- 1CyanoCapture, Oxford Science Park, Oxford, United Kingdom
- 2School of Biological Sciences, University of East Anglia, Norwich Research Park, Norwich, United Kingdom
Atmospheric CO2 removal (CDR) is a fundamentally endergonic process. Performing CDR or Bioenergy with Carbon Capture and Storage (BECCS) at the gigatonne scale will produce a significant additional burden on the planet’s limited renewable energy resources irrespective of the technology employed. Harnessing photosynthesis to drive industrial-scale CO2 fixation has been of significant interest because of its minimal energy requirements and potential low costs. In this review, we evaluated the thermodynamic considerations of performing atmospheric carbon removal using microalgae and cyanobacteria versus physicochemical processes and explore the implications of these energetic costs on the scalability of each respective solution. We review the biomass productivities of recently discovered fast-growing cyanobacterial strains and discuss the prospects of genetically engineering certain metabolic pathways for channeling the fixed carbon into metabolic ‘carbon sinks’ to further enhance their CO2 capture while concurrently extracting value. We share our perspectives on how new highly productive chassis strains combined with advanced flux balance models, essentially coupling synthetic biology with industrial biotechnology, may unlock more favorable methods for CDR, both from an economic and thermodynamic perspective.
1 Introduction
The world produces 40 billion tonnes/year (T/y) in anthropogenic CO2 emissions (Friedlingstein et al., 2023). Based on estimates from the IPCC, approximately 10 billion tonnes of carbon removal is required annually between now and 2100 to meet the requirement to limit global warming to 1.5°C (IPCC, 2018). As of 22nd March 2024 a total of $2.34 billion USD was spent on carbon removal credit purchases for 5.8 million tonnes of CO2 removal, of which only 5.1% have been delivered (CDR, 2024). In order to meet these requirements, carbon removal must increase by four orders of magnitude. Direct Air Capture (DAC) and Bioenergy Carbon Capture & Storage (BECCS) have the largest market capitalization, representing 19 and 64% of the purchases, respectively (CDR, 2024). At the gigatonne scale, the additional renewable energy that would be required to use conventional CO2 capture technologies poses a major feasibility concern (McQueen et al., 2021; Soeherman et al., 2023; Prats-Salvado et al., 2024).
Earth receives approximately 4.3 × 1020 joules of solar irradiance per hour, providing more energy in 1 h than all of the energy consumed by human activity in a year (Lewis and Nocera, 2006). However, the vast majority of this solar capacity remains underutilized (Blankenship et al., 2011). An estimated 115 billion tonnes of CO2 is fixed by photosynthesis annually (Field et al., 1998). Despite the great interest in BECCS, growing fuel crops such as switchgrass to harness photosynthetic productivity at industrial scale still poses a major disadvantage in the areal requirement for arable land. Cyanobacteria and microalgae boast a significantly greater areal productivity and CO2 fixation rate and can yield ~4 fold higher biomass (Subramanian and Sayre, 2022), and 15-300-fold more oil production than terrestrial plants (Chisti, 2007; Hempel et al., 2012; Rehman et al., 2022). Furthermore, cultivation of microalgae and cyanobacteria can be conducted in open ponds or closed vessels on non-arable land, thereby avoiding direct competition for limited agricultural land for the production of biomass (Righelato and Spracklen, 2007). Cyanobacteria contain CO2 concentration mechanisms (CCM), most notably the carboxysome, that allow higher carbon dioxide fixation efficiencies compared to terrestrial plants and macroalgae, leading to higher yields (Savir et al., 2010). Moreover, certain cyanobacterial species can be more rapidly and precisely genetically modified than plants and macroalgae (Gale et al., 2019), suggesting that strains could be further improved to increase biomass yields, aided by recent advances in genetic engineering tools such as CyanoGate (Vasudevan et al., 2019). Despite attempts to utilize microalgae for biofuel production in the 2000s and early 2010s, these initiatives largely faltered due to inadequate biomass yields and the substantial expenses linked to lipid extraction techniques (Abo et al., 2019; Venkata Subhash et al., 2022). Successful implementation of BECCS is therefore dependent on identifying and utilizing faster growing and higher biomass producing strains.
Recently discovered fast growing cyanobacteria, including Synechococcus sp. PCC 11901 and Cyanobacterium aponinum UTEX 3222, have the highest biomass productivity observed among known cyanobacterial and microalgal species (Włodarczyk et al., 2020; Schubert et al., 2023). Synechococcus sp. PCC 11901 is of particular interest due to its natural competence, fast growth (doubling times of less than 2.5 h) and very high productivity (32.6 g/L in 14 days) when cultured under industrially relevant CO2 concentrations of ~5% (e.g., CCGT natural gas power stations). This could potentially make it suitable for cultivation for compounds/biomass production and carbon capture including BECCS, as well as atmospheric carbon removal (Mills et al., 2022; Victoria et al., 2023). Furthermore, other strains of Synechococcus sp. have been well-characterized and possess traits of interest such as native production of commercially valuable compounds (e.g., phycocyanin), fast growth and natural competence, making them potentially useful chassis (Goodchild-Michelman et al., 2023).
Nevertheless, the scalability of employing photosynthesis for mass-scale carbon removal is hindered by inherent efficiency limitations concerning the flux balance at a subcellular level, with only 1–3% of the energy in incident photons being utilized for biomass production (Hann et al., 2022). This poses both an unfortunate bottleneck, as well as an opportunity to make major efficiency gains if carbon flux can be partitioned to pathways with faster reaction kinetics – lending credit to the idea of metabolically engineered carbon sinks (Oliver and Atsumi, 2015; Zhang et al., 2017; Hann et al., 2022). Hence, alongside process improvements, at a physiological level an overall improvement in the efficiency of factors such as biological light capture and CO2 assimilation may lead to productivity thresholds needed to make carbon sequestration at an industrial scale possible while limiting land use and CAPEX (de Vree et al., 2015; Even et al., 2022; Klein and Davis, 2023). Potentially, cyanobacterial biofilms and immobilization in polymer-based compounds could be utilized which has the advantages of consuming less water and allowing high-density growth but in the case of immobilization, cells can no longer be harvested for biomass (Caldwell et al., 2021). Moreover, not all cyanobacteria readily form biofilms.
In this review, we discuss the application of metabolic engineering for improving carbon sinks or other desirable traits to generate fast growing cyanobacterial strains with improved CO2 fixation, thermotolerance and more efficient light-harvesting capacity. A ‘carbon sink’ in this review refers to high flux metabolites or carbon dense compounds that need further downstream processing for permanent sequestration. We categorize each respective approach and evaluate where there is greatest potential for future studies. First, we describe the comparative thermodynamic efficiency limits of performing carbon capture through biological sequestration versus conventional physicochemical methods for atmospheric carbon removal. We illustrate the magnitude of the additional energetic burden posed on the planet when performing carbon capture, irrespective of technology, at the gigatonne scale that is being suggested by the IPCC (Calvin et al., 2023). Second, we conclude that CO2 separation is a fundamentally endergonic process and therefore carbon capture at the gigatonne scale must either become fully powered by renewable energy that has the potential to scale proportionally with CDR demand, or it must be done through a self-powered process like photosynthesis where there is no additional energetic burden. Third, we review the current feasibility of deploying cyanobacteria for BECCS and CDR at scale. Finally, we examine the recent advances in using genetic engineering to further enhance the rate of CO2 fixation in recently discovered fast-growing cyanobacteria that have opened up new possibilities in making this an economically compelling solution capable of scaling rapidly in the near future.
2 Thermodynamics underpinning photosynthesis for CO2 fixation
Both living and non-living systems are bound by the laws of thermodynamics that bear significant relevance to any discourse assessing the viability of performing CO2 sequestration at the gigatonne scale. Animate matter can be considered to be a self-assembling open system, wherein, a separation of substances is achieved between the cell and its environment at the most fundamental level (Popovic, 2019). At the system level, biological life can be conceived as the maintenance of a disequilibrium state against its surroundings (Weber, 2010), increasing its own order and adding entropy of the environment in the process. As the organism creates ordered structures, utilizes energy, divides and responds to the environment, these reactions all have thermodynamic parameters, like any chemical reaction. It is therefore necessary to derive the thermodynamic properties of the reactions that convert CO2 in the environment into biomass, including the Gibbs free energy, entropy and enthalpy calculations, to arrive at a proper comparison against chemical carbon capture technologies. Furthermore, these calculations lend mathematical evidence to why biology may be uniquely resourced to perform the synthesis of more complex molecular structures from CO2 fixation—thus introducing the rationale for metabolic engineering and carbon sinks.
2.1 Second-law efficiencies dictate the minimum energetic requirements for performing carbon removal
Based on the second law of thermodynamics, there is a minimum theoretical energy required to perform separation of CO2 (0.04%) from the air irrespective of the energy efficiency for a given process (House et al., 2011). The theoretical minimum is the net change in exergy of a closed system for a pure separation process. Assuming that the conditions are isothermal and isobaric, this net change in exergy is equal to the change in Gibbs free energy (Equation 1). The minimum work required can be calculated to be 19,385.63 J/mol or 122.35 kWh/T CO2. Because of reversibility, it is impossible in the practical sense to be operating at the theoretical minimum. Therefore, most separation processes operate at anywhere between 2.5-fold (for desalination via reverse osmosis) to 20-fold for DAC (Dahmus and Gutowski, 2007). Separation systems typically require significantly more work done compared to the minimum thermodynamic work required—this ratio is termed the ‘second law efficiency’ which in carbon capture is approximately 5% (House et al., 2011).
Equation 1: the theoretical minimum work to concentrate CO2 from ambient air at 0.04% concentration is 19.39 kJ/mol. Where C denotes the molar concentration of substance k in an ideal gas, denotes change in Gibbs free energy, denotes change in enthalpy and R equals ideal gas constant.
Assuming a 5% second-law efficiency, approximately 388 kJ/mol of energy (2,500 kWh/ T CO2) is needed to separate CO2 from air irrespective of technology. Previous technoeconomic modeling of the cost of DAC systems at scale concluded that this is likely to be only carbon negative if powered by non-CO2 emitting energy sources (House et al., 2011). Taken one step further, we ought to also ask what the opportunity cost is for each additional kWh of renewable energy spent on performing atmospheric carbon removal at this energetic cost for carbon capture compared to the amount of CO2 emission reduction that could be achieved if supplied to the grid to prevent further emissions from combustion of fossil-derived resources. Assuming a 10% second-law efficiency, approximately 1,250 kWh/T CO2 is required—this is what most second generation DAC technologies are projecting to be able to perform at scale using metal–organic frameworks (MOFs; Kumar et al., 2015; Darunte et al., 2016). When capturing from concentrated emission sites such as biogenic power stations emitting 5% CO2 in the flue gas for BECCS-type applications, the minimum work drops to 47.6 kWh/TCO2, making this process significantly more favorable. It is worth noting that the concentration has been increased 125-fold compared to the previous example, but the minimum work against entropy has been reduced by only 60%.
Equation 2: The theoretical minimum work to concentrate CO2 from biogenic emission sources (BECCS) at 5% concentration is 7.55 kJ/mol. Where C denotes the molar concentration of substance k in an ideal gas, denotes change in Gibbs free energy, denotes change in enthalpy and R equals the ideal gas constant.
In a recent multi-component technoeconomic analysis for Direct Air Capture and Storage (DACCS), the process-specific thermodynamic minimum energy required was calculated to be 964, 1,222 and 667 kWh/TCO2 for liquid solvent, solid sorbent and CaO ambient weathering technologies, respectively (Table 1). This is 5–10 fold higher than the thermodynamic minimum calculated in Equation 1 for the separation of CO2 from air (Sievert et al., 2024).
The IPCC targets 10 Gt/year or 420–1,100 Gt of carbon removal in total before 2,100 (5.6–14.7 Gt/year; Carbon Removal | World Resources Institute, 2023; Calvin et al., 2023). Therefore, scaling any atmospheric carbon removal technology at 1,230 kWh/T CO2 (194 kJ/mol), assuming that 10% second-law efficiency is achieved, by 10 Gt/year, results in energy consumption of 12,300 TWh/year (43% of global electricity production). Even assuming that we perform CDR at the thermodynamic minimum of 19.4 kJ/mol at the 10 Gt/year scale, we approach an energy consumption of 1,230 TWh/year, approximately 4.3% of current global energy production of 28,661 TWh/y (based on 2022 estimates; Ritchie et al., 2024). These figures exclude the additional energy that would be required for any processes associated with the permanent storage or compression of the captured CO2.
Approximately 61% of global energy production is from coal, oil and gas (Ritchie et al., 2024). Therefore, CDR would consume 4.3% of the world’s annual electricity production to capture 25% of annual anthropogenic CO2 emissions. Therefore, atmospheric carbon removal is only environmentally practical if powered by low-carbon or renewable energy (McQueen et al., 2021). However, since renewable energy can potentially be better spent decarbonizing the energy mix, it has been widely suggested that gigatonne-scale atmospheric carbon removal ought to come after a near-complete renewable energy transition for it to be justifiable (House et al., 2011). One study reported that, assuming a 20% second law efficiency of atmospheric CO2 removal at 2,500 kWh/T CO2, powering such a technology with 1 kWh of energy would at best, capture approximately the same amount of CO2 that is being emitted by a coal power station to produce 1 kWh. Hence the reason why photosynthesis becomes an interesting approach if it can be engineered (Soeherman et al., 2023). It was concluded from a technoeconomic analysis by Wilcox and colleagues: “Even if the region is no longer building new high-carbon plants but is still running its old ones, shutting down these old plants in favor of low-carbon energy sources is likely to be a better use of these low-carbon sources than powering DAC. Only when the region’s electricity system is nearly completely decarbonized, do the opportunity costs of dedicating a low-carbon electricity source to DAC disappear.”(McQueen et al., 2021). Considering the energy alone involved directly in separating CO2, the cost makes performing atmospheric carbon removal difficult to justify from an efficiency perspective. In contrast, photosynthesis is energetically additional and therefore does not compete for the limited global renewable energy supply to perform CO2 removal.
