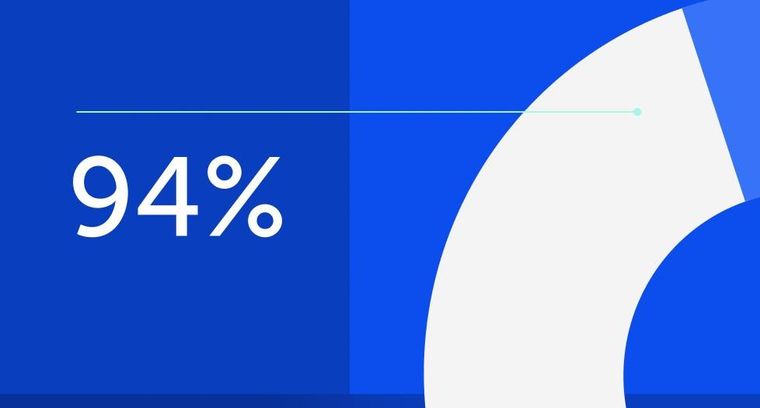
94% of researchers rate our articles as excellent or good
Learn more about the work of our research integrity team to safeguard the quality of each article we publish.
Find out more
REVIEW article
Front. Clim., 19 June 2024
Sec. Carbon Dioxide Removal
Volume 6 - 2024 | https://doi.org/10.3389/fclim.2024.1345224
This article is part of the Research TopicQuantifying Carbon Removal by Negative Emissions TechnologiesView all 11 articles
All pathways which limit global temperature rise to <2°C above pre-industrial temperatures now require carbon dioxide removal (CDR) in addition to rapid greenhouse gas emissions reductions. Novel and durable CDR strategies need to rapidly scale over the next few decades in order to reach Paris Agreement Targets. Terrestrial enhanced weathering (EW) involves the acceleration of natural weathering processes via the deployment of crushed rock feedstocks, typically Ca- and Mg-rich silicates, in soils. While models predict this has the potential to remove multiple gigatonnes of CO2 annually, as an open-system pathway, the measurement (monitoring), reporting, and verification (MRV) of carbon removal and storage is challenging. Here we provide a review of the current literature showing the state-of-play of different methods for monitoring EW. We focus on geochemical characterization of weathering processes at the weathering site itself, acknowledging that the final storage of carbon is largely in the oceans, with potential losses occurring during transfer. There are two main approaches for measuring EW, one focused on solid phase measurements, including exchangeable phases, and the other on the aqueous phase. Additionally, gas phase measurements have been employed to understand CO2 fluxes, but can be dominated by short-term organic carbon cycling. The approaches we review are grounded in established literature from the natural environment, but implementing these approaches for EW CDR quantification has strengths and limitations. The complexity inherent in open-system CDR pathways is navigable through surplus measurement strategies and well-designed experiments, which we highlight are critical in the early stage of the EW CDR industry.
All emissions pathways which limit global average temperatures to <2°C now necessitate gigatonne scale removal of atmospheric carbon dioxide (CO2) in addition to emissions reductions (IPCC, 2022; Smith et al., 2023). In order to meet national and international targets for climate change mitigation, the carbon dioxide removal (CDR) industry has to scale rapidly, delivering high-quality, durable and additional CDR (Campbell et al., 2022; Maesano et al., 2022; Smith et al., 2023). Projected needs for durable CDR range anywhere from 0.06 GtCO2/yr. to over 1 GtCO2/yr. by 2030, alongside less durable forestation-based methods and land management practices, which is a 30–540 fold increase from 2020 levels of 0.002 GtCO2 (Smith et al., 2023). Accelerating CDR deployment within such a short time frame given the financing, policy, technical and engineering challenges associated with many durable CDR methods highlights the importance of rapidly validating those approaches that are available to scale near-tem and that can readily integrate within existing activity. Much of this validation rests on scientific verification of the net atmospheric CO2 removed, as well as the assessment of associated environmental impacts. This review addresses the challenge of scientific verification by detailing the current state-of-play of the multiple options for quantifying and monitoring carbon fluxes associated with terrestrial enhanced weathering (EW).
Enhanced weathering is considered a novel CDR technique which aims to speed up chemical weathering of rocks, increasing the flux of dissolved inorganic carbon (DIC, primarily bicarbonate [HCO3−]) to the oceans (Seifritz, 1990; Schuiling and Krijgsman, 2006; Köhler et al., 2010; Hartmann et al., 2013; Taylor et al., 2016). The premise of this is that CO2 dissolved in rain and soil waters as carbonic acid is neutralized by alkaline minerals, generating stable bicarbonate ions in drainage waters which, when transported to the oceans, stored in groundwater reservoirs or precipitated as carbonate minerals in soils become a durable carbon store (Figure 1).
Figure 1. Illustration of conceptual stages for terrestrial EW CDR. The open system processes can be broadly separated into three stages; (i) capture, (ii) transport and (iii) storage. Note that there is spatial and temporal overlap between the stages. The different stages give a modularity to CDR measurement where we focus here on soil processes, with methods to estimate the primary capture stage of bicarbonate formation.
The global riverine flux of DIC from continental weathering has regulated atmospheric CO2 on Earth over million year timescales, and currently removes ~1 Gt of CO2 per year (Walker et al., 1981; Berner and Berner, 2012). In order to speed up this natural process, crushed reactive rocks (e.g., basalt), minerals (e.g., olivine and wollastonite) or other alkaline materials (e.g., slag, cement kiln dust, or returned concrete) can be applied in agricultural settings (Renforth et al., 2015; Taylor et al., 2016; Renforth, 2019; Amann et al., 2020; Haque et al., 2020; Kelland et al., 2020). Based on generalist model predictions, EW has a global CDR potential in the range of 0.5–4 Gt CO2 per year (Fuss et al., 2018; Beerling et al., 2020; IPCC, 2022), which can be optimized through the choice of feedstock and weathering environment (Beerling et al., 2020; Cipolla et al., 2021, 2022; Baek et al., 2023; Deng et al., 2023; Haque et al., 2023; Jerden et al., 2024). Such a magnitude of CDR can meaningfully contribute to national and international CDR targets (Taylor et al., 2016; Beerling et al., 2020; Kantzas et al., 2022; Smith et al., 2023) with water and energy requirements lower than most industrial removal technologies (Lefebvre et al., 2019; Eufrasio et al., 2022), no required change in land use, all while providing important benefits for crops and communities (Manning and Theodoro, 2020; Swoboda et al., 2022). Moreover, the infrastructure required for deployment already exists.
Silicate rock powders have been researched or used commercially for decades at low application rates as a niche sustainable land management strategy (Goldschmidt and M., 1922; Ilchenko, 1955; Chittenden et al., 1967; Sanz Scovino and Rowell, 1988; Bolland and Baker, 2000; Leonardos et al., 2000; Van Straaten, 2007; Basak et al., 2017; Canadian Wollastonite, 2017; Manning and Theodoro, 2020; Swoboda et al., 2022). Despite the interest, silicate rock powders are not used widely due to financial, social, logistical and technological barriers, which carbon finance can help overcome. Major benefits include raising soil pH, offering a replacement solution for AgLime use which may release CO2 when used in combination with nitrate based fertilizers (West and McBride, 2005), and supplying a range of micro- and macro-nutrients (Swoboda et al., 2022). Agronomic benefits have been observed in tropical, subtropical and temperate soils under both experimental and field conditions (Manning and Theodoro, 2020; Conceição et al., 2022; Swoboda et al., 2022; Beerling et al., 2023; Guo et al., 2023; De Medeiros et al., 2024; Skov et al., 2024; Wang et al., 2024). There are currently no national or international standardized recommendations for the use of silicate rock powders with agriculture, such as guidance on application rates and specific rock-soil combinations to maximize benefits.
