- 1Meise Botanic Garden, Meise, Belgium
- 2Service général de l'Enseignement supérieur et de la Recherche scientifique, Fédération Wallonie-Bruxelles, Brussels, Belgium
- 3CNRS, Univ. Lille, UMR 8198-Evo-Eco-Paleo, Lille, France
Assisted gene flow interventions such as plant translocations are valuable complementary techniques to habitat restoration. Bringing new genetic variants can contribute to increasing genetic diversity and evolutionary resilience, counteract inbreeding depression and improve plant fitness through heterosis. Large, highly genetically variable populations are usually recommended as sources for translocation. Unfortunately, many critically endangered species only occur as small populations, which are expected to show low genetic variation, high inbreeding level, paucity of compatible mates in self-incompatible species, and increased genetic divergence. Therefore, assessment of population genetic status is required for an appropriate choice of the source populations. In this paper, we exemplify the different analyses relevant for genetic evaluation of populations combining both molecular (plastid and nuclear) markers and fitness-related quantitative traits. We assessed the genetic status of the adult generation and their seed progeny (the potential translocation founders) of small populations of Campanula glomerata (Campanulaceae), a self-incompatible insect-pollinated herbaceous species critically endangered in Belgium. Only a few small populations remain, so that the species has been part of a restoration project of calcareous grasslands implementing plant translocations. In particular, we estimated genetic diversity, inbreeding levels, genetic structure in adults and their seed progeny, recent bottlenecks, clonal extent in adults, contemporary gene flow, effective population size (Ne), and parentage, sibship and seed progeny fitness variation. Small populations of C. glomerata presented high genetic diversity, and extensive contemporary pollen flow within populations, with multiple parentage among seed progenies, and so could be good seed source candidates for translocations. As populations are differentiated from each other, mixing the sources will not only optimize the number of variants and of compatible mates in translocated populations, but also representativeness of species regional genetic diversity. Genetic diversity is no immediate threat to population persistence, but small Ne, restricted among-population gene flow, and evidence of processes leading to genetic erosion, inbreeding and inbreeding depression in the seed progeny require management measures to counteract these trends and stochastic vulnerability. Habitat restoration facilitating recruitment, flowering and pollination, reconnecting populations by biological corridors or stepping stones, and creating new populations through translocations in protected areas are particularly recommended.
Introduction
Assisted gene flow interventions such as plant translocations are valuable complementary techniques to habitat restoration based on ecological management practices (Zimmer et al., 2019; Gargiulo et al., 2021). Plant translocations consist of intentionally introducing individuals (seeds, cuttings or plug plants) in genetically depauperate, inbred or extirpated populations that cannot recover through pollen flow or through recolonization by seeds from soil seed banks or from nearby populations (Menges, 2008; Weeks et al., 2011). Bringing new genetic variants can contribute to increasing genetic diversity and evolutionary resilience, counteract inbreeding depression and improve plant fitness through heterosis (Zavodna et al., 2015; Van Rossum et al., 2020; Van Rossum and Le Pajolec, 2021). However, translocation-based population restoration may fail for several reasons. Poor habitat conditions have been reported as a determinant factor in failure, resulting in high mortality of transplants and low recruit establishment (Godefroid et al., 2011; Schäfer et al., 2020; Volis and Blecher, 2021). Other studies found high inbreeding levels in transplants or in their offspring as a result of a low number of genotypes or reduced pollination service in source populations (Krauss et al., 2002; Monks et al., 2021; Van Rossum and Le Pajolec, 2021). If the introduced gene pool is too genetically differentiated from the natural pool or if the propagated transplants originate from several genetically differentiated sources, founders may be maladapted, and outbreeding depression may be found in the outcrossed progeny as a result of a breakdown of the co-adapted genes (Edmands, 2007; Bowles et al., 2015; Barmentlo et al., 2018). Therefore, carefully preparing the translocation is essential for optimizing the success in achieving genetically and demographically viable populations (Godefroid et al., 2016a; Commander et al., 2018) and optimizing population resilience to changing environmental conditions (Sgrò et al., 2011; Borrell et al., 2019; Pazzaglia et al., 2021). Several features have to be taken into account in designing the translocation. Species life-history traits, such as longevity, breeding system (e.g., self-incompatibility, dioecy, autogamy), pollen and seed dispersal abilities and clonal ability determine species sensitivity to habitat fragmentation and species response to restoration by influencing mating processes, gene flow and population genetic composition (Charlesworth, 2006; Dudash and Murren, 2008; Berjano et al., 2013; Wiberg et al., 2016; Bittebiere et al., 2020; Tierney et al., 2020; Garcia-Jacas et al., 2021). The selection of sources for translocation material (e.g., seeds, cuttings, tubers) has to be based on ecological characteristics, genetic composition and seed production and quality (Commander et al., 2018; Hoban et al., 2018; Bragg et al., 2020). Planting design, i.e., the number of planted founders, spatial arrangement and management of the sites before and after plant translocation may also affect demographic dynamics and mating processes (Betz et al., 2013; Albrecht and Long, 2019; Silcock et al., 2019; Van Rossum et al., 2020).
Large, highly genetically variable populations are usually recommended as source (one or several in mixture) for translocation (Basey et al., 2015; Hoban et al., 2018; Schäfer et al., 2020). Unfortunately, many critically endangered species only remain as small, spatially isolated populations. Those populations are expected to show low genetic variation, high inbreeding levels, and for self-incompatible or dioecious species, paucity of compatible mates (e.g., Aguilar et al., 2008; Angeloni et al., 2011; Berjano et al., 2013; Ottewell et al., 2016). As a result, seed production may be reduced and of poor quality (Vergeer et al., 2004; Wiberg et al., 2016; Aguilar et al., 2019; Tierney et al., 2020). Small populations may also consist in remnant, senescing populations, with old, possibly highly clonal, individuals still holding historical (pre-fragmentation) genetic variation (e.g., Van Geert et al., 2015; Gargiulo et al., 2019; Thomas et al., 2021; Van Rossum et al., 2021). However, in the current habitat fragmentation context, contemporary gene flow may be restricted due to spatial isolation and pollination disruption (e.g., Ghazoul, 2005; Aguilar et al., 2008). As a result, the seed progeny intended to be used as source for translocation may be genetically depauperate and inbred (Van Geert et al., 2008; Van Rossum, 2008; Thomas et al., 2021; Van Rossum and Le Pajolec, 2021). Small, isolated populations may also become genetically differentiated from each other, as a result of inbreeding and genetic drift (Ottewell et al., 2016; Wiberg et al., 2016), with the risk of outbreeding depression (Edmands, 2007; Barmentlo et al., 2018). Therefore, assessment of the genetic status of the populations is important for an appropriate selection of the source populations.
Criteria for selecting populations using genetic tools are detailed in many guidelines for plant translocations (e.g., Maschinski and Albrecht, 2017; Commander et al., 2018; Pazzaglia et al., 2021), and practical studies investigating the genetic status of populations for designing translocations are increasing (e.g., Berjano et al., 2013; Wiberg et al., 2016; Van Rossum and Raspé, 2018; St. Clair et al., 2020; Garcia-Jacas et al., 2021; Gargiulo et al., 2021; Thomas et al., 2021; Van Rossum et al., 2021), but studies combining the use of molecular DNA markers and fitness-related quantitative traits for assessing the genetic status still remain uncommon (Gentili et al., 2018; Borrell et al., 2019). Molecular markers allow for quantifying genetic diversity, inbreeding levels, parentage and sibship, pollen and seed dispersal and therefore gene flow, identifying mating processes, and possible pollination failure (e.g., Hardy et al., 2004; Carroll and Fox, 2008; Llaurens et al., 2008; Jones and Wang, 2010; Bragg et al., 2020; Van Rossum and Hardy, 2021). Effective population size (Ne), which represents a more accurate indicator for population viability than census population size, and bottlenecks that populations may have recently incurred, can also be inferred using molecular markers (Chybicki and Burczyk, 2009; Luikart et al., 2010; Wang, 2016). Fitness quantitative traits inform on negative consequences of habitat fragmentation and population size on reproductive success, on inbreeding or outbreeding depression, and on (mal)adaptation, especially when combined with molecular markers (Zavodna et al., 2015; Barmentlo et al., 2018; Borrell et al., 2019; Van Rossum and Le Pajolec, 2021). Identifying phenotypic plasticity and maternal effects may also give insights into adaptive differences and non-neutral genetic variability accounting for population resilience (Nicotra et al., 2015; Hamilton et al., 2017; Van Rossum et al., 2020). Besides, whether the potential source and target populations share common phylogeographic history (based on plastid markers) has been insufficiently examined (Van Rossum and Raspé, 2018; Vera et al., 2020; Bobo-Pinilla et al., 2021; Jacquemart et al., 2021), despite the risk of reproductive isolation and outbreeding depression in hybrids between genetic lineages that show diverging phylogeographic patterns (Edmands, 2007; Martin et al., 2017).
In this paper, we used plastid and nuclear molecular markers together with fitness-related quantitative traits to exemplify the different analyses that can be relevant to evaluate the genetic status of populations, stressing the results potentially leading to translocation failure. More precisely, we investigated the following factors: (1) genetic diversity, inbreeding levels and detection of recent bottlenecks in the adult generation of potential source or target populations and in the seed progeny that are intended for founding the translocated populations; (2) clonal extent in the adult generation; (3) genetic differentiation among populations; (4) contemporary gene flow, parentage, sibship and contemporary Ne; (5) and maternal effects, phenotypic plasticity and inbreeding depression in fitness-related quantitative traits. The study was carried out on Campanula glomerata L. (Campanulaceae), an insect-pollinated herbaceous species that is critically endangered in Belgium, only consisting of small populations, and that has been involved in a restoration project of calcareous grasslands which has implemented plant translocations (Godefroid et al., 2016a,b). We discuss the implications of our findings for species regional conservation and designing plant translocation protocol using small populations as seed sources.
Materials and Methods
Study Species
Campanula glomerata L. (Campanulaceae) is a long-lived perennial, herbaceous species occurring on calcareous grasslands, scrub, open woodlands and sand dunes. It has a wide continental Eurasian distribution range, reaching its margin in northwestern Europe where the species becomes rare and where populations are scattered and disjunct (Hultén and Fries, 1986). The species is declining in Western Europe, as a result of the fragmentation and degradation of its habitats, and populations are often small and isolated (Green et al., 1997; Hill et al., 2004; Bachmann and Hensen, 2007; Godefroid et al., 2016a). In Belgium, the species is critically endangered (Saintenoy-Simon et al., 2006) and a species recovery plan has been designed, involving plant translocations in the framework of a Life project in Belgian Lorraine (southern Belgium). After an exhaustive field survey, only small populations of C. glomerata were found in Belgian Lorraine, but also in the surrounding regions in France and Luxembourg (Godefroid, pers. commun.). It was therefore decided to use several small populations as seed sources and to mix them (Godefroid et al., 2016a).
The plants flower from June to August. The flowering stems are up to 70 cm high and bear on average 20–30 (up to 190) violet-blue protandrous flowers arranged in clusters, which produce nectar (Denisow and Wrzesień, 2015; Strzałkowska-Abramek et al., 2018; Godefroid and Van Rossum, unpublished data). The species is characterized by a self-incompatible breeding system (Gadella, 1964). The main pollinators are bumble bees, solitary bees, honey bees and hoverflies (Albrecht et al., 2007; Denisow and Wrzesień, 2015). The numerous tiny seeds are dispersed over short distances by the shaking of the capsules, and do not form a persistent seed bank in the soil. Plants can live for 25–30 years, and subsist through vegetative spread by rhizomes (Klotz et al., 2002; Hill et al., 2004; Bachmann and Hensen, 2007). Clonal spread was estimated at 0.01–0.25 m/year (Klimešová and Klimeš, 2013).
