- 1University of Alberta, Department of Biological Sciences, Edmonton, AB, Canada
- 2IDEXX Laboratories, Inc., Westbrook, ME, United States
Introduction: Zoonoses associated with urban wildlife are increasingly concerning for human health and include the recent emergence of alveolar echinococcosis (AE) in North America. AE develops following infection with the tapeworm Echinococcus multilocularis. In Alberta, up to 65% of urban coyotes (Canis latrans) are infected with E. multilocularis, and infected scats contain eggs that can be accidentally ingested by people. Our goal was to determine the predictors of infection prevalence and intensity in coyote scats in Edmonton, Canada, and to identify the predictors of coyote scat deposition and content, especially as related to anthropogenic food sources and infrastructure.
Methods: To study infection prevalence and intensity, volunteers collected 269 scats, which were tested for E. multilocularis using polymerase chain reaction. We compared infection prevalence and shedding intensity by habitat and scat content. To determine predictors of scat presence and content, we used snow tracking to identify 1263 scats. We compared landscape characteristics at scats and available points, and among scats with different contents. We used negative binomial regression to predict scat abundance in city-delineated green spaces.
Results: 26.0% of tested scats were positive for E. multilocularis (n = 70), and infection was twice as common as expected near compost and 1.3x more common than expected when scats contained anthropogenic food. Scats were more common than expected near other scats (80% within 1 m of scats, 27% at 11.5 m), buildings (19% at buildings, 16% at 80 m), and the camps of people experiencing homelessness (24% at camps, 20% at 60 m). Scats frequently contained fruit (52.9%), anthropogenic material (36.7%), and birdseed (16.0%), and scats containing anthropogenic material often occurred near human infrastructure, supporting a relationship between anthropogenic attractants and scat accumulation.
Discussion: These results suggest that abundant food sources and anthropogenic food increase coyote aggregation, increasing both scat abundance and infection rates, which in turn increases risk of exposure to zoonotic parasites for humans. Risk to humans might be reduced by preventing coyote access to anthropogenic and aggregated food sources and educating people who are likely to encounter infected soil or vegetation, including gardeners, park users, and people experiencing homelessness.
1 Introduction
A major form of human-wildlife conflict in cities is the potential for urban-dwelling wildlife to transmit zoonotic diseases to human residents (Rothenburger et al., 2017; Gibb et al., 2020). Zoonotic diseases account for ~60% of emerging infectious diseases worldwide (Bengis et al., 2004; Jones et al., 2008) and may be especially prominent in urban areas (Rothenburger et al., 2017; Gibb et al., 2020) where biodiversity is limited, animal density and stress are elevated, and community structure is altered (Wright and Gompper, 2005; Bradley and Altizer, 2007). Cities also offer aggregated food sources where synanthropic species congregate, increasing wildlife disease transmission and prevalence (Prange et al., 2004; Wright and Gompper, 2005; Becker et al., 2015; Murray et al., 2016a; Murray et al., 2016b), especially when weak or sick individuals select these food sources (Murray et al., 2016a; Murray et al., 2019).
An emerging, infectious zoonosis of increasing concern in North American cities is alveolar echinococcosis (AE; Eckert and Deplazes, 2004; Houston et al., 2021; Santa et al., 2021). Humans develop AE following accidental ingestion of microscopic eggs of the zoonotic tapeworm Echinococcus multilocularis present in canine feces (Catalano et al., 2012; Poulle et al., 2017), feline feces (Poulle et al., 2017; Bastien et al., 2018; Umhang et al., 2022) or adhering to soil (Poulle et al., 2017; Bastien et al., 2018) and produce (Lass et al., 2015). Without treatment, mortality in humans exceeds 90% (Brunetti et al., 2010). Previously, AE was nearly absent from North America, but infection rates are now increasing following the appearance of a highly infectious European haplotype of E. multilocularis in North America (Massolo et al., 2019; Houston et al., 2021). While this haplotype has been detected in several Canadian provinces (Schurer et al., 2021; Robbins et al., 2022) and American states (Zajac et al., 2020; Polish et al., 2021; Kuroki et al., 2022; Polish et al., 2022), Alberta is the epicenter of AE in North America, with over 20 confirmed cases (Houston et al., 2021). In Edmonton, Alberta, a large population of urban-adapted coyotes (Canis latrans) represents a reservoir of E. multilocularis (Catalano et al., 2012; Luong et al., 2020; Sugden et al., 2020; Houston et al., 2021), with several studies identifying infection rates between 50 and 65% (Catalano et al., 2012; Luong et al., 2020; Sugden et al., 2020).
Because infected coyote scats are the source of eggs that could cause AE in people, understanding the distribution of scats, especially infected scats, in an urban landscape could facilitate local risk assessment and management. Many carnivores, including coyotes, deposit scat and urine strategically for intraspecific communication (Macdonald, 1980; Brattstrom, 1999; Barja and List, 2014; Apps et al., 2019) and territorial signalling (Bowen and Cowan, 1980; Allen et al., 1999; Gese, 2001). While urine may be the most common deposition for coyote communication (Barrette and Messier, 1980; Wells and Bekoff, 1981; Gese and Ruff, 1997), scat also appears to be used for scent-marking (Brattstrom, 1999; Barja and List, 2014). Scats therefore may accumulate in specific locations, where risk of exposure for humans or pets may increase. For example, previous studies have documented increased scat deposition at the tops of slopes (Brattstrom, 1999), along territorial boundaries (Bowen and Cowan, 1980; Allen et al., 1999; Gese, 2001), near food sources (Bowen and Cowan, 1980; Allen et al., 1999; Barja and List, 2014), and on trails or roads (Brattstrom, 1999; Barja and List, 2014).
The risk that humans encounter scats and are exposed to zoonotic disease likely increases when coyotes occupy human-dominated habitat, for example, by denning under or near buildings (Way, 2009; Raymond and St Clair, 2023a) or by accessing food or attractants near backyards (Murray and St. Clair, 2017; Raymond and St. Clair, 2023b). Spatial overlap of urban wildlife and domestic pets can also expose pet owners to disease (Azocar-Aedo et al., 2014; Mackenstedt et al., 2015; Julien et al., 2021). For example, dog owners may face increased risk of exposure if their pets consume parasites or carry them on their fur (Nonaka et al., 2009; Conraths et al., 2017). Some human demographics may be at increased risk of parasite exposure and, thus, vulnerability to zoonotic disease, including people experiencing homelessness and living rough in green spaces (Leibler et al., 2016), people who work with soil and compost (e.g., gardeners), and pet owners who may also be at increased risk of exposure. Lastly, human exposure to zoonotic disease likely increases where the local rate of scat infection is higher, which may occur when food sources aggregate animals, accelerating trophic transmission (Wright and Gompper, 2005; Becker et al., 2015; Becker et al., 2018) and potentially limiting the development of immunity (Sugden et al., 2023).
The purpose of this study was to determine the environmental factors and human use contexts that increase risk of human exposure to coyote scats and, by extension, to infectious E. multilocularis eggs with three specific objectives. To identify predictors of scat infection, we collected scats (n = 269) from different habitats and compared infection prevalence and intensity by habitat and scat content. Second, to determine the predictors of scat location on the urban landscape, we used a different sample of scats (n = 1263) and a series of remotely sensed natural and anthropogenic landscape features to predict scat presence at a fine scale (immediate scat environment) and a broader scale (city-delineated green spaces). Third, to determine the relationships between anthropogenic landscape features and scat content, we visually examined scats (n = 1173) in situ to determine the presence of several food types, including those associated with human activity.
2 Methods
2.1 Study area
Edmonton is a large (~1 million people), sprawling city (684 km2) in central Alberta, Canada, centered at 53.5472°N, 113.5006°W (City of Edmonton, 2019). Edmonton is located at the interface of the boreal forest to the north and prairie grassland to the south. The local climate is dry with cold winters (Jan average temp = –10.4°C) and warm summers (Jul average temp = 17.7°C; Environment Canada, 2022). The city includes residential, industrial, and commercial developed sectors as well as extensive green spaces and natural areas (City of Edmonton, 2013). Many of these natural areas are large and contiguous with parks located adjacent to the North Saskatchewan River Valley, which bisects the city and provides connectivity for urban wildlife (City of Edmonton, 2007; City of Edmonton, 2013). These areas also support human recreation throughout the year (City of Edmonton, 2013). Red foxes (Vulpes vulpes) occur in the city, but they are very rare (unpublished data). Feral domestic dogs are possible but unlikely owing to rapid reporting by community members and removal by civic officials.
2.2 Infection status
To assess scat infection status among habitats and content, authors and a team of volunteers collected coyote scats within Edmonton between 2017 and 2020. Volunteers searched for scats in areas that represented good habitat for coyotes (e.g., green spaces, naturalized areas, golf courses [Dodge and Kashian, 2013; Murray and St. Clair, 2017; Wurth et al., 2020]), areas where humans could be at increased risk of contacting or ingesting scat particles (e.g., community gardens [Bastien et al., 2018], dog parks [Toews et al., 2021], residential areas), and areas where aggregated resources could increase disease transmission risk (Becker et al., 2015; Becker et al., 2018), such as compost piles (Murray et al., 2016b) and stormwater ponds. Volunteers searched areas by walking a human walkway (e.g., walking trail, sidewalk), while searching visually for scats. Volunteers collected each scat in an inverted heavy-weight freezer bag, sealed the bag, and placed the bagged sample in a second freezer bag. Samples were then frozen (– 80°C) for > 3 days. To ensure volunteer health and safety, all volunteers completed a training session, and received educational materials and personal protective equipment.
Because coyote infection with E. multilocularis varies seasonally (Liccioli et al., 2014), volunteers recorded the collection date, which was later classified as ‘Winter’ (December to February), ‘Spring’ (March to May), ‘Summer’ (June to August), or ‘Autumn’ (September to November). Volunteers classified scats as belonging to one of five ecological types: ‘Forested’ sites were characterised by > 50% cover by mixed wood forest, ‘Maintained’ sites featured mowed grass, and ‘Developed’ sites were characterised by industrial, commercial, or residential infrastructure. ‘Naturalized’ sites had previously been cleared, but were not actively maintained (i.e., unmowed grass and shrub communities < 6 m in height) The final ecological type, ‘Compost’, was used for scats detected within 50 m of a compost pile.
After freezing scats to neutralize parasites, one author (D.S.) visually inspected scats and verified that 269 of the 313 scats collected had been correctly identified as coyote scats. Identification was based on size, morphological criteria, and content (Elbroch and MacFarland, 2019; Raymond and St. Clair, 2023b; Raymond and St. Clair, 2023c.), and scats were distinguished from domestic dog scats based on morphology and the absence of visible grain (Reed and Merenlender, 2011). We used half of each scat sample to qualitatively assess scat content by dismantling scats with a spatula. When we detected apples (Malus spp.), birdseed, garbage, or anthropogenic compost, we classified scats as containing anthropogenic material. We used a mortar and pestle to homogenize the other half of each scat and removed a ~0.5g sample to be tested for Echinococcus multilocularis infection. Infection status was determined using a commercially available real-time polymerase chain reaction (quantitative PCR) assays (IDEXX Laboratories, Echinoccoccus RealPCR™ Panel) as previously described (Porter et al., 2022; Sugden et al., 2023). We used association tests to identify differences in infection prevalence among years, seasons, habitats, and scat content. We used chi square association tests when < 20% of expected values were less than five, and Fisher’s exact tests otherwise, included Yate’s correction when appropriate, and identified important deviations from expected values by calculating standardized residuals (Sharpe, 2015). We used the cycle threshold (CT) value of the quantitative PCR assay as a measure of the relative DNA load, or shedding intensity, in each sample. To compare shedding intensity among habitat types and scat content, we used linear regression to model the CT values among different habitat types and content, using the habitat with median shedding intensity and scats that did not contain anthropogenic food as reference categories. Lastly, we used a chi square association test to compare the occurrence of anthropogenic content among scats detected in different habitats.
