszka et al., 2015). Because of their strong affiliation with sea ice, Arctic marine mammals are seen as sentinels of Arctic Ocean health (see Moore and Gulland, 2014). Some climate change impacts on marine mammal populations have been demonstrated (e.g. Udevitz et al., 2012; Stenson and Hammill, 2014; Øigård et al., 2014; Rode et al., 2022; Vacquiè-Garcia et al., 2024), but logistics challenges and costs of surveys and other data acquisition for these animals have created a lack of base-line comparative data for many populations/species, making it difficult to document or accurately predict trends (see Kovacs et al., 2021a for a review).
Attempts to predict effects of climate change on species fall into three broad categories (Dawson et al., 2011; Pacifici et al., 2015; Willis et al., 2015; Foden and Young, 2016): descriptions of species and habitat traits that make species more vulnerable (e.g., Laidre et al., 2008; Foden et al., 2013); a correlative approach that models shifts in species distribution based on observed niche and projections of future suitable ranges (e.g., MacLeod, 2009; Gregory et al., 2012); and mechanistic models of the effects that climate change will have on physiological or population processes (e.g., Molnár et al., 2010, 2011). These approaches have increasing specificity, and therefore would be expected to provide more accurate predictions as the detailed mechanisms through which climate change affects species are examined. However, as models become more specific, they typically also become more narrowly focused, and therefore can omit interactions between species or other environmental processes that might be essential for the persistence of the focal species. Some studies have combined aspects of several of these approaches to obtain more integrated and complete analyses (e.g., Keith et al., 2008; Fordham et al., 2013a, 2013b; Foden and Young, 2016). Most analyses of this sort are theoretical, and few have been applied to marine mammal species.
Population Viability Analysis (PVA) is an approach that was developed to assess multiple interacting threats that have impacts on a species simultaneously. PVA uses demographic modeling, often in combination with genetic, habitat, and other models, to assess the risks to wildlife populations under various scenarios and evaluate the likely efficacy of protection, recovery, or restoration options (Shaffer, 1990; Boyce, 1992; Beissinger and McCullough, 2002; Morris and Doak, 2002). PVA models have been used to examine pending impacts of climate change on species (e.g., Wichmann et al., 2005; Molnár et al., 2010; Molano-Flores and Bell, 2012), but these studies have generally employed the standard PVA approach of focusing on a single species and its habitat. Thus, they consider other species with which the focal species interacts as not themselves to be undergoing changed population dynamics due to climate change, or at least as being independent systems rather than being tightly coupled with the critical interactions being modified by climate. Even in studies that might be termed multi-species PVAs, addressing climate change or otherwise, usually independent analyses are completed on a set of species. However, if it is the species interactions that are dependent on climatic conditions, then single-species PVA models will often fail to identify the disruptions to ecological communities that will be caused by climate change. However, species focused PVA models can be combined into “metamodels” of tightly interacting species, by linking a PVA model for each species to others, so that the dynamic change in each species can affect the others (Lacy et al., 2013). Each species model can be impacted by common or separate external environmental drivers, and emergent properties of the community’s dynamics can emerge from the metamodel. In such a way, PVA has recently been extended to examine predator-prey interactions (e.g., Shoemaker et al., 2014), impacts of invasive species (Miller et al., 2016), disease-host interactions (e.g., Bradshaw et al., 2012; Wells et al., 2015), and multiple concurrent threats (Prowse et al., 2013).
Herein, we present a metamodel for a top-level predator, the polar bear (Ursus maritimus), and two of its prey species, ringed seals (Pusa hispida) and bearded seals (Erignathus barbatus) that are important to the persistence of the polar bear and in turn are strongly influenced by bear predation. The interactions between these species, as well as other aspects of the biology of each species, are highly dependent upon sea ice. Therefore, changing Arctic sea-ice conditions as the climate continues to warm are likely to have especially profound impacts on the interdependence of these animals. We focused on the populations of the three species around the Svalbard Archipelago (Norway), Franz Josef Land (Russia), and the Barents Sea between these archipelagos, northward to the Arctic ice cap. These High Arctic areas have until recently had extensive ice cover, including the fjord areas within the archipelagos and coastal areas, throughout much of the year. But Svalbard in particular has changed dramatically, with rapid increases in air temperature and reductions in sea ice cover, along with intrusions of Atlantic Water masses (which are increasing in temperature) into the fjords especially on the west side of the archipelago (e.g., De Rovere et al., 2022; Isaksen et al., 2022; Urbanski and Litwicka, 2022). This region is experiencing warming at a rate twice the average for the Arctic, so is a bellwether for other regions (Isaksen et al., 2022). We used a metamodel that links PVAs of the three species in order to examine the impact of declining spring-time land-fast sea ice on the fjords cascades through this predator-prey system. We modeled the ringed seals as the primary prey species; its relationship with polar bears is likely to be strongly influenced by changing ice conditions. We modeled the bearded seal population as an alternative prey for which predation might not be as strongly impacted by changing ice conditions. This metamodel therefore allows us to project possible severity and timing of some climate change induced threats to these Arctic endemic species.
2 Methods
2.1 PVA models
Population models for each of the three species were developed with the Vortex PVA software version 10.6 (Lacy, 2000; Lacy et al., 2023; Lacy and Pollak, 2023; program and manual available at https://scti.tools/vortex). Vortex is an age and sex-structured population model that simulates demographic processes subjected to both deterministic forces and demographic, environmental, and genetic stochastic events. Vortex simulates a population by stepping through a series of events that describe an annual cycle, including mate selection; reproduction; mortality; and dispersal. Each demographic rate can be specified to be a function of individual traits (e.g., age and breeding history), population characteristics (e.g., density and age structure), or external drivers (e.g., habitat characteristics). Vortex is normally used as an individual-based model, projecting population dynamics from the aggregate of fates of individuals, but it has an option to run as a simpler population-based model (analogous to matrix models of demography; Caswell, 2001) with any effects of individual variation around demographic rates assumed to be described adequately by the population means. In either mode, the simulations are iterated to generate the distribution of fates that the population might experience.
Demographic rates for the PVA models were obtained from published sources or, when data were lacking, from expert opinion of the authors and colleagues with validation that derived population statistics such as age structure and population growth rates consistent with the species biology and information on the local population. When available, we relied on data on the respective Svalbard populations; otherwise, we used information from other populations for the actual species. Variation in probabilities of demographic events caused by fluctuations in the environment across years (“environmental variation”, EV, as opposed to directional trends in rates) is modeled in Vortex by sampling the demographic rates each year from binomial distributions with user-specified means and standard deviations. For our models we assumed that fluctuations in annual survival and reproduction are largely affected by the same factors, and we therefore specified that the correlation between reproduction and survival was 1.0 in each of our scenarios. This might exaggerate the annual fluctuations in population demography slightly, compared to a case in which fluctuations in survival and reproduction are independent. However, with species as long-lived as the Arctic seals and the polar bear, environmental variation will be unimportant to long-term projections because fluctuations in demographic rates average out across years. The initial age distributions were set at the stable age distribution calculated from initial fecundity and survival rates. However, the precise age distributions that arise from the complex metamodel dynamics often cannot be calculated analytically, and the first 2 or 3 years of projections sometimes display short-term fluctuations before long-term patterns emerge.
For ringed seals and bearded seals, the models were run as population-based simulations, with no considerations of individual characteristics that might modify fecundity and survival rates. For the polar bears, the Vortex model was run as an individual-based simulation, so that we could include the dynamics of females being available to breed only when they did not have dependent cubs (see below).
2.1.1 Ringed seal population and demography
Ringed seals in the Barents Sea give birth primarily on land-fast sea ice along the coastlines of Svalbard (Norway) and Franz Josef Land (Russia) (Kovacs and Lydersen, 2006). Some pupping is known to occur in the drift ice of the northern Barents Sea (Kovacs and Lydersen, pers. obs), but these areas have never been systematically surveyed. Female polar bears that den on the islands of the Svalbard and Franz Josef Land archipelagos rely heavily on feeding on ringed seals in land-fast sea ice breeding areas upon emergence from their dens in spring, after a long period of fasting in the den (Freitas et al., 2012). Their cubs are small and cannot travel great distances easily and cannot be immersed in water for long periods (Lone et al., 2018). Therefore, we assume that the number of ringed seals breeding on the land-fast sea ice is a critical determinant of the number of polar bear cubs that can be successfully raised.
The total population size of ringed seals in the Barents Sea region was set in our models at 200,000 in the non-breeding season based on surveyed areas in Svalbard and estimated densities in various breeding areas (Lydersen et al., 1990; Lydersen and Ryg, 1991; Smith and Lydersen, 1991; Krafft et al., 2006). These densities were extrapolated to apply also to the land-fast sea ice areas in Franz Josef Land. At the start of the simulation model, we divided this number of seals equally between those that use the land-fast sea ice around the archipelagos and those that use the vast areas of drift ice. A population of this size would produce about 54,000 pups per year (given reproductive rates below), about half of which would be raised on land-fast sea ice in fjords, with approximately half of these pups being born in Svalbard (rather than Franz Josef Land).
Ringed seals are physiologically mature (capable of spermatogenesis or ovulation) at a mean of 4.2 y and 3.5 y for males and females, respectively (Krafft et al., 2006), although maturation was about a year later in the 1980s, and successful breeding might not take place until a year or more after sexual maturation. Lydersen and Gjertz (1987) reported that 63% of males are sexually mature by age 6 y, and 60% of females are sexually mature by 5 y. We therefore modeled the reproductive lifespan of females is from 5 y to 40 y, with males starting to breed about 1 y later (at 6 y).
Most adult female ringed seals produce a single pup each year, with ovulation rates reported to be 91% (Lydersen and Gjertz, 1987) and 86% (Krafft et al., 2006) in Svalbard. We therefore specify in the model that 90% of adult females pup in an average year (with environmental variation across years, EV, of ± 5% SD). Ringed seals are polygynous, with younger and small males and old males being excluded from prime mating areas (Krafft et al., 2007). We assumed that the sex ratio of newborns is 1:1.
Survival rates for ringed seals have not been documented, so we used values that yield population growth rates (r = 0.059) and age structures that are plausible for the species. For females, we specified annual mortality rates of 50% (± 3% SD), 15% (± 1% SD), 12% (± 1% SD), 9% (± 1% SD), 7% (± 1% SD), and 5% (± 1% SD), for the first year, 2nd, 3rd, 4th, 5th, and later years, respectively. For males, we specified annual mortality rates of 50% (± 3% SD), 15% (± 1% SD), 12% (± 1% SD), 9% (± 1% SD), 6% (± 1% SD), and 5% (± 1% SD), for the first year, 2nd, 3rd, 4th, 5th, and later years, respectively. The maximum age for both sexes was set to 45 y (Lydersen and Gjertz, 1987), although fewer than 2% would be expected to live beyond 35 y based on the average annual mortality.
In order to model the interaction of polar bear and prey population dynamics, the annual mortality rate for each age class was divided into mortality attributable to polar bear predation (which will change during the simulation as the ratio of bears to seals changes) and the component due to other sources of mortality (which are assumed to remain constant over time). The mortality of each age class due to polar bear predation was calculated as the number of ringed seals preyed on by polar bears to meet the bears’ energetic demands (see below), multiplied by the proportion of predation occurring on that age class of seals, divided by the number of ringed seals in that age class. The background annual mortality without bear predation was then calculated so that the survival prior to bear predation multiplied by the survival rate due to predation was equal to one minus the total mortality rates listed above. (See annotated input files in the Supplementary Material for further details and equations.) After removing the estimated predation by polar bears, the remaining non-bear mortality rates for females were 29.9%, 0%, 0%, 5.9%, 3.8%, and 1.8%, for the first year, 2nd, 3rd, 4th, 5th, and later years, respectively, and for males they were 29.9%, 0%, 0%, 5.9%, 2.8%, 1.8%, and 1.8%, for the first year, 2nd, 3rd, 4th, 5th, 6th, and later years, respectively. Our calculations of the numbers of 2nd and 3rd year ringed seals killed by bears amounted to the total mortality we had estimated for these two age classes. It is implausible that all mortality of these age classes was due to bear predation, so either some of the bear predation allocated to 2nd and 3rd year seals must be on other juvenile age classes instead, or our estimates of the total mortality experienced by other pre-reproductive age classes included some mortality that should be assigned to 2nd and 3rd year seals. However, given that the total survival to the age of breeding is the important factor in demographic projections determining population growth, the allocation of mortality among specific pre-breeding age classes does not affect population trajectories. The total mortality up to breeding age in our model was 36.5% without bear predation and 68.3% with bear predation.
The carrying capacity (K) was set to 125% of the initial population size for each subpopulation (land-fast sea ice vs drift/pack ice), to allow for population growth to be apparent in any scenarios with optimistic conditions, such as no decline in land-fast sea ice. It is not known to what extent ringed seals move between the areas around the archipelagos and the pack ice, but there are some fragmentary data that suggest high site fidelity in this species (McLaren, 1958; Smith and Hammill, 1981; Kelly and Quakenbush, 1990; Freitas et al., 2008; Kelly et al., 2010; Hamilton et al., 2015, 2016). Dispersal between the two areas was therefore modeled as occurring with 5% of subadults (ages 3y, 4y, and 5y) of both sexes moving to the other area each year. Alternative dispersal rates of 0% to 10% per year were also tested.
Key demographic parameters for the ringed seal populations are summarized in Table 1. Complete listing of the values entered into the Vortex population model are provided in the annotated input in Supplementary Material.