2.2 Photosynthesis performs an enthalpy change in addition to CO2 separation
In contrast to CDR, carbon fixation in photosynthetic organisms is powered by ATP and NADPH produced by a self-repairing electron transport chain in the thylakoid membrane (Lea-Smith and Hanke, 2021). Furthermore, CO2 capture is inextricably coupled to CO2 fixation into organic compounds with higher energy states (Popovic, 2019). These comprise predominantly lipids, carbohydrates and proteins that constitute what is broadly termed as ‘biomass’. The stoichiometry of the formation of biomass from its constituent reactants can be represented in the equations below where Reaction 1 denotes the combustion of Chlorella sp. biomass while Reaction 2 denotes the combustion of Synechococcus sp. biomass (Nagarkar et al., 2004; Quintana et al., 2011; Popovic, 2019; see Appendix 1.3). The laws of thermodynamics render any work done against entropy to be permanently irrecoverable. Therefore, it is of paramount importance to know how much work is done against entropy in comparison to the work done to an enthalpy change, in a reaction that separates CO2 as gas versus a biological system wherein CO2 is captured and converted into biomass. Next, we draw on values for enthalpy and entropy of formation for microorganisms estimated experimentally by Popovic et al., along with Hess’ law to determine the enthalpy of combustion values under standard conditions (Popovic, 2019). To estimate the molecular composition of Synechococcus sp. biomass based on the percentage of protein, lipid, and carbohydrate content, we use their typical composition as a basis (see Appendix 1.1) for the calculation leading to the formula (Nagarkar et al., 2004). The calorific value of microalgal and cyanobacterial biomass in the literature ranges from 2.8 kJ/g to 21 kJ/g dry cell weight (Scragg et al., 2002; Nagarkar et al., 2004). We have taken a conservative estimate, given the experimental calorific value of 2.8 kJ/g dry cell weight (DCW) in Nagarkar et al., for Synechococcus sp. biomass. We then calculated the enthalpy of combustion, which is presented in Table 2 (see Appendix 1.2 for calculation details).
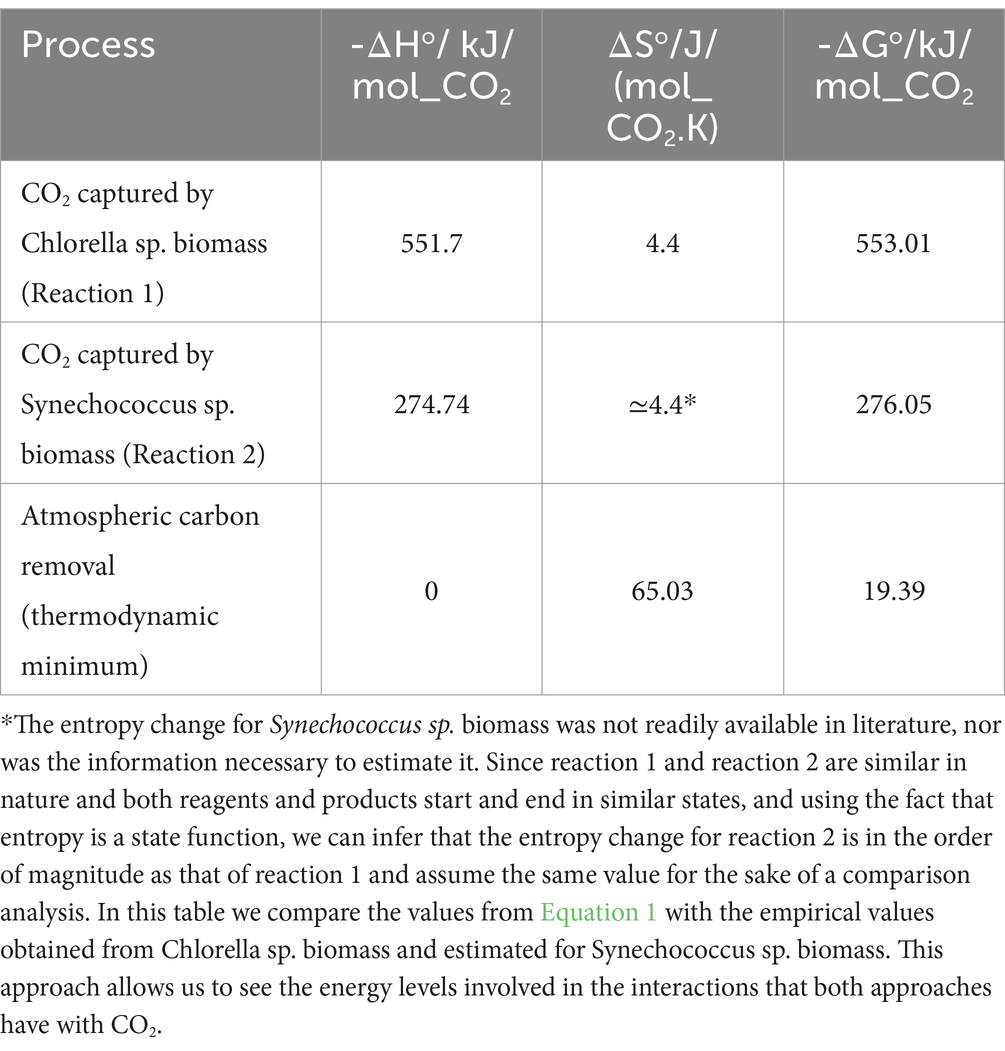
Table 2. Comparison of the changes in enthalpy, entropy and free energy of combustion for Chlorella sp. biomass (reaction 1, Popovic, 2019), Synechococcus sp. biomass (reaction 2) and for DAC under standard conditions.
Since the energetic requirement for biological sequestration includes the enthalpy change, the total amounts to 274 kJ/mol of CO2 of additional work (for Synechococcus) that has been performed by biology to convert CO2 into a higher energy state (biomass), equivalent to an energy gain of 1,729 kWh/T CO2, whereas there is no enthalpy change in the pure separation of CO2 from air.
From Table 2, we can deduce that significantly greater energy is required to capture each mol of CO2 using biological sequestration as biomass compared to a chemical process separating CO2 from air at the thermodynamic minimum (derived from Equation 1). However, most of this energy is consumed by an enthalpy change, meaning that this energy is not lost from the system and therefore, cyanobacteria and microalgae serve as extraordinarily efficient biological factories. To determine whether performing carbon removal makes sense at a fundamental reaction level, these values do not consider the practical energetic costs of CO2 transport, compression and storage for atmospheric carbon removal, nor the energetic costs associated with extraction, cultivation and processing of a biological system. It should also be noted that atmospheric carbon removal can be done to produce CO2 as a substrate for manufacturing processes and that CO2 has a very low standard enthalpy state of formation at −393.5 kJ/mol (Wang et al., 2019). A pure stream of compressed CO2 generated from atmospheric carbon removal can still technically undergo an equivalent enthalpy change through a downstream chemical process such as Fischer-Tropsch, to produce hydrocarbons or organic compounds (Male et al., 2021; Soeherman et al., 2023). However, this is performed at a significant energetic penalty which must be calculated and added on to the Gibbs free energy change for the thermodynamic minimum for atmospheric carbon removal, if we are to make a true comparison of the end-to-end energetic efficiencies of performing biomanufacturing from photosynthesis against chemically converting CO2 after performing direct air capture (Male et al., 2021; Soeherman et al., 2023). Since the enthalpy change undergone by Synechococcus sp. (Table 2) is equivalent to a gain in chemical potential energy (at isothermal conditions), engineering metabolic pathways to favor carbon flux inside a cell toward biosynthesis enables much of this energy to be recovered in the extractable compound of interest, which may facilitate generating economic value from carbon. Hence, unlike conventional carbon capture technologies, with biological sequestration there is a theoretical potential to generate revenues that can offset costs of capture because an enthalpy change has occurred (Zhang et al., 2017; Beal et al., 2018; Onyeaka et al., 2021).
2.3 The low entropic penalty of biomass conversion implies possible room for improvement
The ΔSo for both reactions 1 and 2 are paradoxically lower per mol of biological CO2 fixation than that of the thermodynamic minimum for the separation of CO2 from atmospheric concentrations (Appendix 1.3). It is important to note that, first, this is due to there being two different changes for the state function enthalpy and entropy. Secondly, the thermodynamic analysis focuses on the system changes rather than the universal enthalpy/entropy changes, which can lead to seemingly paradoxical appearances such as a negative entropy change in a system, when one forgets to consider the increased entropy in the surrounding environment for a living organism. One interesting conclusion from this is that this lower system entropy means that the possibility for improving the process is greater in CO2 capture using biological processes, through genetic engineering or otherwise, given that the thermodynamic minimum already has a second-law limit that is 14.8 times bigger in a given physicochemical process of separation than that of Synechococcus sp. (65.03 vs. 4.4 J/molCO2.K; Figure 1).
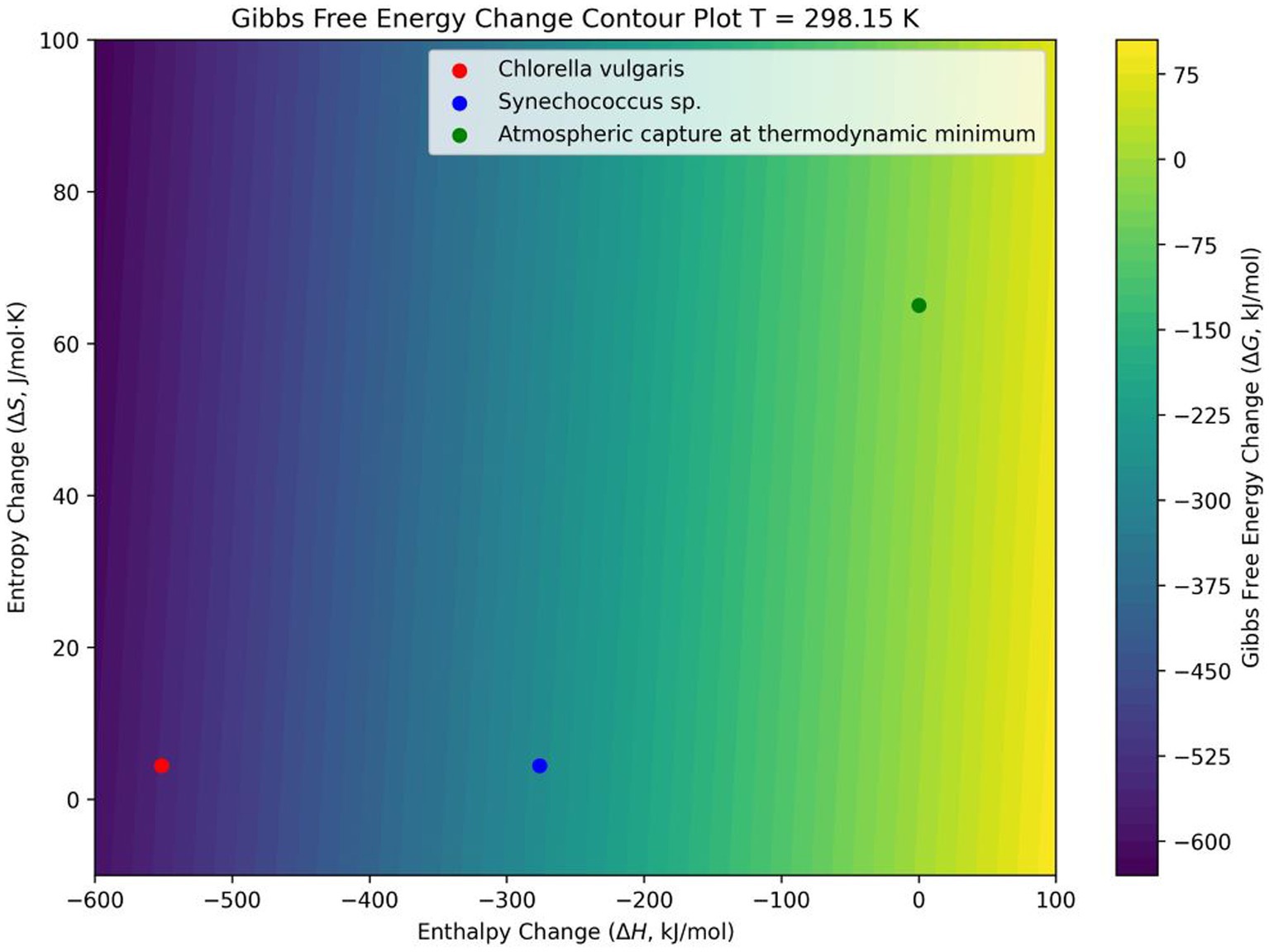
Figure 1. Gibbs free energy change contour plot. This plot illustrates the variation of Gibbs free energy (ΔG) as a function of enthalpy change (ΔH) and entropy change (ΔS) for a hypothetical reaction at an assumed constant temperature of 298.15 K. Contour lines represent levels of constant (ΔG), with darker regions indicating more negative values (energetically favorable conditions) and lighter regions corresponding to less negative or positive values (less favorable or unfavorable conditions). Specific points are marked for ‘Chlorella sp.,’ ‘Synechococcus sp.’ and ‘Atmospheric capture at thermodynamic minimum’, with their positions indicating the respective ΔH and ΔS values. The plot provides insight into the thermodynamic feasibility of these processes, where points falling into darker regions are more spontaneous under the given conditions.
