- 1Department of Wildland Resources, Utah State University, Logan, UT, United States
- 2Jack H. Berryman Institute, Utah State University, Logan, UT, United States
- 3Center for Integrated Spatial Research, Environmental Studies Department, University of California, Santa Cruz, Santa Cruz, CA, United States
- 4Ecology Center, Utah State University, Logan, UT, United States
- 5U.S. Geological Survey, Western Ecological Research Center, Dixon Field Station, Dixon, CA, United States
Translocated animals undergo a phase of behavioral adjustment after being released in a novel environment, initially prioritizing exploration and gradually shifting toward resource exploitation. This transition has been termed post-release behavioral modification. Post-release behavioral modification may also manifest as changes in habitat selection through time, and these temporal dynamics may differ between individuals. We aimed to evaluate how post-release behavioral modification is reflected in temporal dynamics of habitat selection and its variability across individuals using a population of translocated female greater sage-grouse as a case study. Sage-grouse were translocated from Wyoming to North Dakota (USA) during the summers of 2018–2020. We analyzed individual habitat selection as a function of sagebrush cover, herbaceous cover, slope, and distance to roads. Herbaceous cover is a key foraging resource for sage-grouse during summer; thus, we expected a shift from exploration to exploitation to manifest as temporally-varying selection for herbaceous cover. For each individual sage-grouse (N = 26), we tested two competing models: a null model with no time-dependence and a model with time-dependent selection for herbaceous cover. We performed model selection at the individual level using an information-theoretic approach. Time-dependence was supported for five individuals, unsupported for seven, and the two models were indistinguishable based on AICc for the remaining fourteen. We found no association between the top-ranked model and individual reproductive status (brood-rearing or not). We showed that temporal dynamics of post-release habitat selection may emerge in some individuals but not in others, and that failing to account for time-dependence may hinder the detection of steady-state habitat selection patterns. These findings demonstrate the need to consider both temporal dynamics and individual variability in habitat selection when conducting post-release monitoring to inform translocation protocols.
Introduction
Most animals select for areas that have been previously visited – their familiar space (Wolf et al., 2009; Avgar et al., 2015; Ranc et al., 2020b). This behavioral process has been shown to provide benefits in terms of resource acquisition (Merkle et al., 2014; Ranc et al., 2021) and reduction of predation risk (Gehr et al., 2020). Translocated animals experience an unfamiliar landscape and therefore often undergo a phase of behavioral adjustment after being released in a novel environment (Berger-Tal and Saltz, 2014; Berger-Tal et al., 2020). During this initial phase, translocated individuals learn information about their new environment and may undertake exploratory movements to build a cognitive map of their surroundings (Berger-Tal and Saltz, 2014). Exploration and resource exploitation are at opposite ends of a behavioral gradient that translocated animals exhibit after release (Berger-Tal et al., 2014). Initially, the trade-off leans toward exploration; then, individuals gradually shift their behavior toward exploitation as they become more familiar with their new environmental context – a process that underlies the emergence of stable home ranges (Ranc et al., 2020a). The transition from exploration to exploitation has been termed post-release behavioral modification (PRBM; Berger-Tal and Saltz, 2014).
Post-release monitoring is important for understanding the success of translocation efforts (Bubac et al., 2019). Typically, the goal of monitoring is to assess individual behavior (such as habitat selection) and vital rates (such as survival or reproductive success) post-translocation (Armstrong and Seddon, 2008; Parker et al., 2013). This information helps to fine-tune translocation protocols within the context of an adaptive management framework, for example, by adjusting the choice of release sites or individuals to translocate (Letty et al., 2007; Osborne and Seddon, 2012).
When monitoring post-release behavior – for example, using telemetry devices fitted on translocated individuals – not accounting for PRBM may bias or confound estimates of habitat selection. An animal's habitat selection changes depending on current motivation and internal state (Nathan et al., 2008; Roever et al., 2014), and motivation is fundamentally different when animals are focused on exploration vs. exploitation. This issue is frequently ignored, or sometimes dealt with by discarding data for the first few days or weeks after release (e.g., Mondal et al., 2013; Werdel et al., 2021), during which behavior is assumed not to be representative of a steady state. In the latter case, the choice of the temporal cut-off to use is arbitrary and relies on the assumption that every individual in the population behaves similarly.
Evidence is increasing that individual behavioral responses to stimuli are idiosyncratic in wild populations (Cote et al., 2010; Bonnot et al., 2015; Merrick and Koprowski, 2017). Individuals with different temperaments may react differently when exposed to novel environments (Germano et al., 2017; de Azevedo and Young, 2021). Individual differences may manifest, for example, in the degree of risk-aversion exhibited during exploration (Montagne, 2016), stress-tolerance (May et al., 2016), or dispersal distances (Richardson et al., 2017); but individuals may also differ in the time it takes for them to move along the exploration/exploitation continuum, or in how their habitat selection changes as their internal state shifts.
Explicitly modeling temporal dynamics and accounting for individual heterogeneity should improve the quality of our inference on habitat selection in translocated populations. Incorporating these elements into the analysis of post-release behavior may improve translocation protocols aimed at maximizing the probability of successful population restoration. In this study, we evaluated individual variation in temporal dynamics of habitat selection after release in a novel environment using a population of greater sage-grouse (Centrocercus urophasianus; hereafter, sage-grouse) translocated from Wyoming, USA, to North Dakota, USA, as a case study.