Study Populations and Sampling
Leaves were collected in 2013 from 178 adult individuals in the last six populations of C. glomerata from Belgian Lorraine in southern Belgium, and in 2014 from two populations from France located at 57 and 106 km from the Belgian populations, respectively (Figure 1; Supplementary Table S1). Sampling was made on flowering plants. A plant with flowering stalks starting from a single point was considered as one individual and sampled. The number of flowering individuals was counted the same year as leaf sampling. The geographic distance between Belgian populations was 0.68–3.57 km. Seeds were collected as a single cohort collection in 2012 (CHA, FON), 2013 (EMO, LAM, WAT) or 2014 (MAR, VER) according to ENSCONET. (2009) protocol (no seeds could be collected from population CEC) and germinated in 2015 to obtain 2000 seed progeny (218–352 transplants per source population) for plant translocations (for details, see Godefroid et al., 2016a). From these, 31–101 seed progeny individuals per population were sampled for leaves, and rosette diameter was measured 2 months after germination. A total of 680 samples were dried in silica gel.
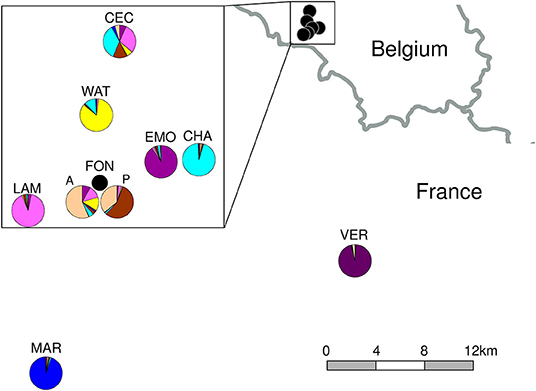
Figure 1. Location of eight studied populations of Campanula glomerata in Belgian Lorraine and northern France. For population details, see Supplementary Table S1. The pie charts correspond to mean membership values at K = 8 clusters for the adult generation (except for FON: A, adults and P, seed progeny) of the structure analysis (Figure 2).
DNA Analyses
DNA Extraction
DNA was extracted from ca. 10–15 mg of dried leaf material using a CTAB method (Doyle and Doyle, 1990) for plastid analyses and with the NucleoMag 96 Plant kit (Macherey Nagel, Duren, Germany) according to the manufacturer's protocol for microsatellite analyses. We estimated the concentration of genomic DNA in the extracts using the Qubit Quantitation Platform (Invitrogen). DNA concentration was standardized to 2 ng/μl.
Plastid DNA Sequencing
Three plastid loci usually showing high polymorphism and commonly used for DNA barcoding (e.g., Hollingsworth et al., 2011; Xia et al., 2019) were tested for amplification and sequencing. The plastid marker rps16 (with rpsF and rpsR2; Oxelman et al., 1997) did not amplify. The two other loci were amplified and sequenced from five individuals per population (excluding FON population) for a total of 35 individuals, with the following with psbA3'f (Sang et al., 1997) and trnHf (Tate and Simpson, 2003), and matK with matK-xF (Ford et al., 2009) and matK-MALPR1 (Dunning and Savolainen, 2010). We followed standard PCR procedures (e.g., Shaw et al., 2005). PCR products were purified by adding 1 U of Exonuclease I and 0.5 U FastAP Alkaline Phosphatase (Thermo Scientific, Germany) and incubated at 37°C for 1 h, followed by inactivation at 80°C for 15 min. Sequencing was performed in both directions by Macrogen Europe (The Netherlands) with PCR primers. Chromatograms were edited and assembled in Geneious (Biomatters). Contig sequences are available from GenBank under accession numbers OL943797 (matK) and OL943798 (trnH-psbA).
Nuclear Microsatellite Analysis
We used 15 polymorphic microsatellite loci (excluding CAM19) previously developed by Van Rossum and Godé (2021), but with the protocol adapted as follows: PCR amplifications were performed in 10 μL reactions including 1X multiplex PCR master Mix (QIAGEN, Germany), 3μl (5–20ng) of genomic DNA, and 2.0–6.4 pmol labeled forward and reverse primers (see Table 2 in Van Rossum and Godé, 2021). The PCR cycling consisted of an initial denaturation at 95°C for 15 min, followed by 35 cycles: denaturation at 95°C for 30 s, annealing at 55°C for 45 s and extension at 72°C for 1 min, and a final extension at 60°C for 30 min. Multiplexes were electrophoresed on an ABI PRISM 3130 sequencer (Applied Biosystems), with 1.5 μl of PCR product diluted with dH2O (1:10), and 9.75 μl of Hi-Di Formamide (Life Technologies, USA) and 0.25 μl of MapMarker 500 labeled with DY-632 (Eurogentec). Alleles were scored with software GeneMapper version 5 (Applied Biosystems) and Geneious Prime (Biomatters).
Data Analyses and Rationale
The research questions to investigate and the possible problems that might lead to translocation failure discussed here below are summarized in Table 1. For suggestion of other potential data analyses and related software, see e.g., Van Rossum and Hardy (2021), and Bourgeois and Warren (2021) for genomic approaches.
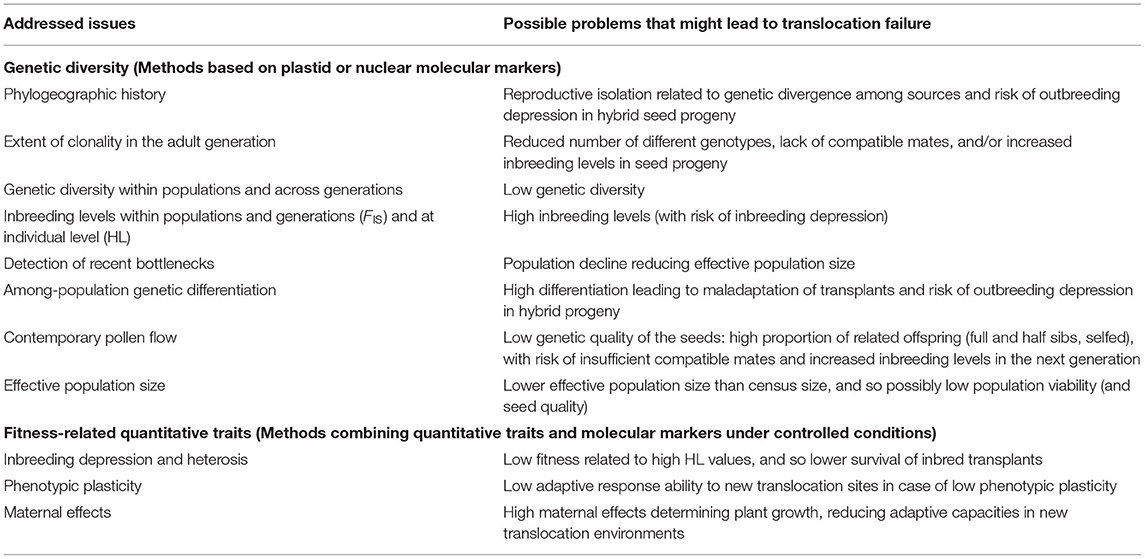
Table 1. Summary of the research questions to investigate and the possible problems that might lead to translocation failure.
Phylogeographic History
Differences in plastid marker sequences among populations may reveal different phylogeographic patterns related to ancient processes, such as different (post)glacial migration histories, and the existence of contact zones where distinct genetic lineages have met (Hewitt, 2004; Hickerson et al., 2010; Van Rossum et al., 2018). The related genetic divergence among, sometimes co-occurring, genetic lineages may induce reproductive isolation and outbreeding depression in hybrid progeny (Edmands, 2007; Martin et al., 2017), requiring to take phylogeographic patterns into account for source sampling (Van Rossum et al., 2018; Vera et al., 2020; Bobo-Pinilla et al., 2021). The other research questions relating to genetic diversity (as described in Table 1) were based on the analysis of nuclear microsatellite markers and are described below.
Extent of Clonal Propagation
Populations characterized by high clonal propagation in the adults sampled for translocation material (cuttings, seeds) may show a reduced number of genotypes and of compatible mates compared to what could be expected given census population size, and high inbreeding levels in the seed progeny intended to be used for translocation (Van Rossum and Raspé, 2018; Bittebiere et al., 2020; Van Rossum and Le Pajolec, 2021; Van Rossum et al., 2021).
In order to estimate clonal propagation in the adult generation, we identified multilocus genotypes, assigned each sampled adult to a multilocus genotype and calculated the probability (pse) of finding the same multilocus genotype a second time in each population using GenAlEx 6.5 (Peakall and Smouse, 2012). Identical multilocus genotypes were likely to be putative clones when pse < 0.05, and to result from sexual reproduction when pse > 0.05 (Parks and Werth, 1993). The locus CAM27 showing a lot of missing data due to a high null allele frequency (Van Rossum and Godé, 2021) and 21 individuals with missing data in the other loci were excluded from the analyses. For population showing putative clones, we calculated the proportion of distinct multilocus genotypes as G/N (Ellstrand and Roose, 1987); G is the number of multilocus genotypes and N the number of sampled adults.
Genetic Variation Within Populations
Source material for translocation should show high genetic diversity to ensure evolutionary resilience, also to cope with changing environmental conditions, and low inbreeding levels to avoid inbreeding depression issues (e.g., Carroll and Fox, 2008; Sgrò et al., 2011; Prati et al., 2016; Commander et al., 2018; Hoban et al., 2018). In particular, attention should be paid when small populations are used as sources as they might be inbred and genetically depauperate, in the adult and/or the seed progeny generations (Vergeer et al., 2004; Van Geert et al., 2008; Wiberg et al., 2016; Tierney et al., 2020; Gargiulo et al., 2021).
The following estimates of genetic variation were calculated for each population for the adults and for the seed progeny using GEN-SURVEY (Vekemans and Lefèbvre, 1997) or FSTAT (Goudet, 2003): allelic richness (A[N]) for a fixed sample size (N = 5, excluding adult data of FON), observed (Ho) and expected (He) heterozygosity and Wright's inbreeding coefficient (FIS), corrected for small sample size. We tested the significance of the FIS values (over all loci) by randomization tests and sequential Bonferroni-type correction. As null alleles were detected at some loci (Van Rossum and Godé, 2021), mean inbreeding coefficient values (FISnull) and their 95% highest posterior density intervals (HPDI) were calculated after adjusting allele frequencies with INEST (Chybicki and Burczyk, 2009) to take a possible effect of null alleles into account on FIS values. We applied the Bayesian approach (IIM) with 106 Markov Chain Monte Carlo iterations, of which the first 105 were discarded as burnin phase, testing two models: a full model (nfb, including null alleles, inbreeding and genotyping failures), and a model (nb) where inbreeding was set at 0. The best fitting model was indicated by the lowest value of the deviation information criterion (DIC). Differences in A[5], Ho, He, and FIS values between adult and seed progeny generations were tested by performing pairwise Wilcoxon matched pairs tests by locus. Relationships between within-population genetic estimates (G/N, A[5], Ho, He, FIS and FISnull) and flowering population size were examined for each generation by Gamma correlation analyses. Analyses were performed using STATISTICA version 12 (Dell Inc.).