3 Recent discovery of fast-growing species unlocks the possibility of industrial-scale CO2 fixation using engineered cyanobacteria
The recent discovery of fast-growing cyanobacteria such as Synechococcus sp. strains PCC 11901 and PCC 11801, provides possible platforms for further improvement of carbon sinks via genetic modification, utilizing knowledge from previous work in model strains such as Synechocystis sp. PCC 6803, Synechococcus sp. PCC 7002 and Synechococcus elongatus PCC 7942 (Table 3). Synechococcus elongatus PCC 7942 has been used as a platform for production of polyhydroxyalkanoates (PHA), ethylene, sucrose, aldehydes, free fatty acids and alcohols from CO2 (Oliver et al., 2013; Dexter et al., 2015; Ungerer et al., 2018b; Santos-Merino et al., 2019). In these species carbon capture at industrial scale was typically a secondary objective. For example, Algenol engineered Synechocystis sp. PCC 6803 and Synechococccus elongatus PCC 7942 for production of ethanol in closed, outdoor photobioreactor systems using CO2 from an emission site (Legere et al., 2017). The discovery of Synechococcus elongatus UTEX 2973, which demonstrates fast doubling times at low cell densities of 2.3 h (Yu et al., 2015), sparked interest in isolating fast growing cyanobacteria and transferring genetic toolkits to this new strain, which possesses high sequence homology with Synechococcus elongatus PCC 7942 (>99%). The two strains differ by only 53 SNPs, a 7.5 kb deletion and a 188 kb inversion, but Synechococcus elongatus UTEX 2973 has a 2.9-fold faster doubling time than Synechococcus elongatus PCC 7942 (Yu et al., 2015). Photophysiological studies demonstrated that Synechococcus elongatus UTEX 2973 had increased expression of photosystem I (PSI), cytochrome b6f and plastocyanin of 1.6, 1.5 and 2.4 fold, respectively (Ungerer et al., 2018a), compared to Synechococcus elongatus PCC 7942, which increased electron flux through the thylakoid electron transport chain. Distinct SNPs were identified in the coding region of rpaA of Synechococcus elongatus UTEX 2973, a known master regulatory gene for the circadian clock. The K134E point mutation in this gene found in Synechococcus elongatus UTEX 2973 is not seen in the genomes of other related strains including Synechococcus sp. PCC 11901, Synechococcus elongatus 7,942 and Synechococcus sp. PCC 7002, although the rpaA gene itself is well conserved across the genus. While Synechococcus elongatus UTEX 2973 has a lysine substitution, both Synechococcus elongatus 7,942 and Synechococcus sp. PCC 7002 maintain glutamate in this locus. Interestingly, fast-growing Synechococcus sp. PCC 11901 also has a point mutation in this exact locus where arginine is substituted instead of glutamate.
Similarly, distinct SNPs in atpA and ppnK were identified in the same enrichment analysis in this study. Y252C substitution in the atpA gene changed cysteine in Synechococcus elongatus PCC 7942 to tyrosine in Synechococcus elongatus UTEX 2973 in the alpha subunit of ATP synthase and it was speculated that this elicits a loss in sensitivity to redox regulation (Wang et al., 2013). The D260E substitution in ppnK results in the change of glutamate in Synechococcus elongatus PCC 7942 to an aspartate in Synechococcus elongatus UTEX 2973. In the encoded NAD+ kinase enzyme, structural analysis has revealed that the shorter negative side chain of the aspartate is electrostatically repelled by the phosphate groups in NAD+ and ATP. Kinetic parameters of the resulting NAD+ kinase confirmed that the Vmax improved from 434 to 625 nmol/min/mg for NAD+ in Synechococcus elongatus PCC 7942 to Synechococcus elongatus UTEX 2973 (Ungerer et al., 2018b), demonstrating a major breakthrough in engineering targets discovered through comparative genomics and bioinformatics. When all five SNPs were introduced into Synechococcus elongatus PCC 7942 through markerless cloning, the doubling time was improved from 6.8 to 2.2 h and conferred high-light tolerance (Ungerer et al., 2018b). It was also noted that the introduction of the SNP in the rpaA promoter and the pair of coding SNPs did not confer any improved growth phenotype when introduced separately. Future comparative genomics studies across the fast-growing strains that have been isolated may identify the determinants of improved photosynthetic yields. However, Synechococcus elongatus UTEX 2973 does not show higher biomass accumulation than Synechocystis sp. PCC 6803 or Synechococcus elongatus PCC 7942, limiting its use in biotechnology (Włodarczyk et al., 2020; Mills et al., 2022).
Synechococcus sp. PCC 11801 and Synechococcus sp. PCC 11802 are closely related fast-growing strains recently isolated in India (Jaiswal et al., 2018, 2020). Synechococcus sp. PCC 11801 is a freshwater strain which shares 83% sequence homology with its two most closely related strains, Synechococcus elongatus UTEX 2973 and Synechococcus elongatus PCC 7942 (Jaiswal et al., 2018). Synechococcus sp. PCC 11802 has 97% sequence homology with Synechococcus sp. PCC 11801, with 94,540 SNPs shared between the two species. Interestingly, of the five major SNPs found in the Synechococcus elongatus UTEX 2973 atpA, ppnK and rpaA genes that conferred faster growth rates, four were also found in Synechococcus sp. PCC 11801 and Synechococcus sp. PCC 11802 (Jaiswal et al., 2020). It was also shown that Synechococcus sp. PCC 11802 has a different CO2 specificity and grows better at 1% CO2, unlike Synechococcus sp. PCC 11801, which has a fourfold increase in productivity when grown in ambient air compared to 1% CO2 conditions.
Synechococcus sp. PCC 7002 has a 2.6-h doubling rate under optimal conditions and is naturally competent, making it another strong candidate since its isolation in the 1960s (Baalen, 1962; Batterton and Van Baalen, 1971; Nomura et al., 2006; Ludwig and Bryant, 2012). However, it was not until the 2010s that major interest in engineering this strain began to emerge. Synechococcus sp. PCC 7002 achieved proof-of-concept as a euryhaline cyanobacterial chassis for the biosynthesis of a range of valuable compounds including mannitol, riboflavin, cellulose and reducing sugars (Zhao et al., 2015; Ruffing et al., 2016; Kachel and Mack, 2020). Under optimal conditions, Synechococcus sp. PCC 7002 was shown to be capable of achieving approximately 9.3 gDCW/L after 8 days in optimized ‘MAD’ media for marine strains, surpassing that of its fast-growing freshwater counterparts, Synechococcus elongatus. PCC 7942 and Synechococcus elongatus UTEX 2973, which both achieve a maximum of 6.5 gDCW/L in the same time period (Włodarczyk et al., 2020). Moreover, systems for precise unmarked editing of this species have been developed, allowing repeated chromosomal manipulation (Jones et al., 2021).
In 2020, the euryhaline strain, Synechococcus sp. PCC 11901, was isolated and shown to significantly surpass the maximum specific growth rate of other cyanobacteria, demonstrating 18.3 gDCW/L in 8 days in 1% CO2 at 30°C under 750 μmol photons m−2 s−1 light, nearly double that of the second most productive strain, Synechococcus sp. PCC 7002 (Włodarczyk et al., 2020; Table 3). Further work was conducted to optimize the growth conditions, showing that when cultured with 5% CO2 in enriched media termed ‘MAD2 media’, Synechococcus sp. PCC 11901 and Synechococcus sp. PCC 7002 could yield 32.6 and 19.2 gDCW/L, respectively, in 14 days. Carbon accounts for almost half of the dry weight of microalgae biomass, hence approximately 1.83 kg of CO2 can be fixed in every 1 kg of microalgae biomass production. The significance of this productivity in the context of industrial biotechnology is that 2.29 g/L/d of biomass productivity or approximately 4.2 gCO2/L/d of CO2 fixation can theoretically be accomplished with Synechococcus sp. PCC 11901 under controlled conditions in laboratory photobioreactors. While productivity losses are to be expected when scaling up in outdoor photobioreactors (Table 3), the robust growth phenotype observed in fast-growing strains may confer an advantage in scale-up translatability when compared against similar strains of microalgae deployed in commercial photobioreactors (Cheah et al., 2015; de Vree et al., 2015; Ullah et al., 2015). To improve Synechococcus sp. PCC 11901 as a chassis for industrial production, further studies characterized the neutral sites for stable genomic integration and also tested a suite of strong promoters for constitutive expression alongside development of a dCas9-based CRISPR interference system for markerless chromosomal genetic modification using CRISPR-Cas12a, thus opening up significant possibilities of using this species as a photoautotrophic workhorse for synthetic biology (Victoria et al., 2023).
Recently, a novel cyanobacterium, Cyanobacterium aponinum UTEX 3222, was identified from a unique marine environment with naturally elevated CO₂ (>98%) and low pH (<6.5; Schubert et al., 2023). Cyanobacterium aponinum UTEX 3222 was shown to outpace most other fast-growing strains with a doubling rate of 2.35 h in liquid medium and a biomass density of >31 gDCW/L in batch culture. In addition, Cyanobacterium aponinum UTEX 3222 was shown to sink more quickly than other fast-growing strains, which was attributed to its larger cell volume (Schubert et al., 2023). This property is extremely useful for industrial-scale cultivation as it reduces the requirement of industrial flocculants or dewatering. However, tools to genetically manipulate this species have not been developed.
4 Using carbon sinks for enhanced CO2 fixation
4.1 Rationale behind carbon sinks as a method of driving CO2 fixation
Electron transport in cyanobacteria has been recently reviewed so we will focus on the energy conversion rates (Lea-Smith and Hanke, 2021). Moreover, there is some variation in the electron transport chain between cyanobacteria, so for simplicity, the predicted pathway in Synechococcus sp. PCC 11901 is shown in Figure 2 (Lea-Smith et al., 2013; Mills et al., 2022). The first step is driven by photosystem II (PSII), where water is split by sunlight into oxygen, protons, and electrons, which replaces energized electrons released from the reaction center. The quantum efficiency of this process is 84% (Wientjes et al., 2013), although the transfer of useful work from the light harvesting complex (LHC) to the water splitting reaction is around 32%, as shown in Figure 2 (Dau and Zaharieva, 2009). Ultimate transfer of the electron to ferredoxin-NADP+-reductase to reduce NADP+ to NADPH and production of a proton gradient across the thylakoid membrane, which drives ATP production via ATP Synthase, results in a direct conversion of light energy into chemical potential energy that is usable by the cell (Dau and Zaharieva, 2009). Dau and colleagues determined that the energy efficiency of this first stage is 10.5% (Dau and Zaharieva, 2009). However, between the light dependent reactions and the Calvin-Benson-Bassam (CBB) cycle, there is a significant loss in energy transfer, resulting in only 1–2% of energy converting to biomass, although this might be higher in certain cyanobacteria and algae, potentially up to 10%, which is close to the theoretical maximum of 12% (Ooms et al., 2016; Hann et al., 2022). During the process of carbon fixation, NADPH and ATP are utilized in the Calvin–Benson–Bassham (CBB) cycle to regenerate ribulose 1,5-bisphosphate, which Ribulose bisphosphate carboxylase/oxygenase (rubisco) catalyzes the addition of CO₂ (CO₂ fixation) to in order to form two molecules of 3-phosphoglycerate, that subsequently enter central metabolism. Rubisco can also catalyze the addition of O2 to ribulose 1,5-bisphosphate, resulting in production of 2-phosphoglycoate, a process called photorespiration, which reduces carbon fixation rates. It has been widely accepted that the CBB cycle in photosynthetic organism is bottlenecked by the slow reaction kinetics of rubisco which fixes only 1–22 CO2 molecules per second per active site (Zhao et al., 2024), in part due to the requirement for specificity of CO2 over O2 binding. However, carboxylation rates are faster in cyanobacterial species, which is likely linked to the lower requirement for CO2 specificity, as rubisco is enclosed in the CO2 enriched carboxysome (Savir et al., 2010). Attempts to structurally engineer rubisco have rarely shown worthwhile improvements in carbon fixation rates, including in cyanobacteria (Oliver and Atsumi, 2015), and it has been suggested that despite its slow catalysis, it is already perfectly optimized to accommodate the environmental CO2 concentration (Tcherkez et al., 2006).
