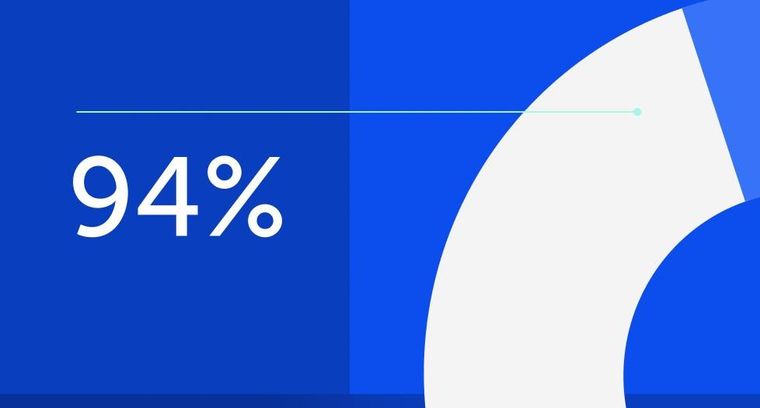
94% of researchers rate our articles as excellent or good
Learn more about the work of our research integrity team to safeguard the quality of each article we publish.
Find out more
PERSPECTIVE article
Front. Clim., 09 September 2024
Sec. Carbon Dioxide Removal
Volume 6 - 2024 | https://doi.org/10.3389/fclim.2024.1430957
This article is part of the Research TopicEnvironmental Engineering Perspectives on Ocean-Based Carbon Dioxide RemovalView all articles
There are many potential approaches to marine carbon dioxide removal (mCDR), of which ocean iron fertilization (OIF) has the longest history of study. However, OIF studies to date were not primarily designed to quantify the durability of carbon (C) storage, nor how wise OIF might be as an mCDR approach. To quantify C sequestration, we introduce a metric called the “centennial tonne,” defined as 1,000 kg of C isolated from atmospheric contact for on average at least 100 years. We present the activities needed to assess OIF from a scientific and technological perspective, and additionally, how it might be responsibly studied and potentially deployed. The five activities include: field studies in the Northeast Pacific; improved modeling for field studies, data assimilation and predictions at larger scales; improvements in monitoring, reporting and verification (MRV) for C, and also MRV for tracking ecological and environmental impacts; and developing new iron sources and their delivery, to increase efficiencies and reduce costs. The fifth activity is to understand whether public and community support exists for OIF, and what governance structures might support further research and possible deployment of OIF. This article is written by a multidisciplinary experts group called Exploring Ocean Iron Solutions (ExOIS) that is organized around a responsible code of conduct. Of the mCDR approaches, OIF has the potential to be low cost, scalable, and rapidly deployable. Reducing CO2 emissions must lead the way, but there is also an urgency to decide under what conditions and whether OIF might be deployed or not.
We need a new way of talking about global warming. As the UN Secretary General has said, the “era of global boiling” has arrived. Despite remarkable progress on a very complex problem over the past 30 years, much more effort is needed to keep the temperature increase to “well below” 2°C as specified in the 2015 Paris Agreement (UNFCCC, 2015). Climate models suggest that this next decade is critical to avert the worst consequences of climate change (IPCC, 2023). Humanity discharges about 37 billion tonnes of carbon dioxide into the atmosphere every year, which equates to about 10 billion tonnes of carbon (CO2:C ratio = 3.7). This release cannot continue unabated, so it is with a sense of urgency that the world must move to reduce greenhouse gas emissions and continue to find ways to adapt and build resilience. In addition, we will need to remove carbon dioxide (CO2) from the atmosphere. Given the ocean’s large capacity for carbon storage—more than 50 times larger than the atmosphere and 15–20 times larger than all land-based plants and soils—enhancing the ocean’s natural ability to store carbon should be considered.
There are many different approaches to marine carbon dioxide removal (mCDR), of which ocean iron fertilization (OIF) has the longest history of study and field testing (NASEM, 2022). Those studies confirmed that adding trace amounts of iron to some parts of the ocean is effective at stimulating phytoplankton growth, therefore supporting the iron hypothesis (Martin, 1990). Through enhanced photosynthesis, CO2 can be removed from the atmosphere, with a fraction of that transferred to the deep sea. However, studies to date were not designed to quantify how durable this storage can be, or how effective OIF might be as an mCDR approach.
The overall goal of the Exploring Ocean Iron Solutions (ExOIS, 2022) consortium is to conduct research to evaluate whether or not OIF is an efficient and responsible method of mCDR. A common metric for success for mCDR would be to achieve net increases in durable (>100 years) carbon storage in the deep sea that can reach Gt CO2 per year levels at a cost of less than $100 per tonne of CO2 sequestered. This must be done while avoiding unacceptable environmental, social, and other impacts.
Ultimately, ExOIS is seeking to establish open-source protocols for at-scale OIF mCDR, which if considered responsible and ethical, may accelerate its eventual implementation by private/public entities. This would require appropriate monitoring, reporting, and verification (MRV) protocols, going beyond just carbon accounting to assess ecological and other non-carbon environmental effects (eMRV). Because of concerns surrounding the ethics of mCDR, ExOIS is organized around a responsible and ethical code of conduct that prioritizes activities for the collective benefit of our planet with an emphasis on open and transparent studies that include engagement of the public, including Indigenous groups (Buesseler et al., 2022).
An important component of what we propose is the prioritization and integration of social science and governance research alongside field trials. These will necessarily involve activities both onshore and in the ocean—a global commons—with social and governance considerations and implications at both the domestic and international levels. Steps are needed to examine if, and under what conditions and configurations, OIF might be legally permissible and socially acceptable (Cooley et al., 2023). We also need to urgently understand the potential impacts of different possible OIF approaches to human communities and the resources that they depend on (e.g., fisheries, food security, incomes, livelihoods), as well as the intangible ways (e.g., esthetic, spiritual, cultural). This work must be fully integrated with the scientific and engineering work associated with OIF field trials.
This article is not a review of prior OIF studies, of which there are several (Boyd et al., 2007; Yoon et al., 2018), but a description of the research required to consider whether or not OIF provides an effective, durable, and safe approach for at-scale implementation of mCDR. Here we present the outcome of a workshop held at the Moss Landing Marine Laboratories in May 2023, which designed a path forward for OIF studies that includes: (1) field studies in the NE Pacific; (2) regional, global, and field study modeling; (3) testing various forms of iron and delivery methods; (4) advancing MRV and eMRV; and (5) advancing social science and governance. These activities are needed to accelerate our understanding of the suitability, efficacy, and impacts of OIF for mCDR.
A new generation of field studies are needed to fill the knowledge gaps and target uncertainties as to whether OIF is sufficiently effective, scalable, and reproducible for mCDR, and what the projected implementation costs would be. This requires field experiments that are significantly larger (>10 times larger spatial scales) and longer in duration (1 year instead of 1 month) than previous mesoscale OIF studies. A set of core measurements will be needed to quantify CO2 drawdown in the surface ocean, the re-equilibration timescales of atmospheric CO2 with the surface ocean, the sinking transport of C to depth, and the portion of this flux that results in carbon sequestration for 100 years or more (Supplementary Table S1). It will be critical that the ecological and environmental consequences of OIF field studies be given significant attention, and will require a combination of remote sensing, in-situ observations, and modeling. The field studies will need to be developed in collaboration with social and governance experts to ensure they are deployed with public engagement and in an equitable and ethical manner, with the appropriate social safeguards in place (see Section 6).
We primarily considered high nutrient low chlorophyll (HNLC) sites where the capacity for CO2 removal using OIF has been estimated at 1–4 Gt CO2/year (GESAMP, 2019; NASEM, 2022). Low nutrient settings also deserve attention, where Fe may stimulate N2 fixation and C drawdown over larger areas of the ocean (e.g., Karl and Letelier, 2008), but we start with what we know works. A summary of the baseline conditions and attributes of the sites considered is presented in Supplementary Tables S2, S3.
Eventually, OIF field studies will need to explore multiple settings, particularly the Southern Ocean where there is the greatest potential for carbon removal, but also the greatest political constraints. However, important next steps can and should be taken at sites that are less challenging from a logistical point of view. We consider the best location for the next OIF field studies to be within the NE Pacific. This region has low eddy kinetic energy, allowing for an iron-fertilized patch of seawater to remain coherent over timescales of several months (Figure 1). The depths to which carbon needs to sink to be sequestered for >100 years defines a metric we refer to as the centennial tonne (see Section 3). This depth is relatively shallow in the NE Pacific, around 500 m (Figure 2).
Figure 1. Proposed location of field studies in the NE Pacific near Ocean Station Papa (insert upper right panel). Prediction of consequences over time (30, 60, and 90 days) of adding 100 tonnes Fe to 100 × 100 km area, and the temporal progression of surface free Fe (nM), surface chlorophyll (mg m−3), POC flux at 200 m (mmol C m−2 d−1), and surface pCO2 drawdown (µatm) (panels left to right respectively). Net CO2 removal is predicted to be equivalent to 100,000–200,000 tonnes CO2 at 200 m (F. Chai personal communication).
Figure 2. Map showing the depth below which 50% of the carbon would be isolated from the surface ocean for at least 100 years. Adapted from a model in Siegel et al. (2021b).
Modeling suggests that drift of the patch center relative to the maximal signal in particulate organic carbon flux at 200 m will be small, 10–30 km, even after 90 days (Figure 1). Furthermore, the site is at the end of the large-scale ocean overturning circulation, and thus downstream impacts and nutrient robbing concerns (i.e., enhanced production leading to lower nutrients downstream; e.g., Matsumoto, 2006) are less than other ocean regions. Accessibility is good, given the location of US and Canadian ports. No island nations are in the proposed study region, though outreach and discussion with regional fisheries and coastal Indigenous groups would be important in the early stages of planning (Section 6).
In addition, there is a wealth of scientific baseline data, including the Canadian time series at Ocean Station Papa OSP (Freeland, 2007; Peña and Bograd, 2007), NOAA moorings at OSP (NASA, 2024), and field data from the recent NASA EXPORTS study (Siegel et al., 2021a), among others. These baseline data will help in experimental planning and permitting under the London Convention and Protocol (Section 6). Finally, we know from experience that adding iron can stimulate diatom-dominated communities and export in this region (Supplementary Tables S2, S3; SERIES; Boyd et al., 2004).
Prior experiments used ship-based deployment of iron (II) sulfate (FeSO4) (Boyd et al., 2007), along with the inert tracer SF6 added to facilitate short-term tracking of the iron-amended patch. The key advantage of FeSO4 is the existing evidence of its efficacy in stimulating blooms in multiple HNLC regions. A disadvantage of FeSO4 is its rapid (temperature dependent) oxidation to insoluble iron (III) species and subsequent rapid precipitation and loss from surface waters. New iron forms and iron delivery methods are being proposed to improve the C:Fe export efficiency (Section 4) but these remain in early experimental stages and are too premature to be selected for application at the proposed scales. In the meantime, we can use FeSO4 to study variation of patch size, fate, and duration of FeSO4 delivered and improve our MRV capabilities.