2.3 Distribution and composition
2.3.1 Data collection
To explore scat distribution, we used a unique sample of scats (n = 1263) detected by one author (S.R.) while following 431 km of coyote paths in 2021 and 2022 using snow tracking (Barrette and Messier, 1980; Bowen and Cowan, 1980; Paquet, 1991; Raymond and St. Clair 2023c). The author responsible for tracking and scat identification used multiple criteria and was certified in wildlife track and sign identification (https://trackercertification.com/). We searched for scats within green spaces > 500m2 that represented high-quality habitat for urban coyotes (Dodge and Kashian, 2013; Raymond and St Clair, 2023a), identified coyote tracks in snow (Elbroch and MacFarland, 2019), followed coyote paths using snow tracking techniques (Bowen and Cowan, 1980; Paquet, 1991), and identified coyote scats. Some of these scats (n = 668) were also used to determine the predictors of coprophagy of coyote scats by black-billed magpies (Pica hudsonia) (Raymond and St. Clair, 2023c). Because scat and urine marks may be used differently by coyotes (Gese and Ruff, 1997), we also recorded the location of urination sites. We could confidently identify urine as belonging to coyotes only when (1) urine was in fresh snow and associated with fresh coyote tracks, or (2) a urine mark was within 1 m of urine coloured by blood (i.e., proestral bleeding), which would be unlikely in domestic dogs. We recorded such double urinations as a single urination site (Figure 1A). Because of the difficulty of distinguishing coyote and dog urine, we did not record urine sites that we could not attribute to coyotes or whether or not urine was present near each scat.
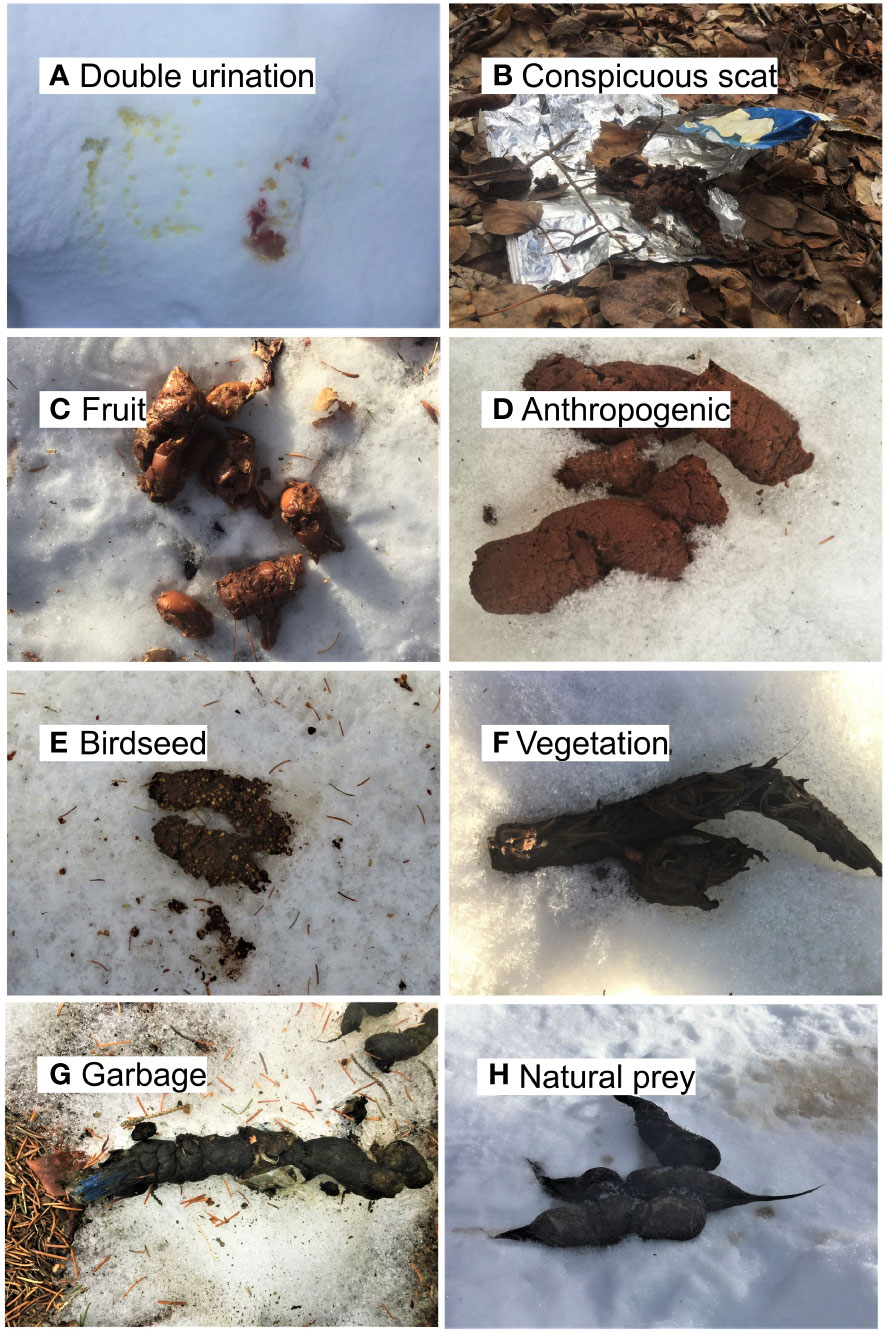
Figure 1 Coyote urination (A) and scat (B–H) detected in Edmonton, Canada, 2021 – 2022. (A) A double marking site where a male and female coyote marked with urine; the female’s urine is coloured with blood. (B) Scat deposited prominently on a chip bag. The remaining images show scat containing various content, including fruit (C), anthropogenic material (D), birdseed (E), vegetation (F), garbage (G), and natural prey (H).
At each scat, we conducted a coarse analysis of diet by visually assessing six categories of content as present or not detected. We classified scats that contained berry or apple seeds or peels as containing fruit (Figure 1C). We noted the presence of birdseed (Figure 1E), undigested vegetation (Figure 1F), garbage (e.g., plastic, cloth; Figure 1G), and natural prey (i.e., bones, fur, feathers, or blood; Figure 1H). We also included a category called ‘presumed anthropogenic material’, which we used for content that did not fit into any of the other categories, but which appeared anthropogenic in origin (Raymond and St. Clair, 2023c). Examples of presumed anthropogenic material resulted in scats that had an orange hue, and a tubular, blunt shape characteristic of a diet containing carbohydrates (Elbroch and MacFarland, 2019). Food items that could result in such scats include cooked meat, grain (e.g., bread, kibble) or digestible material in human compost (Figure 1D). We included ‘Unknown’ as a category to accommodate scat content that did not appear to fit well into any of these categories.
2.3.2 Predictors of scat presence
We measured several remotely sensed landscape characteristics that could influence scat distribution. Prior to modelling, we hypothesized the biological importance of these predictors and developed a priori predictions about how each could influence scat deposition (Table 1). Most predictors pertained to more than one causal mechanism. Briefly, we hypothesized that coyotes seeking to preserve the longevity of scat signals for intraspecific communication (Elbroch and MacFarland, 2019) would preferentially deposit scat where degradation was slower, predicting scats on leeward slopes (i.e., East aspects), flat sites, far from roads, in areas with low road density, and at sites with low anthropogenic or mowed grass land cover and high natural land cover. We hypothesized that coyotes may selectively mark travel corridors to facilitate travel within a territory (Barrette and Messier, 1980; Paquet, 1991; Gese and Ruff, 1997; Barja and List, 2014), and we predicted more scat on maintained trails (Barrette and Messier, 1980; Allen et al., 1999; Brattstrom, 1999; Barja and List, 2014), at maintained trail junctions (Brattstrom, 1999; Barja and List, 2014) and adjacent to water bodies (Paquet, 1991).
We predicted that scat deposition to reinforce territorial boundaries would occur along landscape features that often represent such boundaries (Bowen and Cowan, 1980; Gese and Ruff, 1997; Allen et al., 1999; Gese, 2001), such as riverbanks (Crabtree and Sheldon, 1999), and sites with topographic concavity (Wilkinson and Francis, 1995). Because urban core territories coincide with the boundaries of green spaces (Gese et al., 2012; Murray and St. Clair, 2017), we expected scat accumulation near roads and buildings, at intermediate road and building densities, near natural patch edges, and in areas with high natural edge density. Because territorial marking is characterised by even spacing between marks along territorial borders (Gese and Ruff, 1997; Gese, 2001), territorial signalling would also predict intermediate distance between scats. Lastly, if coyotes deposit scat strictly as a product of the biological need for elimination (Barrette and Messier, 1980; Barja and List, 2014), we expected scat accumulation in high-quality habitat where coyotes presumably spend the most time, predicting increased deposition in areas with natural land cover, and at sites where they may access food (Bowen and Cowan, 1980; Allen et al., 1999; Barja and List, 2014); in cities, such sites include human residences where coyotes access anthropogenic food (Murray and St. Clair, 2017; Raymond and St. Clair, 2023b), temporary camps belonging to people experiencing homelessness, and playgrounds. The elimination hypothesis also predicted reduced scat abundance near roads and buildings and in areas with high road or building density.
2.3.3 Statistical analyses
To determine predictors of scat deposition, we used a resource selection function with a use available design (Boyce and McDonald, 1999). We classified scats as use sites (1), and we developed available sites (0) by randomly generating points constrained along GPS tracks representing coyote paths with a ratio of three available sites per use site. Because our study was exploratory, available literature on coyote defecation in urban areas is limited, and predictors often pertained to several hypotheses, we used all-subsets model selection (Eberhardt, 2003; Stephens et al., 2007; Hegyi and Zsolt Garamszegi, 2011; Symonds and Moussalli, 2011; Tredennick et al., 2021).
We converted Euclidean distances to decay terms using the formula decay distance = 1 – (e−αd), where α and d represent a decay term and the Euclidean distance to the feature, respectively (Nielsen et al., 2009; Raymond and St. Clair, 2023c). We expected the influence of landscape-level features (e.g., proximity to water) to decay slowly, and we expected the influence of microsite features (e.g., proximity to scat) to decay rapidly, so we tested a broad range of α values for each proximity metric as follows: 0.002, 0.004, 0.006, 0.012, 0.03, 0.06, 0.2 (Raymond and St. Clair, 2023c) and selected the decay term that minimized Akaike Information Criterion value (AICc; Supplementary Table S1). We standardized all variables by scaling (1 SD) and mean-centering, and compared the quadratic and linear fit (via AICc and likelihood ratio test) for each variable (Hosmer and Lemeshow, 2000). We emphasized the most important predictors by eliminating predictors associated with P > 0.05 in univariate generalized linear models (GLMs) from further analyses (Supplementary Table S2). We assessed collinearity using Pearson’s correlation values (Dormann et al., 2013) and removed the lower performance predictor for correlated pairs (r > 0.5). To increase simplicity and management applications of models (Starfield, 1997), we used conservative Bayesian Information Criterion (BIC) to select models from all subsets (Aho et al., 2014). We considered models within ΔBIC ≤ 2 to be top main effects models and averaged them conditionally (Symonds and Moussalli, 2011). We developed biologically plausible two-way interactions, iteratively added them to the top main effects model (Hosmer and Lemeshow, 2000) and retained them only if they lowered BIC by > 2 and had confidence intervals that did not overlap zero (Supplementary Table S3). We calculated variance inflation factors (VIF) to confirm the absence of collinearity. We evaluated model performance using four metrics, which included k-fold cross validation (k = 5; Boyce et al., 2002), area under the receiving operator curve (ROC; Cumming, 2000), and McFadden and Nagelkerke pseudo R2 values (McFadden, 1979; Veall and Zimmermann, 1994).
To test for differences between locations where scat vs. urine were deposited, we compared average values for all predictors using student t-tests. We then applied the final scat model to urination data and used a confusion matrix to determine the frequency with which that model correctly predicted a urination event.
Because individual scats may not represent a realistic management unit, we also completed an analysis predicting scat abundance in city-delineated green spaces. We identified green spaces (City of Edmonton, 2022a) where we followed > 25 m of coyote paths, clipped paths by the perimeter of each green space, and determined the number of scats detected and the path length followed in each green space. To develop predictors representative of each green space, we generated 50-m buffers around the paths we followed. We calculated predictors similar to those used for individual scats (Table 1) but modified slightly. Specifically, we calculated density metrics and percent land cover for the 50-m buffers we developed, we included distance to Edmonton’s River valley/ravine parks network, distance to the city center, the total area of the green space, and a binary variable reflecting whether the green space was continuous with the river valley/ravine parks network (Supplementary Table S4). We omitted East index, slope, topographic concavity, and distance to building, road, and natural patch edge. Instead of the distance to the nearest camp and playground, we used the number of camps detected per km of coyote paths followed and the number of playgrounds present within the 50-m buffer divided by its area. We used negative binomial regression (Coxe et al., 2009) and included the path length as an offset term. Because of the smaller sample size, we removed variables associated with P > 0.25 in univariate GLMs, and considered variables to be correlated at r > 0.6 (Supplementary Table S5). We added two-way interaction terms (Supplementary Table S6) and tested model performance as described previously.