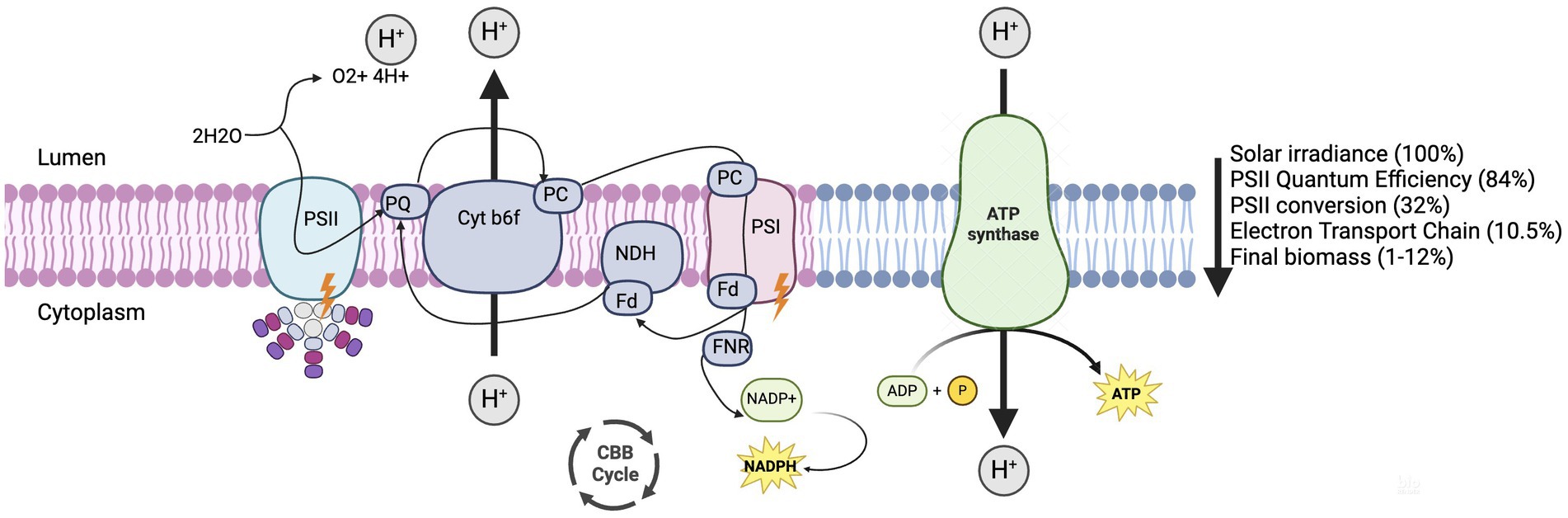
Figure 2. Proposed electron transport chain network in Synechococcus sp. PCC 11901. Efficiency calculations adapted from Dau and Zaharieva (2009) and figure adapted from Lea-Smith and Hanke (2021). ADP, Adenosine diphosphate; ATP, Adenosine triphosphate; CBB cycle, Calvin Benson and Bassham cycle; Cyt, Cytochrome; Fd, Ferridoxin; NADP, Nicotinamide adenine dinucleotide phosphate; NDH, NAD(P)H dehydrogenase; PSII, Photosystem I; PSII, Photosystem II; PQ, Plastoquinone; PC, Plastocyanin.
A significant amount of harvested light energy can be dissipated in deleterious reactions under low CO2 conditions because of the slower reaction kinetics in the CBB cycle, leading to insufficient regeneration of NADP+ and ADP, which in turn results in over-reduction of the electron transport chain (Kanno et al., 2017). As a result, during times of excessive light intensity, instead of capitalizing on this energy to produce more biomass, most of this energy is transferred to O2 via either photorespiration, controlled reactions in terminal oxidases or flavodiiron complexes, or via uncontrolled reactions resulting in formation of reactive oxygen species (ROS), which induces cell stress and photo-oxidative damage, ultimately leading to cellular death (Santana-Sanchez et al., 2019; Lea-Smith and Hanke, 2021). A (re-)balance between light harvesting and energy usage/storage by the cell is needed to optimize the overall light energy conversion efficiency.
The slowness of the reaction kinetics downstream of the CBB cycle creates a metabolic bottleneck (Ducat et al., 2012; Hendry et al., 2016). Several of the metabolic pathways are heavily regulated by proteins sensitive to the cytoplasmic redox state that is used as a proxy for detecting the energetic ‘saturation’ of the cell. These mechanisms, such as cp12, downregulate the upstream pathways involving photosynthesis under these conditions. Rational engineering to redirect carbon partitioning toward less bottlenecked pathways or inserting heterologous enzymes may enable the synthesis of de novo carbon-dense compounds and relieve upstream CO2 fixing steps from inhibitory feedback loops, potentially maintaining a higher rate of carbon fixation for a longer period of time (Oliver and Atsumi, 2015). This is confirmed through experimental findings but is also in line with the conclusions drawn from the thermodynamic calculations concerning the second-law limits of Synechococcus sp. biomass discussed at the end of Section 1.
4.2 Rational engineering of carbon sinks into metabolic pathways
While photosynthesis is energetically more efficient than physicochemical means of CO2 capture, it faces limitations including inefficient light absorption due to pigment selectivity, temperature sensitivity affecting enzyme activity, constraints in CO2 and water availability, photorespiration and CBB cycle inefficiency due to ATP and NADPH availability. Genetic improvements in cyanobacteria have focused on enhancing photosynthetic efficiency, including overexpressing rubisco (Jensen, 2000; Liang and Lindblad, 2017; Tharasirivat and Jantaro, 2023), although this may be limited by CO2 availability and the inability to pack additional rubisco into carboxysomes. Therefore, a more logical approach involves modulating the carbon flux to optimize carbon sinks, ensuring efficient utilization of fixed carbon for biomass production and metabolic processes. This strategy complements efforts to improve carbon fixation by addressing downstream reactions, ultimately maximizing the overall efficiency of photosynthesis and biomass accumulation in cyanobacteria. Redirecting fixed carbon toward the synthesis of C dense sinks not only aids in carbon sequestration but also fosters sustainable production of valuable bioproducts (Oliver and Atsumi, 2015). For this, targeting genes linked to biosynthesis of sugars, glycogen, alkanes, fatty acids, esters etc., has been identified as a strategic approach to optimize C sinks for improved carbon sequestration and bioproduct yields.
In Synechococcus elongatus PCC 7942, heterologous expression of cscB, encoding a sucrose symporter, enabled cytosolic sucrose to be rapidly exported into the media, but also increased biomass productivity and photosynthetic activity (Ducat et al., 2012). Increased flux toward sucrose production was achieved by knocking out glgA, encoding a protein required for synthesis of the alternate glycogen carbon sink, and invA, encoding a protein which breaks down sucrose to glucose and fructose (Figure 3). In an alternate experiment, three genes encoding acetolactate synthase (AlsS), 2-acetolactate decarboxylase (AlsD), and secondary alcohol dehydrogenase (Adh) were expressed to enable production of 2,3-butanediol (2,3-BD), achieving production of 2.4 g/L in 21 days (Figure 3; Oliver et al., 2013). Since pyruvate is a precursor to multiple anabolic pathways and to the 2,3-BD pathway, it is reasonable to expect that increasing the pool of pyruvate would increase the availability for 2,3-BD synthesis as a carbon sink. By overexpressing pgm and pyk encoding phosphogylceromutase and pyruvate kinase respectively, resulting in higher production of pyruvate, the recombinant strains had slight attenuated biomass production but an improved overall carbon and 2,3-butanediol yield of 1.8 and 2.4-fold, respectively (Figure 3; Oliver and Atsumi, 2015). This demonstrates that introducing certain carbon sinks can increase CO2 fixation rates. However, increasing production of free fatty acids to 6.16 mM (1.54 g/L) by knocking out the long-chain-fatty-acid-CoA ligase fadD and inserting a truncated heterologous thioesterase tesA in Synechococcus sp. PCC 11901, resulted in production of 7.5 gDCW/L biomass in 7 days, a reduction compared to the 15 gDCW/L biomass observed in wild-type (Włodarczyk et al., 2020). Contrary to the previous example where the overall carbon yield was increased, in this example, carbon yield was reduced when introducing a novel carbon sink, although excessive free fatty acids in the media could also have reduced growth.
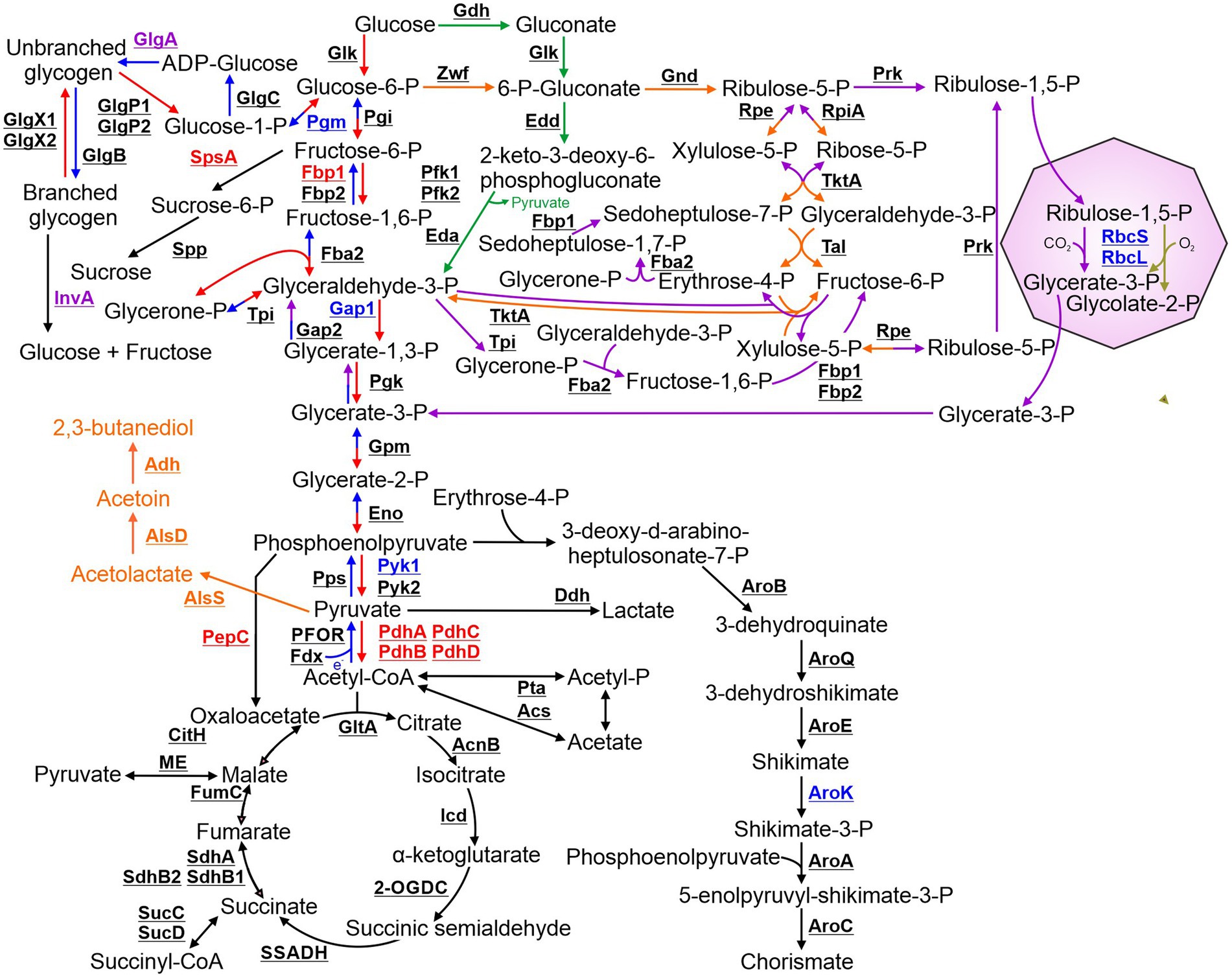
Figure 3. Central metabolism in Synechococcus sp. including proteins altered to increase biomass or compound carbon sinks. Proteins deleted, overexpressed, underexpressed or heterologously expressed are highlighted in purple, blue, red and orange, respectively.
Recently, it was shown that tolerance to high light and high temperature could be conferred in Synechococcus elongatus PCC 7942 hypermutated strains, in which mutS and recA were deleted, by repeatedly culturing the cells under these environment conditions (Sun et al., 2023). This led to generation of a strain with a key point mutation in the non-coding region upstream of aroK, resulting in overexpression of the encoded shikimate kinase, a key enzyme required for biosynthesis of chorismite, a precursor for synthesis of plastoquinone, aromatic amino acids (tyrosine and phenylalanine) and folate (Mills et al., 2020; Moore et al., 2024). Phenotypically, this conferred temperature tolerance up to 45°C and 2,500 μmol photons m−2 s−1 light with optimum growth observed at 42°C and 1,500 μmol photons m−2 s−1. It is unclear how the increased flux through the shikimate pathway directly improves thermotolerance and high light tolerance since the pathway likely has global effects on metabolic function. However it was speculated that this may be the result of enhanced synthesis of plastoquinone, which may reduce the damaging effect of oxidative stress by enabling faster electron dissipation (Block et al., 2013; Spicher et al., 2016; Havaux, 2020). Future studies could focus on inserting these SNPs into faster growing Synechococcus sp. PCC 11901 to confer the same high light and temperature tolerance, which may be desirable traits for industry and reduce the dependence on cooling systems in certain geographies.
Also of interest for rationale engineering of carbon sinks is the regulatory protein CP12, which is activated by an increase in the NADH:NADPH ratio and regulates the CBB cycle by sensing the redox state of the cell (Kanno et al., 2017). Under high light, CP12 downregulates the CBB cycle by repressing glyceraldehyde-3-phosphate dehydrogenase and phosphoribulokinase, thereby reducing carbon flux toward the Embden-Meyerhof-Parnas (EMP) pathway and reducing the conversion of ribulose 5-phosphate to ribulose 1,5-bisphosphate. It was therefore speculated that deletion of cp12 would result in an increase in the activity of the CBB cycle and therefore CO2 fixation. In one study, cp12 was knocked out and rbcLXS, encoding rubisco and its associated chaperone, was overexpressed in a mutant strain of Synechococcus elongatus PCC 7942 engineered to produce 2,3-BD (Figure 3). This combination of CBB cycle disinhibition in a strain resulted in 39% higher production of 2,3-BD from CO2 (Kanno et al., 2017).