Translocation has been used as a management tool to augment declining sage-grouse populations across their range (Reese and Connelly, 1997; Baxter et al., 2008, 2013; Duvuvuei et al., 2017). Sage-grouse populations have undergone severe declines since the 1960s (Garton et al., 2011; Coates et al., 2021). In North Dakota, the extant sage-grouse population experienced a sharp decline due to habitat loss and a West Nile Virus (Flavivirus spp.) disease outbreak in the mid-2000s (Garton et al., 2011). Augmentation of this declining population was initiated in 2017 with translocation of individuals from a large, stable population in central Wyoming (Coates et al., 2021). Using data from this translocation, our objectives were to evaluate: (1) whether sage-grouse exhibited individual variation in habitat selection during PRBM, and (2) whether including time-dependent terms improved inference on individual habitat selection.
Materials and Methods
We captured female sage-grouse during summers 2018–2020 from the source population near Stewart Creek, WY at night using spotlights and dip-nets cast from all-terrain vehicles (Wakkinen et al., 1992). The captured individuals included brood-rearing females (captured in June with their young chicks, 7–40 days old; Meyerpeter et al., 2021) and non-brood-rearing females (captured in June without a brood). All individuals were translocated within 24 h post-capture (Lazenby et al., 2021). We measured and weighed individuals and equipped them with rump-mounted Global Positioning System (GPS) Platform Transmitter Terminal (PTT) ARGOS-enabled tracking devices (23 g, GeoTrak©, Inc.) scheduled to acquire six locations a day at irregular intervals. In the case of brood-rearing females, we also captured their chicks, weighed them, and equipped them with Very High Frequency (VHF) suture-style tracking devices (1.1 g, Holohil Systems©, Ltd.). During transport, females and their chicks were kept separated to prevent injury but within audio-visual contact in specialized transport boxes (Meyerpeter et al., 2021). We translocated captured sage-grouse to Bowman County, ND and released them using a soft-release method, i.e., allowing for an acclimation period (~30–45 min) in a release pen. The source habitat in WY is part of the Wyoming Basin Sage-Grouse Management Zone, characterized by large, uninterrupted sagebrush (Artemisia spp.) steppe (Lazenby et al., 2021). The sage-grouse population in this area is one of the largest remaining across the species range (Coates et al., 2021). The release area in ND is part of the Great Plains Sage-Grouse Management Zone which is a grass-dominated landscape interspersed with small sagebrush patches (Lazenby et al., 2021). The sage-grouse population in this area constitutes a small remnant fragment at the northeastern periphery of the species range (Coates et al., 2021).
To quantify temporal dynamics in post-release habitat selection, we analyzed GPS-tracking data for female sage-grouse in the 60 d following their translocation to ND. Model predictors included percent sagebrush cover, percent herbaceous cover, slope, and distance to roads; based on previous literature, these variables are known to be important predictors of sage-grouse habitat selection across their range (Connelly et al., 2011; Dinkins et al., 2014) as well as specifically in the translocated ND population (Lazenby et al., 2021). We obtained percent sagebrush and herbaceous cover data at a 30-m resolution from the National Land Cover Database (NLCD 2016; https://www.mrlc.gov/national-land-cover-database-nlcd-2016). We obtained data on slope at a 30-m resolution from Landfire (https://landfire.gov/). We obtained data on roads from the USGS National Transportation Dataset (USGS 2014), including paved roads, highways, and interstates as well as gravel or dirt roads. We rasterized the roads vector layer to a 30-m resolution and calculated distance of each pixel to the nearest road. We log-transformed distance values to allow for a spatial decay in the behavioral response to roads. We scaled and centered all predictors before including them in the model.
We used integrated step selection analysis (iSSA; Avgar et al., 2016) to quantify habitat selection. Because iSSA requires data at regular time intervals, we scanned our dataset to find the most frequent regular interval between locations (i.e., 11 h) and only retained 11-h steps. An iSSA simultaneously models the habitat selection process [denoted as w(x)] and the movement process [denoted as ϕ(x)] underlying an animal's space use (Avgar et al., 2016). For all models, we modeled step lengths as realizations of a gamma distribution, which accounts for the heavy-tailed distribution of movement distances typically observed in empirical data; and turning angles as realizations of a von Mises distribution, which accounts for directional persistence. Both distributions were initially parameterized with population-level data (gamma with shape = 0.72 and scale = 725.04; von Mises with mean = 0.00 and concentration = 0.10). Accordingly, our movement process model was ϕ(x) = exp[α1l + α2log(l) + α3cos(θ)], where l is the step length and θ is the turning angle. We sampled 100 available steps for each observed step using these population-level gamma and von Mises distributions.
We tested our hypothesis of temporal modification of habitat selection by fitting competing models for the habitat selection process. We used model selection to evaluate whether individuals exhibited temporal modification of habitat selection by fitting an interaction between a focal predictor and the natural logarithm of time, measured as days since translocation. Herbaceous cover is the primary driver of habitat selection for translocated sage-grouse during summer (our period of investigation) in our study area. In summer, sage-grouse – and especially females with broods – rely on grasses, forbs, and the associated insect community as the main food sources for themselves and their young (Connelly et al., 2000, 2011). In the grass-dominated ecosystem of North Dakota, where sagebrush cover is sparser than in most other areas of the species range, the availability of herbaceous cover plays a more important role than sagebrush cover at fine scales (Kaczor et al., 2011). As such, if time-dependent habitat selection were to emerge in our study system, we would expect it to manifest primarily in the response to herbaceous cover. We expected individual responses to potentially vary through time for resources but not for conditions, thus we chose not to test for time-dependent responses to slope. Sagebrush is not a limiting resource for sage-grouse during summer as much as during winter, when alternative food sources are not available (Connelly et al., 2000; Swanson et al., 2013); although it remains an important broad-scale predictor of sage-grouse habitat selection, we did not expect it to be the main driver of habitat selection at fine scales during summer. Selection for roads may potentially vary through time after release, but we did not expect individual responses to switch from selection to avoidance or vice-versa; thus, we did not test for time-dependent responses to roads.