To detect recent genetic bottlenecks in each population, we performed tests for the excess in heterozygosity on each generation (excluding adult sampling with n <20) using INEST, under the Two-Phase model and with the default settings recommended by Peery et al. (2012). P-values of the Wilcoxon signed-rank tests were determined based on 106 permutations.
Genetic Structure Among Populations
Investigating how populations genetically diverged from each other might indicate the occurrence of several processes, such as genetic drift effects and disruption of gene flow (Aguilar et al., 2008; Ottewell et al., 2016). Too high among-population genetic differentiation might lead to transplant maladaptation and outbreeding depression in hybrid progeny (Edmands, 2007; Bowles et al., 2015). Comparing adult and seed progeny generations allows for identifying contemporary processes, especially pollen dispersal patterns (Hardy et al., 2004; Ritchie and Krauss, 2012; Van Rossum et al., 2020; Van Rossum and Le Pajolec, 2021).
We conducted several complementary analyses to describe the genetic structure of the populations. First, pairwise FST values were calculated between populations and generations according to Weir and Cockerham (1984), excluding FON because of a too small sampling size (n =3), and their significance was tested by randomization tests using FSTAT and Bonferroni correction. Differences in FST values between adult and seed progeny generations were tested by performing pairwise Wilcoxon matched pairs tests on the same 15 population pairs. Pairwise FST values were also calculated with the ENA correction using FreeNa (Chapuis and Estoup, 2007) so that the presence of null alleles could be taken into account. Bootstrap resampling over loci using 10,000 replicates were computed using FreeNa to obtain 95% confidence intervals.
Second, Cavalli-Sforza and Edwards' (1967) genetic distances were computed between all pairs of populations and generations, with the INA correction (taking possible null alleles into account), with bootstrapping over loci, using FreeNa. The patterns of between-population differentiation were summarized by performing a neighbor-joining (NJ) cluster analysis on the matrix of Cavalli-Sforza and Edwards' distances using the software Populations 1.2.3.1 (Olivier Langella, Montpellier). The cluster analysis was visualized using FigTree v.1.4.3 (Rambaut, 2016).
Finally, we inferred population structure using STRUCTURE version 2.3.4 (Pritchard et al., 2000) performed with Structure_threader (Pina-Martins et al., 2017). This Bayesian clustering method identifies the number of clusters (K) of distinct gene pools that differ by a set of allele frequencies at each locus. We performed analyses for K = 1 to K = 15 clusters (30 different runs), using an admixture ancestry model with correlated allele frequencies, run length of burn-in period of 106 iterations, and 2 × 106 Markov Chain Monte Carlo replications. Null alleles were treated as recessive and genotypes with missing data were considered as homozygous for null alleles (Falush et al., 2007). The most likely number of K clusters was inferred based on the ad hoc statistic DeltaK and the highest likelihood value (Evanno et al., 2005), after running STRUCTURE HARVESTER (Earl and vonHoldt, 2012), but results for each K cluster were also checked. The most likely estimated membership (Q) values of the 10 best (with the highest likelihood values) independent runs were obtained with CLUMPP version 1.1.2 (Jakobsson and Rosenberg, 2007) and visualized on barplots using DISTRUCT version 1.1. (Rosenberg, 2004).
Contemporary Pollen Flow and Effective Population Size
Genetic quality of the seeds collected for transplant propagation depends on whether there was effective contemporary pollen dispersal among individuals and populations (Hardy et al., 2004; Vergeer et al., 2004; Menges, 2008; Ritchie and Krauss, 2012; Aguilar et al., 2019). When most seed progeny are genetically related and belong to a few large families, consisting of full sibs (sharing both parents) and half sibs (sharing one of the two parents), or of selfed offspring (in case of self-compatible species), the number of compatible mates can be reduced and high levels of inbreeding may be expected in the next generation (Aguilar et al., 2019; Thomas et al., 2021). Therefore, it is important to verify that parentage contributions were spread over a maximum of parents, especially in case of small populations. Contemporary Ne is a better indicator of population health than census population size, as Ne takes several demographic factors, such as sex ratio, mate compatibility, inbreeding and family size, into account (Carroll and Fox, 2008; Luikart et al., 2010). Recent bottlenecks are interesting to detect, as they may indicate population declines through stochastic demographic or environmental events (partial destruction of the populations, harsh climatic season) and reduce Ne (Peery et al., 2012). However, sibship, Ne and bottlenecks have been still overlooked in genetic assessment for plant translocations (Krauss et al., 2002; Ritchie and Krauss, 2012; Bragg et al., 2020; Gargiulo et al., 2021; Thomas et al., 2021).
Sibship, parentage and contemporary Ne were inferred on seed progeny for each population (excluding CEC), using COLONY version 2.0.6.6 (Wang, 2009; Jones and Wang, 2010). The adult generation was included as candidate (maternal and paternal) parent genotypes (excluding putative clones) in the analyses. The analyses were performed using a full likelihood approach with high likelihood precision, no prior sibship size, medium length of run, and probability of an actual father or mother being included in candidates guessed at 0.8 (or 0.5 for FON and VER), and taking estimations of allelic dropout rates (null allele frequencies) and genotypic error rates into account (Wang, 2018, 2019). The other parameters were set as by default or at 0. For Ne, estimated from sibship frequency of a single cohort (in our case seed progeny generation), 95% confidence intervals were obtained from bootstrapping (Wang, 2016). Note that the sibship assignment method in species with overlapping generations gives an estimate of the effective number of breeders when applied to a single cohort, taking non-random mating (and selfing for self-compatible species) into account (Wang et al., 2010; Wang, 2016). This is particularly useful for detecting population trends when planning translocation experiments in long-lived species. To search for possible contemporary pollen flow between Belgian populations, the analyses were also performed with all sampled adults of the six Belgian populations as candidate parent genotypes (n = 88). The best configuration (with the maximum likelihood) of the seed progeny parentage was visualized as a pedigree graph using Pedigree Viewer version 6.5f (Kinghorn and Kinghorn, 2015).
Data Analyses Based on Fitness-Related Quantitative Traits
Fitness-related quantitative traits grown under controlled conditions can vary according to genetic factors, such as individual inbreeding level (lower fitness expected for homozygous genotypes) and heterosis (higher fitness of the heterozygotes), which may affect transplant survival and reproductive success (Zavodna et al., 2015; Barmentlo et al., 2018; Van Rossum and Le Pajolec, 2021). Differences in fitness among populations or maternal plants can also indicate local processes (genetic drift, selection) leading to genetic variability and potentially differing adaptive capacities (Willi et al., 2007; Nicotra et al., 2015; Hamilton et al., 2017). Phenotypic differences may be plastic and disappear after time, suggesting that plants may adapt to changing environments and translocation sites (Gentili et al., 2018; Van Rossum et al., 2020; Van Rossum and Le Pajolec, 2021). Strong maternal effects may favor plant growth and survival in the original environmental conditions, but might impair them if environmental conditions differ, e.g., in translocation sites (Schuler and Orrock, 2012).
We performed a one-way Analysis of Covariance (ANCOVA) together with pairwise Tukey HSD post hoc tests for testing for differences in seed progeny rosette diameter in relation to population and Homozygosity by locus (HL, as an indicator of inbreeding) as a covariate. This estimate, which varies from 0 (all loci are heterozygous) to 1 (all loci are homozygous; Aparicio et al., 2006), was calculated for each individual, using the software GENHET (Coulon, 2010). CAM27, because of a high frequency of null alleles in all populations (ranging 18–58%), which might falsely inflate HL, was excluded from the analyses. Differences in seed progeny HL between populations was tested by computing a one-way ANOVA together with pairwise Tukey tests. The 18 possibly wrongly labeled MAR seed progeny (see results of the structure analysis below) were excluded from the AN(C)OVAs. We performed a Pearson's correlation analysis between rosette diameter and HL. HL was Box-Cox-transformed to achieve normality and homoscedasticity. The tests were conducted with STATISTICA.
Results
Plastid DNA Sequencing
The sequences of trnH-psbA and matK were 393 and 908 bp long, respectively. No polymorphism was observed in either matK or trnH-psbA, so that concatenating the sequences and building a phylogenetic tree was not relevant.
Loci and Scored Alleles
We found 13 to 39 alleles per locus for a total of 376 alleles, of which 43 were private (1 found in CEC, CHA and FON populations, 2 in EMO, 4 in LAM, 8 in VER and 26 in MAR; there was no private allele in WAT). All loci were polymorphic in all populations and in adult and seed progeny generations.
Extent of Clonal Propagation
Out of 659 sampled individuals, 643 were associated with a single multilocus genotype. The 487 seed progeny individuals showed distinct multilocus genotypes. Seven multilocus genotypes (1–3 per population) were assigned to two or three adult samples in CHA, LAM, MAR or VER, which can be considered as putative clones (pse < 0.05). For CHA, LAM, MAR and VER populations, G/N of the adult generation was 0.96, 0.88, 0.97, and 0.87, respectively, and 1.00 for the other populations. Therefore, clonal spread could be considered as marginal in our studied populations.
Genetic Variation Within Populations and Across Generations
In most populations estimates of genetic variation (A[5], Ho, He) were high. A lower allelic richness A[5] and/or genetic diversity (Ho, He) in the seed progeny than in the adults was found in FON, WAT, LAM, MAR (excluding possibly wrongly labeled individuals) and VER (Table 2; Supplementary Table S2). Significant deviation from Hardy-Weinberg expectations was found for adult or seed progeny in five populations, with positive FIS values (Table 2). The IIM analyses indicated that the presence of null alleles contributed to the significantly positive FIS values, with decreased FISnull values, ranging from 0.016 to 0.073 instead of 0.039 to 0.159 for FIS (Table 2). However, inbreeding was detected for the adult generation in MAR and in the seed progeny for CHA, LAM and VER (lowest DIC for the nfb model in the IIM analysis). There was a significant correlation between flowering population size and adult FIS (Gamma correlation Γ = 0.615, P = 0.040), but the correlation was not significant anymore with adult FISnull (Γ = −0.077, P = 0.797). The correlations between flowering populations size and the other genetic estimates (G/N, A[5], Ho, and He) in both generations and seed progeny FIS and FISnull were not significant (Γ ranging from −0.619 to 0.474, P ≥ 0.060).
