- 1Research Division Future Energy and Industry Systems, Wuppertal Institute for Climate, Environment and Energy, Wuppertal, Germany
- 2Department of Energy Technology, NOWUM-Energy Institute, University of Applied Sciences Aachen, Jülich, Germany
Direct air capture (DAC) combined with subsequent storage (DACCS) is discussed as one promising carbon dioxide removal option. The aim of this paper is to analyse and comparatively classify the resource consumption (land use, renewable energy and water) and costs of possible DAC implementation pathways for Germany. The paths are based on a selected, existing climate neutrality scenario that requires the removal of 20 Mt of carbon dioxide (CO2) per year by DACCS from 2045. The analysis focuses on the so-called “low-temperature” DAC process, which might be more advantageous for Germany than the “high-temperature” one. In four case studies, we examine potential sites in northern, central and southern Germany, thereby using the most suitable renewable energies for electricity and heat generation. We show that the deployment of DAC results in large-scale land use and high energy needs. The land use in the range of 167–353 km2 results mainly from the area required for renewable energy generation. The total electrical energy demand of 14.4 TWh per year, of which 46% is needed to operate heat pumps to supply the heat demand of the DAC process, corresponds to around 1.4% of Germany's envisaged electricity demand in 2045. 20 Mt of water are provided yearly, corresponding to 40% of the city of Cologne‘s water demand (1.1 million inhabitants). The capture of CO2 (DAC) incurs levelised costs of 125–138 EUR per tonne of CO2, whereby the provision of the required energy via photovoltaics in southern Germany represents the lowest value of the four case studies. This does not include the costs associated with balancing its volatility. Taking into account transporting the CO2 via pipeline to the port of Wilhelmshaven, followed by transporting and sequestering the CO2 in geological storage sites in the Norwegian North Sea (DACCS), the levelised costs increase to 161–176 EUR/tCO2. Due to the longer transport distances from southern and central Germany, a northern German site using wind turbines would be the most favourable.
1 Introduction
The correlation between the increase in atmospheric CO2 concentration and the rise in global temperature has been sufficiently documented (IPCC, 2023). In line with the Paris Agreement, the German government's target is to achieve climate neutrality from 2045 by substituting fossil fuels and offsetting unavoidable residual emissions. This offset can be implemented through the use of negative emission technologies that belong to the broader set of carbon dioxide removal (CDR) measures (Renforth et al., 2023). It is, however, unlikely that only one technology alone will be able to meet the demand; rather, a portfolio of negative emission technologies is needed (Minx et al., 2018; Rueda et al., 2021). Current long-term energy and climate scenario analyses focus mainly on a mix of negative emission technologies options strongly related to energy technologies, namely bioenergy with CO2 capture and storage (BECCS) and direct air capture with subsequent CO2 storage (DACCS). Therefore, their possible use must also always be considered in the context of the entire energy system. Each of the options interact with it in different ways and has different impacts on the environment, as discussed by Smith et al. (2016) on the basis of six environmental impact categories. Direct air capture (DAC) is limited primarily by its high demand for electrical and thermal energy. Other factors, such as costs and potential water requirements, can also affect the viability of this technology (Smith et al., 2016; Fasihi et al., 2019; Rueda et al., 2021). While a large number of studies are already available on the use of BECCS, fewer studies and concepts exist with regard to DACCS (Minx et al., 2018). Therefore, this paper focuses solely on the DAC technology.
Although various papers discuss the future development of DAC (National Academies of Sciences, 2019; McQueen et al., 2021b; Ozkan et al., 2022), DAC technology has only been demonstrated at a few plants worldwide so far. As a result, there is at present only limited knowledge about the long-term costs and resource requirements of large-scale implementation (Fasihi et al., 2019; McQueen et al., 2020; Hanna et al., 2021; Lackner and Habib, 2021). The largest plant currently in existence, operated by the Climeworks firm, removes 4,000 tCO2/year from the atmosphere by means of adsorption. A plant with a removal capacity of 1 MtCO2/year is being developed by the Carbon Engineering firm based on absorption (IEA, 2022). However, other processes for capturing CO2 from the atmosphere are also being trialled worldwide (Ozkan et al., 2022).
Up to now, there have been only a few studies that indicate the concrete potential of DAC implementation. Lux et al. (2023) analyse a CDR demand using DAC in the European energy system of 288 MtCO2/year while considering an annual removal of 5% of European emissions in 2050 relative to 1990. For Germany, the demand for DAC is analysed in the context of climate neutrality studies. These studies indicate a need for negative emissions of between 45 and 90 MtCO2/year to achieve climate neutrality in 2045, of which between 17 and 59 MtCO2/year are provided by BECCS and between 15 and 38 MtCO2/year by DACCS (Luderer et al., 2021; Samadi and Lechtenböhmer, 2022; Kullmann and Stolten, 2023). Borchers et al. (2022) analyse 13 CDR options for Germany (various DACCS, BECCS and natural sink enhancement concepts). They attribute a CDR potential of 150 MtCO2/year at centralised DAC farms and an additional 15 MtCO2/year at decentralised plants in combination with ventilation and air conditioning systems. However, none of the studies analysed provide a structural implementation analysis for DAC plants. Specific resources and cost estimates to implement DAC for countries or regions were only found for the U. S. state of California. Baker et al. (2020) describe the possibility of making California carbon-neutral by 2045 by removing 125 MtCO2/year. This includes a DAC implementation strategy of 16 MtCO2/year until 2045.
To the best of the authors' knowledge, there is no assessment of a potential implementation of DAC plants in Germany. The goal of this study is therefore to analyse and comparatively classify the resource requirements (land use, renewable energy and water) and costs of possible DAC implementation pathways for Germany.
The remainder of the paper is organised as follows: Section 2 describes the current state of the art as well as providing a comparison of the existing DAC processes, followed by the methodology and calculation of the chosen indicators. In Section 3, the technical design of four case studies describing potential implementation strategies is presented. Finally, the results regarding the costs and resource consumption are discussed in Section 4, whilst conclusions are drawn in Section 5.
2 Materials and methods
In order to answer the research question, the different DAC technologies are compared and the DAC technology that appears to be most advantageous for Germany in the time horizon considered is selected. We determine a target value for the quantity of CO2 extracted by DAC to achieve German climate neutrality (x MtCO2/year) based on a meta-scenario analysis. This target value serves as the basis for the cost and resource analysis, which we examine in four case studies. Within the case studies, different combinations of technical systems (DAC, heat pump and compressor) and renewable energy systems (PV or wind turbines) are defined using literature-based data and then scaled to the capacity required to achieve German carbon neutrality (Figure 1).
2.1 Selection of a specific DAC technology for the analysis
According to Ozkan et al. (2022), two DAC technology approaches have already been implemented at several demonstration plants and are thus at an advanced stage of development (Table 1). The adsorption process, on the one hand, requires a heat level of ~100°C (“low-temperature”) and is based on a design that enables small-scale solutions on a container basis. Here, adsorption and desorption in vacuum take place cyclically in the same technical space. As a result, the technical components are exposed to strong pressure, temperature and humidity fluctuations, which must be taken into account in the choice of construction materials. Due to the changing process phases, continuous operation is only possible by staggering the operation of several modules (Beuttler et al., 2019). Some “low-temperature” DAC systems produce water as a by-product. The amount varies depending on the adsorption medium and environmental conditions. Fasihi et al. (2019) reported that between 0.8 and 2 tonnes of water are captured per tonne of CO2. However, due to the additional energy required, it is reasonable to minimise the amount of water produced. There are currently 17 adsorption plants in operation, mainly in Europe. All plants are operated by the Climeworks and Global Thermostat (IEA, 2022).
The absorption process, on the other hand, works at a heat level of 900°C (“high-temperature”). The separation of absorption and desorption ensures continuous operation at ambient pressure reduced stress on components due to temperature and pressure swing, as well as protection of the plant components. In contrast to the “low-temperature” approach, this process requires water. According to Ozkan et al. (2022), the water consumption comes to 1–13 tH2O/tCO2. The process can be applied in central large-scale solutions. As both large equipment components such as the calciners or steam slakers and high-temperature process heat are required, significantly larger capacity blocks than the one used in the adsorption process are needed, which makes upscaling by adding more units more complex. The only pilot plant, currently operated by the Carbon Engineering firm, uses natural gas with a downstream carbon capture and storage (CCS) process to produce the required high temperature (Keith et al., 2018). Alternative heat sources could be hydrogen, electricity or concentrating solar power. In addition, McQueen et al. (2021a) describe the possible use of an all-electric calciner, which is not yet commercially available and its development is therefore difficult to predict. Besides the companies mentioned above, numerous other companies are also working on DAC on a laboratory and pilot scale and exploring further DAC approaches (IEA, 2022; Ozkan et al., 2022).
Compared to high-temperature heat, low-temperature heat is much easier to provide using renewable energy or waste heat from industry. Due to its small-scale design, smaller energy potentials can also be utilised or it can be easier to develop smaller sites. In contrast, the generation of high-temperature heat in a system based on renewable energy, as is intended in Germany by 2045 at the latest, would require large-scale DAC plants that burn hydrogen, for example, or use concentrating solar power or nuclear energy. The available climate neutrality studies for Germany, however, assume that green hydrogen, which will be scarce for a long time, should only be consumed in “no-regret” applications like synthetic aviation fuels, feedstocks and green steel production. The use of concentrating solar power is not realistic in Germany, however, due to limited direct normal irradiation potential. The use of an electrical calciner in combination with renewables or nuclear energy is also difficult to predict due to the low level of technological development. Furthermore, the use of nuclear power is not an option due to the German nuclear phase-out by 2023 but would be an option for other countries (McQueen et al., 2021a). Therefore, to perform the intended analysis, the “low-temperature” process was chosen as the basis for a reference plant for a potential rollout in Germany. This technology appears to be most advantageous for Germany in the time horizon under consideration due to its need for process temperatures of around 100°C and its ability to provide (instead of requiring) water in the process (Beuttler et al., 2019; National Academies of Sciences, 2019).
2.2 Selecting a climate neutrality scenario for deriving a DAC target
Based on a meta-scenario analysis, an exemplarily DAC quantity required to achieve climate neutrality in Germany is determined as a target value. This target value serves as the basis for the cost and resource analysis. All scenarios known to the authors, which are in line with the German government's target for a climate-neutral Germany by 2045, require the capture and the permanent storage of CO2 or its use as feedstock. They differentiate between natural and technical sinks as well as between process emission related CCS and negative emissions. However, not all of these options are examined in the various scenarios. In 2045, the need for CO2 capture is given as 55–116 MtCO2/year (Figure 2), whereby different assumptions, framework conditions and focuses of the authors shape the results differently (Boston Consulting Group, 2021; Deutsche Energie-Agentur, 2021; Luderer et al., 2021; Prognos et al., 2021; Fraunhofer-Institut ISI, 2022; Kullmann and Stolten, 2023). The possibility of using DAC is mentioned in all of these studies, but only Prognos et al. (2021), Fraunhofer-Institut ISI (2022), and Kullmann and Stolten (2023) state specific amounts of DACCS in their basic scenarios for 2045. Boston Consulting Group (2021) provide for additional DAC quantities in the event that the measures on land use, land use change and forestry (LULUCF) do not come into effect as envisaged in their baseline scenarios (see the split line). Luderer et al. (2021) rely primarily on the use of BECCS, although they do not exclude the possibility of using other measures for CDR (see the split line).
