- 1Soil Chemistry Group, Wageningen University & Research, Wageningen, Netherlands
- 2Eion Corp., Princeton, NJ, United States
- 3Lithos Carbon, Inc., Seattle, WA, United States
- 4Terradot Soil Inc., San Francisco, CA, United States
Various approaches are currently used to quantify the carbon dioxide removal (CDR) associated with enhanced weathering (EW), which involves amending soils with crushed silicate minerals. We aimed to contribute to the development of a standardized procedure for CDR quantification by complementing the results of a recently published soil column experiment, in which crushed olivine, wollastonite, and albite were added to soils, with total fusion ICP-OES analyses of base cation concentrations. CDR quantified by soil-based mass balance approaches was only comparable to leachate-based total alkalinity measurements after correcting for the weathering products that were retained within the soil profile, which we defined as the retarded fraction. The retarded fraction comprised 92.7–98.3% of the weathered cations, indicating that at least in our short-term study (64 days), the majority of weathering products were retained within the soil. Further investigation of the fate of retarded weathering products showed that small portions precipitated as carbonate minerals (up to 34.0%) or adsorbed to reactive surfaces, such as soil organic matter and clay minerals (up to 32.5%). Hence, a large portion of weathering products may be retained in the soil due to strong adsorption and/or further mineral precipitation reactions (31.6–92.7%), with potentially important implications for the quantification of CDR across time. We conclude that soil-based mass balance approaches are useful in quantifying weathering rates and can infer potential CDR; however, the actual CDR realized for a given time and depth interval can only be constrained after accounting for the retarded fraction.
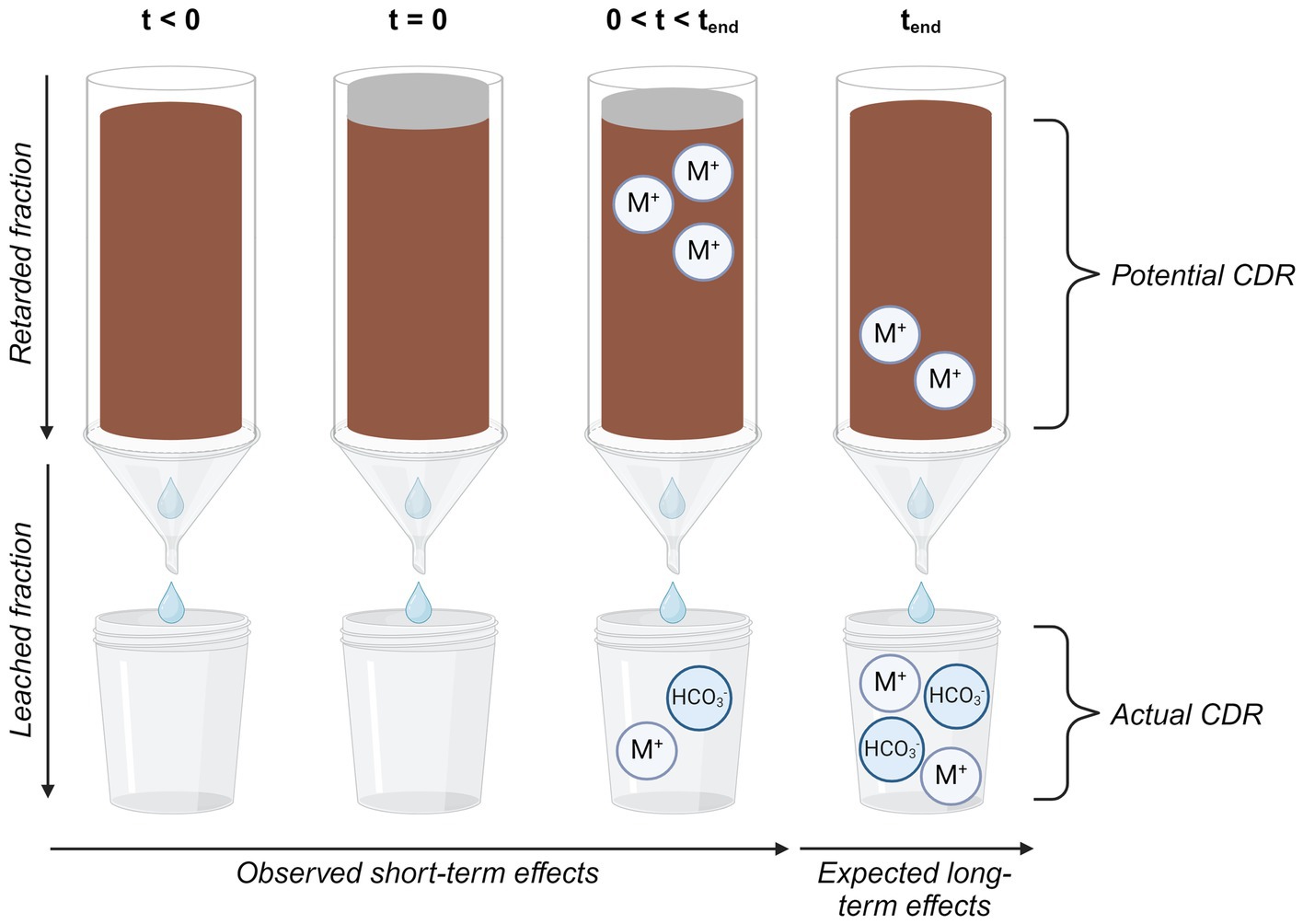
Graphical Abstract. Graphical abstract created in BioRender.com.
1 Introduction
Fossil fuel combustion and land use change have caused global surface temperature to increase 1.1°C compared to pre-industrial levels (IPCC, 2023). Under current emissions, the remaining carbon budget to limit global temperature rise to 1.5°C is likely reached by the year 2029 (Lamboll et al., 2023). These projections stress that, on top of rapid anthropogenic emission reductions, large-scale carbon dioxide (CO2) removal (CDR) needs to be employed in the near future (IPCC, 2018; Smith et al., 2024). CDR involves human activities that result in the capture of atmospheric CO2 and subsequent durable storage in geological, land, or ocean reservoirs (Smith et al., 2024). Enhanced weathering (EW) is a promising and potentially scalable CDR technique, as it may remove CO2 for millennia and longer, can provide co-benefits for agricultural productivity, and the infrastructure for its extensive employment on croplands is available (Beerling et al., 2020; Smith et al., 2024).
EW involves the acceleration of the natural silicate weathering cycle by amending soils with crushed silicate minerals (Beerling et al., 2018; Schuiling and Krijgsman, 2006). The weathering of silicate minerals by CO2 dissolved in water produces bicarbonate ions (HCO3−) and base cations (Ca2+, Mg2+, K+, Na+). To qualify as CDR as defined above, the HCO3− ions produced during the capture of CO2 must either leach out of soils and reach the oceans via rivers or groundwater, or precipitate as stable carbonate minerals (CaCO3) in soils or oceans, producing durable CO2 sequestration on geological time scales (Clarkson et al., 2024; Hartmann et al., 2013). In many soils, especially in non-arid regions and managed croplands, the formation of stable carbonate minerals is unlikely due to continuous dissolution and reprecipitation of CaCO3 (Clarkson et al., 2024). Quantifying CDR based on the leached weathering products, and thus excluding soil carbonate minerals, may result in a potential underestimation but provides conservative CDR estimates. This conservative method still calls for a clear definition of what is considered leachate in a field setting: should weathering products be traced in soil pore water, and if so at which depth(s), or should only the products be traced that have reached rivers? (Mills et al., 2024). In addition to leaching out, weathering products may also precipitate as other secondary minerals or bind to reactive soil surfaces, such as soil organic matter (SOM) and clay minerals, further complicating the quantification of CDR (Kelland et al., 2020).