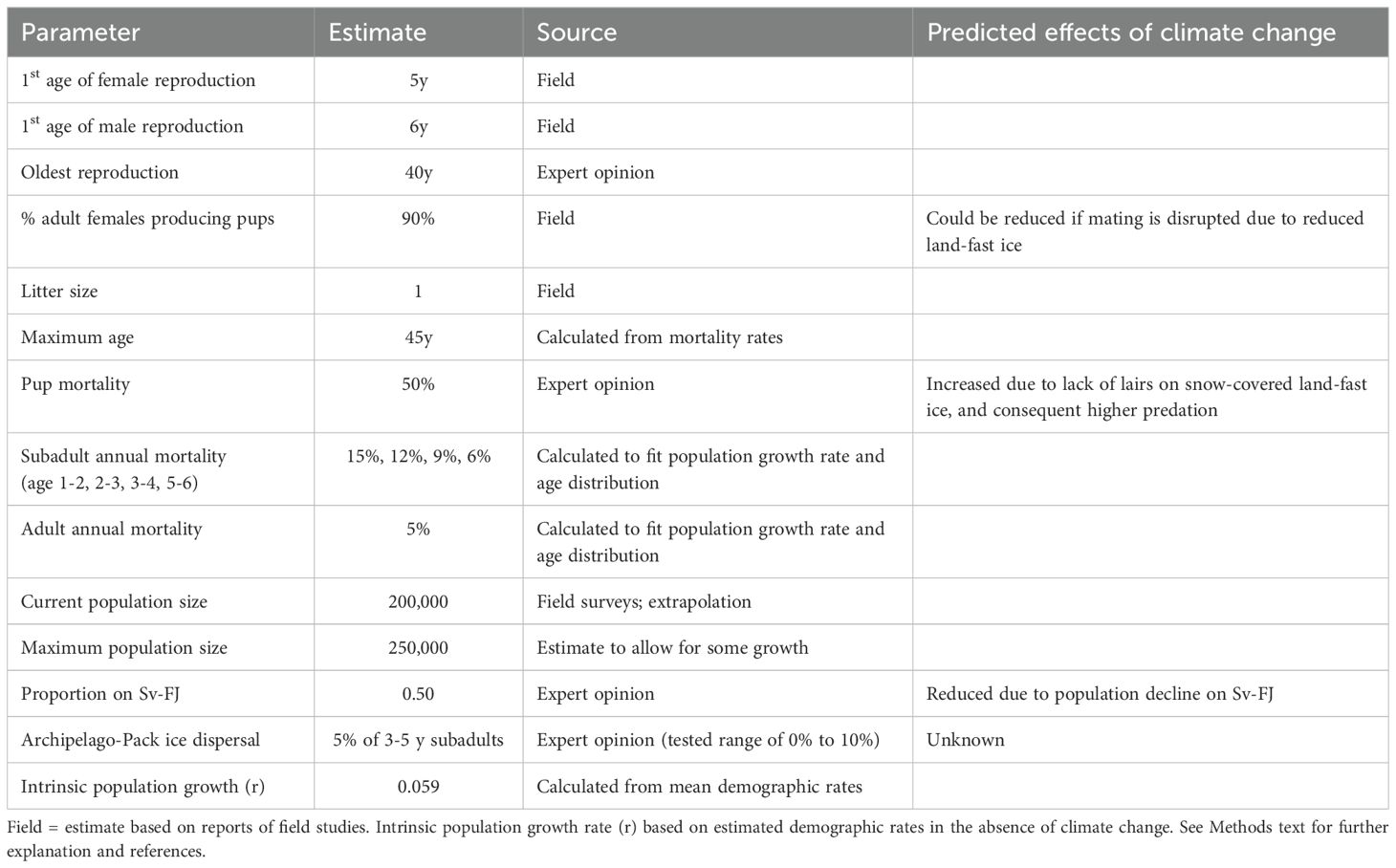
Table 1. Key demographic parameters used to model ringed seal population in the northern Barents Sea.
2.1.2 Bearded seal population and demography
No surveys have been undertaken for bearded seals in the northern Barents Sea, and the population is widely dispersed at variable densities throughout the area (Ahonen et al., 2017; Llobet et al., 2023). We set the total population size of bearded seals in the region at 50,000 in the non-breeding season.
Bearded seals become sexually mature at about 5 y for females and 6 y for males (Andersen et al., 1999), and we assumed that they typically produce their first pup at age 6 y. They can breed throughout a lifetime that can extend to 25 y. Most adult female bearded seals produce a single pup each year, and we specified in the model that 90% (with an EV of ± 5% SD) of adult females give birth in an average year. Bearded seals are polygynous (Van Parijs et al., 2003). We assumed that the sex ratio of newborns is 1:1.
Survival rates for bearded seals have not been documented, so we used values that yield exponential population growth rates (r = 0.048) and age structures that are plausible for the species. For both sexes, we assumed annual mortality rates of 25% (± 3% SD) in the first year and 10% (± 1% SD) each year thereafter. These mortality rates would result in a 6% probability of an animal surviving to the maximum age of 25 y. The annual mortality rate for each age class was divided into mortality due to polar bear predation and a second component arising from other sources of mortality. After removing the estimated predation by polar bears, the remaining non-bear mortality rates for the bearded seal model were 7.1%, 2.9%, 2.9%, and 0.1% in the first year, 2nd, 3rd, and later years, respectively. Our estimates project that most of the bearded seal mortality can be accounted for by predation by polar bears, therefore, we may have underestimated total mortality or overestimated the proportion killed by bears. The carrying capacity (K) was set at 125% of the initial population size to allow for some population expansion.
Key demographic parameters for the bearded seal population are summarized in Table 2. Complete listing of the values entered into the Vortex population model are provided in the annotated input in Supplementary Material.
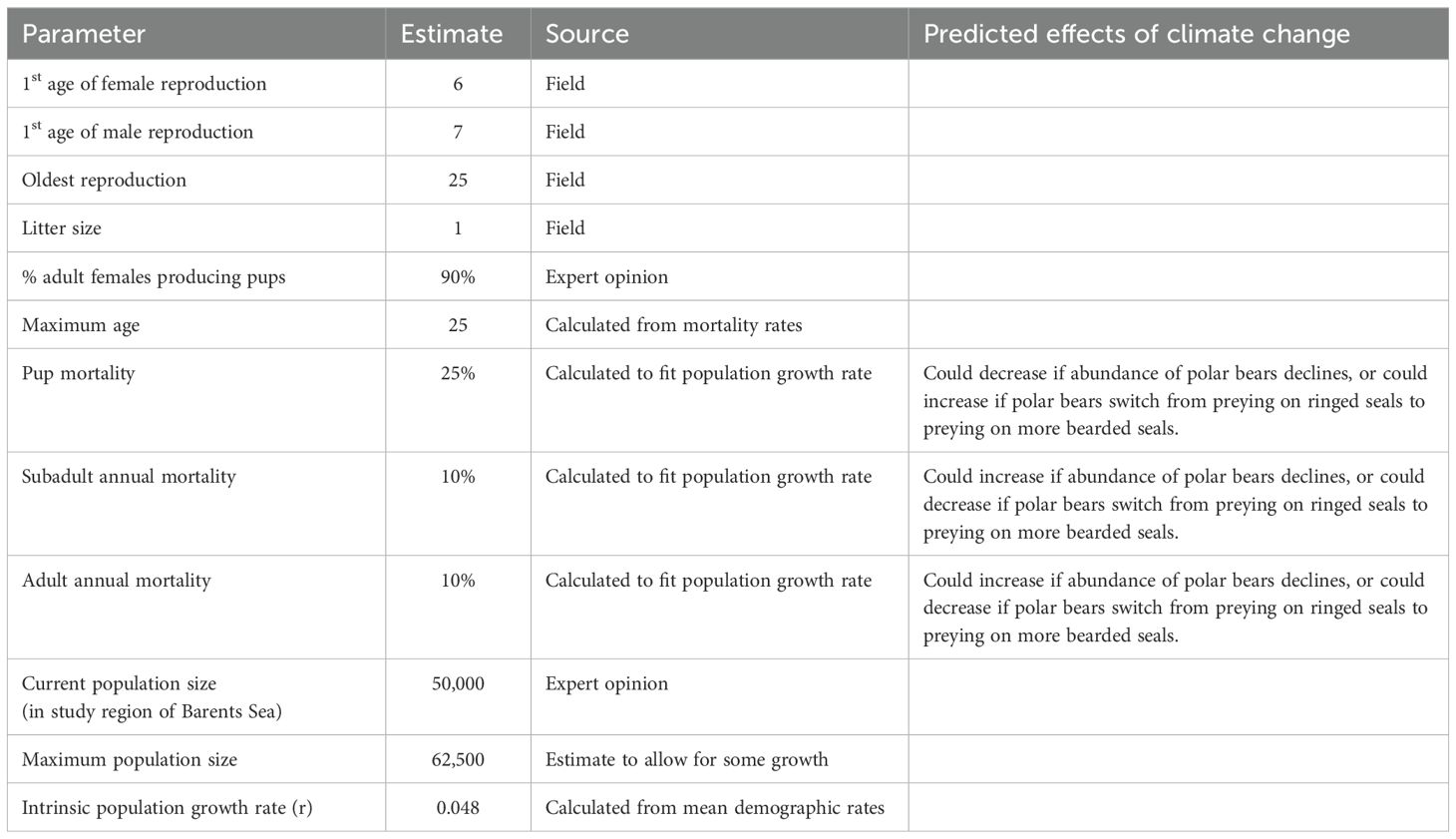
Table 2. Key demographic parameters used to model bearded seal population in the northern Barents Sea.
2.1.3 Polar bear population and demography
The size of the Barents Sea subpopulation of polar bears was estimated to be N = 2,650 in 2004 (Aars et al., 2009), out of a total circumpolar Arctic estimate of approximately 26,000 for the 20 recognized populations combined (Regehr et al., 2016; Laidre et al., 2022). The Barents Sea subpopulation was heavily harvested until 1973 (with an average of 320 bears taken per year from 1945-1970; Derocher, 2005) but has grown in the subsequent decades following protective legislation and is likely still recovering (Aars et al., 2017).
Polar bears in Svalbard usually produce their first litter when females are 6 y (mating at age 5 y) of age or older, although they occasionally have cubs at when they are 5 y (Derocher, 2005). We set the initial age of breeding in the models to be 6 y. Females will not produce a subsequent litter while they still have dependent cubs. Females that successfully wean litters can produce their next litter after a 3-year inter-birth interval. If all cubs in a litter die before weaning, females can breed that year to produce a litter the next year (if the prior litter dies early enough in the year for mating to take place in late spring). The individual-based simulation tracked when females produced cubs and when those cubs died to determine at each year if a female had dependent cubs. If a female lost a litter from one of the prior two years, we specified in the model that she would have a 25% probability of being able to recycle and mate again that year. Otherwise, she could not mate again until the following year.
Of the adult females that are available for mating, we specified that 90% (± 5% environmental variation, EV) produce a litter. Given the restriction on breeding by females with dependent cubs, this leads to a mean of 40% of adult females producing a litter each year in our model, which is similar to the values of 37.5% reported by Derocher (2005) and 41% reported by Wiig (1998). We specified the distribution of litter sizes to be 31% a single cub, 66% twins, and 3% triplets, resulting in the reported mean of 1.72 (Derocher, 2005). We assumed that the sex ratio of newborns is 1:1. We specified in the model that dependent cubs will die if their mother dies.
Males in Svalbard have been seen with females during the mating season from the age of 4 y, but scarring, fresh wounds, and age distribution among males having access to females, suggests that 6 y is a typical age for males to start to reproduce. Derocher et al. (2010) showed that few young adult males were seen with females in mating pairs. However, Zeyl et al. (2009) showed from genetics that young males were more successful than suggested by observations. Based on this combined information, we assumed that young sexually mature males (6-10 y) are 80% as likely as older males to breed in our models.
Mortality rates for polar bears in Svalbard have been estimated for adult females based on data from satellite telemetry collars (Wiig, 1998), and for animals of different ages based on capture/recapture data (Cubaynes et al., 2021). Litter production rates have been reported both in Cubaynes et al. (2021), and in Naciri et al. (2022), where the latter study showed an increase in production with age for females, increasing from young ages up to prime age females, followed by a sharp decrease with old age. The values presented below are based on these sources. First year mortality of cubs was set at 85% for primiparous females and 50% (EV of ± 10% SD) when the mother had experience with at least one prior litter. The annual mortality of dependent cubs in each of the next two years was set to 20% (± 5% SD). Annual mortality from 3 y to physical maturity at 6 y was set to 3% (± 1% SD). For adults, annual mortality was set to 2% (± 1 SD) up to 18 y, 10% from 18 y to 22 y, and 20% after 22 y. Maximum longevity was set to 28 y, and breeding occurred only up through 25 y of age, so that females would be able to survive to rear their last litter to independence. These values generate a maximum population growth rate of 2.1%.
The carrying capacity (K) was modeled as an upper limit on the number of independent bears (3 y and older). When K was exceeded, additional mortality with a probability of (N-K)/N was applied to each individual across all age classes to bring the population size back down to K. Based on harvest levels, the historic population size across the region was likely 6,000 or more, although some of that would have been from Greenland (i.e., outside of the area we are modeling). It is not known if the current carrying capacity is as large as it was for the historic population, because sea ice habitat has declined markedly over the last three decades in the area (Stern and Laidre, 2016; Urbanski and Litwicka, 2022). We can estimate the number of bears that could be supported by the prey base of bearded seals, ringed seals, and other prey – using the energetic calculations described below (also see Stirling and Øritsland, 1995). With the estimates of predator-prey relationships in our metamodel, we observed that predation by the polar bear population on bearded and ringed seals is unsustainable (driving the seal populations down and consequently causing collapse also of the bear population) if the bear population grows to a size such that it takes more than 15% of the combined prey populations each year. Thus, we can define the carrying capacity of the polar bear population as being the size that can be supported by a predation rate of 15% per year (i.e., K = 0.15 x available biomass of ringed seals, bearded seals, and other prey/43.5 required prey per bear [see below]). With the seal populations able to grow to an estimated 125% of current sizes (see above), this leads to an upper limit of K for the population of 3605 independent polar bears, or 4532 total bears including cubs still dependent on their mothers.
Key demographic parameters for the polar bear population are summarized in Table 3. Complete listing of the values entered into the Vortex population model are provided in the annotated input in Supplementary Material.