Prior in situ surface iron amendments were conducted at initial spatial scales from 25 to 300 km2 and observed over 2–6 weeks (Yoon et al., 2018). We propose a much larger study area of >1,000 to 10,000 km2. The larger scale will reduce the impact of lateral mixing processes relative to the size of the bloom, which can lead to dilution and patch heterogeneity (Boyd et al., 2007). This dilution reduces particle aggregation rates and the potential for C export (e.g., Jackson, 2008). Lateral mixing is important to consider for bloom development when scaling up, both mixing iron and phytoplankton out of the patch, and resupplying macro-nutrients (e.g., silicate) into the bloom (Abraham et al., 2000). Entraining macro-nutrients into the bloom reduces the potential for co-limitation, which could otherwise terminate the bloom at larger scales. However, observations show that large natural systems generate higher C export efficiencies (see Section 4 and discussion on natural systems summarized by De Baar et al., 2008), which would be more representative of potential mCDR deployments at scale. A larger patch size also improves the ability to robustly detect C export because subsurface assets like sediment traps (moored or neutrally buoyant), gliders, or other technologies (e.g., BGC-Argo floats, Boyd et al., 2023) are less likely to miss or under-sample export pulses.
Preliminary modeling experiments indicate that a 100 × 100 km patch size in the NE Pacific would result in an additional C export at 200 m equivalent to 100,000–200,000 tonnes CO2 after 90 days (Figure 1). Patch tracking will be accomplished through co-deployment of the inert tracer SF6 (or its replacement SF5CF3; Ho et al., 2008), and as this signal degrades, patch tracking would continue with measurements of biogeochemical proxies, such as chlorophyll, particle concentrations, CO2 levels, nutrient drawdown, and dissolved iron, as measured using ships, remote sensing and an assortment of autonomous vehicles (AVs) (e.g., Law et al., 2006; Boyd et al., 2007; Yoon et al., 2018). We acknowledge the fact that these inert tracers are themselves potent greenhouse gasses (GHGs), however the relative amounts to be used for OIF experiments would be so small as to have a negligible impact on earth’s climate. We estimate that about 10 kg of SF5CF3 would be enough for a 100×100 km experiment in the NE Pacific, and would have the equivalent warming potential as about 180 tonnes of CO2, or about 4.5 billionths of annual anthropogenic emissions (Sturges et al., 2000; Law et al., 2006). This is about 0.1–0.2% of the additional CO2 removal expected by a single experiment of this size. In addition to tracers, seeding of multiple inexpensive surface floats across the patch can be used for tracking water movements, and would be an additional way to access patch location over longer and larger scales after the SF6 signal is lost (e.g., Niiler et al., 1995).
Carbon must be transported below the depth horizon of the main thermocline (pycnocline), as otherwise winter mixing will allow it to return to the atmosphere. In addition, any drawdown of CO2 needs to consider not just how much dissolved CO2 decreases in the surface, but how quickly the surface waters re-equilibrate with the atmosphere prior to sinking (Bach et al., 2023). A combination of observations on seasonal to annual timescales, and modeling for longer timescales, are thus essential for field studies and MRV for carbon and eMRV (Section 5; Boyd et al., 2023).
OIF will have substantial ecological and biogeochemical consequences, some of which (e.g., increased diatom production) are intended and well known, and can be considered positive. Other outcomes are less well defined, and potentially undesirable. Monitoring, reporting, and verification of these environmental and ecological effects (eMRV) is as important as MRV for carbon accounting. This would hold for any mCDR approach, and will differ for OIF depending upon the location, seasonality, and scale of deployment. Some of the potential negative impacts at the surface of at scale OIF include macronutrient removal, and detrimental shifts in plankton and secondary ecosystems. In deeper waters and at the seafloor, the consequences of remineralization of sinking organic C include reductions in oxygen, decreases in pH, and production of other GHGs (e.g., N2O, CH4) (Levin et al., 2023). These eMRV variables have been measured in several prior OIF field studies and have not shown side effects that would negate GHG consequences or cause permanent changes to local ecosystems (summarized in NASEM, 2022). Furthermore, studies of natural ocean iron sources have not observed, nor reported, negative consequences (e.g., harmful algal blooms (HABS), dead zones) in iron enriched ocean regions (e.g., Blain et al., 2007; Pollard et al., 2009). Nevertheless, it is essential that eMRV be conducted to evaluate whether this holds for longer term OIF implementation (Section 5).
To make progress, we need to conduct multiple OIF field studies in the NE Pacific. The priorities would be to: (1) create and track a large bloom; (2) track the export and fate of additional C flux to a depth where C is sequestered on time scales >100 years (MRV for C); (3) assess the ecological and environmental shifts (eMRV); (4) use new observations to improve MRV models and evaluate new MRV technologies; (5) use field data and models to access scalability, costs and climate impacts; and (6) use knowledge gained to optimize mCDR potential in the future.
Field planning must be conducted transparently, with participation by multiple international groups. Other groups would be encouraged to join at the same site for additional observations. Projects would be designed to fully comply with all applicable legal requirements and ensure opportunities for public comment and respect of sovereign national concerns and customs. Major findings would be presented in preliminary format at scientific gatherings, and in short form to the public and funders. Data would be made publicly available in a timely fashion and would be used to better inform the models needed to evaluate the consequences of OIF deployment on climatically relevant scales. Peer review would be encouraged for all findings from the field studies. From permitting, to rights-holders, to public engagement, integration of social science and governance research alongside field trials is needed and discussed in Section 6.
The ultimate goal of the field work is to establish open-source protocols that would be needed if implementing OIF at scale, that can be assessed with a set of standard MRV protocols. Given that ecosystems will be altered by OIF—indeed that is the purpose—it will be critical to establish guidelines that identify thresholds for acceptable change, beyond which will lead to a decision to halt the experiment. Although this next generation of field studies will be at larger scales than prior OIF experiments, we should be clear they are far smaller than natural OIF events. Showing the public and others that these studies can be done in a responsible way that minimizes and manages risks of undesirable impacts is key if OIF is to be a viable and acceptable climate change mitigation tool.
Modeling will be needed for experimental planning, to synthesize field trial results, and to predict and extrapolate results to regional and global scales, which will be required to evaluate C impacts, climate effects and overall changes to the ocean C cycle and ecosystem. We break down these modeling efforts into a series of related activities below, with models specific to MRV discussed in Section 5.
OIF efforts require models with realistic representations of physical, biogeochemical and ecosystem processes. Physical model components are grounded in well-established primitive equations, which are solved at various spatial scales relevant to OIF efforts. These range from (sub)mesoscales for processes occurring at the immediate site of OIF deployments, to basin scales for downstream impacts, to the global scale for carbon sequestration and durability. In contrast, ocean biogeochemical models differ significantly in their complexity, structure, and parameterizations. Most rely on a functional group approach (Quéré et al., 2005), representing the interaction of fundamental ecosystem components with the cycles of carbon, nutrients, and other elements. This approach has been successful in reproducing present-day carbon cycle and ecosystem processes—typically focusing on lower-trophic levels and surface ocean processes. Biogeochemical models have also been used to generate projections under future climate scenarios, albeit with significant inter-model variability (Kwiatkowski et al., 2020). In principle, most of today’s models can be used to assess a variety of OIF impacts, from carbon air-sea fluxes, export, and sequestration, to nutrient robbing and oxygen loss. However key impacts such as harmful algal blooms and marine ecosystem feedbacks to climate are not well modeled. In addition, many processes that influence the biological carbon pump are not well parameterized in models, and thus predictions about the magnitude and change of C flux in the future are widely variable (Henson et al., 2022).
Accurate representation of iron cycling processes is essential to model the effects of OIF on marine ecosystems and carbon sequestration. The cycle of iron in natural seawater is distinct from that of other nutrients due to the extremely low iron concentration in dissolved form and complex interactions with particulate matter (Boyd and Ellwood, 2010; Tagliabue et al., 2017). One main target for models is to capture the correct residence time of dissolved iron in the ocean (Tagliabue et al., 2016). A rapid, tight balance between sources and sinks of iron leads to one of the shortest residence times among the nutrients required by phytoplankton.
A diversity of iron sources and sinks complicates modeling efforts. Among the sources, atmospheric deposition (Duce and Tindale, 1991), release from sediment (Johnson et al., 1999), and river runoff (Rijkenberg et al., 2014) are the main iron sources in surface waters. At high latitudes, inputs from icebergs, glaciers (Raiswell et al., 2008), and sea ice meltwater (Lannuzel et al., 2016) can become important, and there is evidence to suggest a source from submarine groundwater discharges (Windom et al., 2006). Hydrothermal vents also release stabilized dissolved iron into the water column at depth (Bennett et al., 2008). The uncertainty is large enough that models differ significantly in the total input of iron to the ocean, ranging from as low as 1.4 to as high as 195.4 Gmol per year (Tagliabue et al., 2016). To further complicate the picture, iron introduced by different sources is characterized by a range of solubilities and, ultimately, bioavailability (Shaked et al., 2020).
In parallel, multiple processes remove bioavailable iron from seawater. The oxidized form Fe(III) dominates in the ocean, but is characterized by sub-nanomolar solubilities, and thus quickly aggregates and precipitates (Wu et al., 2001). Dissolved iron is rapidly incorporated into marine particles by biological uptake and abiotic scavenging (Tagliabue et al., 2017). Chemical complexation enhances iron solubility in seawater, and ligand-bound iron represents most of the bioavailable iron in the ocean (Gledhill, 2012). Furthermore, iron incorporation into phytoplankton and zooplankton spans a wide range of cellular quotas (Sunda and Huntsman, 1997; Twining and Baines, 2013), and consumption and recycling by bacteria, viruses, and zooplankton complicate the picture (Strzepek et al., 2005).
These diverse and intertwined processes pose a significant challenge for models. For example, if ligand production is tied to organic matter synthesis and remineralization (Boyd et al., 2010; Pham and Ito, 2019), purposeful OIF may stimulate ligand release, enhancing iron retention (a positive feedback) (Maldonado et al., 2001). On the other hand, if ligand production by phytoplankton is higher under iron-limited conditions (Völker and Ye, 2022), it may be reduced by OIF, limiting its effectiveness (a negative feedback). Likewise, increased iron precipitation and scavenging at higher iron concentrations, including by slowly sinking authigenic particles (the colloidal pump, Tagliabue et al., 2023a), could provide negative feedbacks on OIF.