As an open-system CDR pathway, EW faces challenges for directly monitoring weathering rates, and ultimately calculating net CDR. The complexity of this open system presents a significant barrier to scaling the technology (Santos et al., 2023). Under the definition of CDR as a process involving both the capture and durable storage of atmospheric carbon dioxide (Smith et al., 2023), the capture phase refers to the formation of bicarbonate at the weathering site with storage occurring primarily as dissolved bicarbonate in the ocean and long lived aquifers (Campbell et al., 2022; Smith et al., 2023) or, less commonly, through the precipitation of carbonate minerals in the soil with certain feedstocks and soil systems (Renforth et al., 2009; Haque et al., 2020). Ocean storage is considered permanent (~105 years) by all current carbon management definitions. Pedogenic carbonate in soils has previously been considered as a durable store of carbon (~104 years, Zamanian et al., 2016; Andrews and Taylor, 2019). However, these estimates are based on arid natural environments, and may not be representative of carbonate formation during EW (Zamanian et al., 2016). Further research is required regarding carbonate storage due to the potential for remobilization (Berner et al., 1983; Zamanian et al., 2016; Renforth and Henderson, 2017; Kanzaki et al., 2023). Moreover, the storage pathways differ in their CDR efficiency with up to a 50% loss of captured CO2 occurring during carbonate precipitation (see Campbell et al., 2022).
There are currently numerous approaches to estimating carbon capture at a weathering site, drawn from established soil science, agronomy, geochemistry and geology literature. These approaches can be broadly separated into solid, water and gas based categories. In addition, the use of soil exchangeable cation concentrations presents a hybrid between solid and water based categories, but would be conducted on the samples taken for solid phase analysis. Each category contains multiple geochemical measurement strategies which each have scientific or operational benefits and limitations, with no standard method prevailing in the field (Table 1). Given the nascent phase of EW research and deployment, early crediting methodologies from standard setting bodies allow for a diversity of approaches for CDR quantification (Carbon Standards International, 2022; Puro.earth, 2022) although an example of stronger requirements can be seen more recently (Isometric; Sutherland et al., 2024) focused on collecting surplus data and reflecting the approaches reviewed here. Provided that data are shared transparently, this emerging methodological framework facilitates operational research and field advancement in the private sector at a scale and urgency that cannot be achieved in academia alone, while maintaining social and environmental safeguards.
Transport of aqueous bicarbonate through the lithosphere-hydrosphere system occurs post-capture and pre-storage (Figure 1), often associated with ‘downstream’ CO2 losses that decrease CDR efficiency. In order to make a claim for a CDR credit (Puro.earth, 2022; Sutherland et al., 2024), downstream CO2 losses should also be quantified as, by the definition above, CDR typically refers to the CO2 that has been both captured and stored. Downstream losses are more difficult to monitor directly compared to capture processes. As such, models and publicly available riverine geochemistry datasets may show promise for addressing open system losses (Calabrese et al., 2022; Kanzaki et al., 2022; Knapp and Tipper, 2022; Zhang et al., 2022; Bertagni and Porporato, 2022; Harrington et al., 2023; Kanzaki et al., 2023; Zhang et al., 2024) but industry guidance on how to handle them in CDR claims is under refinement. Upstream losses (i.e., operational or embodied emissions) must also be quantified by life cycle analysis (Nunes et al., 2014; Lefebvre et al., 2019; Zhang et al., 2023) and included in net-CDR calculations (Carbon Standards International, 2022; Puro.earth, 2022; Sutherland et al., 2024). We further stress that full assessment of additionality and robust environmental and social safeguarding policies are essential components of responsible EW CDR projects. Many of these safeguards can be incorporated into measurement approaches for quantifying weathering rates and carbon capture.
Carbon removal claims for EW are made based on the enhancement of inorganic carbon cycling, and would typically not include any increased carbon removal by organic carbon pathways, due to the major differences in permanence and durability of the different fractions (Brander et al., 2021). That being said, EW feedstocks may have complex effects on different soil organic carbon fractions (Ramos et al., 2022, 2024), as addition of rock powder may stimulate mineralization of the labile organic carbon pool, while potentially increasing the long-term stable mineral-associated organic matter (Slessarev et al., 2022; Buss et al., 2024). As such, while we focus on inorganic approaches here, it is important to consider wholescale carbon budgeting approaches to assess the impact of EW on existing carbon management practices, such as soil organic carbon (SOC) storage (Kantola et al., 2023) and wider associated ecosystem carbon removal (Goll et al., 2021).
This review provides an orientation point for EW students, researchers, operators, regulators and wider stakeholders, detailing the basis of current measurement approaches. We outline the current state-of-play and summarize the strengths and limitations of different measurements for EW, primarily focusing on quantification of weathering rates and captured CO2. We consider downstream CO2 losses to be beyond the scope of this paper, but highlight that this is a topic of ongoing research. This review sits alongside a broad review of experimental design strategies (Almaraz et al., 2022), a recent set of thorough analytical recommendations for geochemical CDR (Campbell et al., 2023), and a toolkit developed by CarbonPlan (Holzer et al., 2023b) that outlines system wide considerations for EW.
In its simplest chemical formulation, EW can be considered as an acid–base neutralization reaction. Carbonic acid (containing atmospheric or biogenic CO2) is neutralized by the dissolution of an alkaline material or mineral, such as a silicate mineral, producing dissolved bicarbonate, dissolved silica, and major dissolved cations (Ca2+, Mg2+, Na+, K+). This reaction converts CO2 dissolved in water to stable bicarbonate ions which are primarily durably stored as dissolved bicarbonate in the ocean or, in a minority of cases, as carbonate minerals. The dissolution of most silicate minerals is incongruent, meaning that mobile cations (Ca2+, Mg2+, Na+, K+) are removed via drainage waters, and immobile elements (e.g., Al, Ti) either remain in the soil in recalcitrant minerals or are incorporated into secondary minerals such as clays. These reactions are illustrated in Equations 1 and 2, showing the dissolution of two idealized feldspar mineral endmembers (anorthite and albite) that are common in silicate rock feedstocks. In this instance, for anorthite, Ca2+ and bicarbonate are dissolved in water, whereas for albite, Na+, bicarbonate and silicon are dissolved. In both cases Al and some Si remain in the soil as clay minerals.
