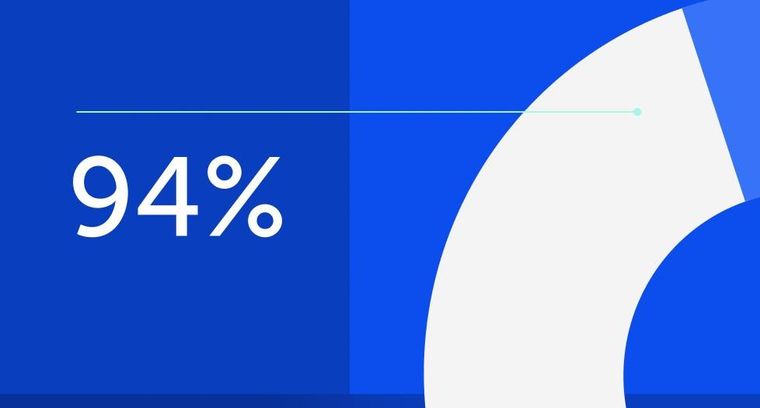
94% of researchers rate our articles as excellent or good
Learn more about the work of our research integrity team to safeguard the quality of each article we publish.
Find out more
PERSPECTIVE article
Front. Clim., 04 April 2025
Sec. Carbon Dioxide Removal
Volume 7 - 2025 | https://doi.org/10.3389/fclim.2025.1582786
As climate mitigation efforts lag, dependence on anthropogenic CO2 removal increases. Enhanced rock weathering (ERW) is a rapidly growing CO2 removal approach. In terrestrial ERW, crushed rocks are spread on land where they react with CO2 and water, forming dissolved inorganic carbon (DIC) and alkalinity. For long-term sequestration, these products must travel through rivers to oceans, where carbon remains stored for over 10,000 years. Carbon and alkalinity can be lost during river transport, reducing ERW efficacy. However, the ability of biological processes, such as aquatic photosynthesis, to affect the fate of DIC and alkalinity within rivers has been overlooked. Our analysis indicates that within a stream-order segment, aquatic photosynthesis uptakes 1%–30% of DIC delivered by flow for most locations. The effect of this uptake on ERW efficacy, however, depends on the cell-membrane transport mechanism and the fate of photosynthetic carbon. Different pathways can decrease just DIC, DIC and alkalinity, or just alkalinity, and the relative importance of each is unknown. Further, data show that expected river chemistry changes from ERW may stimulate photosynthesis, amplifying the importance of these biological processes. We argue that estimating ERW’s carbon sequestration potential requires consideration and better understanding of biological processes in rivers.
Carbon dioxide removal (CDR) has become an increasingly prevalent tool in IPCC modeled mitigation pathways that limit global warming to 1.5°C or 2°C relative to the preindustrial period (IPCC, 2022). There is still vast uncertainty and debate around the need, cost, efficacy, scalability, and ethics of using CDR to meet climate objectives (Anderson and Peters, 2016; Rockström et al., 2016; Hollnaicher, 2022). Nonetheless, the likelihood of CDR dependence grows with the ongoing failure of pledged and actualized emissions reductions to meet what is needed to limit warming (Boehm et al., 2022). In response, the CDR industry is growing rapidly as it prepares to meet future demand (Smith et al., 2024; CDR.fyi, 2025).
In the durable CDR landscape—i.e., carbon removal for >1,000 years—enhanced rock weathering (ERW) is an emerging and rapidly growing approach. Currently, 18 ERW companies exist and ~19% of the total tons of carbon sold in the durable carbon market is attributed to ERW (CDR.fyi, 2025). However, the CDR potential of ERW at scale is uncertain (Buma et al., 2024). Given the proliferating CDR landscape and increasing use of ERW as a carbon offset tool, it is crucial to fully understand the approach’s efficacy in order to avoid emitting more carbon than is actually sequestered.
In ERW, silicate or carbonate rocks are crushed and spread on land—usually agricultural fields—where the minerals react with CO2 and water to form bicarbonate (HCO3−) and cations. For ERW to successfully and durably remove CO2, the weathering products must reach a long-term reservoir. For most deployments, that reservoir is the ocean where the residence time of carbon is long enough [>10,000 years (Middelburg et al., 2020)] to offset fossil emissions, which stay in the atmosphere for thousands of years (Archer et al., 2009).
The journey from fields, where weathering occurs, to the ocean, where sequestration occurs, involves streams, rivers, lakes, and reservoirs. In these freshwater systems, many processes can pull or release weathering products from water, altering the amount delivered to the ocean. Thus far, the ERW community has only considered the ability of abiotic processes—namely carbonate precipitation (Knapp and Tipper, 2022; Zhang et al., 2022; Harrington et al., 2023) and CO2 degassing (Zhang et al., 2025)—to facilitate the loss of ERW products within rivers.