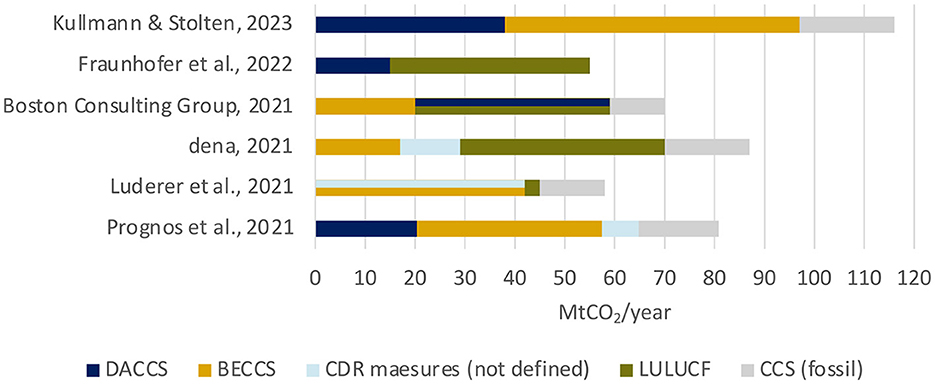
Figure 2. Comparison of German climate neutrality scenarios for the reference year 2045 regarding their assumed CO2 capture measures. Source: own representation.
The demand for negative emissions in our analysis is based on Prognos et al. (2021), using their CNGE 2045 (‘‘Climate-Neutral Germany”) scenario. This scenario was chosen because it appears to be the most transparent scenario regarding the assumptions on different CO2 capture measures, for example the use of “low-temperature” DAC and its assumed energy consumption. Furthermore, it presents a kind of a middle-of-the-road scenario among the three scenarios that envisage the use of DACCS [CO2 capture rates of 15, 20, and 38 MtCO2/year according to Prognos et al. (2021), Fraunhofer-Institut ISI (2022), Kullmann and Stolten (2023), respectively]. The CNGE 2045 scenario represents an integrated scenario that takes into account greenhouse gas emissions from the energy, industry, transport, buildings, agriculture and waste management sectors and considering the manifold interconnections between these sectors. In this way the emissions are reduced by 65% to 438 MtCO2/year in 2030 and by 95% to 65 MtCO2/year in 2045, compared to 1990 levels. So-called unavoidable, remaining emissions in 2045 result primarily from agriculture and, to a low extent, from individual industrial sectors and waste management, whose emissions cannot be further reduced for various reasons. To compensate for these, the authors envisage negative emissions through BECCS and DACCS (along with other options). Together, the demand for CDR measures is increased to 65 MtCO2/year in 2045, of which 20 MtCO2/year is removed by DACCS. This 20 MtCO2 is defined as our target value for the quantity of CO2 to be extracted by DAC.
2.3 Definition of the case studies
In order to assess the impacts of installing the derived DAC capacity, in Section 3, four case studies on possible DAC implementation pathways are defined. They differ in terms of the choice of region and the respective renewable energy generation, namely onshore wind turbines and photovoltaics (PV). Regions in northern, central and southern Germany are selected in order to analyse different framework conditions with regard to the renewable energy potential or the CO2 transport distance. Electrical air-source heat pumps are considered to meet the heat demand of the DAC plants. It is assumed that the CO2 extracted from the atmosphere will be prepared for transport to the coast via CO2 pipelines. Based on current corporate plans, the port of Wilhelmshaven (WHV), located on the German part of the North Sea, is taken as a possible port for CO2 transfer (OGE, 2023) to potential storage sites in the North Sea. The cost and resource analysis is conducted for each case study and the results are presented both specifically (per tonne of CO2) and in absolute figures. All assumptions regarding costs and resources are for 2045, using literature-based expectations regarding technical developments and extrapolations.
2.4 Carbon capture efficiency
Even if only renewable energy or waste heat is used to operate the DAC plant, the carbon capture efficiency, defined as the ratio of carbon captured to carbon removed, is usually <1. This is because, in addition to the emissions from operation, the embodied emissions generated during the production processes of the facilities required must also be taken into account. Deutz and Bardow (2021), for example, calculate an efficiency of 96.5% for a similar DAC system in the case of wind and PV, based on 2050. Terlouw et al. (2021) analyse the highest efficiency in countries with a low-carbon electricity mix and the use of waste heat. Since we assume a nearly climate-neutral economy in Germany in 2045, there will be almost no emissions from domestic production processes. However, since the UN Framework Convention on Climate Change (UNFCCC) only considers domestic greenhouse gas emissions in the national GHG emission balance, we neglect possible embodied emissions from production abroad in order to be consistent with the chosen CNGE 2024 scenario. We therefore assume that the carbon removed by the DAC plants installed in Germany corresponds to the same amount of carbon captured.
The efficiency of the DAC process is also strongly influenced by the ambient conditions and the process engineering parameters. Wurzbacher et al. (2012) demonstrate that the adsorption time and humidity have a direct influence on the adsorbed amount of H2O and CO2, while the temperature has only a minor effect. This only becomes relevant when it gets warmer than 50°C (Sendi et al., 2022), which is not the case in Germany. The higher the humidity in the environment, the more CO2 and H2O can be bound by the adsorbent. However, the amount of adsorbed H2O increases more than CO2. Therefore, a favourable CO2/H2O ratio for the adsorption process occurs at low humidity (Sendi et al., 2022). This advantage is based on the fact that the desorption of water causes an additional thermal energy requirement, which should be avoided for efficiency reasons. As the humidity only varies slightly between the analysed regions in Germany over the course of the year (Climate Data, 2024), we assume no difference in energy demand. Furthermore, it is assumed that the adsorption and desorption processes of the DAC systems in the different case studies are operated at the same rhythm, so that the adsorption time is also assumed to be constant.
2.5 Defining central energy demand parameters
In every case study, the electricity required to operate the technical systems (DAC, heat pump and compressor) is assumed to be supplied by renewable energy plants based on an annual balance. This means that the amount of energy produced over the course of a year is equal to the amount of energy consumed by the technical systems over the same period. The parameters described below, and the corresponding sources are listed in Table 3 for the technologies selected in the case studies.
The required capacity of the renewable energy systems (PRE) in kW is derived from the total annual electrical energy demand of the technical systems (Eel,total) in kWh and the annual specific electrical energy yield of the renewable energy systems based on the region-specific full load hours (FLHRE) in h (Equation 1). Eel,total is divided into the direct electricity demand (Eel,direct) of DAC and compression unit and the electricity demand (Equations 2, 3). Eel,PowerToHeat resulting from the heat (Eth,hp), that is required by the DAC technology and provided by a heat pump. Eel,PowerToHeat is calculated by applying the heat pump's coefficient of performance (COP) (Equation 4). The specific heat demand (eth,sp) and the specific electricity demand (eel,sp), defined as the energy demand per tonne of CO2, are multiplied by the annual amount of CO2 removed (nCO2) to determine the total energy demand per DAC, air-source heat pump and compression unit (Eel,DAC, Eth,hp and Eel,c, respectively) (Equations 5–7).
where
Storage options for heat and electricity and storage capacities are not considered in this analysis. Instead, it is assumed that surplus electricity is fed into the grid and taken out of the grid at the time of underproduction (the resulting possible margin of error is addressed in the discussion). The energetic and material flows as well as the interconnection of the modules are shown schematically in Figure 3.
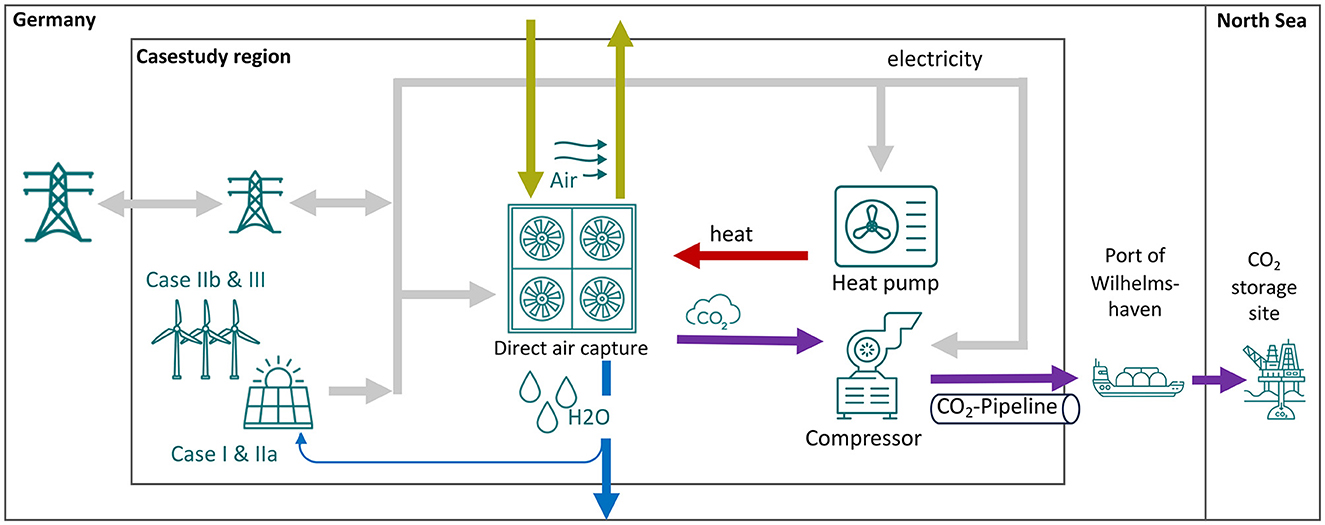
Figure 3. Schematic illustration of the basic energetic and material flows in the modelled cases. Source: own illustration.
Using hourly feed-in time series for renewable energy plants and an assumed constant demand profile for the DAC modules with maintenance intervals, the extent to which the DAC modules can be supplied self-sufficiently is examined. For this purpose, the degree of self-sufficiency (D) is defined, which indicates what percentage of Eel,total can be provided directly by the renewable energy systems (Eel,RE). To determine Eel,RE, the energy quantities provided by the renewable energy systems at time t [ERE(t)] are summed up over 8,760 hours (Equation 8). If the energy production at time t exceeds the energy demand Eel(t), that means if ERE(t) > Eel(t), only the share of renewable energies required to cover the energy demand is added.