A generalized silicate weathering pathway (Figure 2) illustrates that there are two primary geochemical targets for tracking weathering processes: 1) the constituents of minerals being weathered (e.g., Ca2+, Mg2+, Na+, K+, Si), and 2) the carbon consumed or transformed by weathering reactions (CO2, or HCO3−) (Almaraz et al., 2022; Amann and Hartmann, 2022). Of these, the former approach is a proxy for mineral dissolution, allowing a calculation of weathering rates and CDR potential, and by extension – with significant assumptions – carbon export. The latter provides a more direct measure of carbon export, but also requires assumptions on mobility and losses.
Figure 2. Summary of the weathering process and potential complicating processes that result in downstream CO2 loss. The primary aim of measurement techniques are to trace the loss or gain of weathering products in different phases; solid, liquid or gas. This can be relatively straightforward in cases where carbonic acid is the dominant weathering acid, but in cases where fertilizers produce nitric acid, weathering products may be released without concomitant CO2 removal. Strong acids also drive carbonate dissolution that is a CO2 source. CO2 can also be lost during transport and at the final storage location, however a detailed review of these processes is beyond the scope of this paper.
An estimate of the maximum CDR potential by EW can be determined using the modified Steinour equation (Steinour, 1959; Renforth, 2012; Renforth, 2019). This is achieved by calculating the amount of alkali and alkaline cations (wt% of CaO, MgO, Na2O, K2O) contained in a rock powder feedstock, and then using charge balance to estimate the maximum potential for CO2 removal (Epot):
Where MW = molecular weight (g mol−1), α, β ε, θ are coefficients which account for redox speciation as a function of pH (1 for pH between 3 and 10; Renforth, 2019) and = the carbon drawdown per (double-charged) cation flux to the ocean ( =1.5 to 1.7 for typical earth surface environmental conditions; Renforth, 2012, 2019). Hypothetically, if the primary carbon storage pathway is through carbonate mineral formation (carbon mineralization), rather than bicarbonate, then = 1, reflecting the loss of CDR potential through re-release of CO2 upon carbonate precipitation. It is important to notice that an =1.5 to 1.7 already accounts for some downstream losses of carbon (Renforth, 2012, 2019).
Most applications of Epot only focus on Ca and Mg, as the contribution of K and Na to the Epot of proposed feedstocks for EW is typically ~25% of that of Ca and Mg (Lewis et al., 2021). Moreover, K (and to a lesser extent Na) are common constituents of chemical fertilizers which may complicate cation budgeting based on field samples. That said, all four cations are considered in the recent crediting methodology of Sutherland et al. (2024). The Steinour equation can also be modified to account for sulfate and phosphate in the rock powder (Renforth, 2019), sourced from salts (e.g., gypsum) pyrite or apatite. In these cases, rock weathering results in cations that are charge balanced by phosphate and sulfate, rather than bicarbonate.
Understanding maximum Epot is important for evaluating various rock powders in order to maximize CDR gains, for initial life cycle assessments (LCA) or first-order global scale models (e.g., Renforth, 2012; Beerling et al., 2020; Zhang et al., 2022). Even though Epot can be calculated by the cation concentration in the rock, it does not account for the mineralogical composition and reactivity of the rock powders, where some minerals are more easily dissolved than others, nor does it account for temporal aspects of the reactions. Thus, it is a single, time integrated estimate assuming fully congruent weathering of the feedstock. Its use is also limited by knowledge of the internal variability of the feedstock’s chemical composition. Actual CDR from in situ weathering will always be smaller than Epot due to the complexity of mineral weathering and downstream CO2 losses.
The loss of reactants from mineral phases can be used to calculate weathering rates, which may be converted to an initial carbon capture estimate using the Epot of a feedstock. Total soil + rock powder mobile cation concentrations (e.g., [Ca] and [Mg]) are expected to decrease as mineral dissolution proceeds and mobile cations are lost to solution (Figure 3). The main advantage of analyzing solid phases in this manner is that it creates a time-integrated signal, whereas other approaches, discussed below, are heavily influenced by climatological and pedogenic parameters leading to more temporally variable signals. Additionally, this approach may be integrated with current agronomic practices as soil samples may be routinely taken by farmers to assess other parameters such as soil pH and cation exchange capacity (CEC). Furthermore, it is possible to constrain weathering signals on a field-by-field basis.
Figure 3. Schematic view of approaches to monitoring weathering activity and idealized examples of total solid and exchangeable results. Different measurement pathways illustrate different components of the weathering mass balance, which must be comprehensively quantified to estimate weathering rates and calculate CDR. Data serve an illustrative purpose only and are not based on measurements.
Examining total solids traces the loss of cations in the soil and rock powder to determine weathering rates, but it does not track their export, so converting a rate of cation loss to CDR equivalents will be a maximum estimate of carbon actually exported into the river-ocean system (i.e., it tracks realized CDR potential, not eventual CDR after loss processes). Calculating carbon export requires further assumptions (Sutherland et al., 2024). Additionally, it does not uniquely identify the action of carbonic acid over other potential acids in the soil, which would cause an overestimate of carbon capture at the weathering site.
A key challenge faced by soil-based cation mass balance is that the contribution of applied rock powder to cation concentrations in the soil and rock powder mixture is not certain, given inhomogeneous application and mixing, sampling constraints, and potential physical erosion or remobilization. This means that cation accounting based on a generalized rock powder application rate for the entire field can result in an over- or under-estimation of the initial cation concentration, even when samples are averaged across a field.
The problem of calculating the initial cation contribution from applied rock powder can be addressed by measuring the concentration of cations in the soil relative to an immobile trace element (such as Ti, Al, Th, Zr) (Reershemius et al., 2023). These principles also underlie the patent of Wolf et al. (2023) who, focusing on the rare earth elements (REE) and other immobile tracers, suggest that combinations of immobile tracers can be used to calculate rock powder application rates (see also Kantola et al., 2023; Reershemius and Suhrhoff, 2024; Wolf et al., 2024 for a discussion of limitations). The premise of using immobile tracers in this manner is grounded in traditional sediment geochemistry in that the loss or gain of geochemical components must be expressed relative to a conservative or immobile element that is not involved in the primary reaction (Brimhall and Dietrich, 1987). This creates a normalization procedure that, in this case, accounts for the variable mixtures of rock powder and soil at the sample point, or any erosive loss of rock powders (Figure 4). In effect, by comparing the concentrations of mobile cations to that of an immobile trace element, a soil + rock powder sample can be pinned along a compositional mixing line between the soil baseline and the rock powder, as shown in Figure 4. Actual variability in rock powder application and weathering activity must then be captured by ensuring a high number of samples across the entire site covering representative environmental gradients.
Figure 4. Illustration of cation mass balance rooted in the concentration of an immobile trace element, Ti, modified from Reershemius et al. (2023). Start and end values serve illustration purposes only and have not yet been measured. Here we show Mg as an example of a mobile cation, j. Panel (A) demonstrates the mixing line created by the baseline soil plus rock powder, where any combination of soil + rock powder would fall on this mixing line. (B) illustrates how a measure of immobile Ti at the end of weathering ([Ti]end) can be used to calculate [Mg]add. (C) illustrates the loss of [Mg] during weathering, gained by the measurement of [Mg]end, and is expressed as the change in [Mg] (Δ[Mg]). (D) demonstrates the cases where application amounts vary and weathering rates remain the same, meaning the fraction of [Mg] lost relative to Mg]add (Fd), remains constant (dashed lines). Three examples are shown for no weathering (Fd = 0), majority weathering (Fd = 0.7) and complete weathering (Fd = 1). Erosive loss of powder would be effectively the same as variable powder addition (D), illustrated by the sample moving toward [Mg]soil, [Ti]soil with a slope of approximately constant Fd.