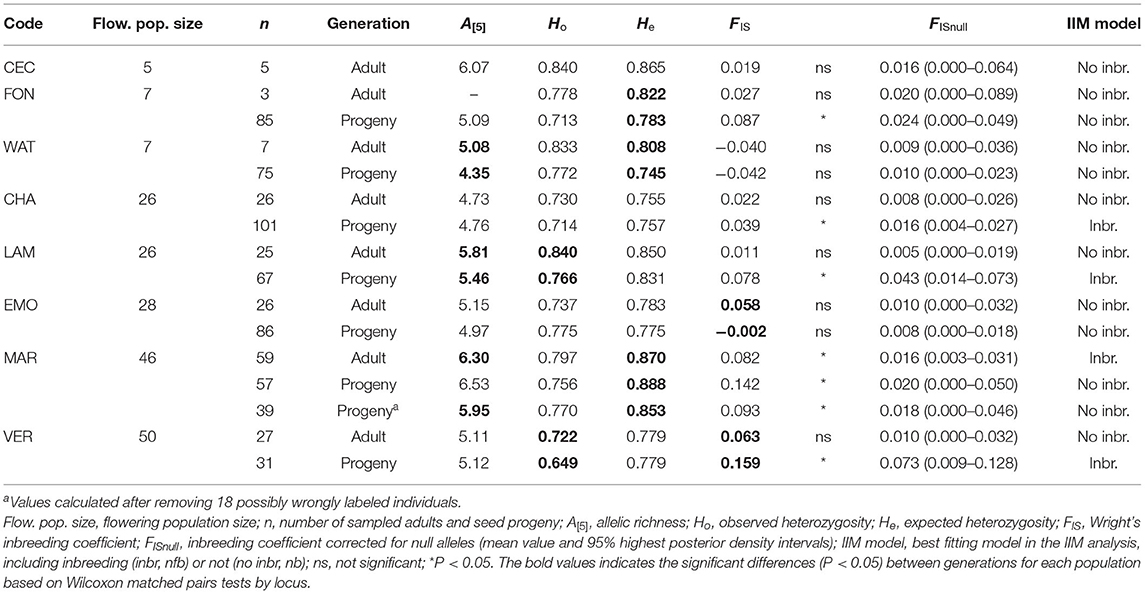
Table 2. Estimates of within-population genetic variation for eight populations of Campanula glomerata at 15 nuclear microsatellite loci.
Recent genetic bottlenecks were detected in LAM population for both adult and seed progeny generations (Wilcoxon signed-rank tests Z = 2.44 and 2.10, P = 0.006 and 0.018, respectively) and for WAT seed progeny (Z = 2.78, P = 0.002). No evidence of recent bottlenecks was found for the other populations (Z ranging from −1.42 to 0.28, P ≥ 0.402).
Genetic Structure Among Populations and Between Generations
Genetic differentiation between adults and their seed progeny (within populations) was absent or low, with FST values varying from 0.000 to 0.012 and mostly not significant (P > 0.05). By contrast, genetic differentiation between populations was moderate to high and significant (P > 0.05 after Bonferroni correction) for most population pairs (Supplementary Table S3). FST values were higher for seed progeny than for adults (FST values between population pairs ranging 0.035–0.154 and 0.082–0.159, respectively; Wilcoxon matched pairs test Z = 3.01, P = 0.003). Values were similar when applying the ENA correction (Supplementary Table S3).
The tree based on Cavalli-Sforza and Edwards' genetic distances with the INA correction for null alleles and the STRUCTURE analysis (best modal clusters at K = 2 and 8, Supplementary Figure S1) showed similar patterns of among-population genetic differentiation, with two main clusters separating the two French populations (MAR and VER) from the Belgian populations and adult and seed progeny generations of the same population clustering together (Figure 2). At K = 8, the Bayesian clustering allowed to distinguish the populations based on high membership Q values (> 80%), except CEC whose individuals were assigned to several clusters. In FON progeny, individuals were assigned to two different clusters (dark and light brown), some individuals being admixed between the two clusters. One cluster (dark brown) was not found in the sampled adults, which suggested that it might correspond to not genotyped adults. In MAR (France), 18 seed progeny individuals appeared to belong to another cluster (light blue) typical of CHA from Belgium, with very high Q values (≥ 87%), so with no indication of admixture, and not found in the adult generation. After verification, and as these 18 individuals belonged to one same range of numbering, a possible explanation was wrong labeling during seedling pricking or juvenile repotting. For this reason, the other data analyses were performed again excluding these 18 seed progeny individuals. The barplots obtained for the other K clusters did not bring additional information (results not shown).
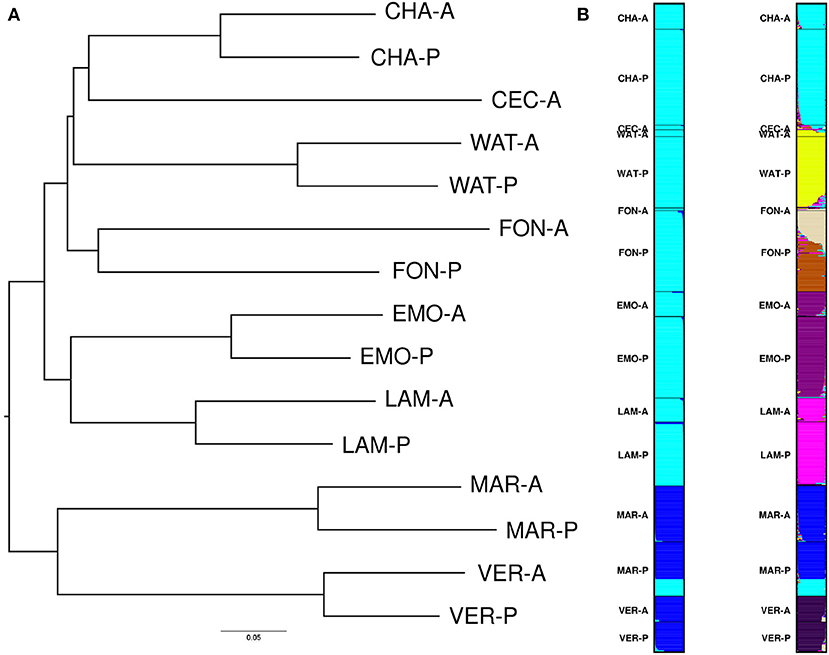
Figure 2. (A) Neighbor-joining tree based on Cavalli-Sforza and Edwards' genetic distances with the INA correction for null alleles and (B) results of Bayesian clustering (modal K = 2 and 8) for two generations (A, adults and P, seed progeny) in eight populations of Campanula glomerata. In the barplot, each individual is represented by a horizontal bar, showing the membership (Q) to each of the 2 or 8 modal clusters.
Contemporary Pollen Flow and Effective Population Size
Sibship and parentage assignments using COLONY showed low proportions of full sibs and half sibs and usually small full sib family sizes, so that most seed progeny individuals were not closely related in most populations, except in WAT (Table 3; Supplementary Figure S2). The number of offspring per inferred (maternal or paternal) parent genotype was small, except for a few parents and in WAT (Table 3; Supplementary Figure S2). These results indicated representative seed sampling of the maternal plants and high multiple paternity. Ne estimated from sibship assignments ranged from 6 to 40, and was similar (EMO, LAM, WAT) or higher (CHA, FON) than flowering population size (Table 2) in most populations, but lower for MAR and VER (Table 3). The number of seed progeny whose at least one maternal or paternal parent was assigned from sampled adult genotypes was usually low, except for VER and WAT where candidate parents were identified as sampled adult genotypes for more than two third of the seed progeny (Table 3). Only one offspring (from EMO) had an assigned parent from sampled adults of another population (CHA).
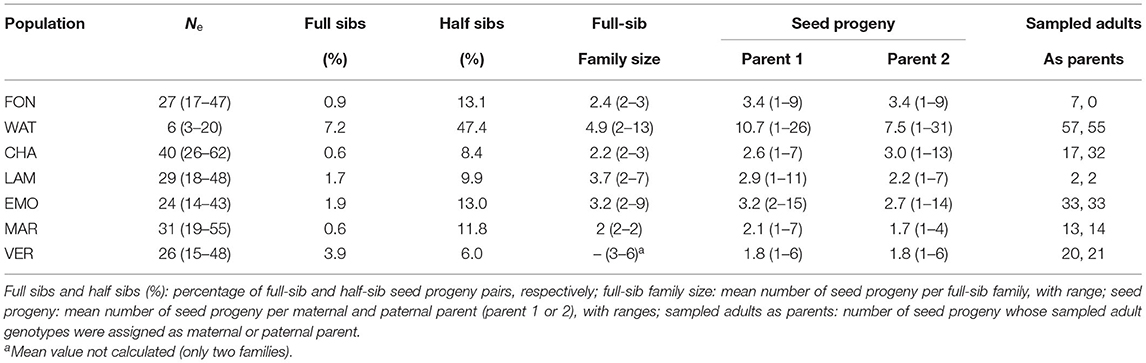
Table 3. Effective population size (Ne, with 95% confidence intervals), sibship and parentage for seven populations of Campanula glomerata.
Fitness of the Seed Progeny
The one-way ANCOVA [R2 = 0.228, F(7, 476) = 20.09, P < 0.001] revealed a significant population effect [F(6, 476) = 20.29, P < 0.001] on plant size (after 2 months of growth), with VER-MAR and LAM showing the lowest and highest rosette diameter values, respectively (pairwise Tukey HSD post hoc tests, P < 0.001, Figure 3A). Rosette diameter was negatively correlated with HL [β estimate = −0.150, F(1, 476) = 12.87, P < 0.001; univariate Pearson's correlation coefficient r = −0.175, P < 0.001, Supplementary Figure S3] and with flowering population size (using mean values per population, Γ = −0.789, P = 0.018). Populations of similar flowering population sizes (e.g., CHA and LAM) significantly differed from each other (Tukey tests, P < 0.001; Figure 3A). There were significant differences between populations in HL (R2 = 0.072, F(6, 477) = 6.16, P < 0.001), with VER showing higher HL values than WAT, LAM, EMO and MAR (Tukey tests, P ≤ 0.016; Figure 3B), but there was no significant correlation between HL and flowering population size (Γ = 0.158, P = 0.636).
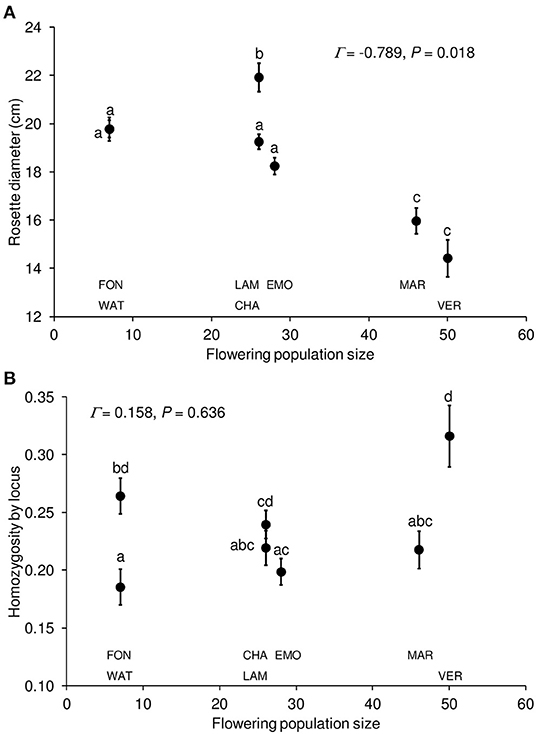
Figure 3. Rosette diameter (A) and homozygosity by locus (B) (mean value ± SE) in function of flowering population size for seven populations (CHA, EMO, FON, LAM, MAR, VER and WAT) of Campanula glomerata. Different letters indicate significant (P < 0.05) differences based on Tukey tests between populations. Γ = Gamma correlation coefficient.
Discussion
No Evidence of Distinct Genetic Lineages Related to Past Events
The lack of polymorphism in the sequenced plastid DNA markers suggests no strong genetic divergence among populations related to past phylogeographic processes. Consequently, the existence of divergent, reproductively isolated genetic lineages amongst the studied populations of C. glomerata is unlikely. A similar pattern was also reported for Arnica montana (Van Rossum and Raspé, 2018) and in the bird seed-dispersed Juniperus communis (Jacquemart et al., 2021), both being critically endangered in southern Belgium. By contrast, distinct genetic lineages were found for A. montana (Vera et al., 2020) and for Jacobaea auricula (Bobo-Pinilla et al., 2021) in Spain, and reproductively isolated genetic lineages were found for Silene nutans in southern Belgium (Martin et al., 2017).