In current literature, various approaches are being used to quantify CDR associated with EW. Studies in which CDR is fully based on the dissolved fraction of base cations generally find low estimates compared to those including solid phases. Based on magnesium (Mg2+) leaching, Amann et al. (2020) reported 0.02–0.05 t CO2 removal ha−1 y−1 after applying 220 t olivine-rich dunite ha−1 in a mesocosm experiment. Buckingham et al. (2022) calculated total alkalinity (TA) using the dissolved concentrations of base cations measured at several depths along their soil columns, sequestering only 0.01 t CO2 ha−1 y−1 following 100 t ha−1 basalt amendment. Both authors conclude that cation adsorption, secondary mineral precipitation, and plant uptake may at least partly explain their low CDR estimates. In contrast, other studies report CDR estimates that are orders of magnitude higher through monitoring the change in solid inorganic carbon (Haque et al., 2020; Khalidy et al., 2023), notably excluding the produced TA. Although TA titrations can be considered a direct measurement of CDR, these measurements are labor-intensive and highly variable in space and time, consequently making them unsuitable for large-scale applications (Clarkson et al., 2024). Using electrical conductivity as a proxy for TA is restricted to soils with a low cation exchange capacity (CEC), SOM, and clay content, excluding the majority of soils (Rieder et al., 2024). Therefore, mass balance approaches combining base cations in soil, plants, and leachate are frequently used (Kelland et al., 2020; te Pas et al., 2023; ten Berge et al., 2012). Here, the fate of base cations within the soil and the extraction method used affect the CDR estimates. For example, a 0.01 M CaCl2 extraction is frequently used to approximate the cations in soil solution, thereby excluding cations adsorbed to reactive binding sites (Houba et al., 2000). After constructing mass balances based on the Mg contents of soils extracted with 0.01 M CaCl2 and plants, ten Berge et al. (2012) concluded that the 2.69 t CO2 sequestration ha−1 calculated following a 32-week pot experiment in which soils were amended with 204 t olivine ha−1 was likely an underestimation. Te Pas et al. (2023) used a strong acid extraction (0.43 M HNO3) but only after removing the thin layer of applied minerals at the soil surface to avoid unintended mineral dissolution by the extractant. However, this is not possible for most experiments in which the applied minerals are mixed with the upper soil layer. Recently, Reershemius et al. (2023) validated a soil-based mass balance approach that overcomes this issue by measuring changes in the concentrations of mobile base cations relative to immobile trace elements in pre- and post-treatment samples. Their results indicate good agreement with the measurement of weathering products in soil, plants, and leachate combined. Based on their approach, US field experiments amended with 50 t basalt ha−1 y−1 sequestered approximately 3.8 t CO2 ha−1 in the first year (Beerling et al., 2024; Kantola et al., 2023), indicating possibly higher CDR by EW compared to quantifications by earlier studies using different methods.
The development of a standardized quantification method for CDR associated with EW is required to allow Monitoring, Reporting, and Verification (MRV). Here, we contribute to this objective by complementing the results of a recently published soil column experiment amended with olivine, wollastonite, and albite (te Pas et al., 2023) with analyses of the concentrations of mobile base cations. Our aim for this study is three-fold. First, we compare the CDR quantified by two soil-based elemental mass balance approaches to leachate-based total alkalinity. Second, we quantify the retarded fraction, i.e., the fraction of weathering products that is retained within the soil profile. Finally, we provide insights on the nature of the adsorption and precipitation mechanisms of retarded weathering products within the soil.
2 Materials and methods
2.1 Materials
The soil samples used in the study by te Pas et al. (2023) originated from the topsoil of a sandy grassland (pH-H2O = 5.16) in Wageningen, the Netherlands (51°59′39.3″N 5°40′05.9″E). Based on the location, the soil was classified as Gleyic Phaeozem according to the FAO World Reference Base system. Crushed olivine minerals were supplied by the Åheim Gusdal Mine located in Steinsvik, Norway. Crushed wollastonite minerals were retrieved from a mine located at Saint Lawrence Wollastonite Deposit, Ontario, Canada. Albite was obtained from a mine at Bancroft, Ontario, Canada, and was crushed using a jaw crusher. Table 1 shows the major elemental composition and specific surface area (SSA) of the baseline soil and the three minerals used for the soil column experiment. For further details on the soil and minerals, we refer to te Pas et al. (2023).
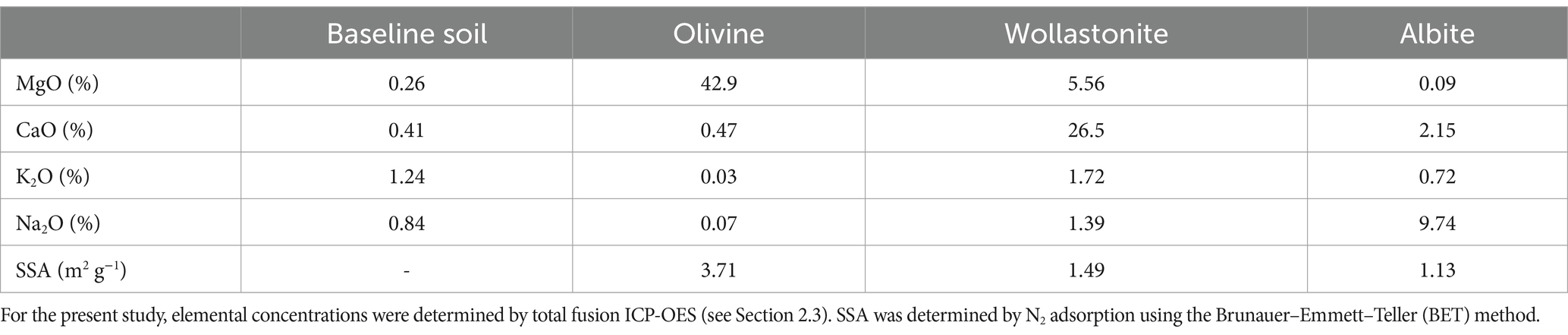
Table 1. Major elemental composition and specific surface area (SSA) of the soil and minerals used in the experiment of te Pas et al. (2023).
2.2 Experimental set-up
The experimental set-up was described in te Pas et al. (2023). In brief, a down-flow soil column experiment was conducted for 64 days in a conditioned room of 20 ± 2°C. Soil columns (180 mL, Ø 5 cm) were connected via a funnel to a closed container, in which the leachate was collected. Columns were filled with 150 g soil and 100 mL de-mineralized water using a five-step wet packing procedure (Gilbert et al., 2014; ISO, 2019), followed by 2 days of pre-incubation in which redundant water leached from the columns. On day 1 of the experiment, 18.75 g (125 g kg−1 soil) of olivine, wollastonite, and albite were applied to different columns as a layer on top of the soil. Each treatment was studied in duplicate, and one control soil without silicate amendment was used. Here, we present additional analyses for one of the duplicates, which were referred to in te Pas et al. (2023) as Olivine 1 (Ol1), Wollastonite 2 (Wo2), and Albite 1 (Al1). Every 2 to 4 days, 40 mL de-mineralized water was applied to the top of the soil columns. Afterward, leachate was collected in the containers below the soil columns and sampled at 9 time points between 1 and 64 days for analyses of base cation concentrations (Ca2+, Mg2+, K+, Na+) using inductively coupled plasma optical emission spectrometry (ICP-OES), and at 21 time points for pH measurements and titrations with 0.02 M HCl to determine TA, with a decreasing frequency over time. Upon termination of the experiment, the thin layer of applied minerals on top of the soil was removed based on visual observations, producing two separate samples of the post-treatment soil and the layer of applied minerals for each treatment. Although separated, the layer of applied minerals very likely contained some soil particles and vice versa. The samples were separately dried at 40°C, sieved over 2 mm, and homogenized.