Mass balance approaches using any immobile tracer are predicated on knowing precisely the baseline soil immobile tracer (i) and cation (j) concentrations, rock powder i and j concentrations, and the concentrations of i and j in the mixture after weathering. Essentially, the immobile tracer is used to calculate the amount of cations added to the soil through rock powder spreading, and measurements of post weathering samples of soil + rock powder mixtures inform on the remaining cation amount. The difference in cation concentrations can then be expressed as a fraction of rock powder dissolved (Fd; Figure 4D) and related back to carbon capture through Equation 3.
This approach makes two major assumptions. Firstly, the immobile tracer must be demonstrably immobile in the chosen environment. Loss of some immobile tracer from the rock powder will result in a conservative estimate for weathering, but if reprecipitated elsewhere in the soil column may produce a mixed signal for rock powder addition in some samples and therefore distort whole-field weathering estimates. Secondly, cations must be considered fully mobile and generally lost to solution, and will therefore not be present in any solid soil + rock powder sample after weathering. This second assumption, however, is not always applicable as cations can be (sometimes temporarily) incorporated into exchangeable phases or secondary minerals (Amann et al., 2020; Fuhr et al., 2022; Wood et al., 2023). Standard operating procedures for dealing with such exchangeable phases require further development, with some users including pre-leaching treatments to remove them. Sieving of samples to remove larger residual rock fragments and organic debris is usually practiced.
While operationally scalable, one major limitation of using the solid phases for these estimates is the low signal to noise ratios due to high soil cation concentrations. Hence it can be difficult to resolve small differences between the soil–rock mixtures, before and after weathering. To counter this problem, isotope dilution inductively-coupled plasma mass spectrometry (ID-ICP-MS) may be used to improve measurement precision and be better able to resolve small changes in cation concentrations (Reershemius et al., 2023). Infield heterogeneity can also be the limiting factor rather than analytical precision. There may be cases where such high precision is not required, such as where higher rock powder application rates are used, in higher intensity weathering environments, or, generally, when soil and feedstock compositions are very different (Suhrhoff et al., 2024).
Given the requirement for a precise baseline concentration to be assumed, heterogeneity in soil trace and major element composition represents an appreciable barrier to implementing solid-phase mass balance approaches to tracking weathering at the field scale. This may be at least partly addressed through implementing soil sampling protocols that increase the replicability of measurements (e.g., pooling, geospatial referencing). Statistical treatments of whole-field i and j concentrations of baseline and post-application, post-weathering samples will likely be necessary to robustly quantify field-scale compositional changes as a result of EW feedstock application and subsequent dissolution. A key challenge for practitioners will be to assess the minimum sampling requirements for resolvability of these signals in a range of settings, which is currently under-explored in the literature and a recommended priority for ongoing research.
Lastly, the choice of immobile tracer i is an important consideration. The concentration of immobile tracer in the rock powder must be significantly higher than in the soil, because: (1) this reduces the requisite analytical precision for measuring a signal for rock powder addition above background noise; and (2) otherwise, at low Δirock powder - soil, the dissolution of feedstock contributes to a stronger concentration effect of i, resulting in a pronounced overestimate of rock powder addition (Reershemius and Suhrhoff, 2024). This effect is true of any element i, but can be corrected easily for those elements i where concentration in rock powder is several times greater than in soil (Reershemius et al., 2023). Low Δirock powder - soil of Th, Nb, Y, and REEs precludes these immobile tracers from being used for most rock powder-soil combinations; this is also true of Ti and Al for most ultramafic rocks, where Cr, Ni and Fe might be the only realistic tracers (Suhrhoff et al., 2024). Some proposed rock powders for EW, such as wollastonite, do not contain any immobile trace elements in sufficient abundance to be used for this purpose. A tool for assessing the applicability of different immobile tracers is now available to help guide operational decision making (Suhrhoff et al., 2024).
An additional consideration for an immobile tracer i that must be tested thoroughly is the extent of mobility during weathering processes in a range of settings; especially those elements, such as Cr, Ni, Fe, that can also have limited mobility depending on properties such as soil redox state, availability of organic colloids, and pH (Alloway, 2013). While immobility might still be maintained for these elements, heterogeneity in their concentrations vertically and laterally due to diagenetic processes, may lead to biased results.
Kantola et al. (2023) suggest using multiple immobile trace elements in a single calculation to increase the signal strength from rock powder addition in solid phase samples. This involves fitting a regression to the observed concentration increase of multiple immobile trace elements and using the slope of this line to calculate the rock powder application rate (Kantola et al., 2023). However, when using elements that are not particularly enriched in the feedstock, this approach must account for the fact that the concentration increase of multiple immobile trace elements following rock powder addition to a soil is also a function of the relative concentration differences between rock powder and soil for each individual element, and will be affected by feedstock dissolution (Reershemius and Suhrhoff, 2024). Moreover, analytical uncertainties must be appropriately propagated to ensure accuracy and significance of interpretations based on regression models. A different approach to increasing the strength of a signal for rock powder addition may instead be to pin to a ratio of immobile tracers, where the denominator is a tracer that is less concentrated in the rock powder than in the soil (e.g., Th; Suhrhoff et al., 2024).
It is clear that more work is needed to develop methods to refine soil-based mass balance for estimating in-field weathering rates of rock powder: primarily to improve sampling and analytical practices while limiting their cost, to resolve spatial variability in estimates of field-scale weathering rates, and to take into account processes such as fertilizer addition and background weathering of soil constituents that may interfere with signals. Moreover, assumptions on tracer (im)mobility require testing in more local environments before such methods can be robustly used for widespread CDR crediting purposes.
With some reactive feedstocks (e.g., slag and wollastonite) or under certain climatic conditions (low rainfall, high soil pH) carbonate precipitation may be favored over aqueous bicarbonate creation, termed carbonation or CO2 mineralization (Campbell et al., 2022). The CDR efficiency of the mineralization pathway is up to half that of EW with bicarbonate formation due to CO2 release during carbonation (Figure 2). In these cases, the formation of carbonate can be monitored as total inorganic carbon (TIC). At high enough concentrations, TIC is relatively simple to quantify using calcimetry or thermogravimetric analysis and, if enough carbonate is generated, can be combined with techniques such as XRD analysis to characterize the carbonate mineralogy (Dudhaiya et al., 2019; Haque et al., 2019, 2020, 2023; Khalidy et al., 2021). Additionally, the source of carbon and calcium can be quantified using isotopic tracers (see Section 2.5; radiogenic Sr. isotopes to confirm the source of Ca, and stable carbon and oxygen isotopes to confirm the source of carbon) to ensure the accumulation of TIC came from mineralization of silicate minerals (Knapp et al., 2023).