Genetic Status of the Remaining Populations
Unlike Arnica montana, Dianthus deltoides and Linnaea borealis, whose small populations were found to be highly clonal and showed low genetic variation (Wiberg et al., 2016; Van Rossum and Raspé, 2018; Van Rossum et al., 2021), clonal propagation in populations of C. glomerata was only marginal, so that the number of adult genets was similar to the number of ramets. Moreover, despite the small size of the populations, genetic diversity within populations (He = 0.76–0.87) was high compared to other self-incompatible insect-pollinated declining plant species occurring in similarly fragmented habitats, e.g., A. montana (He = 0.32–0.60; Van Rossum and Raspé, 2018), Primula vulgaris (He = 0.33–0.83; Van Geert et al., 2015), and Pulmonaria officinalis (He = 0.35–0.71; Meeus et al., 2012). Given the high longevity (25–30 years) of the plants, which may also subsist as non-sprouting rhizomes in the soil (Bachmann and Hensen, 2007), we may assume that the adults consist of old genets still retaining pre-disturbance genetic diversity (Van Geert et al., 2008; Thomas et al., 2021). Besides, most populations showed no inbreeding in the adult generation, as expected for species characterized by a self-incompatible breeding system (Charlesworth, 2006; Dudash and Murren, 2008). Five populations showed a slight loss of allelic richness or genetic diversity in the seed progeny generation compared to the adults, and three populations showed low but significant inbreeding levels in seed progeny, suggesting that the processes (genetic drift effects, crosses between relatives leading to biparental inbreeding) usually observed in fragmented populations (e.g., Aguilar et al., 2008, 2019; Berjano et al., 2013; Wiberg et al., 2016) may have started. There was also evidence of recent genetic bottlenecks in LAM and WAT populations, suggesting that these populations may have incurred demographic reductions (Peery et al., 2012), as found for the self-incompatible Sesili farrenyi (Garcia-Jacas et al., 2021). No effect of flowering population size was observed on the genetic variation estimates, but the size range may be too narrow to detect any relationship.
Extensive Contemporary Pollen Flow Within Populations but Limited Among Populations
Adults and seed progeny generations were genetically similar (low FST values) in all studied populations of C. glomerata. The small size of full-sib and half-sib families for most parents indicated high multiple paternity, and contemporary Ne estimates were similar or even higher than flowering population sizes for most populations. These findings suggest extensive contemporary pollen flow within populations. Flowers of C. glomerata represent an important resource in nectar and pollen for many insects and so attract a variety of pollinators, such as bumble bees, solitary bees, honey bees and hoverflies (Albrecht et al., 2007; Denisow and Wrzesień, 2015; Strzałkowska-Abramek et al., 2018), which may favor extensive pollen flow across the whole population (Ghazoul, 2005; Van Rossum, 2010; DiLeo et al., 2018). High multiple paternity was also found in an isolated, but large population of the self-incompatible Arabidopsis halleri, with only 4% of offspring sharing the same father (Llaurens et al., 2008), while a value of 20% was found in a population of 96 flowering plants of the self-incompatible Centaurea corymbosa (Hardy et al., 2004).
By contrast, the high genetic differentiation among populations (for both adult and seed progeny generations) indicated by the moderate to high FST values, and the lack of admixture indicated by genetic structure and parentage analyses based on nuclear microsatellite markers suggest that contemporary gene flow may be limited or marginal among the Belgian populations, despite the short geographic distances separating them (<3.6 km). Although seed dispersal efficiency is known to be low, barely over a few meters (Bachmann and Hensen, 2007), such pattern is surprising concerning pollen dispersal, given flight and pollen dispersal abilities reported over such distances for some pollinators of C. glomerata (Ghazoul, 2005; Van Rossum, 2010; Zurbuchen et al., 2010). It suggests the existence of dispersal barriers between populations or that small flowering size of the populations reduced the probability for pollinators to encounter the neighboring populations, so that maintenance of constancy in pollinator foraging behavior (especially for bumble bees and honey bees) can be compromised (Ghazoul, 2005; Van Rossum and Triest, 2010, 2012; DiLeo et al., 2018).
However, a low number of sampled adults could be assigned as parents of the seed progeny in most populations, suggesting that the parentage analyses might lack accuracy. Several factors may have limited the accuracy of a parentage analysis: the 15 microsatellite markers might not have been sufficiently informative (Jones and Wang, 2010); the sampling of adults might not have been exhaustive enough (Hardy et al., 2004); sampling of the seed cohort in 2012 and of adults in 2013 for two populations might have led to discrepancies between generations, related to fluctuating population sizes, disturbance (mowing, bottlenecks) or weather conditions affecting pollen production (Bachmann and Hensen, 2006, 2007; Denisow and Wrzesień, 2015).
Fitness of the Seed Progeny
Reduced plant fitness is usually expected in small populations due to genetic erosion, inbreeding and deteriorated habitat conditions, especially in the seed progeny (e.g., Vergeer et al., 2004; Zavodna et al., 2015; Aguilar et al., 2019). In the studied populations of C. glomerata, inbred progeny plants (with high HL values) were indeed smaller, despite good cultivation conditions, suggesting some inbreeding depression (related to biparental inbreeding) at individual level (Willi et al., 2005; Barmentlo et al., 2018). Surprisingly, plants in small populations tended to be larger, but populations also differed in plant size apart from population size, suggesting maternal effects at early growth stages (Roach and Wulff, 1987) and high genetic adaptive variability (Basey et al., 2015; Hamilton et al., 2017). Moreover, the degraded nutrient-poor calcareous grasslands of Western Europe are usually reforested and eutrophicated (e.g., Betz et al., 2013; Hautekèete et al., 2015), leading to increased edge effects and competition in closing vegetation (Jacquemyn et al., 2003; Oostermeijer et al., 2003; Maurice et al., 2012). Plant height of C. glomerata was reported to increase under competition in dense cover vegetation (Bachmann et al., 2005), and the sampled populations also differed in local ecological conditions, e.g., they occurred on sandy or marly soils (Godefroid et al., 2016a). Therefore, the observed differences in plant size and maternal effects on progeny at early growth stages may reflect local adaptation of old, resilient genotypes (Oostermeijer et al., 2003; Willi et al., 2007), which might lead to maladaptation if translocation is implemented in restored nutrient-poor grasslands (Reckinger et al., 2010; Schuler and Orrock, 2012). Differences in plant growth, however, might also reflect phenotypic plasticity (Nicotra et al., 2015; Gentili et al., 2018), so that among-source population differences might disappear after translocation and in the newly established generations (Van Rossum et al., 2020; Van Rossum and Le Pajolec, 2021).
Implications for Translocation Design
The small populations of C. glomerata investigated in this study presented a good potential as providers of a highly diverse genetic pool (at both neutral and adaptive traits), with multiple parentage among the seed progenies, and so can be considered as good candidates as seed sources for translocations. As populations are differentiated from each other, each population only contains a fraction of species' regional genetic diversity. Therefore, mixing the sources will not only optimize the number of variants and of compatible mates in the translocated populations, but also representativeness of species regional genetic diversity.
Genetic diversity poses no immediate threat for the persistence of these populations, but small effective population sizes (and so a limited number of compatible mates) indicate that populations are vulnerable to stochastic extinction (Carroll and Fox, 2008). Moreover, only one of the Belgian populations is located in a protected area (CEC), while the others occur on roadsides or along a quarry exploited for sand (EMO), so that they are particularly vulnerable to anthropogenic disturbances. Alerting the roadside managers to the presence of such valuable populations for critically endangered species is essential. Given the evidence of the onset of processes leading to genetic erosion, inbreeding and inbreeding depression, management measures need to be implemented to counteract these trends. Therefore, population size has to be increased through habitat restoration facilitating recruitment, flowering and pollination, and gene flow has to be restored, by identifying and removing the possible barriers to pollen flow, and reconnecting populations by biological corridors or stepping-stone populations (Van Rossum and Triest, 2012; Ottewell et al., 2016). Creating new populations through translocations in protected and restored areas will also contribute to safeguarding the species' regional genetic diversity and to improve its regional conservation status.
Conclusions
The present study represents a good illustration of the use of genetic tools combining neutral nuclear and plastid molecular markers and adaptive fitness-related quantitative markers to assess the population genetic status of a critically endangered species in the framework of a restoration program involving plant translocations. In particular, estimating parentage, sibship and Ne of the source material intended to be used for translocations can be a useful approach for assessing the genetic quality of seeds and contemporary pollen flow processes.
Data Availability Statement
The datasets presented in this study can be found in online Zenodo repository at https://doi.org/10.5281/zenodo.5676272 and https://doi.org/10.5281/zenodo.5676286 (Le Pajolec et al., 2021; Van Rossum and Godé, 2021).
Le Pajolec, S., Vandelook, F., Van Rossum, F., and Godefroid, S. (2021). Fitness measures of seed progeny from seven natural populations of Campanula glomerata. Zenodo Digital Repository. https://doi.org/10.5281/zenodo.5676272.
Van Rossum, F., and Godé, C. (2021). Individual multilocus genotypes of adults and seed progeny from eight natural populations of Campanula glomerata. Zenodo Digital Repository. https://doi.org/10.5281/zenodo.5676286.
Author Contributions
FVR conceived and designed the research, analyzed the data, and wrote the manuscript. OR conducted the plastid analyses. SLP conducted the germination and growth experiment. CG conducted the microsatellite analyses and genotyping. All authors critically reviewed the manuscript. All authors contributed to the article and approved the submitted version.
Funding
This study was funded by the European Union LIFE+ Nature and Biodiversity Program (Project No. LIFE11 NAT/BE/001060).
Conflict of Interest
The authors declare that the research was conducted in the absence of any commercial or financial relationships that could be construed as a potential conflict of interest.
Publisher's Note
All claims expressed in this article are solely those of the authors and do not necessarily represent those of their affiliated organizations, or those of the publisher, the editors and the reviewers. Any product that may be evaluated in this article, or claim that may be made by its manufacturer, is not guaranteed or endorsed by the publisher.
Acknowledgments
We thank the Département de la Nature et des Forêts (Service Public de Wallonie) and Natagora for giving access to the study sites and authorization to collect plant material, S. Godefroid for help in collecting leaf material and rosette diameter measurement, P. Bardin for collecting material in MAR population, F. Vandelook for seed progeny propagation in the greenhouse, A. Destombes and S. Contreras (Genoscreen, France) for microsatellite development, P. Asselman for help with primer pair selection, W. Baert for plastid molecular analyses, S. Gallina (Evo-Eco-Paleo, Univ. Lille, France) for the STRUCTURE analyses that were carried out using Structure_threader, and R. Gargiulo and two reviewers for constructive comments on the manuscript.