2.3.4 Scat content
To assess the influence of anthropogenic landscape features on scat content, we used an all-subsets modelling approach following the procedure described for determining predictors of scat deposition. We repeated this procedure six times, with one iteration for each of the six categories of scat content we detected. We used scat as the unit of replication, and we coded scats containing the target content item as 1 and those in which we did not detect the target content item as 0. Because we were primarily interested in the relationship between scat content and anthropogenic landscape features, we included only predictors that related to human presence or infrastructure, which included building, road, and natural edge density, distance to building, road, natural edge, camp, maintained trail, playground and scat, and anthropogenic and mowed grass land cover. Owing to smaller sample size, we included only variables associated with P < 0.25 in the all-subsets approach, we considered predictors correlated when r > 0.6, and we did not include interaction terms (Supplementary Table S7). We reported 95% confidence intervals for all analyses. We used ArcGIS version 10.8.2 to conduct spatial analyses and we completed statistical analyses and developed figures using R Studio version 4.0.3.
3 Results
3.1 Infection status
Between 2017 and 2020, authors and volunteers collected 269 scats. Of these scats, 70 (26.0%) tested positive for E. multilocularis via qPCR. Among scats collected in 2017 (n = 19), 2018 (n = 20), 2019 (n = 74) and 2020 (n = 156), infection rate ranged between 20.0% (2018) and 28.2% (2020), but there was no difference in infection among years (Fisher exact test, P = 0.806). Most scats were collected in winter (n = 130, 48.3%) and spring (n = 105, 39.0%), with fewer in summer (n = 16, 5.9%) and autumn (n = 18, 6.7%). Infection rate was highest in summer (37.5%), similar in spring (27.6%) and winter (26.2%), and lowest in autumn (0.06%), but these differences were not statistically significant (Fisher exact test, P = 0.130). Infection status differed for 249 scats with recorded habitat associations (χ2 = 14.3, df = 4, P = 0.006, Figure 2A), with double the expected number of infected scats in compost areas. We could confidently analyse scat content for 264 scats, of which 53.8% (n = 142) contained anthropogenic material. Scats containing anthropogenic material were infected 1.3 x more frequently than expected (χ2Yates = 8.52, df = 1, P = 0.004, Figure 2B). Among the 62 infected scats for which habitat was also recorded, mean shedding intensity was highest at maintained sites (CT value of 34.6 ± 2.6 SD and compost sites (34.8 ± 3.3) and lowest at naturalized sites (37.4 ± 0.6; Figure 2C). A GLM predicting shedding intensity revealed that scats detected at compost sites had greater shedding intensity (i.e., lower CT values; β = –1.58, P = 0.10, CI = –3.4–0.3). For the 69 infected scats where we could assess content, anthropogenic material increased shedding intensity (β = –1.82, P = 0.02, CI = –3.3– –0.4), with a mean CT value of 35.1 ± 2.6 for scats containing anthropogenic food and 36.3 ± 3.5 for those without; Figure 2D). The number of scats containing anthropogenic content differed among the five habitat types (χ2 = 9.76, df = 4, P = 0.045), with compost, maintained, and developed sites characterized by scats with more anthropogenic food than expected and scats at naturalized and forested sites having fewer scats containing anthropogenic material than expected (n = 244).
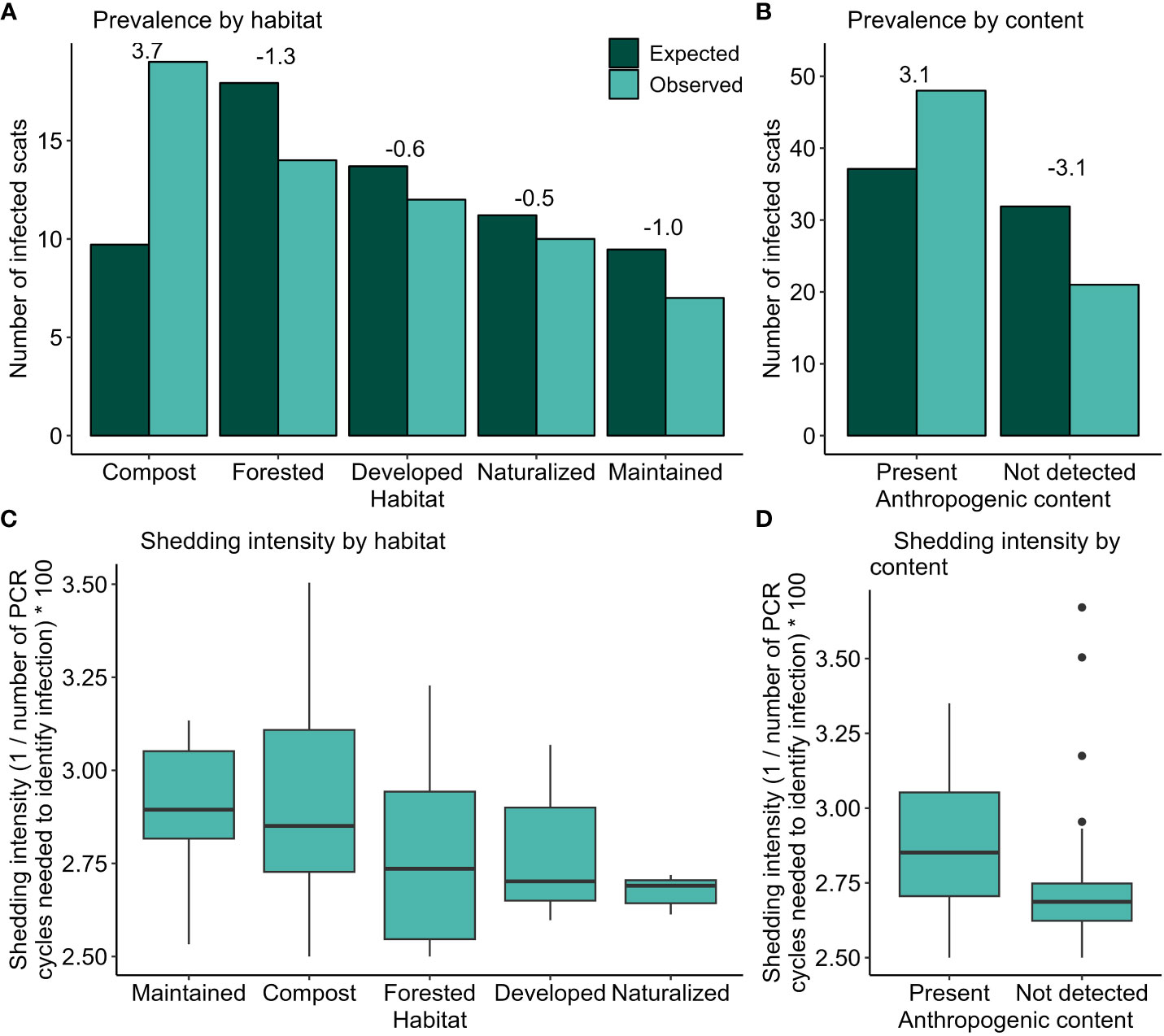
Figure 2 Prevalence and intensity of E. multilocularis infection at coyote scats collected in Edmonton, Canada, 2017 – 2020. Infection prevalence was greatest in areas with compost (A, n = 249) and when scats contained anthropogenic material (B, n = 264). Scat shedding intensity did not vary significantly among habitats (C, n = 62), but scats containing anthropogenic material had greater shedding intensity (D, n = 69).
3.2 Scat distribution
Between January 2021 and March 2022, we followed 431 km of coyote paths and recorded the location of 1263 scats (2.9 scats/km) and 53 urination sites (0.12 urination sites/km). Of the 53 urination sites, 16 were double marks where both a male and female had marked (Figure 1A). We detected proestral blood in urine between 05 February and 16 March. Because we relied on snow tracking to find scats, we found most scats during the winter (n = 748, 59.2%), but we also detected scats during the spring (n = 444, 35.1%) and autumn (n = 71, 5.7%). We found several scats deposited on prominent or elevated surfaces (Figure 1B), including logs (n = 14), roots (n = 7), branches (n = 3), in a pile of discarded kitty litter (n = 1), on a potato chip bag (n = 1), in the middle of a coyote-killed bird (n = 1), on a newspaper (n = 1), on a denim jacket (n = 1), and on various unidentified pieces of anthropogenic garbage (n = 8). We detected urination sites during the winter (n = 40, 75.5%), early spring (n = 11, 20.8%), and late autumn (n = 2, 3.8%).
We included 13 predictors in the all-subsets modelling approach to predict scat distribution: five linear terms (East index, topographic concavity, road density, distance to building, decay distance to camp) and eight quadratic terms (natural land cover, slope, natural edge density, distance to water, natural patch edge, and maintained trail junction, decay distance to scat, and maintained trail). Univariate modelling of these predictors revealed that the most important one was decay distance to the nearest scat, which suggested that coyotes deposited scat near existing scats, but that this relationship declined with increasing distance (Supplementary Table S2). This univariate model was characterised by a Nagelkerke pseudo R2 value 0.246 higher and an AICc value 942.7 lower than the next highest performing univariate GLM.
The all-subsets approach to model selection resulted in eight main effects models within Δ2 BIC (Supplementary Figure S1). Addition of an interaction term between natural land cover and decay distance to nearest scat also met our criteria for inclusion (Supplementary Table S3). Of models within Δ2 BIC, the lowest BIC model was weighted at 0.9, so we avoided the complexities of model averaging and proceeded with this model as our final one. It revealed that coyotes deposited scats near other coyote scats, but the attractive influence of scats decreased with increasing distance; we detected an 80% chance of marking within 1-m of an existing scat, and a 27% chance of marking 11.5 m from an existing scat (Figure 3A, Figure 4A). Coyotes avoided depositing scats at intermediate distances of approximately 850 m from natural patch edges (Figure 3A), and scats were more prevalent at sites with high levels of natural land cover and near both camps (Figure 4B) and buildings (Figure 4C). Coyotes also selected convex locations, such as peaks and tops of slopes to deposit scats. Lastly, the interaction term suggested that scats deposited near other scats were less affected by percent natural land cover compared to scats that were less clustered (Supplementary Figure S2). Excepting predictors that were included as both linear and quadratic terms and interaction terms, VIFs were low (≤ 1.12), suggesting limited collinearity. Nagelkerke’s pseudo R2 (0.317) and McFadden’s pseudo R2 (0.214) suggested the model fit the data moderately well. The ROC area under the curve was 0.784, suggesting the model distinguished well between used and available sites. Cross validation resulted in an accuracy of 81.1% and Cohen’s kappa of 0.397, suggesting high agreement among folds. Comparing average predictor values where urine and scat were deposited showed that most predictors did not vary between the two site types (Supplementary Table S8), but the final scat model correctly predicted only 20 of 53 urination sites, for an accuracy of 37.7%, which suggests that coyotes may select different sites for urination and defecation in urban areas.