Dietzen and Rosing (2023) suggest monitoring cation accumulation in exchangeable phases as a pathway to carbon capture estimates, which may potentially be easier to resolve compared to total solids approaches. In principle, this approach is based on the opposite assumption compared to solid phase approaches: namely that all released mobile cations are not leaving the top soil but are retained on exchangeable sites for the period in question. Exchangeable phases are geochemically reactive components (clays, oxides and organic matter) within a soil that have the capacity to weakly bind cations to their negatively charged surfaces. These reactions are typically considered to be rapid, operating on the order of seconds to days and may include sorption and surface precipitation (Brady and Weil, 2008). Often the exchangeable phases include elements bound to clays and oxides minerals, but not the elements incorporated into these minerals on longer timescales due to diagenetic stabilization. Elements weakly bound to organic matter can also be included, but not those directly incorporated into more recalcitrant organic substances (Brady and Weil, 2008). Note that some literature sources refer to ‘bioavailable’, ‘reactive’ or ‘labile’ pools to distinguish phases that are separate to non-reactive, inert mineral phases (Bhatia et al., 2013; Larkin et al., 2021). There will be overlap between such phases and the exchangeable pool, however, in reality, all of these terminologies are operationally defined based on leaching protocols and are rarely exclusive in their extraction (Brown, 1943; Tessier et al., 1979; Groenenberg et al., 2017; Vienne et al., 2022; Vink et al., 2022; Dietzen and Rosing, 2023).
The precision of this approach relies on correctly timing soil sampling, and any loss of cations from top soils to ground water will not be detected. Given that water (and hence the flushing of cations from top soils) is a necessary condition for weathering to occur, the validity of these assumptions and the temporal decoupling of weathering and cation transport can be questioned. Dietzen and Rosing (2023) suggest that baseline monitoring of exchangeable and fluid phases could be used to identify time periods where the loss of cations to fluids is minimal, allowing measurements from the exchangeable fraction from particular seasonal points to be used for CDR estimates. But, there will always be a risk of underestimating cation losses.
In some EW experiments, there is no evidence for significant cation removal by exchangeable phases (Renforth et al., 2015), while others demonstrate quantitatively important interactions between cations and secondary minerals, such as clays (Pogge von Strandmann et al., 2019, 2021; Dietzen and Rosing, 2023), even up to a 100 x the concentrations seen in leachate data (Kelland et al., 2020). Given the potential control of exchangeable pools onto the weathering mass balance, they may be a limiting factor for identifying weathering products from solution based methods, and a suggested contributor for the lack of observed weathering signals in outflow waters of field trials, particularly in low pH soils (Larkin et al., 2022). Such behavior will depend on the base saturation state of the exchange sites as well as soil permeability, pH, net cation exchange capacity (CEC) and surplus rainfall. If there is a low CEC or high pH, base saturated soil, it will be more likely that cations are transported out of the soil.
With a similar logic to examining exchangeable phases, accumulation of weathering products in aqueous phases can also produce a wealth of information on cations and anions, including carbon species (Renforth et al., 2015; Shao et al., 2015; Vienne et al., 2022; Te Pas et al., 2023). In experimental setups, such as columns, pots and mesocosms, the aqueous phase is often collected as a ‘leachate’; that is a fluid that has penetrated the soil, mobilized weathering products and exited the experimental setup into a collector. Infield sampling may be done via the sampling of pore waters through Macro Rhizon syringes, via soil lysimeters (e.g., suction cup or tension lysimeters) or even shallow wells at specific depths in the soil column (see Almaraz et al., 2022). Artificial cation-exchange resins in the soil have also been suggested as a method to sample waters by the patent of Wolf et al. (2023) but to date, there is no published research validating this approach. Anion exchange resins have also been used to measure nitrate and ammonia (Kantola et al., 2023).
Catchment or watershed scale monitoring on rivers or channels have been applied to larger scales of deployment, providing integrated total system fluxes (Andrews and Taylor, 2019; Larkin et al., 2022; Knapp et al., 2023). Indeed, monitoring of dissolved loads at the riverine catchment scale is the primary method by which current global CO2 removal via natural weathering is measured (Gaillardet et al., 1999b; Jacobson and Blum, 2003; Viers et al., 2007; Hartmann et al., 2014; Moon et al., 2014; Hilton and West, 2020) hinting at the scalability of this approach. It may also be possible to monitor drainage waters via carefully designed artificial drainage systems (e.g., tile drains) to capture water exiting a site (Andrews and Taylor, 2019).
We note that there are differences in chemistry between natural and agricultural catchments which may affect the resolvability of EW. Typically, agricultural catchments may have higher nitrate, sulfate and alkalinity than natural catchments (Hamilton et al., 2007; Perrin et al., 2008; Stets et al., 2014). Dissolved organic carbon (DOC) concentrations in waters draining croplands may be lower than in other natural systems (such as permafrost, tundra or boreal forests, (Liu and Wang, 2022), but may also be transiently raised by high organic amendments (such as manure). Agriculture may also result in salinization and increasing Cl− concentrations, due to fertilizer amendments such as KCl (Meybeck, 2003). Liming with carbonates (limestone, dolomite) leads to increasing HCO3− concentrations if weathering occurs via the carbonic acid weathering pathway (Hamilton et al., 2007; Raymond et al., 2008). Literature estimates ~15% of excess acidity from nitrogen fertilizer application may react with ag-lime (Knapp and Tipper, 2022), meaning that not all weathering of agricultural lime will result in an increase in HCO3.
In the field, the location, depth and timing of solution sampling will have implications for how measurements will be related back to carbon capture estimates. For pore-water samples, data will likely primarily reflect reactions at the weathering site including primary dissolution and secondary uptake of weathering products. Water moving through a soil may be subject to additional geochemical processing, such as adhesion to cation exchange sites (Pogge von Strandmann et al., 2019), that could modify the EW signal. By contrast, measurements made at the catchment scale can provide total system fluxes, averaging out small scale heterogeneities.
For all solution-based analysis in the field environment, challenges exist in the temporal signature of aqueous phases that can vary seasonally, for example with rainfall amount and intensity that affect dissolution kinetics, dilution, water flow paths through the soil, transit times and total cation export (Calabrese et al., 2017; Wen et al., 2022), requiring detailed temporal consideration on sampling strategies (Dietzen and Rosing, 2023) and how measurements are scaled to annual CDR estimates. Additionally, particularly at large scales, it might take years for a resolvable trend to emerge (Taylor et al., 2021) and so long-term monitoring, large-scale and carefully designed experiments might be required, in combination with scientific guidance on sampling intervals for robust CDR estimates.
Solution based analysis requires assessment of water flow in order to convert concentrations into a flux (the total amount of inorganic carbon exiting to catchment waters per year) and ultimately calculate the mass of carbon exiting the system. Typically there is a measurement resolution mismatch between how often a discharge measurement is taken or modeled (resolution of minutes to daily) and how often a concentration measurement is made (at least biweekly, sometimes monthly). Therefore, a load estimation method is required to calculate an annual flux (e.g., Moatar and Meybeck, 2005). Chosen load estimation methods require some assumptions to be made about concentration behavior in between sampling points. Measurement of discharge at the watershed scale may be more straightforward with the proper installation and calibration of a gaging station. At the smaller field scale, modeling or calculation of predicted flow based on measured climatic parameters (via an onsite weather station) and physical soil properties can be used to estimate discharge (Alley, 1984; McCabe and Markstrom, 2007).