Supplementary Material
The Supplementary Material for this article can be found online at: https://www.frontiersin.org/articles/10.3389/fcosc.2022.829332/full#supplementary-material
References
Aguilar, R., Cristóbal-Pérez, E. J., Balvino-Olvera, F. J., de Jesús Aguilar-Aguilar, M., Aguirre-Acosta, N., Ashworth, L., et al. (2019). Habitat fragmentation reduces plant progeny quality: a global synthesis. Ecol. Lett. 22, 1163–1173. doi: 10.1111/ele.13272
Aguilar, R., Quesada, M., Ashworth, L., Herrerias-Diego, Y., and Lobo, J. (2008). Genetic consequences of habitat fragmentation in plant populations: susceptible signals in plant traits and methodological approaches. Mol. Ecol. 17, 5177–5188. doi: 10.1111/j.1365-294X.2008.03971.x
Albrecht, M., Duelli, P., Müller, C., Kleijn, D., and Schmid, B. (2007). The Swiss agri-environment scheme enhances pollinator diversity and plant reproductive success in nearby intensively managed farmland. J. Appl. Ecol. 44, 813–822. doi: 10.1111/j.1365-2664.2007.01306.x
Albrecht, M. A., and Long, Q. G. (2019). Habitat suitability and herbivores determine reintroduction success of an endangered legume. Plant Divers. 41, 109–117. doi: 10.1016/j.pld.2018.09.004
Angeloni, F., Ouborg, N. J., and Leimu, R. (2011). Meta-analysis on the association of population size and life history with inbreeding depression in plants. Biol. Conserv. 144, 35–43. doi: 10.1016/j.biocon.2010.08.016
Aparicio, J. M., Ortego, J., and Cordero, P. J. (2006). What should we weigh to estimate heterozygosity, alleles or loci? Mol. Ecol. 1, 4659–4665. doi: 10.1111/j.1365-294X.2006.03111.x
Bachmann, U., and Hensen, I. (2006). Are population sizes of Campanula glomerata on the decline following the abandonment of traditional land-use practices? Feddes Repert. 117, 164–171. doi: 10.1002/fedr.200511086
Bachmann, U., and Hensen, I. (2007). Is declining Campanula glomerata threatened by genetic factors? Plant Species Biol. 22, 1–10. doi: 10.1111/j.1442-1984.2007.00170.x
Bachmann, U., Hensen, I., and Partzsch, M. (2005). Is Campanula glomerata threatened by competition of expanding grasses? Plant Ecol. 180, 257–265. doi: 10.1007/s11258-005-3788-0
Barmentlo, S. E., Meirmans, P. G., Luijten, S. H., Triest, L., and Oostermeijer, J. G. B. (2018). Outbreeding depression and breeding system evolution in small, remnant populations of Primula vulgaris: consequences for genetic rescue. Conserv. Genet. 19, 545–554. doi: 10.1007/s10592-017-1031-x
Basey, A. C., Fant, J. B., and Kramer, A. T. (2015). Producing native plant materials for restoration: 10 rules to collect and maintain genetic diversity. Native Plants J. 16, 37–53. doi: 10.3368/npj.16.1.37
Berjano, R., Gauthier, P., Fisogni, A., Doblas, D., Pons, V., and Thompson, J. D. (2013). Mate limitation in populations of the endangered Convolvulus lineatus L.: a case for genetic rescue? J. Nat. Conserv. 21, 334–341. doi: 10.1016/j.jnc.2013.05.001
Betz, C., Scheuerer, M., and Reisch, C. (2013). Population reinforcement—a glimmer of hope for the conservation of the highly endangered Spring Pasque flower (Pulsatilla vernalis). Biol. Conserv. 168, 161–167. doi: 10.1016/j.biocon.2013.10.004
Bittebiere, A.-K., Benot, M.-L., and Mony, C. (2020). Clonality as a key but overlooked driver of biotic interactions in plants. Perspect. Plant Ecol. 43, 125510. doi: 10.1016/j.ppees.2020.125510
Bobo-Pinilla, J., Salmerón-Sánchez, E., Mota, J. M., and Peñas, J. (2021). Genetic conservation strategies of endemic plants from edaphic habitat islands: the case of Jacobaea auricula (Asteraceae). J. Nat. Conserv. 61, 126004. doi: 10.1016/j.jnc.2021.126004
Borrell, J. S., Zohren, J., Nichols, R. A., and Buggs, R. J. A. (2019). Genomic assessment of local adaptation in dwarf birch to inform assisted gene flow. Evol. Appl. 13, 161–175. doi: 10.1111/eva.12883
Bourgeois, Y. X. C., and Warren, B. H. (2021). An overview of current population genomics methods for the analysis of whole-genome resequencing data in eukaryotes. Mol. Ecol. 30, 6036–6071. doi: 10.1111/mec.15989
Bowles, M. L., McBride, J. L., and Bell, T. J. (2015). Long-term processes affecting restoration and viability of the federal threatened Mead's milkweed (Asclepias meadii). Ecosphere 6, 11. doi: 10.1890/ES14-00240.1
Bragg, J. G., Cuneo, P., Sherieff, A., and Rossetto, M. (2020). Optimizing the genetic composition of a translocation population: incorporating constraints and conflicting objectives. Mol. Ecol. Resour. 20, 54–65. doi: 10.1111/1755-0998.13074
Carroll, S. P., and Fox, C. W. (2008). Conservation Biology: Evolution in Action. Oxford, UK: Oxford University Press.
Cavalli-Sforza, L. L., and Edwards, A. W. F. (1967). Phylogenetic analysis: models and estimation procedures. Am. J. Hum. Genet. 19, 233–257. doi: 10.1111/j.1558-5646.1967.tb03411.x
Chapuis, M.-P., and Estoup, A. (2007). Microsatellite null alleles and estimation of population differentiation. Mol. Biol. Evol. 24, 621–631. doi: 10.1093/molbev/msl191
Charlesworth, D.. (2006). Evolution of plant breeding systems. Curr. Biol. 16, R726–R735. doi: 10.1016/j.cub.2006.07.068
Chybicki, I. J., and Burczyk, J. (2009). Simultaneous estimation of null alleles and inbreeding coefficients. J. Hered. 100, 106–113. doi: 10.1093/jhered/esn088
Commander, L. E., Coates, D., Broadhurst, L., Offord, C. A., Makinson, R. O., and Matthes, M. (2018). Guidelines for the Translocation of Threatened Plants in Australia (3rd ed.) Canberra: Australian Network for Plant Conservation.
Coulon, A.. (2010). GENHET: an easy-to-use R function to estimate individual heterozygosity. Mol. Ecol. Resour. 10, 167–169. doi: 10.1111/j.1755-0998.2009.02731.x
Denisow, B., and Wrzesień, M. (2015). The habitat effect on the diversity of pollen resources in several Campanula spp.—an implication for pollinator conservation. J. Apicult. Res. 54, 62–71. doi: 10.1080/00218839.2015.1030243
DiLeo, M. F., Holderegger, R., and Wagner, H. H. (2018). Contemporary pollen flow as a multiscale process: Evidence from the insect-pollinated herb, Pulsatilla vulgaris. J Ecol. 106, 2242–2255. doi: 10.1111/1365-2745.12992
Dudash, M. R., and Murren, C. J. (2008). “The influence of breeding systems and mating systems on conservation genetics and conservation decisions,” in Conservation Biology: Evolution in Action, eds S.P. Carroll, C.W. Fox (Oxford, UK: Oxford University Press), 68–80.
Dunning, L. T., and Savolainen, V. (2010). Broad-scale amplification of matK for DNA barcoding plants, a technical note. Bot. J. Linn. Soc. 16, 1–9. doi: 10.1111/j.1095-8339.2010.01071.x
Earl, D. A., and vonHoldt, B. M. (2012). STRUCTURE HARVESTER: a website and program for visualizing STRUCTURE output and implementing the Evanno method. Conserv. Genet. Resour. 4, 359–361. doi: 10.1007/s12686-011-9548-7
Edmands, S.. (2007). Between a rock and a hard place: evaluating the relative risks of inbreeding and outbreeding for conservation and management. Mol. Ecol. 16, 463–475. doi: 10.1111/j.1365-294X.2006.03148.x
Ellstrand, N. C., and Roose, M. L. (1987). Patterns of genotypic diversity in clonal plant species. Am. J. Bot. 71, 123–131. doi: 10.1002/j.1537-2197.1987.tb08586.x
ENSCONET. (2009). ENSCONET Seed Collecting Manual for Wild Species. European Native Seed Conservation Network. Available online at: http://ensconet.maich.gr/PDF/Collecting_protocol_English.pdf (accessed November 11, 2021).
Evanno, G., Regnaut, S., and Goudet, J. (2005). Detecting the number of clusters of individuals using the software STRUCTURE: a simulation study. Mol. Ecol. 14, 2611–2620. doi: 10.1111/j.1365-294X.2005.02553.x
Falush, D., Stephens, M., and Pritchard, J. K. (2007). Inference of population structure using multilocus genotype data: dominant markers and null alleles. Mol. Ecol. Notes 7, 574–578. doi: 10.1111/j.1471-8286.2007.01758.x
Ford, C. S., Ayres, K. L., Toomey, N., Haider, N., Van Alphen Stahl, J., Kelly, L. J., et al. (2009). Selection of candidate coding DNA barcoding regions for use on land plants. Bot. J. Linn. Soc. 159, 1–11. doi: 10.1111/j.1095-8339.2008.00938.x
Gadella, T. W. J.. (1964). Cytotaxonomic studies in the genus Campanula. Wentia 11, 1–104. doi: 10.1111/j.1438-8677.1964.tb00014.x
Garcia-Jacas, N., Requena, J., Massó, S., Vilatersana, R., Blanché, C., and López-Pujol, J. (2021). Genetic diversity and structure of the narrow endemic Seseli farrenyi (Apiaceae): implications for translocation. PeerJ 9, e10521. doi: 10.7717/peerj.10521
Gargiulo, R., Adamo, M., Cribb, P. J., Bartolucci, F., Sarasan, V., Alessandrelli, C., et al. (2021). Combining current knowledge of Cypripedium calceolus with a new analysis of genetic variation in Italian populations to provide guidelines for conservation actions. Conserv. Sci. Pract. 3, e513. doi: 10.1111/csp2.513
Gargiulo, R., Worswick, G., Arnold, C., Pike, L. J., Cowan, R. S., Hardwick, K. A., et al. (2019). Conservation of the threatened species, Pulsatilla vulgaris Mill. (Pasqueflower), is aided by reproductive system and polyploidy. J. Hered. 110, 618–628. doi: 10.1093/jhered/esz035
Gentili, R., Solari, A., Diekmann, M., Duprè, C., Monti, G. S., Armiraglio, S., et al. (2018). Genetic differentiation, local adaptation and phenotypic plasticity in fragmented populations of a rare forest herb. PeerJ. 6, e4929. doi: 10.7717/peerj.4929
Ghazoul, J.. (2005). Pollen and seed dispersal among dispersed plants. Biol. Rev. 80, 413–443. doi: 10.17/S1464793105006731
Godefroid, S., Le Pajolec, S., and Van Rossum, F. (2016a). Pre-translocation considerations in rare plant reintroductions: implications for designing protocols. Plant Ecol. 217, 1693–1182. doi: 10.1007/s11258-015-0526-0
Godefroid, S., Le Pajolec, S., and Van Rossum, F. (2016b). Rescuing critically endangered species in Belgium—an ambitious reintroduction program of the Botanic Garden Meise. BGJournal 13, 24–27.
Godefroid, S., Piazza, C., Rossi, G., Buord, S., Stevens, A.-D., Aguraiuja, R., et al. (2011). How successful are plant species reintroductions? Biol. Conserv. 144, 672–682. doi: 10.1016/j.biocon.2010.10.003
Goudet, J.. (2003). FSTAT (ver. 2.9.4), A Program to Estimate and Test Population Genetics Parameters. Available online at: https://www2.unil.ch/popgen/softwares/fstat.htm. Updated from Goudet (1995) (accessed November 11, 2021).