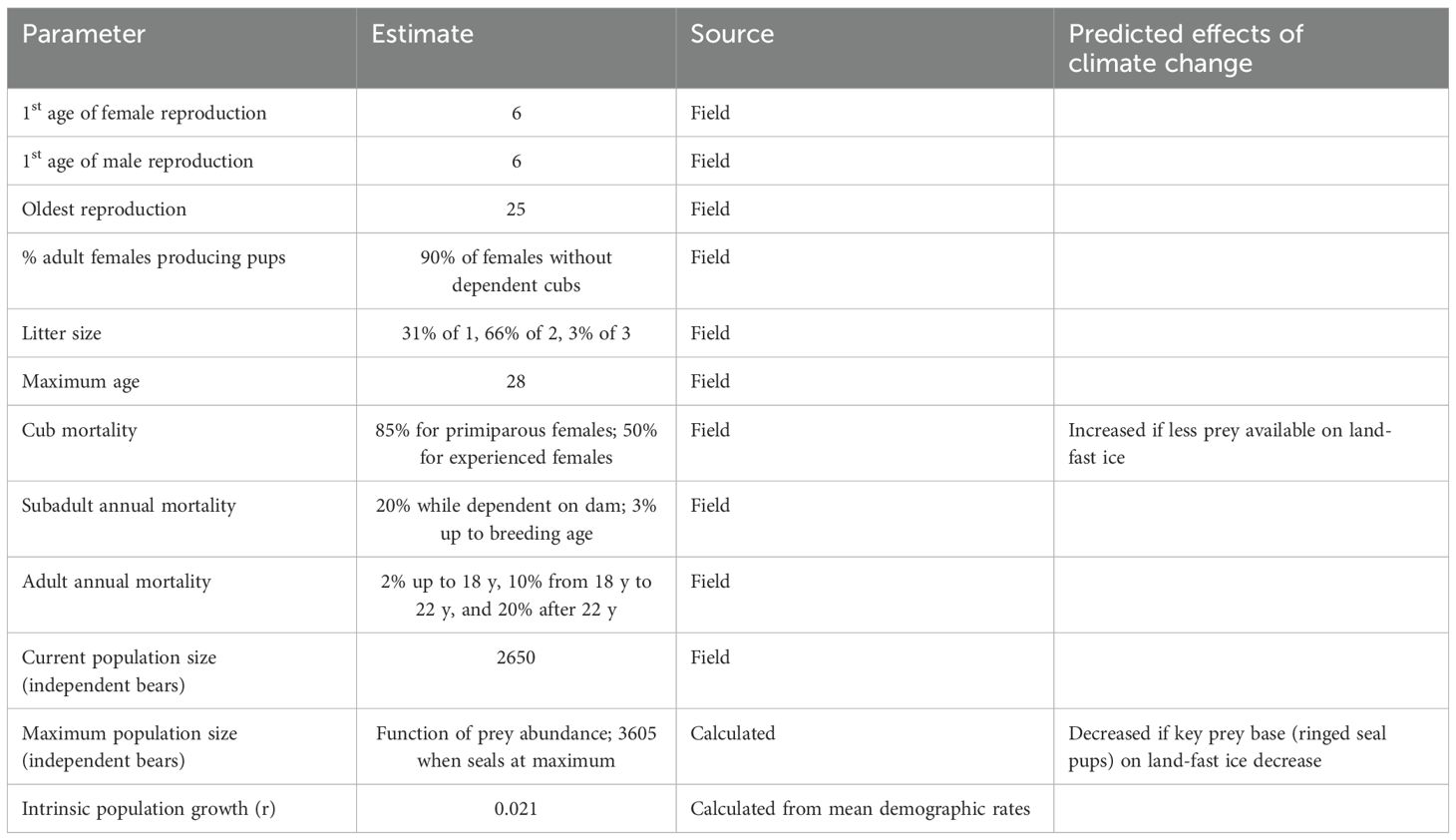
Table 3. Key demographic parameters used to model polar bear population in the northern Barents Sea.
2.1.4 Interactions between the species
Figure 1 illustrates linkages between the species that we considered within the metamodel. Increases in stocks of prey increase the total prey available to polar bears and thereby increases the carrying capacity for bears (K-adults) but decreases the relative predation (having a positive impact) on each prey type because that prey becomes proportionately less of the prey base for the bears. An increase in the bear population increases predation on all of the prey species (although “other” prey in our model is assumed to be a constant, being a range of individually minor prey species that are not themselves strongly affect by polar bear predation). The number of bear cubs that can be reared (K-cubs) is positively related to the number of ringed seals on the land-fast sea ice, while the number of ringed seal pups that can be raised on the land-fast sea ice (K-pups) is dependent on the springtime ice and snow cover (Kovacs et al., 2024). The numbers of ringed seals in each of the two subpopulations receives augmentation from immigrants dispersing from the other population (but in turn is reduced by the number of emigrants). Ice cover around the archipelagos is modeled as a direct effect only on the number of ringed seal pups that can be raised on the land-fast sea ice but has indirect impacts on other parts of the system through the various interdependencies.
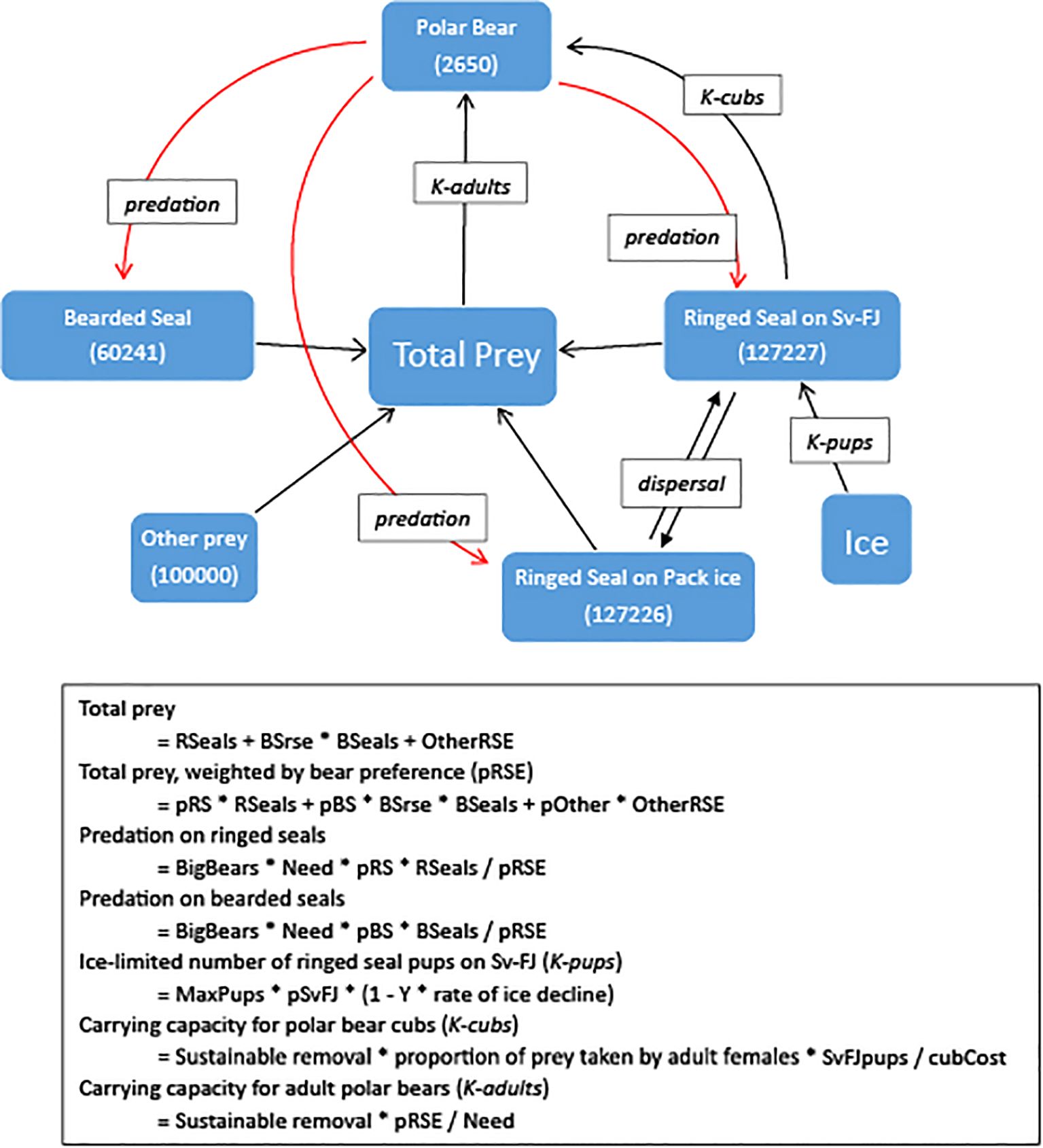
Figure 1. Linkages between components of this predator-prey system considered within the metamodel. Starting values for each population are given in parentheses. Black and red arrows representing positive and negative relationships, respectively. Italicized text boxes note the mechanisms through which the relationships act, according to equations given below, with parameters “OtherRSE” – other prey, scaled as “ringed seal equivalents” of energy value; “BSrse” – energetic value of a bearded seal relative to a ringed seal; “pRS”, “pBS”, and “pOther” – proportional predation by polar bears, relative to abundance of prey species; “BigBears” – Polar bears independent from dam; “Need” – Polar bear energetic need (43.5 RSE/y); “MaxPups” – number of ringed seal pups produced when seal population is at carrying capacity; “pSvFJ” – proportion of ringed seals on Svalbard-Franz Josef; “SvFJpups” – number of ringed seal pups on Svalbard-Franz Josef; “cubCost” – Energy required to raise a cub (7.35 RSE).
To model how the effects of changing sea ice conditions cascade through this predator-prey system, we specified quantitative relationships that describe ways in which the species impact each other and how each species and their interactions depend on the ice. The amount of predation by polar bears on the prey species was estimated from energetic calculations of the prey availability, food value, and energy requirements of the bears. The total available prey to support the polar bear population was estimated by assuming that the prey base is comprised of the ringed seals (the primary prey), bearded seals (less abundant prey), and all other prey (harp seals (Pagophilus groenlandicus) and other seals (including walruses Odobenus rosmarus), bird eggs (Prop et al., 2015), reindeer (Rangifer tarandus platyrhynchus; Stempniewicz et al., 2021), and whale carcasses (Aars et al., 2015; Laidre et al., 2018)). Mature ringed seals are 50-100 kg (Kovacs et al., 2021b); while an adult bearded seal is about 250 kg (Andersen et al., 1999). However, bears prey on young seals more heavily than adults. At birth, ringed seals average 4.5 kg, and they grow to 20 kg by the time of weaning at six weeks of age (Lydersen and Kovacs, 1999). Bearded seals average 38 kg at birth, and they grow rapidly to 80-120 kg when weaned at three weeks of age (Kovacs et al., 2020). Without knowing precise proportions of seals preyed upon at each age for each species, we cannot determine the relative energy value of an average ringed seal vs bearded seal eaten. However, based on average sizes of the two species, we assumed that the average bearded seal taken by a polar bear is 8 times larger than the average ringed seal that is taken. We also tested some scenarios with a 5:1 ratio of energy value of bearded to ringed seals (see Sensitivity analyses, below).
Other prey species are individually much less abundant in the diet of polar bears and typically provide much less energy to bears. However, collectively and over the entire year they might provide an amount of food equivalent to that which the bears derive from predation on ringed seals. For the model, it was assumed that these other food sources would remain stable through the model run time frames.
Reports of polar bear diets suggest that proportions of various prey species vary by location, season, and for individual bears. Derocher et al. (2002) reported a distribution of 63% ringed seal, 13% bearded seal, 8% harp seal, and 16% unknown species (with a biomass distribution of 30% ringed, 55% bearded, and 15% harp seals) in the diet of polar bears in Svalbard and the Barents Sea. Iversen et al. (2013) reported that polar bear scats in Svalbard contained mostly ringed seal pups, but bearded seals might be a much larger part of the diet of polar bears feeding offshore (not sampled in that study). We assumed that polar bears prey on ringed seals, bearded seals, and other prey in proportion to their availability. Because bearded seals (at the ages taken by polar bears) are larger than ringed seals, but about 25% as abundant, this means that polar bears would take ringed seals about 4x as often as bearded seals, but the polar bear diet would consist of twice as much biomass of bearded seals compared to ringed seals. With estimated current abundances of adults and annual production of pups, the dietary biomass distribution at the outset of our simulation would be 30% ringed seal, 58% bearded seal, and 12% other prey, closely corresponding to the estimates of Derocher et al. (2002). If we estimate a lower relative energy value for bearded seals (e.g., 5-fold more than ringed seals), the proportion of each prey in the diet would remain the same, but more of each prey species would need to be consumed to meet the energetic needs of the bears, and the distribution of biomass would shift (e.g., to 39%, 46%, 15%). We also tested a few models in which we specified a greater preference for ringed seals (see Sensitivity analyses, below), which shifts both the numeric distribution and biomass distribution of prey consumed.
In order to determine how many polar bears (K) can be supported by the prey base, and how many ringed seals and other prey species are killed annually by the bears, it is necessary to estimate the energetic requirements of the polar bear population. The energy needs for an “average bear” (range 5-500 kg, young, subadults, adult females and adult males) per year was approximated based on a simplification of Stirling and Øritsland (1995) calculations, using a mass of circa 200 kg. The basal metabolic rate (BMR) estimated according to Kleiber (1975) is 70 x BM^0.75 = 3.72 Mcal/day (15.6 MJ/day). The field metabolic rate (FMR) of free-living animals is generally somewhere between 2-4 times the BMR (Nagy, 1987). Polar bears are able to conserve energy in periods with little food by lowering their metabolic rate, and FMR was thus set to 2 x BMR. (See Stirling and Øritsland (1995) for discussion of this topic). Thus, the annual energy requirement for the average polar bear would be 3.72 Mcal/day x 2 x 365 = 2 715.6 Mcal/year (11 362 MJ/y). According to Stirling and Øritsland (1995) an adult ringed seal provides about 150 000 kcal of energy for a polar bear, subadults provide about 50 000 kcal, and pups provide about 10 000 kcal for the first two weeks after birth, 50 000 kcal for the next two weeks, and about 100 000 kcal thereafter. If the proportion of kills are 50% pups under two weeks of age, 30% 2-4 weeks, and 20% older, then the average ringed seal pup provides about 40 000 kcal to a bear. Polar bear predation on ringed seals was estimated to be about 56% on pups, 26% on 1-2 y subadults, and 18% on adult-size seals by Stirling and Øritsland (1995). Therefore, the average ringed seal killed provides 62 400 kcal (261 MJ), and a bear needs to kill 43.5 such “ringed seal units” to meet its annual energy requirements. Adult male polar bears weigh about twice as much as females, but without dependent cubs - males might travel farther in search of prey. If our calculations underestimate the average energy requirements for adult bears, we might be overestimating the number of bears that can be supported by the prey base. If so, the upper limit on the number of polar bears projected in the early years of our models (before there is significant loss of sea-ice) would be less, and the subsequent decline in polar bears as ringed seal populations on the archipelago decline would be accelerated. The metamodel parameters defining the predator-prey linkages are summarized in Table 4.