Our two competing models of the habitat selection process for each individual were:
• Model T0 did not include any time-dependence in the habitat selection process (null model);
• Model T1 included time-dependent selection for herbaceous cover and no time-dependence for selection for the other predictors;
In the equations above, w(x) denotes the habitat selection function, Sx, Hx, Lx, and Rx denote percent sagebrush cover, percent herbaceous cover, slope, and distance to roads at location x, respectively, and Tx denotes days since translocation when the GPS location x was taken. Because the total number of parameters in the more complex model (T1) was 8, we only fit the models to individuals with at least 80 observed steps (using a minimum of 10 steps per estimated parameter as a rule of thumb to ensure adequate power). We ranked models for each individual based on Akaike's Information Criterion corrected for small sample sizes (AICc). We grouped individuals based on their top-ranked model: group T0 had model T0 as top-ranked (ΔAICc >2 for model T1) and group T1 had model T1 as top-ranked (ΔAICc >2 for model T0). Individuals for which the second-ranked model had ΔAICc ≤2 were not attributed to either group. For individuals in groups T0 and T1, we evaluated mean parameter estimates at the individual level under both models. We used standard errors to calculate 95% confidence intervals around mean parameter estimates using a large-sample approximation. Then, for all individuals, we calculated mean predictions of log Relative Selection Strength (log-RSS; Avgar et al., 2017) for herbaceous cover under model T1 as a function of days since translocation. Log-RSS is the natural logarithm of the ratio of the exponential habitat selection function for two sets of predictor values (Avgar et al., 2017); we chose to evaluate selection for the 3rd quartile of herbaceous cover (52%) vs. the mean value across the dataset (46%), but because our model is linear on the log-scale, these patterns would hold for any values of herbaceous cover separated by 6%.
Results
The final dataset included 26 translocated sage-grouse. Of these, 19 were brood-rearing females and seven were non-brood-rearing females. Time-dependence in habitat selection was supported for five individuals, while the absence of time-dependence (i.e., null model T0) was best supported for seven individuals; the two models were indistinguishable based on AICc for the remaining 14 individuals (Table 1; see Supplementary Material for individual model selection tables). We found no evidence of association between the occurrence of time-dependence in habitat selection and individual status (brood-rearing or non-brood-rearing; Fisher's exact test; p > 0.05). Out of seven individuals in group T0, model T0 indicated selection for herbaceous cover in four, avoidance in one, and no significant response for two; we detected no significant responses to the other three predictors (Figure 1). Out of five individuals in group T1, model T1 indicated initial selection for herbaceous cover with a shift toward avoidance for two and initial avoidance with a shift toward selection for three (Figures 1, 2); we also detected selection and avoidance of steeper slopes for one individual, respectively (Figure 1). When fitting model T0 to group T1, the sign of the response to herbaceous cover was reversed with respect to model T1 for four out of five individuals (Figure 1). When fitting model T1 to group T0, the mean parameter estimate was estimated accurately albeit with greater uncertainty (Figure 1). For individuals that exhibited time-dependent habitat selection for herbaceous cover, the switch from selection to avoidance (or vice-versa) occurred within 1–3 days after release (Figure 2).
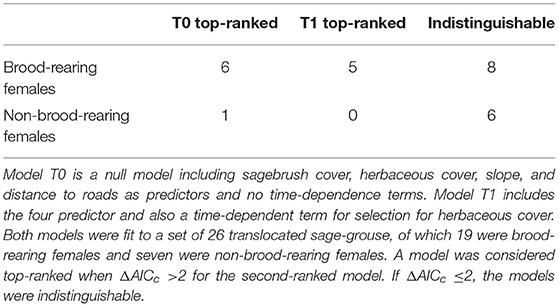
Table 1. Summary of model ranking based on AICc for translocated female sage-grouse in North Dakota in 2018–2020.
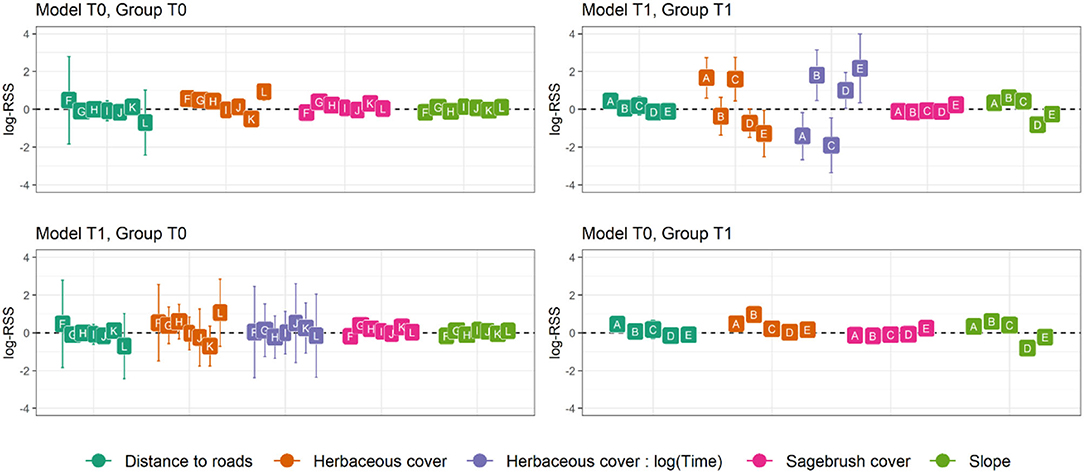
Figure 1. Mean parameter estimates and 95% confidence intervals for habitat selection of translocated sage-grouse in North Dakota, 2018–2020, in the 60 days after release. Model T0 includes no time-dependent terms, while model T1 includes time-dependent selection for herbaceous cover, a key foraging resource during summer. Both models were fitted to each individual separately and ranked based on AICc, then individuals were grouped based on their top-ranked model. Individuals in group T0 had model T0 as top-ranked (ΔAICc >2 for model T1), while individuals in group T1 had model T1 as top-ranked (ΔAICc >2 for model T0). Parameter values express log-Relative Selection Strength (sensu Avgar et al., 2017), i.e., the selection coefficient for a 1-unit increase in the covariate value.