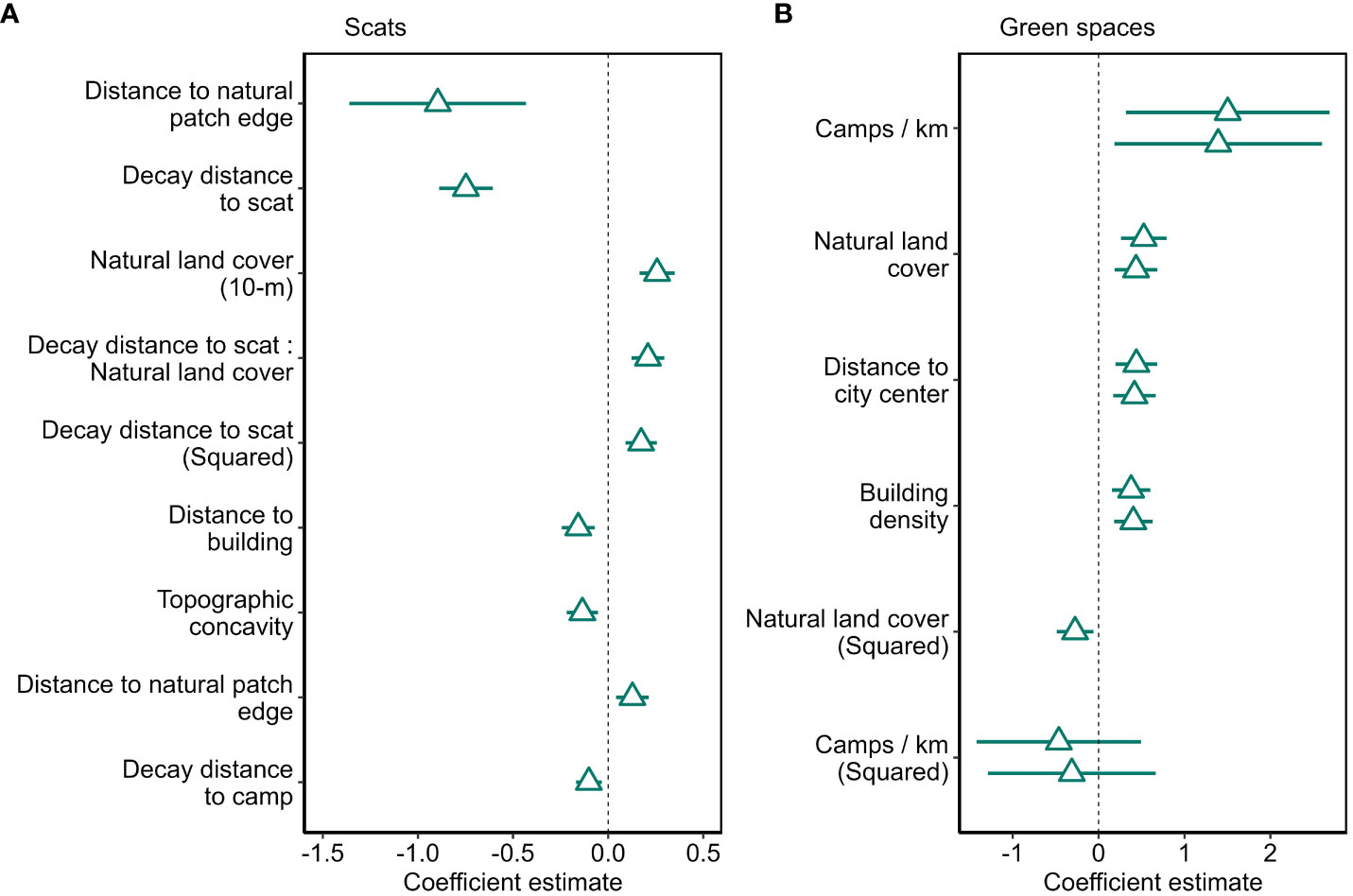
Figure 3 Standardized (1 SD) and mean-centered coefficient estimates and 95% confidence intervals for the final model predicting scat deposition (A) and the two models included in model averaging to predict the number of coyote scats within city-delineated green spaces (B) in Edmonton, Canada, 2021 – 2022.
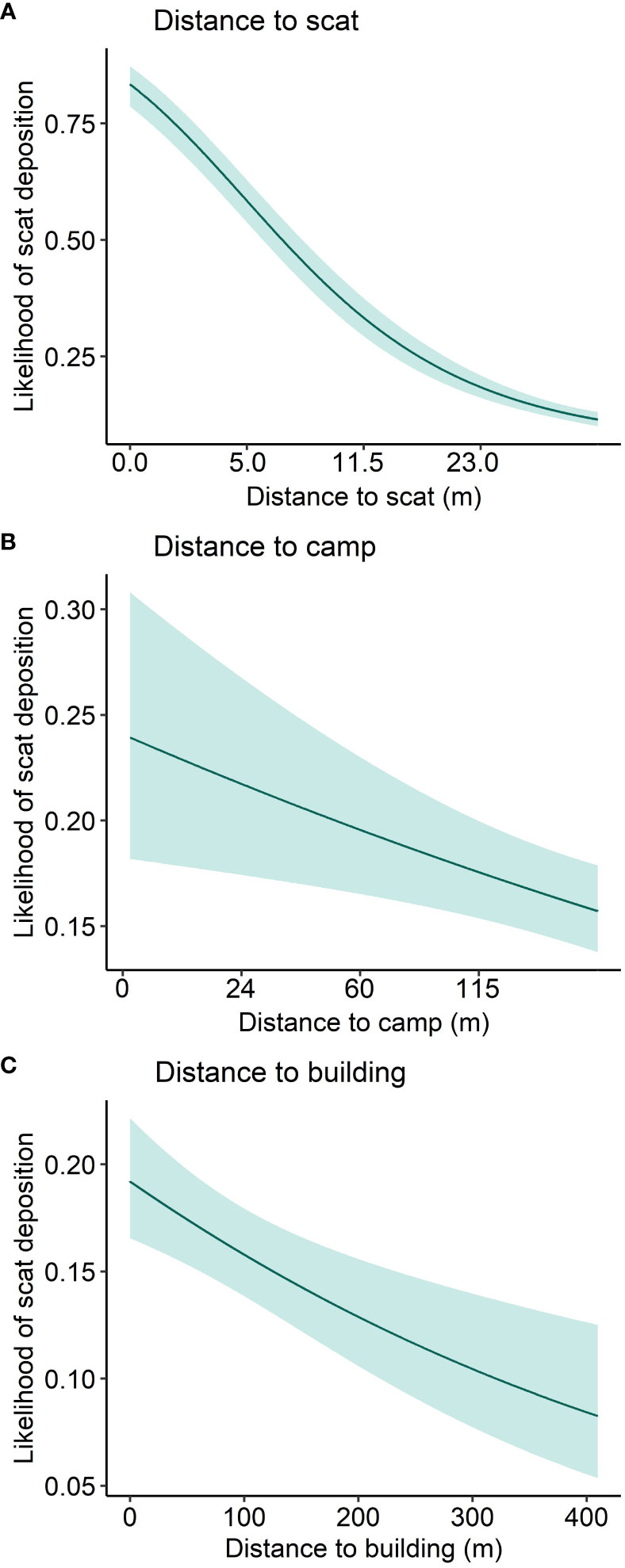
Figure 4 Marginal effect plots showing the predicted likelihood of scat deposition in response to proximity to the nearest scat (A), camp (B), and building (C) in Edmonton, Canada, 2021–2022. Scat likelihood decreased with increasing distance from the target feature.
To determine characteristics of green spaces with greater scat abundance, we used 301.6 km of coyote paths that occurred in 123 green spaces and over which we detected 1009 scats. Path length within green spaces varied from 37 m to 27 km, and the number of scats per km of coyote path varied from 0 to 98 scats/km (mean = 4.5 scats/km, SD = 11.4). We retained eight predictors in the all-subsets modelling approach, which included five quadratic terms (road density, natural land cover, camps/km, distance to water, and city center) and three linear terms (anthropogenic land cover, decay distance to playground, building density; Supplementary Table S5). The all-subsets modelling approach resulted in two models within Δ2 BIC, for which we averaged coefficients (Figure 3B) and excluded interaction terms because they did not improve model performance (Supplementary Table S2). The final model indicated that scat abundance increased in green spaces with more camps belonging to unhoused people, when green spaces were further from city center, and when building density was high. A negative quadratic term suggested that scat abundance was highest when green spaces had intermediate levels of natural land cover, peaking at about 60%. Both models included in averaging demonstrated limited collinearity (VIF ≤ 1.33). Nagelkerke (0.40, 0.37) and Mcfadden’s (0.10, 0.09) pseudo R2 metrics indicated moderate fit. Multiclass ROC AUC was high at 0.875 and 0.870 for the first and second models, respectively.
3.3 Scat content
Of the 1173 scats where we could assess diet, the most common dietary components were fruit (present in 52.9% of scats, n = 620), and presumed anthropogenic material (36.7%, n = 431; Figure 5A). Birdseed (n = 188), natural prey (n = 184), and vegetation (n = 181) were similarly common in scats (15 – 16%), and garbage was rarely detected (3.2%, n = 37; Figure 5A). For each type of scat content, we developed a list of predictors for inclusion in an all-subsets modelling approach. This approach resulted in four models within Δ2 BIC predicting scats containing birdseed, three predicting those containing garbage, two predicting scats containing fruit, natural prey, and vegetation, and a single model predicting scats containing anthropogenic material (Figure 5). Generally, scats containing some type of anthropogenic material occurred near human infrastructure. Scats containing garbage or unknown anthropogenic material occurred where road density was high (Figures 5C, G). Scats containing birdseed occurred near buildings and playgrounds, and where anthropogenic land cover was high (Figure 5D). Scats near camps often contained unknown anthropogenic material (Figure 5C). Scats containing garbage occurred near maintained trails (Figure 5G), and those containing fruit occurred near playgrounds (Figure 5B), where fruit trees are common. Scats containing fruit and unknown anthropogenic material were predicted by proximity to other scats, suggesting these carbohydrate-rich foods aggregate coyotes and their scats (Figures 5B, C); by contrast, scats containing natural prey or vegetation were predicted by increasing distance from other scats, suggestive of lesser aggregation (Figures 5E, F). Scats containing natural foods were located far from human infrastructure; scats containing natural prey were located far from camps and buildings (Figure 5E), and those containing vegetation were located far from buildings and playgrounds (Figure 5F). Although nearly all beta coefficients were associated with confidence intervals that did not overlap zero, the performance metrics for most models were poor (Table 2), indicating limited ability to discriminate between scats containing the target content and those that did not.
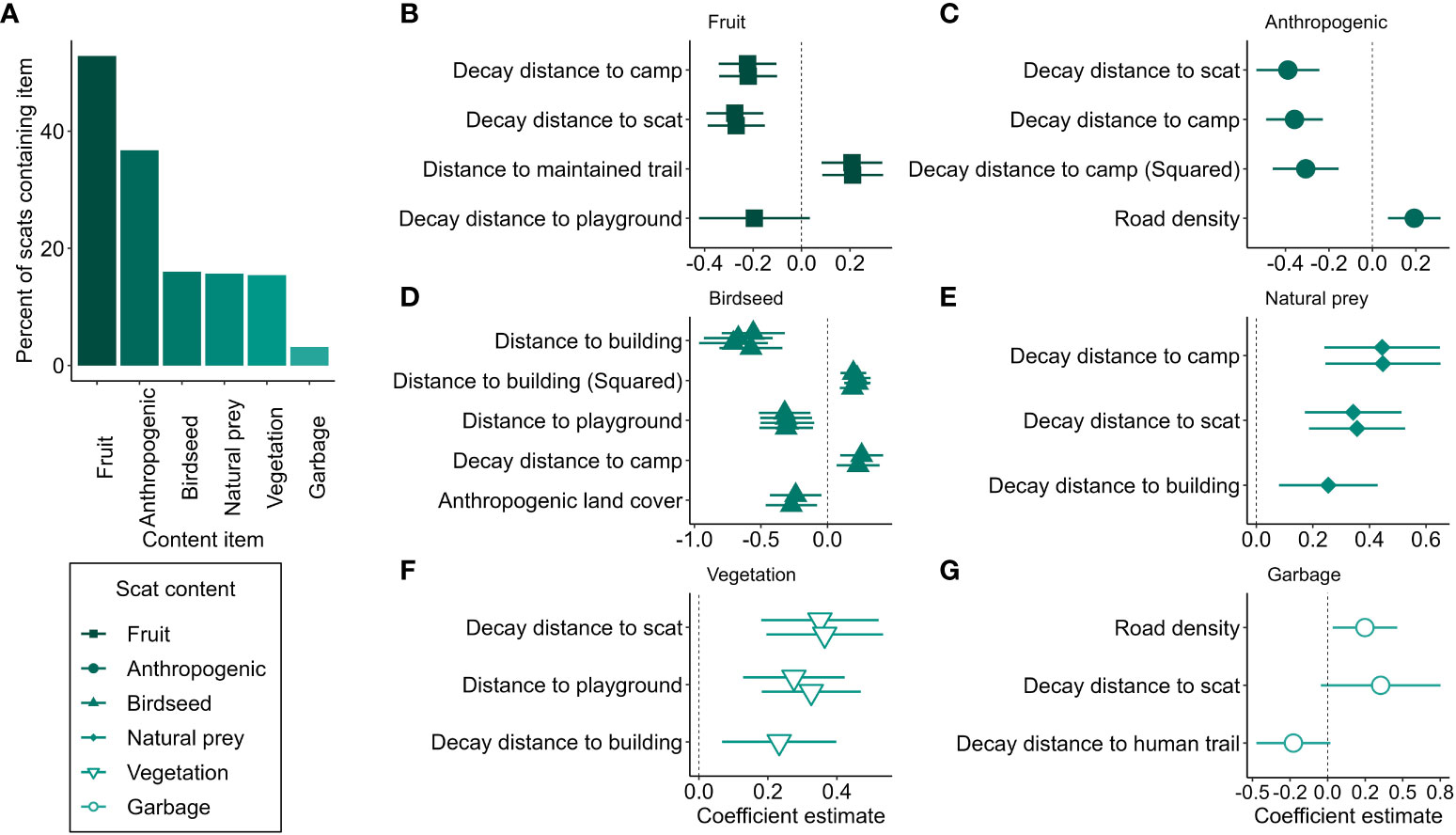
Figure 5 (A) The percentage of coyote scats (n = 1173) that contained each of six categories of content detected in Edmonton, Canada, 2021 – 2022. (B–G) show the standardized (1 SD) and mean-centered parameter estimates and 95% confidence intervals for all main effects models within Δ 2 BIC predicting scats containing each category of content.