The electrical capacity of the heat pump (Php) is calculated by dividing the electric demand of the heat pump (Eel,PowerToHeat) by the FLHhp of the air-source heat pump (Equation 9). Similarly, the electrical capacity of the compressor (Pc) is determined by the electric demand of the compressor (Eel,c) and the FLHc of the compressor (Equation 10).
2.6 Deriving resource consumption and costs
Table 3 lists the main resource consumptions and cost assumptions described below as well as the corresponding sources. The land use is differentiated with regard to the building area of the technical systems (Ap) and the area for renewable energy provision (ARE), including the necessary clearance areas due to shading. For this purpose, the specific building area of the individual technical systems (DAC technology, compressors and air-source heat pumps) (asp,tech.sys) and the renewable energy plants (asp,RE) is multiplied by the number of the corresponding plants capacity (Ptech.sys and PRE, respectively) (Equations 11, 12).
The total water production (Wtotal) is calculated using the specific water production values of the DAC system (Wsp.production) times the amount of CO2 removed (nCO2) minus the operational water consumption of the renewable energy plants, which is the specific consumption (wsp.consum) per kWh times the electricity provided by the renewable energy plants (Eel,total) in kWh (Equation 13). Only the direct water use is considered, while any water consumption in the life cycle of the technical systems is not included in our analysis.
The capture costs are expressed both as the total investment costs (CAPEXtotal) and the levelised cost of carbon dioxide (LCOCO2DAC) and refer to newly built plants of the year 2045. The CAPEX results from the sum of the costs of the technical systems (CAPEXtech.sys) plus the renewable energy systems (CAPEXRE; see Table 3) (Equation 14).
The LCOCO2DAC consist of the annuity CAPEX, the annual fixed costs (OPEXfix) and the annual variable costs (OPEXvar) (Equation 15). The interest rate, expressed as weighted average cost of capital (WACC), and the depreciation period (m) to determine the annuity factor (a) are determined individually for each technology, based on literature values. All costs are allocated to the annual amount of CO2 removed (nCO2) (Equation 16). It is assumed that the WACC for the DAC plants, heat pumps, and compressors are of the same order of magnitude, while PV and wind turbines have lower WACC. The basic assumption for the latter is that there is less uncertainty about the economic viability of the renewable energies. Although the CO2 capture and treatment by the DAC technology, along with the associated compressor and heat pump, are subject to a common risk of failure, the renewable energy plants can still operate even if the CO2 capture and treatment fails. This represents a separate business model based on electricity marketing, resulting in a lower risk of capital loss and therefore, a lower WACC.
Additionally, the cost of CO2 transport and sequestration are estimated. Since detailed network planning is not part of this paper and costs may vary considerably depending on the future CO2 grid design and the scaling, only rough figures from the literature are used. In order to make the LCOCO2DAC from the different case studies comparable, first the onshore transport cost LCOCO2Trans.Onshore from the respective regions to the port of WHV are estimated, taking into account the transport volume of 20 MtCO2/year in each case study. The cost of DAC plus the onshore transport to WHV are then calculated in Equation 17 as,
In order to provide the total cost of DACCS, the costs for offshore CO2 transport and storage (LCOCO2WHVtoS) must be added (Equation 18). Here, it is assumed that the captured CO2 will be transported by offshore pipeline to a potential storage site in the Norwegian North Sea.
3 Design of the case studies
3.1 Defining the location of DAC plants
In order to highlight the different impacts of powering DAC facilities using renewable energy from either PV or wind turbines, the resource impacts and the costs are analysed along four case studies. These differ in the choice of region (variation of both the distance to the port of WHV and the renewable energy potential) for the technical systems (Table 2, Figure 4). Regions in southern, central, and northern Germany were chosen so that they are close to trunk lines for CO2 transport as outlined for a planned CO2 network by OGE (2023).
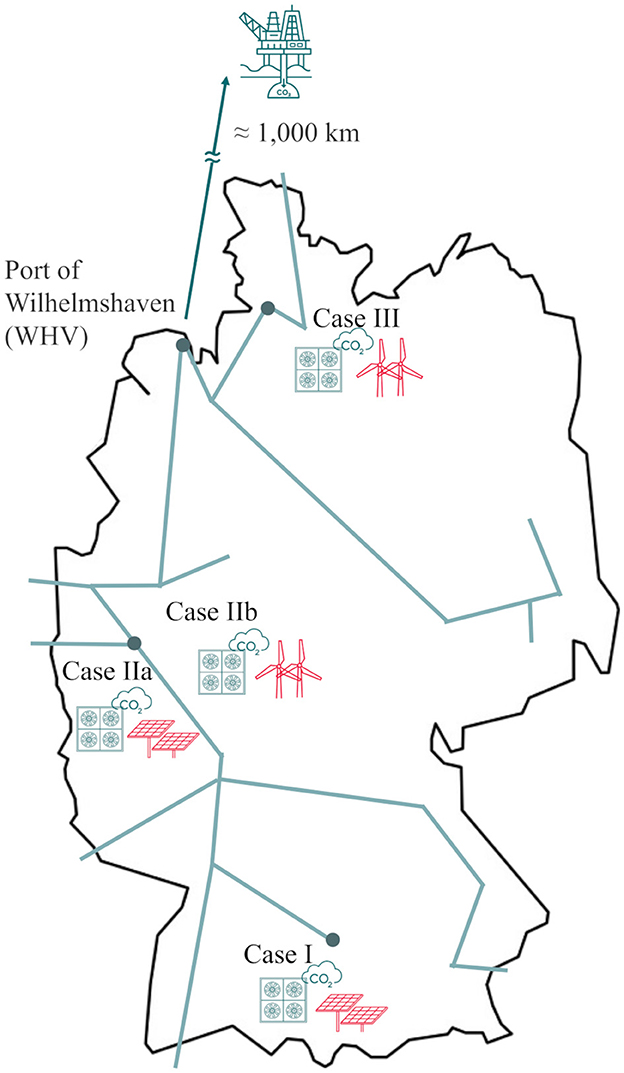
Figure 4. Illustration of the locations of the selected regions in cases I–III. Source: own illustration, based on a CO2 trunk line as part of a future CO2 network roughly outlined by OGE (2023).
Case I: In south Germany, Ulm (750 km to WHV), which is close to the start of the planned CO2 network, is selected. Due to the high solar irradiation in southern Germany (Borchers et al., 2022), only coupling with PV is considered.
Case IIa and case IIb: In central Germany, a CO2 grid feed-in from the Rhenish lignite mining area (Garzweiler, close to the city of Cologne) is considered (400 km to WHV). Here, the “Delta Rhine Corridor,” “Rhenish coalfield cluster,” and “WHVCO2logne” (OGE, 2023) projects overlap, which is why the probability of implementing a CO2 strategy is high. Furthermore, because of the planned coal phase out in Germany by 2030, the Rhenish lignite mining area is presently undergoing a strong transition with major renewable energy projects being planned (MWIKE.NRW, 2022) and new innovative industries potentially emerging. In this regard, both the application of PV and the implementation of wind turbines with regional average values are being considered. This region might also become highly relevant due to its proximity to the Ruhr area, as climate-neutral CO2 will also be needed in the future to supply the chemical clusters there for the production of green feedstock.
Case III: In northern Germany (distance to WHV 100 km), only the combination of coastal near onshore wind turbines using the prevailing high wind potential (Borchers et al., 2022) and DAC is examined.
In line with the CNGE 2045 scenario, the thermal energy is provided by electrically driven air-heat pumps in all cases. This avoids the need to build additional infrastructure to transport heat or hydrogen for heat generation. In addition, compression of the captured CO2 to 150 bar is considered, which prepares the CO2 for transport. Other possible cases, such as the use of industrial waste heat, are considered in the discussion section.
3.2 Framework conditions
Since high FLH are required to operate DAC plants economically (Fasihi et al., 2019), 7,000 FLH are assumed for the DAC plants. This is also in accordance with the selected CNGE 2045 scenario. However, due to the assumption of high DAC-FLH compared to the much lower FLH of renewable electricity production, the question arises as to which grade of DAC plant self-sufficiency might still be justifiable. On the one hand, the direct coupling of DAC plants with electricity producers does not place any load on the power grid. On the other hand, volatile renewables are unlikely to match the steady energy demand of a DAC plant, so that full self-sufficiency would lead to high investment costs. Therefore, connexion to the German electricity grid is assumed. Since the general conditions of the energy market in 2045 are very uncertain, it is assumed for the sake of simplicity that the exchange itself is cost- and resource-neutral. The degree of self-sufficiency D (Equation 8) is used as an auxiliary variable to represent additional storage requirements. In order to determine D, feed-in time series given by Entsoe (2021) are adapted to the selected regions. They are compared with the assumed constant demand profile of the DAC modules with maintenance intervals, and the times of over- or underproduction are identified. This is then used to analyse the times when electricity has to be drawn from the grid or fed into the grid. Alternative designs, such as excessively increasing the size of renewable energy plants and feeding surplus energy into the national power grid, were not taken into consideration.
3.3 Technology data
In this section, the core data used for the calculation and the assumed technological progress of the main technologies (DAC, heat pump, compressor, and PV and wind turbines) are derived (see Table 3). Cost data from literature sources have been inflation adjusted to the base year 2020 using data from the Statistisches Bundesamt (2023).
3.3.1 Direct air capture
When considering the key figures for DAC technology, it is important to bear in mind that this is a technology at the demonstration stage. Projections up to 2045 are subject to great uncertainty and depend on many factors. In this paper, a DAC plant capacity of 4,000 tCO2/year is assumed, which corresponds to the largest “low-temperature” DAC plant (“Orca”) currently in operation. The small-scale container-based design, however, will allow larger or smaller plants to be built in the future by interconnecting several containers (“Orca” also already consists of stacked containers). While the selected plant size equals 0.26 m2/(tCO2·year), larger plants are expected to reduce the specific land use to 0.07 m2/(tCO2·year) (Deutz and Bardow, 2021). However, since the DAC plant area is only a fraction of the area covered by PV and wind energy plants used for electricity and heat generation (Terlouw et al., 2021), this upscaling effect is negligible. Moreover, larger DAC plants would not necessarily be more energy efficient than smaller systems, since their main energy consumption results from the temperature increase of the adsorbent in order to release the CO2 from the adsorbent (Wurzbacher et al., 2012; Sendi et al., 2022). Therefore, we have refrained from analysing a virtually upscaled large plant.