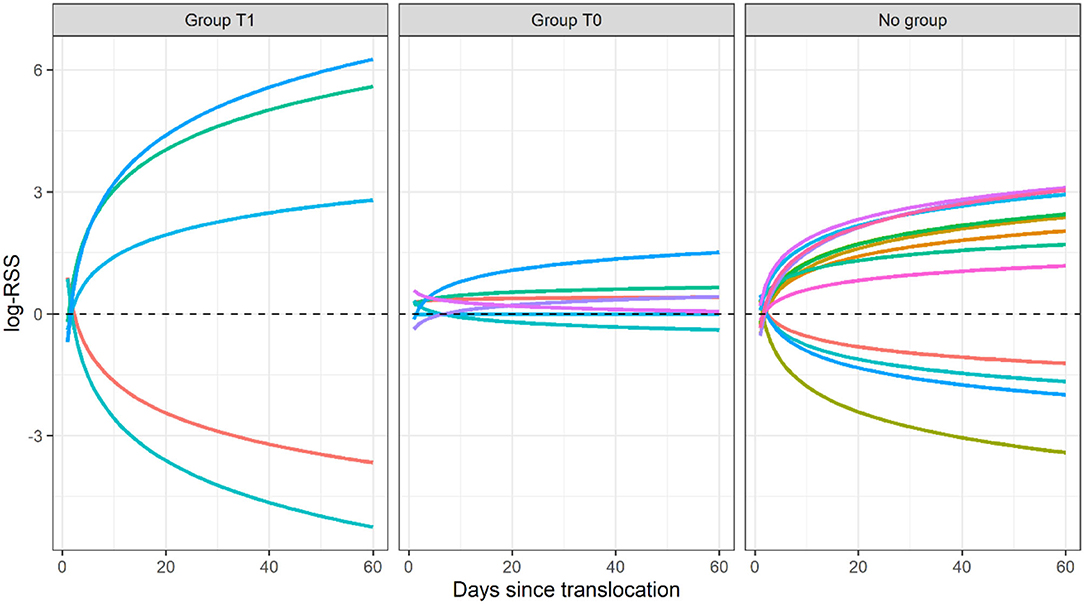
Figure 2. Predictions of log-Relative Selection Strength from model T1 in response to herbaceous cover as a log-function of time (days since translocation) in translocated female sage-grouse in North Dakota, 2018–2020, in the 60 days after release. Predictions were evaluated using individual-level parameter estimates. Group T1 included individuals for which the model with time-dependent selection for herbaceous cover was top-ranked (ΔAICc >2 for model T0), group T0 included individuals for which the model without time-dependence was top-ranked (ΔAICc >2 for model T1), and the “no group” label denotes individuals for which the two models were indistinguishable (ΔAICc ≤ 2 for the second-ranked model).
Discussion
Using data for translocated greater sage-grouse, we showed that temporal dynamics of post-release habitat selection may manifest in some individuals but not in others, and that not accounting for time-dependence may hinder correct interpretation of habitat selection patterns. These findings showcase the need to consider both temporal dynamics and individual variability in habitat selection when conducting post-release monitoring of translocated animals.
Five translocated sage-grouse (all brood-rearing females) exhibited a temporally varying response to herbaceous cover within the first 60 days post-release. For two of these individuals, selection for higher herbaceous cover was strongest immediately after release and gradually decreased through time, switching to avoidance (Figure 2). The remaining three individuals initially exhibited avoidance of higher herbaceous cover and then switched to selection (Figure 2). A switch from avoidance to selection for herbaceous cover during post-release behavioral modification is what we expected based on theory: translocated animals should initially prioritize exploration of their new surroundings and move through the landscape regardless of the distribution of key resources, and they should gradually shift to resource exploitation once acclimated (Berger-Tal and Saltz, 2014). The trend we observed is also compatible with natal habitat preference induction, where translocated individuals select for habitat that is most similar to their natal habitat before they gradually adjust to the new conditions (Davis and Stamps, 2004). Indeed, sage-grouse habitat at the source population consisted of contiguous sagebrush steppe, where sagebrush was the dominant vegetation type and herbaceous cover was sparse; sage-grouse were then released in a grass-dominated system. Some translocated individuals may have responded to this change by initially avoiding unfamiliar features and then gradually selecting for higher herbaceous cover as they became familiar with the release area. Individuals who initially selected and then started avoiding higher herbaceous cover may have done so because their brood fledged or died within the 60 days after release, which relaxed their dependence on herbaceous cover for foraging.