The effectiveness of any sampling strategy is ultimately determined by the accuracy with which the observations can be used to reconstruct reality—the state of the natural system being measured. In this context, reality is an elusive metric, for property distributions in the ocean rarely (if ever) have been oversampled. Given the dearth of opportunity for testing sampling strategies against objective criteria with purely observational means, OSSEs offer an attractive framework for investigation of these issues (e.g., McGillicuddy et al., 2001; Kourafalou et al., 2016; Gwyther et al., 2023).
The approach begins with constructing a simulation characteristic of the natural system. The model run serves as a representation of reality, which is then subsampled in a specified fashion to produce a simulated dataset and synthesized into a reconstruction of reality. Comparison of the reconstructed field with the “truth” as defined by the original simulation thus provides a quantitative evaluation of that sampling strategy. Of course there is an important caveat: the OSSEs are based on simulations that are an imperfect representation of the real world.
OSSEs will be needed to determine the observing system requirements for field trials. For example, what is the resolution needed in observational networks in three-dimensions, and over what time scales? Future OIF studies will increasingly involve autonomous floats such as BGC-Argo (Claustre et al., 2020) and gliders (Chai et al., 2020), and their deployment scheme is key to the next generation of longer/larger for field studies.
We are calling OSSE-designed observing systems for OIF “Ocean Iron Fertilization Observatories” (OIFOs). It is important to recognize that such OIFOs must be put in place in advance of the iron fertilization field trial and persist for some time afterward, the timescale of which will be guided by OSSEs when designing the experiment, but in essence would need to capture the pre-OIF conditions through to the end of winter mixing.
Carbon accounting of an OIF CDR action requires an assessment of both the amount of atmospheric carbon sequestered as well as the timescale that the carbon remains within the deep ocean. For a passive tracer injected within the ocean, there will be a distribution of contact times (Siegel et al., 2021b). This is because there are an infinite number of paths connecting a given location in the ocean interior to anywhere at the sea surface.
Durability assessments can be based upon steady-state assumptions of present-day ocean circulation processes (DeVries et al., 2012; Siegel et al., 2021b, 2023), or they can use time-dependent circulation reflecting various climate change scenarios (Baker et al., 2022). The depths mapped out in Figure 2 also assume that air-sea gas exchange occurs instantaneously (Siegel et al., 2021b). However, in much of the ocean, the carbonate system equilibration time can be many months to several years, and circulation and mixing process can limit the time that the upper ocean is in contact with the atmosphere (Bach et al., 2023; Nowicki et al., 2024). Both of these processes will reduce initial in-gassing of atmospheric CO2, especially in high latitude regions where deep seasonal mixing and slow CO2 system equilibration rates are found (Ito and Follows, 2013; Nowicki et al., 2024).
Overall, the efficacy of OIF as a climate change mitigation strategy can be conceptualized along axes of additionality and durability (Figure 3). We introduce a metric we call the centennial tonne, defined as 1,000 kg of carbon isolated from atmospheric ventilation for on average at least 100 years. By virtue of the profile of remineralization of sinking particulate material in the ocean (the so-called Martin curve, Martin et al., 1987), that centennial tonne of carbon will be part of a continuum of higher fluxes in the upper water column that decrease at greater depths, with correspondingly lower durability in the upper water column, and higher durability at depth. The rationale for a century timescale is pragmatic and based on the anticipation of carbon markets and durability around carbon credits and climate solutions. Note that removal does not have to be permanent for mCDR to be an effective tool in reducing human and environmental losses due to climate change.
Figure 3. Conceptual diagram related to the durability and uncertainty around measuring the “centennial tonne” of carbon. This concept requires observations of additional carbon flux to depth (y-axis) and model determinations of durability (x-axis). In this manner, intersection of the 100 year time scale and a given C flux (green shading) would give an estimate of how many, or X centennial tonnes of C were sequestered. Uncertainties around this estimate can be predicted as shown by the blue shading. Note this curve would vary with time and location in response to OIF and it is the integral of these measurements over the deployment area that is required to determine net additional and durable carbon storage. Also, adjustments for surface CO2 exchange would be needed (e.g., Bach et al., 2023) to determine net impacts of OIF on atmospheric CO2.
Uncertainty in the centennial tonne grows with decreasing additionality (shaded area in Figure 3), as smaller fluxes are more difficult to measure and distinguish with certainty against a variable background. Uncertainty also grows with increasing durability, as longer timescales are less well constrained in ocean biogeochemical models. One outcome of the models and observations will be quantifying and putting uncertainties on our centennial tonne benchmark. Also, adjustments for surface CO2 exchange would be needed (e.g., Bach et al., 2023) to determine net impacts of OIF on atmospheric CO2.
Because of structural model differences, even models calibrated to reproduce current conditions may differ in their response to perturbations. This variability is exemplified by divergent model projections of primary production and carbon export under climate change (Laufkötter et al., 2015; Henson et al., 2022). Such structural uncertainty likely extends to the models’ responses to OIF. Within this context, model intercomparison projects (MIPs), will be key to addressing our ability to robustly simulate OIF responses.
Successful MIPs are structured around well-defined scientific questions and require coordinated efforts among participating modeling groups and rights-holders (Eyring et al., 2016). The last iron model intercomparison project (FeMIP) was conducted nearly a decade ago (Tagliabue et al., 2016), and since then, there has been significant progress in model formulations and skills (e.g., Black et al., 2020; Somes et al., 2021; Tagliabue et al., 2023a). Moreover, FeMIP primarily focused on the natural iron cycle in global models and did not target OIF interventions.
Key to a successful OIF-MIP effort will be coordinated simulations under a common OIF scenario, conducted using structurally different models, and potentially including a comparison of responses at different scales, from local, to regional, to global. OIF scenarios could include (1) past purposeful OIF experiments enabling an evaluation against observed responses (e.g., Yoon et al., 2018), and (2) future OIF experiments exploring a range of planned locations and Fe delivery strategies.
We argue that a new, coordinated OIF-MIP would provide a variety of benefits, including: (1) quantification of the uncertainty arising from model structure; (2) community consensus on the design, set up, and diagnostics of OIF simulations (i.e., standardization); (3) increased consensus toward planning OIF field experiments; (4) multi-model output available for OSSE (addressing structural uncertainty); (5) identification of gaps and directions for OIF model refinement; (6) advancement in modeling technology, including toward operational use and data assimilation for MRV applications; and (7) increased collaboration and transparency of OIF efforts.
A remarkable diversity of iron sources has been proposed for OIF with differing advantages and disadvantages and state of knowledge. The goal is to optimize iron solubility, bioavailabilty, persistence and ecological compatibility. As noted earlier, FeSO4 has been used in past field studies, but several exciting new approaches (Supplementary Table S4) include engineered nanomaterials (Babakhani et al., 2022), release of Fe electrochemically from floating platforms (Taqieddin et al., 2024), to lower cost methods that use buoyant rice husks (such as shown for dissolved silica; Shetye et al., 2023), and even additions such as clay materials, to enhance export of C via aggregation and ballasting (Sharma et al., 2024).
A range of delivery methods have been proposed as well. These range from the traditional approach of pumping dissolved iron from a vessel, to sprays of aerosolized liquid or powder, to electrochemical dissolution of metal plates. Methods and materials will each be suited to different craft for deployment, from autonomous gliders to boats to airplanes, with each method needing its own environmental, ethical, and social evaluation.
The efficiency of iron fertilization for previous mesoscale OIF experiments has generally been low, with estimates suggesting that only ≈1% of added iron was required to stimulate additional surface biomass (Bowie et al., 2001; Yoon et al., 2018). Much of the added iron is quickly converted to particulate forms and is lost to sinking before it can be used by phytoplankton. Some of the added iron is bound to weak or non-bioavailable ligands causing it to precipitate before biological uptake can occur. Phytoplankton may also take up more iron than needed (so called “luxury uptake”; Twining et al., 2004).
Evidence from natural OIF suggests that much higher iron fertilization efficiencies should be possible. Sustained releases of iron into the Southern Ocean from islands, wildfire ash, and volcanic ash have all led to larger increases in C productivity and/or export per amount of Fe added than prior OIF experiments (Blain et al., 2007; De Baar et al., 2008; Pollard et al., 2009; Hamme et al., 2010; Tang et al., 2021). The biological C:Fe ratio for uptake into Southern Ocean phytoplankton is greater than 20,000 on a per mole basis (Twining et al., 2004), yet the typical C:Fe ratios of sinking phytoplankton can be lower (Frew et al., 2006), suggesting that much iron can sink without bringing carbon with it (Twining et al., 2014). In warmer regions, the opposite seems to be true, with phytoplankton showing the capacity to recycle iron for growth in the surface ocean even while driving the downwards export of macronutrients and carbon (Rafter et al., 2017; Hawco et al., 2022). A key factor in the efficiency of natural OIF may be the gradual and continuous addition of iron over extended time periods, which allows phytoplankton blooms to sustain high rates of growth until the phytoplankton reach a high density and aggregate, promoting sinking into the deep ocean.
Initial tests should seek to determine iron solubility in the presence and absence of biology (Fe-limited phytoplankton and zooplankton grazers), both because of the potential for microbial solubilization (e.g., production of organic ligands and siderophores), and because microbial production of particles may seed aggregation of inorganic iron colloids (Tagliabue et al., 2023a). In addition to solubility, biological assays can be used to test for iron bioavailability, such as the ability of added materials to relieve iron limitation in cultured and natural phytoplankton communities. Another key factor is iron persistence. Some materials are designed to release iron slowly over time, providing a continual source of new dissolved iron to the oceans, while other materials provide a rapid one-time iron release. The persistence of iron is certain to depend on both the chemical and biological activity of seawater, and thus experiments will need to involve organic ligands, living phytoplankton, and microbial communities. Finally, to achieve social license, the sourcing and processing of iron must have minimal side effects and demonstrated ecological compatibility.
Like a bake-off among pastry chefs, we recommend that a series of land-based experiments of increasing difficulty and complexity be conducted, with only the highest-performing “contestants” taken forward to the final, most complex experiments. This intercomparison would be the first step to develop protocols and test a broad group of natural and potentially engineered Fe minerals and materials with potential for eventual deployment at scale, allowing a wide diversity of approaches to be compared. Secondly, the core activity of the bake-off would be moving experiments toward more naturalistic conditions. Recently developed 110 L PERIcosms (Pelagic Ecosystem Research Incubators) are designed to incubate complex, diverse, natural ocean microbial communities for timescales of weeks to months in trace-metal clean conditions (Seelen et al., 2024). PERIcosms are designed with side ports for sampling the dissolved phase and a sediment trap to collect sinking material. We envision a series of PERIcosm experiments taking place, on shore or at sea, using natural ocean water from HNLC regions and other locations, enabling testing in naturalistic ocean conditions. Ultimately, the goal is to identify material that most effectively stimulates phytoplankton growth and C export, while also enabling technical feasibility and cost effectiveness for deployment at large scales. Using large floating mesocosms at sea is another option that might be considered (e.g., Böttjer-Wilson et al., 2021), though even these scales of containment (on order 60 m3) are both logistically difficult to deploy and cannot track full ecosystem responses over multiple months and larger scales needed to assess OIF efficiencies and impacts.