In contrast to widely varying information on the current energy demand, assumptions for 2045 are becoming increasingly similar. Our assumptions rely on Hanna et al. (2021) and National Academies of Sciences (2019) who state the minimum energy demand of a DAC system as 944 kWhth/tCO2 and 286 kWhel/tCO2. Fasihi et al. (2019) indicate an energy demand of 1,102 kWhth/tCO2 and 182 kWhel/tCO2 by 2050.
In contrast, there is great uncertainty regarding the future costs. Hanna et al. (2021) calculate the CAPEX in 2050 at 711 EUR/(tCO2·year), while Fasihi et al. (2019) assume cost of 199 EUR/tCO2·year. Both estimate the OPEX at 4% of the annuity investment cost. While Hanna et al. (2021) assume an expansion of DAC capacity of 50 MtCO2/year by 2030 (inter alia, due to a supposed lack of production capacity), Fasihi et al. (2019) assume a capacity expansion of up to 473 MtCO2/year by 2030 in their base case. This is the main reason for the large cost differences, as Fasihi et al. (2019) already achieve the highest cost reduction by 2030 due to early assumed learning effects. This paper is based on the conservative figures taken from Hanna et al. (2021), as it seems unlikely that the capacities assumed by Fasihi et al. (2019) for 2030 will be reached in the next 6 years at the current level of expansion.
The annuity factor (Equation 16) is determined using a WACC of 8% and a depreciation period of 25 years (IEA, 2022). The assumption for the WACC is also in line with the current trend regarding the average WACC according to KPMG (2023). IEA (2022) reports a water production range of 0.8–2 tH2O/tCO2, depending on the respective climate conditions. Since a decrease in water production results in energy savings, for this study a constant production rate of 1 tH2O/tCO2 is assumed until 2045.
3.3.2 Air-heat pumps
A heat level of ~100°C is required to operate the DAC process. The temperature level can be provided by a variety of technologies or by using waste heat. This paper focuses on heat supply via an industrial air compression heat pump. Other heat sources like geothermal, ocean or seawater are not considered, in line with the assumption of the CNGE2045 scenario. The assumed COP of 2.85 is in line with Deutz and Bardow (2021) and DEA (2016b). The depreciation period is set at 20 years and the WACC at 8%. The CAPEX are assumed to be 720,000 EUR/MW and the OPEX are estimated at 700 EUR/(MW·year) (OPEXfix) and 1.6 EUR/MWh (OPEXvar) (DEA, 2016b). There is no requirement for water consumption and the land requirement is estimated at 600 m2/MW (DEA, 2016b).
3.3.3 Photovoltaics
To generate electricity from PV, large ground-mounted tracking systems with a generation capacity of 40 MW per unit are considered. The yearly performance per MW for 2045 is estimated at 1,306 MWh/MW in case I and 1,197 MWh/MW in case IIa. The values were calculated based on Kost et al. (2021) with an annual increase in FLH of 0.34% and correspond to a performance of 1,200 and 1,100 MWh/MW in 2020, respectively (World Bank Group, 2023). The depreciation period is estimated to be 30 years (DEA, 2016a) and the WACC at 3.7% (Kost et al., 2021). The specific space requirement is 14,000 m2/MW, while the CAPEX are estimated at 350,000 EUR/MW and the OPEX at 9,000 EUR/MW (DEA, 2016a). The operational water consumption, in particular that which is required to clean the PV surface, is assumed to be 0.045 tH2O/MWh (Jin et al., 2019).
3.3.4 Onshore wind turbines
Electricity generation from large-scale onshore plants is assumed to take place in cases IIb and III with a generation capacity of 6 MW per unit. For this paper, values from Kost et al. (2021) were used, which indicate yields of 1,800 MWh/MW from inland wind turbines (case IIb) and 3,200 MWh/MW from wind turbines near the coast (case III) for 2020. Accounting for a yearly increase in FLH of 0.5%, the turbines are predicted to produce 2,039 and 3,625 MWh/MW in 2045, respectively. The depreciation period of the plants is estimated at 25 years (DEA, 2016a), while the WACC stands at 4.2% (Kost et al., 2021). The land requirement is differentiated according to building area and distance area, whereby the distance area includes the building area. For a 6 MW wind turbine, a building area of 4,800 m2 (800 m2/MW) and a distance area of 300,000 m2 (50,000 m2/MW) is set. The CAPEX are estimated at 1,020,000 EUR/MW. The OPEXfix and the OPEXvar are estimated at 12,000 EUR/MW and 1,3 EUR/MWh, respectively. According to Jin et al. (2019), no operational water consumption is assumed.
3.3.5 Compressors
The CO2 is compressed to a pressure of 150 bar and prepared for transport via pipeline. In line with the operating time of the DAC plants, 7,000 FLH are assumed. The estimated depreciation period is 25 years, the WACC is set at 8%, and the electricity demand is estimated at 101 kWh/tCO2 (Deutz and Bardow, 2021). The CAPEX are assumed at 4 EUR/(tCO2·year) and the OPEX are estimated at 5% of CAPEX. The required space is calculated by multiplying the throughput per hour with the specific value of 0.3 m2/(tCO2·hour) (DEA, 2021).
3.4 CO2 transport and storage
CO2 transport cost estimates vary significantly from source to source (Sleiti et al., 2022). Since the development of a potential CO2 transport net in Germany is also currently highly speculative, CO2 transport cost can only be calculated very roughly. For a rough estimate, data from Sleiti et al. (2022) are taken, who compiled corresponding data from ZEP (2011) for transporting a capacity of 20 MtCO2/year, the capacity we also consider for DAC. The onshore transport cost LCOCO2Trans.Onshore for transport from the different regions to the port of WHV are calculated using the figures given for onshore pipelines, inflation-adjusted from 2010 to 2023.
LCOCO2Trans.Onshore (case I) = 1 EUR/tCO2 assuming a distance of 100 km,
LCOCO2Trans.Onshore (cases IIa and IIb) = 4 EUR/tCO2 assuming a distance of 400 km,
LCOCO2Trans.Onshore (case III) = 7.5 EUR/tCO2 assuming a distance of 750 km.
For the offshore transport cost, it is assumed that the captured CO2 will be transported by offshore pipeline to a potential storage site in the Norwegian North Sea, 1,000 km away from the port of WHV. Similar to the onshore transport, inflation-adjusted costs of 14 EUR/tCO2 are derived.
Storage cost of 18 EUR/tCO2 for a potential deep saline aquifer are conservatively assumed in line with DEA (2021) based on a WACC of 8%. Transport and storage costs together result in LCOCO2WHVtoStorage of 34 EUR/tCO2.
4 Results
Table 4 shows the values resulting from the implementations of the DAC technology in 2045. In addition, the key figures are compared with the consumption for Cologne, the fourth largest city in Germany with 1.1 million inhabitants. However, it should not be assumed that the resource and energy consumption is directly related to the city of Cologne. The purpose is to illustrate the order of magnitude of the calculated values.
4.1 Energy demand
Through the use of heat pumps, the entire final energy demand of the DAC plants is supplied electrically. The electric heat generation accounts for 46% of the final energy demand. The total electrical energy demand of 14.4 TWh/year corresponds to 1.4% of Germany's electricity demand as determined in the CNGE 2045 scenario for 2045. It corresponds to 45% of the electricity demand of private households in the city of Cologne in 2019 (Stadt Köln, 2022). It is about one third lower than what is assumed in the CNGE 2045 scenario for the operation of the DAC plants (20.7 TWh/year). This might be due to the fact that the scenario assumes more conservative learning rates for the DAC technology, resulting in a higher specific energy demand.
Due to the different energy producers and the different FLH, the electrical capacity to be installed varies for the use of PV from 11.0 GW (case I) to 12.0 GW (case IIa), which represents 3.0 and 3.3% of the PV capacity that is assumed to be installed for the whole of Germany by 2045. In the case of wind turbines, it varies from 7.1 GW (case IIb) to 4.0 GW (case III), which is equivalent to 4.9 and 2.8% of what is assumed for Germany by 2045, respectively. While the onshore wind turbines achieve 81% (case IIb) and 82% (case III) self-sufficiency, the use of PV modules only leads to 44% (cases I and IIa) self-sufficiency. This is due to the fact that the 7,000 FLH assumed for the DAC plants cover only 80% of the year, which leads to a structural undersupply, especially in months with limited solar hours.
4.2 Land use
The land required by the DAC plants itself amounts to 7.5 km2 which corresponds to 2% of the area of the city of Cologne. The land use due to electricity production is more than 90% greater. 161.4–175.4 km2 are required for PV construction, which corresponds to 42–44% of the area of the city of Cologne. However, the extent to which an area can be optimally used for solar energy generation depends on its orientation and location and additionally influences the area required. While the building area for onshore wind turbines is about 4.0–2.4 km2, large areas of 352.5–198.3 km2 are required between the turbines. This area corresponds to 51 and 89% of the area of Cologne.
4.3 Water use
It is assumed that water is produced as a by-product in the DAC process at a rate of 1 tH2O/tCO2. In addition, only the process-related water consumption or process-related water utilisation is considered; the upstream and downstream demand or production in the life cycle is not assessed. The operational water demand of PV, in particular for cleaning the PV modules, leads to a reduction in the water produced by ~0.032 tH2O/tCO2, while no operational demand is assumed in the case of wind energy (see Section 2.6). Thus, a quantity of 19.4 MtH2O/year is provided in the cases I and II.a, and 20 MtH2O/year in the cases II.b and III. These figures correspond to 42 and 44% of Cologne's water demand, respectively (based on average German per capita water consumption of 45.6 KtH2O/year) (Bdew, 2023). 2.1 Mt (cases I and II.a) and 2.2 Mt (cases II.b and III) of hydrogen could be produced stoichiometrically if the water were used as a feedstock. These figures only serve to illustrate the order of magnitude. The provision of potable water or hydrogen involves further expenses for facilities and infrastructure that have not been considered here.
4.4 Cost of direct air capture
Figure 5 illustrates both the resulting cost of capturing CO2 (coloured areas) and the cost of subsequent transport and storage of CO2 (grey areas). Just considering the capture and compression of 20 MtCO2/year, costs of 2.4–2.7 billion EUR/year are incurred, which equal specific costs of 125, 126, 138, and 126 EUR/tCO2 in cases I, IIa, IIb, and III, respectively. The largest cost driver is the DACCAPEX (62–68% of the total cost), which makes reducing the technology cost key to cost-effective operation. It should be noted that the absolute values of DACCAPEX and DACOPEX do not change across the four case studies, as no regional differences in operating parameters were assumed (Section 2.3). The renewable energy plants share increases from 13% (case I) to 21% (cases IIa and III) to 20% (case IIb) if CO2 is only captured. The higher value of wind energy in case IIb compared to case III is explained by the fact that only 50% of the FLH is achieved inland compared to the coast. Including transport and storage too, the share of renewables decreases to a range of 9–16%, respectively.