Seven sage-grouse (six brood-rearing and one non-brood-rearing female) did not exhibit time-dependent habitat selection for herbaceous cover; most of them selected for herbaceous cover, one avoided it, but their behavior did not change through time (Figures 1, 2). Although our sample size did not give us statistical power to make definitive claims, we did not find a link between temporal dynamics of habitat selection exhibited by translocated individuals and their status (brood-rearing or non-brood-rearing). Rather, the individual variation we observed in temporal dynamics of post-release habitat selection may be a result of intrinsic characteristics of each individual (e.g., personality; Carere and Eens, 2005).
Our results demonstrate that model formulation affects inference, reinforcing that failure to consider temporal dynamics and individual variation may substantially change the conclusions drawn about habitat selection in translocated populations. When using a model without time-dependence terms to predict habitat selection for individuals that exhibit time-dependence, our estimates of steady-state habitat selection were altered. In some cases, opposite trends canceled out – e.g., initial avoidance neutralized steady-state selection, resulting in a non-significant pattern (Figure 1). In others, the initial response outweighed the steady-state behavior resulting in a reversed pattern (Figure 1). These findings demonstrate that failing to account for time-dependent habitat selection in individuals that exhibit it is likely to lead us to inaccurate conclusions. By contrast, including time-dependence terms still resulted in accurate estimates for steady-state selection for herbaceous cover in individuals that did not exhibit temporal dynamics, albeit with greater uncertainty (Figure 1). All in all, these results show that the inclusion of time-dependence terms, at least during data exploration, allows for detection of temporal dynamics where they occur without compromising accuracy in estimating steady-state habitat selection.
The time-dependent model was indistinguishable from the null for the remaining 14 individuals based on AICc. This is likely because the response to herbaceous cover in these individuals was too weak to outweigh the penalty from an additional model parameter. Nonetheless, our results show that fitting a model that includes time-dependent terms to individuals that do not exhibit time-dependent habitat selection increases uncertainty but does not affect the accuracy of steady-state estimates. Thus, one possible approach is to begin data exploration by fitting time-dependence models to all individuals and then further refine individual parameter estimates by removing unnecessary time-dependence terms on an individual basis.
Responses to other predictors in our models were weak, with a few exceptions: one individual selected to be near roads, four individuals selected for high sagebrush cover, one avoided steeper slopes and three selected for them (Figure 1). One possible explanation for selection for roads is that linear features may provide conspicuous landmarks for some individuals and help with orientation in an unknown landscape. Landmarks play an important role in spatial orientation and learning in vertebrates (Bingman and Cheng, 2005; Lewis et al., 2020), and previous studies have shown that, in some bird species, translocated individuals are able to use anthropogenic landmarks (including roads, e.g., Bélisle and St. Clair, 2002) to navigate across the landscape. Selection for high sagebrush cover and avoidance of steep slopes are typical habitat selection responses observed in sage-grouse across their range (Connelly et al., 2000, 2011). Selection for steeper slopes in some individuals was surprising, although less so when contextualized within the study area, where even the steepest slopes are only moderately steep. The fact that, overall, responses of translocated sage-grouse to key predictors were weak could be attributed to several reasons. One possible explanation is that some translocated sage-grouse were unable to behave adaptively in an environment so different from their natal habitat, at least during the first 2 months after release, and thus behaved similarly to random walkers using resources in close proportion to their availability. Another possible reason for the lack of strong responses, which is not necessarily in contradiction with the first one, is that habitat selection in translocated sage-grouse may be stronger at coarser orders of selection, but at finer scales, once settled within a general area, sage-grouse behavior becomes closer to a random walk. Finally, the release environment was rather homogeneous, and the lack of landscape heterogeneity may have resulted in weak responses.
In our study system, sage-grouse exhibited weak to moderate habitat selection responses, but accounting for temporal dynamics may be even more important in highly heterogeneous landscapes. When evaluating time-dependent habitat selection, it is beneficial to decide a priori on ecologically meaningful model formulations based on existing knowledge of the study species and system, especially when data availability constraints limit the complexity of the models. In this study, the size of our individual datasets did not allow us to fit time-dependent terms for multiple predictors at a time. Higher-frequency, temporally regular location data should allow researchers to include several time-dependent terms within one model and test alternative hypotheses regarding time-dependent habitat selection for multiple resources. Potentially, individuals might display time-dependent selection for different predictors and reveal new, intriguing patterns of individual heterogeneity.
Temporal dynamics in habitat selection provide insight into how long it takes for translocated individuals to reach steady-state behavior in the release area. Results from this study illustrate that (1) the time-to-steady-state may differ between individuals, (2) the acclimation phase may be shorter (or longer) than expected a priori, and (3) some individuals may not display any time-dependent responses. In principle, the time it takes for individuals to reach steady-state habitat selection may also differ between predictors. Often, researchers deal with post-release behavioral modification by discarding data from the first few days or weeks after release. Explicitly modeling temporal dynamics of individual behavior may inform the choice of cut-off to use when discarding data or entirely remove the need for it, because the model structure already accommodates the potential for behavioral adjustments.