As we move beyond pilot studies and narrow down choices of the form of iron, larger scale engineering of how iron (liquid or solid) could be most efficiently delivered would be needed. The costs to fully implement such delivery will depend upon the scale of the field studies, but certainly testing and engineering of systems to deliver hundreds of tonnes of iron will be needed. Systems engineering to design these would require dedicated engineering teams working with OIF iron specialists. Along with optimizing the form of iron, the amount added, and rate of introduction are also important variables that control the growth response and mCDR consequences. The impact of varying iron concentrations on phytoplankton growth and carbon sequestration has not been tested from the perspective of maximizing carbon export. In addition, regarding the timing of delivery, previous natural and deliberate OIF events suggest that adding iron slowly over a long period of time may lead to the greatest increases in phytoplankton biomass. Finally, we note that all the metrics discussed above are likely to be differentially expressed in different biological regimes. Thus, it will be important to test materials and methods in a variety of settings.
The aim of this work is to quickly determine the handful of methods that are most promising for OIF. These methods should be relatively inexpensive, have a low carbon footprint and low propensity for other negative environmental side effects, and be highly efficient in terms of shuttling carbon into the deep ocean. Developing OIF should involve and engage scientists from across the globe, and testing the various forms of iron provides a key opportunity to develop international collaborations early. In addition to testing the Fe-rich materials in Supplementary Table S4, testing of soils as substrates for iron addition is a pathway to engage scientists from numerous countries. Depending upon the chosen form of Fe, delivery methods could be optimized and would include air or sea based AV’s. These selected methods would then be integrated into the larger Phase II field studies (Section 7).
Monitoring, reporting, and verification (MRV) of the effects of ocean iron fertilization activities are of key importance, not just for OIF, but for any mCDR study and eventual deployment. MRV activities need to assess the additionality, leakage, and durability of carbon uptake, while eMRV activities assess the ecological and environmental impacts. For MRV, additionality refers to the amount of carbon uptake that can be specifically attributed to the OIF intervention, relative to a baseline or control scenario with no intervention. Leakage here refers to the loss of sequestered CO2 back to the atmosphere. Durability refers to the timescale that the atmospheric CO2 taken up by the OIF action remains within the ocean. Examples of some of the key technologies from MRV are shown in Supplementary Figure S1.
If OIF is to be conducted at scale as a CDR solution, the MRV of any OIF action almost assuredly will involve using data-assimilating models. Models are required as it is not possible to fully observe additionality, leakage, durability, and desired and undesired environmental effects over the required spatial areas and time periods, which range from regional to global and months to centuries, respectively. This necessity is common to all mCDR methods and has been discussed extensively in the literature (e.g., Bach et al., 2023; Ho et al., 2023; Palter et al., 2023). If OIF is to receive the social support to be conducted at scale, these models must provide not only information for carbon accounting of the OIF action (MRV), but also an assessment of the ecological and biogeochemical impacts of the OIF action (eMRV). Social research (see Section 6) will be needed to understand how people view the consequences of our current path of climate induced damage relative to the consequences of mCDR, which if successful, would lower atmospheric CO2.
Local (km) to basin (1000’s of km) scale MRV assessments will require data-assimilative model systems, based upon the OIF models and OIFOs designed with OSSEs at a much larger scale. These modeling systems need to assimilate regional-scale data as well as appropriate local-scale observations to assess both the critical carbon flows for MRV, but also the ecological and biogeochemical impacts needed for eMRV. Field studies will provide the intensive data needed to develop and validate these coupled MRV/eMRV modeling systems for OIF. Most importantly, these initial field studies will help identify the most important data observations needed to accurately and economically assess the efficacy and impacts of OIF CDR when or if conducted at scale. This process of iterative model tuning to observed data from OIF pilot studies will also provide bounds for model prediction uncertainty needed to help value carbon credits or other instruments in financial markets.
MRV will require measurements of carbon fluxes and key biological properties that influence additionality (how much C export is caused by OIF), negative ecosystem feedbacks (such as production of other greenhouse gasses), and durability (the interaction between circulation and the remineralization depth of the exported carbon). During the next generation field experiments, these measurements may be constrained through intensive observations rather than models (with the data used for both method and model development and validation). These C fluxes include: net organic carbon flux out of the surface layer via the biological pump, with an emphasis on the gravitational pump as it is the dominant pathway for C sequestration on longer time scales, i.e., durability (Boyd et al., 2019); net air-sea CO2 flux into the surface layer before it subducts; and the carbon remineralization rate profile below the surface layer, to determine the variations in C, N, P, bSi (biogenic silica), and Fe remineralization length scales required to properly assess long term and far field impacts.
Organic carbon fluxes and carbon remineralization rates can be observed via several methods, some ship-supported and others autonomous (Supplementary Table S5; Supplementary Figure S1). There are no standards for these measurements and careful intercalibration, especially for emerging autonomous techniques that could be used for large-scale MRV model validation, will be a critical task during the initial field experiments. Remote sensing will allow for temporal and spatial sampling of many biological properties and physical conditions in the surface (Westberry et al., 2013). To determine the additionality, all parameters need to be constrained both at the treated site and at unamended control sites with similar characteristics, and will be managed by deploying an appropriately sized OIFO array for a given iron-amended patch.
The assessment of unpredictable ecological impacts will require broader, less-targeted measurements of community composition and biomass distributions. These should include any commercially or socially valuable species, as determined by social science research and engagement accompanying field trial planning, in addition to lower trophic levels. This set of eMRV measurements is less targeted than those required for MRV because of the need to identify unexpected changes, but shifts that include HABs are particularly of interest and relatively easy to monitor.
Biogeochemical shifts inside and outside of the iron-amended region will require measurements that can resolve decreases in subsurface O2, increases in subsurface CO2 (which can lead to hypercapnia and acidification), increases in non-CO2 greenhouse gasses (N2O, CH4), production of dimethyl sulfide, and consumption of limiting nutrients other than iron, including macronutrients and other key trace metals (some of which may be co-limiting with iron). In particular, upon alleviation of iron stress, the consumption of other nutrients may have impacts on downstream productivity elsewhere in the ocean (e.g., Oschlies et al., 2010; Tagliabue et al., 2023b), which over most timescales will need to be simulated with a modeling approach. The depth dependencies of nutrient consumption and remineralization, and the carbon-to-nutrient stoichiometries of exported organic matter, are thus key eMRV observational requirements (Supplementary Table S5). Measuring profiles vs. depth of water properties are key to parameterize remineralization in models to determine the downstream and regional impacts.
In addition to the specific modeling and observational requirements for MRV and eMRV, there are general needs that apply to both. Key developments for MRV technologies are summarized in Supplementary Table S5. Distributed measurements at scale for assimilation into models will require suitable sensors on autonomous sampling platforms. Decreases in platform cost, renewable power sources, recoverability, and ability to log more complex data streams and perform on-board data reduction would be helpful. Another early opportunity for method refinement would be the intercalibration of existing methods, for both geochemical parameters and for assessment of biological community composition.
MRV observations must accompany all OIF field activities. For some parameters there are no automated, scalable observational methods, and so traditional ship-supported observations will be required. These will serve a dual purpose—first, to support decision making about the efficacy and wisdom of OIF, and second, to provide intercalibration opportunities for emerging, scalable MRV technologies. Many of the MRV and eMRV requirements are not unique to OIF. Investments in new sensors for MRV for C (e.g., ARPA-E SEA-CO2 Program, 2023), and encouraging instruments to measure ecological shifts and non-C parameters are needed for all mCDR approaches.
As discussed above, OIF field trials must be accompanied by extensive social science and governance research. Given OIF’s legacy of historical controversies (Kintisch, 2009; Buck, 2018), it will be important to explore how OIF is governed under international and domestic law, as well as its social support for further research and any kind of deployments. It will also be essential to involve public groups in discussions regarding about whether, where, when, under what conditions, and at what scale OIF might be responsibly implemented. The following section describes key components of this research agenda.
Understanding public perceptions of OIF is essential (Cooley et al., 2023). Existing work to date suggests that OIF is not favorably viewed by many public groups (Ipsos MORI, 2010; Bostrom et al., 2012; Amelung and Funke, 2015; Bertram and Merk, 2020; Jobin and Siegrist, 2020; Shrum et al., 2020; Nawaz et al., 2023). While this research is limited in geographic scope and OIF has primarily been studied as single items in surveys, there is still reason to believe that public groups may not support OIF deployment at scale. Further research is needed to understand whether both the general public and potentially affected groups support, as well as how they might view different possible applications of OIF (e.g., across locations, materials, dispersal methods, and governance arrangements). Particular attention should be given to how Indigenous and Global South groups might view OIF as this is essential to just implementation of OIF and has been the subject of little prior research (Sovacool, 2023). In addition to the qualitative research discussed in the following section, one way to approach this might be via quantitative survey research methods, with a particular focus on factors such as site selection, materials used, dispersal mechanisms, and political-economic considerations. Other methods, such as deliberative polling, citizens’ assemblies (Cox et al., 2022), or world-wide views, may also be important means to facilitate not only greater understanding of public perceptions but also greater community participation in this research.
Social research must involve a broad range of groups in any region being considered for research and deployment in order to explore specific decisions about regional implementation. Community engagement might be in the form of deliberative small groups (Bellamy, 2022; Cox et al., 2022), but other methods might also be explored, particularly those that build decision making capacity at local or regional levels. Engagement should aim, to the extent possible, to understand the key concerns and priorities of these groups (e.g., regarding fisheries, economic livelihoods), so that these can be integrated into the broader OIF research agenda. In this community engagement research, it will be important to give particular attention to groups that have been historically excluded from decision-making on ocean spaces. It is important, in particular, that Indigenous communities are included in the process and that OIF research respects principles of meaningful consent, following the United Nations Declaration on Rights of Indigenous Peoples. Beyond this, it is important that engagement research explores how all coastal communities might shape the design of further research and any possible deployment projects of OIF.