We contend that biological processes—namely photosynthesis—by submerged plants and algae represent an important but overlooked loss pathway for ERW products in freshwater systems. Quantifying the impact of biological processes on these products is necessary to correctly estimate net CDR associated with ERW.
Here we briefly review ERW as a CDR technology, use existing data from river systems to explore the importance of aquatic photosynthesis on carbon budgets, and consider how large-scale deployment of ERW could feedback and alter biological processes.
Carbonic-acid weathering of carbonate and silicate minerals is one of the natural processes that controls CO2 concentrations in the atmosphere. It is estimated that, currently, natural rock weathering on land removes ~1 GtCO2 yr.−1 from the atmosphere (Ciais et al., 2014). The goal of ERW is to augment and speed up this natural weathering process by crushing and spreading rocks. Assuming the weathered products enter the ocean, both reactions hold potential to remove CO2 from the atmosphere on timescales of interest to humans (i.e., hundreds to thousands of years) (Middelburg et al., 2020; Zhang et al., 2022).
Ultimately, carbonic-acid weathering converts CO2 gas into dissolved inorganic carbon (DIC) and generates alkalinity. We distinguish between DIC and alkalinity because, while the two are tightly coupled, there are processes that can affect one while not influencing the other (Biedunkova and Kuznietsov, 2024). Both are important for CDR. ERW-generated DIC delivered to the deep ocean is the metric of interest for sequestration. Alkalinity affects DIC when waters equilibrate with CO2 in the atmosphere, but full equilibration does not always occur (Raymond et al., 2013; Campeau et al., 2017; Zhou et al., 2025).
There have been multiple theoretical assessments of the carbon-sequestration potential of ERW on agricultural fields (Kantola et al., 2017; Beerling et al., 2020; Kantzas et al., 2022; Baek et al., 2023) as well as analyses of potential life-cycle carbon budgets associated with mining, transporting, crushing, and spreading rock (Moosdorf et al., 2014; Zhang et al., 2023; Li et al., 2024). These analyses have concluded that ERW on agricultural fields has potential to sequester a meaningful amount of carbon (0.5 to 3 Gt CO2 yr.−1). However, many of these ERW CDR estimates did not account for loss of carbon and/or alkalinity in rivers as ERW products travel from fields to ocean. Estimates that included river losses only considered abiotic processes or were process-agnostic. The impact of river biology was neglected.
Measurements of total DIC in rivers represent a snapshot of dynamic bio-physical and chemical interactions that are constrained by the buffering capacity of the aquatic environment (Campeau et al., 2017). Upon entering open waters, DIC can be lost to the atmosphere as CO2 (Butman and Raymond, 2011), incorporated into submerged aquatic biomass through the uptake of either CO2(aq) or HCO3− during photosynthesis (Hotchkiss et al., 2015), remain dissolved and exported downstream, or removed from solution through carbonate precipitation (Tobias and Böhlke, 2011) (Figures 1A–C).
Figure 1. Riverine processes affecting weathering products. Processes are numbered for reference. (A) Processes that affect dissolved inorganic carbon concentrations, but not alkalinity: 1. Equilibrium degassing, 2. Respiration, and 3a. Photosynthesis where either dissolved CO2 is used or bicarbonate use is coupled with H+ transport across the cell membrane. (B) Processes that affect both dissolved inorganic carbon concentrations and alkalinity: 4. Acid inputs that drive degassing, 5. Calcite precipitation, and 3b. Photosynthesis where bicarbonate uptake occurs via anion exchange. (C) Process that do not affect dissolved inorganic carbon concentrations but do affect alkalinity: 6. Uptake of nitrogen and phosphorous species that contribute to alkalinity (i.e., N-alkalinity and P-alkalinity)—phosphorous ions are transported across the cell membrane using H+ transport, as illustrated in panel A—and 7. Cation uptake by aquatic plants and algae that is coupled with H+ transport across the cell membrane.
The relative importance of each loss pathway is a function of the physical environment (light, temperature, gas exchange velocity, channel slope, and stream flow), the biological composition of aquatic and riparian primary producers that alter aqueous chemical conditions (pH, alkalinity), and the inputs of geochemically complex groundwater. Perhaps the strongest control on the fate and transport of DIC is hydrology. Water velocity controls the residence time of carbon (Rehn et al., 2023) and the rate of CO2 degassing (Hall and Ulseth, 2020). Seasonal changes in run-off can drive extreme shifts in the magnitude of CO2 emissions across stream networks (Conroy et al., 2023).
Here we recognize that rivers drive toward equilibrium but often never reach it (Raymond et al., 2013; Campeau et al., 2017), and are often not at steady state, particularly smaller tributaries (Durighetto et al., 2020; Brinkerhoff et al., 2024). Thus, our discussion considers how riverine biological processes can affect DIC and alkalinity within rivers, but it does not presume how these biologically driven changes in turn affect other processes occurring within the river, such as pH shifts, degassing, and carbonate precipitation.