2.3 Chemical analyses of soils and minerals
The baseline soil, the three mineral feedstocks (olivine, wollastonite, and albite), the post-treatment soils, and the separated layers of applied minerals were collected at the end of the experiment (10 samples in total) and used for further analysis using total fusion ICP-OES. Total fusion was conducted by mixing samples with a flux of lithium metaborate and lithium tetraborate to induce a fused sample within an induction furnace. The resultant molten melt was poured into a 5% nitric acid solution until completely dissolved prior to analysis. The final processed samples were then analyzed using ICP-OES.
2.4 Methods used to quantify CDR
We present three methods to calculate CDR associated with EW (Table 2). Method 1 (M1) is a soil-based mass balance approach using base cation concentrations measured in the thin layer of applied minerals and mineral feedstock, determined by total fusion ICP-OES. Since olivine, wollastonite, and albite amendments were applied to the soil surface, the characterization of elements in the separated layer of applied minerals was used to calculate weathering rates. Method 2 (M2) was based on the elemental mass balance (EMB) method presented in te Pas et al. (2023), which is derived from the base cations measured in the leachate and in a strong acid (0.43 M HNO3) extract of the soil, analyzed using ICP-OES. Mass balances (M1 and M2) were constructed using the major base cation present in the mineral feedstock, being Mg for olivine, calcium (Ca) for wollastonite, and sodium (Na) for albite (Table 1). Finally, method 3 (M3) involved TA titrations of the leachates.
2.4.1 Calculations method 1
The first method uses a base cation mass balance approach to quantify weathering rates, CDR, and the retarded fraction based on analyses on the mineral feedstock and the thin layer of applied minerals (see Supplementary Table S1 for the mass of the collected layers). First, the change in base cations (Δcation) was calculated by subtracting the concentration of the base cation in the thin layer of applied minerals (mineral layer) after the experiment (tend) and at the start of the experiment (t0) using Equation 1.
Equation 2 was used to quantify the fraction of mineral amendment that had weathered (Fw) based on the loss of weathering products from the layer of applied minerals for the major base cation present in the mineral feedstock.
Since the layer of applied minerals collected at the end of the experiment likely also contained some soil particles, is expected to be underestimated when the cation concentration in the applied mineral is higher than that in the soil. As such, this method may lead to an overestimation of Fw based on how much soil was mixed in with the collected layer of applied minerals. CDR (Equation 3) was calculated by multiplying Fw by the mineral dose applied (which was 18.75 g for each treatment), and the CDR potential of the mineral feedstock divided by the mass of soil in the column (masssoil; 0.15 kg). The CDR potential of a mineral feedstock (CDRpot) was defined as the maximum theoretical CO2 removal potential (g CO2 g−1 mineral weathered) based on elemental composition and was calculated by multiplying the molar mass of CO2 by the valence of each cation and by the sum of the molar concentrations of the four base cations as measured for the mineral feedstocks using total fusion ICP-OES (Reershemius et al., 2023).
Base cation concentrations were also directly quantified in the leachate of each column (te Pas et al., 2023). The total mass of retarded cations is the difference between ∆cation and the sum of cations in the leachate. The retarded fraction, i.e., the fraction of weathering products that is retained within the soil profile, was quantified as the concentration of weathered base cations normalized to the base cations directly measured in the leachate ([Cation]leachate) (Equation 4).
The leached C that left the soil column was quantified using Equation 5 by multiplying the calculated CDR (Equation 3) by the fraction of weathering products that was not retarded (Equation 4).
2.4.2 Calculations method 2
CDR was calculated in te Pas et al. (2023) using two methods (here M2 and M3, with minor adaptations). The elemental mass balances (EMB-method) will be referred to here as method 2 (M2). The baseline soil and the soil samples at the end of the experiment were extracted with 0.43 M HNO3 followed by ICP-OES analysis of Mg, Ca, and Na concentrations ([Cation]soil; Groenenberg et al., 2017). For M2, the thin layer of applied minerals at the soil surface that also contained some soil particles was excluded from analysis with this strong acid to avoid unintended mineral dissolution by the extractant as much as possible. Base cation concentrations measured in the leachate samples were linearly interpolated over time to determine cumulative leaching ([Cation]leachate). Equation 6 was used to determine Fw, in which [Cation]applied, i.e., the total amount of Mg, Ca, or Na applied through mineral amendment, was based on total fusion ICP-OES analysis.
Then, Equation 3 was used to calculate CDR, which differs from te Pas et al. (2023) through the use of a calculated CDRpot (see Section 2.4.1) instead of assuming stoichiometry. Finally, leached C was calculated using Equation 7.
2.4.3 Calculations method 3
CDR was also quantified in te Pas et al. (2023) based on TA titrations of collected leachate samples and soil inorganic carbon content using Scheibler calcimetry (IC-method). Since we focus here on the leached C, only the TA titrations of the leachate were used for method 3 (M3). M3 is thus an adaptation of the IC-method presented in te Pas et al. (2023). Hence, this method can only be used to derive CDR (not Fw), which equals the leached C and excludes the retarded fraction. TA concentrations were linearly interpolated over time to calculate cumulative TA leached (mol). We multiplied the difference in TA between mineral-treated (TAtreatment) and control soils (TAcontrol) by the molar mass of CO2 (g mol−1) and divided by the mass of soil in the column (0.15 kg; Equation 8).
2.5 Additional analysis on the retarded fraction
The concentrations of soil exchangeable base cations (Ca2+, Mg2+, K+, Na+) were determined using a 0.1 M BaCl2 extraction followed by ICP-OES measurements (ISO, 2018). Soil carbonate content was determined using Scheibler calcimetry (Allison, 1960; ISO, 1995). Both soil pools were corrected for the concentrations measured in the baseline soil and compared to the results obtained by total fusion ICP-OES analysis, with the aim of retrieving the fate of weathering products in the retarded fraction. We then defined an unknown soil pool by subtracting the weathering products traced in the leachate, the exchangeable pool and precipitated as carbonate minerals from the weathered fraction defined by total fusion ICP-OES analysis. This unknown soil pool represents the weathering products that were retarded in the soil column but not in the exchangeable fraction or as carbonate minerals.
3 Results
3.1 CDR quantification by different methods
The CDR calculated using three methods for the three different minerals is shown in Figure 1A. For the olivine treatment, 15.5 g CO2 kg−1 soil was calculated using M1, which involved a mass balance using the major base cation present (Mg) based on total fusion ICP-OES analyses of the layer of applied minerals. M2 involved a mass balance using the Mg concentrations measured in the leachate and the soil extracted with 0.43 M HNO3, indicating 1.39 g CO2 kg−1 soil. M3, i.e., TA titrations of leachate samples, resulted in the lowest estimates of 0.21 g CO2 kg−1 soil. For the wollastonite treatment, the highest CDR estimate was also found for M1, namely, 12.0 g CO2 kg−1 soil. Lower CDR estimates were found for M2, 1.44 g CO2 kg−1 soil, and M3, 0.12 g CO2 kg−1 soil. M1 also produced the highest CDR estimates for the albite treatment, 3.67 g CO2 kg−1 soil, followed by 0.36, and 0.14 g CO2 kg−1 soil using M2 and M3, respectively. Figure 1B shows the carbon that was actually leached during the experiment, producing values that were all at least an order of magnitude lower compared to the calculated CDR, except for M3 where calculated CDR equals leached C. Furthermore, the difference in estimated leached C between the four methods was <0.2 g CO2 kg−1 soil for all treatments, showing values of 0.21–0.26, 0.12–0.22, and 0.14–0.27 g CO2 leached kg−1 soil for the olivine, wollastonite, and albite treatments, respectively.