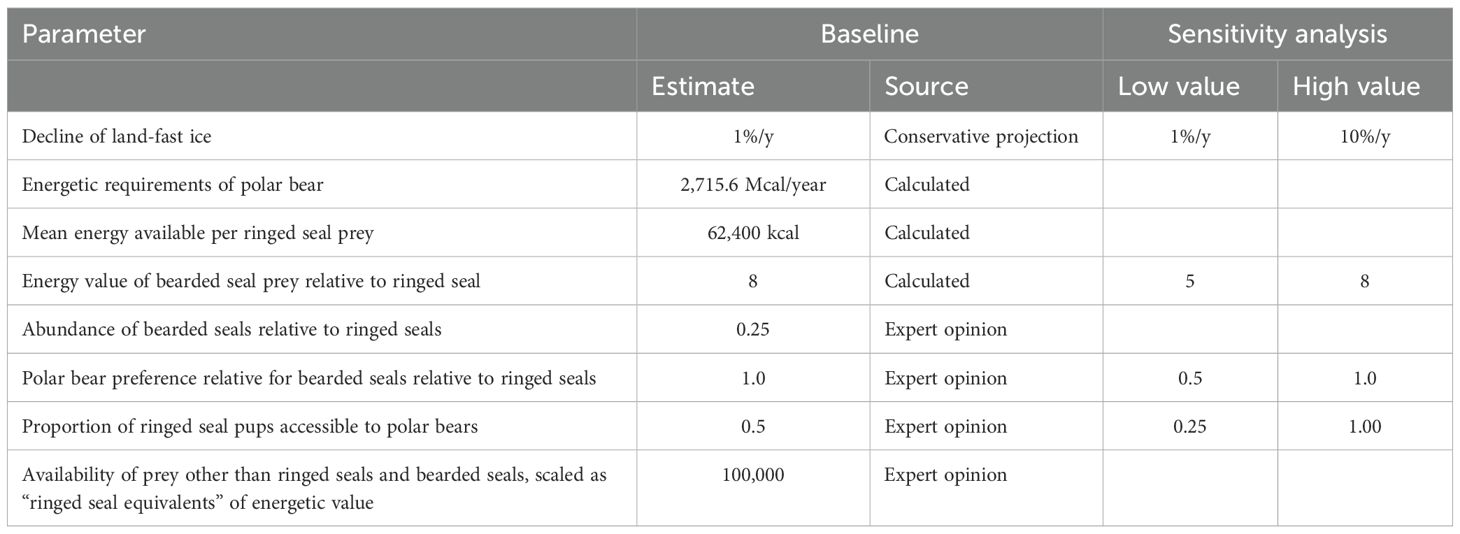
Table 4. Metamodel parameters defining predator-prey linkages, with best estimates as used in Baseline model and ranges evaluated in sensitivity analyses.
In each year of the simulation, the total prey consumed by bears was calculated from the number of independent bears and the energy requirements per bear, and this predation was allocated among the three types of prey based on the relative availability of their biomass. Predation was further allocated among the age classes of ringed seals and bearded seals. For ringed seals, bear predation has been reported (Stirling and Øritsland, 1995) as 56% on pups, 26% on 1–2-year-olds, and 18% on adults. For bearded seals, lacking field data, we assumed that predation is distributed as 30% on pups, 15% on 1–2-year-olds, and 55% on adults, because bearded seal pups are able to escape predation at an earlier age than are ringed seals.
The critical dependency of female polar bears with cubs-of-the-year on the availability of ringed seals in the breeding areas on the land-fast sea ice around the archipelagos when the bears emerge from the den was modeled by assuming that the number of bear cubs that can be raised each year is limited by the availability of ringed seals on the land-fast sea ice at this time of the year. We assumed that 50% of the ringed seal pups were accessible to bears. If this proportion was not taken, polar bear cub survival declined because of the lower prey capture rate. Other values for this limiting of the harvest rate were tested during sensitivity analyses (see below). The ringed seal population model (with the parameter values given above) estimates that currently 29% of ringed seal pups are killed by bears. We also assumed that half of the predation on ringed seals would be by bears other than females with cubs. To raise cubs, a female bear needs to kill on average 12.5 ringed seals, which is 7.35 ringed seals per bear cub (with a mean litter size of 1.7). We therefore set the number of bear cubs that could be raised each year to be no more than the number of ringed seal pups in Svalbard and Franz Josef Land x maximum proportion harvested (0.5) x proportion killed by females with cubs (0.5), divided by the requirement for 7.35 seals/bear cub. Female polar bears that are raising cubs prey on all ringed seal age classes, but we assume that without the available ringed seal pups in breeding areas, the bears could not get enough high energy food when they emerge from dens in the spring to be able to successfully raise their cubs. Thus, survival of polar bear cubs can be estimated as a function of the abundance of ringed seal pups on the land-fast sea ice. At the outset of the simulation, this equates to a maximum of 926 cubs that female bears can raise in Svalbard and Franz Josef Land. Given the age structure and reproductive rates described above, the current bear population would be producing an estimated 609 cubs per year, indicating that in our model the availability of ringed seal pups is not currently a limiting factor restricting the number of bear cubs surviving. The limitation on the number of bear cubs if the ringed seal population declines in the future was modeled by specifying that when the maximum number of cubs for a year was exceeded by births, then cub survival was decreased proportionately to the excess of cubs born.
In models projecting sea ice cover over time, it was assumed that reduced pupping habitat for ringed seals in Svalbard and Franz Josef Land reduces the number of ringed seals pups available to mother bears emerging from their dens with cubs. It was assumed that the reduction in ice cover has no impact on the subpopulation of ringed seals that gives birth on the pack ice (at this point in time). In addition, it was assumed that the total carrying capacity for adult ringed seals was not diminished by the loss of ice.
Although the decline in the ringed seal subpopulations that pup on the fjord ice of Svalbard or Franz Josef Land could perhaps have been modeled as a direct consequence of polar bears being able to prey on more of the pups if they become more densely packed under reduced sea ice conditions, rather than being projected to decline linearly with ice cover, other species such as arctic foxes (Vulpes lagopus) and glaucous gulls (Larus hyperboreus) also exploit the easy availability of ringed seal pups when ice and snow conditions do not provide an opportunity for ringed seals to create lairs on the ice to protect the pups (Lydersen and Smith, 1989). Without knowing the details of the feeding behaviors and abundances of these other predators, mechanistic models of the causes of decline for ringed seals are not possible; the critical dependency of ringed seals on the ice for reproduction was thus modelled simply with the local carrying capacity being linearly related to the ice cover available for raising pups.
2.1.5 Projections of changing ice cover
Seasonal minima and maxima projected for large regions from global climate models provide the most robust predictions of average changes to Arctic climate (IPCC, 2023). However, for the polar bear – ringed seal relationships around Svalbard, what matters is the extent of land-fast sea ice cover in April (when seals have pups and polar bears have emerged from dens with cubs), and the snow cover that has accumulated on that ice (Hezel et al., 2012). Sea ice on the Barents Sea in April declined by about 13.4% per decade from 1979 to 2021 (Isaksen et al., 2022) and Hezel et al. (2012) project a 70% decline in snow-covered ice adequate for ringed seal lairs by 2100. We therefore initially tested the impact of a conservative, linear 1% annual decline in the springtime land-fast sea ice in our models. However, documented increases in the rate of change of Arctic sea ice, along with increasing water temperatures led us to explore faster rates of decline as well, projecting impacts of sea ice declines up to 10% per year (i.e., complete loss of sea ice over the next decade.
2.1.6 Metamodeling approach
The three PVA models were created in the Vortex software, and we examined the projections for each species to verify that population growth rates and age structures were consistent with the species biology when initial baseline values for the abundances of the other species were entered as fixed parameters. The three PVAs were then linked into a metamodel via the MetaModel Manager software (Lacy et al., 2013; Pollak and Lacy, 2020; program and source code available at https://scti.tools/metamodelmanager). The functional relationships connecting the species were specified in the three Vortex PVA models via state variables that track changing parameters (such as prey abundances) and derived variables (such as the maximum number of cubs that can be raised), which are in turn used in functions that specify demographic rates (such as cub survival). The current value of each state variable during the simulation was shared among the three PVA models via the MetaModel Manager program, with specification that in each year of the simulation control would cycle among the PVA models in the sequence ringed seal, bearded seal, and then polar bear. For example, in each year of the simulation, the ringed seal PVA model would set a state variable for the number of pups born on the land-fast sea ice, and this variable would be passed to the other PVA models so that it could be used as a determinant of bear cub survival that year. In data flow back to the seal PVA models, the bear PVA would set a state variable calculating the number of independent bears each year, and that variable would then be used in the ringed seal and bearded seal PVAs to determine the number of each species killed by the bears.
Sensitivity analyses were run to explore plausible alternative parameters describing the interactions among the species, including: higher or lower rates of movement of ringed seals between breeding on the land-fast sea ice and the pack ice; greater preference of polar bears for ringed seals; reduced average food value of bearded seals; changed proportion of ringed seal pups available to the bears; and annual rates of decline of land-fast sea ice from 1% to 10%.
Each scenario was simulated for 100 years, and simulations were each repeated 100 times to obtain estimates of means and SD for the population size over time. All input files for the three Vortex models and MetaModel Manager are available on the Zenodo repository at DOI 10.5281/zenodo.11265382. Notes included in the Vortex input files and provided in the Supplementary Material provide further explanations of model structure and parameter estimates.
3 Results
3.1 Baseline model
In the absence of any changes to the springtime ice cover, the metamodel generates predator-prey dynamics that are consistent with the existing populations and their recent trajectories (Figure 2). The seal populations are able to support the polar bear population. Within 5 to 6 years, the ringed seals and bearded seal populations grow to their carrying capacities, set at 125% of the initial population sizes, with growth rates in the first few years of 4.3% and 4.7%, respectively. After a small initial decline while the metamodel dynamics modified the starting age structure, the polar bear population was also projected to grow, although at a slower rate (about 1.3%) than the prey populations. After about 20 years, the bear population had increased by about 30%, and the seal populations consequently began to decline slightly. After about 50 years, all three species stabilized at numbers above the starting sizes, with prey populations about 18% to 21% above initial sizes, and the polar bear population about 50% above its initial size. The projections showed fluctuations around these predator-prey dynamics, with predicted sizes that varied with a SD across independent iterations of about ± 10% of the means.
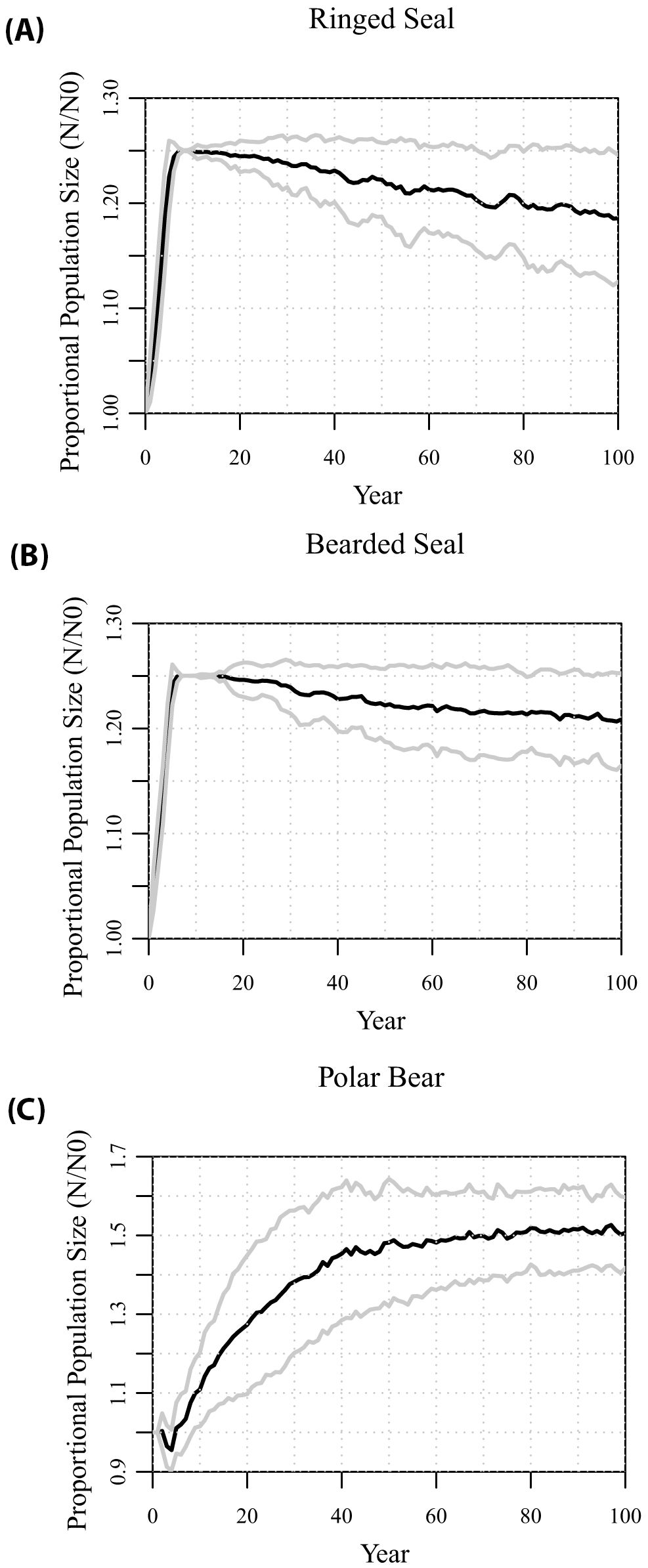
Figure 2. Projections of population sizes relative to starting numbers of (A) ringed seals, (B) bearded seals, and (C) polar bears from 100 iterations – with an assumption of no decline in land-fast sea ice. Gray lines show variation across iterations as ± 1 SD.
3.2 Sensitivity analyses of metamodel parameters in the absence of climate change
Alternative values for a number of the uncertain model parameters, which describe the linkages between the species, were tested. Scenarios with either 0% or 10% annual dispersal of subadult ringed seals between the population breeding on the land-fast sea ice and the population on pack ice generated mean trajectories that were virtually indistinguishable from the baseline metamodel above (results not shown). This occurred because in the absence of a decline in ice cover, the population of ringed seals on the land-fast sea ice grows to, and remains near, carrying capacity even with increasing polar bear predation (see above).