Aquatic photosynthesis requires uptake of either dissolved CO2 or bicarbonate—submerged plants and algae cannot access atmospheric CO2 for photosynthesis. The bicarbonate carbon fixation pathway dominates over the CO2(aq) fixation pathway in river systems with high bicarbonate concentrations (Aho et al., 2021; Kaijser et al., 2021). In fact, the proportion of HCO3− vs. CO2(aq) affects aquatic plant assemblages. Systems that experienced increases in available HCO3− through agricultural liming shifted species composition to those most able to actively take up HCO3− for photosynthesis (Brandrud, 2002; Iversen et al., 2019).
Uptake of charged ions, such as bicarbonate, by plants requires transport of other ions across cell walls to maintain internal pH balance and ionic equilibrium. Figure 1 illustrates the various HCO3− uptake pathways that are known to exist for aquatic macroalgae and seagrass (Poschenrieder et al., 2018). Membrane transport of bicarbonate by vegetation is not well documented (Poschenrieder et al., 2018). One set of known uptake pathways involves active transport of H+ out of the cell followed by passive outward transport of OH− (Figure 1A). The H+ then is either co-transported back into the cell with HCO3− or it reacts with HCO3− and generates CO2(aq) that then diffuses through the cell membrane. Both carbon uptake pathways remove DIC from the river water but have no impact on alkalinity.
The other set of known carbon uptake pathways involves anion exchange across the cell membrane (Figure 1B). A chloride ion exits the cell while either a single bicarbonate or two bicarbonates and a sodium ion enter the cell. This uptake pathway reduces DIC and alkalinity in the surrounding river water.
Aquatic plant productivity has additional indirect impacts on river DIC and alkalinity. First, benthic algae promote calcite precipitation, which decreases both DIC and alkalinity (Figure 1B); the algal mats provide a surface for crystal growth while mat photosynthesis increases pH and the saturation index of calcite in local microenviornments (Hoffer-French and Herman, 1989; Hayashi et al., 2012). Second, associated uptake of nitrogen (NH3) and phosphorous (H3PO4, H2PO4−, HPO42−, PO43−) by plants can affect the non-carbonate components of alkalinity. Finally, in addition to nitrogen and phosphorous, plants require cations and uptake of these can be coupled with the release of H+ out of the cell (Babourina and Rengel, 2010) (Figure 1C). If H+ is released, it reduces alkalinity in the surrounding water. However, cation uptake is not always coupled with H+ release. Sometimes, uptake of a given cation, like Ca2+, is coupled with the release of another non-acidic cation, like Na+ (Babourina and Rengel, 2010), which will not alter alkalinity.
Uptake pathways used by aquatic vegetation, as illustrated by Figure 1, strongly determine the impact plant productivity has on DIC and alkalinity in rivers. However, it is extremely difficult to discern the actual membrane transport pathways used in a field setting; detailed, cell-level investigations are most likely required. In the context of ERW, a conservative approach is to assume that all photosynthetic activity reduces both DIC and alkalinity (i.e., plants are using bicarbonate uptake via anion exchange) and all cation uptake reduces alkalinity (i.e., plants take up cations using H+ exchange) in rivers.
Recent advances in sensor technology and modeling approaches have allowed scientists to estimate river gross primary production (GPP) across hundreds of rivers (Appling et al., 2018). We harnessed this dataset along with USGS stream chemistry data (US Geological Survey, 2016) to determine the magnitude of plant productivity and to put its influence in context.
The two datasets shared at least three overlapping time points at 70 USGS gaging stations; the median number of overlapping points was 16. A majority of the stations overlapped with agricultural areas (Supplementary Figure S1) and had balanced carbonate systems (i.e., DIC-to-alkalinity ratios near 1) (Supplementary Figure S2). The combined dataset included 1,600 datapoints spanning from 2007 to 2016. The GPP dataset reports GPP in terms of O2 concentrations. Although photosynthetic quotients have been shown to vary between 0.8 and 1.2 (Trentman et al., 2023), here we assumed a 1:1 molar ratio between CO2 and O2.
We used a stream-order framework (Figure 2B) to compare the rate of carbon transport to that of photosynthesis. We harnessed the power-law relationship between average stream-order length and median discharge (Downing et al., 2012; McManamay and DeRolph, 2019) (Figure 2A) to calculate a stream-order length for each gage location (Figure 2D). With this length set, we calculated a stream-order photosynthesis fraction for each time point. The stream-order photosynthesis fraction was defined as the ratio between the amount of carbon removed by photosynthesis within the stream-order section to the amount of carbon delivered to the stream-order section by water flow (Figure 2C). Resulting fractions are plotted in Figures 2E,F.