A last feature of a social science research agenda should involve developing a decision framework for evaluating how to select the next steps for OIF research. Trade-offs are certain to arise between different sites, implementation methods, and research timelines—and many of these trade-offs may have implications for local communities and disadvantaged groups. Decision processes are needed to explicitly articulate trade-offs and ensure that different groups are represented. To do this, social research can bring together local communities with experts to collaboratively explore the possible risks, co-benefits, and trade-offs associated with different OIF locations and technical approaches (Harrison et al., 2018; Armoškaitė et al., 2020). This work should give attention not only to tangible but also intangible resources such as cultural and spiritual (Martin et al., 2016).
Legal research is needed to assess strengths and weaknesses in existing governance frameworks for OIF, and possible legal reforms to advance safe, responsible, and just outcomes (NASEM, 2022). While there has been much discussion of the regulation of OIF studies under the London Convention and Protocol, less attention has been given to the treatment of OIF under other international agreements, including the new Biodiversity Beyond National Jurisdiction (BBNJ) treaty and agreements that make up the global climate change regime. It is important to explore the potential for developing a new international framework or compact governing OIF, or perhaps mCDR more broadly, and is one of the recommendations in the NASEM (2022) report. Finally, domestic laws relevant to the OIF field trials, and any subsequent longer-term deployments, will need to be assessed.
OIF studies need to be conducted in iron limited waters, which are largely outside of national EEZs. Even so, the studies will be regulated under the domestic law of the country in which the vessel used is registered and/or was loaded. In many countries, the regulatory approach taken is likely to be influenced by recent developments under the London Convention and Protocol. In 2008, the parties to the London Convention and Protocol adopted a non-binding resolution, which allows for “legitimate scientific research” into OIF but restricts deployment. In 2010, the parties adopted an “assessment framework” for evaluating research projects, which provides for an “initial assessment” to determine whether a project has “proper scientific attributes to qualify as legitimate scientific research” followed by an environmental impact assessment. This approach—i.e., allowing certain research projects after a comprehensive evaluation but preventing deployment—was codified in a 2013 amendment to the London Protocol. That amendment has not yet entered into force, however. The recently adopted BBNJ Treaty might, in the future, provide another avenue for international governance of OIF and other mCDR activities (Webb, 2024).
While these international developments must be considered when planning OIF field studies, it is important to note that the permit and other legal requirements that apply to such studies will ultimately depend on where and how they are conducted. Studies that involve the use of vessels registered or loaded within the U.S. (regardless of where the studies themselves take place) will be subject to regulation by the U.S. Environmental Protection Agency (EPA) under the domestic law that implements the London Convention [i.e., the Marine Protection, Research, and Sanctuaries Act (MPRSA)]. While the EPA has provided preliminary guidance on the application of the MPRSA to OIF and other mCDR activities (EPA, 2024), its permitting processes continue to evolve, and concerted effort will be needed to secure the necessary approvals and otherwise obtain the necessary social and political support for mCDR studies in the open ocean.
Social and governance research should be an integral component of the overall ExOIS research strategy to run alongside the early-stage field trials (Supplementary Table S6), and therefore help to inform decision-making about scaling up trials (Nawaz et al., 2023). This process will begin with the scoping of groups to be engaged with by carrying out a rights-holder mapping exercise, and should be carried out in collaboration with key communities and rights-holder groups to ensure a thorough and transparent process. Engaging in a dialog with governments and decisionmakers has already begun, and will be an ongoing and evolving process as the science on mCDR develops and our understanding of OIF matures. The social science and governance research needs to work in synergy with the fieldwork to ensure the best research outcomes and policy decisions.
The acceleration of climate-induced changes in the earth and ocean systems highlights the urgent need for research into mCDR, including OIF. We have the tools and models to begin field research in parallel with technical developments for MRV, shore-based studies of iron forms and delivery, improvements in modeling, as advancements in governance and policy continue. A concern is that rapid commercialization of mCDR before we have evidence of carbon efficiencies, biogeochemical and ecological changes, and societal implications almost certainly could hurt, or even shut off, marine climate intervention efforts. Quite simply, using OIF for marketable carbon credits, or for governments to meet purported climate goals, is premature.
We describe a 5-year Phase I research plan for parallel research activities, though the timing and progress along these paths may move independently as a function of available human and financial resources. The early activities would be dominated by field planning, improving and intercomparing our models, initiating the iron “bake- off” activities, encouraging developments in new technologies for MRV and eMRV, and initiating social science studies and governance activities (including, but not limited to, securing permits for field trials). The following several years would include several next-generation field experiments in the NE Pacific.
Taken together, we would be ready at the end of Phase I to decide whether to make operational the larger OIFOs for replicated studies at multiple sites in Phase II. By then, we would have determined the most efficient and bioavailable forms of iron and delivery systems, and expanded the use of AVs for iron delivery, with improved MRV and eMRV to establish OIFOs. These operational improvements in Phase I will make working in other sites more efficient and cost effective as we move to significantly more challenging settings such as the Southern Ocean, where the high nutrient concentrations increase the potential for OIF to impact global atmospheric CO2 removal.
Phase II would be used to better define regional differences in both high- and low-nutrient sites. Studies over larger spatial scales would focus on increasing C efficiencies and finding optimal locations to increase climate impacts with acceptable consequences. Emphasis would be placed on transition of OIF studies to larger scale deployment by laying out common protocols and standards for MRV and eMRV. This transition to larger scales would need to involve commercial partners and training of the next generation of mCDR operators and MRV verifiers. During Phase II, we would continue to engage with the public, governments and decision makers on larger issues of mCDR and global climate regulation, that would ensure equitable and sustained benefit to humanity and the environment.
As we proceed, field planning will have to establish sentinels of critical response parameters (biological and chemical), markers of social acceptance to proceed with deployment, along with decision-based thresholds for halting iron release or further experimentation. Critical go/no-go decisions would include whether or not C efficiencies can be quantified and verified and at what cost. Without this ability there is no need to continue OIF studies for mCDR. If ecological and geochemical impacts are detrimental (e.g., deoxygenation, N2O, HABS) relative to those we are experiencing already with climate change or outside regulatory limits, then further study will also not be warranted. To be clear, determining how or whether these scenarios develop is one of the key goals of the project: establish the potential limitations of OIF before operational scale mCDR can be allowed to occur. Based on previous OIF experiments, the ocean systems quickly return to ambient conditions once iron infusions stop—a natural brake on such perturbations—so the field experimentation proposed here, in progressive steps with effective oversight and safeguards, is unlikely to lead to lasting impacts on the ocean systems as discussed in Section 2 on eMRV.
The next decade of climate losses and interplay between public, economic, and political forces, will require the flexibility to accelerate and/or rescope these plans. While a decade may seem like a long time, climate models suggest that this window is critical for reducing CO2 emissions while also seeking effective CDR protocols to assist in achieving net zero by mid-century, and thus avert the worst consequences of climate change. We have learned in the past that conducting inexpensive and hence inconclusive field trials, or moving ahead without appropriate checks and oversight, will shut down progress as governments and the public pushback against the unknown risks of OIF and mCDR (Service, 2012), while ignoring its potential.
To move forward on OIF R&D requires a significant amount of support. The NASEM report (NASEM, 2022) estimated that $290 M would be needed over 5–10 years to evaluate OIF as a method of mCDR. ExOIS has put forward a budget of $160 M over 5 years for the activities described in this manuscript. To remain independent and to apply for research permits under the London Convention and Protocol, will require support from national agencies, philanthropies and/or as gifts from high wealth individuals and private entities, rather than by directly selling C credits or sharing IP.
Importantly, and embedded within the strategies here, will be the requirement to compare OIF mCDR costs and consequences against the accelerating rate of climate-driven ecological changes, socioeconomic losses, and human suffering without implementation of climate intervention policies (emissions reductions plus CDR). As the program proceeds, more emphasis will be directed to life-cycle analyses (Bach et al., 2024) and costs to better inform us on the options of OIF vs. other land- and ocean-based CDR approaches. The most recent estimates on cost per ton CO2 removed using OIF with appropriate MRV ranges from <$25/ton CO2 in best and intermediate cases and > $400/ton CO2 in worst case scenarios (Emerson et al., 2024). That study sees a small advantage for air vs. ship delivery of iron (≈25% reduction in cost) but suggests that studies to improve C efficiencies for export, as well as increasing biological responses to Fe would have the greatest impact in reducing costs (by factors of 2–3 for each). Field studies, as well as on shore work on optimizing Fe bioavailability and then improving delivery of new forms of iron, will be essential outcomes of the activities recommended here and key variables that impact costs. At the same time, attention to social dimensions, governance considerations, and public perceptions of OIF are essential and would need to increase as we move ahead in this decadal plan, as mCDR will require robust regulation and governance procedures and a favorable view of mCDR and the benefit of OIF as one of a portfolio of GHG reduction approaches.
Today, we have the opportunity and obligation to invest in the knowledge necessary to ensure that we can make scientifically and ethically sound decisions for the future of our planet. There is no path forward to solving the climate crisis that excludes the oceans for CDR, which along with emissions reduction will be necessary to reduce human suffering and environmental and ocean damage due to climate change. Of the mCDR approaches, OIF has the potential to be cheap, scalable, and rapidly deployable. Hence there is an urgency to proceed with the plans outlined in this article to decide under what conditions OIF might be deployed or not. Now is the time for actionable studies to begin.
The original contributions presented in the study are included in the article/Supplementary material, further inquiries can be directed to the corresponding authors.
KB: Writing – original draft, Writing – review & editing. DB: Writing – original draft, Writing – review & editing. FC: Writing – original draft, Writing – review & editing. JC: Writing – original draft, Writing – review & editing. ME: Writing – original draft, Writing – review & editing. NH: Writing – original draft, Writing – review & editing. SJ: Writing – original draft, Writing – review & editing. DM: Writing – original draft, Writing – review & editing. PM: Writing – original draft, Writing – review & editing. SN: Writing – original draft, Writing – review & editing. JN: Writing – original draft, Writing – review & editing. AP: Writing – original draft, Writing – review & editing. KR: Writing – original draft, Writing – review & editing. DaS: Writing – original draft, Writing – review & editing. SS: Writing – original draft, Writing – review & editing. DeS: Writing – original draft, Writing – review & editing. KT-K: Writing – original draft, Writing – review & editing. BT: Writing – original draft, Writing – review & editing. RW: Writing – original draft, Writing – review & editing. MW: Writing – original draft, Writing – review & editing. AW: Writing – original draft, Writing – review & editing. PX: Writing – original draft, Writing – review & editing. J-EY: Writing – original draft, Writing – review & editing.
The author(s) declare that financial support was received for the research, authorship, and/or publication of this article. Financial support for the MLML workshop was provided by the Astera Institute along with the Biological Oceanography Lab at MLML, and more generally from the MLML, San José State University. Support for production of this report was provided by the Astera Institute, the McConnell Family Foundation, and the Woods Hole Oceanographic Institution.