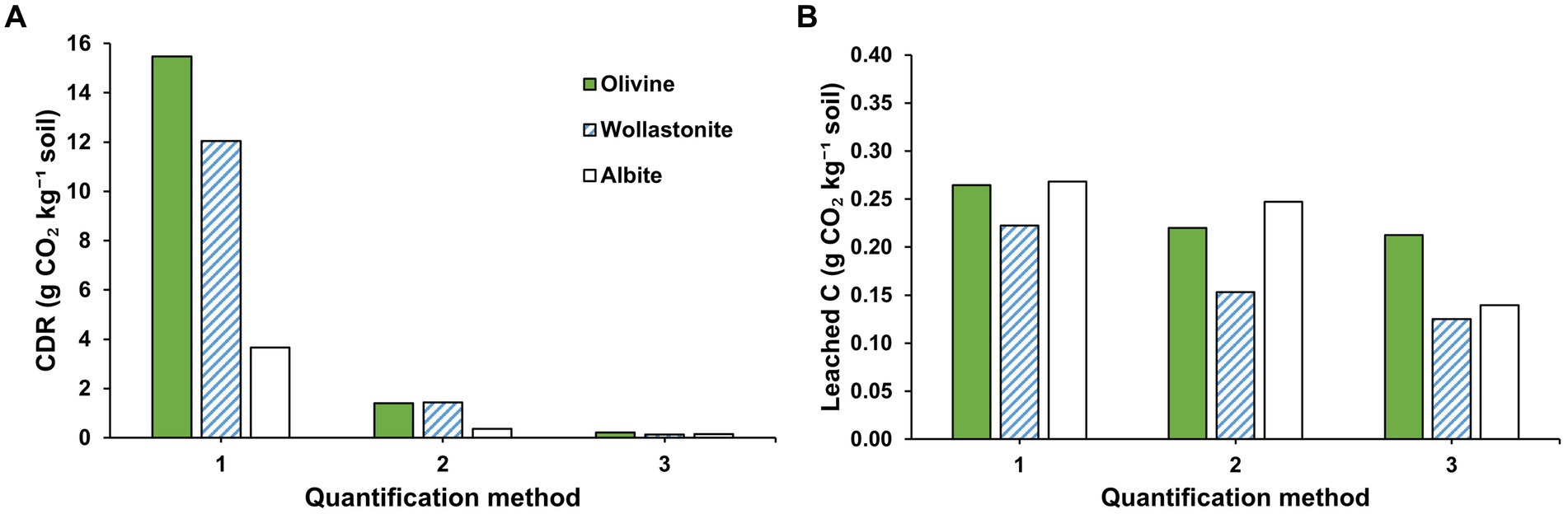
Figure 1. (A) Carbon dioxide removal (CDR) quantified using different methods at the end of the soil column experiment of te Pas et al. (2023); (B) Carbon that was leached during the experiment (see Sections 2.4.1–2.4.3). M1 and M2 are based on the major base cation of the applied mineral, being Mg for olivine, Ca for wollastonite, and Na for albite. Note that the y-axis of B is 2.5% of A and that M3 shows the same data in both figures.
3.2 Quantifying the fraction of retarded weathering products
Using M1, the fraction of retarded weathering products was quantified (Table 3). For the olivine treatment, 98.3% of the weathered Mg was calculated to be retained within the soil profile during the experiment. Of the Ca weathered from the wollastonite amendment, 98.2% was retained in the soil column. For the albite treatment, a retarded fraction of 92.7% was calculated for Na. Additional analyses were conducted to gain insights into the fate of base cations in the retarded fraction (Supplementary Table S2). A comparison of the base cation concentrations extracted with 0.1 M BaCl2 to the concentrations measured with total fusion ICP-OES showed that 1.47% (albite), 6.48% (olivine), and 12.3% (wollastonite) of the weathered cations measured with total fusion ICP-OES could be traced in 0.1 M BaCl2 extractions (not corrected for the baseline soil). While albite produced a soil CaCO3 content below that of the baseline soil (56.4 mg per 150 g soil), soil CaCO3 content increased to 149 mg for olivine and 190 mg for wollastonite per 150 g soil in the column (Supplementary Table S2).
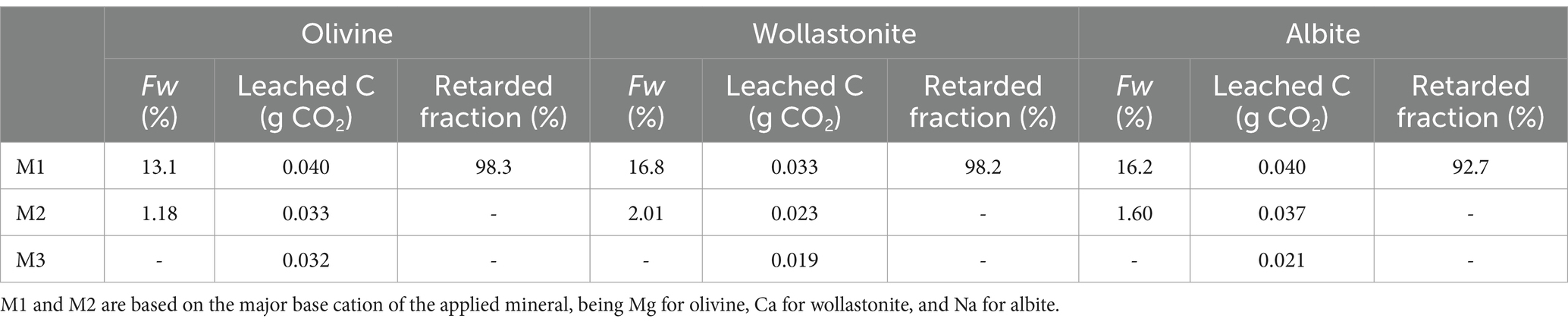
Table 3. Overview of the weathered fractions (Fw, %), leached C (g CO2 leached from the column corrected for the control), and retarded fractions (%) quantified using different methods (see Sections 2.4.1–2.4.3) at the end of the soil column experiment of te Pas et al. (2023).
4 Discussion
4.1 Comparison of methods to quantify CDR
We showed that the CDR associated with EW quantified by soil-based mass balance approaches was one or two orders of magnitude higher than leachate-based TA measurements (Figure 1A). The calculated fractions of retarded weathering products were all nearing 100% (Table 3), indicating that the vast majority was retained within the soil profile. When correcting for this retarded fraction, the calculated leached C (M1 and M2) closely overlapped with the measured leachate-based TA (M3) (Figure 1B; Table 3). Hence, soil-based mass balance approaches are only able to fully quantify CDR, involving both the capture of CO2 and its durable storage (see definition in Section 1), over a given time and depth interval (here 2 months and 6 centimeters of soil) when accounting for the retarded fraction. Our conclusions are consistent with those of Reershemius et al. (2023). In addition, our study allows a quantitative assessment of the importance of the fraction of retarded weathering products and we provide calculations for a range of elements and mineral feedstocks.
Large differences in quantified CDR were also observed among the different soil-based mass balance approaches, where M2 yielded substantially lower Fw and CDR than M1 (Figure 1A; Table 3). M2 was based on base cations measured in the leachate and extracted from soils, excluding the thin layer of applied minerals that besides the applied mineral also contained some soil particles. Other soil column and mesocosm studies found the dissolved base cation concentrations in the bottom layer to be about an order of magnitude lower than in the top layer (Amann et al., 2020; Buckingham et al., 2022). As a result of the limited mobility of weathering products in our experiment, as confirmed by the quantified retarded fraction, it is likely that weathered base cations were primarily retained in the upper part of the soil profile, directly below the layer of applied minerals. Since the fraction of weathering products retained in this thin upper layer was not considered in M2, we conclude that M2 underestimates Fw and CDR. However, M1 is based on the assumption that base cations are truly mobile (Clarkson et al., 2024), while our quantified retarded fractions suggest otherwise. Methods such as those used by Reershemius et al. (2023) incorporate pre-treatment steps that leach out labile forms of cations bound to reactive soil surfaces, thereby removing part of the cations in the retarded fraction. We did not deploy such methods and instead extracted total concentrations through total fusion, hence allowing for the quantification of both the full retarded and leached fractions of weathering products. Our approach allows for a continued improvement of our understanding of cation behavior within soil and supports the development of soil-based mass balance methods for CDR quantification.