Figure 2. Magnitude of aquatic photosynthesis quantified using a stream-order mass-balance framework. (A) Power-law relationship between average stream-order length and stream-order discharge based on published literature (Downing et al., 2012; McManamay and DeRolph, 2019). (B) Example stream-order network. (C) Mass balance calculation producing stream-order photosynthesis fraction (f), which is the ratio between the amount of carbon removed by photosynthesis within the stream-order section (Mp) to the amount of carbon delivered to the stream-order section by water flow (Min). Mp was calculated as the photosynthesis rate or GPP (photo) x river width (W) x stream order length (L). Min was calculated as discharge (Q) × DIC concentration. (D) Histogram of stream-order lengths calculated for the dataset using median discharge rates and the relationship in panel A. (E) Resulting stream-order photosynthesis fraction versus photosynthesis rate. Colors indicate DIC concentrations. Circles are individual data points and squares are site medians. The solid line marks the power-law relationship for individual points (R2 = 0.5). Dashed line marks the power-law relationship for site medians (R2 = 0.3). (F) Histogram of stream-order photosynthesis fraction for site medians.
The stream-order photosynthesis fraction for individual time points ranged from less than 0.01% to greater than 1,000%. The median fraction for gage sites ranged from 0.2 to 125%, with most sites falling between 1% and 30% (Figure 2F). These results indicate that photosynthesis can process a notable portion of the carbon moving through a stream order. The fraction processed increased as photosynthesis rates increased and DIC concentrations decreased. In fact, the stream-order photosynthesis fraction and photosynthesis rate had a positive power-law relationship (R2 = 0.48 for individual points, solid black line, R2 = 0.30 for site medians, dashed black line, Figure 2E). The photosynthesis fraction did not systematically vary across stream orders; longer stream-order lengths were not associated with a greater fraction of carbon processed by photosynthesis.
The calculated fractions apply to a single stream order. A river is composed of series of stream orders. For a given carbon input, it is possible to estimate the total fraction taken up by photosynthesis across a series stream orders by assuming a perfectly ideal river network (e.g., Figure 2B) and no cycling of carbon taken up by plants. In this simplified situation, the fraction leaving a given stream order is 1 minus the stream-order photosynthesis fraction (Figure 2C). This is then the amount entering the next higher stream order within which photosynthesis can uptake an additional fraction of the original carbon input. Thus, total fractional uptake of a given carbon input due to photosynthesis across n stream orders can be calculated as: Fn = 1– (1–f1) (1–f2) (1–f3)…(1–fn), where fn is the photosynthesis fraction within the nth stream order. With this framework, relatively small fractions within individual stream orders can grow to a large total photosynthesis fraction across a river network. For example, if a single carbon input travels through 10 stream orders and plants take up 5% of the received input in each stream order (i.e., the network has a consistent 0.05 stream-order photosynthesis fraction), by the end of the journey, 40% of the original input will be lost from the water due to plant uptake.
The effect of aquatic photosynthesis on ERW-generated DIC and alkalinity depends on the membrane transport pathway used by the plant during carbon uptake (Figures 1A,B) as well as on the occurrence of processes associated with photosynthesis, such as calcite precipitation (Figure 1B) and uptake of nutrients or cations (Figure 1C). The net effect, in turn, that these biologically driven DIC and alkalinity shifts have on CDR efficacy depends on what happens to the vegetation (discussed in the next section) and the impact these DIC and alkalinity shifts have on other riverine processes (e.g., CO2 air-water exchange).
Carbon and alkalinity taken up by aquatic vegetation is stored as plant biomass. Biomass is subsequently lost from plants through respiration, degradation, or consumption by herbivores. The fate of plant carbon and alkalinity differs depending on which of these processes occurs and if the vegetation is covered by water. Aquatic vegetation can cycle between submersion and exposure to the atmosphere as river levels change; remarkably more than half of USA runoff is sourced from ephemeral streams (Brinkerhoff et al., 2024).
While the vegetation is alive, it releases carbon as CO2 during respiration. If the vegetation is covered by water, respired CO2 re-enters the water, returning carbon, but not alkalinity, to the river system (Figure 1A). If instead respiration occurs when the vegetation is not covered by water, respired CO2 directly enters the atmosphere. Degradation is similar to respiration in that CO2 is released either back into river water or the atmosphere depending on if the plant matter is covered by water. However, full degradation also releases alkalinity, represented by the excess base cations stored in the plant biomass (Figure 1C), back into the river channel. If instead aquatic biomass is consumed by aquatic or terrestrial herbivores, its carbon and alkalinity are transferred to the consuming organism. At this point, tracking the carbon and alkalinity becomes highly challenging because they can continue moving through either the aquatic or terrestrial food web.