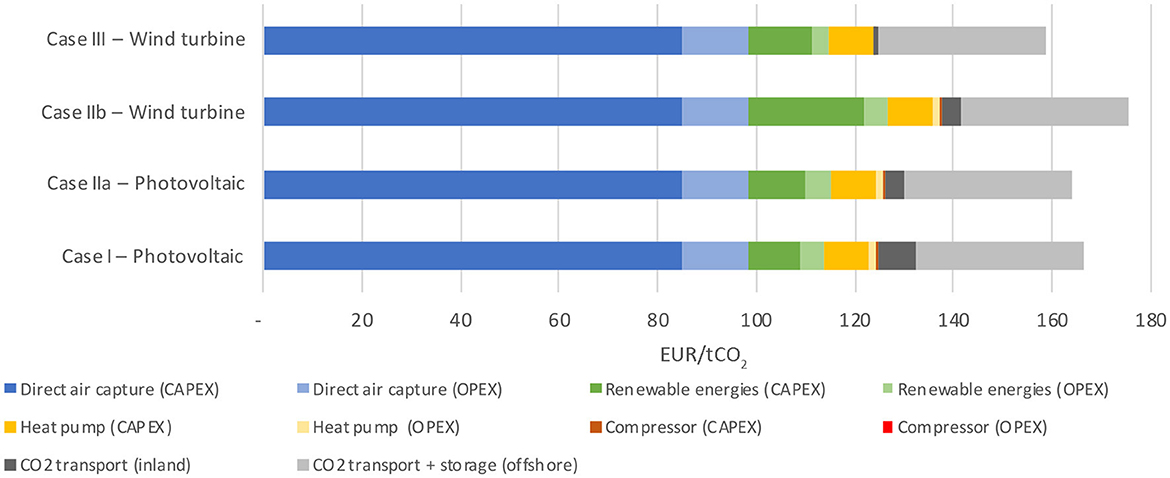
Figure 5. Comparison of the levelised costs LCOCO2 of the four cases as analysed for 2045 (coloured areas refer to DAC only, while the full bars refer to DACCS). Costs are shown in Euro per tonne of CO2. Source: own illustration.
The LCOCO2DAC in case I represents the most cost-effective removal cost, but the removal costs in cases IIa and III are also of about the same order of magnitude (difference of 0.6 and 0.4%). However, due to the assumption that the captured CO2 will be transported to the port of WHV, the much longer transport distance from the location in southern Germany results in higher additional costs (7.5 EUR/tCO2 in case I) than for the sites in central Germany (4 EUR/tCO2 in the cases IIa and IIb) and northern Germany (1 EUR/tCO2 in case III).
As a result, case III achieves the most cost-effective LCOCO2DACtoWHV of 127 EUR/tCO2. Case IIa also achieves lower LCOCO2DACtoWHV costs than case I (130 instead of 132 EUR/tCO2). Both cases benefit from shorter transport distances to the port. However, it should be noted that wind turbines achieve a higher proportion in terms of energy supply, whereas PV is subject to higher generation fluctuations. Compensating for this volatility is associated with costs that are not calculated in this study, but are discussed below.
If also considering the transport of CO2 from Germany to the assumed storage sites in the Norwegian North Sea with subsequent storage, an additional 34 EUR/tCO2 must be added. This finally results in total LCOCO2DACCS of 166, 164, 176 and 161 EUR/tCO2 in cases I, IIa, IIb, and III, respectively.
5 Discussion
5.1 Classification of the findings
To capture and subsequently compress CO2 to 150 bar within Germany, LCOCO2DAC of 125 to 138 EUR/tCO2 were determined for 2045. Including transport to the port of WHV and the subsequent offshore transport and storage, the LCOCO2DACCS were found to be 161–176 EUR/tCO2. These results are within the range of Lux et al. (2023), who calculated costs of 60 to 140 EUR/tCO2 with progressive assumptions and 160–270 EUR/tCO2 with conservative assumptions for a capture rate of up to 288 MtCO2/year in the EU. Their figures also include the costs of storing the CO2 of about 10 EUR/tCO2. Lehtveer and Emanuelsson (2021) state costs of 170–180 EUR/tCO2, calculated for both sunny and windy regions. Capture costs are lower in sunny regions (central Spain, therefore a higher PV share) than in windy regions (Ireland, therefore a higher wind share). As in Lux et al. (2023), their cost assumptions include the cost of sequestration. Furthermore, the reported specific costs for 2050 in Hanna et al. (2021) of between 130 and 220 EUR/tCO2, depending on the renewable energy supply and whether or not energy storage is used, are of about the same order of magnitude. Young et al. (2023) point out that all previous cost studies have set the cost escalation up to a FOAK plant too low and therefore assume excessive cost reductions due to the learning process starting too early. According to their assumptions, globally, costs of 200–550 USD/tCO2, corresponding to 185–500 EUR/tCO2, result for the solid sorbent technology considered here in 2050.
Apart from the amount of land used by the renewable energy plants and the transportation distance to the coast, the results of the three regions differ primarily in terms of the costs of renewable energies. Thereby, the combination of PV systems in south Germany with the DAC process (case I) was determined to be the most cost-effective option for CO2 capture (without transport and storage). However, this is only valid under the simplified assumption of cost- and resource-neutral feed-in and feed-out to the electricity grid. In reality, only 44% self-sufficiency is achieved. Compared to cases IIb and III, both using wind turbines with 81% and 82% self-sufficiency, this implies that a larger amount of electricity has to be provided and taken up by the electricity grid. This is likely to result in additional costs that are currently not included in the cost calculations.
As the future market situation cannot be estimated, the potential additional costs are discussed on the basis of a worst-case scenario in 2023. When analysing electricity spot market prices from 2023, it can be seen that 87% of the trading volume was between 0 and 0.15 EUR/kWh and around 99% between −0.05 and 0.2 EUR/kWh (Netztransparenz, 2024). Considering only the 87% case and assuming that non-usable amounts of electricity from our case regions are sold at the least favourable time (0 EUR/kWh) and the same amounts are purchased at the most expensive time (0.15 EUR/kWh), additional costs of 0.15 EUR/kWh may be incurred. This would result in additional LCOCO2DAC of 60, 60, 20, and 19 EUR/tCO2 in cases I, IIa, IIb, and III, meaning a surcharge of 49, 49, 15, and 16%, respectively. Figure 6 illustrates the development of the potential additional costs in general as a function of the electricity procurement costs that cannot be covered by the direct use of the assumed renewable energy plants. It further shows that the use of wind turbines in combination with DAC is advantageous compared to PV from prices of <0.01 EUR/kWh (case III) and <0.05 EUR/kWh (case IIb). The higher self-sufficiency of cases IIb and III thus leads to a much lower burden on the electricity grid, which might be advantageous for the energy transition strategy. This is in line with Lehtveer and Emanuelsson (2021) who conclude that DAC technology demonstrates high performance especially in interaction with wind turbines.
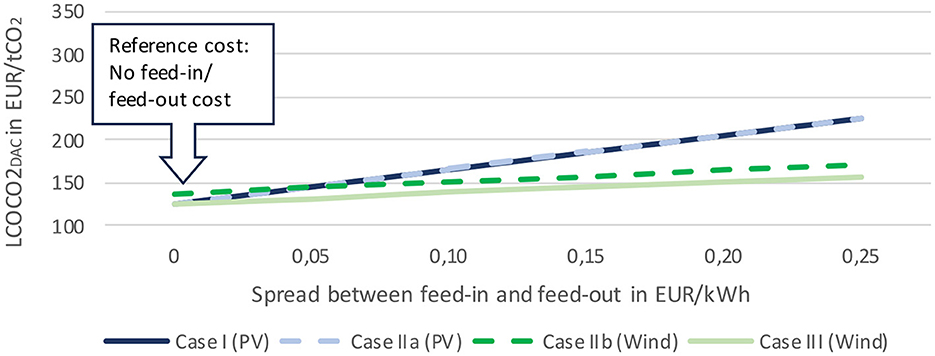
Figure 6. Comparison of the LCOCO2DAC of the four cases with additional electricity procurement costs for the energy feed-in and feed-out from the grid. Source: own representation.
A large-scale implementation of DAC facilities in coastal areas of northern Germany has the further advantage of heavily reduced CO2 transport costs from inland to a port if the captured CO2 is to be transported farther to storage sites in the North Sea. Considering both the greater self-sufficiency and lower transport costs, our results suggest that a northern German DAC implementation strategy in combination with onshore and offshore wind parks is to be considered advantageous compared to the south.
Overall, the specific CO2 removal costs must be put into perspective when considering that the European Union's emissions trading scheme price is already moving between 80 and 100 EUR/tCO2 in 2023. Since the price can be expected to rise in line with the political targets in the EU and globally, even higher costs than calculated would become economically viable. For example, a maximum allowances' price of around 160 EUR/tCO2 in the EU by 2030, inflation adjusted to EUR2022, is expected according to a survey conducted by Kopernikus-Projekt Ariadne (2022). Furthermore, other advantages are to be expected from rolling out a German DAC strategy, such as national value creation, the generation of technical know-how, leading by example and, associated with this, a large export market.
5.2 Uncertainties
This paper only provides an initial overview of the costs and resources involved in DAC implementation. The energy yields from renewable energy plants and the calculated land requirement correspond to high potential values that can be achieved in Germany. The figures thus represent the minimum land requirement and most effective energy yield, which will probably be exceeded if a national DACCS strategy is implemented. Analysing and forecasting renewable energy yields from different locations is a highly complex process that depends on a wide range of factors. Simplified assumptions for energy production have been used, which are associated with certain inaccuracies and are not applicable to specific locations. However, since the share of the costs for renewable energy production (PV or wind turbines) is only 13–21% of the LCOCO2DAC, even considerably worse locations would not have a major impact on the resulting costs.
As shown above, the DACCAPEX contributes 62–68% of the total cost of CO2 capture. Therefore, three key parameters are varied to determine their influence on the DACCAPEX, which are the technology learning rate, the FLH and the WACC.
(1) The greatest uncertainty with regard to the LCOCO2 is the 2045 cost assumption for the DAC technology due to its relatively low technology readiness level of 6 on a scale of 1–10 (IEA, 2022). The literature data on the DACCAPEX, which are based on learning rates and other assumptions, vary by up to 260% (Fasihi et al., 2019; Breyer et al., 2020; DEA, 2021; Hanna et al., 2021). If the relatively low DACCAPEX of 196 EUR/(tCO2·year) (Breyer et al., 2020, see Section 3.3.1) is applied instead of the DACCAPEX of 724 EUR/(tCO2·year) (Hanna et al., 2021) we used in our calculation, the LCOCO2DAC would decrease from 125 to 138 EUR/tCO2 to 58 to 68 EUR/tCO2. If such a low DACCAPEX were reached in the future, the share of the costs for renewable energy production would increase to 25–37% in our four cases. In that case, it would be essential to actually look for high-potential renewable energy regions.