The major dissolved cations in natural waters are Ca2+, Mg2+, Na+ and K+. Sodium (Na+) and potassium (K+) are highly mobile and hence are likely to remain in the aqueous phase, but are limited by their availability in CDR rock powders and have a lower CDR potential per mass due to forming singly charged cations. Calcium and Mg2+ are more abundant in CDR rock powders but are more likely to adhere to the exchangeable sites (Whitworth, 1998; Bergaya et al., 2006; Dietzen and Rosing, 2023), and Ca2+ will form pedogenic carbonates in some EW operations (Haque et al., 2020; Khalidy et al., 2021). Potassium (K+) and Ca2+ are more likely to be uptaken by plants, with potassium being a key nutrient. Numerous studies suggest that Mg2+ is the most suitable cation for estimating weathering rates in the exchangeable fraction and plays a larger role in potential CO2 uptake (Renforth et al., 2015; Pogge von Strandmann et al., 2021; Dietzen and Rosing, 2023). Moreover, given that Mg2+ is not as readily incorporated into carbonate minerals or uptaken by plants compared to Ca2+, it may be easier to trace in solutions.
Similarly, Si will be a major component of many EW feedstocks and its presence in solution could directly indicate weathering activity. It is more difficult, however, to relate Si concentrations back to carbon capture given the mineral partitioning of Si within the feedstock. For example, the presence of Si in non-alkaline silicate minerals (including amorphous phases) could supply Si without cations. Silicon can also be removed from solution to form secondary minerals (e.g., Kelland et al., 2020). It has furthermore been suggested that silica saturation may limit feedstock dissolution and the CDR potential of EW (Köhler et al., 2010; Hartmann et al., 2013; Harrington et al., 2023), however the role of silica saturation in limiting enhanced weathering is debated (Schuiling et al., 2011).
In natural waters at neutral range pH, the dominant dissolved anions are sulfate, nitrate, chloride and bicarbonate. Minor contributions from phosphate and borate may be present in some waters. The sum of major anions (Cl−, SO42−, NO3− HCO3−) minus the sum of major cations (Ca2+, Mg2+, Na+ and K+) in equivalents is equal to zero due to the law of electroneutrality. The normalized inorganic charge balance (NCIB = (Σ + − Σ−)/(Σ+ + Σ−) in %, where Σ + is the sum of cations and Σ − is the sum of anions, in equivalents) or charge balance error (CBE) is a key indicator of water data quality, with values typically greater than ±5% for high quality measurements, and should always be within ±10% (Fritz, 1994).
Measurement of anions are required to understand not only the quality of the measurement but also to understand the acidity source (carbonic acid vs. strong acids; see section 3.2), and to correct cation measurements for rainwater inputs (cyclic salts) and dissolution of evaporites (such as gypsum that is commonly used as an agricultural amendment). Bicarbonate concentrations in neutral range pH waters may be calculated using major anion and cation measurements via charge balance, assuming that all remaining positive charge after subtracting Cl−, SO42−, NO3− (in equivalents) is charge balanced by HCO3− (e.g., Galy and France-Lanord, 1999).
Dissolved inorganic carbon (DIC) is a term used to encompass all inorganic carbon species in a liquid, including CO2, carbonate, bicarbonate and carbonic acid. Thus, it can give the most direct measurement of bicarbonate release from mineral weathering (Amann et al., 2020; Almaraz et al., 2022). DIC measurements can be performed with several types of equipment that require minimal technical skills (e.g., coulometry). For soil pore waters, the main challenge is that the partial pressure of CO2 (pCO2) is much higher than atmospheric pCO2 due to respiration. Thus, samples need to be hermetically saved (and with minimum headspace), from the moment they are collected in the field until they are analyzed, to avoid degassing of molecular CO2 (Reiman and Xu, 2019). Special care needs to be taken for samples at lower pH values in which the concentration of dissolved molecular CO2 is higher. In other cases, samples can be left open to the air to equilibrate with atmospheric CO2, after which DIC can be measured a second time, simulating equilibration which would have happened when the soil pore-waters enter a watershed (Figure 5).
Figure 5. Bjerrum plot (created with PHREEQC, v.3, with input values, DIC = 1 mol/kg, t = 25°C) showing the concentrations of carbonate species in solution, and total alkalinity (TA). The typical pH range for natural waters is between 6.3 and 10.3, where the dominant species of dissolved inorganic carbon is HCO3−.
In neutral pH waters, DIC can be approximated by total alkalinity (TA) measurements. This is only true, however, if the only proton (H+) receptors (the bases) are largely from hydroxide (OH−), bicarbonate (HCO3−) and carbonate (CO32−), and pH is between 6.3 and 10.3 (see Bjerrum plot, Figure 5). More strictly, TA refers to the milliequivalents (mEq) of H+ used while titrating a water sample with an acid of known concentration, usually via methods such as Gran Titration (Gran, 1952; Stumm and Morgan, 1996; Wolf-Gladrow et al., 2007). Thus, the measured TA is an approximation for DIC, which in most natural freshwaters is bicarbonate, and hence can be used to estimate CDR. Any two of alkalinity, pH, pCO2 and DIC alongside temperature can be used to calculate bicarbonate concentrations in waters. These calculations can be performed in PHREEQC (Parkhurst, 1995).
The advantage of TA is that it is a simple measurement to make with no specialist equipment needed, and is routinely measured during watershed monitoring by, for example, governmental bodies (Hartmann et al., 2014; USGS, 2019). Total alkalinity is a conventional parameter used to calculate the bicarbonate concentration of natural freshwaters at circum-neutral pH (Andrews et al., 2016; Amann and Hartmann, 2022; Knapp et al., 2023; Holzer et al., 2023a). Caution should be maintained, however, as TA can be affected by the presence of other accepting bases in addition to DIC. For example, in waters with high dissolved organic carbon (DOC), including some agricultural systems with organic fertilizers, total alkalinity might be a poor approximation for bicarbonate concentrations (Wolf-Gladrow et al., 2007; Kerr et al., 2021).
Electrical conductivity (EC) reflects the total ion concentration of a solution and hence can proxy the accumulation of weathering products, offering a potentially more scalable solution to CDR monitoring. The benefit is that EC can potentially be monitored in real time using sensors, while TA requires ex-situ lab analysis. Amman & Hartmann (2022) identified consistent correlations between TA and EC, thought to reflect the covariation of TA and cation concentrations. Soil porewater conductivity and volumetric water content may furthermore be used to constrain carbonate alkalinity in soils with low CEC (Rieder et al., 2024). This potentially means EC could be used in specific situations, coupled with pH and CEC measurements, where assumptions about TA and DIC speciation can be made and bicarbonate concentrations can be calculated successfully using speciation modeling software such as PHREEQC (Parkhurst, 1995). Robust and representative calibration datasets should be used to validate and monitor the use of EC in field environments as the relationship of EC to TA will vary with different ionic compositions.