If polar bears are assumed to prey preferentially on ringed seals, relative to their abundance, then the predator-prey dynamics would be projected to be unstable, with ringed seals unable to sustain the initial predation rates, and polar bears subsequently also declining after the prey base is diminished (the lines showing shallower or steeper declines for scenarios “BS preference = 0.75” and “BS preference = 0.50”, respectively, in Supplementary Figures S1A, S1C). This suggests that scenarios with a reduced preference (or ability) by polar bears to prey on bearded seals relative to ringed seals are not good representations of the system, because ringed seals, bearded seals, and polar bears do currently co-exist in the Barents Sea. Other parameter values examined in the sensitivity analyses all resulted in stable predator-prey dynamics after initial growth phases, although the initial growth of the bearded seal population was slower when they provide less energy value to the bears, as a consequence of the bears taking more seals to meet their energy needs (see Supplementary Figure S2B), and the final population size of the bears was less if they could effectively access a smaller percent of the ringed seal pups or if bearded seals provided less energy (Supplementary Figures S2C, S3C). Small differences among scenarios in the final population sizes of the seals were as expected: both prey species remain closer to their carrying capacities in the two scenarios in which polar bears are less abundant because of more limited availability of prey (“BS = 5 RSE”, Supplementary Figure S2; “25% RS pups accessible” Supplementary Figure S3). There was moderate variation among the independent iterations for those scenarios in which the populations did not remain near their carrying capacities (see 90% confidence intervals in Supplementary Figures S1–S3), but the baseline model showed stable population sizes with minimal variation across iterations. These sensitivity analyses verify that the baseline model provides a reasonable scenario that represents the predator-prey dynamics better than models with some plausible alternative estimates of the species interactions.
3.3 Population projections in scenarios with declining ice cover
With a conservative, projected 1% annual decline in land-fast sea ice in April, the ringed seal population in Svalbard and Franz Josef Land initially starts to grow but after a decade enters a decline (Figure 3A). Consequently, the polar bear population is projected to start to decline from its peak size after about three decades (Figure 3C). The decline in the bear population lags behind the decline in ringed seals by a few decades, because the impact of declining ice is on ringed seal recruitment rather than on adult survival, and the subsequent impact of declining ringed seal pups is on bear recruitment. In the decades around the peak bear numbers, the ringed seals on the pack ice (not shown) and the bearded seals (Figure 3B) show small temporary declines, as a result of the still high levels of bear predation. After the polar bears begin to decline, then the bearded seals recover and remain near carrying capacity.
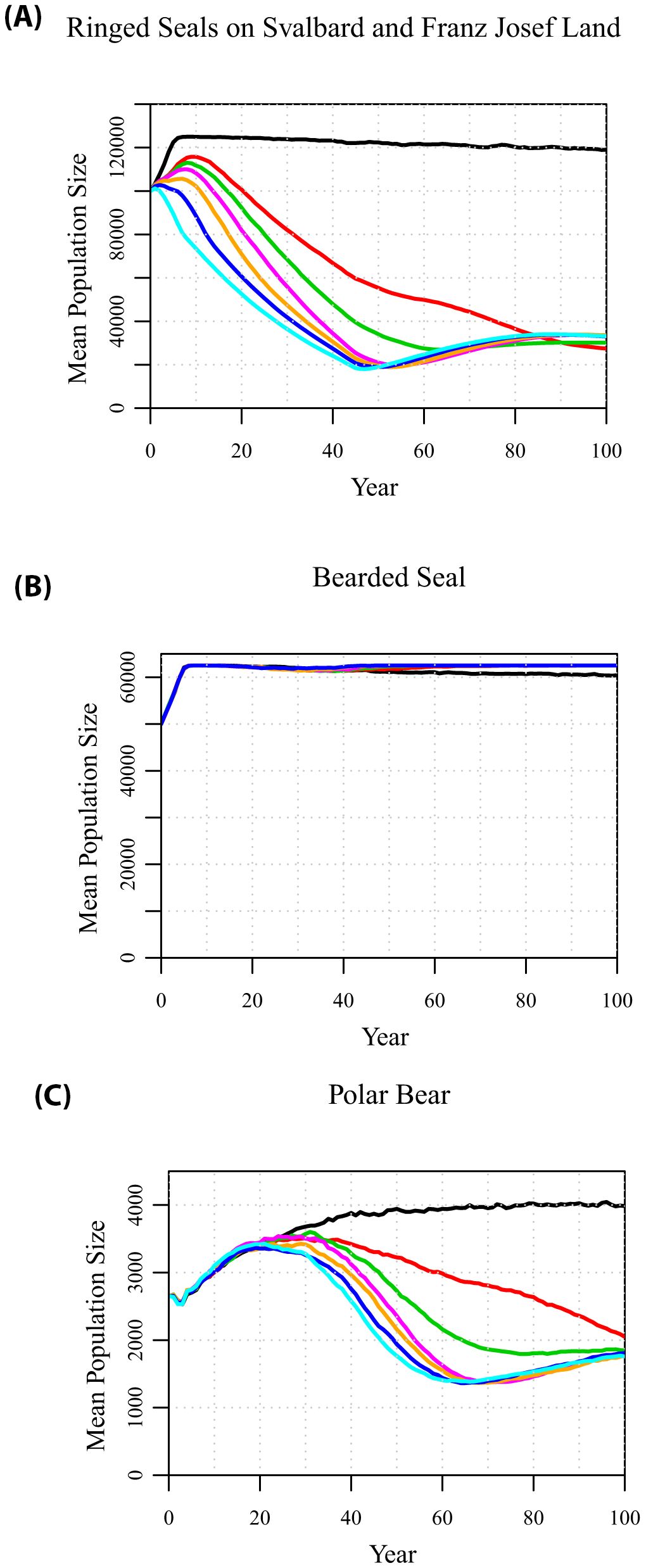
Figure 3. Projections of population sizes of (A) ringed seals in Svalbard and Franz Josef Land, (B) bearded seals, and (C) polar bears, when there is a 0%, 1%, 1.5%, 2%, 3%, 5%, or 10% annual decline (from top to bottom) in the springtime land-fast sea ice.
If sea ice cover declines are more similar to current expectations, occurring at rates of 1.5% to 3% per year, the decline in ringed seals, followed by decline in polar bears, occurs more quickly and to a greater extent. After 50 to 60 years, by which time there would be little or no spring land-fast sea ice around the archipelagos, the ringed seal population around Svalbard and Franz Josef Land hits a minimum at about 20% of its initial size. The polar bear population hits its minimum about a decade later, and this allows the ringed seal population to recover slightly. This remnant population of ringed seals persists because the initial model assumes that each year 5% of subadult ringed seals from the pack ice subpopulation move down to Svalbard or Franz Josef Land. Thus, the pack ice subpopulation of ringed seals remains near carrying capacity, while the steady flow of ringed seals moving down to the archipelagos provide an ongoing (although much reduced) annual production of ringed seal pups, which provides food for polar bears emerging from dens with cubs. Even higher rates of ice loss, 5% to 10% per decade (i.e., complete loss of land-fast sea ice on the fjords in 20 or 10 years), lead to only marginally faster declines in ringed seals (Figure 3A) and polar bears (Figure 3C), due to the assumed immigration from the pack ice population of ringed seals sustaining a remnant population of polar bears.
As springtime ice cover on the fjords of the archipelagos diminishes, it would become maladaptive for ringed seals to move from the pack ice into the declining population near the coast, where they have no prospect of experiencing conditions necessary to rear pups. If there is no dispersal of ringed seals from the pack ice to Svalbard and Franz Josef Land, then the decline of ringed seals on the land-fast sea ice and of the polar bears that depend on them accelerates (Supplementary Figure S4). At higher rates of loss of spring sea ice (e.g., 2 to 3% per year), the ringed seal population breeding around the archipelagos disappears after 55 to 65 years if it is not continually replenished from the population breeding on the pack ice, and extirpation of the polar bear population denning and breeding on the archipelagos follows about 20 years later.
To explore further the sensitivity of the collapse of the seal and bear populations to immigration of ringed seals from the pack ice, Supplementary Figure S5 compares mean population trajectories for the scenarios with a 2% annual decline in sea ice cover and dispersal of subadult seals between the pack ice and the archipelagos at annual rates varying from 0% to 5%. Even a low rate of movement of pack ice seals into the archipelagos to attempt to breed there (although without any success after the spring land-fast sea ice cover is completely gone) is projected to sustain a small breeding population of polar bears. However, this projection is dependent on the assumptions that the alternative prey base will continue to be sufficient to support the adult bears.
4 Discussion
Ringed seals are key prey for polar bears and human inhabitants in coastal Arctic communities across the circumpolar Arctic. Their range extends further north than most other seals because they can maintain breathing holes through quite thick sea ice. However, they are dependent on quite specific ice and snow conditions in the spring in order to construct snow lairs in which they rear their pups. They are therefore highly vulnerable to the ongoing declines in sea ice in the Arctic (Laidre et al., 2008; Kovacs et al., 2011, 2012, 2021a, 2024; Reimer et al., 2019). Bearded seals are another important Arctic seal species throughout the circumpolar Arctic, that is a favored hunting target for some Alaskan communities and a significant contributor to polar bear diets (Derocher et al., 2002; Nelson et al., 2019). Bearded seal pups are large at birth, and swim within the first hours of life, making them somewhat less accessible to polar bears than ringed seal pups (Lydersen et al., 1994; Lydersen and Kovacs, 1999; Kovacs et al., 2020), and adults spend relatively little time hauled out, reducing their availability to polar bears somewhat (Hamilton et al., 2017). Because bearded seals forage on a wide variety of prey species, will more readily haul out on land, and have pups large enough to use glacier ice pieces (or potentially land), they might have more climate resilience than ringed seals, though they are still likely to be negatively impacted by global warming (Kovacs et al., 2020). There are few data available to examine the changes that are occurring in population trends for these seals species, so we have used modelling forecasts herein, combined with similar projections to follow their principal predator, the polar bear in the Barents Region.
Female polar bears are dependent on finding high calorie, easily accessible prey quickly after den emergence in order to recover fat reserves lost during the fasting period in the den and to fuel increased milk production for the active, growing cubs when they shift out of the den (Archer et al., 2023). Females with cubs of the year are not free to swim in open-water or loose ice areas, so not surprisingly they focus their hunting effort in areas with land-fast sea ice where ringed seals lairs are most dense, such as at glacier fronts in Svalbard (Freitas et al., 2012; Lydersen et al., 2014). Alternative prey, such as bearded seals, probably cannot fill the critical energetic needs in the early spring when females have very young cubs. Spring feeding on ringed seals is also important to other polar bears (males and juveniles) that acquire most of their annual energy intake at this time (Stirling and Øritsland, 1995). Later in the season other prey can be utilized (Hamilton et al., 2017), although the energetic values of birds, reindeer and other terrestrial prey is unlikely to fully compensate for the loss of ice-seals from the polar bear’s diet (Whiteman et al., 2017; Hunter et al., 2010; Pagano et al., 2024).
In this study we used multiple Population Viability Analysis models linked into a metamodel to examine the inter-dependencies of ringed seals, bearded seals, and polar bears in the Barents Region and to project potential impact scenarios of changing sea ice on this predator-prey system. To build these models, estimates of detailed demographic parameters on each species were required in addition to understanding the constraints on how the species interact, data on the energetic values of prey and requirements of the predator, and projections of changing sea ice conditions. Data are not available to provide accurate estimates for all of the demographic rates needed for these three arctic species, so informed estimates were often used as inputs to our models. Moreover, the impacts of changing climate on these species will almost certainly include additional processes/stressors that we have not modeled. Even so, the dynamics revealed in the metamodel of a predator-prey system in which the relationships among species are strongly determined by the sea ice conditions in the Arctic likely do represent reasonable approximations of the impacts that can be expected. The projected trends indicate that currently abundant species are likely to be highly vulnerable to effects of climate change and experience significant declines. Our metamodel also provides a framework for including new data into future projections of climate change impacts as they become available.
The most dramatic and easily intuited prediction from our metamodel is that as the sea ice conditions that allow ringed seals to raise pups in snow-covered lairs on the frozen fjords of Svalbard and Franz Josef Land decline or even disappear (see Kim et al., 2023) our marine mammal populations will be negatively impacted. Ringed seal populations will collapse due to lack of successful recruitment. Consequently, the polar bear populations that depend on ringed seal pups to be able to raise their own offspring are likely to follow similar trends. Dispersal of ringed seals from the pack ice might slow extirpation of coastal subpopulations, with the ringed seals that use land-fast sea ice becoming demographic sinks rather than the strongholds for the species that they have been in the past. Interestingly, our models suggest that the populations of ringed seals using drift ice, and bearded seals, are projected to maintain slightly larger population sizes in the long-term, because the decline in the regional population of polar bears reduces predation pressure. However, our models do not reflect the on-going reductions in drift ice, which we fixed as a constant, although this ice has become thinner, more fractionated, more mobile, and less extensive (Isaksen et al., 2022). In the coming decades remaining sea ice in the Arctic will largely occur over deeper, off-shelf waters. Although there are signals of increasing primary production in the Arctic Ocean, the deep ocean is less productive than the Arctic shelf seas (Ardyna and Arrigo, 2020).
Although the directions of change to the populations modeled herein have been predicted for some time (e.g. Tynan and DeMaster, 1997; Laidre et al., 2008; Durner et al., 2009; Stirling and Derocher, 2012) the dramatic magnitudes of the estimated changes are perhaps surprising. Our estimated timeline is almost certainly overly optimistic given that the rate of sea ice decline has accelerated over recent years in the Barents Region and drift ice, similar to land-fast sea ice, has declined markedly (Isaksen et al., 2022; Urbanski and Litwicka, 2022). Land-fast sea ice coverage in open fjords in western Svalbard collapsed in 2006 and has not recovered since; sill fjords still retain more ice, but there are fewer tide-water glaciers calving pieces onto the annual ice and less precipitation in winter comes as snow (there is more rain) so there are fewer drifts even on available ice. The predicted declines in ringed seals and bearded seals will almost certainly lead to a collapse of polar bears in the Barents Sea area. At very least the remaining polar bear population will be smaller than current numbers (Aars et al., 2009, 2017) despite the various foraging and distributional plasticity being shown currently by the polar bears in the region (Prop et al., 2015; Hamilton et al., 2017; Bengtsson et al., 2021; Stempniewicz et al., 2021).
Our metamodel also shows that with long-lived species, impacts that reduce reproductive success, even to almost zero, might not be easily observable from census data on adults until a decade or more after climate changes have in fact disrupted the community. Field monitoring of demographic changes combined with models that project the consequent population dynamics will be required to reveal the full scope of the consequences of climate change impacts that are already underway. Our models project an initial rise in both predator and prey populations, for about a decade, as a consequence of reduced hunting compared to the historical situation. The subsequent decline in adult ringed seals becomes noticeable only in the second decade after the start of the steady decline in sea ice on which they depend; this time frame is actually at-hand given that in hind-sight west coast sea ice in Svalbard collapsed in 2006 (Vihtakari et al., 2018). The polar bear population would not be expected to enter into decline until the lack of recruitment of ringed seals results in the depletion of breeding aged ringed seals – starting in the third decade and dropping below the initial population size only after about 50 years. Indeed, the lack of suitable ice and snow conditions for ringed seal lairs might result in polar bears being able to prey on ringed seals in birthing areas more easily than in the past initially, because of increased densities of seals in the remaining land-fast sea ice areas (Rosing Asvid, 2006). Hunting success by polar bears will not decline until there are fewer seal pups in lairs and on open ice around the archipelagos.