(2) The FLH of the DAC plant is a factor that affects the CO2 removal capacity per DAC unit. Assuming 8,500 instead of 7,000 FLH, which we used in our calculation, the LCOCO2DAC decreases by 17–18% from 125 to 138 EUR/tCO2 to 102.9 to 115.1 EUR/tCO2. At 5,500 FLH, the LCOCO2DAC increases by 18–20% from 150.2 to 162.4 EUR/tCO2 (Figure 7). Due to higher FLH and the resulting change in the total electricity demand over time, the degree of self-sufficiency decreases from 44 to 40% in cases I and IIa, from 81 to 72.6% in case IIb, and from 82 to 73.3% in case III. In contrast, it increases to 53% in cases I and IIa, to 87.6% in case IIb, and to 89.2% in case III when the FLH is reduced. This shows that lower FLH requires a higher number of DAC units, resulting in higher investment costs. Conversely, a lower number of DAC units resulting from higher FLH results in a lower investment cost. Higher FLH requires a more constant energy supply, which is contrary to the operation of renewable energies and is reflected in the development of the degree of self-sufficiency. The resulting increase in feed-in and feed-out processes into and from the electricity grid leads to higher LCOCO2, as discussed in Section 5.1. Conversely, lower FLH results in a higher degree of self-sufficiency and fewer exchange processes with the electricity grid, leading to lower additional costs. The optimal FLH therefore depends on various influencing factors that needs to be analysed and evaluated individually for each specific site.
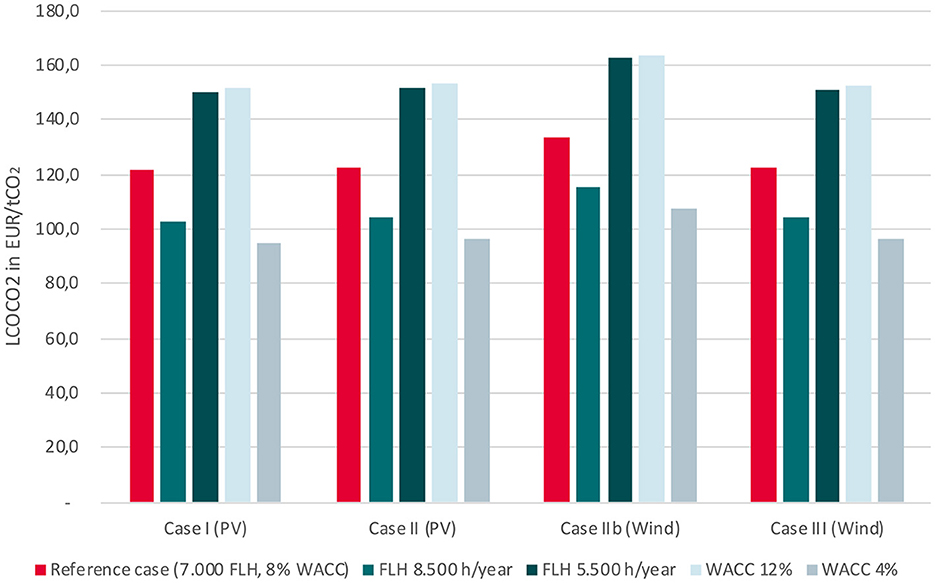
Figure 7. The impact of full load hours (FLH) and weighted average cost of capital (WACC) on the development of the LCOCO2. Source: own representation.
(3) Different WACC rates are applied in the studies on the cost development of DAC, be it 2% by Lux et al. (2023), 5% by Sendi et al. (2022), or 7% as an “average” of 5.6% and 11.7% by Fasihi et al. (2019). Hanna et al. (2021) use a WACC of 0%, based on the assumption of government-mandated expenses. This illustrates both the range of economic assumptions and the uncertainty that exists in this area. Varying the WACC by −4% or +4% compared to the 8% we used in our calculation (4 and 12%) shows that the LCOCO2DAC decreases by around 22 to 24% and reaches a range of 95.1–107.3 EUR/tCO2 or rises by 19–21% to 151.5–163.8 EUR/tCO2, respectively (Figure 7).
Figure 7 also illustrates that both an increase in the FLH (second column) and a decrease in the WACC (the fifth column), can achieve similar cost reductions. However, increasing the FLH is associated with high uncertainties regarding the feed-in and feed-out processes in a future energy market (Section 5.1), while a lower in the WACC could be brought about by creating a positive investment environment. This is in line with Young et al. (2023), who emphasise that larger projects need political incentives to reduce the cost of capital in the long term. Possible approaches include institutional financing, state-owned enterprises, the application of feed-in tariffs, production tax credits, or, according to Hanna et al. (2021), government-mandated expenses.
What will be crucial for large-scale availability is the production capacity of DAC technology, as not only Germany but also a large part of the international community will have a demand for negative emissions. While Hanna et al. (2021) assume a global DAC demand of up to 2.3 GtCO2/year by 2050 regarding capacity constraints, Fuss et al. (2018) even estimate a demand of up to 5 GtCO2/year from 2050 onwards. The IPCC scenarios calculate a requirement of 220–360 GtCO2 in the second half of century (IPCC, 2023), which corresponds to rates of 4.4–7.2 GtCO2/year (of which DAC, however, would only account for part). To meet such a high demand, industrial production lines are needed, which usually involve long planning, construction and start-up phases, which is why the industry's growth rate can be quite a critical obstacle (Hanna et al., 2021). At the same time, large-scale production of DAC plants offers the opportunity to quickly realise cost reductions in manufacturing. This needs to be considered in a global context and has not been analysed in this paper.
Another uncertainty describes the cost of CO2 transport, since an estimate of transport volumes heavily depends on future development of German or European CO2 transport networks. The continued use of fossil fuels combined with CCS would lead to a high CO2 transport volume, while greater electrification and a hydrogen strategy, only taking into account CCS for “unavoidable” CO2 emissions such as from cement factories, would reduce the CO2 transport demand. Since the transport costs depend significantly on the transport distance, solutions with short transport distances within north Germany would have cost advantages.
5.3 Further needs of research
In addition to the case studies considered here, a number of other factors are conceivable that could have a relevant influence on costs and resource consumption. These should be weighed against each other in an integrated analysis in order to pinpoint optimal locations.
In order to reduce or avoid CO2 transportation costs, an alternative would be to instal the DAC plants close to CO2 storage sites. However, since previous publications do not indicate any political ambition to enable CO2 storage in Germany at this point in time (Merk et al., 2022), an approach using offshore storage was chosen. If regional storage sites were to be included, an optimal relationship between renewable energy yields, storage capacity and transportation costs might be found. In addition to the issue of transport costs, onshore CO2 storage may lead to greater public acceptance, which could, for example, in turn lead to shorter implementation periods and cost reductions in planning.
Furthermore, the question of an appropriate heat supply is likely to have a relevant influence both on resources and costs. Since about 46% of the electricity demand is converted into heat if heat pumps are assumed as in our model, a large amount of electricity could be saved if waste heat were to be used. Borchers et al. (2022) and Baus and Nehr (2022) describe the possibility of combining DAC with ventilation and air conditioning systems. Another option would be the use of waste heat from industry, for which various studies have identified a high potential (Brueckner et al., 2017; König et al., 2019). For the most part, the existing waste heat is in the low-temperature range and would therefore harmonise well with the “low-temperature” DAC technology. The small-scale design, as a further benefit of the “low-temperature technology,” allows the DAC capacity to be customised based on the quantity of waste heat or moving air mass in the air conditioning systems. While low-cost or even cost-neutral heat would lead to a reduction in the LCOCO2, an adapted decentralised use and transport strategy would have to be developed which would involve the bundling of small quantities of CO2 and might therefore be more costly. Additionally, it should be considered that DAC systems require high FLH to be operated economically, which depend on a relatively constant energy supply.
This also raises the question of the optimal size of the DAC systems. The system capacity assessed in this paper corresponds to a CO2 removal of 4,000 tCO2/year, which means that 6,258 DAC facilities would be required to remove 20 MtCO2/year from the atmosphere, considering the assumed 7,000 FLH. Larger DAC systems, such as the “Mammoth” system planned by Climeworks which will sequester 36,000 tCO2/year, would only require 696 facilities. The size of the plants in an optimised system could then depend on the proximity to industrial waste heat sources and the distance to CO2 pipelines. Furthermore, scaling the system size could also reduce the specific space requirement.
Lehtveer and Emanuelsson (2021) state that considering LCOCO2 alone can be misleading and that, instead, the impact of such technical systems on the overall energy system needs to be considered in order to facilitate informed decisions. Therefore, an extended analysis should consider the integration of DAC plants into the energy system. A cost optimisation of the overall system consisting of electricity and DAC plants under the secondary condition of the amount of CO2 to be captured and the available land potential might provide further information for an implementation strategy.
Another question arises regarding the process- and environment-dependent parameters described in Section 2.3. To make strategic decisions in favour for or against a German or European DAC (CS) strategy, it is necessary to conduct a more detailed analysis of the resource consumption and costs. Additionally, external factors such as humidity or the availability of a continuous renewable energy supply must be further examined and their impact presented. As a continuation of this work, site-specific analyses and implementation strategies might be employed in regions that are identified as more advantageous than Germany.
In general, the intended implementation of such a complex new system also requires far more than just a techno-economic analysis (Viebahn et al., 2019; Fujimori et al., 2023). From a holistic point of view, further assessment aspects such as ecological effects, legal considerations regarding the concrete regulatory framework and questions of social acceptance as well as synergy effects with other infrastructure projects such as CO2 transport for conventional CCS or the planning of a hydrogen pipeline network should be examined. These and similar questions should be addressed in a comprehensive technology assessment and investigated with broad stakeholder participation, as is the case with other technologies of this scale.
In addition to the amounts of DAC for geological storage considered here, there could be further demand for DAC in the provision of green feedstock for industry. This is needed to produce synthetic chemicals and fuels such as sustainable aviation fuels from green hydrogen. Cases IIa and IIb in particular were chosen as exemplary locations close to energy-intensive industry (for example refineries in Cologne and chemical parks in the Ruhr area). Only low transportation costs would then have to be added to the LCOCO2 derived here. But the conversion of the Rhenish lignite mining area itself to a climate-neutral region is also likely to increase the demand for CO2 for power-to-x processes. Accordingly, further studies on the integration of DAC into a regional economy would be necessary.