Of these fates, only aquatic degradation fully reverses the removal of DIC and alkalinity from the stream associated with aquatic photosynthesis. The other fates either drive carbon emissions (directly or indirectly) or move DIC and alkalinity out of the stream and into food webs where they are nearly impossible to track. Given these outcomes, we contend that when aquatic plants uptake DIC and alkalinity generated by ERW, a portion of it likely never reaches the ocean. The remainder that does reach the ocean is delayed relative to water flow due to time spent incorporated in aquatic biomass.
These results are relevant for ERW carbon crediting. Credits should only be awarded once the carbon is durably stored or when there is a high degree of confidence that it will not be released before it reaches the durable reservoir. Carbon that is returned to the “fast” carbon cycle, where CO2 is taken up and released by organic matter, should not count as removal until more is known about the fate of photosynthetic carbon. Standard practice today assumes a ~15% loss of ERW-generated carbon during transport between the field and ocean (Puro Earth, 2024); our analysis indicates the actual losses are potentially higher and hard to predict.
Today, ERW deployments are small and sparse enough that weathering products are essentially undetectable once they reach a river. In this situation, we can assume that ERW products behave and partition similarly to background riverine DIC and alkalinity. However, as ERW scales, it will begin to have measurable impacts on river chemistry, in particular, increases in DIC concentrations and alkalinity, and changes in pH (Oh and Raymond, 2006). Such chemical changes can feedback and impact the biological processes discussed above.
The existing USGS chemistry data show that photosynthesis rates tend to increase as pH and DIC concentrations increase (Supplementary Figure S3). This trend could reflect a general photosynthesis response to higher DIC concentrations, or a specific response to increased bicarbonate concentrations. However, other explanations exist. For example, pH can affect sorption of elements to sediment. In particular, phosphorous, a key nutrient for biological activity, has been shown to sorb less to sediment and remain more dissolved in water at higher pH values (Temporetti et al., 2019). It is also possible that the data are showing the existing impact of agricultural runoff, which has higher pH, DIC, and nutrient concentrations (Barnes and Raymond, 2009), on aquatic photosynthesis. Regardless of the mechanisms, the analysis indicates that chemical changes in rivers induced by ERW hold potential to enhance the rate at which plants process carbon and alkalinity. The net impact of this chemical-biological feedback on the fate of ERW products in rivers is hard to predict.
The analyses demonstrate that riverine biological processes should not be ignored by the ERW community. At most locations within our dataset, aquatic photosynthesis currently processes 1 to 30% of DIC moving through a stream-order section (Figure 2). The cumulative proportion processed by plants quickly increases as photosynthesis operates across an entire stream network composed of multiple stream orders. Our analysis also indicated that as ERW scales, the anticipated changes to downstream water chemistry will likely feedback and enhance aquatic photosynthesis. The fate of DIC and alkalinity removed from the stream by aquatic photosynthesis is unclear and should be an area of further study. We contend that, most likely, a portion of DIC and alkalinity transformed into aquatic biomass never makes it into a durable storage reservoir, introducing uncertainty to the estimates of downstream losses that are currently used for ERW carbon crediting.
Publicly available datasets were analyzed in this study. Data can be found at: https://www.sciencebase.gov/catalog/item/59eb9c0ae4b0026a55ffe389; https://waterdata.usgs.gov/nwis/qw.
RN: Conceptualization, Data curation, Formal analysis, Funding acquisition, Investigation, Methodology, Project administration, Visualization, Writing – original draft, Writing – review & editing. TK: Formal analysis, Methodology, Validation, Writing – review & editing. SZ: Data curation, Funding acquisition, Writing – review & editing. DB: Conceptualization, Funding acquisition, Writing – review & editing.
The author(s) declare that financial support was received for the research and/or publication of this article. The work presented in this manuscript was supported by National Science Foundation awards 2422780 and 2419565 to RN.
A preprint of the manuscript was published on CDRXIV (Neumann et al., 2025).
The authors declare that the research was conducted in the absence of any commercial or financial relationships that could be construed as a potential conflict of interest.
The authors declare that no Gen AI was used in the creation of this manuscript.
All claims expressed in this article are solely those of the authors and do not necessarily represent those of their affiliated organizations, or those of the publisher, the editors and the reviewers. Any product that may be evaluated in this article, or claim that may be made by its manufacturer, is not guaranteed or endorsed by the publisher.