Although lags between climate change and impacts on the fauna are expected, the sea ice conditions have already changed dramatically around Svalbard, and the collapse of this Arctic species assemblage might already be underway. Projecting the changes occurring to seals and bears in the broader Barents Sea Region is difficult because large-scale models of average ice cover in the Arctic do not provide the necessary specificity about the local ice (and snow) conditions that will matter most to the fauna. For ringed seal-polar bear dynamics, the critical ice environment is the extent of ice on the fjords in April and the snow cover over that ice (Kovacs et al., 2024). For other species, different but equally specific environmental conditions might be critical. The results of the sea ice in the Pacific Arctic retracting over the deep Arctic Ocean have been seen in vast changes to distribution of walruses, particularly mothers and calves. Mothers normally stayed on the off-shore ice through the summer, but with the retreat of the sea ice northward into the deep Arctic Ocean, females now use land-based haul-out sites along the coasts of Alaska and Russia (Jay et al., 2012). Stampedes in these new, mixed sex, high density haul-outs herds are resulting in increased calf mortality at levels that can negatively impact population demographics (Udevitz et al., 2012).
Although the general trends that we project are likely indicative of major changes to the ice dependent fauna of the Arctic, we acknowledge that the extent and rate of changes will be dependent on a number of factors that are as yet poorly known. Some of the key uncertainties about the system include: do individual ringed seals switch between breeding on land-fast sea ice and breeding on pack ice; are there two distinct breeding strategies such that the dynamics of the two subpopulations are largely independent; do polar bears have flexible enough prey selection to find alternative high-energy food adequate to raise cubs; how do changing ice conditions affect bearded seals; and are there interactions with other species in the system that can play key roles? Additionally, increased distance between land and the northern ice may have some implications for polar bears that have not been directly addressed in this study. Polar bears in the Barents Sea area depend on sea ice bridges providing access to denning areas on land during the fall, for females to give birth in mid-winter. Merkel and Aars (2022) showed that denning habitat is becoming less available (shown earlier by empirical capture data, Derocher et al., 2011) because sea ice no longer forms around islands in time to provide easy access to land. It is clear that there are already changes in bear distribution given that Maduna et al. (2021) described how genetic variability has decreased in Svalbard in recent years, as sea ice connecting the islands with the ice edge has been reduced, creating metapopulation structure that was not seen 30 years ago in this polar bear population.
We emphasize that the predictions arising from our metamodel are contingent on other aspects of Arctic community-function not being disrupted. We modeled only three of the ice-dependent Arctic species, we examined only the populations in the northern Barents Sea region, and we projected the impact of only one aspect of the sea ice environment (ice extent). Changing climatic conditions will undoubtedly have many more effects on these species than we have explored – due to interdependencies on other species, other features of the physical environment (freshening of fjords from glacial and riverine run-off), increasing acidification of Arctic water masses and other aspects of the species’ biology. For example, the ringed seals mating system has been structured around males defending areas under the ice where several females have their (multiple) lairs. If the ice is not stable through the nursing period, it is likely that females will be much more mobile, with unknown effects on breeding behavior and pregnancy rates.
Changes to ice conditions are expected to cause cascading impacts throughout Arctic food webs, and we have not modeled possible effects of changing availability of prey for the seals. Freitas et al. (2008) suggested that foraging within drift ice areas north of Svalbard might not be energetically profitable for coastal ringed seals when sea ice retreats more than 700 km north of the islands in the archipelago. If this is true, and all ringed seals must feed in coastal areas, prey availability might become a limiting factor. Coastal ringed seals already show signs of retracting into very small refugia areas near tide-water glacier fronts (Hamilton et al., 2019), likely tracking their favorite food polar cod (Boreogadus saida) (Bengtsson et al., 2021). This small Arctic fish is already in decline in the Barents Sea area (ICES, 2018), likely because of Atlantification of the Barents Sea system (Fossheim et al., 2015). Other predators might also become more important, particularly the killer whale (Orcinus orca), which has already extended its range northward in the Northwest Atlantic Arctic (Higdon et al., 2014). New or increased competition from subarctic and temperate marine mammal species shifting their distributions northward are also a concern (Kovacs et al., 2011). However, some of these species may also contribute to alternative food sources for polar bears (which in our model we assumed to be constant). For example, walrus, harbor seal (Phoca vitulina), and reindeer populations have increased significantly in recent years in Svalbard (Kovacs et al., 2014; Le Moullec et al., 2019). Harbor seals are now hunted by polar bears in Svalbard and this species is definitely a climate winner that is expanding its range on the west coast of Svalbard (Merkel et al., 2013; Bengtsson et al., 2021). Recent data also indicate that bears are better at catching reindeer than was believed previously; they use several different techniques and kills have been documented in several areas of Svalbard (Stempniewicz et al., 2021). An important research need is to determine how flexible polar bears are with respect to switching to other prey species as the Arctic ecosystem changes in response to climate. Further, focus should be on the energetic value of alternative prey and if they can be sufficient to ensure survival and reproduction for Svalbard bears over time, with less availability of sea ice and the ice-associated seals.
Other areas of the Arctic might experience slower changes to the ice-dependent fauna than we predict for the Barents Sea. For example, some areas in Northwest Greenland might retain sea ice in the coming decades (ACIA, 2005). Such areas should be targeted for conservation of Arctic endemic species. The disruption and possible collapse of ecological communities dependent on specific climatic conditions can be expected in many places, even if these changes do not occur as quickly or as dramatically as is likely for ice-dependent species in the Barents Sea.
5 Conclusions
The risks of climate change to species and ecosystems are becoming increasingly well known, but they are still usually described as effects that will likely occur in the future. However, in parts of the Arctic, climate change has already exceeded the limits set by various climate protocols, and impacts on species are already occurring. The ice-dependent fauna of the Arctic is clearly especially vulnerable.
Climate change poses a special challenge to the Red List process used to identify species at risk (e.g., Akçakaya et al., 2014; Foden and Young, 2016). Our modeling suggests that noticeable species declines might not occur until several decades after the environmental changes that set in motion the ultimately threatening processes. Thus, observed population declines might be discovered too late to allow for conservation actions to attempt mitigation.
While the impacts of ongoing climate change on rare species with limited distributions are of concern, the ecological effects that result from collapse (even if not to extinction) of currently abundant species that are keystone species supporting ecological communities might be more pervasive and damaging to entire ecological communities. Multi-species models including predator-prey interactions, competition, and species dependencies are required to predict cascading effects of climate change on ecological communities. As more data become available on the species we modeled, other Arctic species, their interactions, the projections of climate change in the Arctic, and the effects of climate change on species and their inter-relationships, we expect that the metamodel approach we have used herein can serve as a template for further analyses that can generate more accurate and robust conclusions about the threats that climate change poses to species and ecological communities.
Data availability statement
The datasets presented in this study can be found in online repositories. The names of the repository/repositories and accession number(s) can be found in the article/Supplementary Material.
Author contributions
RL: Formal analysis, Methodology, Software, Writing – original draft, Writing – review & editing. KK: Conceptualization, Data curation, Investigation, Writing – original draft, Writing – review & editing, Funding acquisition. CL: Data curation, Investigation, Writing – review & editing, Writing – original draft. JA: Data curation, Investigation, Writing – review & editing, Writing – original draft.
Funding
The author(s) declare financial support was received for theresearch, authorship, and/or publication of this article. This work was funded by the Norwegian Polar Institute and the Norwegian Research Council (ARK - project number 313678).
Acknowledgments
The Vortex and MetaModel Manager software programs were made freely available by the sponsors of the Species Conservation Toolkit Initiative (www.scti.tools). Discussions with colleagues in the IUCN Climate Change Specialist Group (led by Wendy Foden) stimulated the initiation of the study. The authors thank Pedro Duarte for helpful discussions in the early development of the PVA models. Two reviewers made many helpful suggestions that helped us improve the final manuscript.
Conflict of interest
The authors declare that the research was conducted in the absence of any commercial or financial relationships that could be construed as a potential conflict of interest.
Publisher’s note
All claims expressed in this article are solely those of the authors and do not necessarily represent those of their affiliated organizations, or those of the publisher, the editors and the reviewers. Any product that may be evaluated in this article, or claim that may be made by its manufacturer, is not guaranteed or endorsed by the publisher.
Supplementary material
The Supplementary Material for this article can be found online at: https://www.frontiersin.org/articles/10.3389/fcosc.2024.1439386/full#supplementary-material
References
Aars J., Andersen M., Brenière A., Blanc C. (2015). White-beaked dolphins trapped in the ice and eaten by polar bears. Polar Res. 34, 26612. doi: 10.3402/polar.v34.26612
Aars J., Marques T. A., Buckland S. T., Andersen M., Belikov S., Boltunov A., et al. (2009). Estimating the Barents Sea polar bear subpopulation size. Mar. Mammal Sci. 25, 35–52. doi: 10.1111/j.1748-7692.2008.00228.x
Aars J., Marques T. A., Lone K., Andersen M., Wiig Ø., Bardalen Fløystad I. M., et al. (2017). Polar bear population structure and trend in the western Barents Sea. Polar Res. 36, 1374125. doi: 10.1371080/17518369.17512017.11374125
Ahonen H., Stafford K. M., de Steur L., Lydersen C., Wiig Ø., Kovacs K. M. (2017). The underwater soundscape in the western Fram Strait breeding ground of Spitsbergen’s endangered bowhead whales. Mar. Pollut. Bull. 123, 97–112. doi: 10.1016/j.marpolbul.2017.09.019
Akçakaya H. R., Butchart S. H. M., Watson J. M., Pearson R. G. (2014). Preventing species extinctions resulting from climate change. Nat. Climate Change 4, 1048–1049. doi: 10.1038/nclimate2455
AMAP (2021). AMAP Arctic Climate Change Update 2021: Key Trends and Impacts (Tromsø, Norway: Arctic Monitoring and Assessment Programme (AMAP), viii+148pp.
Andersen M., Hjelset A. M., Gjertz I., Lydersen C., Gulliksen B. (1999). Growth, age at sexual maturity and condition in bearded seals (Erignathus barbatus) from Svalbard, Norway. Polar Biol. 21, 179–185. doi: 10.1007/s003000050350
Archer L. C., Atkinson S. N., Pagano A. M., Penk S. R., Molnár P. K. (2023). Lactation performance in polar bears is associated with fasting time and energetic state. Mar. Ecol. Prog. Ser. 720, 175–189. doi: 10.3354/meps14382
Ardyna M., Arrigo K. R. (2020). Phytoplankton dynamics in a changing Arctic Ocean. Nat. Climate Change 10, 892–903. doi: 10.1038/s41558-020-0905-y
Beissinger S. R., McCullough D. R. (Eds.) (2002). Population Viability Analysis (Chicago: Chicago University Press), 577 pp.
Bengtsson O., Hamilton C. D., Lydersen C., Andersen M., Kovacs K. M. (2021). Distribution and habitat characteristics of pinnipeds and polar bears (Ursus maritimus) around the Svalbard Archipelago, based on observations from 2005-2018. Polar Res. 40, 1–20. doi: 10.33265/polar.v40.5326
Blanchet M. A., Primicerio R., Frainer A., Kortsch S., Skern-Mauritzen M., Dolgov S. V., et al. (2019). The role of marine mammals in the Barents Sea foodweb. ICES J. Mar. Sci. 76, i37–i53. doi: 10.1093/icesjms/fsz136
Boyce M. S. (1992). Population viability analysis. Annu. Rev. Ecol. Systematics 23, 481–506. doi: 10.1146/annurev.es.23.110192.002405
Bradshaw C. J. A., McMahon C. R., Miller P. S., Lacy R. C., Watts M. J., Verant M. L., et al. (2012). Novel coupling of individual-based epidemiological and demographic models predicts realistic dynamics of tuberculosis in alien buffalo. J. Appl. Ecol. 49, 268–277. doi: 10.1111/j.1365-2664.2011.02081.x
CAFF (2013). Arctic biodiversity assessment. Status and trends in Arctic biodiversity (Akureyri, Iceland: Conservation of Flora and Fauna).
CAFF (2017). State of the Arctic marine biodiversity report (Akureyri, Iceland: Conservation of Arctic Flora and Fauna).
Cubaynes S., Aars J., Yoccoz N. G., Pradel R., Wiig Ø., Ims R. A., et al. (2021). Modeling the demography of species providing extended parental care: A capture-recapture multievent model with a case study on polar bears (Ursus maritimus). Ecol. Evol. 11, 3380–3392. doi: 10.1002/ece3.v11.7
Dawson T. P., Jackson S. T., House J. I., Prentice I. C., Mace M. (2011). Beyond predictions: Biodiversity conservation in a changing climate. Science 332, 53–58. doi: 10.1126/science.1200303
Derocher A. E. (2005). Population ecology of polar bears at Svalbard, Norway. Population Ecol. 47, 267–275. doi: 10.1007/s10144-005-0231-2
Derocher A. E., Andersen M., Wiig Ø., Aars J. (2010). Sexual dimorphism and the mating ecology of polar bears (Ursus maritimus) at Svalbard. Behav. Ecol. Sociobiology 64, 939–946. doi: 10.1007/s00265-010-0909-0
Derocher A. E., Andersen M., Wiig Ø., Aars J., Hansen E., Biuw M. (2011). Sea ice and polar bear den ecology at Hopen Island, Svalbard. Mar. Ecology-Progress Ser. 441, 273–279. doi: 10.3354/meps09406
Derocher A. E., Wiig Ø., Andersen M. (2002). Diet composition of polar bears in Svalbard and the western Barents Sea. Polar Biol. 25, 448–452. doi: 10.1007/s00300-002-0364-0
De Rovere F., Langone L., Schroeder K., Miserocchi S., Giglio F., Aliani S., et al. (2022). Water masses variability in inner Kongsfjorden (Svalbard) during 2010.2020. Front. Mar. Sci. 9. doi: 10.3389/fmars.2022.741075
Durner G. M., Douglas D. C., Nielson R. M., Amstrup S. C., McDonald T. L., Stirling I., et al. (2009). Predicting 21st-century polar bear habitat distribution from global climate models. Ecol. Monogr. 79, 25–58. doi: 10.1890/07-2089.1
Foden W. B., Butchart S. H. M., Stuart S. N., Vié J.-C., Akcakaya H. R., Angulo A., et al. (2013). Identifying the world’s most climate change vulnerable species: A systematic trait-based assessment of all birds, amphibians and corals. PloS One 8, e65427. doi: 10.1371/journal.pone.0065427
Foden W. B., Young B. E. (Eds.) (2016). “IUCN SSC guidelines for assessing species’ Vulnerability to climate change,” in Version 1.0. Occasional Paper of the IUCN Species Survival Commission No. 59 (IUCN Species Survival Commission, Cambridge, UK and Gland, Switzerland), X+114pp.