Green, P. R., Green, I. P., and Crouch, G. A. (1997). The Atlas Flora of Somerset. Privately Published by the Authors, Wayford and Yeovil. p.[i-ii +] 1–291.
Hamilton, J. A., Royauté, R., Wright, J. W., Hodgskiss, P., and Ledig, F. T. (2017). Genetic conservation and management of the California endemic, Torrey pine (Pinus torreyana Parry): implications of genetic rescue in a genetically depauperate species. Ecol. Evol. 7, 7370–7381. doi: 10.1002/ece3.3306
Hardy, O. J., González-Martínez, S. C., Fréville, H., Boquien, G., Mignot, A., Colas, B., et al. (2004). Fine-scale genetic structure and gene dispersal in Centaurea corymbosa (Asteraceae) I. Pattern of pollen dispersal. J. Evol. Biol. 17, 795–806. doi: 10.1111/j.1420-9101.2004.00713.x
Hautekèete, N.-C., Frachon, L., Luczak, C., Toussaint, B., Van Landuyt, W., Van Rossum, F., et al. (2015). Habitat type shapes long-term plant biodiversity budgets in two densely populated regions in north-western Europe. Diversity Distrib. 21, 631–642. doi: 10.1111/ddi.12287
Hewitt, G. M.. (2004). Genetic consequences of climatic oscillations in the Quaternary. Phil. Trans. R. Soc. Lond. B. 359, 183–195. doi: 10.1098/rstb.2003.1388
Hickerson, M. J., Carstens, B. C., Cavender-Bares, J., Crandall, K. A., Graham, C. H., Johnson, J. B., et al. (2010). Phylogeography's past, present, and future: 10 years after Avise, 2000. Mol. Phylogenet. Evol. 54, 291–301. doi: 10.1016/j.ympev.2009.09.016
Hill, M. O., Preston, C. D., and Roy, D. B. (2004). PLANTATT—Attributes of British and Irish Plants: Status, Size, Life History, Geography and Habitats. Huntingdon: NERC Centre for Ecology and Hydrology.
Hoban, S., Griffith, P., Richards, C., and Way, M. (2018). “Chapter 9. How many individuals? How many populations?” in BGCI and IABG's Species Recovery Manual, eds V. Heywood, K. Shaw, Y. Harvey-Brown, P. Smith (Richmond, UK: Botanic Gardens Conservation International), 60–67.
Hollingsworth, P. M., Graham, S. W., and Little, D. P. (2011). Choosing and using a plant DNA barcode. PLoS ONE 6, e19254. doi: 10.1371/journal.pone.0019254
Hultén, E., and Fries, M. (1986). Atlas of North European Vascular Plants: North of the Tropic of Cancer I-III. Königstein: Koeltz Scientific Journals.
Jacquemart, A.-L., Buyens, C., Delescaille, L.-M., and Van Rossum, F. (2021). Using genetic evaluation to guide conservation of remnant Juniperus communis (Cupressaceae) populations. Plant Biol. J. 23, 193–204. doi: 10.1111/plb.13188
Jacquemyn, H., Van Rossum, F., Brys, R., Endels, P., Hermy, M., Triest, L., et al. (2003). Effects of agricultural land use and fragmentation on genetics, demography and population persistence of the rare primula vulgaris, and implications for conservation. Belg. J. Bot. 136, 5–22. doi: 10.2307/20794510
Jakobsson, M., and Rosenberg, N. A. (2007). CLUMPP: a cluster matching and permutation program for dealing with label switching and multimodality in analysis of population structure. Bioinformatics 23, 1801–1806. doi: 10.1093/bioinformatics/btm233
Jones, O., and Wang, J. (2010). COLONY: a program for parentage and sibship inference from multilocus genotype data. Mol. Ecol. Resour. 10, 551–555. doi: 10.1111/j.1755-0998.2009.02787.x
Kinghorn, B., and Kinghorn, S. (2015). Pedigree Viewer Version 6.5f. Available online at: https://bkinghor.une.edu.au/pedigree.htm (accessed November 21, 2021).
Klimešová, J., and Klimeš, L. (2013). Clo-Pla3—database of clonal growth of plants from Central Europe. Available online at: http://clopla.butbn.cas.cz/ (accessed December 20, 2021).
Klotz, S., Kühn, I., and Durka, W. (2002). Biolflor – Eine Datenbank mit biologisch-ökologischen Merkmalen zur Flora von Deutschland. Schriftenr. Vegetationskd. 38, 1–334.
Krauss, S., Dixon, B., and Dixon, K. W. (2002). Rapid genetic decline in a translocated population of the endangered plant Grevillea scapigera. Conserv. Biol. 14, 986–994. doi: 10.1046/j.1523-1739.2002.01105.x
Llaurens, V., Castric, V., Austerlitz, F., and Vekemans, X. (2008), High paternal diversity in the self-incompatible herb Arabidopsis halleri despite clonal reproduction spatially restricted pollen dispersal. Mol. Ecol. 17, 1577–1588. doi: 10.1111/j.1365-294X.2007.03683.x
Luikart, G., Ryman, N., Tallmon, D. A., Schwartz, M. K., and Allendorf, F. W. (2010). Estimation of census and effective population sizes: the increasing usefulness of DNA-based approaches. Conserv. Genet. 11, 355–373. doi: 10.1007/s10592-010-0050-7
Martin, H., Touzet, P., Dufay, M., Godé, C., Schmitt, E., Lahiani, E., et al. (2017). Lineages of Silene nutans developed rapid, strong, asymmetric postzygotic reproductive isolation in allopatry. Evolution 71, 1519–1531. doi: 10.1111/evo.13245
Maschinski, J., and Albrecht, M. A. (2017). Center for plant conservation's best practice guidelines for the reintroduction of rare plants. Plant Divers. 39, 390–395. doi: 10.1016/j.pld.2017.09.006
Maurice, T., Colling, G., Muller, S., and Matthies, D. (2012). Habitat characteristics, stage structure and reproduction of colline and montane populations of the threatened species Arnica montana. Plant Ecol. 21, 831–842. doi: 10.1007/s11258-012-0045-1
Meeus, S., Honnay, O., Brys, R., and Jacquemyn, H. (2012). Biased morph ratios and skewed mating success contribute to loss of genetic diversity in the distylous Pulmonaria officinalis. Ann. Bot. 109, 227–235. doi: 10.1093/aob/mcr272
Menges, E. S.. (2008). Restoration demography and genetics of plants: when is a translocation successful? Austr. J. Bot. 56, 187–196. doi: 10.1071/BT07173
Monks, L., Standish, R., McArthur, S., Dillon, R., Byrne, M., and Coates, D. (2021). Genetic and mating system assessment of translocation success of the long-lived perennial shrub Lambertia orbifolia (Proteaceae). Restor. Ecol. 29, e13369. doi: 10.1111/rec.13369
Nicotra, A. B., Beever, E. A., Robertson, A. L., Hofmann, G. E., and O'Leary, J. (2015). Assessing the components of adaptive capacity to improve conservation and management efforts under global change. Conserv. Biol. 29, 1268–1278. doi: 10.1111/cobi.12522
Oostermeijer, J.G.B., Luijten, S.H., and den Nijs, J.C.M. (2003). Integrating demographic and genetic approaches in plant conservation. Biol. Conserv. 113, 389–398. doi: 10.1016/S0006-3207(03)00127-7
Ottewell, K. M., Bickerton, D. C., Byrne, M., and Lowe, A. J. (2016). Bridging the gap: a genetic assessment framework for population-level threatened plant conservation prioritization and decision-making. Divers. Distrib. 22, 174–188. doi: 10.1111/ddi.12387
Oxelman, B., Lidén, M., and Berglund, D. (1997). Chloroplast rps16 intron phylogeny of the tribe Sileneae (Caryophyllaceae). Plant. Syst. Evol. 206, 393–410. doi: 10.1007/BF00987959
Parks, J. C., and Werth, C.R. (1993). A study of spatial features of clones in a population of bracken fern, Pteridium aquilinum (Dennstaedtiaceae). Am. J. Bot. 80, 537–544. doi: 10.2307/2445369
Pazzaglia, J., Nguyen, H. M., Santillán-Sarmiento, A., Ruocco, M., Dattolo, E., Marín-Guirao, L., et al. (2021). The genetic component of seagrass restoration: what we know and the way forwards. Water 13, 829. doi: 10.3390/w13060829
Peakall, R., and Smouse, P. E. (2012). GenAlEx 6.5: genetic analysis in Excel. Population genetic software for teaching and research-an update. Bioinformatics 28, 2537–2539. doi: 10.1093/bioinformatics/bts460
Peery, M. Z., Kirby, R., Reid, B. N., Stoelting, R., Doucet-Bëer, E., Robinson, S., et al. (2012). Reliability of genetic bottleneck tests for detecting recent population declines. Mol. Ecol. 21, 3403–3418. doi: 10.1111/j.1365-294X.2012.05635.x
Pina-Martins, F., Silva, D. N., Fino, J., and Paulo, O. S. (2017). Structure_threader: an improved method for automation and parallelization of programs structure, fastStructure and MavericK on multicore CPU systems. Mol. Ecol. Resour. 17, e268–e274. doi: 10.1111/1755-0998.12702
Prati, D., Peintinger, M., and Fischer, M. (2016). Genetic composition, genetic diversity and small-scale environmental variation matter for the experimental reintroduction of a rare plant. J. Plant Ecol. 9, 805–813. doi: 10.1093/jpe/rtv067
Pritchard, J. K., Stephens, M., and Donnelly, P. (2000). Inference of population structure using multilocus genotype data. Genetics 155, 949–959. doi: 10.1093/genetics/155.2.945
Rambaut, A.. (2016). FigTree Tree Figure Drawing Tool Version 1.4.3. Available online at: http://tree.bio.ed.ac.uk/software/figtree/ (accessed November 11, 2021).
Reckinger, C., Colling, G., and Matthies, D. (2010). Restoring populations of the endangered plant Scorzonera humilis: influence of site conditions, seed source, and plant stage. Restor. Ecol. 18, 904–913. doi: 10.1111/j.1526-100X.2009.00522.x
Ritchie, A. L., and Krauss, S. L. (2012). A genetic assessment of ecological restoration success in Banksia attenuata. Restor. Ecol. 20, 441–449. doi: 10.1111/j.1526-100X.2011.00791.x
Roach, D. A., and Wulff, R. D. (1987). Maternal effects in plants. Annu. Rev. Ecol. Evol. Syst. 18, 209–235. doi: 10.1146/annurev.es.18.110187.001233
Rosenberg, N. A.. (2004). DISTRUCT: a program for the graphical display of population structure. Mol. Ecol. Notes 4, 137–138. doi: 10.1046/j.1471-8286.2003.00566.x
Saintenoy-Simon, J., Barbier, Y., Delescaille, L.-M., Dufrêne, M., Gathoye, J.-L., and Verté, P. (2006). Première liste des espèces rares, menacées et protégées de la Région Wallonne (Ptéridophytes et Spermatophytes). Version 1 (7/3/2006). Available online at: http://biodiversite.wallonie.be/fr/plantes-protegees-et-menacees.html?IDC=3076 (accessed November 10, 2021).