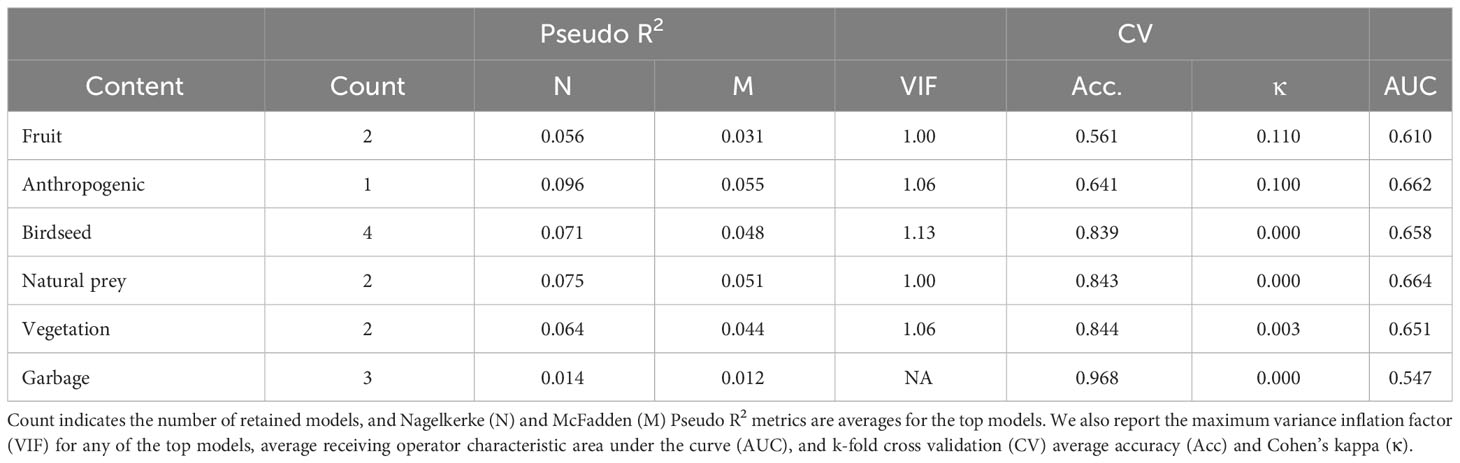
Table 2 Performance metrics associated with models predicting the presence of six categories of content in coyote scats in Edmonton, Canada, 2021 – 2022.
4 Discussion
The recent establishment of AE as an emerging zoonotic disease in North America (Massolo et al., 2019; Houston et al., 2021; Santa et al., 2021) highlights the necessity to understand the patterns and processes that determine coyote scat infection and abundance, particularly on urban landscapes where the parasite is prevalent, such as Edmonton, Alberta. Understanding these patterns could facilitate assessment of human risk and the development of management actions to mitigate risk. Our results showed that scat infection was most prevalent in areas with compost and, within parks, most abundant near other scats and human infrastructure, potentially increasing exposure risk for some human demographics. Our assessment of scat content supported the idea that access to anthropogenic foods increases coyote use of and defecation in spaces used by people, which, combined with increased infection associated with aggregated food sources, increases zoonotic disease risk. These results show that patterns of coyote scat infection and deposition are partially predictable, therefore suggesting how strategic landscape and attractant management could limit zoonotic disease risk to humans.
We found that 26.0% of scats contained E. multilocularis DNA, similar to the infection rate detected in scats from Calgary, Alberta, where 21.4% of scats were infected (Liccioli et al., 2014). Infection prevalence was lower than that detected in coyote intestines from Edmonton (> 50%; (Catalano et al., 2012; Luong et al., 2020; Sugden et al., 2020), presumably because of inconsistent shedding of eggs (Liccioli et al., 2014) or degradation of DNA in scats. That the infection rate was greatest in areas with compost and when scats contained anthropogenic food reflects a well-documented relationship between aggregated food sources accessed by multiple species and increased interspecific and intraspecific disease transmission (Becker et al., 2015; Gottdenker et al., 2015; Becker et al., 2018; Murray et al., 2019), including the known attraction of diseased coyotes to compost in Edmonton (Murray et al., 2016b). In this city, coyote scats near large compost piles had 10 times more Taeniidae (tapeworm) eggs compared to scats found in natural areas (Murray et al., 2016b), and mange was more prevalent in individuals detected near compost piles. Compost piles (Murray et al., 2015b) and other anthropogenic subsidies (Towns et al., 2009; Yeakel et al., 2009; Goodrich et al., 2011; Murray et al., 2016a) are most frequently accessed by individuals that are weak or diseased, meaning that infected scats may accumulate near these sites. When rodents access the same anthropogenic food sources, they likely ingest E. multilocularis eggs deposited in coyote scat and become infected as intermediate hosts (Thompson, 2017; Houston et al., 2021). Coyotes that prey on intermediate hosts are in turn infected or reinfected, and the cycle of disease transmission is perpetuated and enhanced. Low quality and anthropogenic food, including compost, may also degrade immune systems and limit disease immunity (Murray et al., 2016b; Sugden et al., 2020), including immunity to E. multilocularis, that might otherwise develop in older individuals (Sugden et al., 2023). Because compost often occurs near human residences and is used for gardening, this relationship speaks to increased risk of exposure for humans.
Understanding the natural history that dictates scat deposition could improve mitigation of human exposure to infected scats. Scats were deposited non-randomly, often on conspicuous objects, which increases their visual and olfactory impact (Bowen and Cowan, 1980; Allen et al., 1999; Barja, 2009; Barja and List, 2014). Scat deposition patterns in our study supported multiple mechanistic hypotheses (Table 1), especially territoriality (Bowen and Cowan, 1980; Allen et al., 1999) and temporal clustering of scats associated with elimination (Wells and Bekoff, 1981; Gese and Ruff, 1997). Several authors suggest that scat is deposited to achieve elimination in a time-dependent manner, and urine is the primary method of marking in coyotes (Barrette and Messier, 1980; Bowen and Cowan, 1980; Wells and Bekoff, 1981; Paquet, 1991; Gese and Ruff, 1997). However the dry climate and cold winters of Edmonton may favour scat for marking because urine quickly evaporates (Barja and List, 2014) or freezes. Urbanization may also favour scat-based signalling because food is abundant enough to produce it regularly, whereas wolves (Canis lupus) may rely more on urine because inconsistent feeding limits scat availability (Asa et al., 1985). That our scat model predicted only 38% of urination sites suggests there may be a difference in the relative importance of scat and urination (Barrette and Messier, 1980; Bowen and Cowan, 1980; Wells and Bekoff, 1981; Gese and Ruff, 1997), but our small sample size for urine sites limits the interpretation of this result.
The most important predictor of scat deposition was proximity to another scat, which is consistent with previous observations that coyotes respond strongly to existing marks (Bowen and Cowan, 1980) and overmarking behaviour, in which scats are deposited on or near existing scats (Ferkin and Pierce, 2007). Although several biological hypotheses explain overmarking (e.g., reinforcement of territorial boundaries, mate attraction, navigation, group cohesion), animals respond primarily to the top-most mark (Woodward et al., 2000; Ferkin and Pierce, 2007), which may encourage defecation on existing scats. Selection for scat deposition on convex slopes (i.e., peaks, ridges, top of bank) supports the territoriality hypothesis because territorial boundaries often coincide with topographical features (Moorcroft et al., 2006), including convex sites (Wilkinson and Francis, 1995). Because urban coyotes with stable home ranges primarily occupy naturalized areas (Gehrt et al., 2009; Gese et al., 2012; Dodge and Kashian, 2013; Mitchell et al., 2015; Murray et al., 2015b; Murray and St. Clair, 2017), the ecotone between naturalized and human-dominated areas likely demarcates territorial boundaries, so observed scat deposition near buildings and natural patch edges also supports the territoriality hypothesis. In Edmonton, selection of top of bank and ecotones for scat deposition likely increases exposure risk for humans because these areas often coincide with backyards and playgrounds. The tendency for scats to aggregate increases the value of determining correlates of their distribution for minimizing human exposure risk.
Selection of natural areas by coyotes within urban spaces is common (Gehrt et al., 2009; Gese et al., 2012; Dodge and Kashian, 2013; Mitchell et al., 2015; Murray and St. Clair, 2017) and presumably explains why scat increased where natural land cover was high, as predicted by the elimination hypothesis. Proximity to buildings and camps could also support this hypothesis if coyotes access food in these locations; coyote food and attractants are often present in yards (Elliot et al., 2016; Murray and St. Clair, 2017; Raymond and St. Clair, 2023b), and we frequently observed food and chewed anthropogenic objects in camps belonging to people experiencing homelessness (authors’ pers. obs.). The observed proximity between scats and human residences, including camps, suggests that some residents and people experiencing homelessness may have greater exposure to E. multilocularis. This population is vulnerable to several zoonoses and other kinds of infections (Leibler et al., 2016), which may occur simultaneously to make diagnosis more difficult (Raoult et al., 2001). Because aggregated food attracts coyotes and increases both wildlife disease and scat abundance, people who spend time and feed wildlife at the edge of their residence may be particularly vulnerable. We observed this circumstance often, sometimes in association with picnic and children’s play areas, in the form of bird, squirrel and deer feeders at the edges of unfenced yards that back on ravines in Edmonton (Raymond and St. Clair, 2023b). Wildlife feeding is more common in urban than rural areas (Tryjanowski et al., 2015) and may have increased during the COVID-19 pandemic (Hockenhull et al., 2021).
Models predicting scat abundance in city-delineated green spaces revealed that small parks in heavily urbanized matrices are associated with greater scat prevalence and, hence, disease risk. This situation characterized several small natural areas that may concentrate animals in limited preferred habitat (Gese et al., 2012) and increase local disease risk for coyotes and people. We provide the first detailed study of the relationship between encampments belonging to those experiencing homelessness and metrics of coyote use and associated zoonotic risk. Greater scat abundance in green spaces with more encampments could reflect attraction to camps because of garbage and other food subsidies that were common in these areas, or it could reflect that green spaces selected by people experiencing homelessness for camping (Koprowska et al., 2020) are similar to the natural areas selected by coyotes (Gehrt et al., 2009; Gese et al., 2012; Mitchell et al., 2015; Murray and St. Clair, 2017). The apparent abundance of scat in green spaces with intermediate camp density could reflect that, when camp density is very high, associated human presence limits habitat suitability for coyotes. Because site selection for defecation occurs at a fine scale (Brattstrom, 1999; Barja, 2009), the low performance of these models relative to those predicting scat deposition could suggest that site selection occurs at a finer scale than we measured in this analysis.