Fordham D. A., Akcakaya H. R., Brook B. W., Rodriguez A., Alves P. C., Civantos E., et al. (2013a). Adapted conservation measures are required to save the Iberian lynx in a changing climate. Nat. Climate Change 3, 899–903. doi: 10.1038/nclimate1954
Fordham D. A., Mellin C., Russell B. D., Akcakaya H. R., Bradshaw C. A., Aiello-Lammens M. E., et al. (2013b). Population dynamics can be more important than physiological limits for determining range shifts under climate change. Global Change Biol. 19, 3224–3237. doi: 10.1111/gcb.2013.19.issue-10
Fossheim M., Primicerio R., Johannesen E., Ingvaldsen R. B., Aschan M. M., Dolgov A. V., et al. (2015). Recent warming leads to a rapid borealization of fish communities in the Arctic. Nature Climate 5, 673–677. doi: 10.1038/NCLIMATE2647
Freitas C., Kovacs K. M., Andersen M., Aars J., Sandven S., Skern-Mauritzen M., et al. (2012). Importance of fast ice and glacier fronts for female polar bears and their cubs during spring in Svalbard, Norway. Mar. Ecol. Prog. Ser. 447, 289–304. doi: 10.3354/meps09516
Freitas C., Kovacs K. M., Ims R. A., Fedak M. A., Lydersen C. (2008). Ringed seal post-moulting movement tactics and habitat selection. Oecologia 155, 193–204. doi: 10.1007/s00442-007-0894-9
Gilg O., Kovacs K. M., Aars J., Fort J., Gauthier G., Gramillet D., et al. (2012). Climate change and the ecology and evolution of Arctic vertebrates. Ann. New York Acad. Sci. 1249, 166–190. doi: 10.1111/j.1749-6632.2011.0641.x
Gregory S. D., Brook B. W., Goossens B., Ancrenaz M., Alfred R., Ambu L. A., et al. (2012). Long-Term field data and climate-habitat models show that orangutan persistence depends on effective forest management and greenhouse gas mitigation. PloS One 7, e43846. doi: 10.1371/journal.pone.0043846
Hamilton C. D., Kovacs K. M., Ims R. A., Aars J., Lydersen C. (2017). An Arctic predator-prey system in flux: climate change impacts on space use by polar bears and ringed seals. J. Anim. Ecol. 86, 1054–1064. doi: 10.1111/jane.2017.86.issue-5
Hamilton C. D., Lydersen C., Ims R. A., Kovacs K. M. (2015). Predictions replaced by facts: a keystone species' behavioural responses to declining arctic sea-ice. Biol. Lett. 11, 20150803. doi: 10.1098/rsbl.2015.0803
Hamilton C. D., Lydersen C., Ims R. A., Kovacs K. M. (2016). Coastal habitat use by ringed seals Pusa hispida following a regional sea-ice collapse: importance of glacial refugia in a changing Arctic. Mar. Ecol. Prog. Ser. 545, 261–277. doi: 10.3354/meps11598
Hamilton C. D., Vacquié-Garcia J., Kovacs K. M., Ims R. A., Lydersen C. (2019). Contrasting changes in space use induced by climate change in two Arctic marine mammal species. Biol. Lett. 15, 20180834. doi: 10.1098/rsbl.2018.0834
Hezel P., Zhang X., Bitz C. M., Kelly B. P., Massonnet F. (2012). Projected decline in spring snow depth on Arctic sea ice caused by progressively later autumn open ocean freeze-up this century. Geophysical Res. Lett. 39, L17505. doi: 10.1029/2012GLO52794
Higdon J. W., Westdal K. W., Ferguson S. H. (2014). Distribution and abundance of killer whales (Orcinus orca) in Nunavut, Canada – an Inuit knowledge survey. J. Mar. Biol. Assoc. United Kingdom 94, 1293–1304. doi: 10.1017/S0025315413000921
Hunter C. M., Caswell H., Runge M. C., Regehr E. V., Amstrup S. C., Stirling I. (2010). Climate change threatens polar bear populations: a stochastic demographic analysis. Ecology 91, 2883–2897. doi: 10.1890/09-1641.1
ICES (2018). Interim Report of the Working Group on the Integrated Assessments of the Barents Sea (WGIBAR) Vol. 04 (Copenhagen, Denmark: International Council for Exploration of the Sea), 210. ICES Document CM IEASG.
IPCC (2023). “Summary for Policymakers,” in Climate Change 2023: Synthesis Report. Contribution of Working Groups I, II and III to the Sixth Assessment Report of the Intergovernmental Panel on Climate Change. Eds. Lee H., Romero J. (IPCC, Geneva, Switzerland), 1–34. Core Writing Team. doi: 10.59327/IPCC/AR6-9789291691647.001
Isaksen K., Nordli Ø., Ivanov B., Køltzow M. A. Ø., Aaboe S., Gjelten H. M., et al. (2022). Exceptional warming over the Barents area. Sci. Rep. 12, 9371. doi: 10.1038/s41598-022-13568-5
Iversen M., Aars J., Haug T., Alsos I., Lydersen C., Bachmann L., et al. (2013). The diet of polar bears (Ursus maritimus) from Svalbard, Norway, inferred from scat analysis. Polar Biol. 36, 561–571. doi: 10.1007/s00300-012-1284-2
Jahn A., Holland M. M., Kay J. E. (2024). Projections of an ice-free arctic ocean. Nat. Rev. Earth Environ. 5, 164–176. doi: 10.1038/S43017-023-00515-9
Jay C., Fishchbach A. S., Kochnev A. A. (2012). Walrus area use in the Chukchi Sea during sparse sea ice cover. Mar. Ecol. Prog. Ser. 468, 1–13. doi: 10.3354/meps10057
Keith D. A., Akcakaya H. R., Thuiller W., Midgley G. F., Pearson R. G., Phillips S. J., et al. (2008). Predicting extinction risks under climate change: coupling stochastic population models with dynamic bioclimatic habitat models. Biol. Lett. 4, 560–563. doi: 10.1098/rsbl.2008.0049
Kelly B. P., Badajos O. H., Kunnasranta M., Moran J. R., Martinez-Bakker M., Wartzok D., et al. (2010). Seasonal home ranges and fidelity to breeding sites among ringed seals. Polar Biol. 33, 1095–1109. doi: 10.1007/s00300-010-0796-x
Kelly B. P., Quakenbush L. T. (1990). Spatiotemporal use of lairs by ringed seals (Phoca hispida). Can. J. Zoology 68, 2503–2512. doi: 10.1139/z90-350
Kim Y.-H., Min S.-K., Gillett N. P., Notz D., Malinina E. (2023). Observationally constrained projections of an ice-free Arctic even under a low emission scenario. Nat. Commun. 14, 3139. doi: 10.1038/s41467-023-38511-B
Kiszka J. J., Heithaus M. R., Wirsing A. J. (2015). Behavioural drivers of the ecological roles and importance of marine mammals. Mar. Ecol. Prog. Ser. 523, 267–281. doi: 10.3354/meps11180
Kleiber M. (1975). The fire of life: an introduction to animal energetics (Huntington, New York: Krieger).
Kovacs K. M., Aars J., Lydersen C. (2014). Walruses recovering after 60+ years of protection at Svalbard, Norway. Polar Res. 33, 26034. doi: 10.3402/polar.v33.26034
Kovacs K. M., Aguilar A., Aurioles D., Burkanov V., Campagna C., Gales N., et al. (2012). Global threats to pinnipeds. Mar. Mammal Sci. 28, 414–436. doi: 10.1111/j.1748-7692.2011.00479.x
Kovacs K. M., Boveng P., Desportes G., Ferguson S., Hansen R., Laidre K., et al. (2021a). 2021 State of the Arctic Marine Biodiversity Report. (SAMBR) Update: Marine Mammals (Akureyri, Iceland: Conservation of Arctic Flora and Fauna International Secretariat).
Kovacs K. M., Citta J., Brown T., Dietz R., Ferguson S., Harwood L., et al. (2021b). Variation in body size of ringed seals (Pusa hispida hispida) across the circumpolar Arctic: evidence of morphs, ecotypes or simply extreme plasticity? Polar Res. 40, 5753. doi: 10.33265/polar.v40.5753
Kovacs K. M., Krafft B., Lydersen C. (2020). Bearded seal (Erignathus barbatus) pup growth - body size, behavioral plasticity and survival in a changing climate. Mar. Mammal Sci. 36, 276–284. doi: 10.1111/mms.12647
Kovacs K. M., Liston G. E., Reinking A. K., Gerland S., Lydersen C. (2024). Reproductive specialization can increase extinction risk in the face of Arctic climate change. Ecol. Model. 495, 110790. doi: 10.1016/j.ecolmodel.2024.110790
Kovacs K. M., Lydersen C. (2006). Birds and mammals of Svalbard (Tromsø, Norway: Norwegian Polar Institute). No. 13.
Kovacs K. M., Lydersen C., Overland J. E., Moore S. E. (2011). Impacts of changing sea-ice conditions on Arctic marine mammals. Mar. Biodiversity 41, 181–194. doi: 10.1007/s12526-010-0061-0
Krafft B. A., Kovacs K. M., Haug T., Lydersen C. (2006). Growth and population parameters of ringed seals (Pusa hispida) from Svalbard, Norway 2002-2004. ICES J. Mar. Sci. 63, 1136–1144. doi: 10.1016/j.icesjms.2006.04.001
Krafft B. A., Kovacs K. M., Lydersen C. (2007). Distribution of sex and age groups of ringed seals Pusa hispida in the fast-ice breeding habitat of Kongsfjorden, Svalbard. Mar. Ecol. Prog. Ser. 335, 199–206. doi: 10.3354/meps335199
Lacy R. C. (2000). Structure of the VORTEX simulation model for population viability analysis. Ecol. Bulletins 48, 191–203.
Lacy R. C., Miller P. S., Nyhus P. J., Pollak J. P., Raboy B. E., Zeigler S. (2013). Metamodels for transdisciplinary analysis of population dynamics. PloS One 8, e84211. doi: 10.1371/journal.pone.0084211
Lacy R. C., Miller P. S., Traylor-Holzer K. (2023). Vortex 10 User’s Manual (Apple Valley, Minnesota, USA: IUCN SSC Conservation Planning Specialist Group, and Chicago Zoological Society).
Lacy R. C., Pollak J. P. (2023). VORTEX: A Stochastic Simulation of the Extinction Process (Brookfield, Illinois, USA: Chicago Zoological Society). Version 10.6.0.
Laidre K. L., Stern H., Kovacs K. M., Lowry L., Moore S. E., Regehr E. V., et al. (2015). Arctic marine mammal population status, sea ice habitat loss, and conservation recommendations for the 21st century. Conserv. Biol. 29, 724–737. doi: 10.1111/cobi.12474
Laidre K. L., Stirling I., Estes J. A., Kochnev A., Roberts J. (2018). Historical and potential future importance of large whales as food for polar bears. Front. Ecol. Environ. 16, 515–524. doi: 10.1002/fee.2018.16.issue-9
Laidre K. L., Stirling I., Lowry L. F., Wiig Ø., Heide-Jørgensen M. P., Ferguson S. H. (2008). Quantifying the sensitivity of arctic marine mammals to climate-induced habitat change. Ecol. Appl. 18, S97–S125. doi: 10.1890/06-0546.1
Laidre K. L., Supple M. A., Born E. W., Regehr E. V., Wiig Ø., Ugarte F., et al. (2022). Glacial ice supports a distinct and undocumented polar bear subpopulation persisting in late 21st-century sea-ice conditions. Science 376, 1333–1338. doi: 10.1126/science.abk2793
Le Moullec M., Pedersen Å.Ø., Stien A., Rosvold J., Hansen B. B. (2019). A century of conservation: The ongoing recovery of Svalbard reindeer. J. Wildlife Manage. 83, 1676–1686. doi: 10.1002/jwmg.21761
Llobet S. M., Ahonen H., Lydersen C., Kovacs K. M. (2023). The Arctic and the future Arctic? Soundscapes and marine mammal communities on the east and west sides of Svalbard characterized through acoustic data. Front. Mar. Sci. Front. Mar. Sci. 10. doi: 10.3389/fmars.2023.1208049
Lone K., Kovacs K. M., Lydersen C., Fedak M., Andersen M., Lovell P., et al. (2018). Aquatic behaviour of polar bears (Ursus maritimus) in an increasingly ice-free Arctic. Sci. Rep. 8, 9677. doi: 10.1038/s41598-018-27947-4
Lydersen C., Assmy P., Falk-Petersen S., Kohler J., Kovacs K. M., Reigstad M., et al. (2014). The importance of tidewater glaciers for marine mammals and seabirds in Svalbard, Norway. J. Mar. Syst. 129, 452–471. doi: 10.1016/j.jmarsys.2013.09.006
Lydersen C., Gjertz I. (1987). Population parameters of ringed seals (Phoca hispida Schreber 1775) in the Svalbard area. Can. J. Zoology 65, 1021–1027. doi: 10.1139/z87-162
Lydersen C., Hammill M. O., Kovacs K. M. (1994). Diving activity in nursing bearded seal (Erignathus barbatus) pups. Can. J. Zoology 72, 96–103. doi: 10.1139/z94-013
Lydersen C., Jensen P. M., Lydersen E. (1990). A survey of the Van Mijen fiord, Svalbard, as habitat for ringed seals, Phoca hispida. Holarctic Ecol. 13, 130–133. doi: 10.1111/j.1600-0587.1990.tb00598.x
Lydersen C., Kovacs K. M. (1999). Behaviour and energetics of ice-breeding, North Atlantic phocid seals during the lactation period. Mar. Ecol. Prog. Ser. 187, 265–281. doi: 10.3354/meps187265
Lydersen C., Ryg M. (1991). Evaluation breeding habitat and populations of ringed seals Phoca hispida in Svalbard fjords. Polar Rec. 27, 223–228. doi: 10.1017/S0032247400012614
Lydersen C., Smith T. G. (1989). Avian predation on ringed seal Phoca hispida pups. Polar Biol. 9, 489–490. doi: 10.1007/BF00261031
MacLeod C. D. (2009). Global climate change, range changes and potential implications for the conservation of marine cetaceans: a review and synthesis. Endangered Species Res. 7, 125–136. doi: 10.3354/esr00197
Maduna S. N., Aars J., Fløystad I., Klutsch C. F. C., Zeyl Fiskebeck E. M. L., Wiig Ø., et al. (2021). Sea ice reduction drives genetic differentiation among Barents Sea polar bears. Proc. R. Soc. B 288, 20211741. doi: 10.1098/rspb.2021.1741
McLaren I. A. (1958). “The biology of the ringed seal, Phoca hispida, in the eastern Canadian Arctic,” in Bulletin of the Fisheries Research Board of Canada, vol. 118. (Fisheries Research Board of Canada, Ottawa).