Likewise to the possible need for DAC for carbon capture and utilisation, the need for DACCS is also very difficult to estimate. While the CNGE 2045 scenario selected assumes 20 MtCO2/year for DACCS in 2045, Kullmann and Stolten (2023) indicate a need for DACCS of up to 38 MtCO2/year. This could increase significantly if other negative emission potentials such as BECCS or natural sinks cannot be used (Figure 2). Moreover, if the ambitious reduction pathway to reduce greenhouse gas emissions by 95% cannot be implemented (“overshooting”), additional residual emissions would need to be offset by negative emissions. Furthermore, none of the existing climate neutrality scenarios for Germany takes into account the non-CO2 effects of aviation which might also have to be offset by further removals from the atmosphere. In particular, much greater efforts will be needed to achieve the high levels of net-negative emissions (getting below zero) mentioned that the IPCC considers necessary in its scenarios (IPCC, 2023).
Last but not least, the need for larger CO2 removal capacities requires further analyses for planning and implementation. If, for example, 200 MtCO2/year had to be offset by DACCS instead of the 20 MtCO2/year analysed, this would mean 10 times the building area for the DAC systems of 7.5 km2 under the given assumptions. A 10-fold electricity demand in the amount of 144 TWh/year would correspond to 14% of the total German electricity demand in 2045. Taking into account the higher energy demand and land use, the question arises as to whether Germany can meet the infrastructural, energy, environmental, and political implications of such a scenario or whether cooperation with countries with more favourable renewable energy (“hot spots”) and larger areas might be necessary.
6 Conclusion
The costs and resources examined in this paper show that the main challenges for implementing DAC in Germany are the space requirements for the energy supply itself. Since long runtimes are important to operate DAC economically, the combination with fluctuating renewable energies leads to an imbalance in the generation and use of electrical energy. In this paper, energy storage technologies have not been considered, which means that surplus electrical energy must be fed into the grid and additional electrical energy must be purchased in times of low renewable energy generation.
Our results show that the cost of DAC in Germany might reach 125–138 EUR/tCO2 (only capture and compression) and 161–176 EUR/tCO2 (including transport and sequestration), if no additional electricity balancing costs are considered. The combination of onshore wind turbines in the north with the DACCS process was identified as the most cost-effective option. Even though the combination of DAC and PV has slightly lower costs for capturing CO2 (0.4%), it only achieves 44% self-sufficiency. In contrast, the use of wind turbines has a self-sufficiency of up to 82%. This is also shown by the worst-case analysis, according to which possible additional costs for feed-in and feed-out processes to and from the electricity net come to 60 EUR/tCO2 in the case of photovoltaics and only 19 EUR/tCO2 in the case of wind energy in the north. Further advantages include the resulting lower load on the electricity grid (and possible associated cost savings), as well as the short transport distances to CO2 storage sites in the North Sea. Furthermore, significant quantities of electricity are currently being transported from north to south Germany due to regional imbalances between generation and demand, which means that additional consumers with low space requirements are better off in the north. This leads to a reduction in the load on the power grid. Therefore, the expansion of energy-intensive DAC plants should be encouraged in northern Germany.
Data availability statement
The raw data supporting the conclusions of this article will be made available by the authors, without undue reservation.
Author contributions
SB: Conceptualisation, Data curation, Methodology, Resources, Visualisation, Writing – original draft, Validation. PV: Conceptualisation, Funding acquisition, Methodology, Project administration, Resources, Supervision, Writing – review & editing. CJ: Conceptualisation, Methodology, Writing – review & editing.
Funding
The author(s) declare financial support was received for the research, authorship, and/or publication of this article. The authors gratefully acknowledge the financial support of the German Federal Ministry for Research and Education (BMBF) for the development of advanced power-to-x processes (grant no. 03SF0627C). Sole responsibility for the content of this paper lies with the authors.
Acknowledgments
This paper is based on the master's thesis Block (2021), parts of which were published in the journal Annales des Mines (Block and Viebahn, 2022). For this paper, both the methodology and the results were revised and significantly expanded.
Conflict of interest
The authors declare that the research was conducted in the absence of any commercial or financial relationships that could be construed as a potential conflict of interest.
Publisher's note
All claims expressed in this article are solely those of the authors and do not necessarily represent those of their affiliated organizations, or those of the publisher, the editors and the reviewers. Any product that may be evaluated in this article, or claim that may be made by its manufacturer, is not guaranteed or endorsed by the publisher.
Abbreviations
BECCS, bioenergy with CO2 capture and storage; CDR, carbon dioxide removal; CNGE, climate-neutral Germany; DAC, direct air capture; DACCS, direct air capture with subsequent CO2 storage; FLH, full load hours; LULUCF, land use, land use change and forestry; WHV, Wilhelmshaven.
References
Baker, S. E., Stolaroff, J. K., Peridas, G., Pang, S., Goldstein, M., Lucci, F. R., et al. (2020). Getting to Neutral: Options for Negative Carbon Emissions in California. Livermore, CA: Lawrence Livermore National Laboratory, LLNL-TR-796100.
Baus, L., and Nehr, S. (2022). Potentials and limitations of direct air capturing in the built environment. Build. Environ. 208:108629. doi: 10.1016/j.buildenv.2021.108629
Bdew (2023). Entwicklung des personenbezogenen Wassergebrauchs. Available online at: https://www.bdew.de/service/daten-und-grafiken/entwicklung-des-personenbezogenen-wassergebrauches/ (accessed November 23, 2023).
Beuttler, C., Louise, C., and Wurzbacher, J. (2019). The role of direct air capture in mitigation of anthropogenic greenhouse. Front. Clim. 1:10. doi: 10.3389/fclim.2019.00010
Block, S. (2021). Auslegung, Analyse und Bewertung von Direct Air Capture (DAC)-Anlagen zur Nutzung für Power-to-X-Prozesse und zur Erzielung “negativer Emissionen” in Deutschland. (Master's thesis), Fachhochschule Aachen, Aachen, Germany; Wuppertal Institut, Wuppertal, Germany.
Block, S., and Viebahn, P. (2022). Direct Air Capture (DAC) in Germany: resource implications of a possible rollout in 2045. Annales des Mines – Responsabilité et Environnement. 105, 78–82. doi: 10.3917/re1.105.0078
Borchers, M., Thrän, D., Chi, Y., Dahmen, N., Dittmeyer, R., Dolch, T., et al. (2022). Scoping carbon dioxide removal options for Germany-What is their potential contribution to Net-Zero CO2? Front. Clim. 4:810343. doi: 10.3389/fclim.2022.810343
Boston Consulting Group (2021). CLIMATE PATHS 2.0 - A Program for Climate and Germany's Future Development. Available online at: https://www.bcg.com/germany/klimapfade (accessed November 23, 2023).
Breyer, C., Fasihi, M., and Aghahosseini, A. (2020). Carbon dioxide direct air capture for effective climate change mitigation based on renewable electricity: a new type of energy system sector coupling. Mitig. Adapt. Strateg. Glob. Chang. 25, 43–65. doi: 10.1007/s11027-019-9847-y
Brueckner, S., Arbter, R., Pehnt, M., and Laevemann, E. (2017). Industrial waste heat potential in Germany-a bottom-up analysis. Energy Efficiency 10, 513–525. doi: 10.1007/s12053-016-9463-6
Climate Data (2024). Klimadaten für Städte, Orte und Reiseziele weltweit. Available online at: https://de.climate-data.org/ (accessed January 12, 2024).
DEA (2016a). Technology Data - Energy Plants for Electricity and District Heating Generation. Copenhagen: Danish Energy Agency and Energinet. Available online at: https://ens.dk/sites/ens.dk/files/Analyser/technology_data_catalogue_for_el_and_dh.pdf (accessed July 15, 2023).
DEA (2016b). Technology Data for Individual Heating Plants. Copenhagen: Danish Energy Agency and Energinet. Available online at: https://ens.dk/sites/ens.dk/files/Analyser/version_03_-_technology_data_catalogue_for_heating_installations.pdf (accessed July 15, 2023).
DEA (2021). Technology Data - Carbon Capture, Transport and Storage. Copenhagen: Danish Energy Agency and Energinet. Available online at: https://ens.dk/sites/ens.dk/files/Analyser/technology_data_for_carbon_capture_transport_and_storage.pdf (accessed July 15, 2023).
Deutsche Energie-Agentur (2021). dena-Leitstudie Aufbruch Klimaneutralit?t. [Dena Lead Study Setting of Climate Neutrality]. Available online at: https://www.dena.de/en/newsroom/news/dena-pilot-study-towards-climate-neutrality/ (accessed November 23, 2023).
Deutz, S., and Bardow, A. (2021). Life-cycle assessment of an industrial direct air capture process based on temperature-vacuum swing adsorption. Nat. Energy 6, 203–213. doi: 10.1038/s41560-020-00771-9
Entsoe (2021). Actual Generation per Production Type. Available online at: https://transparency.entsoe.eu/generation/r2/actualGenerationPerProductionType/show (accessed July 27, 2023).
Fasihi, M., Efimova, O., and Breyer, C. (2019). Techno-economic assessment of CO2 direct air capture plants. J. Clean. Prod. 224, 957–980. doi: 10.1016/j.jclepro.2019.03.086
Fraunhofer-Institut ISI, Consentec, ifeu, TU Berlin. (2022). Treibhausgasneutrale Szenarien 2045 - Industriesektor. Available online at: https://langfristszenarien.de/enertile-explorer-wAssets/docs/LFSIII_Webinar16.11.2022_Industrie_final.pdf (accessed November 20, 2023).
Fujimori, S., Nishiura, O., Oshiro, K., Hasegawa, T., Shiraki, H., Shiogama, H., et al. (2023). Reconsidering the lower end of long-term climate scenarios. PLoS Clim. 2:318. doi: 10.1371/journal.pclm.0000318
Fuss, S., Lamp, W. F., Callaghan, M. W., Hilaire, J., Creutzig, F., Amann, T., et al. (2018). Negative emissions-Part 2: costs, potentials and side effects. Environ. Res. Lett. 13:e063002. doi: 10.1088/1748-9326/aabf9f
Hanna, R., Abdulla, A., Xu, Y., and Victor, D. (2021). Emergency deployment of direct air capture as a response to the climate crisis. Nat. Commun. 12:368. doi: 10.1038/s41467-020-20437-0
IEA (2022). Direct Air Capture 2022. Available online at: https://www.iea.org/reports/direct-air-capture-2022 (accessed February 19, 2023).
IPCC (2023). Climate Change 2023: Synthesis Report. Contribution of Working Groups I, II and III to the Sixth Assessment Report of the Intergovernmental Panel on Climate Change [Core Writing Team, H. Lee and J. Romero (eds.)] (Geneva: IPCC), 184.