Similar to other studies, our analysis of scat content showed that urban coyotes extensively access anthropogenic food, including birdseed, garbage, compost, and other anthropogenic material (Murray et al., 2015b; Larson et al., 2020; Sugden et al., 2021; Raymond and St. Clair, 2023b), in addition to natural food items (Morey et al., 2007; Gehrt and Riley, 2010; Murray et al., 2015b; Newsome et al., 2015). Fruit was an important winter food source that appeared in 52.9% of scats, reflecting consumption of native mountain ash berries (Sorbus lacustre), non-native crab apples, and human-provisioned compost or fruit. Performance for models predicting scat content was low, perhaps because of the non-random deposition sites of scat (above) and gut transit time, both of which cause geographically separated sites of consumption and defecation. Despite model limitations, we found positive associations between several anthropogenic features (e.g., road density, proximity to buildings, camps, playgrounds, anthropogenic land cover) and scats containing anthropogenic material (e.g., unknown anthropogenic material, birdseed, garbage), which supports the well-documented relationship between anthropogenic habitat and diet in coyotes (Newsome et al., 2015; Larson et al., 2020). Coyotes that consume anthropogenic food are associated with larger and more urbanized home ranges (Murray et al., 2015b; Newsome et al., 2015), greater incidence of disease (Murray et al., 2015b), and greater use of human-dominated areas (Murray and St. Clair, 2017; Larson et al., 2020), all of which promote human-wildlife conflict (Murray et al., 2015a). Both our data, and other studies suggest that consumption of low-quality food reduces coyote immunity and increases disease (Murray et al., 2016b; Sugden et al., 2021), so the scats containing anthropogenic material and located near human infrastructure may be more likely to expose people to disease risk. By contrast, scats containing natural foods (i.e., natural prey and vegetation) were predicted by greater distance from camps, buildings, and playgrounds, showing that some urban-adapted coyotes retain a natural diet (Gehrt and Riley, 2010; Newsome et al., 2015; Larson et al., 2020). Studies in bears (Hopkins et al., 2010) and coyotes (Murray et al., 2015b) suggest that maintenance of a natural diet reduces the likelihood of food conditioning. Importantly, the observation that scats containing fruit and unknown anthropogenic material are predicted by proximity to other scats suggests that these carbohydrate-rich foods aggregate animals and scats, likely accelerating disease transmission and increasing infection prevalence (Becker et al., 2015) and exposure risk for humans.
Our study had some limitations that bear on management implications. For our analysis of infection status using qPCR, our inability to calculate effort or more equally stratify scat collection spatially or temporally limited the sensitivity of our statistical analyses. Our finding that infection rate was lowest in autumn but did not statistically differ among seasons contrasts the results of another study, which found that coyote scat infection was most frequent in spring and depended on relative abundance of intermediate hosts (Liccioli et al., 2014). For our analysis of scat deposition patterns, our use of snow tracking limited data collection to months with snow, despite differences in coyote behaviour and territoriality among biological seasons (Allen et al., 1999). We did not account for scat removal from the landscape, by weathering, snowfall, or coprophagy (Lonsinger et al., 2016), and we did not record whether scats were accompanied by urine, which could have different value as scent marks (Bowen and Cowan, 1980). Because we identified scat content visually, we relied on diet items that were indigestible and discernible that may have underestimated well-digested material. A previous study showed that coyote scat content assessed visually differed considerably from content assessed via DNA metabarcoding, with metabarcoding often detecting well-digested anthropogenic material that could not be visually discerned (Henger et al., 2022).
Despite those limitations, our study provides information that might be used to mitigate the risk of exposure for people to a new haplotype of the zoonotic tapeworm, E. multilocularis, that is emerging as alveolar echinococcosis in Alberta (Massolo et al., 2019; Houston et al., 2021; Santa et al., 2021). Elsewhere in North America, this haplotype has been detected in dogs (Zajac et al., 2020; Kuroki et al., 2022), foxes (Polish et al., 2022; Robbins et al., 2022), and people (Houston et al., 2021; Polish et al., 2021; Schurer et al., 2021; Polish et al., 2022). We showed that compost and consumption of anthropogenic food are associated with a higher prevalence of infected coyote scats, suggesting that aggregations of food that attract both coyotes and prey increase rates of transmission and infection. These sources should be secured from coyotes. Similarly, household compost, garbage, birdseed, fallen fruit, and other wildlife attractants should be managed to prevent attraction by coyotes and aggregated feeding (Elliot et al., 2016; Murray and St. Clair, 2017; Henger et al., 2022; Raymond and St. Clair, 2023b). Intentional feeding of wildlife undoubtedly concentrates scats, raises disease risk to humans from many zoonoses, and is consequently prohibited in Edmonton (City of Edmonton, 2021) and many other cites (Clement and Bunce, 2022), but may not be adequately communicated as a disease risk. Because scats are more abundant near natural patch edges, where natural cover is high and where slopes are convex, this combination of microhabitat features could be avoided where human behaviour increases risk of contact with scat or soil (e.g., playgrounds, schools, community gardens, dog parks). Avoidance could be achieved through proactive landscape management (e.g., fencing) at existing facilities and by locating new facilities strategically. Because coyotes are stimulated to mark with scat where other individuals have marked (Bowen and Cowan, 1980), removal of coyote scats near human-use facilities could discourage that behaviour. The abundance of scats near camps and in green spaces with abundant camps suggests that those experiencing homelessness may be at increased risk of AE, especially given barriers to accessing healthcare (Cernadas and Fernandez, 2021) and high rates of immunocompromise (Raoult et al., 2001). Safe, affordable, and inclusive housing options and awareness of symptoms among health care professionals that care for those experiencing homelessness could mitigate risk. In small green spaces within heavily urbanized habitat, where scats are more abundant, signage or targeted education campaigns could be valuable. Fruit is important winter food for coyotes in Edmonton, and fruit-bearing trees and shrubs appear to increase scat abundance and likely increase disease risk. We therefore recommend that non-fruit-bearing, native species be planted near playgrounds and urban parks, especially near picnic sites and human residences. In Edmonton and perhaps other cities, naturalization campaigns have favoured berry-producing shrubs. Where these species are favoured for their utility to people or wildlife, fencing and education could reduce risk of exposure to tapeworm eggs.
Data availability statement
The datasets presented in this study can be found in online repositories. The names of the repository/repositories and accession number(s) can be found below: https://github.com/sageraymond/CoyoteScat.
Ethics statement
Ethical approval was not required for the study involving animals in accordance with the local legislation and institutional requirements because observation and collection of scats from the landscape does not require ethics approval.
Author contributions
SR: Conceptualization, Data curation, Formal analysis, Funding acquisition, Investigation, Methodology, Project administration, Software, Visualization, Writing – original draft, Writing – review & editing. DS: Conceptualization, Data curation, Funding acquisition, Investigation, Methodology, Project administration, Writing – review & editing. MAS: Data curation, Investigation, Methodology, Resources, Writing – review & editing. CCSC: Conceptualization, Formal analysis, Funding acquisition, Investigation, Methodology, Project administration, Resources, Supervision, Writing – review & editing.
Funding
The author(s) declare financial support was received for the research, authorship, and/or publication of this article. DS and SR were each supported by a Canada Graduate Scholarship (Master’s) from the Natural Sciences and Engineering Research Council of Canada (NSERC), a Walter H. Johns Graduate Fellowship from the University of Alberta, and an Alberta Graduate Excellence Fellowship from the Province of Alberta. Additional financial support was provided by an NSERC Discovery Grant (RGPIN-2017-05915) and a University of Alberta Faculty of Science Research Fellowship awarded to CCSC.
Acknowledgments
We thank many undergraduate students and community members that collected the 269 scats used to study infection status.
Conflict of interest
Author MAS was employed by the company IDEXX Laboratories, Inc. This laboratory offers the Echinococcus RealPCR™ Panel on a commercial basis and performed the testing described in this study. IDEXX played no role in the study design, in the collection and interpretation of data, or in the decision to submit the manuscript for publication.
The remaining authors declare that the research was conducted in the absence of any commercial or financial relationships that could be construed as a potential conflict of interest.
Publisher’s note
All claims expressed in this article are solely those of the authors and do not necessarily represent those of their affiliated organizations, or those of the publisher, the editors and the reviewers. Any product that may be evaluated in this article, or claim that may be made by its manufacturer, is not guaranteed or endorsed by the publisher.
Supplementary material
The Supplementary Material for this article can be found online at: https://www.frontiersin.org/articles/10.3389/fcosc.2023.1294693/full#supplementary-material
References
Aho K., Derryberry D., Peterson T. (2014). Model selection for ecologists: the worldviews of AIC and BIC. Ecology 95, 631–636. doi: 10.1890/13-1452.1
Allen J. J., Bekoff M., Crabtree R. L. (1999). An observational study of coyote (Canis latrans) scent-marking and territoriality in Yellowstone National Park. Ethology 105, 289–302. doi: 10.1046/j.1439-0310.1999.00397.x
Apps P., Rafiq K., McNutt J. W. (2019). “Do carnivores have a world wide web of interspecific scent signals?,” in Chemical Signals in Vertebrates, vol. 14 . Ed. Buesching C. D. (Cham: Springer International Publishing), 182–202.
Asa C., Mech L., Seal U. (1985). The use of urine, feces, and anal-gland secretions in scent-marking by a captive wolf (Canis-lupus) pack. Anim. Behav. 33, 1034–1036. doi: 10.1016/S0003-3472(85)80043-9
Azocar-Aedo L., Smits H. L., Monti G. (2014). Leptospirosis in dogs and cats: Epidemiology, clinical disease, zoonotic implications and prevention. Archivos Medicina Veterinaria 46, 337–348. doi: 10.4067/S0301-732X2014000300002
Barja I. (2009). Decision making in plant selection during the faecal-marking behaviour of wild wolves. Anim. Behav. 77, 489–493. doi: 10.1016/j.anbehav.2008.11.004
Barja I., List R. (2014). The role of spatial distribution of faeces in coyote scent marking behaviour. Polish J. Ecol. 62, 373–383. doi: 10.3161/104.062.0215
Barrette C., Messier F. (1980). Scent-marking in free-ranging coyotes, Canis-latrans. Anim. Behav. 28, 814–819. doi: 10.1016/S0003-3472(80)80141-2
Bastien M., Vaniscotte A., Combes B., Umhang G., Germain E., Gouley V., et al. (2018). High density of fox and cat faeces in kitchen gardens and resulting rodent exposure to Echinococcus multilocularis and Toxoplasma gondii. Folia Parasitologica 65, 002. doi: 10.14411/fp.2018.002
Becker D. J., Hall R. J., Forbes K. M., Plowright R. K., Altizer S. (2018). Anthropogenic resource subsidies and host-parasite dynamics in wildlife. Philos. Trans. R. Soc. B-Biological Sci. 373, 20170086. doi: 10.1098/rstb.2017.0086
Becker D. J., Streicker D. G., Altizer S. (2015). Linking anthropogenic resources to wildlife-pathogen dynamics: a review and meta-analysis. Ecol. Lett. 18, 483–495. doi: 10.1111/ele.12428
Bengis R. H., Leighton F. A., Fischer J. R., Artois M., Morner T., Tate C. M. (2004). The role of wildlife in emerging and re-emerging zoonoses. Rev. Scientifique Et Technique-Office Int. Des. Epizooties 23, 497–511. doi: 10.20506/rst.23.2.1498
Bowen W. D., Cowan I. (1980). Scent marking in coyotes. Can. J. Zoology-Revue Can. Zoologie 58, 473–480. doi: 10.1139/z80-065
Boyce M. S., McDonald L. L. (1999). Relating populations to habitats using resource selection functions. Trends Ecol. Evol. 14, 268–272. doi: 10.1016/S0169-5347(99)01593-1
Boyce M. S., Vernier P. R., Nielsen S. E., Schmiegelow F. K. A. (2002). Evaluating resource selection functions. Ecol. Model. 157, 281–300. doi: 10.1016/S0304-3800(02)00200-4
Bradley C. A., Altizer S. (2007). Urbanization and the ecology of wildlife diseases. Trends Ecol. Evol. 22, 95–102. doi: 10.1016/j.tree.2006.11.001
Brunetti E., Kern P., Vuitton D. A. (2010). Expert consensus for the diagnosis and treatment of cystic and alveolar echinococcosis in humans. Acta Tropica 114, 1–16. doi: 10.1016/j.actatropica.2009.11.001
Catalano S., Lejeune M., Liccioli S., Verocai G. G., Gesy K. M., Jenkins E. J., et al. (2012). Echinococcus multilocularis in urban coyotes, Alberta, Canada. Emerging Infect. Dis. 18, 1625–1628. doi: 10.3201/eid1810.120119
Cernadas A., Fernandez A. (2021). Healthcare inequities and barriers to access for homeless individuals: A qualitative study in Barcelona (Spain). Int. J. Equity Health 20, 84. doi: 10.1186/s12939-021-01409-2
City of Edmonton (2007) Natural Connections Strategic Plan. Available at: https://www.edmonton.ca/public-files/assets/document?path=PDF/Natural_Connections_-_Strategic_Plan_JUNE_09.pdf.