Measuring isotope compositions is more expensive than most of the MRV approaches introduced thus far, and requires more specialist infrastructure, thus they are less likely to be an integral part of routine MRV approaches. However, isotope-based approaches can yield additional important information that are complementary to other methods and provide a fuller picture of the EW process. In particular, they can play a fundamental role in determining some of the underlying processes and in calibrating reactive transport models.
The approaches in the previous sections all have in common that they assess the absolute concentrations of certain elements or ions. While it is essential to assess elemental reservoirs and fluxes, isotope ratios can yield additional information such as the nature of the sources and sinks of elemental fluxes, or processes that modulate these fluxes (Faure and Mensing, 2005). For example, stable isotope systems are often used to infer mixing of different element sources, as different sources can have different isotopic signatures. They are also used to infer processes such as the formation of secondary phases or cation sorption onto exchangeable sites because some isotopes are easier to exchange, for example as a result of higher or lower mass (i.e., they fractionate them).
Radiogenic isotope systems are typically used to trace sources rather than processes, as they are routinely corrected for instrumental mass fractionation during measurement (e.g., radiogenic Nd and Sr. isotopes). This correction means that any natural mass fractionation is not observed, and as a result, sample composition typically depends on the isotope composition of the source mineralogical phases.
Stable carbon and oxygen isotopes (δ13C and δ18O) are relatively simple to measure and can inform on the source of C in soil fluids and carbonates. If the δ13C of each carbon source is distinct and known, then measured δ13C of water outflows could inform on the relative proportion of each source (Manning et al., 2013). For example, CO2 in soil waters is typically derived from organic respiration, giving it a low δ13C value −27 to −12.5‰; (Vogel, 1993), whereas carbonate rocks and carbonate amendments (‘AgLime’), derived from marine carbonate deposits, have higher, and distinct, δ13C values. Potentially δ13C, coupled with δ18O, can help separate sources of C and identify contributions from silicate weathering vs. carbonate weathering in fluids as well as pedogenic and lithogenic carbonates in soils (Cerling, 1984; Schulte et al., 2011). To date, δ13C and δ18O have been used to partition sources in carbonates at enhanced weathering sites (Knapp et al., 2023).
Radiocarbon (14C-dating) is expensive to analyze and requires both specialist preparation of samples, and equipment for measurement (e.g., accelerator mass spectrometry). However, radiocarbon can provide valuable insights into the age of products derived from enhanced weathering, and can help to pin-point modern atmospheric carbon sources with lower uncertainty than traditional stable carbon and oxygen isotopes (Knapp et al., 2023). Radiocarbon may be incorporated into the products of enhanced weathering, such as carbonates, derived from atmospheric CO2 which currently has a fixed 14C content from cosmic production in the atmosphere. Once incorporated, 14C starts to decay and the age of the carbonate since formation can be measured. Older sources of carbon (> ~ 60 kyrs), such as bedrock carbonate and shales, have isotopically ‘dead’ C, where 14C has fully decayed, potentially helping separate carbon supplied by EW from other sources of C in the system. Coupled with total carbon content, it is possible to extract a rate of atmospheric C removal using this technique (Knapp et al., 2023). Nevertheless, caution should be exercised when using both radiocarbon and stable carbon isotopes as these methods are affected by CO2 exchange and kinetic fractionation that may lead to misleading interpretations (Stubbs et al., 2023).
Radiogenic strontium (Sr) isotopes (87Sr/86Sr) are a common tracer for natural weathering reactions and sources of weathering fluxes (Blum and Erel, 2003; Faure and Mensing, 2005). Radiogenic Sr (87Sr) is produced from 87Rb through radioactive (β-) decay; hence the name radiogenic. Radiogenic Sr is usually normalized to non-radiogenic, stable 86Sr to facilitate comparison between phases with different Sr. concentrations (Blum, 1995; Blum and Erel, 2003). Silicate rock 87Sr/86Sr differ according to rock type (mantle or crustal, as Rb and Sr are fractionated during partial melting, with Rb concentrated in the melt) and age (Blum and Erel, 2003; Faure and Mensing, 2005). Carbonates and evaporites can inherit 87Sr/86Sr values from the water in which they are deposited, and have values which are distinct, and typically lower, than silicates. As a result, different rock types have distinct isotope compositions that can be used to trace the dissolution of silicate feedstocks in EW.
Strontium cations are relatively mobile, and Sr readily substitutes for Ca in minerals as they have similar atomic radii. Strontium enters pore waters and streams on a similar timescale as major cations, and therefore can be used to trace cation sources, in particular Ca. Hence, the isotope composition of Sr in effluent water can be used to investigate what mineralogical phases contribute to the dissolved signals through their dissolution (Peters et al., 2004; Andrews et al., 2016; Larkin et al., 2022). If the Sr isotope composition of soils and feedstocks, as well as Sr concentrations of the feedstock, are known, this can be used to estimate lithology-specific weathering rates at catchment scales (Négrel et al., 1993; Gaillardet et al., 1999a; Suhrhoff et al., 2022). This approach has also been applied to EW and used to estimate the relative cation flux contribution of carbonate and silicate weathering, and hence calculate CDR using cation stoichiometry (Larkin et al., 2022). Caution should be applied when using radiogenic Sr if EW feedstocks contain carbonates, as carbonates dissolve much quicker than silicate minerals and even trace amounts of carbonates are sufficient to dominate Sr isotope signatures of weathering fluxes, particularly in early weathering stages (Harris et al., 1998; Aubert et al., 2001; Jacobson et al., 2002).
Novel isotope tracers, such as magnesium (Mg), lithium (Li), silicon (Si), stable strontium (Sr) and calcium (Ca) can be used to trace many additional processes, including secondary mineral formation and plant uptake (Pistiner and Henderson, 2003; Georg et al., 2007; Opfergelt and Delmelle, 2012; Andrews et al., 2016; Pogge von Strandmann et al., 2021; Nelson et al., 2022). The majority of work to date on these isotope systems has revolved around quantifying fractionation factors and natural element cycling (Penniston-Dorland et al., 2017; Teng et al., 2017), but EW-specific applications are increasing (Pogge von Strandmann et al., 2021; Vienne et al., 2023). For these isotopic tracers strong fractionations are induced by interactions with secondary minerals, thus potentially allowing quantification of secondary mineral formation using isotope mass balance, that could inform on cation loss to exchangeable phases, or potential re-release of CO2 during secondary mineral formation (see section 3.3; Campbell et al., 2022). The initial isotope signature of different feedstocks might also be sufficiently unique to use as a source tracing tool, similar to radiogenic Sr.