The Supplementary material for this article can be found online at: https://www.frontiersin.org/articles/10.3389/fclim.2025.1582786/full#supplementary-material
Aho, K. S., Hosen, J. D., Logozzo, L. A., McGillis, W. R., and Raymond, P. A. (2021). Highest rates of gross primary productivity maintained despite CO2 depletion in a temperate river network. Limnol. Oceanogr. Lett. 6, 200–206. doi: 10.1002/lol2.10195
Anderson, K., and Peters, G. (2016). The trouble with negative emissions. Science 354, 182–183. doi: 10.1126/science.aah4567
Appling, A. P., Read, J. S., Winslow, L. A., Arroita, M., Bernhardt, E. S., Griffiths, N. A., et al. (2018). The metabolic regimes of 356 rivers in the United States. Sci. Data 5:180292. doi: 10.1038/sdata.2018.292
Archer, D., Eby, M., Brovkin, V., Ridgwell, A., Cao, L., Mikolajewicz, U., et al. (2009). Atmospheric lifetime of fossil fuel carbon dioxide. Annu. Rev. Earth Planet. Sci. 37, 117–134. doi: 10.1146/annurev.earth.031208.100206
Babourina, O., and Rengel, Z. (2010). “Ion transport in aquatic plants” in Waterlogging Signalling and tolerance in plants. eds. S. Mancuso and S. Shabala (Berlin, Heidelberg: Springer), 221–238.
Baek, S. H., Kanzaki, Y., Lora, J. M., Planavsky, N., Reinhard, C. T., and Zhang, S. (2023). Impact of climate on the global capacity for enhanced rock weathering on croplands. Earth’s Future 11:e2023EF003698. doi: 10.1029/2023EF003698
Barnes, R. T., and Raymond, P. A. (2009). The contribution of agricultural and urban activities to inorganic carbon fluxes within temperate watersheds. Chem. Geol. 266, 318–327. doi: 10.1016/j.chemgeo.2009.06.018
Beerling, D. J., Kantzas, E. P., Lomas, M. R., Wade, P., Eufrasio, R. M., Renforth, P., et al. (2020). Potential for large-scale CO2 removal via enhanced rock weathering with croplands. Nature 583, 242–248. doi: 10.1038/s41586-020-2448-9
Biedunkova, O., and Kuznietsov, P. (2024). Investigation of the formation and variability of dissolved inorganic carbon and dissolved organic carbon in the water of a small river (on the example of the Styr River, Ukraine). Environ. Monit. Assess. 196, 1115–1117. doi: 10.1007/s10661-024-13309-3
Boehm, S., Jeffery, L., Levin, K., Hecke, J., Schumer, C., Fyson, C., et al. (2022). State of climate action 2022. Available online at: https://www.wri.org/research/state-climate-action-2022 (Accessed September 20, 2024).
Brandrud, T. E. (2002). Effects of liming on aquatic macrophytes, with emphasis on Scandinavia. Aquat. Bot. 73, 395–404. doi: 10.1016/S0304-3770(02)00032-3
Brinkerhoff, C. B., Gleason, C. J., Kotchen, M. J., Kysar, D. A., and Raymond, P. A. (2024). Ephemeral stream water contributions to United States drainage networks. Science 384, 1476–1482. doi: 10.1126/science.adg9430
Buma, B., Gordon, D. R., Kleisner, K. M., Bartuska, A., Bidlack, A., DeFries, R., et al. (2024). Expert review of the science underlying nature-based climate solutions. Nat. Clim. Chang. 14, 402–406. doi: 10.1038/s41558-024-01960-0
Butman, D., and Raymond, P. A. (2011). Significant efflux of carbon dioxide from streams and rivers in the United States. Nat. Geosci. 4, 839–842. doi: 10.1038/ngeo1294
Campeau, A., Wallin, M. B., Giesler, R., Löfgren, S., Mörth, C.-M., Schiff, S., et al. (2017). Multiple sources and sinks of dissolved inorganic carbon across Swedish streams, refocusing the lens of stable C isotopes. Sci. Rep. 7:9158. doi: 10.1038/s41598-017-09049-9
CDR.fyi (2025). CDR.fyi. Available online at: https://www.cdr.fyi/ (Accessed September 24, 2024).
Ciais, P., Sabine, C., Bala, G., Bopp, L., Brovkin, V., Canadell, J., et al. (2014). “Carbon and other biogeochemical cycles” in Climate change 2013 – The physical science basis. ed. Intergovernmental Panel on Climate Change (Cambridge: Cambridge University Press), 465–570.