In studies conducted in Synechococcus elongatus PCC 7942, 1,3-propanediol (1,3-PDO) production of 1,3-PDO was improved by optimization of gene expression and growth conditions. First, a synthetic pathway consisting of genes derived from Escherichia coli, Saccharomyces cerevisiae and Klebsiella pneumoniae were introduced to produce 1,3-PDO directly from CO2. The strain produced 0.29 g/L 1,3-PDO, however, it also accumulated 1.32 g/L glycerol as an intermediate product (Hirokawa et al., 2016). To reduce glycerol accumulation and improve 1,3-PDO production, the weaker promoter, PLlacO1, was replaced with a stronger promoter (Ptrc), and induction timing for gene expression and medium composition were optimized. This resulted in higher 1,3-PDO production (1.22 g/L) and reduced glycerol accumulation (0.87 g/L; Hirokawa et al., 2017a).
4.3 Metabolic flux balance analysis and CRISPR-interference pooled screening identifies novel gene targets
Earlier generations of metabolic models in cyanobacteria were developed considering the subsystems in isolation, such as the circadian clock (Miyoshi et al., 2007), light dependent reactions (Jablonsky and Lazar, 2008) and the CBB cycle (Badger et al., 1985), have been useful in elucidating reaction kinetics. Flux balance analysis (FBA) enables a comprehensive reconstruction of cellular metabolism and has been used to test the phenotypic effects of genetic perturbations (Orth et al., 2010). However, this is significantly harder to perform in a photoautotrophic organism with an internal circadian rhythm because of the additional dynamic parameters introduced, compared to well-characterized heterotrophs such as Escherichia coli (Reimers et al., 2016). Since FBA assumes a steady-state, introducing a temporal component to the model where light intensity varies will overcome the major limitations in the earlier genome-scale metabolic modeling of photoautotrophic organisms, as has been demonstrated for Synechococcus species (Vijayakumar and Angione, 2017; Sarkar et al., 2019; Vijayakumar et al., 2020). Combined with multi-omics data and computational approaches, dynamic FBA is a powerful tool capable of elucidating novel genetic targets and quantifying the predicted change in carbon partitioning. For example, the synthetic metabolic pathway successfully tested by Hirokawa et al. (2016) for 1,3-PDO production was incorporated into the genome-scale model of Synechococcus elongatus PCC 7942 for FBA. This FBA model allowed the authors to identify novel NADPH dehydrogenase 1 (NDH-1) complexes and disruption of the gene encoding the NdhF1 subunit of NDH-1, resulting in production of 0.34 g/L 1,3-PDO and 2.79 g/L glycerol (Hirokawa et al., 2017b).
Though FBA has been developed for Synechocystis sp. PCC 6803, Synechococcus elongatus UTEX 2973, Synechococcus sp. PCC 7002 and Synechococcus elongatus PCC 7942, transcriptomics and metabolomics data for the fastest growing strain, Synechococcus sp. PCC 11901, are not yet available and FBA has not been modeled (Table 2). Furthermore, performing FBA in scaled up outdoor cultivation conditions under diurnal light cycles will be of significant value to industry and may implicate a different set of genetic targets for a more efficient carbon sink target.
4.4 Improving carbon flux through bicarbonate transporters
Cyanobacteria concentrate dissolved inorganic carbon (DIC) species inside the cell at up to 1,000-fold the concentration of the surrounding environment, due to its unique CO2 concentrating mechanisms (CCM; Badger and Price, 2003). Central to the CCM is the carboxysome which contains rubisco and carbonic anhydrase, which converts HCO3− into CO2 and improves the overall reaction kinetics by increasing substrate and enzyme concentrations. It has previously been shown that there are several modes of DIC uptake in cyanobacteria. Two of the four NDH-1 complexes found in the thylakoid membrane, NDH1-MS and NDH1-MS’, are involved (Price et al., 2013; Hualing, 2022). Cyanobacteria also express plasma membrane bound bicarbonate transporters with different binding affinities to CO2 and bicarbonate ions: BCT1 which is a medium affinity ATP-binding cassette transporter; SbtA, an inducible sodium ion-dependent, low flux bicarbonate transporter; and BicA, a constitutively expressed sodium ion-dependent high flux transporter (Gupta et al., 2020). Transcriptomic studies revealed increased expression of bicA and sbtA under carbon limitation. Overexpression of sbtA and bicA under strong inducible promoters in Synechococcus sp. PCC 7002 was shown to increase biomass dry cell weight yields by 50% compared to wild-type (Gupta et al., 2020). These mutations, in combination with an engineered carbon sink, may be synergistic in developing improved strains for carbon sequestration.
4.5 CRISPR-interference pooled screening of carbon sink expressing mutants
Yao et al. pioneered the use of pooled CRISPR interference libraries for targeted gene repression to screen genes in cyanobacteria (Yao et al., 2020). With this method, a pool of 10,498 clones of Synechocystis sp. PCC 6803 were generated, each expressing dCas9 and an sgRNA that targeted a unique protein-coding open reading frame or a non-coding RNA. This enabled a fitness score to be ascribed to each of these genes after screening the mutant pool under multiple growth conditions and performing enrichment analysis post-next generation sequencing (NGS).
Building on this, Li et al. demonstrated the use of CRISPRi in Synechococcus elongatus PCC 7942, specifically in a CRISPR-engineered mutant expressing heterologous alsS, alsD and adh to rewire carbon flux from pyruvate to 2,3-BD synthesis as a carbon sink (Li et al., 2022). Four enzymes were identified which catalyzed reactions that directly competed with the partitioning of carbon toward pyruvate from the CBB cycle. Fructose bisphosphatase (Fbp) and sucrose phosphate (SpsA) convert fructose-1,6-bisphosphate (FBP) to fructose-6-phosphate (F6P) and then F6P to sucrose, respectively, thus drawing carbon out of the CBB cycle. Phosphoenolpyruvate (PEP) is produced from 3-phosophoglycerate (3PG) that directly exits the CBB cycle and is a precursor to pyruvate. PEP is also converted into oxaloacetate (OAA) by the PEP carboxylase (Ppc) enzyme which directly competes with the flux of PEP to pyruvate. Lastly, pyruvate is also converted into acetyl-CoA by pyruvate dehydrogenase (Pdh; Figure 3). Therefore, it was speculated that repression of these genes with CRISPRi may improve 2,3-BD yields as a carbon sink. By inserting sgRNA cassettes that target these four genes, they were knocked down both independently and in combination. The multiplex suppression of all four genes resulted in 2,3-BD titers increasing from 792.8 mg/L to 1,583.8 mg/L, without incurring any biomass growth inhibition. CRISPRi pooled libraries may be useful for elucidating the genetic basis of improved CO2 fixation rates in newly discovered fast growing strains such as Synechococcus sp. PCC 11901 and may also serve as a powerful tool for improving fast-growing strains to produce carbon sinks by enhancing carbon flux toward desirable pathways by knocking down/out competing enzymes in a combinatoric approach.
4.6 Challenges and future perspectives
It has been suggested that microalgal BECCS at 15 g/m2/d productivity achieves a comparable cost and electrical requirement but a significantly lower heating requirement than current DACCS technologies (Even et al., 2022). A recent smaller scale, continuous cultivation, outdoor trial with Synechococcus elongatus UTEX 2973 engineered to express limonene synthase, demonstrated productivities of up to 48 g/m2/d (Long et al., 2022). This strain, however, has not been tested in larger scale photobioreactors (Table 3). Improved biomass accumulating strains such as Synechococcus sp. PCC 11901 may achieve even higher yields but, to the best of our knowledge, have not been tested in larger photobioreactors outdoors. Bridging this translational gap from bench-to-pilot scale remains a challenge for deploying microorganisms for atmospheric carbon removal or BECCS (Khan et al., 2019). This may be of increasing interest as a solution to achieving gigatonne scale decarbonization if global energy availability becomes a constraint. Focusing on carbon sinks is also a potential strategy for channeling carbon flux toward more appropriate biosynthesis pathways. However, the majority of this work has been performed through rational design, and there has only been limited evidence in the literature of where the chosen targets were guided precisely by advanced screening techniques such as CRISPRi or multi-omics data. Metabolic engineering through targeted genetic manipulation presents a promising avenue to enhance carbon fixation rates and adaptability to flue gases, while simultaneously offering routes to diversify revenue streams, thereby improving commercial viability (Satta et al., 2023). However, the relatively limited repertoire of sophisticated genetic regulatory components tailored for precise control of introduced pathways in cyanobacteria renders this field years behind biomanufacturing industries using more well-established organisms like E. coli and Saccharomyces cerevisiae (Jones, 2014; Hitchcock et al., 2020; Guillaume and Branco dos Santos, 2023). Recent efforts have focused on characterizing standardized modular cloning parts in cyanobacteria, such as the CyanoGate system, yet the advancement of such parts could be significantly boosted by establishing robust and modular expression libraries (Vasudevan et al., 2019; Caicedo-Burbano et al., 2020). Furthermore, expanding genomic, expression, and mutant libraries may provide deeper insights into cyanobacterial physiology, facilitating metabolic engineering efforts.
While CRISPR-based genome editing and interference approaches have shown promise in regulating gene expression by targeting multiple loci simultaneously, concerns regarding toxicity and off-target effects of CRISPR nucleases may limit their application in cyanobacterial genome modification (Guo et al., 2023). Efforts to mitigate this issue include controlling the expression of CRISPR nucleases post-introduction into cyanobacteria (Mengstie et al., 2024). Despite these challenges, the ongoing development of CRISPR-Cas systems in cyanobacteria holds tremendous potential for strain engineering. Future research in synthetic biology aimed at optimizing cyanobacteria as a chassis for metabolic carbon sinks should benefit significantly from the proven approaches using multi-omics guided flux balance models, and high-throughput screening methods. Additionally, the establishment of scalable cultivation systems and outdoor testing platforms is crucial for validating engineered strains under real-world conditions and also gaining a better understanding of how flux balance changes according to diurnal light cycles—for which there is still limited understanding in the field.
Performing carbon removal through photosynthesis has long been compared against competing chemical technologies. It must be noted that drawing an energetic comparison against non-biological systems is difficult, since biology is unable to uncouple its entropy and enthalpy changing activities. A cell performs three combined functions analogous to a PV panel (thylakoid), a direct air capture unit (Ci-transporters) and a CO2 upcycling system (CBB-cycle), that cannot be separated. Strictly speaking, since the separation of CO2 from the air requires only an entropic change, from the perspective of maximizing just CO2 separation, biological systems that perform additional work to undergo an enthalpy change is unnecessary or wasteful. However, from the more pragmatic perspective, we make the argument that the inevitable enthalpy change undergone by CO2 in photosynthesis ought not to be considered as an inefficiency, but rather, a mechanism by which carbon removal at the 10 Gt/year scale can become net profitable instead of being a permanent cost to the planet. For any carbon removal method that does not create an enthalpy change, CO2 separation will always be at a net cost if captured CO2 is being buried, with some models projecting costs upward of $3 trillion/year at the 10 Gt/year scale (Sievert et al., 2024). A CO2 drawdown of 10 Gt/year is equivalent to 2.7 Gt of carbon atoms by mass fraction, which to put into perspective, is equivalent to 63% of the mass of carbon atoms contained in all the crude oil that the world produces each year (4.3 Gt; IEA, 2023). Therefore, the chemical potential energy that biological systems can efficiently absorb from sunlight to transform carbon opens up possibilities for a new era of carbon-negative industrial manufacturing that can offset the costs of co-performing carbon sequestration as biomass—which may be a more sustainable way to continuously conduct 10Gt/y of carbon removal (Cestellos-Blanco et al., 2020; Stephens et al., 2021).
Without taking into account energy gained in enthalpy change for a given area of cyanobacterial cultivation, the overall mass of CO2 sequestered and converted into biomass per square meter of solar irradiance is significantly less than the mass of CO2 that would be separated from the air by employing a given atmospheric carbon removal technology using photovoltaics. Assuming that a PV panel can power a given atmospheric carbon removal method that uses 1,250 kWh/T CO2 to perform separation, if the energy is produced from a m2 of photovoltaic panels, assuming that there is a 100% energy transfer efficiency between the PV panel and carbon removal technology, we can draw a direct comparison against the areal efficiency of biological CO2 sequestration based solely on its areal biomass productivity. Taking the solar irradiance data for the month of September in Arizona to draw this comparison, we estimate that a typical PV panel receives 6.8 kWh/m2/day of light energy and is capable of producing 0.8 kWh/m2/day of electrical energy, assuming a standard 16% overall efficiency, 14.08% system losses, 96% inverter efficiency and 20.2% DC capacity factor (Abdelhamid et al., 2016). Taking this further, assuming that atmospheric carbon removal can be done at 1,250 kWh/T CO2, approximately 640 g/d of CO2 can be captured for each m2 of additional PV capacity built for powering carbon removal based on these assumptions. In one recent technoeconomic model at a similar latitude in Nevada, it was proposed that a 100 MW capacity of PV and 300 MWh battery would be required to remove 100,000 T CO2/year using direct air capture and storage powered by solar energy (DACCS). Assuming that 1 m2 of solar PV produces 0.16 kW of electricity, a 100 MW capacity PV system would equate to 625,000 m2 of required capacity removal or 438 g CO2/day at a levelized cost of $877/T CO2, assuming a carbon removal efficiency of 88% (NREL PVWatts Calculator, 2014; Gutsch and Leker, 2024). Comparing this to cyanobacterial cultivation, 43.3 g/m2/d of biomass yield was achieved using an outdoor pond-design photobioreactor with Synechococcus elongatus UTEX 2973 in September 2021 in Texas at a similar latitude, an equivalent CO2 fixation of 81 g/m2/d, assuming a biomass to CO2 stoichiometric ratio of 1:1.88 (Long et al., 2022). Outdoor algal cultivation trials in Arizona by NREL at a similar latitude showed 19.9 g/m2/d productivity in September with the microalgal strain Picochlorum celeri (Klein and Davis, 2023). Therefore, it can be assumed that from the perspective of areal efficiency of converting incident solar energy into CO2 removal, PV panels convert enough solar energy to deliver 5–8 times the amount of CO2 removal at 1,250 kWh/TCO2 than using Synechococcus elongatus UTEX 2973 at this productivity rate. Therefore, biomass productivities need to be improved if biological carbon sequestration is to reach parity with PV-powered atmospheric carbon removal for a given area of incident solar energy.