We are pleased to acknowledge the contributions of all the participants at the Moss Landing Marine Laboratories (MLML) ExOIS workshop. In addition to the manuscript co-authors, others at the MLML ExOIS workshop included: David Koweek, Ocean Visions; Dan Whaley, Hypothesis; Neil Cohn, CodeGreen; Michael Behrenfeld, Oregon State University; Yui Takeshita, MBARI; Douglas Hamilton, NC State University; Bill Cochlan SF State, University; Francisco Chavez, MBARI; Matthew Church, University of Montana; Colleen Durkin, MBARI; Andrea Fassbender, NOAA/PMEL; Ken Johnson, MBARI; Raphael Kudela, UCSC; Matthew Long, UCAR/[C]Worthy. Assistance at that workshop was provided by several students from MLML as well as Hans Schwing. Additional help with organization of the workshop and in the writing of the workshop report was provided by Jessica Drysdale, Laura Castañón, Ken Kostel, and Eric Taylor at WHOI. Finally, we thank two reviewers for their thoughtful and thorough reviews.
The authors declare that the research was conducted in the absence of any commercial or financial relationships that could be construed as a potential conflict of interest.
All claims expressed in this article are solely those of the authors and do not necessarily represent those of their affiliated organizations, or those of the publisher, the editors and the reviewers. Any product that may be evaluated in this article, or claim that may be made by its manufacturer, is not guaranteed or endorsed by the publisher.
The Supplementary material for this article can be found online at: https://www.frontiersin.org/articles/10.3389/fclim.2024.1430957/full#supplementary-material
Abraham, E. R., Law, C. S., Boyd, P. W., Lavender, S. J., Maldonado, M. T., and Bowie, A. R. (2000). Importance of stirring in the development of an iron-fertilized phytoplankton bloom. Nature 407, 727–730. doi: 10.1038/35037555
Amelung, D., and Funke, J. (2015). Laypeople's risky decisions in the climate change context: climate engineering as a risk-defusing strategy? Hum. Ecol. Risk. Assess. 21, 533–559. doi: 10.1080/10807039.2014.932203
Armoškaitė, A., Puriņa, I., Aigars, J., Strāķe, S., Pakalniete, K., Frederiksen, P., et al. (2020). Establishing the links between marine ecosystem components, functions and services: an ecosystem service assessment tool. Ocean Coast. Manag. 193:105229. doi: 10.1016/j.ocecoaman.2020.105229
ARPA-E SEA-CO2 Program (2023). Sensing Exports of anthropogenic carbon through ocean observation. Available at: https://arpa-e.energy.gov/technologies/programs/sea-co2 (Accessed May 7, 2024).
Babakhani, P., Phenrat, T., Baalousha, M., Soratana, K., Peacock, C. L., Twining, B. S., et al. (2022). Potential use of engineered nanoparticles in ocean fertilization for large-scale atmospheric carbon dioxide removal. Nat. Nanotechnol. 17, 1342–1351. doi: 10.1038/s41565-022-01226-w
Bach, L. T., Ho, D. T., Boyd, P. W., and Tyka, M. D. (2023). Toward a consensus framework to evaluate air–sea CO2 equilibration for marine CO2 removal. Limnol. Oceanogr. Lett. 8, 685–691. doi: 10.1002/lol2.10330
Bach, L. T., Vaughan, N. E., Law, C. S., and Williamson, P. (2024). Implementation of marine CO2 removal for climate mitigation: the challenges of additionality, predictability, and governability. Elem. Sci. Anth. 12:1. doi: 10.1525/elementa.2023.00034
Baker, C. A., Martin, A. P., Yool, A., and Popova, E. (2022). Biological carbon pump sequestration efficiency in the North Atlantic: a leaky or a Long-term sink? Glob. Biogeochem. Cycles 36:e2021GB007286. doi: 10.1029/2021GB007286
Bellamy, R. (2022). Mapping public appraisals of carbon dioxide removal. Glob. Environ. Chang. 76:102593. doi: 10.1016/j.gloenvcha.2022.102593
Bennett, S. A., Achterberg, E. P., Connelly, D. P., Statham, P. J., Fones, G. R., and German, C. R. (2008). The distribution and stabilisation of dissolved Fe in deep-sea hydrothermal plumes. Earth Planet. Sci. Lett. 270, 157–167. doi: 10.1016/j.epsl.2008.01.048
Bertram, C., and Merk, C. (2020). Public perceptions of ocean-based carbon dioxide removal: the nature-engineering divide? Front Clim 2:594194. doi: 10.3389/fclim.2020.594194
Black, E. E., Kienast, S. S., Lemaitre, N., Lam, P. J., Anderson, R. F., Planquette, H., et al. (2020). Ironing out Fe residence time in the dynamic Upper Ocean. Glob. Biogeochem. Cycles 34:e2020GB006592. doi: 10.1029/2020GB006592
Blain, S., Quéguiner, B., Armand, L., Belviso, S., Bombled, B., Bopp, L., et al. (2007). Effect of natural iron fertilization on carbon sequestration in the Southern Ocean. Nature 446, 1070–1074. doi: 10.1038/nature05700
Böttjer-Wilson, D., White, A. E., Björkman, K. M., Church, M. J., Poulos, S., Shimabukuro, E., et al. (2021). Effects of nutrient enrichments on oligotrophic phytoplankton communities: a mesocosm experiment near Hawai‘i, USA. Aquat. Microb. Ecol. 87, 167–183. doi: 10.3354/ame01977
Bostrom, A., O’Connor, R. E., Böhm, G., Hanss, D., Bodi, O., Ekström, F., et al. (2012). Causal thinking and support for climate change policies: International survey findings. Global Environ. Change 22, 210–222.
Bowie, A. R., Maldonado, M. T., Frew, R. D., Croot, P. L., Achterberg, E. P., Mantoura, R. F. C., et al. (2001). The fate of added iron during a mesoscale fertilisation experiment in the Southern Ocean. Deep-Sea Res. II Top. Stud. Oceanogr. 48, 2703–2743. doi: 10.1016/S0967-0645(01)00015-7
Boyd, P., Claustre, H., Legendre, L., Gattuso, J.-P., and Le Traon, P.-Y. (2023). Operational monitoring of Open-Ocean carbon dioxide removal deployments: detection, attribution, and determination of side effects. Oceanography 36, 2–10. doi: 10.5670/oceanog.2023.s1.2
Boyd, P. W., Claustre, H., Levy, M., Siegel, D. A., and Weber, T. (2019). Multi-faceted particle pumps drive carbon sequestration in the ocean. Nature 568, 327–335. doi: 10.1038/s41586-019-1098-2
Boyd, P. W., and Ellwood, M. J. (2010). The biogeochemical cycle of iron in the ocean. Nat. Geosci. 3, 675–682. doi: 10.1038/ngeo964
Boyd, P. W., Ibisanmi, E., Sander, S. G., Hunter, K. A., and Jackson, G. A. (2010). Remineralization of upper ocean particles: implications for iron biogeochemistry. Limnol. Oceanogr. 55, 1271–1288. doi: 10.4319/lo.2010.55.3.1271
Boyd, P. W., Jickells, T., Law, C. S., Blain, S., Boyle, E. A., Buesseler, K. O., et al. (2007). Mesoscale iron enrichment experiments 1993-2005: synthesis and future directions. Science 315, 612–617. doi: 10.1126/science.1131669
Boyd, P. W., Law, C. S., Wong, C. S., Nojiri, Y., Tsuda, A., Levasseur, M., et al. (2004). The decline and fate of an iron-induced subarctic phytoplankton bloom. Nature 428, 549–553. doi: 10.1038/nature02437
Buck, H. J. (2018). “Village science meets global discourse: the Haida Salmon restoration Corporation’s ocean iron fertilisation experiment” in Geoengineering our climate? eds. J. J. Blackstock and S. Low (London: Routledge).
Buesseler, K., Leinen, M., and Ramakrishna, K. (2022). Removing carbon dioxide: first, do no harm. Nature 606:864. doi: 10.1038/d41586-022-01774-0
Chai, F., Johnson, K. S., Claustre, H., Xing, X., Wang, Y., Boss, E., et al. (2020). Monitoring ocean biogeochemistry with autonomous platforms. Nat. Rev. Earth Environ. 1, 315–326. doi: 10.1038/s43017-020-0053-y
Claustre, H., Johnson, K. S., and Takeshita, Y. (2020). Observing the Global Ocean with biogeochemical-Argo. Annu. Rev. Mar. Sci. 12, 23–48. doi: 10.1146/annurev-marine-010419-010956
Cooley, S. R., Klinsky, S., Morrow, D. R., and Satterfield, T. (2023). Sociotechnical considerations about ocean carbon dioxide removal. Annu. Rev. Mar. Sci. 15, 41–66. doi: 10.1146/annurev-marine-032122-113850
Cox, E., Spence, E., and Pidgeon, N. (2022). Deliberating enhanced weathering: public frames, iconic ecosystems and the governance of carbon removal at scale. Public Underst. Sci. 31, 960–977. doi: 10.1177/09636625221112190
De Baar, H. J. W., Gerringa, L. J. A., Laan, P., and Timmermans, K. R. (2008). Efficiency of carbon removal per added iron in ocean iron fertilization. Mar. Ecol. Prog. Ser. 364, 269–282. doi: 10.3354/meps07548
DeVries, T., Primeau, F., and Deutsch, C. (2012). The sequestration efficiency of the biological pump. Geophys. Res. Lett. 39:2012GL051963. doi: 10.1029/2012GL051963
Duce, R. A., and Tindale, N. W. (1991). Atmospheric transport of iron and its deposition in the ocean. Limnol. Oceanogr. 36, 1715–1726. doi: 10.4319/lo.1991.36.8.1715
Emerson, D., Sofen, L. E., Michaud, A. B., Archer, S. D., and Twining, B. S. (2024). A cost model for ocean iron fertilization as a means of carbon dioxide removal that compares ship- and aerial-based delivery, and estimates verification costs. Earth’s Future 12:e2023EF003732. doi: 10.1029/2023EF003732
EPA (2024). Permitting for mCDR and mSRM. Available at: https://www.epa.gov/ocean-dumping/permitting-mcdr-and-msrm (Accessed May 9, 2024).
ExOIS (2022). Exploring Ocean iron solutions. Available at: https://oceaniron.org/ (Accessed May 7, 2024).