Meredith M., Sommerkorn M., Cassotta S., Derksen C., Ekaykin A., Hollowed A., et al. (2019). “Polar regions,” in IPCC Special Report on the Ocean and Cryosphere in a Changing Climate. Eds. Pörtner H.-O., Roberts D. C., Masson-Delmotte V., Zhai P., Tignor M., Poloczanska E., Mintenbeck K., Alegría A., Nicolai M., Okem A., Petzold J., Rama B., Weyer N. M. (Cambridge University Press, Cambridge, UK and New York, NY, USA), 203–320. doi: 10.1017/9781009157964.005
Merkel B., Aars J. (2022). Shifting polar bear Ursus maritimus denning habitat availability in the European Arctic. Polar Biol. 45, 481–490. doi: 10.1007/s00300-022-03016-5
Merkel B., Lydersen C., Yoccoz N. G., Kovacs K. M. (2013). The world's northernmost harbour seal population - how many are there? PlosOne 8, e67576. doi: 10.1371/journal.pone.0067576
Miller P. S., Lacy R. C., Medina-Miranda R., López-Ortiz R., Díaz-Soltero H. (2016). Confronting the invasive species crisis with meta-model analysis: An explicit, two-species demographic assessment of an endangered bird and its brood parasite in Puerto Rico. Biol. Conserv. 196, 124–132. doi: 10.1016/j.biocon.2016.01.029
Molano-Flores B., Bell T. J. (2012). Projected population dynamics for a federally endangered plant under different climate change emission scenarios. Biol. Conserv. 145, 130–138. doi: 10.1016/j.biocon.2011.10.022
Molnár P. K., Derocher A. E., Klanjscek T., Lewis M. A. (2011). Predicting climate change impacts on polar bear litter size. Nat. Commun. 2, 186. doi: 10.1038/ncomms1183
Molnár P. K., Derocher A. E., Thiemann G. W., Lewis M. A. (2010). Predicting survival, reproduction, and abundance of polar bears under climate change. Biol. Conserv. 143, 1612–1622. doi: 10.1016/j.biocon.2010.04.004
Moore S. E., Gulland F. M. D. (2014). Linking marine mammal and ocean health in the “new normal“. Arctic. Ocean Coast. Manage. 102, 55–57. doi: 10.1016/j.ocecoaman.2014.08.011
Morris W. F., Doak D. F. (2002). Quantitative Conservation Biology. Theory and Practice of Population Viability Analysis (Sunderland, Mass: Sinauer), 480 pp.
Naciri M., Aars J., Blanchet M. A., Gimenez O., Cubaynes S. (2022). Reproductive senescence in polar bears in a variable environment. Front. Ecol. Evol. 10. doi: 10.3389/fevo.2020.920481
Nagy K. A. (1987). Field metabolic rate and food requirement scaling in mammals and birds. Ecol. Monogr. 57, 111–128. doi: 10.2307/1942620
Nelson M., Quakenbush L., Taras B., Ice Seal C. (2019). Subsistence harvest of ringed, bearded, spotted and ribbon seals in Alaska is sustainable. Endangered Species Res. 40, 1–16. doi: 10.3354/esr00973
Øigård T. A., Haug T., Nilssen K. T. (2014). Current status of hooded seals in the Greenland Sea. Victims of climate change and predation? Biol. Conserv. 172, 29–36. doi: 10.1016/j.biocon.2014.02.007
Pacifici M., Foden W. B., Visconti P., Watson J. E. M., Butchart S. H. M., Kovacs K. M., et al. (2015). Assessing species vulnerability to climate change. Nat. Climate Change 5, 215–224. doi: 10.1038/nclimate2448
Pagano A. M., Rode K. D., Lunn N. J., McGreachy D., Atkinson S. N., Farley S. D., et al. (2024). Polar bear energetic and behavioral strategies on land with implications for surviving the ice-free period. Nat. Commun. 15, 947. doi: 10.1038/s41467-023-44682-1
Pollak J. P., Lacy R. C. (2020). MetaModel Manager (Brookfield, Illinois: Chicago Zoological Society). Available at: http://www.scti.tools/metamodelmanager. Version 1.0.6.
Previdi M., Smith K. L., Polvani L. M. (2021). Arctic amplification of climate change: a review of underlying mechanisms. Environ. Res. Lett. 16, 093003. doi: 10.1088/1748-9326/ac1c29
Prop J., Aars J., Bardsen B. J., Hanssen S. A., Bech C., Bourgeon S., et al. (2015). Climate change and the increasing impact of polar bears on bird populations. Front. Ecol. Evol. 3. doi: 10.3389/fevo.2015.00033
Prowse T. A. A., Johnson C. N., Lacy R. C., Bradshaw C. J. A., Pollak J. P., Watts M. J., et al. (2013). No need for disease: testing extinction hypotheses for the thylacine using multi-species metamodels. J. Anim. Ecol. 82, 355–364. doi: 10.1111/jane.2013.82.issue-2
Rantanen M., Karpechko A. Y., Lipponen A., Nordling K., Hyvärinen O., Ruosteenoja K., et al. (2022). The Arctic has warmed nearly four times faster than the globe since 1979. Commun. Earth Environ. 3, 168. doi: 10.1038/s43247-022-00498-3
Reeves R. R., Ewins P. J., Agbayani S., Heidi-Jorgensen M. P., Kovacs K. M., Lydersen C., et al. (2014). Distribution of endemic cetaceans in relation to hydrocarbon development and commercial shipping in a warming Arctic. Mar. Policy 44, 375–389. doi: 10.1016/j.marpol.2013.10.005
Regehr E. V., Laidre K. M., Akcakaya H. R., Amstrup K. S., Atwood T. C., Lunn N. J., et al. (2016). Conservation status of polar bears (Ursus maritimus) in relation to projected sea-ice declines. Biol. Lett. 12, 20160556. doi: 10.20161098/rsbl.20162016.20160556
Regehr E. V., Lunn N. J., Amstrup S. C., Stirling I. (2007). Effects of earlier sea ice breakup on survival and population size of polar bears in western Hudson Bay. J. Wildlife Manage. 71, 2673–2683. doi: 10.2193/2006-180
Reimer J. R., Caswell H., Derocher A. E., Lewis M. A. (2019). Ringed seal demography in a changing climate. Ecol. Appl. 29, e01855. doi: 10.1002/eap.1855
Rode K. D., Douglas D. C., Atwood T. C., Durner G. M., Wilson R. R., Pagano A. M. (2022). Observed and forecasted changes in land use by polar bears in the Beaufort and Chukchi Seas 1985-2040. Global Ecol. Conserv. 40, e02319. doi: 10.1016/j.gecco.2022.e02319
Rosing Asvid A. (2006). The influence of climate variability on polar bear (Ursus maritimus) and ringed seal (Pusa hispida) population dynamics. Can. J. Zoology 84, 357–365. doi: 10.1139/Z06-001
Scheffers B. R., De Meester L., Bridge T. C. L., Hoffmann A. A., Pandolfi J. M., Corlett R. L., et al. (2016). The broad footprint of climate change from genes to biomes to people. Science 354, aaf7671. doi: 10.1126/science.aa.f7671
Shaffer M. L. (1990). Population viability analysis. Conserv. Biol. 4, 39–40. doi: 10.1111/j.1523-1739.1990.tb00265.x
Shoemaker K. T., Lacy R. C., Verant M. L., Brook B. W., Liveri T. M., Miller P. S., et al. (2014). Effects of prey metapopulation structure on the viability of black-footed ferrets in plague-impacted landscapes: a metamodeling approach. J. Appl. Ecol. 51, 735–745. doi: 10.1111/jpe.2014.51.issue-3
Shu Q., Wang Q., Årthun M., Wang S., Song Z., Zhang M., et al. (2022). Arctic Ocean Amplification in a warming climate in CMIP6 models. Sci. Adv. 8, eabn9755. doi: 10.1126/sciadv.abn9755
Smith T. G., Hammill M. O. (1981). Ecology of the ringed seal, Phoca hispida, in its fast-ice breeding habitat. Can. J. Zoology 59, 966–981. doi: 10.1139/z81-135
Smith T. G., Lydersen C. (1991). Availability of suitable land-fast ice and predation as factors limiting ringed seal populations, Phoca hispida, in Svalbard. Polar Res. 10, 585–594. doi: 10.1111/j.1751-8369.1991.tb00676.x
Stanton J. C., Shoemaker K. T., Pearson R. G., Akçakaya H. R. (2015). Warning times for species extinctions due to climate change. Global Change Biol. 21, 1066–1077. doi: 10.1111/gcb.2015.21.issue-3
Stempniewicz L., Kulaszewicz I., Aars J. (2021). Yes, they can: polar bears Ursus maritimus successfully hunt Svalbard reindeer Rangifer tarandus platyrhynchus. Polar Biol. 44, 2199–2206. doi: 10.1007/s00300-021-02954-w
Stenson G. B., Hammill M. O. (2014). Can ice breeding seals adapt to habitat loss in a time of climate change? ICES J. Mar. Sci. 71, 1977–1986. doi: 10.1093/icesjms/fsu074
Stern H., Laidre K. L. (2016). Sea-ice indicators of polar bear habitat. Cryosphere 10, 1–15. doi: 10.5194/tc-10-2027-2016
Stirling I., Derocher A. E. (2012). Effects of climate warming on polar bears: a review of the evidence. Global Change Biol. 18, 2694–2706. doi: 10.1111/j.1365-2486.2012.02753.x
Stirling I., Øritsland N. A. (1995). Relationships between estimates of ringed seal (Phoca hispida) and polar bears (Ursus maritimus) populations in the Canadian Arctic. Can. J. Fisheries Aquat. Sci. 52, 2594–2612. doi: 10.1139/f95-849
Taylor P. C., Boeke R. C., Boisvert L. N., Feldl N., Henry M., Huang Y., et al. (2022). Process drivers, inter-model spread, and the path forward - a review of amplified Arctic warming. Front. Earth Sci. 9. doi: 10.3389/feart.2021.758361
Tynan C., DeMaster D. P. (1997). Observations and predictions of Arctic climatic change: potential effects on marine mammals. Arctic 50, 308–322. doi: 10.14430/arctic1113
Udevitz M. S., Taylor R. L., Garlich-Miller J. L., Quakenbush L. T., Snyder J. A. (2012). Potential population-level effects of increased haul-out mortality of Pacific walrus calves. Polar Biol. 36, 291–298. doi: 10.1007/s00300-012-1259-3
Urbanski J. A., Litwicka D. (2022). The decline of Svalbard land-fast sea ice extent as a result of climate change. Oceanologia 64, 535–545. doi: 10.1016/j.oceano.2022.03.008
Vacquiè-Garcia J., Spitz J., Hammill M., Stenson G. B., Kovacs K. M., Lydersen C., et al. (2024). Foraging habits of Northwest Atlantic hooded seals over the past 30 years: future habitat suitability under global warming. Global Change Biol. 30, e17186. doi: 10.1111/gcb.17186
Van Parijs S. M., Lydersen C., Kovacs K. M. (2003). Vocalizations and movements suggest alternate mating tactics in male bearded seals. Anim. Behav. 65, 273–283. doi: 10.1006/anbe.2003.2048
Vihtakari M., Welcker J., Moe B., Chastel O., Tartu S., Hop H., et al. (2018). Black-legged kittiwakes as messengers of Atlantification in the Arctic. Sci. Rep. 8, 1178. doi: 10.1038/s41598-017-19118-8
Wells K., Brook B. W., Lacy R. C., Mutze G. J., Peacock D. E., Sinclair R. G., et al. (2015). Timing and severity of immunizing diseases in rabbits is controlled by seasonal matching of host and pathogen dynamics. J. R. Soc. Interface 12, 2014184. doi: 10.1098/rsif.2014.1184
Whiteman J. P., Harlow H. J., Durner G. M., Regehr E. V., Amstrup S., Ben-David M. (2017). Phenotypic plasticity and climate change: can polar bears respond to longer Arctic summers with an adaptive fast? Oecologia 186, 369–381. doi: 10.1007/s004442-017-4023-0
Wichmann M. C., Groeneveld J., Jeltsch F., Grimm V. (2005). Mitigation of climate change impacts on raptors by behavioural adaptations: ecological buffering mechanisms. Global Planetary Change 47, 273–281. doi: 10.1016/j.gloplacha.2004.10.016
Willis S. G., Young B., Rondinini C. (2015). Assessing species vulnerability to climate change. Nat. Climate Change 5, 215–224. doi: 10.1038/nclimate2448
Keywords: Arctic, climate change, ringed seal, polar bear, bearded seal, population viability analysis, metamodel
Citation: Lacy RC, Kovacs KM, Lydersen C and Aars J (2024) Linking PVA models into metamodels to explore impacts of declining sea ice on ice-dependent species in the Arctic: the ringed seal, bearded seal, polar bear complex. Front. Conserv. Sci. 5:1439386. doi: 10.3389/fcosc.2024.1439386
Received: 27 May 2024; Accepted: 30 October 2024;
Published: 19 November 2024.
Edited by:
Carlos R. Ruiz-Miranda, Universidade Estadual do Norte Fluminense, BrazilReviewed by:
Karin Charlotta Harding, University of Gothenburg, SwedenBarry Noon, Colorado State University, United States
Copyright © 2024 Lacy, Kovacs, Lydersen and Aars. This is an open-access article distributed under the terms of the Creative Commons Attribution License (CC BY). The use, distribution or reproduction in other forums is permitted, provided the original author(s) and the copyright owner(s) are credited and that the original publication in this journal is cited, in accordance with accepted academic practice. No use, distribution or reproduction is permitted which does not comply with these terms.
*Correspondence: Robert C. Lacy, cmxhY3lAaXgubmV0Y29tLmNvbQ==