Missing from the above calculation is a consideration of the enthalpy change that is recoverable against the irrecoverable work done against entropy in both of those examples (Table 2). Synechococcus sp. have an advantage in that there is an additional gain of 274 kJ/mol CO2 fixed as biomass as an enthalpy change (1,729 kWh/T CO2 in chemical potential energy gained), which is theoretically recoverable as work, unlike work done against entropy. It is also interesting that the work done against system entropy is significantly lower in biological solutions that sequester CO2 than the minimum work done to perform CO2 separation. Therefore, a strong argument exists based on thermodynamics to use engineered bacteria to produce carbon sinks as a method of deriving chemical (and economic) value from this enthalpy change instead of the burial of carbon, even if only partially. Secondly, even if we were to, for the sake of argument, nullify the energetic gain as a sunk cost, the cost of deploying a fully PV powered DAC infrastructure imposes a levelized cost of capture of $877/T CO2 based on recent models (Gutsch and Leker, 2024). Conversely, an algal system amounts to $320/T CO2 (calculated from the minimum biomass selling price of $602/T biomass) based on NREL’s technoeconomic analysis which assumes a modest average of 18.5 g/m2/d of annual productivity (Klein and Davis, 2023), before any consideration of possible additional revenue routes from engineered carbon sinks and bioproducts. Therefore, gigatonne-scale carbon removal will be economically burdensome irrespective of technology, unless further value can be generated from the captured CO2, which fundamentally requires an enthalpy increase. If this route is pursued, from a thermodynamic perspective, it would be reasonable to speculate that this may be significantly more favorable to perform biochemically than by stacking atmospheric CO2 separation with a chemical reaction converting CO2 into precursor compounds, which would be illustrated in the comparison of the total Gibbs free energy change as aforementioned. However, further work is required to harness light-driven biosynthesis and there is considerable process scale-up challenges, as well as legislative challenges concerning the use of modified organisms that need to first be overcome before this technology can become viable.
5 Conclusion
CDR through harnessing cyanobacteria for CO2 fixation presents promising avenues for mitigating the impacts of climate change. At the crux of the issue, the thermodynamics underlying atmospheric carbon removal presents a major problem concerning competing renewable energy resources as well as an economic burden when imagined at the 10 Gt/year scale. Using photosynthesis has limitations such as the surface area required, although it has the advantage of being self-powered, rendering it energetically additional and independent of renewable energy sourcing constraints. Furthermore, since it undergoes both an entropic and enthalpy change, it presents a possibility of becoming a net profitable operation if some economic value can be derived from the additional work done to convert CO2 into higher energy state compounds by the cell. The genetic engineering of carbon sink pathways unlocks a new route by which CO2 removed from the atmosphere can be converted into value while carbon partitioned toward cell growth is locked for permanent carbon removal. The carbon sinks in the evidence discussed in this review shows that biosynthesis does not necessarily impede overall CO2 fixation rates, but rather can increase it further if the metabolic flux is carefully considered. Further multi-disciplinary research on using fast-growing cyanobacteria, such as Synechococcus sp. PCC 11901, may lead to commercially viable, industrial-scale CO2 fixation. Genetic modification and advanced multi-omics analyses have led to significant enhancements in carbon sink capacities and overall CO2 fixation rates. Integration of metabolic FBA and CRISPRi pooled screening facilitates the identification of novel gene targets crucial for further improving CO2 fixation efficiency in cyanobacteria. With continued research efforts focusing on key metabolic pathways, genetic engineering strategies, and industrial-scale cultivation techniques, the prospect of performing carbon removal that circumvents many of the major foreseeable challenges concerning energy and thermodynamic limitations with currently proposed non-biological methods.
Author contributions
DK: Conceptualization, Investigation, Visualization, Formal Analysis, Writing – original draft. JM-C: Investigation, Writing – original draft. ES: Investigation, Visualization, Formal Analysis, Writing – original draft. DJL-S: Visualization, Writing – original draft, Writing – review & editing. US: Investigation, Writing – original draft, Writing - review & editing.
Funding
The author(s) declare that financial support was received for the research, authorship, and/or publication of this article. This work is funded by CyanoCapture Ltd. DJL-S acknowledges funding from the Natural Environmental Research Council, United Kingdom (NE/X014428), and the Biotechnology and Biological Sciences Research Council, United Kingdom (BB/S020365/1; BB/Y008332/1). DK, JM-C and US received funding from Innovate UK (Grant number: 10073574).
Conflict of interest
DK, JM-C, ES and US are affiliated with CyanoCapture Ltd.—a biotechnology company conducting research into cyanobacterial physiology and genetics.
The remaining author declares that the research was conducted in the absence of any commercial or financial relationships that could be construed as a potential conflict of interest.
The author(s) declared that they were an editorial board member of Frontiers, at the time of submission. This had no impact on the peer review process and the final decision.
Publisher’s note
All claims expressed in this article are solely those of the authors and do not necessarily represent those of their affiliated organizations, or those of the publisher, the editors and the reviewers. Any product that may be evaluated in this article, or claim that may be made by its manufacturer, is not guaranteed or endorsed by the publisher.
Supplementary material
The Supplementary material for this article can be found online at: https://www.frontiersin.org/articles/10.3389/fclim.2024.1412232/full#supplementary-material
References
Abdelhamid, M., Pilla, S., Singh, R., Haque, I., and Filipi, Z. (2016). A comprehensive optimized model for on-board solar photovoltaic system for plug-in electric vehicles: energy and economic impacts. Int. J. Energy Res. 40, 1489–1508. doi: 10.1002/er.3534
Abo, B. O., Odey, E. A., Bakayoko, M., and Kalakodio, L. (2019). Microalgae to biofuels production: a review on cultivation, application and renewable energy. Rev. Environ. Health 34, 91–99. doi: 10.1515/reveh-2018-0052
Baalen, C. V. (1962). Studies on marine blue-green algae. Botanica Marina 4, 129–139. doi: 10.1515/botm.1962.4.1-2.129
Badger, M. R., Bassett, M., and Comins, H. N. (1985). A model for HCO3− accumulation and photosynthesis in the cyanobacterium Synechococcus sp. Plant Physiol. 77, 465–471. doi: 10.1104/pp.77.2.465
Badger, M. R., and Price, G. D. (2003). CO2 concentrating mechanisms in cyanobacteria: molecular components, their diversity and evolution. J. Exp. Bot. 54, 609–622. doi: 10.1093/jxb/erg076
Batterton, J. C., and Van Baalen, C. (1971). Growth responses of blue-green algae to sodium chloride concentration. Arch. Mikrobiol. 76, 151–165. doi: 10.1007/BF00411789
Beal, C. M., Archibald, I., Huntley, M. E., Greene, C. H., and Johnson, Z. I. (2018). Integrating algae with bioenergy carbon capture and storage (ABECCS) increases sustainability. Earths Future 6, 524–542. doi: 10.1002/2017EF000704
Blankenship, R. E., Tiede, D. M., Barber, J., Brudvig, G. W., Fleming, G., Ghirardi, M., et al. (2011). Comparing photosynthetic and photovoltaic efficiencies and recognizing the potential for improvement. Science 332, 805–809. doi: 10.1126/science.1200165
Block, A., Fristedt, R., Rogers, S., Kumar, J., Barnes, B., Barnes, J., et al. (2013). Functional modeling identifies paralogous Solanesyl-diphosphate synthases that assemble the side chain of Plastoquinone-9 in plastids *. J. Biol. Chem. 288, 27594–27606. doi: 10.1074/jbc.M113.492769
Broddrick, J. T., Rubin, B. E., Welkie, D. G., Du, N., Mih, N., Diamond, S., et al. (2016). Unique attributes of cyanobacterial metabolism revealed by improved genome-scale metabolic modeling and essential gene analysis. Proc. Natl. Acad. Sci. USA 113, E8344–E8353. doi: 10.1073/pnas.1613446113
Caicedo-Burbano, P., Smit, T., Pineda Hernández, H., Du, W., and Branco dos Santos, F. (2020). Construction of fully segregated genomic libraries in Polyploid organisms such as Synechocystis sp. PCC 6803. ACS Synth. Biol. 9, 2632–2638. doi: 10.1021/acssynbio.0c00353
Caldwell, G. S., In-na, P., Hart, R., Sharp, E., Stefanova, A., Pickersgill, M., et al. (2021). Immobilising Microalgae and Cyanobacteria as Biocomposites: New Opportunities to Intensify Algae Biotechnology and Bioprocessing. Energies. 14:2566. doi: 10.3390/en14092566
Calvin, K., Dasgupta, D., Krinner, G., Mukherji, A., Thorne, P. W., Trisos, C., et al. (2023). “IPCC, 2023: Climate change 2023: Synthesis report” in Contribution of working groups I, II and III to the sixth assessment report of the intergovernmental panel on climate change. eds. Core writing team , H. Lee and J. Romero (IPCC, Geneva, Switzerland: Intergovernmental Panel on Climate Change (IPCC)).
Carbon Removal | World Resources Institute . (2023). URL: https://www.wri.org/initiatives/carbon-removal (accessed 4.4.24).
CDR . Fyi. (2024). URL: https://www.cdr.fyi/ (accessed 3.22.24).
Cestellos-Blanco, S., Zhang, H., Kim, J. M., Shen, Y., and Yang, P. (2020). Photosynthetic semiconductor biohybrids for solar-driven biocatalysis. Nat. Catal. 3, 245–255. doi: 10.1038/s41929-020-0428-y
Cheah, W. Y., Show, P. L., Chang, J.-S., Ling, T. C., and Juan, J. C. (2015). Biosequestration of atmospheric CO2 and flue gas-containing CO2 by microalgae. Bioresour. Technol. Advan Biofuels and Chemicals from algae 184, 190–201. doi: 10.1016/j.biortech.2014.11.026
Chisti, Y. (2007). Biodiesel from microalgae. Biotechnol. Adv. 25, 294–306. doi: 10.1016/j.biotechadv.2007.02.001
Dahmus, J. B., and Gutowski, T. G. (2007). What gets recycled: an information theory based model for product recycling. Environ. Sci. Technol. 41, 7543–7550. doi: 10.1021/es062254b
Darunte, L. A., Oetomo, A. D., Walton, K. S., Sholl, D. S., and Jones, C. W. (2016). Direct air capture of CO2 using amine functionalized MIL-101(Cr). ACS Sustain. Chem. Eng. 4, 5761–5768. doi: 10.1021/acssuschemeng.6b01692
Dau, H., and Zaharieva, I. (2009). Principles, efficiency, and blueprint character of solar-energy conversion in photosynthetic water oxidation. Acc. Chem. Res. 42, 1861–1870. doi: 10.1021/ar900225y
Davies, F. K., Fricker, A. D., Robins, M. M., Dempster, T. A., McGowen, J., Charania, M., et al. (2021). Microbiota associated with the large-scale outdoor cultivation of the cyanobacterium Synechococcus sp. PCC 7002. Algal Res. 58:102382. doi: 10.1016/j.algal.2021.102382
de Vree, J. H., Bosma, R., Janssen, M., Barbosa, M. J., and Wijffels, R. H. (2015). Comparison of four outdoor pilot-scale photobioreactors. Biotechnol. Biofuels 8:215. doi: 10.1186/s13068-015-0400-2
Dexter, J., Armshaw, P., Sheahan, C., and Pembroke, J. T. (2015). The state of autotrophic ethanol production in Cyanobacteria. J. Appl. Microbiol. 119, 11–24. doi: 10.1111/jam.12821
Ducat, D. C., Avelar-Rivas, J. A., Way, J. C., and Silver, P. A. (2012). Rerouting carbon flux to enhance photosynthetic productivity. Appl. Environ. Microbiol. 78, 2660–2668. doi: 10.1128/AEM.07901-11