Sang, T., Crawford, D. J., and Stuessy, T. F. (1997). Chloroplast DNA phylogeny, reticulate evolution and biogeography of Paeonia (Paeoniaceae). Am. J. Bot. 84, 1120–1136. doi: 10.2307/2446155
Schäfer, D., Vincent, H., Fischer, M., and Kempel, A. (2020). The importance of genetic diversity for the translocation of eight threatened plant species into the wild. Global Ecol. Conserv. 24, e01240. doi: 10.1016/j.gecco.2020.e01240
Schuler, M. S., and Orrock, J. L. (2012). The maladaptive significance of maternal effects for plants in anthropogenically modified environments. Evol. Ecol. 26, 475–481. doi: 10.1007/s10682-011-9499-1
Sgrò, C. M., Andrew, J., Lowe, A. J., and Hoffmann, A. A. (2011). Building evolutionary resilience for conserving biodiversity under climate change. Evol. Appl. 4, 326–337. doi: 10.1111/j.1752-4571.2010.00157.x
Shaw, J., Lickey, E. B., Beck, J. T., Farmer, S. B., Liu, W., Miller, J., et al. (2005). The tortoise and the hare II: relative utility of 21 noncoding chloroplast DNA sequences for phylogenetic analysis. Am. J. Bot. 92, 142–166. doi: 10.3732/ajb.92.1.142
Silcock, J. L., Simmons, C. L., Monks, L., Dillon, R., Reiter, N., Jusaitis, M., et al. (2019). Threatened plant translocation in Australia: a review. Biol. Conserv. 236, 211–222. doi: 10.1016/j.biocon.2019.05.002
St. Clair, A. B., Dunwiddie, P. W., Fant, J. B., Kaye, T. N., and Kramer, A. T. (2020). Mixing source populations increases genetic diversity of restored rare plant populations. Restor. Ecol. 28, 583–593. doi: 10.1111/rec.13131
Strzałkowska-Abramek, M., Jachuła, J., Wrzesień, M., Bozek, M., Dabrowska, A., and Denisow, B. (2018). Nectar production in several Campanula species (Campanulaceae). Acta Sci. Polonorum Hortorum Cult. 17, 127–136. doi: 10.24326/asphc.2018.3.13
Tate, J. A., and Simpson, B. B. (2003). Paraphyly of Tarasa (Malvaceae) and diverse origins of the polyploid species. Syst. Bot. 28, 723–737. doi: 10.1043/02-64.1
Thomas, W. J. W., Anthony, J. M., Dobrowolski, M. P., and Krauss, S. L. (2021). Optimising the conservation of genetic diversity of the last remaining population of a critically endangered shrub. AoB Plants 13, plab005. doi: 10.1093/aobpla/plab005
Tierney, D., Ahrens, C., Rymer, P., and Auld, T. D. (2020). The interaction of clonality, breeding system and genomics for a highly threatened plant species and the management implications. Biodivers. Conserv. 29, 3009–3029. doi: 10.1007/s10531-020-02012-7
Van Geert, A., Van Rossum, F., and Triest, L. (2008). Genetic diversity in adult and seedling populations of Primula vulgaris in a fragmented agricultural landscape. Conserv. Genet. 9, 845–853. doi: 10.1007/s10592-007-9409-9
Van Geert, A., Van Rossum, F., and Triest, L. (2015). Perspectives for genetic rescue of the extremely fragmented Primula vulgaris populations in The Netherlands: reflecting the future of Belgian populations? Plant Ecol. Evol. 148, 329–334. doi: 10.5091/plecevo.2015.1101
Van Rossum, F.. (2008). Conservation of long-lived perennial forest herbs in an urban context: Primula elatior as study case. Conserv. Genet. 9, 119–128. doi: 10.1007/s10592-007-9314-2
Van Rossum, F.. (2010). Reproductive success and pollen dispersal in urban populations of an insect-pollinated hay-meadow herb. Perspect. Plant Ecol. 12, 21–29. doi: 10.1016/j.ppees.2009.08.002
Van Rossum, F., Destombes, A., and Raspé, O. (2021). Are large census-sized populations always the best sources for plant translocations? Restor. Ecol. 29, e13316. doi: 10.1111/rec.13316
Van Rossum, F., and Godé, C. (2021). Development of highly polymorphic microsatellite markers for Campanula glomerata L. (Campanulaceae). Mol. Biol. Rep. 49, 805–810. doi: 10.1007/s11033-021-06839-3
Van Rossum, F., and Hardy, O. J. (2021). Guidelines for genetic monitoring of translocated plant populations. Conserv. Biol. doi: 10.1111/cobi.13670. [Epub ahead of print].
Van Rossum, F., Hardy, O. J., Le Pajolec, S., and Raspé, O. (2020). Genetic monitoring of translocated plant populations in practice. Mol. Ecol. 29, 4040–4058. doi: 10.1111/mec.15550
Van Rossum, F., and Le Pajolec, S. (2021). Mixing gene pools to prevent inbreeding issues in translocated populations of clonal species. Mol. Ecol. 30, 2756–2771. doi: 10.1111/mec.15930
Van Rossum, F., Martin, H., Le Cadre, S., Brachi, B., Christenhusz, M., and Touzet, P. (2018). Phylogeography of a widely distributed species reveals a cryptic assemblage of distinct genetic lineages needing separate conservation strategies. Perspect. Plant Ecol. 35, 44–51. doi: 10.1016/j.ppees.2018.10.003
Van Rossum, F., and Raspé, O. (2018). Contribution of genetics for implementing population translocation of the threatened Arnica montana. Conserv. Genet. 19, 1185–1198. doi: 10.1007/s10592-018-1087-2
Van Rossum, F., and Triest, L. (2010). Pollen dispersal in an insect-pollinated wet meadow herb along an urban river. Landscape Urban Plann. 95, 201–208. doi: 10.1016/j.landurbplan.2010.01.004
Van Rossum, F., and Triest, L. (2012). Stepping-stone populations in linear landscape elements increase pollen dispersal between urban forest fragments. Plant Ecol. Evol. 145, 332–340. doi: 10.5091/plecevo.2012.737
Vekemans, X., and Lefèbvre, C. (1997). On the evolution of heavy-metal tolerant populations in Armeria maritima: evidence from allozyme variation and reproductive barriers. J. Evol. Biol. 10, 175–191. doi: 10.1046/j.1420-9101.1997.10020175.x
Vera, M., Mora, G., Rodríguez-Guitián, M. A., Blanco, A., Casanova, A., Real, C., et al. (2020). Living at the edge: population differentiation in endangered Arnica montana from NW Iberian Peninsula. Plant Syst. Evol. 306, 44. doi: 10.1007/s00606-020-01673-9
Vergeer, P., Sonderen, E., and Ouborg, N. J. (2004). Introduction strategies put to the test: local adaptation versus heterosis. Conserv. Biol. 18, 812–821. doi: 10.1111/j.1523-1739.2004.00562.x
Volis, S., and Blecher, M. (2021). Translocation success in Iris atrofusca: importance of replicating sites and long-term monitoring. Restor. Ecol. 2021, e13502. doi: 10.1111/rec.13502
Wang, J.. (2009). A new method for estimating effective population sizes from a single sample of multilocus genotypes. Mol. Ecol. 18, 2148–2164. doi: 10.1111/j.1365-294X.2009.04175.x
Wang, J.. (2016). A comparison of single-sample estimators of effective population sizes from genetic marker data. Mol. Ecol. 25, 4692–4711. doi: 10.1111/mec.13725
Wang, J.. (2018). Estimating genotyping errors from genotype and reconstructed pedigree data. Methods Ecol. Evol. 9, 109–120. doi: 10.1111/2041-210X.12859
Wang, J.. (2019). Pedigree reconstruction from poor quality genotype data. Heredity 122, 719–728. doi: 10.1038/s41437-018-0178-7
Wang, J., Brekke, P., Huchard, E., Knapp, L., and Cowlishaw, G. (2010). Estimation of parameters of inbreeding and genetic drift in populations with overlapping generations. Evolution 64, 1704–1718. doi: 10.1111/j.1558-5646.2010.00953.x
Weeks, A. R., Sgrò, C. M., Young, A. G., Frankham, R., Mitchell, N. L., Miller, K. A., et al. (2011). Assessing the benefits and risks of translocations in changing environments: a genetic perspective. Evol. Appl. 4, 709–725. doi: 10.1111/j.1752-4571.2011.00192.x
Weir, B. S., and Cockerham, C. C. (1984). Estimating F-statistics for the analysis of population structure. Evolution 38, 1358–1370. doi: 10.1111/j.1558-5646.1984.tb05657.x
Wiberg, R. A. W., Scobie, A. R., A'Hara, S. W., Ennos, R. A., and Cottrell, J. E. (2016). The genetic consequences of long term habitat fragmentation on a self-incompatible clonal plant, Linnaea borealis L. Biol. Conserv. 201, 405–413. doi: 10.1016/j.biocon.2016.07.032
Willi, Y., Van Buskirk, J., and Fischer, M. (2005). A threefold genetic Allee effect: population size affects cross-compatibility, inbreeding depression and drift load in the self-incompatible Ranunculus reptans. Genetics 169, 2255–2265. doi: 10.1534/genetics.104.034553
Willi, Y., Van Buskirk, J., Schmid, B., and Fischer, M. (2007). Genetic isolation of fragmented populations is exacerbated by drift and selection. J. Evol. Biol. 20, 534–542. doi: 10.1111/j.1420-9101.2006.01263.x
Xia, W., Zhang, B., Xing, D., Li, Y., Wu, W., Xiao, Y., et al. (2019). Development of high-resolution DNA barcodes for Dioscorea species discrimination and phylogenetic analysis. Ecol. Evol. 9, 10843–10853. doi: 10.1002/ece3.5605
Zavodna, M., Abdelkrim, J., Pellissier, V., and Machon, N. (2015). A long-term genetic study reveals complex population dynamics of multiple-source plant reintroductions. Biol. Conserv. 192, 1–9. doi: 10.1016/j.biocon.2015.08.025
Zimmer, H., Auld, T., Cuneo, P., Offord, C., and Commander, L. (2019). Conservation translocation—An increasingly viable option for managing threatened plant species. Austr. J. Bot. 67, 501–509. doi: 10.1071/BT19083
Keywords: bottlenecks, Campanula glomerata, biparental inbreeding, effective population size, inbreeding depression, parentage, plant translocations, sibship
Citation: Van Rossum F, Le Pajolec S, Raspé O and Godé C (2022) Assessing Population Genetic Status for Designing Plant Translocations. Front. Conserv. Sci. 3:829332. doi: 10.3389/fcosc.2022.829332
Received: 05 December 2021; Accepted: 05 January 2022;
Published: 07 February 2022.
Edited by:
Roberta Gargiulo, Royal Botanic Gardens, Kew, United KingdomReviewed by:
Ashley B. Morris, Furman University, United StatesJoyce Maschinski, Center for Plant Conservation, United States
Copyright © 2022 Van Rossum, Le Pajolec, Raspé and Godé. This is an open-access article distributed under the terms of the Creative Commons Attribution License (CC BY). The use, distribution or reproduction in other forums is permitted, provided the original author(s) and the copyright owner(s) are credited and that the original publication in this journal is cited, in accordance with accepted academic practice. No use, distribution or reproduction is permitted which does not comply with these terms.
*Correspondence: Fabienne Van Rossum, ZmFiaWVubmUudmFucm9zc3VtJiN4MDAwNDA7Ym90YW5pY2dhcmRlbm1laXNlLmJl
†Present address: Olivier Raspé, School of Science, Mae Fah Luang University, Chiang Rai, Thailand