Time-dependence in habitat selection could be implemented using different formulations. A shortcoming of the formulation we used is that the natural logarithm of time since release constrains the shape of the temporal-dependence curve, making it range from 0 on the first day post-release to 1 at the maximum number of days post-release (in our case, 60). At the same time, a benefit of this formulation is that our temporal dependence curve is still a linear curve that can be fit using standard software. More complex, non-linear formulation of time-dependence curves would allow for explicit estimation of individual time-dependence parameters over time-series of varying lengths. However, this implementation would require custom non-linear models, which are sometimes beyond the reach of non-statisticians; to overcome this barrier, some quantitative biologists may prefer a Bayesian solution. Custom form models would also be data-hungry due to their non-linear nature.
Temporal dynamics and individual variation in habitat selection are often viewed as a nuisance to remove; instead, we argue that they should be treated as processes on which to make inference. Our results showed that including time-dependent responses and evaluating habitat selection at the individual level may provide a detailed picture of how translocated populations respond to their new environment. Our study indicates benefits of approaches that explicitly incorporate temporal dynamics and accommodate individual variation when examining post-release habitat selection of translocated animals. Accounting for individual differences in habitat selection and post-release behavioral modification should ultimately improve population-level inference as well, by ensuring, first, that population-level analyses focus on steady-state behavior only, and second, that opposite patterns of selection between groups of individuals do not cancel each other out when analyzed collectively. Formal tests of a priori hypotheses on post-release behavior via appropriately designed experimental translocation have the potential to provide valuable information for adaptive management (Armstrong and Seddon, 2008). These benefits warrant high interest in future research aimed to test hypotheses on drivers of individual heterogeneity in post-release behavior.
Data Availability Statement
The data are available on Dryad, https://doi.org/10.5061/dryad.44j0zpcf5. The code is available on GitHub, https://github.com/picardis/picardi-et-al_2021_sage-grouse_frontiers-in-conservation.
Ethics Statement
The animal study was reviewed and approved by Utah State University Institutional Animal Care and Use Committee.
Author Contributions
SP and NR conceived the idea. SP, NR, and BS conducted the analysis. DD and PC supervised the project and provided the data. SM curated the field data. SP wrote the initial manuscript draft. All authors contributed to manuscript editing and revision.
Funding
This research was primarily funded by the North Dakota Game and Fish Department. The Wyoming Game and Fish Department also contributed funding.
Conflict of Interest
The authors declare that the research was conducted in the absence of any commercial or financial relationships that could be construed as a potential conflict of interest.
Publisher's Note
All claims expressed in this article are solely those of the authors and do not necessarily represent those of their affiliated organizations, or those of the publisher, the editors and the reviewers. Any product that may be evaluated in this article, or claim that may be made by its manufacturer, is not guaranteed or endorsed by the publisher.
Acknowledgments
We thank numerous technicians and volunteers for field data collection. We thank the U.S. Geological Survey Ecosystems Mission Area, Wyoming Game and Fish Dept., North Dakota Game and Fish Dept., the Jack Berryman Institute, Utah State University Cooperative Extension, and the Wildlife Federation for their support on this project. Any use of trade, firm, or product names is for descriptive purposes only and does not imply endorsement by the U.S. Government.
Supplementary Material
The Supplementary Material for this article can be found online at: https://www.frontiersin.org/articles/10.3389/fcosc.2021.703906/full#supplementary-material
References
Armstrong, D. P., and Seddon, P. J. (2008). Directions in reintroduction biology. Trends Ecol. Evol. 23, 20–25. doi: 10.1016/j.tree.2007.10.003
Avgar, T., Baker, J. A., Brown, G. S., Hagens, J. S., Kittle, A. M., Mallon, E. E., et al. (2015). Space-use behaviour of woodland caribou based on a cognitive movement model. J. Anim. Ecol. 84, 1059–1070. doi: 10.1111/1365-2656.12357
Avgar, T., Lele, S. R., Keim, J. L., and Boyce, M. S. (2017). Relative selection strength: quantifying effect size in habitat- and step-selection inference. Ecol. Evol. 7, 5322–5330. doi: 10.1002/ece3.3122
Avgar, T., Potts, J. R., Lewis, M. A., and Boyce, M. S. (2016). Integrated step selection analysis: bridging the gap between resource selection and animal movement. Methods Ecol. Evol. 7, 619–630. doi: 10.1111/2041-210X.12528
Baxter, R. J., Flinders, J. T., and Mitchell, D. L. (2008). Survival, movements, and reproduction of translocated greater sage-grouse in Strawberry Valley, Utah. J. Wildl. Manage. 72, 179–186. doi: 10.2193/2006-402
Baxter, R. J., Larsen, R. T., and Flinders, J. T. (2013). Survival of resident and translocated greater sage-grouse in Strawberry Valley, Utah: a 13-year study. J. Wildl. Manage. 77, 802–811. doi: 10.1002/jwmg.520
Bélisle, M., and St. Clair, C. C. (2002). Cumulative effects of barriers on the movements of forest birds. Conserv. Ecol. 5. Available online at: https://www.jstor.org/stable/26271813 (accessed April 21, 2021).