City of Edmonton (2013). Edmonton, your gateway to scenic settings (Edmonton, Canada: Edmonton Economic Development Corporation). Available at: https://passionpassport.com/edmonton-guide-gateway-north/.
City of Edmonton (2014). “Urban primary land and vegetation inventory (uPLVI). Interpretation manual,” in Prepared for: Parks and Biodiversity Section, Sustainable Development, the City of Edmonton, Alberta, 4th ed. (Edmonton Alberta: Greenlink Forestry Inc).
City of Edmonton (2019) 2019 Municipal Census Results. Available at: https://www.edmonton.ca/city_government/facts_figures/municipal-census-results.aspx.
City of Edmonton (2021) The City of Edmonton Bylaw 19553 Feeding of wildlife bylaw. Available at: https://pub-edmonton.escribemeetings.com/filestream.ashx?DocumentId=81118 (Accessed 19 Feb 2023).
City of Edmonton (2022a) Parks: List of all Park Spaces in Edmonton. Available at: https://data.edmonton.ca/Outdoor-Recreation/Parks/gdd9-eqv9.
City of Edmonton (2022b) City of Edmonton’s Open Data Portal. Available at: https://data.edmonton.ca/ (Accessed 21 Jul 2022).
Clement B., Bunce S. (2022). Coyotes and more-than-human commons: exploring co-existence through Toronto’s Coyote Response Strategy. Urban Geogr. 44, 2144–2162. doi: 10.1080/02723638.2022.2068826
Conraths F. J., Probst C., Possenti A., Boufana B., Saulle R., La Torre G., et al. (2017). Potential risk factors associated with human alveolar echinococcosis: Systematic review and meta-analysis. PloS Negl. Trop. Dis. 11, e0005801. doi: 10.1371/journal.pntd.0005801
Coxe S., West S. G., Aiken L. S. (2009). The analysis of count data: A gentle introduction to Poisson regression and its alternatives. J. Pers. Assess. 91, 121–136. doi: 10.1080/00223890802634175
Crabtree R. L., Sheldon J. W. (1999). The ecological role of coyotes on Yellowstone’s northern range. Yellowstone Sci. 7, 15–23.
Cumming G. S. (2000). Using between-model comparisons to fine-tune linear models of species ranges. J. Biogeography 27, 441–455. doi: 10.1046/j.1365-2699.2000.00408.x
Dodge W. B., Kashian D. M. (2013). Recent distribution of coyotes across an urban landscape in southeastern Michigan. J. Fish Wildlife Manage. 4, 377–385. doi: 10.3996/062013-jfwm-040
Dormann C. F., Elith J., Bacher S., Buchmann C., Carl G., Carre G., et al. (2013). Collinearity: A review of methods to deal with it and a simulation study evaluating their performance. Ecography 36, 27–46. doi: 10.1111/j.1600-0587.2012.07348.x
Eberhardt L. L. (2003). What should we do about hypothesis testing? J. Wildlife Manage. 67, 241–247. doi: 10.2307/3802765
Eckert J., Deplazes P. (2004). Biological, epidemiological, and clinical aspects of echinococcosis, a zoonosis of increasing concern. Clin. Microbiol. Rev. 17, 107–135. doi: 10.1128/CMR.17.1.107-135.2004
Elbroch M., MacFarland C. (2019). Mammal Tracks & Sign: A Guide to North American Species. 2nd ed. (Lanham (MD: Rowman & Littlefield Publishing).
Elliot E. E., Vallance S., Molles L. E. (2016). Coexisting with coyotes (Canis latrans) in an urban environment. Urban Ecosyst. 19, 1335–1350. doi: 10.1007/s11252-016-0544-2
Environment Canada (2022) Canadian climate normals 1981–2010 station data. Available at: https://climate.weather.gc.ca/climate_normals/results_1981_2010_e.html?searchType=stnName%26txtStationName=edmonton%26searchMethod=contains%26txtCentralLatMin=0%26txtCentralLatSec=0%26txtCentralLongMin=0%26txtCentralLongSec=0%26stnID=1867%26dispBack=0.
Ferkin M. H., Pierce A. A. (2007). Perspectives on over-marking: Is it good to be on top? J. Ethology 25, 107–116. doi: 10.1007/s10164-006-0012-1
Gehrt S. D., Anchor C., White L. A. (2009). Home range and landscape use of coyotes in a metropolitan landscape: Conflict or coexistence? J. Mammalogy 90, 1045–1057. doi: 10.1644/08-MAMM-A-277.1
Gehrt S. D., Riley S. P. D. (2010). “Coyotes (Canis latrans),” in Urban carnivores: ecology, conflict, and conservation. Eds. Gehrt S. D., Riley S. P. D., Cypher B. L. (Baltimore (MD: The Johns Hopkins University Press), 79–95.
Gese E. M. (2001). Territorial defense by coyotes (Canis latrans) in Yellowstone National Park, Wyoming: Who, how, where, when, and why. Can. J. Zoology 79, 980–987. doi: 10.1139/z01-054
Gese E. M., Morey P. S., Gehrt S. D. (2012). Influence of the urban matrix on space use of coyotes in the Chicago metropolitan area. J. Ethology 30, 413–425. doi: 10.1007/s10164-012-0339-8
Gese E. M., Ruff R. L. (1997). Scent-marking by coyotes, Canis latrans: The influence of social and ecological factors. Anim. Behav. 54, 1155–1166. doi: 10.1006/anbe.1997.0561
Gibb R., Redding D. W., Chin K. Q., Donnelly C. A., Blackburn T. M., Newbold T., et al. (2020). Zoonotic host diversity increases in human-dominated ecosystems. Nature 584, 398–402. doi: 10.1038/s41586-020-2562-8
Goodrich J. M., Seryodkin I., Miquelle D. G., Bereznuk S. L. (2011). Conflicts between Amur (Siberian) tigers and humans in the Russian Far East. Biol. Conserv. 144, 584–592. doi: 10.1016/j.biocon.2010.10.016
Gottdenker N. L., Streicker D. G., Faust C. L., Carroll C. R. (2015). Anthropogenic land use change and infectious diseases: A review of the evidence. EcoHealth 11, 619–632. doi: 10.1007/s10393-014-0941-z
Hegyi G., Zsolt Garamszegi L. (2011). Using information theory as a substitute for stepwise regression in ecology and behavior. Behav. Ecol. Sociobiology 65, 69–76. doi: 10.1007/s00265-010-1036-7
Henger C. S., Hargous E., Nagy C. M., Weckel M., Wultsch C., Krampis K., et al. (2022). DNA metabarcoding reveals that coyotes in New York City consume wide variety of native prey species and human food. Peerj 10, e13788. doi: 10.7717/peerj.13788
Hockenhull J., Squibb K., Cameron A. (2021). How has the COVID-19 pandemic affected the way we access and interact with the countryside and the animals within it? Animals 11, 2281. doi: 10.3390/ani11082281
Hopkins J. B., Herrero S., Shideler R. T., Gunther K. A., Schwartz C. C., Kalinowski S. T. (2010). A proposed lexicon of terms and concepts for human-bear management in North America. Ursus 21, 154–168. doi: 10.2192/URSUS-D-10-00005.1
Hosmer D. W., Lemeshow S. (2000). Applied Logistic Regression (New York (NY: John Wiley & Sons, Inc.).
Houston S., Belga S., Buttenschoen K., Cooper R., Girgis S., Gottstein B., et al. (2021). Epidemiological and clinical characteristics of alveolar echinococcosis: An emerging infectious disease in Alberta, Canada. Am. J. Trop. Med. hygiene 104, 1863–1869. doi: 10.4269/ajtmh.20-1577
Jenness (2013). DEM Surface Tools (Flagstaff, USA: Jenness Enterprises). Available at: http://www.jennessent.com/arcgis/surface_area.htm.
Jones K. E., Patel N. G., Levy M. A., Storeygard A., Balk D., Gittleman J. L., et al. (2008). Global trends in emerging infectious diseases. Nature 451, 990–993. doi: 10.1038/nature06536
Julien D. A., Sargeant J. M., Filejski C., Versluis A. M., Waind V., Harper S. L. (2021). Unleashing the literature: A scoping review of canine zoonotic and vectorborne disease research in Canis familiaris in North America. Anim. Health Res. Rev. 22, 26–39. doi: 10.1017/S1466252320000237
Koprowska K., Kronenberg J., Kuzma I. B., Laszkiewicz E. (2020). Condemned to green? Accessibility and attractiveness of urban green spaces to people experiencing homelessness. Geoforum 113, 1–13. doi: 10.1016/j.geoforum.2020.04.017
Kuroki K., Morishima Y., Dorr L., Cook C. R. (2022). Alveolar echinococcosis in a dog in Missouri, USA. J. Veterinary Diagn. Invest. 34, 746–751. doi: 10.1177/10406387221104754
Larson R. N., Brown J. L., Karels T., Riley S. P. D. (2020). Effects of urbanization on resource use and individual specialization in coyotes (Canis latrans) in southern California. PLoS One 15, 1–23. doi: 10.1371/journal.pone.0228881
Lass A., Szostakowska B., Myjak P., Korzeniewski K. (2015). The first detection of Echinococcus multilocularis DNA in environmental fruit, vegetable, and mushroom samples using nested PCR. Parasitol. Res. 114, 4023–4029. doi: 10.1007/s00436-015-4630-9
Leibler J. H., Zakhour C. M., Gadhoke P., Gaeta J. M. (2016). Zoonotic and vector-borne infections among urban homeless and marginalized people in the United States and Europe 1990-2014. Vector-Borne Zoonotic Dis. 16, 435–444. doi: 10.1089/vbz.2015.1863
Liccioli S., Kutz S. J., Ruckstuhl K. E., Massolo A. (2014). Spatial heterogeneity and temporal variations in Echinococcus multilocularis infections in wild hosts in a North American urban setting. Int. J. Parasitol. 44, 457–465. doi: 10.1016/j.ijpara.2014.03.007
Lonsinger R. C., Gese E. M., Knight R. N., Johnson T. R., Waits L. P. (2016). Quantifying and correcting for scat removal in noninvasive carnivore scat surveys. Wildlife Biol. 22, 45–54. doi: 10.2981/wlb.00179
Luong L. T., Chambers J. L., Moizis A., Stock T. M., St. Clair C. C. (2020). Helminth parasites and zoonotic risk associated with urban coyotes (Canis latrans) in Alberta, Canada. J. Helminthology 94, e25. doi: 10.1017/S0022149X1800113X
Macdonald D. W. (1980). Patterns of scent marking with urine and faeces amongst carnivore communities (London, United Kingdom: Symposia of the Zoological Society of London), 107–139.
Mackenstedt U., Jenkins D., Romig T. (2015). The role of wildlife in the transmission of parasitic zoonoses in peri-urban and urban areas. Int. J. Parasitology-Parasites Wildlife 4, 71–79. doi: 10.1016/j.ijppaw.2015.01.006
Massolo A., Klein C., Kowalewska-Grochowska K., Belga S., MacDonald C., Vaughan S., et al. (2019). European Echinococcus multilocularis identified in patients in Canada. New Engl. J. Med. 381, 384–385. doi: 10.1056/NEJMc1814975
McFadden D. (1979). “Quantitative methods for analysing travel behaviour of individuals,” in Behavioural Travel Modelling (London, UK: Routledge), 279–318.