Uncertainties in mass balance approaches mainly arise from three primary factors. First, analytical errors may propagate through the largest term in the mass balance, i.e., the soil (Pogge von Strandmann et al., 2021). Second, error is introduced by analyzing a small sub-sample of a larger mass of soil, as discussed in Reershemius et al. (2023). Third, uncertainty was introduced by using the replicates of te Pas et al. (2023) for which the columns experienced reduced through-flow of water over time and (likely temporarily) anoxic soil conditions. These specific conditions were observed in all three treatments presented here and, while not invalidating the method, may have impacted cation mobility and the fate of cations within the soil. Further research should therefore include replicates to provide insights in the uncertainty and variability of the presented methods to quantify the retarded fraction.
4.2 Accounting for the retarded fraction
Recent EW studies indicated that weathering signals measured in soil may not be reflected in significant changes in leachate alkalinity (Kelland et al., 2020; Vienne et al., 2024). This phenomenon can be explained by the retarded fraction of weathering products. Using the results for M1-olivine as an example (similar values were observed for wollastonite and albite), we observed a Fw of 13.1% over the course of the experiment, while the retarded fraction represented 98.3% of the weathered amount of Mg. The latter means that of the weathered Mg, only 1.71% could be traced in the leachate. Hence, the actual CDR is lower than the potential CDR. The C that is leached from soil (as measured by leachate-based TA titrations (Equation 8) or calculated by Equations 5, 7) is what we define as the actual CDR realized over a given time and depth interval (here 2 months and 6 centimeters of soil; Figure 1B). The potential CDR equals the CDR calculated based on Fw over a given time and depth interval (Figure 1A) and represents the CDR that could be achieved once the weathering products are fully leached from the soil column (assuming all weathering is driven by carbonic acid). Referring to the definition of CDR (see Section 1 and Smith et al., 2024), the actual CDR involves both the capture and durable storage of CO2 in soils or oceans, while the potential CDR involves the capture of CO2 through mineral weathering which is for a large part not (yet) followed by durable storage (i.e., C leaching). The 98.3% of Mg that originated from weathered olivine (M1) and was retarded within the soil profile in the initial stage of weathering can thus result in actual CDR once leached from the soil. We must consider that the potential CDR may involve base cations lost through plant uptake followed by harvest, retention in less-easily exchangeable pools by strong binding to reactive soil surfaces, and/or mineral precipitation. Whether weathered base cations in those pools are truly lost without further contribution to CDR depends on their fate, i.e., decomposition of crop residues, cation desorption, and mineral redissolution may (partly) return those base cations to soil. The relevance of adsorption and precipitation mechanisms in our experiment will be discussed in Section 4.3. Plant uptake was not possible in our soil column experiment but was found to have a minor impact under mesocosm and field conditions (Beerling et al., 2024; Kelland et al., 2020). Note that potential CDR should not be confused with CDRpot, which is the maximum theoretical CO2 removal potential of a mineral feedstock (g CO2 g−1 mineral weathered) based on its elemental composition (see Section 2.4.1 and Reershemius et al., 2023). In summary, soil-based mass balance approaches can be used to quantify the Fw and potential CDR but are unable to quantify the actual CDR over a given time and depth interval without correcting for the retarded fraction, while leachate-based TA concentrations can only indicate the actual CDR over a given time and depth interval but provide no indication of the Fw.
The retardation of weathering products implies a time lag between potential and actual CDR, with implications for upscaling CDR and MRV associated with EW. Spatial upscaling seems feasible but is complicated by defining the boundaries of the system. Here, we used the entire soil column for CDR quantification, while in the field one may define the system based on the topsoil only (i.e., the mixing layer) or include also the subsoil where weathering products are (temporarily) retained. Temporal upscaling of CDR may be even more complicated due to the time lag introduced by the retarded fraction. Limited knowledge on the fate and thus residence time of this retarded fraction (see Section 4.3) and consequently when leaching of retarded cations will occur (i.e., when potential CDR will become actual CDR) challenge the temporal upscaling of CDR. These obstacles for spatial and temporal upscaling of CDR may hinder the development of cost-effective MRV and carbon credit systems for EW. However, our methods allow quantification of the retarded fraction in a way that is relatively straightforward without the need to use isotopes (Kelland et al., 2020; Larkin et al., 2022), which is important for the economical scaling of EW (Clarkson et al., 2024). The construction of lysimeter set-ups to properly monitor the migration of weathering products and leaching of C is a one-time costly and time-consuming step, but this will drastically improve our understanding of retarded weathering products and the time lag between actual and potential CDR. The duration of our experiment was limited to 64 days, as in te Pas et al. (2023) and similar to several other small-scale EW studies (e.g., Dietzen et al., 2018; Haque et al., 2019; Kelland et al., 2020). It is important to note that the retarded fraction is a function of time. As soil transport processes take place over time, this fraction is expected to change. Although our results may not be representative of long-term scenarios, the calculation methods that we present are relevant to apply in EW experiments of any duration.
4.3 Initial insights on the fate of retarded weathering products
Our final aim for this study was to provide insights on the fate of retarded weathering products and the potential binding and/or precipitation mechanisms responsible for the retarded fraction. A comparison of the results of total fusion ICP-OES to 0.1 M BaCl2 extractions shows that of the major base cations in the retarded fraction 0% (albite), 9.07% (olivine), and 32.5% (wollastonite) could be traced in 0.1 M BaCl2 extractions after correction for the baseline soil (Figure 2). Hence, only a small portion of the retarded base cations was adsorbed onto soil exchange sites, such as SOM and clay minerals (Weil and Brady, 2016), from which these base cations can be desorbed by exchange with 0.1 M Ba2+ ions. For albite, the limited contribution of soil CEC to the retarded fraction (Supplementary Table S2) may be explained by the low strength of adsorption of Na to reactive soil surfaces (Weil and Brady, 2016). However, the retarded fraction found for albite-Na (92.7%; Table 3) was substantially higher than expected based on the high mobility of Na. Given that incongruent albite weathering results in the formation of the non-Na-bearing kaolinite (Hartmann et al., 2013), we cannot yet provide an explanation for the nature of this apparently retained Na fraction. In addition, the quantified base saturation for the albite treatment is substantially lower than for the olivine and wollastonite treatments (Supplementary Table S2), despite fairly similar final pH values (7.30 for olivine, 7.93 for wollastonite, 7.42 for albite; te Pas et al., 2023) under which base saturation is expected to be approaching 100% (Magdoff and Bartlett, 1985). Together with the low contribution of Na to the overall CEC, this suggests that using 0.1 M BaCl2 may not effectively extract the retained Na, as was earlier observed in a study comparing methods for quantifying exchangeable base cations (Jönsson et al., 2002). Although our analyses indicate that the contribution of soil CEC to the retarded fraction of cations is small (Supplementary Table S2), the application of EW also increases soil CEC through the increase in soil pH and potentially via secondary mineral formation resulting from incongruent weathering (te Pas et al., 2023). Despite the limited contribution of soil CEC to the retarded fraction on the short term, the binding of weathered base cations to soil exchange sites may enhance over time as soil CEC increases.