Berger-Tal, O., Blumstein, D. T., and Swaisgood, R. R. (2020). Conservation translocations: a review of common difficulties and promising directions. Anim. Conserv. 23, 121–131. doi: 10.1111/acv.12534
Berger-Tal, O., Nathan, J., Meron, E., and Saltz, D. (2014). The exploration-exploitation dilemma: a multidisciplinary framework. PLoS ONE 9:e95693. doi: 10.1371/journal.pone.0095693
Berger-Tal, O., and Saltz, D. (2014). Using the movement patterns of reintroduced animals to improve reintroduction success. Curr. Zool. 60, 515–526. doi: 10.1093/czoolo/60.4.515
Bingman, V. P., and Cheng, K. (2005). Mechanisms of animal global navigation: comparative perspectives and enduring challenges. Ethol. Ecol. Evol. 17:9522584. doi: 10.1080/08927014.2005.9522584
Bonnot, N., Verheyden, H., Blanchard, P., Cote, J., Debeffe, L., Cargnelutti, B., et al. (2015). Interindividual variability in habitat use: evidence for a risk management syndrome in roe deer? Behav. Ecol. 26, 105–114. doi: 10.1093/beheco/aru169
Bubac, C. M., Johnson, A. C., Fox, J. A., and Cullingham, C. I. (2019). Conservation translocations and post-release monitoring: identifying trends in failures, biases, and challenges from around the world. Biol. Conserv. 238:108239. doi: 10.1016/j.biocon.2019.108239
Carere, C., and Eens, M. (2005). Unravelling animal personalities: how and why individuals consistently differ. Behaviour 142, 1149–1157. doi: 10.1163/156853905774539436
Coates, P. S., Prochazka, B. G., O'Donnell, M. S., Aldridge, C. L., Edmunds, D. R., Monroe, A. P., et al. (2021). Range-wide greater sage-grouse hierarchical monitoring framework—implications for defining population boundaries, trend estimation, and a targeted annual warning system. U. S. Geological Survey Open-File Report 2020–1154, 243. doi: 10.3133/ofr20201154
Connelly, J. W., Rinkes, E. T., and Braun, C. E. (2011). Characteristics of greater sage-grouse habitats: a landscape species at micro and macro scales. Stud. Avian Biol. 38, 69–83. doi: 10.1525/california/9780520267114.003.0005
Connelly, J. W., Schroeder, M. A., Sands, A. R., and Braun, C. E. (2000). Guidelines to manage sage grouse populations and their habitats. Wildlife Soc. Bull. 28, 967–985.
Cote, J., Clobert, J., Brodin, T., Fogarty, S., and Sih, A. (2010). Personality-dependent dispersal: characterization, ontogeny and consequences for spatially structured populations. Philos. Trans. Royal Soc. B Biol. Sci. 365, 4065–4076. doi: 10.1098/rstb.2010.0176
Davis, J. M., and Stamps, J. A. (2004). The effect of natal experience on habitat preferences. Trends Ecol. Evol. 19, 411–416. doi: 10.1016/j.tree.2004.04.006
de Azevedo, C. S., and Young, R. J. (2021). Animal personality and conservation: basics for inspiring new research. Animals 11:1019. doi: 10.3390/ani11041019
Dinkins, J. B., Conover, M. R., Kirol, C. P., Beck, J. L., and Frey, S. N. (2014). Greater Sage-Grouse (Centrocercus urophasianus) select habitat based on avian predators, landscape composition, and anthropogenic features. Condor 116, 629–642. doi: 10.1650/CONDOR-13-163.1
Duvuvuei, O. V., Gruber-Hadden, N. W., Messmer, T. A., Guttery, M. R., and Maxfield, B. D. (2017). Contribution of translocated greater sage-grouse to population vital rates. J. Wildl. Manage. 81, 1033–1041. doi: 10.1002/jwmg.21264
Garton, E. O., Connelly, J. W., Horne, J. S., Hagen, C. A., Moser, A., and Schroeder, M. A. (2011). Greater sage-grouse population dynamics and probability of persistence. Stud. Avian Biol. 38, 293–381. doi: 10.1525/california/9780520267114.003.0016
Gehr, B., Bonnot, N. C., Heurich, M., Cagnacci, F., Ciuti, S., Hewison, A. J. M., et al. (2020). Stay home, stay safe—site familiarity reduces predation risk in a large herbivore in two contrasting study sites. J. Anim. Ecol. 89, 1329–1339. doi: 10.1111/1365-2656.13202
Germano, J. M., Nafus, M. G., Perry, J. A., Hall, D. B., and Swaisgood, R. R. (2017). Predicting translocation outcomes with personality for desert tortoises. Behav. Ecol. 28, 1075–1084. doi: 10.1093/beheco/arx064
Kaczor, N. W., Herman-Brunson, K. M., Jensen, K. C., Rumble, M. A., Klaver, R. W., and Swanson, C. C. (2011). Chapter Twelve. Resource Selection During Brood-Rearing by Greater Sage-Grouse. University of California Press. Available online at: https://www.degruyter.com/document/doi/10.1525/9780520950573-014/html (accessed August 17, 2021).
Lazenby, K. D., Coates, P. S., O'Neil, S. T., Kohl, M. T., and Dahlgren, D. K. (2021). Nesting, brood rearing, and summer habitat selection by translocated greater sage-grouse in North Dakota, USA. Ecol. Evol. 11, 2741–2760. doi: 10.1002/ece3.7228
Letty, J., Marchandeau, S., and Aubineau, J. (2007). Problems encountered by individuals in animal translocations: lessons from field studies. Écoscience 14, 420–431. doi: 10.2980/1195-6860(2007)14[420:PEBIIA]2.0.CO;2
Lewis, M., Fagan, W., Auger-Methe, M., Frair, J., Fryxell, J., Gros, C., et al. (2020). Learning and animal movement. Front. Ecol. Evol. 9:681704. doi: 10.3389/fevo.2021.681704
May, T. M., Page, M. J., and Fleming, P. A. (2016). Predicting survivors: animal temperament and translocation. Behav. Ecol. 27, 969–977. doi: 10.1093/beheco/arv242
Merkle, J. A., Fortin, D., and Morales, J. M. (2014). A memory-based foraging tactic reveals an adaptive mechanism for restricted space use. Ecol. Lett. 17, 924–931. doi: 10.1111/ele.12294
Merrick, M. J., and Koprowski, J. L. (2017). Should we consider individual behavior differences in applied wildlife conservation studies? Biol. Conserv. 209, 34–44. doi: 10.1016/j.biocon.2017.01.021
Meyerpeter, M. B., Lazenby, K. D., Coates, P. S., Ricca, M. A., Mathews, S. R., Gardner, S. C., et al. (2021). Field methods for translocating female greater sage-grouse (Centrocercus urophasianus) with their broods. Wildlife Soc. Bull. doi: 10.1002/wsb.1199
Mondal, K., Bhattacharjee, S., Gupta, S., Sankar, K., and Qureshi, Q. (2013). Home range and resource selection of “problem” leopards trans-located to forested habitat. Curr. Sci. 105, 338–345.