Eyring, V., Bony, S., Meehl, G. A., Senior, C. A., Stevens, B., Stouffer, R. J., et al. (2016). Overview of the coupled model Intercomparison project phase 6 (CMIP6) experimental design and organization. Geosci. Model Dev. 9, 1937–1958. doi: 10.5194/gmd-9-1937-2016
Freeland, H. (2007). A short history of Ocean Station papa and line P. Prog. Oceanogr. 75, 120–125. doi: 10.1016/j.pocean.2007.08.005
Frew, R. D., Hutchins, D. A., Nodder, S., Sanudo-Wilhelmy, S., Tovar-Sanchez, A., Leblanc, K., et al. (2006). Particulate iron dynamics during FeCycle in subantarctic waters southeast of New Zealand. Glob. Biogeochem. Cycles 20:2005GB002558. doi: 10.1029/2005GB002558
Gledhill, M. (2012). The organic complexation of iron in the marine environment: a review. Front. Microbiol. 3:69. doi: 10.3389/fmicb.2012.00069
Gwyther, D. E., Keating, S. R., Kerry, C., and Roughan, M. (2023). How does 4DVar data assimilation affect the vertical representation of mesoscale eddies? A case study with observing system simulation experiments (OSSEs) using ROMS v3.9. Geosci. Model Dev. 16, 157–178. doi: 10.5194/gmd-16-157-2023
Hamme, R. C., Webley, P. W., Crawford, W. R., Whitney, F. A., DeGrandpre, M. D., Emerson, S. R., et al. (2010). Volcanic ash fuels anomalous plankton bloom in subarctic Northeast Pacific. Geophys. Res. Lett. 37:2010GL044629. doi: 10.1029/2010GL044629
Harrison, P. A., Dunford, R., Barton, D. N., Kelemen, E., Martín-López, B., Norton, L., et al. (2018). Selecting methods for ecosystem service assessment: a decision tree approach. Ecosyst. Serv. 29, 481–498. doi: 10.1016/j.ecoser.2017.09.016
Hawco, N. J., Yang, S., Pinedo-González, P., Black, E. E., Kenyon, J., Ferrón, S., et al. (2022). Recycling of dissolved iron in the North Pacific subtropical gyre. Limnol. Oceanogr. 67, 2448–2465. doi: 10.1002/lno.12212
Henson, S. A., Laufkötter, C., Leung, S., Giering, S. L. C., Palevsky, H. I., and Cavan, E. L. (2022). Uncertain response of ocean biological carbon export in a changing world. Nat. Geosci. 15, 248–254. doi: 10.1038/s41561-022-00927-0
Ho, D. T., Bopp, L., Palter, J. B., Long, M. C., Boyd, P. W., Neukermans, G., et al. (2023). Monitoring, reporting, and verification for ocean alkalinity enhancement. State Planet oae2023:2-oae2023. doi: 10.5194/sp-2-oae2023-12-2023
Ho, D. T., Ledwell, J. R., and Smethie, W. M. (2008). Use of SF 5 CF 3 for ocean tracer release experiments. Geophys. Res. Lett. 35:2007GL032799. doi: 10.1029/2007GL032799
IPCC (2023). “Climate change 2023: synthesis report” in Contribution of working groups I, II and III to the sixth assessment report of the intergovernmental panel on climate change.
Ito, T., and Follows, M. J. (2013). Air-sea disequilibrium of carbon dioxide enhances the biological carbon sequestration in the Southern Ocean. Glob. Biogeochem. Cycles 27, 1129–1138. doi: 10.1002/2013GB004682
Jackson, G. A. (2008). Effect of mixed layer depth on phytoplankton removal by coagulation and on the critical depth concept. Deep-Sea Res. I Oceanogr. Res. Pap. 55, 766–776. doi: 10.1016/j.dsr.2008.03.004
Jobin, M., and Siegrist, M. (2020). Support for the deployment of climate engineering: a comparison of ten different technologies. Risk Anal. 40, 1058–1078. doi: 10.1111/risa.13462
Johnson, K. S., Chavez, F. P., and Friederich, G. E. (1999). Continental-shelf sediment as a primary source of iron for coastal phytoplankton. Nature 398, 697–700. doi: 10.1038/19511
Karl, D. M., and Letelier, R. M. (2008). Nitrogen fixation-enhanced carbon sequestration in low nitrate, low chlorophyll seascapes. Mar. Ecol. Prog. Ser. 364, 257–268. doi: 10.3354/meps07547
Kintisch, E. (2009). Controversy in their wake, geoengineering experiment in Southern Ocean to begin. ScienceInsider.
Kourafalou, V. H., Androulidakis, Y. S., Halliwell, G. R., Kang, H. S., Mehari, M. M., le Hénaff, M., et al. (2016). North Atlantic Ocean OSSE system development: nature run evaluation and application to hurricane interaction with the Gulf stream. Prog. Oceanogr. 148, 1–25. doi: 10.1016/j.pocean.2016.09.001
Kwiatkowski, L., Torres, O., Bopp, L., Aumont, O., Chamberlain, M., Christian, J. R., et al. (2020). Twenty-first century ocean warming, acidification, deoxygenation, and upper-ocean nutrient and primary production decline from CMIP6 model projections. Biogeosciences 17, 3439–3470. doi: 10.5194/bg-17-3439-2020
Lannuzel, D., Vancoppenolle, M., van der Merwe, P. P., de Jong, J., Meiners, K. M., Grotti, M., et al. (2016). Iron in sea ice: Review and new insights. Elementa 4:000130. doi: 10.12952/journal.elementa.000130
Laufkötter, C., Vogt, M., Gruber, N., Aita-Noguchi, M., Aumont, O., Bopp, L., et al. (2015). Drivers and uncertainties of future global marine primary production in marine ecosystem models. Biogeosciences 12, 6955–6984. doi: 10.5194/bg-12-6955-2015
Law, C. S., Crawford, W. R., Smith, M. J., Boyd, P. W., Wong, C. S., Nojiri, Y., et al. (2006). Patch evolution and the biogeochemical impact of entrainment during an iron fertilisation experiment in the sub-Arctic Pacific. Deep-Sea Res. II Top. Stud. Oceanogr. 53, 2012–2033. doi: 10.1016/j.dsr2.2006.05.028
Levin, L. A., Alfaro-Lucas, J. M., Colaço, A., Cordes, E. E., Craik, N., Danovaro, R., et al. (2023). Deep-sea impacts of climate interventions. Science 379, 978–981. doi: 10.1126/science.ade7521
Maldonado, M. T., Boyd, P. W., LaRoche, J., Strzepek, R., Waite, A., Bowie, A. R., et al. (2001). Iron uptake and physiological response of phytoplankton during a mesoscale Southern Ocean iron enrichment. Limnol. Oceanogr. 46, 1802–1808. doi: 10.4319/lo.2001.46.7.1802
Martin, J. H. (1990). Glacial-interglacial CO2 change: the iron hypothesis. Paleoceanography 5, 1–13. doi: 10.1029/PA005i001p00001
Martin, J. H., Knauer, G. A., Karl, D. M., and Broenkow, W. W. (1987). VERTEX: carbon cycling in the Northeast Pacific. Deep Sea Res. A Oceanogr. Res. Papers 34, 267–285. doi: 10.1016/0198-0149(87)90086-0
Martin, C. L., Momtaz, S., Gaston, T., and Moltschaniwskyj, N. A. (2016). A systematic quantitative review of coastal and marine cultural ecosystem services: current status and future research. Mar. Policy 74, 25–32. doi: 10.1016/j.marpol.2016.09.004
Matsumoto, K. (2006). Model simulations of carbon sequestration in the Northwest Pacific by patch fertilization. J. Oceanogr. 62, 887–902. doi: 10.1007/s10872-006-0106-y
McGillicuddy, D. J., Lynch, D. R., Wiebe, P., Runge, J., Durbin, E. G., Gentleman, W. C., et al. (2001). Evaluating the synopticity of the US GLOBEC Georges Bank broad-scale sampling pattern with observational system simulation experiments. Deep-Sea Res. II Top. Stud. Oceanogr. 48, 483–499. doi: 10.1016/S0967-0645(00)00126-0
NASA (2024). National Oceanic and Atmospheric Administration, Ocean Station papa. Available at: https://www.pmel.noaa.gov/ocs/Papa (Accessed March 28, 2024).
NASEM (2022). National Academies of sciences, engineering, and medicine. A research strategy for ocean-based carbon dioxide removal and sequestration. Washington, DC: The National Academies Press.
Nawaz, S., Peterson St-Laurent, G., and Satterfield, T. (2023). Public evaluations of four approaches to ocean-based carbon dioxide removal. Clim. Pol. 23, 379–394. doi: 10.1080/14693062.2023.2179589
Niiler, P. P., Sybrandy, A. S., Bi, K., Poulain, P. M., and Bitterman, D. (1995). Measurements of the water-following capability of holey-sock and TRISTAR drifters. Deep-Sea Res. I Oceanogr. Res. Pap. 42, 1951–1964. doi: 10.1016/0967-0637(95)00076-3
Nowicki, M., DeVries, T., and Siegel, D. A. (2024). The influence of Air-Sea CO 2 disequilibrium on carbon sequestration by the Ocean’s biological pump. Glob. Biogeochem. Cycles 38:e2023GB007880. doi: 10.1029/2023GB007880
Oschlies, A., Koeve, W., Rickels, W., and Rehdanz, K. (2010). Side effects and accounting aspects of hypothetical large-scale Southern Ocean iron fertilization. Biogeosciences 7, 4017–4035. doi: 10.5194/bg-7-4017-2010
Palter, J., Cross, J., Long, M., Rafter, P., and Reimers, C. (2023). The science we need to assess marine carbon dioxide removal. Eos 104. doi: 10.1029/2023EO230214
Peña, M. A., and Bograd, S. J. (2007). Time series of the Northeast Pacific. Prog. Oceanogr. 75, 115–119. doi: 10.1016/j.pocean.2007.08.008
Pham, A. L. D., and Ito, T. (2019). Ligand binding strength explains the distribution of iron in the North Atlantic Ocean. Geophys. Res. Lett. 46, 7500–7508. doi: 10.1029/2019GL083319
Pollard, R. T., Salter, I., Sanders, R. J., Lucas, M. I., Moore, C. M., Mills, R. A., et al. (2009). Southern Ocean deep-water carbon export enhanced by natural iron fertilization. Nature 457, 577–580. doi: 10.1038/nature07716
Quéré, C. L., Harrison, S. P., Colin Prentice, I., Buitenhuis, E. T., Aumont, O., Bopp, L., et al. (2005). Ecosystem dynamics based on plankton functional types for global ocean biogeochemistry models. Glob. Chang. Biol. 11, 2016–2040. doi: 10.1111/j.1365-2486.2005.1004.x
Rafter, P. A., Sigman, D. M., and Mackey, K. R. M. (2017). Recycled iron fuels new production in the eastern equatorial Pacific Ocean. Nat. Commun. 8:1100. doi: 10.1038/s41467-017-01219-7
Raiswell, R., Benning, L. G., Davidson, L., and Tranter, M. (2008). Nanoparticulate bioavailable iron minerals in icebergs and glaciers. Mineral. Mag. 72, 345–348. doi: 10.1180/minmag.2008.072.1.345
Rijkenberg, M. J. A., Middag, R., Laan, P., Gerringa, L. J. A., van Aken, H. M., Schoemann, V., et al. (2014). The distribution of dissolved iron in the West Atlantic Ocean. PLoS One 9:e101323. doi: 10.1371/journal.pone.0101323
Seelen, E. A., Townsend, E., Gleich, S. J., Caron, D. A., Dugenne, M., White, A. E., et al. (2024). “Pelagic ecosystem research incubators (PERIcosms): optimized incubation tanks to investigate natural communities under long term, low nutrient, and low metal conditions” in Limnology and Oceanography: Methods.