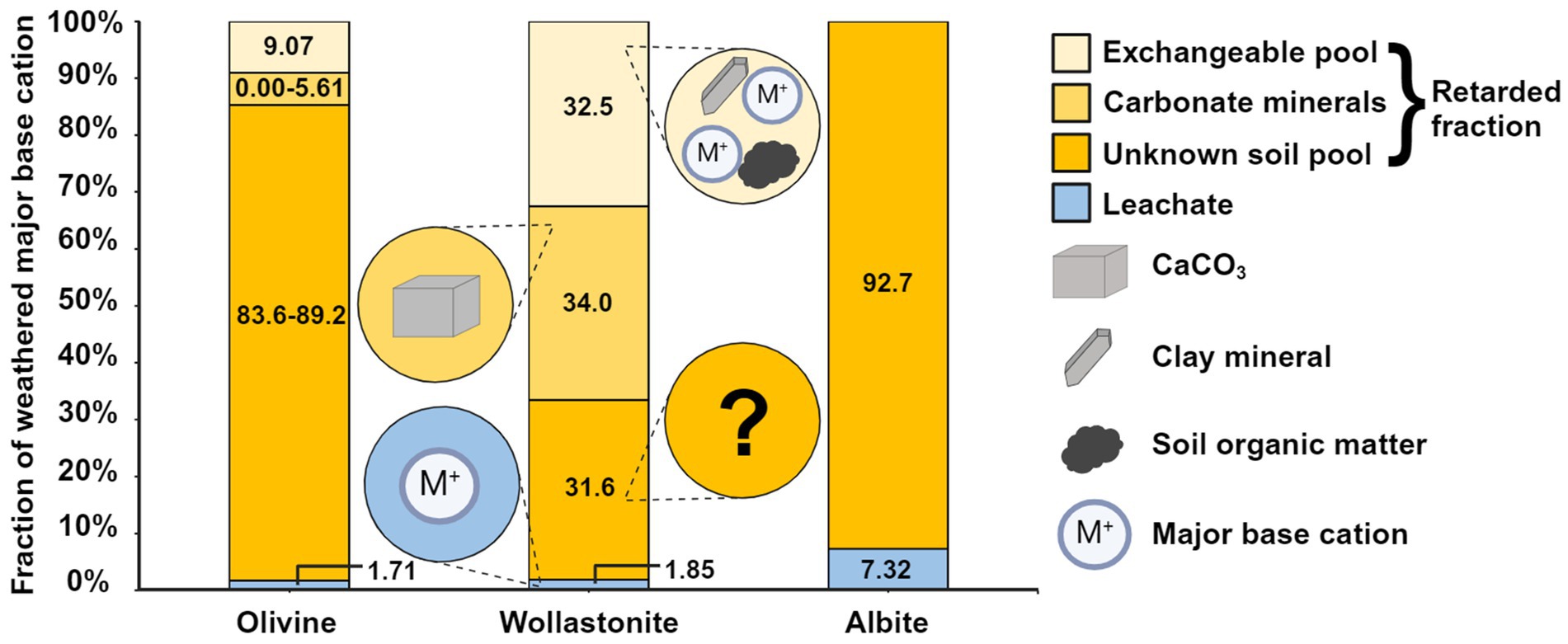
Figure 2. Fate of the weathered major base cation of the applied mineral, being Mg for olivine, Ca for wollastonite, and Na for albite, in the soil column. We quantified the distribution of the weathered base cations over the exchangeable pool (determined by a 0.1 M BaCl2 extraction followed by ICP-OES measurements), carbonate minerals (determined by Scheibler calcimetry), both corrected for the baseline soil (Supplementary Table S2), and the leachate (determined by ICP-OES measurements). The total weathered amount of each cation was determined as the sum of the concentrations measured with total fusion ICP-OES for the soil and ICP-OES for the leachate. The unknown soil pool was calculated as the difference between the total weathered amount and the quantified fates. The carbonate mineral fraction was calculated based on two assumptions: the formation of CaCO3 is most likely, and the major base cation of the applied mineral results in carbonate mineral formation, producing a range for the carbonate pool and the unknown soil pool for olivine and albite (as MgCO3 and Na2CO3 formation is unlikely, see Section 4.3). See Section 2.5 for details. Figure created in BioRender.com.
Another potential mechanism removing base cations from soil is CaCO3 formation, which is especially likely for wollastonite (Figure 2; Khalidy et al., 2023; te Pas et al., 2023). Based on Scheibler calcimetry, 34.0% of the retarded Ca was found to have precipitated as CaCO3 in the wollastonite treatment corrected for the baseline soil. Given that another 32.5% of retarded Ca for this treatment was bound to exchange sites, a third of the retarded base cations was retained otherwise (Figure 2). For olivine and albite, CaCO3 precipitation is more likely than the formation of MgCO3 and Na2CO3 due to their high solubilities, despite Mg and Na being the major base cations of the applied minerals. While the exact carbonate mineral formed is unknown, it is likely that Ca co-precipitated with other cations to form carbonate minerals (Li et al., 2020; Molnár et al., 2021; Mucci and Morse, 1983). Therefore, we calculated both the formation of CaCO3 and MgCO3 (olivine) or Na2CO3 (albite) based on Scheibler calcimetry results, producing a range for the fractions of the major base cations retained as carbonate minerals and in the unknown soil pool (Figure 2). For olivine and albite, the unknown soil pool is even larger (83.6–92.7%) relative to wollastonite. Ca, but also Mg, may be bound to reactive groups on organic compounds in the soil (Tipping, 2002), while several studies have shown that 0.1 M BaCl2 may not fully extract organically bound cations (Dauer and Perakis, 2013; Rosenstock et al., 2019). Other retention mechanisms not (fully) retrieved using a 0.1 M BaCl2 extraction may include incorporation in microbial biomass, adsorption into the interlayers of clay minerals such as vermiculite (Weil and Brady, 2016), and ternary complex formation of phosphate and Ca or Mg when binding to iron oxides (Mendez and Hiemstra, 2020). Considering that these mechanisms can (substantially) delay or potentially even hinder actual CDR generation on a range of timescales depending on the specific mechanism, we recommend further research on defining the underlying mechanisms through geochemical modeling and high-resolution microscopic and spectroscopic analyses. In addition, sequential extractions, as deployed by Niron et al. (2024), can provide insights on the fraction of weathering products that is readily exchangeable and the fraction that is bound to SOM, carbonate minerals and oxide minerals.
The likely involvement of weathered base cations in strong adsorption and/or mineral precipitation reactions in soil has important implications for the quantification of actual CDR and MRV. In current literature, authors often assume that the retention of weathered base cations within the soil profile is temporary with no effect on actual CDR, and therefore, corrections for the retarded fraction are overlooked (Beerling et al., 2024; Kantola et al., 2023; Reershemius et al., 2023). However, we show that this assumption cannot be made before progressing our mechanistic understanding of the fate of the retarded fraction within the soil. In conclusion, soil-based mass balance approaches can be used to quantify weathering rates and potential CDR; however, a correction for retarded weathering products through additional calculations as those shown here, and/or leachate-based TA titrations, is needed to constrain actual CDR for a given time and depth interval.