Conroy, H. D., Hotchkiss, E. R., Cawley, K. M., Goodman, K., Hall, R. O. Jr., Jones, J. B., et al. (2023). Seasonality drives carbon emissions along a stream network. Journal of geophysical research. Biogeosciences 128:e2023JG007439. doi: 10.1029/2023JG007439
Downing, J. A., Cole, J. J., Duarte, C. M., Middelburg, J. J., Melack, J. M., Prairie, Y. T., et al. (2012). Global abundance and size distribution of streams and rivers. Inland Waters 2, 229–236. doi: 10.5268/IW-2.4.502
Durighetto, N., Vingiani, F., Bertassello, L. E., Camporese, M., and Botter, G. (2020). Intraseasonal drainage network dynamics in a headwater catchment of the Italian Alps. Water Resour. Res. 56:e2019WR025563. doi: 10.1029/2019WR025563
Hall, R. O. Jr., and Ulseth, A. J. (2020). Gas exchange in streams and rivers. WIREs Water 7:e1391. doi: 10.1002/wat2.1391
Harrington, K. J., Hilton, R. G., and Henderson, G. M. (2023). Implications of the riverine response to enhanced weathering for CO2 removal in the UK. Appl. Geochem. 152:105643. doi: 10.1016/j.apgeochem.2023.105643
Hayashi, M., Vogt, T., Mächler, L., and Schirmer, M. (2012). Diurnal fluctuations of electrical conductivity in a pre-alpine river: effects of photosynthesis and groundwater exchange. J. Hydrol. 450-451, 93–104. doi: 10.1016/j.jhydrol.2012.05.020
Hoffer-French, K. J., and Herman, J. S. (1989). Evaluation of hydrological and biological influences on CO2 fluxes from a karst stream. J. Hydrol. 108, 189–212. doi: 10.1016/0022-1694(89)90283-7
Hollnaicher, S. (2022). On economic modeling of carbon dioxide removal: values, bias, and norms for good policy-advising modeling. Global Sustainability 5:e18. doi: 10.1017/sus.2022.16
Hotchkiss, E. R., Hall, R. O. Jr., Sponseller, R. A., Butman, D., Klaminder, J., Laudon, H., et al. (2015). Sources of and processes controlling CO2 emissions change with the size of streams and rivers. Nat. Geosci. 8, 696–699. doi: 10.1038/ngeo2507
IPCC (2022). Climate change 2022: Mitigation of climate change. Contribution of working group III to the sixth assessment report of the intergovernmental panel on climate change. Cambridge, UK and New York, NY, USA: Cambridge University Press.
Iversen, L. L., Winkel, A., Baastrup-Spohr, L., Hinke, A. B., Alahuhta, J., Baattrup-Pedersen, A., et al. (2019). Catchment properties and the photosynthetic trait composition of freshwater plant communities. Science 366, 878–881. doi: 10.1126/science.aay5945
Kaijser, W., Lorenz, A. W., Birk, S., and Hering, D. (2021). The interplay of nutrients, dissolved inorganic carbon and algae in determining macrophyte occurrences in rivers. Sci. Total Environ. 781:146728. doi: 10.1016/j.scitotenv.2021.146728
Kantola, I. B., Masters, M. D., Beerling, D. J., Long, S. P., and DeLucia, E. H. (2017). Potential of global croplands and bioenergy crops for climate change mitigation through deployment for enhanced weathering. Biol. Lett. 13:20160714. doi: 10.1098/rsbl.2016.0714
Kantzas, E. P., Val Martin, M., Lomas, M. R., Eufrasio, R. M., Renforth, P., Lewis, A. L., et al. (2022). Substantial carbon drawdown potential from enhanced rock weathering in the United Kingdom. Nat. Geosci. 15, 382–389. doi: 10.1038/s41561-022-00925-2
Knapp, W. J., and Tipper, E. T. (2022). The efficacy of enhancing carbonate weathering for carbon dioxide sequestration. Front. Clim. 4:928215. doi: 10.3389/fclim.2022.928215
Li, Z., Planavsky, N. J., and Reinhard, C. (2024). Geospatial assessment of the cost and energy demand of feedstock grinding for enhanced rock weathering in the coterminous United States. Front. Clim. 6:1380651. doi: 10.3389/fclim.2024.1380651
McManamay, R. A., and DeRolph, C. R. (2019). A stream classification system for the conterminous United States. Sci. Data 6:190017. doi: 10.1038/sdata.2019.17
Middelburg, J. J., Soetaert, K., and Hagens, M. (2020). Ocean alkalinity, buffering and biogeochemical processes. Rev. Geophys. 58:e2019RG000681. doi: 10.1029/2019RG000681
Moosdorf, N., Renforth, P., and Hartmann, J. (2014). Carbon dioxide efficiency of terrestrial enhanced weathering. Environ. Sci. Technol. 48, 4809–4816. doi: 10.1021/es4052022
Neumann, R. B., Kukla, T., Zhang, S., and Butman, D. E. (2025). Photosynthesis in rivers as a loss pathway for ERW-derived DIC and alkalinity. CDRXIV. Available online at: https://cdrxiv.org/preprint/329 (Accessed February 12, 2025).
Oh, N.-H., and Raymond, P. A. (2006). Contribution of agricultural liming to riverine bicarbonate export and CO2 sequestration in the Ohio River basin. Glob. Biogeochem. Cycles 20:GB3012. doi: 10.1029/2005GB002565
Poschenrieder, C., Fernández, J. A., Rubio, L., Pérez, L., Terés, J., and Barceló, J. (2018). Transport and use of bicarbonate in plants: current knowledge and challenges ahead. Int. J. Mol. Sci. 19:1352. doi: 10.3390/ijms19051352
Puro Earth (2024). Enhanced rock weathering methodology for CO2 removal. Available online at: https://7518557.fs1.hubspotusercontent-na1.net/hubfs/7518557/ERW%20Standards/Enhanced_Rock_Weathering_2022_2.pdf (Accessed January 27, 2025).