Montagne, J.-P. (2016). The effect of personality on movement and survival following translocation of california ground squirrels (Otospermophilus beecheyi). M.Sc., Biology Thesis, San Diego State University, California, USA.
Nathan, R., Getz, W. M., Revilla, E., Holyoak, M., Kadmon, R., Saltz, D., et al. (2008). A movement ecology paradigm for unifying organismal movement research. PNAS 105, 19052–19059. doi: 10.1073/pnas.0800375105
Osborne, P. E., and Seddon, P. J. (2012). “Selecting suitable habitats for reintroductions: variation, change and the role of species distribution modelling,” in Reintroduction Biology: Integrating Science and Management, eds J. G. Ewen, D. P. Armstrong, K. A. Parker, and P. J. Seddon (Oxford: Blackwell Publishing Ltd), 73–104. doi: 10.1002/9781444355833.ch3
Parker, K., Ewen, J., and Seddon, P. (2013). Post-release monitoring of bird translocations. Notornis 60, 85–92.
Ranc, N., Cagnacci, F., and Moorcroft, P. R. (2020a). Memory drives the formation of animal home ranges: evidence from a reintroduction. bioRxiv [Preprint]. doi: 10.1101/2020.07.30.229880
Ranc, N., Moorcroft, P. R., Hansen, K. W., Ossi, F., Sforna, T., Ferraro, E., et al. (2020b). Preference and familiarity mediate spatial responses of a large herbivore to experimental manipulation of resource availability. Sci. Rep. 10:11946. doi: 10.1038/s41598-020-68046-7
Ranc, N., Moorcroft, P. R., Ossi, F., and Cagnacci, F. (2021). Experimental evidence of memory-based foraging decisions in a large wild mammal. PNAS 118:e2014856118. doi: 10.1073/pnas.2014856118
Reese, K. P., and Connelly, J. W. (1997). Translocations of sage grouse Centrocercus urophasianus in North America. wbio 3, 235–241. doi: 10.2981/wlb.1997.029
Richardson, K. M., Ewen, J. G., Brekke, P., Doerr, L. R., Parker, K. A., and Armstrong, D. P. (2017). Behaviour during handling predicts male natal dispersal distances in an establishing reintroduced hihi (Notiomystis cincta) population. Anim. Conserv. 20, 135–143. doi: 10.1111/acv.12296
Roever, C. L., Beyer, H. L., Chase, M. J., and Aarde, R. J. van (2014). The pitfalls of ignoring behaviour when quantifying habitat selection. Divers. Distribut. 20, 322–333. doi: 10.1111/ddi.12164
Swanson, C. C., Rumble, M. A., Grovenburg, T. W., Kaczor, N. W., Klaver, R. W., Herman-Brunson, K. M., et al. (2013). Greater sage-grouse winter habitat use on the eastern edge of their range. J. Wildl. Manage. 77, 486–494. doi: 10.1002/jwmg.484
Wakkinen, W. L., Reese, K. P., and Connelly, J. W. (1992). Sage Grouse nest locations in relation to leks. J. Wildl. Manage. 56, 381–383. doi: 10.2307/3808838
Werdel, T. J., Jenks, J. A., Kanta, J. T., Lehman, C. P., and Frink, T. J. (2021). Space use and movement patterns of translocated bighorn sheep. Mamm. Biol. 101, 329–344. doi: 10.1007/s42991-021-00107-4
Keywords: resource selection, post-release behavioral modification, individual behavior, individual heterogeneity, translocation, greater sage-grouse
Citation: Picardi S, Ranc N, Smith BJ, Coates PS, Mathews SR and Dahlgren DK (2021) Individual Variation in Temporal Dynamics of Post-release Habitat Selection. Front. Conserv. Sci. 2:703906. doi: 10.3389/fcosc.2021.703906
Received: 01 May 2021; Accepted: 06 September 2021;
Published: 30 September 2021.
Edited by:
David Saltz, Ben-Gurion University of the Negev, IsraelReviewed by:
Wenke Bai, China West Normal University, ChinaTessa L. Behnke, University of Nevada, Reno, United States
Copyright © 2021 Picardi, Ranc, Smith, Coates, Mathews and Dahlgren. This is an open-access article distributed under the terms of the Creative Commons Attribution License (CC BY). The use, distribution or reproduction in other forums is permitted, provided the original author(s) and the copyright owner(s) are credited and that the original publication in this journal is cited, in accordance with accepted academic practice. No use, distribution or reproduction is permitted which does not comply with these terms.
*Correspondence: Simona Picardi, c2ltb25hLnBpY2FyZGlAdXN1LmVkdQ==