5 Conclusion and outlook
Here, we contributed to the development of a standardized quantification method of CO2 removal associated with enhanced weathering. The results of a recently published soil column experiment were complemented with total fusion ICP-OES analyses of base cation concentrations. Two soil-based mass balance methods were constructed and compared to leachate-based total alkalinity concentrations for soil columns treated with olivine, wollastonite, and albite. The calculated fractions of retarded weathering products were all nearing 100%, indicating that the majority of weathering products were not leached but retained within the soil profile during our short-term (64 days) experiment. Only after accounting for the retarded fraction, the CDR quantified with soil-based mass balance approaches was comparable to leachate-based TA concentrations. Further analyses of the fate of retarded weathering products within the soil showed that a small portion was precipitated as carbonate minerals (up to 34.0%) or adsorbed onto reactive soil surfaces (up to 32.5%). Hence, 31.6–92.7% of the retarded weathering products were retained otherwise in less-easily exchangeable pools, e.g., via mineral precipitation or strong binding to reactive soil surfaces. Considering that these reactions may substantially impact the upscaling of CDR associated with EW and the development of MRV systems, we recommend continued research on the fate of weathered base cations within the soil and accounting for the retarded fraction. Future studies should assess the validity of the calculation methods that we present to quantify the retarded fraction under spatially heterogeneous field conditions and in long-term studies. Furthermore, the fate of retarded weathering products that could not be traced in the exchangeable or carbonate mineral pools requires further research using sequential extractions, geochemical modeling, and/or microscopic and spectroscopic analyses. We conclude that soil-based mass balance approaches can be used to quantify weathering rates and potential CDR; however, the actual CDR realized over a given time and depth interval can only be constrained after accounting for the retarded fraction.
Data availability statement
The original contributions presented in the study are included in te Pas et al. (2023). Additional analyses are presented in this article/Supplementary material. Further inquiries can be directed to the corresponding author or found in the online data repository: https://doi.org/10.4121/68023c4b-7d87-499f-8cf9-537a68486e99.
Author contributions
EP: Conceptualization, Investigation, Methodology, Visualization, Writing – original draft. EC: Conceptualization, Investigation, Methodology, Visualization, Writing – review & editing. AM: Conceptualization, Investigation, Methodology, Visualization, Writing – review & editing. RC: Supervision, Writing – review & editing. MH: Conceptualization, Methodology, Supervision, Writing – review & editing.
Funding
The author(s) declare that financial support was received for the research, authorship, and/or publication of this article. This work was supported primarily through internal funding at Wageningen University & Research. Funding to support analytical costs and in-kind staff time contributions were made by Eion Corp. In-kind staff time contributions were also provided by Lithos Carbon and Terradot.
Acknowledgments
We thank the reviewers for their constructive feedback that improved the quality of this manuscript.
Conflict of interest
EC was employed at Eion Corp. and Lithos Carbon, Inc., both enhanced rock weathering companies operating in the United States, scaling terrestrial EW practices in agricultural systems.
The remaining authors declare that the research was conducted in the absence of any commercial or financial relationships that could be construed as a potential conflict of interest.
Generative AI statement
The authors declare that no Gen AI was used in the creation of this manuscript.
Publisher’s note
All claims expressed in this article are solely those of the authors and do not necessarily represent those of their affiliated organizations, or those of the publisher, the editors and the reviewers. Any product that may be evaluated in this article, or claim that may be made by its manufacturer, is not guaranteed or endorsed by the publisher.
Supplementary material
The Supplementary material for this article can be found online at: https://www.frontiersin.org/articles/10.3389/fclim.2024.1524998/full#supplementary-material
References
Allison, L. E. (1960). Wet-combustion apparatus and procedure for organic and inorganic carbon in soil. Soil Sci. Soc. Am. J. 24, 36–40. doi: 10.2136/sssaj1960.03615995002400010018x
Amann, T., Hartmann, J., Struyf, E., de Oliveira Garcia, W., Fischer, E. K., Janssens, I., et al. (2020). Enhanced weathering and related element fluxes – a cropland mesocosm approach. Biogeosciences 17, 103–119. doi: 10.5194/bg-17-103-2020
Beerling, D. J., Epihov, D. Z., Kantola, I. B., Masters, M. D., Reershemius, T., Planavsky, N. J., et al. (2024). Enhanced weathering in the US Corn Belt delivers carbon removal with agronomic benefits. Proc. Natl. Acad. Sci. 121:e2319436121. doi: 10.1073/pnas.2319436121
Beerling, D. J., Kantzas, E. P., Lomas, M. R., Wade, P., Eufrasio, R. M., Renforth, P., et al. (2020). Potential for large-scale CO2 removal via enhanced rock weathering with croplands. Nature 583, 242–248. doi: 10.1038/s41586-020-2448-9
Beerling, D. J., Leake, J. R., Long, S. P., Scholes, J. D., Ton, J., Nelson, P. N., et al. (2018). Farming with crops and rocks to address global climate, food and soil security. Nat. Plants 4, 138–147. doi: 10.1038/s41477-018-0108-y
Buckingham, F. L., Henderson, G. M., Holdship, P., and Renforth, P. (2022). Soil core study indicates limited CO2 removal by enhanced weathering in dry croplands in the UK. Appl. Geochem. 147:105482. doi: 10.1016/j.apgeochem.2022.105482
Clarkson, M. O., Larkin, C. S., Swoboda, P., Reershemius, T., Suhrhoff, T. J., Maesano, C. N., et al. (2024). A review of measurement for quantification of carbon dioxide removal by enhanced weathering in soil. Front. Clim. 6. doi: 10.3389/fclim.2024.1345224
Dauer, J. M., and Perakis, S. S. (2013). Contribution of calcium oxalate to soil-exchangeable calcium. Soil Sci. 178, 671–678. doi: 10.1097/SS.0000000000000029
Dietzen, C., Harrison, R., and Michelsen-Correa, S. (2018). Effectiveness of enhanced mineral weathering as a carbon sequestration tool and alternative to agricultural lime: an incubation experiment. Int. J. Greenhouse Gas Control 74, 251–258. doi: 10.1016/j.ijggc.2018.05.007
Gilbert, O., Hernández, M., Vilanova, E., and Cornellà, O. (2014). Guidelining protocol for soil-column experiments assessing fate and transport of trace organics. Brussels, Belgium: Demeau.
Groenenberg, J. E., Römkens, P. F. A. M., van Zomeren, A., Rodrigues, S. M., and Comans, R. N. J. (2017). Evaluation of the single dilute (0.43 M) nitric acid extraction to determine geochemically reactive elements in soil. Environ. Sci. Technol. 51, 2246–2253. doi: 10.1021/acs.est.6b05151
Haque, F., Santos, R. M., and Chiang, Y. W. (2020). CO2 sequestration by wollastonite-amended agricultural soils – an Ontario field study. Int. J. Greenhouse Gas Control 97:103017. doi: 10.1016/j.ijggc.2020.103017
Haque, F., Santos, R. M., Dutta, A., Thimmanagari, M., and Chiang, Y. W. (2019). Co-benefits of wollastonite weathering in agriculture: CO2 sequestration and promoted plant growth. ACS Omega 4, 1425–1433. doi: 10.1021/acsomega.8b02477
Hartmann, J., West, A. J., Renforth, P., Köhler, P., De La Rocha, C. L., Wolf-Gladrow, D. A., et al. (2013). Enhanced chemical weathering as a geoengineering strategy to reduce atmospheric carbon dioxide, supply nutrients, and mitigate ocean acidification. Rev. Geophys. 51, 113–149. doi: 10.1002/rog.20004
Houba, V., Temminghoff, E., Gaikhorst, G., and Van Vark, W. (2000). Soil analysis procedures using 0.01 M calcium chloride as extraction reagent. Commun. Soil Sci. Plant Anal. 31, 1299–1396. doi: 10.1080/00103620009370514
ISO 10693. (1995). Soil Quality -Determination of Carbonate Content -Volumetric Method. Geneva, Switzerland: International Organization for Standardization.
ISO 11260. (2018). Soil Quality -Determination of Effective Cation Exchange Capacity and Base Saturation Level Using Barium Chloride Solution. Geneva, Switzerland: International Organization for Standardization.
ISO 21268-3. (2019). Soil Quality -Leaching Procedures for Subsequent Chemical and Ecotoxicological Testing of Soil and Soil-Like Materials -Part 3: Up-Flow Percolation Test. Geneva, Switzerland: International Organization for Standardization.