Service, R. F. (2012). Legal? Perhaps. But controversial fertilization experiment may produce little science. ScienceInsider.
Shaked, Y., Buck, K. N., Mellett, T., and Maldonado, M. T. (2020). Insights into the bioavailability of oceanic dissolved Fe from phytoplankton uptake kinetics. ISME J. 14, 1182–1193. doi: 10.1038/s41396-020-0597-3
Sharma, D., Menon, V. G., Desai, M., Niu, D., Bates, E., Kandel, A., et al. (2024). Organoclay floc formation drives carbon export from the sea surface. Sci. Rep. In review
Shetye, S., Pratihary, A., Shenoy, D., Kurian, S., Gauns, M., Uskaikar, H., et al. (2023). Rice husk as a potential source of silicate to oceanic phytoplankton. Sci. Total Environ. 879:162941. doi: 10.1016/j.scitotenv.2023.162941
Shrum, T. R., Markowitz, E., Buck, H., Gregory, R., Van Der Linden, S., Attari, S. Z., et al. (2020). Behavioural frameworks to understand public perceptions of and risk response to carbon dioxide removal: Behavioral Framework for CDR. Interface Focus 10. doi: 10.1098/rsfs.2020.0002
Siegel, D. A., Cetinić, I., Graff, J. R., Lee, C. M., Nelson, N., Perry, M. J., et al. (2021a). An operational overview of the EXport processes in the ocean from RemoTe sensing (EXPORTS) Northeast Pacific field deployment. Elem. Sci. Anth. 9:00107. doi: 10.1525/elementa.2020.00107
Siegel, D. A., DeVries, T., Cetinić, I., and Bisson, K. M. (2023). Quantifying the Ocean’s biological pump and its carbon cycle impacts on global scales. Annu. Rev. Mar. Sci. 15, 329–356. doi: 10.1146/annurev-marine-040722-115226
Siegel, D. A., DeVries, T., Doney, S. C., and Bell, T. (2021b). Assessing the sequestration time scales of some ocean-based carbon dioxide reduction strategies. Environ. Res. Lett. 16:104003. doi: 10.1088/1748-9326/ac0be0
Somes, C. J., Dale, A. W., Wallmann, K., Scholz, F., Yao, W., Oschlies, A., et al. (2021). Constraining global marine iron sources and ligand-mediated scavenging fluxes with GEOTRACES dissolved iron measurements in an ocean biogeochemical model. Glob. Biogeochem. Cycles 35:e2021GB006948. doi: 10.1029/2021GB006948
Sovacool, B. K. (2023). Expanding carbon removal to the global south: thematic concerns on systems, justice, and climate governance. Energy Clim. Change 4:100103. doi: 10.1016/j.egycc.2023.100103
Strzepek, R. F., Maldonado, M. T., Higgins, J. L., Hall, J., Safi, K., Wilhelm, S. W., et al. (2005). Spinning the “ferrous wheel”: the importance of the microbial community in an iron budget during the FeCycle experiment. Glob. Biogeochem. Cycles 19:2005GB002490. doi: 10.1029/2005GB002490
Sturges, W. T., Wallington, T. J., Hurley, M. D., Shine, K. P., Sihra, K., Engel, A., et al. (2000). A potent greenhouse gas identified in the atmosphere: SF5CF3. Science 289, 611–613. doi: 10.1126/science.289.5479.611
Sunda, W. G., and Huntsman, S. A. (1997). Interrelated influence of iron, light and cell size on marine phytoplankton growth. Nature 390, 389–392. doi: 10.1038/37093
Tagliabue, A., Aumont, O., DeAth, R., Dunne, J. P., Dutkiewicz, S., Galbraith, E., et al. (2016). How well do global ocean biogeochemistry models simulate dissolved iron distributions? Glob. Biogeochem. Cycles 30, 149–174. doi: 10.1002/2015GB005289
Tagliabue, A., Bowie, A. R., Boyd, P. W., Buck, K. N., Johnson, K. S., and Saito, M. A. (2017). The integral role of iron in ocean biogeochemistry. Nature 543, 51–59. doi: 10.1038/nature21058
Tagliabue, A., Buck, K. N., Sofen, L. E., Twining, B. S., Aumont, O., Boyd, P. W., et al. (2023a). Authigenic mineral phases as a driver of the upper-ocean iron cycle. Nature 620, 104–109. doi: 10.1038/s41586-023-06210-5
Tagliabue, A., Twining, B. S., Barrier, N., Maury, O., Berger, M., and Bopp, L. (2023b). Ocean iron fertilization may amplify climate change pressures on marine animal biomass for limited climate benefit. Glob. Chang. Biol. 29, 5250–5260. doi: 10.1111/gcb.16854
Tang, W., Llort, J., Weis, J., Perron, M. M. G., Basart, S., Li, Z., et al. (2021). Widespread phytoplankton blooms triggered by 2019–2020 Australian wildfires. Nature 597, 370–375. doi: 10.1038/s41586-021-03805-8
Taqieddin, A., Sarrouf, S., Ehsan, M. F., Buesseler, K., and Alshawabkeh, A. N. (2024). Electrochemical Ocean iron fertilization and alkalinity enhancement approach toward CO2 sequestration. npj Ocean Sustain. 3:28. doi: 10.1038/s44183-024-00064-8
Twining, B. S., and Baines, S. B. (2013). The trace metal composition of marine phytoplankton. Annu. Rev. Mar. Sci. 5, 191–215. doi: 10.1146/annurev-marine-121211-172322
Twining, B. S., Baines, S. B., and Fisher, N. S. (2004). Element stoichiometries of individual plankton cells collected during the Southern Ocean iron experiment (SOFeX). Limnol. Oceanogr. 49, 2115–2128. doi: 10.4319/lo.2004.49.6.2115
Twining, B. S., Nodder, S. D., King, A. L., Hutchins, D. A., LeCleir, G. R., DeBruyn, J. M., et al. (2014). Differential remineralization of major and trace elements in sinking diatoms. Limnol. Oceanogr. 59, 689–704. doi: 10.4319/lo.2014.59.3.0689
Völker, C., and Ye, Y. (2022). Feedbacks between ocean productivity and organic iron complexation in reaction to changes in ocean iron supply. Front. Mar. Sci. 9:777334. doi: 10.3389/fmars.2022.777334
Webb, R. M. (2024). International governance of ocean-based carbon dioxide removal: Recent developments and future directions : Sabin Center for Climate Change Law, Columbia Law School.
Westberry, T. K., Behrenfeld, M. J., Milligan, A. J., and Doney, S. C. (2013). Retrospective satellite ocean color analysis of purposeful and natural ocean iron fertilization. Deep-Sea Res. I Oceanogr. Res. Pap. 73, 1–16. doi: 10.1016/j.dsr.2012.11.010
Windom, H. L., Moore, W. S., Niencheski, L. F. H., and Jahnke, R. A. (2006). Submarine groundwater discharge: a large, previously unrecognized source of dissolved iron to the South Atlantic Ocean. Mar. Chem. 102, 252–266. doi: 10.1016/j.marchem.2006.06.016
Wu, J., Boyle, E., Sunda, W., and Wen, L.-S. (2001). Soluble and colloidal iron in the oligotrophic North Atlantic and North Pacific. Science 293, 847–849. doi: 10.1126/science.1059251
Yoon, J.-E., Yoo, K.-C., Macdonald, A. M., Yoon, H. I., Park, K. T., Yang, E. J., et al. (2018). Reviews and syntheses: ocean iron fertilization experiments – past, present, and future looking to a future Korean iron fertilization experiment in the Southern Ocean (KIFES) project. Biogeosciences 15, 5847–5889. doi: 10.5194/bg-15-5847-2018
Keywords: marine carbon dioxide removal, ocean iron fertilization, carbon sequestration, climate ethics, exploring ocean iron solutions, centennial tonne, monitoring reporting and verification, ecological environmental monitoring reporting and verification
Citation: Buesseler KO, Bianchi D, Chai F, Cullen JT, Estapa M, Hawco N, John S, McGillicuddy DJ, Morris PJ, Nawaz S, Nishioka J, Pham A, Ramakrishna K, Siegel DA, Smith SR, Steinberg D, Turk-Kubo KA, Twining BS, Webb RM, Wells M, White A, Xiu P and Yoon J-E (2024) Next steps for assessing ocean iron fertilization for marine carbon dioxide removal. Front. Clim. 6:1430957. doi: 10.3389/fclim.2024.1430957
Received: 10 May 2024; Accepted: 25 July 2024;
Published: 09 September 2024.
Edited by:
Olivier Sulpis, UMR7330 Centre Européen de Recherche et d’enseignement de Géosciences de l’environnement (CEREGE), FranceReviewed by:
Phillip Williamson, University of East Anglia, United KingdomCopyright © 2024 Buesseler, Bianchi, Chai, Cullen, Estapa, Hawco, John, McGillicuddy, Morris, Nawaz, Nishioka, Pham, Ramakrishna, Siegel, Smith, Steinberg, Turk-Kubo, Twining, Webb, Wells, White, Xiu and Yoon. This is an open-access article distributed under the terms of the Creative Commons Attribution License (CC BY). The use, distribution or reproduction in other forums is permitted, provided the original author(s) and the copyright owner(s) are credited and that the original publication in this journal is cited, in accordance with accepted academic practice. No use, distribution or reproduction is permitted which does not comply with these terms.
*Correspondence: Ken O. Buesseler, a2J1ZXNzZWxlckB3aG9pLmVkdQ==; Paul J. Morris, cG1vcnJpc0B3aG9pLmVkdQ==
Disclaimer: All claims expressed in this article are solely those of the authors and do not necessarily represent those of their affiliated organizations, or those of the publisher, the editors and the reviewers. Any product that may be evaluated in this article or claim that may be made by its manufacturer is not guaranteed or endorsed by the publisher.
Research integrity at Frontiers
Learn more about the work of our research integrity team to safeguard the quality of each article we publish.