Mitchell N., Strohbach M. W., Pratt R., Finn W. C., Strauss E. G. (2015). Space use by resident and transient coyotes in an urban-rural landscape mosaic. Wildlife Res. 42, 461–469. doi: 10.1071/WR15020
Moorcroft P. R., Lewis M. A., Crabtree R. L. (2006). Mechanistic home range models capture spatial patterns and dynamics of coyote territories in Yellowstone. Proc. R. Soc. B-Biological Sci. 273, 1651–1659. doi: 10.1098/rspb.2005.3439
Morey P. S., Gese E. M., Gehrt S. (2007). Spatial and temporal variation in the diet of coyotes in the Chicago metropolitan area. Am. Midland Nat. 158, 147–161. doi: 10.1674/0003-0031(2007)158[147:SATVIT]2.0.CO;2
Murray M., Cembrowski A., Latham A. D. M., Lukasik V. M., Pruss S., St. Clair C. C. (2015a). Greater consumption of protein-poor anthropogenic food by urban relative to rural coyotes increases diet breadth and potential for human-wildlife conflict. Ecography 38, 1235–1242. doi: 10.1111/ecog.01128
Murray M., Edwards M. A., Abercrombie B., St. Clair C. C. (2015b). Poor health is associated with use of anthropogenic resources in an urban carnivore. Proc. R. Soc. B-Biological Sci. 282, 20150009. doi: 10.1098/rspb.2015.0009
Murray M., Hill J., Whyte P., St. Clair C. C. (2016b). Urban compost attracts coyotes, contains toxins, and may promote disease in urban-adapted wildlife. EcoHealth 13, 285–292. doi: 10.1007/s10393-016-1105-0
Murray M. H., Becker D. J., Hall R. J., Hernandez S. M. (2016a). Wildlife health and supplemental feeding: A review and management recommendations. Biol. Conserv. 204, 163–174. doi: 10.1016/j.biocon.2016.10.034
Murray M. H., Sanchez C. A., Becker D. J., Byers K. A., Worsley-Tonks K. E. L., Craft M. E. (2019). City sicker? A meta-analysis of wildlife health and urbanization. Front. Ecol. Environ. 17, 575–583. doi: 10.1002/fee.2126
Murray M. H., St. Clair C. C. (2017). Predictable features attract urban coyotes to residential yards. J. Wildlife Manage. 81, 593–600. doi: 10.1002/jwmg.21223
Newsome S. D., Garbe H. M., Wilson E. C., Gehrt S. D. (2015). Individual variation in anthropogenic resource use in an urban carnivore. Oecologia 178, 115–128. doi: 10.1007/s00442-014-3205-2
Nielsen S. E., Cranston J., Stenhouse G. B. (2009). Identification of priority areas for grizzly bear conservation and recovery in Alberta, Canada. J. Conserv. Plann. 5, 38–60.
Nonaka N., Kamiya M., Kobayashi F., Ganzorig S., Ando S., Yagi K., et al. (2009). Echinococcus multilocularis infection in pet dogs in Japan. Vector-Borne Zoonotic Dis. 9, 201–205. doi: 10.1089/vbz.2008.0097
Paquet P. C. (1991). Scent-marking behavior of sympatric wolves (Canis-lupus) and coyotes (C-latrans) in Riding-Mountain-National-Park. Can. J. Zoology-Revue Can. Zoologie 69, 1721–1727. doi: 10.1139/z91-240
Polish L. B., O’Connell E. M., Barth T. F. E., Gottstein B., Zajac A., Gibson P. C., et al. (2022). European haplotype of Echinococcus multilocularis in the United States. New Engl. J. Med. 387, 1902–1904. doi: 10.1056/NEJMc2210000
Polish L. B., Pritt B., Barth T. F. E., Gottstein B., O’Connell E. M., Gibson P. C. (2021). First European haplotype of Echinococcus multilocularis identified in the United States: An emerging disease? Clin. Infect. Dis. 72, 1117–1123. doi: 10.1093/cid/ciaa245
Porter E., Seguin M. A., Estrada M., Szlosek D., Massolo A., Visscher D. R. (2022). Assessing the potential for infections of Echinococcus multilocularis in dogs in a hotspot of human alveolar echinococcosis infections in North America. Veterinary Parasitology- Regional Stud. Rep. 29, 100704. doi: 10.1016/j.vprsr.2022.100704
Poulle M.-L., Bastien M., Richard Y., Josse-Dupuis E., Aubert D., Villena I., et al. (2017). Detection of Echinococcus multilocularis and other foodborne parasites in fox, cat and dog faeces collected in kitchen gardens in a highly endemic area for alveolar echinococcosis. Parasite 24, 29. doi: 10.1051/parasite/2017031
Prange S., Gehrt S. D., Wiggers E. P. (2004). Influences of anthropogenic resources on raccoon (Procyon lotor) movements and spatial distribution. J. Mammalogy 85, 483–490. doi: 10.1644/BOS-121
Raoult D., Foucault C., Brouqui P. (2001). Infections in the homeless. Lancet Infect. Dis. 1, 77–84. doi: 10.1016/S1473-3099(01)00062-7
Raymond S., St Clair C. C. (2023a). Urban coyotes select cryptic den sites near human development where conflict rates increase. J. Wildlife Manage. 87, e22323. doi: 10.1002/jwmg.22323
Raymond S., St. Clair C. C. (2023b). Coyotes access diverse anthropogenic attractants at the ecotone between natural and residential urban areas. Urban Ecosyst. 26, 1589–1605. doi: 10.1007/s11252-023-01402-3
Raymond S., St. Clair C. C. (2023c). Urban magpies frequently feed on coyote scats and may spread an emerging zoonotic tapeworm. EcoHealth. doi: 10.1007/s10393-023-01664-5
Reed S. E., Merenlender A. M. (2011). Effects of management of domestic dogs and recreation on carnivores in protected areas in northern California. Conserv. Biol. 25, 504–513. doi: 10.1111/j.1523-1739.2010.01641.x
Robbins W. T., Galeuzzi O., Graham K., Greenwood S. J., Jones M. E. B., Buote M., et al. (2022). Echinococcus multilocularis infection in a red fox (Vulpes vulpes) on Prince Edward Island, Canada. Can. veterinary J. = La Rev. veterinaire Can. 63, 962–966.
Rothenburger J. L., Himsworth C. H., Nemeth N. M., Pearl D. L., Jardine C. M. (2017). Environmental factors and zoonotic pathogen ecology in urban exploiter species. EcoHealth 14, 630–641. doi: 10.1007/s10393-017-1258-5
Santa M. A., Musiani M., Ruckstuhl K. E., Massolo A. (2021). A review on invasions by parasites with complex life cycles: The European strain of Echinococcus multilocularis in North America as a model. Parasitology 148, 1532–1544. doi: 10.1017/S0031182021001426
Schurer J. M., Tsybina P., Gesy K. M., Kolapo T. U., Skinner S., Hill J. E., et al. (2021). Molecular evidence for local acquisition of human alveolar echinococcosis in Saskatchewan, Canada. J. Infect. Dis. 223, 1015–1018. doi: 10.1093/infdis/jiaa473
Sharpe D. (2015). Chi-square test is statistically significant: Now what? Pract. Assessment Research Eval. 20, 1–10.
Starfield A. M. (1997). A pragmatic approach to modeling for wildlife management. J. Wildlife Manage. 61, 261–270. doi: 10.2307/3802581
Stephens P. A., Buskirk S. W., del Rio C. M. (2007). Inference in ecology and evolution. Trends Ecol. Evol. 22, 192–197. doi: 10.1016/j.tree.2006.12.003
Sugden S., Murray M., Edwards M. A., St. Clair C. C. (2021). Inter-population differences in coyote diet and niche width along an urban–suburban–rural gradient. J. Urban Ecol. 7, 1–12. doi: 10.1093/jue/juab034
Sugden S., Sanderson D., Ford K., Stein L. Y., St. Clair C. C. (2020). An altered microbiome in urban coyotes mediates relationships between anthropogenic diet and poor health. Sci. Rep. 10, 22207. doi: 10.1038/s41598-020-78891-1
Sugden S., Steckler D. K., Sanderson D., Abercrombie B., Abercrombie D., Seguin M. A., et al. (2023). Age-dependent relationships among diet, body condition, and Echinococcus multilocularis infection in urban coyotes. PLoS One 18, e0290755. doi: 10.1371/journal.pone.0290755
Symonds M. R. E., Moussalli A. (2011). A brief guide to model selection, multimodel inference and model averaging in behavioural ecology using Akaike’s information criterion. Behav. Ecol. Sociobiology 65, 13–21. doi: 10.1007/s00265-010-1037-6
Thompson R. (2017). “Chapter two - biology and systematics of Echinococcus,” in Advances in Parasitology, vol. 95 . Eds. Thompson R. C. A., Deplazes P., Lymbery A. J. (London, UK: Academic Press), 65–109.
Toews E., Musiani M., Checkley S., Visscher D., Massolo A. (2021). A global assessment of Echinococcus multilocularis infections in domestic dogs: Proposing a framework to overcome past methodological heterogeneity. Int. J. Parasitol. 51, 379–392. doi: 10.1016/j.ijpara.2020.10.008
Towns L., Derocher A. E., Stirling I., Lunn N. J., Hedman D. (2009). Spatial and temporal patterns of problem polar bears in Churchill, Manitoba. Polar Biol. 32, 1529–1537. doi: 10.1007/s00300-009-0653-y
Trailforks Mapping Inc (2022) Trailforks Trail Layer for Edmonton, Alberta, Canada. Available at: https://www.trailforks.com/ (Accessed 1 Aug 2022).
Tredennick A. T., Hooker G., Ellner S. P., Adler P. B. (2021). A practical guide to selecting models for exploration, inference, and prediction in ecology. Ecology 102, e03336. doi: 10.1002/ecy.3336
Tryjanowski P., Skorka P., Sparks T. H., Biadun W., Brauze T., Hetmanski T., et al. (2015). Urban and rural habitats differ in number and type of bird feeders and in bird species consuming supplementary food. Environ. Sci. pollut. Res. 22, 15097–15103. doi: 10.1007/s11356-015-4723-0
Umhang G., Bastien M., Bastid V., Poulle M.-L., Boué F. (2022). High variability in the number of E. multilocularis eggs in cat feces collected in the field. Parasitol. Int. 89, 102583. doi: 10.1016/j.parint.2022.102583
Veall M. R., Zimmermann K. (1994). Evaluating pseudo R2s for binary probit models. Qual. Quantity 28, 151–164. doi: 10.1007/BF01102759
Way J. G. (2009). Observations of coywolves, Canis latrans x lycaon, crossing bridges and using human structures on Cape Cod, Massachusetts. Can. Field-Naturalist 123, 206–209. doi: 10.22621/cfn.v123i3.965
Wells M., Bekoff M. (1981). An observational study of scent-marking in coyotes, Canis-latrans. Anim. Behav. 29, 332–350. doi: 10.1016/S0003-3472(81)80093-0
Woodward R. L., Bartos K., Ferkin M. H. (2000). Meadow voles (Microtus pennsylvanicus) and prairie voles (M-ochrogaster) differ in their responses to over-marks from opposite- and same-sex conspecifics. Ethology 106, 979–992. doi: 10.1046/j.1439-0310.2000.00618.x
Wright A. N., Gompper M. E. (2005). Altered parasite assemblages in raccoons in response to manipulated resource availability. Oecologia 144, 148–156. doi: 10.1007/s00442-005-0018-3
Wurth A. M., Ellington E. H., Gehrt S. D. (2020). Golf courses as potential habitat for urban coyotes. Wildlife Soc. Bull. 44, 333–341. doi: 10.1002/wsb.1081
Yeakel J. D., Patterson B. D., Fox-Dobbs K., Okumura M. M., Cerling T. E., Moore J. W., et al. (2009). Cooperation and individuality among man-eating lions. Proc. Natl. Acad. Sci. U. S. A. 106, 19040–19043. doi: 10.1073/pnas.0905309106
Keywords: Canis latrans, coyote, diet, disease ecology, habitat selection, scat, urban ecology, urban wildlife
Citation: Raymond S, Steckler DK, Seguin MA and St. Clair CC (2024) Coyote scat in cities increases risk of human exposure to an emerging zoonotic disease in North America. Front. Conserv. Sci. 4:1294693. doi: 10.3389/fcosc.2023.1294693
Received: 15 September 2023; Accepted: 12 December 2023;
Published: 08 January 2024.
Edited by:
Hiroshi Tsunoda, Center for Environmental Science, JapanCopyright © 2024 Raymond, Steckler, Seguin and St. Clair. This is an open-access article distributed under the terms of the Creative Commons Attribution License (CC BY). The use, distribution or reproduction in other forums is permitted, provided the original author(s) and the copyright owner(s) are credited and that the original publication in this journal is cited, in accordance with accepted academic practice. No use, distribution or reproduction is permitted which does not comply with these terms.
*Correspondence: Sage Raymond, cnJheW1vbjFAdWFsYmVydGEuY2E=