IPCC (2018). Global warming of 1.5°C. Special report of the intergovernmental panel on climate change. Incheon, South Korea: Intergovernmental Panel on Climate Change.
IPCC (2023). Climate change 2023: synthesis report. Contribution of working groups I, II and III to the sixth assessment report of the intergovernmental panel on climate change. Geneva, Switzerland: Intergovernmental Panel on Climate Change.
Jönsson, U., Rosengren, U., Nihlgard, B., and Thelin, G. (2002). A comparative study of two methods for determination of pH, exchangeable base cations, and aluminum. Commun. Soil Sci. Plant Anal. 33, 3809–3824. doi: 10.1081/CSS-120015924
Kantola, I. B., Blanc-Betes, E., Masters, M. D., Chang, E., Marklein, A., Moore, C. E., et al. (2023). Improved net carbon budgets in the US Midwest through direct measured impacts of enhanced weathering. Glob. Chang. Biol. 29, 7012–7028. doi: 10.1111/gcb.16903
Kelland, M. E., Wade, P. W., Lewis, A. L., Taylor, L. L., Sarkar, B., Andrews, M. G., et al. (2020). Increased yield and CO2 sequestration potential with the C4 cereal Sorghum bicolor cultivated in basaltic rock dust-amended agricultural soil. Glob. Chang. Biol. 26, 3658–3676. doi: 10.1111/gcb.15089
Khalidy, R., Chiang, Y. W., and Santos, R. M. (2023). Fate and migration of enhanced rock weathering products through soil horizons; implications of irrigation and percolation regimes. Catena 233:107524. doi: 10.1016/j.catena.2023.107524
Lamboll, R. D., Nicholls, Z. R., Smith, C. J., Kikstra, J. S., Byers, E., and Rogelj, J. (2023). Assessing the size and uncertainty of remaining carbon budgets. Nat. Clim. Chang. 13, 1360–1367. doi: 10.1038/s41558-023-01848-5
Larkin, C. S., Andrews, M. G., Pearce, C. R., Yeong, K. L., Beerling, D. J., Bellamy, J., et al. (2022). Quantification of CO2 removal in a large-scale enhanced weathering field trial on an oil palm plantation in Sabah, Malaysia. Front. Clim. 4:959229. doi: 10.3389/fclim.2022.959229
Li, X., Zhang, Q., and Yang, B. (2020). Co-precipitation with CaCO3 to remove heavy metals and significantly reduce the moisture content of filter residue. Chemosphere 239:124660. doi: 10.1016/j.chemosphere.2019.124660
Magdoff, F. R., and Bartlett, R. J. (1985). Soil pH buffering revisited. Soil Sci. Soc. Am. J. 49, 145–148. doi: 10.2136/sssaj1985.03615995004900010029x
Mendez, J. C., and Hiemstra, T. (2020). Ternary complex formation of phosphate with ca and mg ions binding to ferrihydrite: experiments and mechanisms. ACS Earth Space Chem. 4, 545–557. doi: 10.1021/acsearthspacechem.9b00320
Mills, J. V., Sanchez, J., Olagaray, N. Y., Wang, H., and Tune, A. K. (2024). Foundations for carbon dioxide removal quantification in ERW deployments. Massachusetts: Cascade Climate.
Molnár, Z., Pekker, P., Dódony, I., and Pósfai, M. (2021). Clay minerals affect calcium (magnesium) carbonate precipitation and aging. Earth Planet. Sci. Lett. 567:116971. doi: 10.1016/j.epsl.2021.116971
Mucci, A., and Morse, J. W. (1983). The incorporation of Mg2+ and Sr2+ into calcite overgrowths: influences of growth rate and solution composition. Geochim. Cosmochim. Acta 47, 217–233. doi: 10.1016/0016-7037(83)90135-7
Niron, H., Vienne, A., Frings, P., Poetra, R., and Vicca, S. (2024). Exploring the synergy of enhanced weathering and Bacillus subtilis: a promising strategy for sustainable agriculture. Glob. Chang. Biol. 30:e17511. doi: 10.1111/gcb.17511
Pogge von Strandmann, P. A. E., Renforth, P., West, A. J., Murphy, M. J., Luu, T. H., and Henderson, G. M. (2021). The lithium and magnesium isotope signature of olivine dissolution in soil experiments. Chem. Geol. 560:120008. doi: 10.1016/j.chemgeo.2020.120008
Reershemius, T., Kelland, M. E., Jordan, J. S., Davis, I. R., D’Ascanio, R., Kalderson-Asael, B., et al. (2023). Initial validation of a soil-based mass-balance approach for empirical monitoring of enhanced rock weathering rates. Environ. Sci. Technol. 57, 19497–19507. doi: 10.1021/acs.est.3c03609
Rieder, L., Amann, T., and Hartmann, J. (2024). Soil electrical conductivity as a proxy for enhanced weathering in soils. Front. Clim. 5:1283107. doi: 10.3389/fclim.2023.1283107
Rosenstock, N. P., Stendahl, J., Van Der Heijden, G., Lundin, L., McGivney, E., Bishop, K., et al. (2019). Base cations in the soil bank: non-exchangeable pools may sustain centuries of net loss to forestry and leaching. Soil 5, 351–366. doi: 10.5194/soil-5-351-2019
Schuiling, R. D., and Krijgsman, P. (2006). Enhanced weathering: an effective and cheap tool to sequester CO2. Climate Change 74, 349–354. doi: 10.1007/s10584-005-3485-y
Smith, S. M., Geden, O., Gidden, M. J., Lamb, W. F., Nemet, G. F., Minx, J. C., et al. (2024). The state of carbon dioxide removal 2024 Available at: https://osf.io/f85qj/ (Accessed June 6, 2024).
te Pas, E. E. E. M., Hagens, M., and Comans, R. N. J. (2023). Assessment of the enhanced weathering potential of different silicate minerals to improve soil quality and sequester CO2. Front. Clim. 4:954064. doi: 10.3389/fclim.2022.954064
ten Berge, H. F. M., van der Meer, H. G., Steenhuizen, J. W., Goedhart, P. W., Knops, P., and Verhagen, J. (2012). Olivine weathering in soil, and its effects on growth and nutrient uptake in ryegrass (Lolium perenne L.): a pot experiment. PLoS One 7, 7:e42098. doi: 10.1371/journal.pone.0042098
Vienne, A., Frings, P., Poblador, S., Steinwidder, L., Rijnders, J., Schoelynck, J., et al. (2024). Earthworms in an enhanced weathering mesocosm experiment: effects on soil carbon sequestration, base cation exchange and soil CO2 efflux. Soil Biol. Biochem. 199:109596. doi: 10.1016/j.soilbio.2024.109596
Keywords: enhanced weathering, soil-based mass balances, leachate-based total alkalinity, olivine, wollastonite, albite
Citation: te Pas EEEM, Chang E, Marklein AR, Comans RNJ and Hagens M (2025) Accounting for retarded weathering products in comparing methods for quantifying carbon dioxide removal in a short-term enhanced weathering study. Front. Clim. 6:1524998. doi: 10.3389/fclim.2024.1524998
Edited by:
Ben W. Kolosz, University of Hull, United KingdomReviewed by:
Diego S. De Medeiros, Federal University of Rio Grande do Sul, BrazilMark Mba Wright, Iowa State University, United States
Copyright © 2025 te Pas, Chang, Marklein, Comans and Hagens. This is an open-access article distributed under the terms of the Creative Commons Attribution License (CC BY). The use, distribution or reproduction in other forums is permitted, provided the original author(s) and the copyright owner(s) are credited and that the original publication in this journal is cited, in accordance with accepted academic practice. No use, distribution or reproduction is permitted which does not comply with these terms.
*Correspondence: Emily E. E. M. te Pas, ZW1pbHkudGVwYXNAd3VyLm5s