Jin, Y., Behrens, P., Tukker, A., and Scherera, L. (2019). Water use of electricity technologies: a global meta-analysis. Renew. Sustain. Energy Rev. 115:109391. doi: 10.1016/j.rser.2019.109391
Keith, D. W., Holmes, G., St Angelo, D., and Heidel, K. (2018). A process for capturing CO2 from the atmosphere. Joule 2, 1573–1594. doi: 10.1016/j.joule.2018.05.006
König, J., Sommer, M., Groß, B., Hoffmann, P., Winkler, M., Bach, D., et al. (2019). Erhebung, Abschätzung und Evaluierung von industrieller Abwärme in Deutschland - Potentiale und Forschungsbedarf: Abschlussbericht des Verbundvorhabens: Fraunhofer Institut für Physikalische Messtechnik (IPM).
Kopernikus-Projekt Ariadne (2022). The EU-ETS Price Through 2030 and Beyond: A Closer Look at Drivers, Models and Assumptions. Input Material and Takeaways From a Workshop in Brussels, 30 November 2022. Available online at: https://ariadneprojekt.de/media/2023/01/Ariadne-Documentation_ETSWorkshopBruessel_December2022.pdf (accessed November 09, 2023).
Kost, C., Shammugam, S., Fluri, V., Peper, D., Dovoodi Memar, A., and Schlegl, T. (2021). Stromgestehungskosten erneuerbare Energien. Freiburg: Fraunhofer ISE. Available online at: https://www.ise.fraunhofer.de/de/veroeffentlichungen/studien/studie-stromgestehungskosten-erneuerbare-energien.html (accessed November 09, 2023).
KPMG (2023). Cost of Capital Study 2023. Available online at: https://kpmg.com/de/en/home/insights/2023/10/cost-of-capital-study-2023.html (accessed January 12, 2024).
Kullmann, F., and Stolten, D. (2023). Energieperspektiven 2030. Available online at: https://www.fz-juelich.de/de/iek/iek-3/aktuelles/meldungen/energieperspektiven-2030 (accessed November 23, 2023).
Lackner, K., and Habib, A. (2021). Buying down the cost of dircet air capture. Industr. Eng. Chem. Res. 60, 8196–8208. doi: 10.1021/acs.iecr.0c04839
Lehtveer, M., and Emanuelsson, A. (2021). BECCS and DACCS as negative emission providers in an intermittent electricity system: why levelized cost of carbon may be a misleading measure for policy. Decisions Front. Clim. 3:647276. doi: 10.3389/fclim.2021.647276
Luderer, G., Kost, C., and Sörgel, D. (2021). Deutschland auf dem Weg zur Klimaneutralität 2045 - Szenarien und Pfade im Modellvergleich (Potsdam: Potsdam Institute for Climate Impact Research), 359.
Lux, B., Schneck, N., Pfluger, B., Männer, W., and Sensfuß, F. (2023). Potentials of direct air capture and storage in a greenhouse gas-neutral European energy system. Energy Strategy Rev. 45:101012. doi: 10.1016/j.esr.2022.101012
McQueen, N., Desmond, M. J., Socolow, R. H., Psarras, P., and Wilcox, J. (2021a). Natural gas vs. electricity for solvent-based direct air capture. Front. Clim. 2:618644. doi: 10.3389/fclim.2020.618644
McQueen, N., Psarras, P., Pilorge, H., Liguori, S., He, J., Yuan, M., et al. (2020). Cost analysis of direct air capture and sequestration coupled to low-carbon thermal energy in the United States. Environ. Sci. Technol. 54, 7542–7551. doi: 10.1021/acs.est.0c00476
McQueen, N., Vaz Gomes, K., McCormick, C., Blumanthal, K., Pisciotta, M., and Wilcox, J. (2021b). A review of direct air capture (DAC): scaling up commercial technologies and innovating for the future. Prog. Energy 3:e032001. doi: 10.1088/2516-1083/abf1ce
Merk, C., Nordø, Å. D., Andersen, G., Lægreid, O. M., and Tvinnereim, E. (2022). Don't send us your waste gases: public attitudes toward international carbon dioxide transportation and storage in Europe. Energy Res. Soc. Sci. 87:102450. doi: 10.1016/j.erss.2021.102450
Minx, J. C., Lamb, W. F., Callaghan, M. W., Fuss, S., Hilaire, J., Lenzi, D., et al. (2018). Negative emissions: part 1: research landscape and synthesis. Environ. Res. Lett. 13:e063001. doi: 10.1088/1748-9326/aabf9b
MWIKE.NRW (2022). Land und 50 Kommunen, Energie-Unternehmen und Projektträger schließen Gigawattpakt für Erneuerbare im Rheinischen Revier. Ministerium für Wirtschaft, Industrie, Klimaschutz und Energie des Landes Nordrhein-Westfalen. Available online at: https://www.wirtschaft.nrw/pressemitteilung/gigawattpakt-rheinisches-revier (accessed November 23, 2023).
National Academies of Sciences Engineering, and Medicine. (2019). Negative Emissions Technologies and Reliable Sequestration: A Research Agenda. Washington, DC: The National Academies Press.
Netztransparenz (2024). Spotmarktpreis nach § 3 Nr. 42a EEG. Available online at: https://www.netztransparenz.de/de-de/Erneuerbare-Energien-und-Umlagen/EEG/Transparenzanforderungen/Marktpr%C3%A4mie/Spotmarktpreis-nach-3-Nr-42a-EEG (accessed January 14, 2024).
OGE (2023). CO2-Netz. Available online at: https://co2-netz.de/de#co2-netz (accessed March 03, 2023).
Ozkan, M., Nayak, S. P., Ruiz, A. D., and Jiang, W. (2022). Current status and pillars of direct air capture technologies. iScience 24:103990. doi: 10.1016/j.isci.2022.103990
Prognos Öko-Institut, and Wuppertal Institut. (2021). Towards a Climate-Neutral Germany by 2045. How Germany Can Reach Its Climate Targets Before 2050. Executive Summary Conducted for Stiftung Klimaneutralität, Agora Energiewende and Agora Verkehrswende. Berlin: Stiftung Klimaneutralität, Agora Energiewende and Agora Verkehrswende.
Renforth, P., Bellamy, R., Beerling, D., Boettcher, M., Bonalumi, D., Brandão, M., et al. (2023). Specialty grand challenge: renaming our section to “Carbon Dioxide Removal”. Front. Clim. 5:1279109. doi: 10.3389/fclim.2023.1279109
Rueda, O., Moggollon, J. M., Tukker, A., and Scherer, L. (2021). Negative-emissions technology portfolios to meet the 1.5 °C target. Glob. Environ. Change 67:102238. doi: 10.1016/j.gloenvcha.2021.102238
Samadi, S., and Lechtenböhmer, S. (2022). Klimaneutralität bis 2045 - Vergleich der Entwicklungen im Energiesystem in aktuellen Szenarien für Deutschland. Energiewirtschaftliche Tagesfragen 72:3. Available online at: https://nbn-resolving.org/urn:nbn:de:bsz:wup4-opus-79259 (accessed February 15, 2024).
Sendi, M., Bui, M., Mac Dowell, N., and Fennell, P. (2022). Geospatial analysis of regional climate impacts to accelerate cost-efficient direct air capture deployment. One Earth 5, 1153–1164. doi: 10.1016/j.oneear.2022.09.003
Sleiti, A. K., Al-Ammari, W. A., Vesely, L., and Kapat, J. S. (2022). Carbon dioxide transport pipeline systems: overview of technical characteristics, safety, integrity and cost, and potential application of digital twin. J. Energy Resour. Technol. 144:092106. doi: 10.1115/1.4053348
Smith, P., Steven, J. D., Creutzig, F., Fuss, S., Minx, J., Gabrielle, B., et al. (2016). Biophysical and economic limits to negative CO2 emissions. Nat. Clim. Change 6, 42–50. doi: 10.1038/nclimate2870
Stadt Köln (2022). Nachhaltigkeitsbericht der Stadt Köln 2022. Available online at: https://www.stadt-koeln.de/mediaasset/content/pdf15/nachhaltigkeitsbericht.pdf (accessed January 19, 2024).
Statistisches Bundesamt (2023). Verbraucherpreisindex und Inflationsrate. Available online at: https://www.destatis.de/DE/Themen/Wirtschaft/Preise/Verbraucherpreisindex/_inhalt.html#sprg475762 (accessed January 19, 2024).
Terlouw, T., Treyer, K., Bauer, C., and Mazzotti, M. (2021). Life cycle assessment of direct air carbon capture and storage with low-carbon energy sources. Environ. Sci. Technol. 55, 11397–11411. doi: 10.1021/acs.est.1c03263
Viebahn, P., Scholz, A., and Zelt, O. (2019). The potential role of direct air capture in the German energy research program: results of a multi-dimensional analysis. Energies 12:18. doi: 10.3390/en12183443
World Bank Group, ESMAP, and Solargis. (2023). Global Solar Atlas. Available online at: https://globalsolaratlas.info/map (accesses November 22, 2023).
Wurzbacher, J. A., Gebald, C., Piatkowski, N., and Steinfeld, A. (2012). Concurrent separation of CO2 and H2O from air by a temperature - vacuum swing adsorption/desorption cycle. Environ. Sci. Technol., 46, 9191–9198. doi: 10.1021/es301953k
Young, J., McQueen, N., Charalambous, C., Renforth, P., Garcia, S., van der Spek, M., et al. (2023). The cost of direct air capture and storage can be reduced via strategic deployment but is unlikely to fall below stated cost targets. One Earth 6, 899–917. doi: 10.1016/j.oneear.2023.06.004
ZEP (2011). The Costs of CO2 Transport: Post-Demonstration CCS in the EU. Brussels: European Technology Platform for Zero Emission Fossil Fuel Power Plants. Available online at: https://zeroemissionsplatform.eu/document/the-costs-of-co2-capture-transport-and-storage/ (accessed November 22, 2023).
Keywords: direct air capture, DAC, climate neutrality, carbon dioxide removal, negative emissions, Germany, economics, rollout
Citation: Block S, Viebahn P and Jungbluth C (2024) Analysing direct air capture for enabling negative emissions in Germany: an assessment of the resource requirements and costs of a potential rollout in 2045. Front. Clim. 6:1353939. doi: 10.3389/fclim.2024.1353939
Received: 11 December 2023; Accepted: 08 February 2024;
Published: 26 February 2024.
Edited by:
Ben W. Kolosz, University of Hull, United KingdomReviewed by:
Volker Sick, University of Michigan, United StatesStephen McCord, University of Michigan, United States
Copyright © 2024 Block, Viebahn and Jungbluth. This is an open-access article distributed under the terms of the Creative Commons Attribution License (CC BY). The use, distribution or reproduction in other forums is permitted, provided the original author(s) and the copyright owner(s) are credited and that the original publication in this journal is cited, in accordance with accepted academic practice. No use, distribution or reproduction is permitted which does not comply with these terms.
*Correspondence: Simon Block, c2ltb24uYmxvY2tAd3VwcGVyaW5zdC5vcmc=