Even, C., Hadroug, D., Boumlaik, Y., and Simon, G. (2022). Microalgae-based bioenergy with carbon capture and storage quantified as a negative emissions technology. Energy Nexus 7:100117. doi: 10.1016/j.nexus.2022.100117
Field, C. B., Behrenfeld, M. J., Randerson, J. T., and Falkowski, P. (1998). Primary production of the biosphere: integrating terrestrial and oceanic components. Science 281, 237–240. doi: 10.1126/science.281.5374.237
Friedlingstein, P., O’Sullivan, M., Jones, M. W., Andrew, R. M., Bakker, D. C. E., Hauck, J., et al. (2023). Global Carbon Budget 2023. Earth Syst. Sci. Data 15, 5301–5369. doi: 10.5194/essd-15-5301-2023
Gale, G. A. R., Schiavon Osorio, A. A., Mills, L. A., Wang, B., Lea-Smith, D. J., and McCormick, A. J. (2019). Emerging species and genome editing tools: future prospects in cyanobacterial synthetic biology. Microorganisms 7:409. doi: 10.3390/microorganisms7100409
Goodchild-Michelman, I. M., Church, G. M., Schubert, M. G., and Tang, T.-C. (2023). ‘Light and Carbon: Synthetic Biology toward New Cyanobacteria-Based Living Biomaterials’. Materials Today Bio. 19:100583. doi: 10.1016/j.mtbio.2023.100583
Guillaume, M. C., and Branco dos Santos, F. (2023). Assessing and reducing phenotypic instability in cyanobacteria. Curr. Opin. Biotechnol. 80:102899. doi: 10.1016/j.copbio.2023.102899
Guo, C., Ma, X., Gao, F., and Guo, Y. (2023). Off-target effects in CRISPR/Cas9 gene editing. Front. Bioeng. Biotechnol. 11:1143157. doi: 10.3389/fbioe.2023.1143157
Gupta, J. K., Rai, P., Jain, K. K., and Srivastava, S. (2020). Overexpression of bicarbonate transporters in the marine cyanobacterium Synechococcus sp. PCC 7002 increases growth rate and glycogen accumulation. Biotechnol. Biofuels 13:17. doi: 10.1186/s13068-020-1656-8
Gutsch, M., and Leker, J. (2024). Co-assessment of costs and environmental impacts for off-grid direct air carbon capture and storage systems. Commun. Eng. 3, 1–14. doi: 10.1038/s44172-023-00152-6
Haines, M., Vadlamani, A., Daniel Loty Richardson, W., and Strous, M. (2022). Pilot-scale outdoor trial of a cyanobacterial consortium at pH 11 in a photobioreactor at high latitude. Bioresour. Technol. 354:127173. doi: 10.1016/j.biortech.2022.127173
Hann, E. C., Overa, S., Harland-Dunaway, M., Narvaez, A. F., Le, D. N., Orozco-Cárdenas, M. L., et al. (2022). A hybrid inorganic–biological artificial photosynthesis system for energy-efficient food production. Nat. Food 3, 461–471. doi: 10.1038/s43016-022-00530-x
Havaux, M. (2020). Plastoquinone in and beyond photosynthesis. Trends Plant Sci. 25, 1252–1265. doi: 10.1016/j.tplants.2020.06.011
Hempel, N., Petrick, I., and Behrendt, F. (2012). Biomass productivity and productivity of fatty acids and amino acids of microalgae strains as key characteristics of suitability for biodiesel production. J. Appl. Phycol. 24, 1407–1418. doi: 10.1007/s10811-012-9795-3
Hendry, J. I., Gopalakrishnan, S., Ungerer, J., Pakrasi, H. B., Tang, Y. J., and Maranas, C. D. (2019). Genome-scale Fluxome of Synechococcus elongatus UTEX 2973 using transient 13C-labeling Data1[OPEN]. Plant Physiol. 179, 761–769. doi: 10.1104/pp.18.01357
Hendry, J. I., Prasannan, C. B., Joshi, A., Dasgupta, S., and Wangikar, P. P. (2016). Metabolic model of Synechococcus sp. PCC 7002: prediction of flux distribution and network modification for enhanced biofuel production. Bioresour. Technol. 213, 190–197. doi: 10.1016/j.biortech.2016.02.128
Hirokawa, Y., Maki, Y., and Hanai, T. (2017a). Improvement of 1,3-propanediol production using an engineered cyanobacterium, Synechococcus elongatus by optimization of the gene expression level of a synthetic metabolic pathway and production conditions. Metab. Eng. 39, 192–199. doi: 10.1016/j.ymben.2016.12.001
Hirokawa, Y., Maki, Y., Tatsuke, T., and Hanai, T. (2016). Cyanobacterial production of 1,3-propanediol directly from carbon dioxide using a synthetic metabolic pathway. Metab. Eng. 34, 97–103. doi: 10.1016/j.ymben.2015.12.008
Hirokawa, Y., Matsuo, S., Hamada, H., Matsuda, F., and Hanai, T. (2017b). Metabolic engineering of Synechococcus elongatus PCC 7942 for improvement of 1,3-propanediol and glycerol production based on in silico simulation of metabolic flux distribution. Microb. Cell Factories 16:212. doi: 10.1186/s12934-017-0824-4
Hitchcock, A., Hunter, C. N., and Canniffe, D. P. (2020). Progress and challenges in engineering cyanobacteria as chassis for light-driven biotechnology. Microb. Biotechnol. 13, 363–367. doi: 10.1111/1751-7915.13526
House, K. Z., Baclig, A. C., Ranjan, M., van Nierop, E. A., Wilcox, J., and Herzog, H. J. (2011). Economic and energetic analysis of capturing CO2 from ambient air. Proc. Natl. Acad. Sci. 108, 20428–20433. doi: 10.1073/pnas.1012253108
Hualing, M. (2022). Cyanobacterial NDH-1 complexes. Front. Microbiol. 13:933160. doi: 10.3389/fmicb.2022.933160
IPCC (2018). IPCC 2018: global warming of 1.5°C: IPCC special report on impacts of global warming of 1.5°C above pre-industrial levels in context of strengthening response to climate change, sustainable development, and efforts to eradicate poverty. Glob. Warm. 15°C IPCC spec. Rep. Impacts glob. Warm. 15°C pre-Ind. Levels context strength. Response Clim. Change sustain. Dev. Efforts eradicate poverty, 1–24. doi: 10.1017/9781009157940.001
Jablonsky, J., and Lazar, D. (2008). Evidence for intermediate S-states as initial phase in the process of oxygen-evolving complex oxidation. Biophys. J. 94, 2725–2736. doi: 10.1529/biophysj.107.122861
Jaiswal, D., Nenwani, M., and Wangikar, P. P. (2023). Isotopically non-stationary 13 C metabolic flux analysis of two closely related fast-growing cyanobacteria, Synechococcus elongatus PCC 11801 and 11802. Plant J. Cell Mol. Biol. 116, 558–573. doi: 10.1111/tpj.16316
Jaiswal, D., Sengupta, A., Sengupta, S., Madhu, S., Pakrasi, H. B., and Wangikar, P. P. (2020). A novel cyanobacterium Synechococcus elongatus PCC 11802 has distinct genomic and Metabolomic characteristics compared to its neighbor PCC 11801. Sci. Rep. 10:191. doi: 10.1038/s41598-019-57051-0
Jaiswal, D., Sengupta, A., Sohoni, S., Sengupta, S., Phadnavis, A. G., Pakrasi, H. B., et al. (2018). Genome features and biochemical characteristics of a robust, fast growing and naturally transformable cyanobacterium Synechococcus elongatus PCC 11801 isolated from India. Sci. Rep. 8:16632. doi: 10.1038/s41598-018-34872-z
Jensen, R. G. (2000). Activation of rubisco regulates photosynthesis at high temperature and CO2. Proc. Natl. Acad. Sci. USA 97, 12937–12938. doi: 10.1073/pnas.97.24.12937
Jones, P. R. (2014). Genetic instability in Cyanobacteria – an elephant in the room? Front. Bioeng. Biotechnol. 2:12. doi: 10.3389/fbioe.2014.00012
Jones, C. M., Parrish, S., and Nielsen, D. R. (2021). Exploiting polyploidy for Markerless and plasmid-free genome engineering in Cyanobacteria. ACS Synth. Biol. 10, 2371–2382. doi: 10.1021/acssynbio.1c00269
Kachel, B., and Mack, M. (2020). Engineering of Synechococcus sp. strain PCC 7002 for the photoautotrophic production of light-sensitive riboflavin (vitamin B2). Metab. Eng. 62, 275–286. doi: 10.1016/j.ymben.2020.09.010
Kanno, M., Carroll, A. L., and Atsumi, S. (2017). Global metabolic rewiring for improved CO2 fixation and chemical production in cyanobacteria. Nat. Commun. 8:14724. doi: 10.1038/ncomms14724
Khan, A. Z., Bilal, M., Mehmood, S., Sharma, A., and Iqbal, H. M. N. (2019). State-of-the-art genetic modalities to engineer Cyanobacteria for sustainable biosynthesis of biofuel and fine-chemicals to meet bio–economy challenges. Lifestyles 9:54. doi: 10.3390/life9030054
Klein, B., and Davis, R. (2023). Algal biomass production via open pond algae farm cultivation: 2022 state of technology and future research. Renew. Energy. 1–27.
Kumar, A., Madden, D. G., Lusi, M., Chen, K.-J., Daniels, E. A., Curtin, T., et al. (2015). Direct air capture of CO2 by Physisorbent materials. Angew. Chem. Int. Ed. 54, 14372–14377. doi: 10.1002/anie.201506952
Lea-Smith, D. J., and Hanke, G. T. (2021). “Electron transport in Cyanobacteria and its potential in bioproduction” in Cyanobacteria biotechnology. eds. P. Hudson (John Wiley & Sons, Ltd), 33–63.
Lea-Smith, D. J., Ross, N., Zori, M., Bendall, D. S., Dennis, J. S., Scott, S. A., et al. (2013). Thylakoid terminal oxidases are essential for the cyanobacterium Synechocystis sp. PCC 6803 to survive rapidly changing light intensities. Plant Physiol. 162, 484–495. doi: 10.1104/pp.112.210260
Legere, E., Roessler, P., Miller, H., Belicka, L., Yuan, Y., and Chance, R. (2017). Recovery act – Integrated pilot-scale biorefinery for producing ethanol from hybrid algae (no. DE –-EE0002867). Myers, FL (United States): Algenol Biotech LLC, Ft.
Lewis, N. S., and Nocera, D. G. (2006). Powering the planet: chemical challenges in solar energy utilization. Proc. Natl. Acad. Sci. 103, 15729–15735. doi: 10.1073/pnas.0603395103
Li, H., Pham, N. N., Shen, C. R., Chang, C.-W., Tu, Y., Chang, Y.-H., et al. (2022). Combinatorial CRISPR interference library for enhancing 2,3-BDO production and elucidating key genes in Cyanobacteria. Front. Bioeng. Biotechnol. 10:913820. doi: 10.3389/fbioe.2022.913820
Liang, F., and Lindblad, P. (2017). Synechocystis PCC 6803 overexpressing RuBisCO grow faster with increased photosynthesis. Metab. Eng. Commun. 4, 29–36. doi: 10.1016/j.meteno.2017.02.002
Long, B., Fischer, B., Zeng, Y., Amerigian, Z., Li, Q., Bryant, H., et al. (2022). Machine learning-informed and synthetic biology-enabled semi-continuous algal cultivation to unleash renewable fuel productivity. Nat. Commun. 13:541. doi: 10.1038/s41467-021-27665-y
Ludwig, M., and Bryant, D. A. (2012). Synechococcus sp. strain PCC 7002 transcriptome: acclimation to temperature, salinity, oxidative stress, and Mixotrophic growth conditions. Front. Microbiol. 3:354. doi: 10.3389/fmicb.2012.00354
Male, J. L., Kintner-Meyer, M. C. W., and Weber, R. S. (2021). The U.S. energy system and the production of sustainable aviation fuel from clean electricity. Front. Energy Res. 9:765360. doi: 10.3389/fenrg.2021.765360
McQueen, N., Desmond, M. J., Socolow, R. H., Psarras, P., and Wilcox, J. (2021). Natural gas vs. Electricity for Solvent-Based Direct air Capture. Front. Clim. 2:618644. doi: 10.3389/fclim.2020.618644
Mengstie, M. A., Azezew, M. T., Dejenie, T. A., Teshome, A. A., Admasu, F. T., Teklemariam, A. B., et al. (2024). Recent advancements in reducing the off-target effect of CRISPR-Cas9 genome editing. Biol. Targets Ther. 18, 21–28. doi: 10.2147/BTT.S429411
Mills, L. A., McCormick, A. J., and Lea-Smith, D. J. (2020). Current knowledge and recent advances in understanding metabolism of the model cyanobacterium Synechocystis sp. PCC 6803. Biosci. Rep. 40:BSR20193325. doi: 10.1042/BSR20193325
Mills, L. A., Moreno-Cabezuelo, J. Á., Włodarczyk, A., Victoria, A. J., Mejías, R., Nenninger, A., et al. (2022). Development of a biotechnology platform for the fast-growing cyanobacterium Synechococcus sp. PCC 12:872. doi: 10.3390/biom12070872
Miyoshi, F., Nakayama, Y., Kaizu, K., Iwasaki, H., and Tomita, M. (2007). A mathematical model for the Kai-protein-based chemical oscillator and clock gene expression rhythms in cyanobacteria. J. Biol. Rhythm. 22, 69–80. doi: 10.1177/0748730406295749
Moore, L. R., Caspi, R., Campbell, D. A., Casey, J. R., Crevecoeur, S., Lea-Smith, D. J., et al. (2024). CyanoCyc cyanobacterial web portal. Front. Microbiol. 15:1340413. doi: 10.3389/fmicb.2024.1340413
Nagarkar, S., Williams, G. A., Subramanian, G., and Saha, S. K. (2004). “Cyanobacteria-dominated biofilms: a high quality food resource for intertidal grazers” in Asian Pacific phycology in the 21st century: Prospects and challenges. ed. P. O. Ang (Netherlands, Dordrecht: Springer), 89–95.