Raymond, P. A., Hartmann, J., Lauerwald, R., Sobek, S., McDonald, C., Hoover, M., et al. (2013). Global carbon dioxide emissions from inland waters. Nature 503, 355–359. doi: 10.1038/nature12760
Rehn, L., Sponseller, R. A., Laudon, H., and Wallin, M. B. (2023). Long-term changes in dissolved inorganic carbon across boreal streams caused by altered hydrology. Limnol. Oceanogr. 68, 409–423. doi: 10.1002/lno.12282
Rockström, J., Schellnhuber, H. J., Hoskins, B., Ramanathan, V., Schlosser, P., Brasseur, G. P., et al. (2016). The world’s biggest gamble. Earth’s Future 4, 465–470. doi: 10.1002/2016EF000392
Smith, S. M., Geden, O., Gidden, M. J., Lamb, W. F., Nemet, G. F., Minx, J. C., et al. (2024). The state of carbon dioxide removal - 2nd edition. The State of Carbon Dioxide Removal. Available at: https://osf.io/f85qj/ (Accessed May 23, 2024).
Temporetti, P., Beamud, G., Nichela, D., Baffico, G., and Pedrozo, F. (2019). The effect of pH on phosphorus sorbed from sediments in a river with a natural pH gradient. Chemosphere 228, 287–299. doi: 10.1016/j.chemosphere.2019.04.134
Tobias, C., and Böhlke, J. K. (2011). Biological and geochemical controls on diel dissolved inorganic carbon cycling in a low-order agricultural stream: implications for reach scales and beyond. Chem. Geol. 283, 18–30. doi: 10.1016/j.chemgeo.2010.12.012
Trentman, M. T., Hall, R. O. Jr., and Valett, H. M. (2023). Exploring the mismatch between the theory and application of photosynthetic quotients in aquatic ecosystems. Limnol. Oceanogr. Lett. 8, 565–579. doi: 10.1002/lol2.10326
US Geological Survey (2016). USGS water-quality data for the nation. Available online at: https://waterdata.usgs.gov/nwis/qw (Accessed February 14, 2024).
Zhang, B., Kroeger, J., Planavsky, N., and Yao, Y. (2023). Techno-economic and life cycle assessment of enhanced rock weathering: a case study from the Midwestern United States. Environ. Sci. Technol. 57, 13828–13837. doi: 10.1021/acs.est.3c01658
Zhang, S., Planavsky, N. J., Katchinoff, J., Raymond, P. A., Kanzaki, Y., Reershemius, T., et al. (2022). River chemistry constraints on the carbon capture potential of surficial enhanced rock weathering. Limnol. Oceanogr. 67, S148–S157. doi: 10.1002/lno.12244
Zhang, S., Reinhard, C. T., Liu, S., Kanzaki, Y., and Planavsky, N. J. (2025). A framework for modeling carbon loss from rivers following terrestrial enhanced weathering. Environ. Res. Lett. 20:024014. doi: 10.1088/1748-9326/ada398
Keywords: carbon dioxide removal (CDR), enhanced rock weathering, aquatic photosynthesis, enhanced mineral weathering, carbon, alkalinity, dissolved inorganic carbon
Citation: Neumann RB, Kukla T, Zhang S and Butman DE (2025) Riverine photosynthesis influences the carbon sequestration potential of enhanced rock weathering. Front. Clim. 7:1582786. doi: 10.3389/fclim.2025.1582786
Received: 24 February 2025; Accepted: 20 March 2025;
Published: 04 April 2025.
Edited by:
Ben W. Kolosz, University of Hull, United KingdomReviewed by:
Devon Kerins, University College Dublin, IrelandCopyright © 2025 Neumann, Kukla, Zhang and Butman. This is an open-access article distributed under the terms of the Creative Commons Attribution License (CC BY). The use, distribution or reproduction in other forums is permitted, provided the original author(s) and the copyright owner(s) are credited and that the original publication in this journal is cited, in accordance with accepted academic practice. No use, distribution or reproduction is permitted which does not comply with these terms.
*Correspondence: Rebecca B. Neumann, cmJuZXVtQHV3LmVkdQ==
Disclaimer: All claims expressed in this article are solely those of the authors and do not necessarily represent those of their affiliated organizations, or those of the publisher, the editors and the reviewers. Any product that may be evaluated in this article or claim that may be made by its manufacturer is not guaranteed or endorsed by the publisher.
Research integrity at Frontiers
Learn more about the work of our research integrity team to safeguard the quality of each article we publish.