Nomura, C. T., Sakamoto, T., and Bryant, D. A. (2006). Roles for heme–copper oxidases in extreme high-light and oxidative stress response in the cyanobacterium Synechococcus sp. PCC 7002. Arch. Microbiol. 185, 471–479. doi: 10.1007/s00203-006-0107-7
NREL PVWatts Calculator . (2014). PVWatts version 5 manual (no. NREL/TP-6A20-62641):1158421. doi: 10.2172/1158421
Oliver, J. W. K., and Atsumi, S. (2015). A carbon sink pathway increases carbon productivity in cyanobacteria. Metab. Eng. 29, 106–112. doi: 10.1016/j.ymben.2015.03.006
Oliver, J. W. K., Machado, I. M. P., Yoneda, H., and Atsumi, S. (2013). Cyanobacterial conversion of carbon dioxide to 2,3-butanediol. Proc. Natl. Acad. Sci. 110, 1249–1254. doi: 10.1073/pnas.1213024110
Onyeaka, H., Miri, T., Obileke, K., Hart, A., Anumudu, C., and Al-Sharify, Z. T. (2021). Minimizing carbon footprint via microalgae as a biological capture. Carbon Capture Sci. Technol. 1:100007. doi: 10.1016/j.ccst.2021.100007
Ooms, M. D., Dinh, C. T., Sargent, E. H., and Sinton, D. (2016). Photon management for augmented photosynthesis. Nat. Commun. 7:12699. doi: 10.1038/ncomms12699
Orth, J. D., Thiele, I., and Palsson, B. Ø. (2010). What is flux balance analysis? Nat. Biotechnol. 28, 245–248. doi: 10.1038/nbt.1614
Popovic, M. (2019). Thermodynamic properties of microorganisms: determination and analysis of enthalpy, entropy, and Gibbs free energy of biomass, cells and colonies of 32 microorganism species. Heliyon 5:e01950. doi: 10.1016/j.heliyon.2019.e01950
Prats-Salvado, E., Jagtap, N., Monnerie, N., and Sattler, C. (2024). Solar-powered direct air capture: techno-economic and environmental assessment. Environ. Sci. Technol. 58, 2282–2292. doi: 10.1021/acs.est.3c08269
Price, G. D., Pengelly, J. J. L., Forster, B., Du, J., Whitney, S. M., von Caemmerer, S., et al. (2013). The cyanobacterial CCM as a source of genes for improving photosynthetic CO2 fixation in crop species. J. Exp. Bot. 64, 753–768. doi: 10.1093/jxb/ers257
Quintana, N., Van der Kooy, F., Van de Rhee, M. D., Voshol, G. P., and Verpoorte, R. (2011). Renewable energy from Cyanobacteria: energy production optimization by metabolic pathway engineering. Appl. Microbiol. Biotechnol. 91, 471–490. doi: 10.1007/s00253-011-3394-0
Rehman, M., Kesharvani, S., Dwivedi, G., and Gidwani Suneja, K. (2022). Impact of cultivation conditions on microalgae biomass productivity and lipid content. Mater. Today Proc., international conference on materials, machines and information Technology-2022 56, 282–290.
Reimers, A.-M., Knoop, H., Bockmayr, A., and Steuer, R. (2016). Evaluating the stoichiometric and energetic constraints of cyanobacterial diurnal growth. Arxiv.
Righelato, R., and Spracklen, D. V. (2007). Carbon mitigation by biofuels or by saving and restoring forests? Science 317:902. doi: 10.1126/science.1141361
Ritchie, H., Rosado, P., and Roser, M. (2024). Electricity mix : Our World Data. Available at: https://ourworldindata.org/electricity-mix
Ruffing, A. M., Jensen, T. J., and Strickland, L. M. (2016). Genetic tools for advancement of Synechococcus sp. PCC 7002 as a cyanobacterial chassis. Microb. Cell Factories 15:190. doi: 10.1186/s12934-016-0584-6
Santana-Sanchez, A., Solymosi, D., Mustila, H., Bersanini, L., Aro, E.-M., and Allahverdiyeva, Y. (2019). Flavodiiron proteins 1–to-4 function in versatile combinations in O2 photoreduction in cyanobacteria. eLife 8:e45766. doi: 10.7554/eLife.45766
Santos-Merino, M., Singh, A. K., and Ducat, D. C. (2019). New applications of synthetic biology tools for cyanobacterial metabolic engineering. Front. Bioeng. Biotechnol. 7:33. doi: 10.3389/fbioe.2019.00033
Sarkar, D., Mueller, T. J., Liu, D., Pakrasi, H. B., and Maranas, C. D. (2019). A diurnal flux balance model of Synechocystis sp. PCC 6803 metabolism. PLoS Comput. Biol. 15:e1006692. doi: 10.1371/journal.pcbi.1006692
Satta, A., Esquirol, L., and Ebert, B. E. (2023). Current metabolic engineering strategies for photosynthetic bioproduction in Cyanobacteria. Microorganisms 11:455. doi: 10.3390/microorganisms11020455
Savir, Y., Noor, E., Milo, R., and Tlusty, T. (2010). Cross-species analysis traces adaptation of rubisco toward optimality in a low-dimensional landscape. Proc. Natl. Acad. Sci. 107, 3475–3480. doi: 10.1073/pnas.0911663107
Sawant, K. R., Savvashe, P., Pal, D., Sarnaik, A., Lali, A., and Pandit, R. (2021). Progressive transitional studies of engineered Synechococcus from laboratory to outdoor pilot-scale cultivation for production of ethylene. Bioresour. Technol. 341:125852. doi: 10.1016/j.biortech.2021.125852
Schubert, M.G., Tang, T.-C., Goodchild-Michelman, I.M., Ryon, K.A., Henriksen, J.R., and Chavkin, T. (2023). Cyanobacteria newly isolated from marine volcanic seeps display rapid sinking and robust, high density growth. BIoRxiv.
Scragg, A. H., Illman, A. M., Carden, A., and Shales, S. W. (2002). Growth of microalgae with increased calorific values in a tubular bioreactor. Biomass Bioenergy 23, 67–73. doi: 10.1016/S0961-9534(02)00028-4
Sievert, K., Schmidt, T. S., and Steffen, B. (2024). Considering technology characteristics to project future costs of direct air capture. Aust. Dent. J. 8, 979–999. doi: 10.1016/j.joule.2024.02.005
Soeherman, J. K., Jones, A. J., and Dauenhauer, P. J. (2023). Overcoming the entropy penalty of direct air capture for efficient Gigatonne removal of carbon dioxide. ACS Eng. Au 3, 114–127. doi: 10.1021/acsengineeringau.2c00043
Spicher, L., Glauser, G., and Kessler, F. (2016). Lipid antioxidant and Galactolipid remodeling under temperature stress in tomato plants. Front. Plant Sci. 7:167. doi: 10.3389/fpls.2016.00167
Stephens, S., Mahadevan, R., and Allen, D. G. (2021). Engineering photosynthetic bioprocesses for sustainable chemical production: a review. Front. Bioeng. Biotechnol. 8:610723. doi: 10.3389/fbioe.2020.610723
Subramanian, S., and Sayre, R. T. (2022). The right stuff; realizing the potential for enhanced biomass production in microalgae. Front. Energy Res. 10:979747. doi: 10.3389/fenrg.2022.979747
Sun, H., Luan, G., Ma, Y., Lou, W., Chen, R., Feng, D., et al. (2023). Engineered hypermutation adapts cyanobacterial photosynthesis to combined high light and high temperature stress. Nat. Commun. 14:1238. doi: 10.1038/s41467-023-36964-5
Tcherkez, G. G. B., Farquhar, G. D., and Andrews, T. J. (2006). Despite slow catalysis and confused substrate specificity, all ribulose bisphosphate carboxylases may be nearly perfectly optimized. Proc. Natl. Acad. Sci. USA 103, 7246–7251. doi: 10.1073/pnas.0600605103
Tharasirivat, V., and Jantaro, S. (2023). Increased biomass and Polyhydroxybutyrate production by Synechocystis sp. PCC 6803 overexpressing RuBisCO genes. Int. J. Mol. Sci. 24:6415. doi: 10.3390/ijms24076415
Ullah, K., Ahmad, M., Sofia Sharma, V. K., Lu, P., Harvey, A., Zafar, M., et al. (2015). Assessing the potential of algal biomass opportunities for bioenergy industry: a review. Fuel 143, 414–423. doi: 10.1016/j.fuel.2014.10.064
Ungerer, J., Lin, P.-C., Chen, H.-Y., and Pakrasi, H. B. (2018a). Adjustments to photosystem stoichiometry and Electron transfer proteins are key to the remarkably fast growth of the cyanobacterium Synechococcus elongatus UTEX 2973. MBio 9, e02327–e02317. doi: 10.1128/mBio.02327-17
Ungerer, J., Wendt, K. E., Hendry, J. I., Maranas, C. D., and Pakrasi, H. B. (2018b). Comparative genomics reveals the molecular determinants of rapid growth of the cyanobacterium Synechococcus elongatus UTEX 2973. Proc. Natl. Acad. Sci. 115, E11761–E11770. doi: 10.1073/pnas.1814912115
Vasudevan, R., Gale, G. A. R., Schiavon, A. A., Puzorjov, A., Malin, J., Gillespie, M. D., et al. (2019). CyanoGate: a modular cloning suite for engineering Cyanobacteria based on the plant MoClo syntax. Plant Physiol. 180, 39–55. doi: 10.1104/pp.18.01401
Venkata Subhash, G., Rajvanshi, M., Raja Krishna Kumar, G., Shankar Sagaram, U., Prasad, V., Govindachary, S., et al. (2022). Challenges in microalgal biofuel production: a perspective on techno economic feasibility under biorefinery stratagem. Bioresour. Technol. 343:126155. doi: 10.1016/j.biortech.2021.126155
Victoria, A. J., Selão, T. T., Moreno-Cabezuelo, J. Á., Mills, L. A., Gale, G. A. R., Lea-Smith, D. J., et al. (2023). Engineering the highly productive cyanobacterium Synechococcus sp. PCC 11901, 1–22. doi: 10.1101/2023.08.04.552009
Vijayakumar, S., and Angione, C. (2017). “Multi-omic data integration elucidates Synechococcus adaptation mechanisms to fluctuations in light intensity and salinity” in Bioinformatics and biomedical engineering. eds. I. Rojas and F. Ortuño (Cham: Springer International Publishing), 220–229.
Vijayakumar, S., Rahman, P. K. S. M., and Angione, C. (2020). A hybrid flux balance analysis and machine learning pipeline elucidates metabolic adaptation in Cyanobacteria. iScience 23:101818. doi: 10.1016/j.isci.2020.101818
Wang, J., Jia, C.-S., Li, C.-J., Peng, X.-L., Zhang, L.-H., and Liu, J.-Y. (2019). Thermodynamic properties for carbon dioxide. ACS Omega 4, 19193–19198. doi: 10.1021/acsomega.9b02488
Wang, S.-B., Murray, C. I., Chung, H. S., and Van Eyk, J. E. (2013). Redox regulation of mitochondrial ATP synthase. Trends Cardiovasc. Med. 23, 14–18. doi: 10.1016/j.tcm.2012.08.005
Weber, B. H. (2010). What is life? Defining life in the context of emergent complexity. Orig. Life Evol. Biosphere. J. Int. Soc. Study Orig. Life 40, 221–229. doi: 10.1007/s11084-010-9203-4
Wientjes, E., van Amerongen, H., and Croce, R. (2013). Quantum yield of charge separation in photosystem II: functional effect of changes in the antenna size upon light acclimation. J. Phys. Chem. B 117, 11200–11208. doi: 10.1021/jp401663w
Włodarczyk, A., Selão, T. T., Norling, B., and Nixon, P. J. (2020). Newly discovered Synechococcus sp. PCC 11901 is a robust cyanobacterial strain for high biomass production. Commun. Biol. 3, 215–214. doi: 10.1038/s42003-020-0910-8
Yao, L., Shabestary, K., Björk, S. M., Asplund-Samuelsson, J., Joensson, H. N., Jahn, M., et al. (2020). Pooled CRISPRi screening of the cyanobacterium Synechocystis sp PCC 6803 for enhanced industrial phenotypes. Nat. Commun. 11:1666. doi: 10.1038/s41467-020-15491-7
Young, J., McQueen, N., Charalambous, C., Foteinis, S., Hawrot, O., Ojeda, M., et al. (2023). The cost of direct air capture and storage can be reduced via strategic deployment but is unlikely to fall below stated cost targets. One Earth 6, 899–917. doi: 10.1016/j.oneear.2023.06.004
Yu, J., Liberton, M., Cliften, P. F., Head, R. D., Jacobs, J. M., Smith, R. D., et al. (2015). Synechococcus elongatus UTEX 2973, a fast growing cyanobacterial chassis for biosynthesis using light and CO₂. Sci. Rep. 5:8132. doi: 10.1038/srep08132
Zhang, A., Carroll, A. L., and Atsumi, S. (2017). Carbon recycling by cyanobacteria: improving CO2 fixation through chemical production. FEMS Microbiol. Lett. 364:fnx165. doi: 10.1093/femsle/fnx165
Zhao, L., Cai, Z., Li, Y., and Zhang, Y. (2024). Engineering rubisco to enhance CO2 utilization. Synthetic Syst. Biotechnol. 9, 55–68. doi: 10.1016/j.synbio.2023.12.006
Keywords: synechococcus, cyanobacteria, carbon removal, CO2 capture, genetic manipulation
Citation: Kim DS, Moreno-Cabezuelo JÁ, Schulz EN, Lea-Smith DJ and Sagaram US (2024) Recent advances in engineering fast-growing cyanobacterial species for enhanced CO2 fixation. Front. Clim. 6:1412232. doi: 10.3389/fclim.2024.1412232
Edited by:
Melissa Paola Mezzari, Independent researcher, Houston, United StatesReviewed by:
Jianping Yu, National Renewable Energy Laboratory (DOE), United StatesJags Pandhal, The University of Sheffield, United Kingdom
Copyright © 2024 Kim, Moreno-Cabezuelo, Schulz, Lea-Smith and Sagaram. This is an open-access article distributed under the terms of the Creative Commons Attribution License (CC BY). The use, distribution or reproduction in other forums is permitted, provided the original author(s) and the copyright owner(s) are credited and that the original publication in this journal is cited, in accordance with accepted academic practice. No use, distribution or reproduction is permitted which does not comply with these terms.
*Correspondence: David S. Kim, ZGF2aWQua2ltQGN5YW5vY2FwdHVyZS5jb20=