Less research is available on the use of gas phase measurement for tracing EW in agricultural settings, which has been demonstrated in soil-less mine waste EW applications (e.g., Stubbs et al., 2022). However, in principle, changes in soil pCO2 and soil CO2 efflux could be used to indirectly calculate CDR. In theory, inorganic CDR by EW could potentially lead to measurable decrease in the CO2 efflux from respiration at the soil surface relative to a suitable control. Nevertheless, resolving an inorganic EW signal via this method is unlikely for two reasons. Firstly, it is not possible to distinguish between an organic and inorganic carbon effect on CO2 efflux via measurement of CO2 alone. Secondly, organic carbon fluxes dominate this measurement, and are around an order of magnitude higher (~25 tCO2.ha-1.yr-1; Weil and Brady, 2017; Lockhart et al., 2023) than any reduction that might be seen due to changes in inorganic fluxes from EW (<2 tCO2.ha-1.yr-1, normalized for 50 t rock powder/ha; Vandeginste et al., 2024; see SI). This means that a reduction in efflux from bicarbonate production during EW would be unlikely to be detected relative to a control, and that these measurements will be noisy (Weil and Brady, 2017). In practical terms, when the main aim is durable inorganic carbon removal, CO2 flux measurements are unlikely to be used for crediting purposes for EW in areas with soil and vegetation. Gas measurements still have utility in EW for due diligence, to ensure that the organic carbon cycle is not substantially perturbed, as CO2 may be released from increased respiration and microbial stimulation (Dietzen et al., 2018). Additionally, monitoring of other greenhouse gas fluxes like CH4 and N2O, has importance as these are also influenced by rock powder application (Chiaravalloti et al., 2023).
Varying interpretations about the effects of rock powders on gas fluxes have been obtained to date using gas flux chambers. Dietzen et al. (2018) found no significant increase for cumulative CO2 emissions for high rates of olivine application in an incubation experiment with organic rich acidic topsoil (0–10 cm), although corresponding lime application increased CO2 by 221%. In a similar experiment, Yan et al. (2023) found significantly increased CO2 emissions due to soil organic carbon mineralization when mixing 12 different soil types with wollastonite (although wollastonite application was extremely high at 10 wt%). Vienne et al. (2023) found significantly reduced CO2 emissions in a mesocosm experiment with high (100 t ha−1) basalt application rates compared to their control, although the addition of earthworms to the basalt plots increased the emissions. Gas measurements with the flux chamber LI-COR system confirm the CO2 drawdown potential of ultramafic rocks and oxides (Rausis et al., 2022; Stubbs et al., 2022), although these experiments were conducted without soil.
Despite being important contributions to the nascent field of gas phase measurements, a major limitation of the flux chamber approach is that they are point measurements at some moment during a highly variable daily CO2 flux curve. The extrapolations of these point measurements might result in significantly different results depending on the spatio-temporal pattern with which measurements have been taken. Besides the importance of feedstock mineralogy, these studies found the major parameters influencing CO2 efflux measurements to be water content, porosity, and permeability. Large scale measurements have been employed through eddy covariance towers (Kantola et al., 2023). These measurement towers yield valuable data about overall ecosystem carbon dynamics. However, the sensor height of somewhere between 1 to 2.5 m might cause significant dilution of the gas phase through the overlay of various fluxes, and thus may not provide the necessary resolution needed for in-depth discrimination of driving mechanisms.
A comprehensive measurement strategy will capture variability in CDR estimates incorporating natural infield spatial and temporal variability, systematic uncertainty from measurement approaches, and analytical external reproducibility. We expect a multidimensional approach would create a pool of CDR estimates and would allow for a data distribution to be generated. Such an intercomparison would also allow for internal consistency checks and quality control on all data generated, including identification and investigation of any outliers. The most conservative estimate of CDR would be to utilize the lowermost capture estimates, as this corresponds to a high probability of removal. However, combining different measurement methods and spatial scales would allow for statistical treatment of all datasets and a distribution to be produced, resulting in an overall CDR estimate with appropriate confidence intervals. Understanding any systematic biases and assumptions, due to different measurement approaches, will be paramount to produce the most accurate and precise CDR value. Understanding and appropriate consideration of statistical significance, heterogeneity and error propagation are central to proper interpretation of any data generated. Compounded with uncertainties from measurement approaches, all methods are limited by the ability to produce data that is representative of the EW activity area, but baseline sampling using agronomic and geochemical indicators could help refine sample plans to ensure variability is covered.
Additional uncertainties may derive from the estimates of rock powder application rate. The logistically simplest method to estimate application rates is via spreading operation data. Alternatively, there is potential for using immobile trace elements in pre- and post- application soils to either estimate true applications rates, or use as a normalization procedure to account for application rate variance (Kantola et al., 2023; Reershemius et al., 2023; Wolf et al., 2023). These solid phase approaches, however, rely on accurate extrapolation across a deployment area. Importantly, high application rates (50–100 t ha−1) that exceed practical agronomic application rates (1–20 t /ha−1, Swoboda et al., 2022) are typical for most ongoing EW experiments in order to obtain clear signals. However, such large amounts, especially when surface applied or mixed only in a shallow (0–10 cm) soil layer, might introduce alkalinity hotspots upon dissolution and thereby significantly alter the micro- and macropore saturation states, which could in turn slow dissolution kinetics. Thus, extrapolating CO2 drawdown rates from such high application amounts might be prone to error.
Temporal uncertainty is perhaps one of the largest limiting factors for EW measurement approaches. The weathering of silicate minerals is a continuum, meaning that the removal of CO2 on site follows a weathering curve which may continue on decadal to centennial timescales (Kanzaki et al., 2022). Each method outlined here is applicable to certain time windows, with only solid phase measurements providing a temporally integrated weathering measure. In addition to this, there is spatial and temporal overlap between mineral dissolution, transport and storage (Figure 1). While beyond the scope of this review, we call for clear guidance from regulators as to what point in time a credit can be issued relative to measurement taken at the weathering site.
The long term use of chemical, nitrogen and phosphorus based fertilizers in nutrient poor soils may lead to complications for tracing enhanced weathering as well as reducing the CDR efficiency (Andrews and Taylor, 2019). Application of certain fertilizers may lead to the formation of strong mineral acids, including nitric and phosphoric acid. Sulfuric acid can also be present if feedstocks, such as some ultramafic rock types, contain sulfide minerals (Lerman and Wu, 2006; Horan et al., 2019; Relph et al., 2021). Organic acid-driven weathering is also likely in some agronomic settings, such as with the application of high organic fertilizers, presence of humic acids (Basak et al., 2017; Swoboda et al., 2021; Busato et al., 2022) or due to plant-derived organic acids (Vicca et al., 2022). Non-carbonic acids present multiple complications for CDR estimates (Taylor et al., 2021; Zhang et al., 2022). Firstly, mineral acids are far stronger than carbonic acids, and hence readily provide acidity for mineral dissolution. This means that alkaline silicate minerals can be dissolved by non-carbonic acids, releasing cations (red-dashed arrow, Figure 2), but with no CO2 sequestration (no HCO3− formation), effectively decreasing the CDR potential for the rock powder. That said, the export of cations from the weathering site could help buffer downstream processes and CO2 loss.
As a result of unknown acid dissolutions, any CDR method that measures only cation concentrations as a weathering product (such as soil only approaches) cannot distinguish which acid caused weathering and hence the true amount of CO2 removed. For liquid based approaches, however, it may be possible to use the relationship of HCO3− vs. [Ca2+ + Mg2+] as evidence for carbonic acid weathering (on a charge equivalence basis after correcting for rainfall and fertilizer salts; Hamilton et al., 2007; Perrin et al., 2008; Larkin et al., 2022). Non-carbonic acid weathering supplies cations without HCO3−, creating anomalous bicarbonate-cation relationships. Furthermore, water NO3− concentrations in effluent water or burried resin capsules (Kantola et al., 2023) could be used to estimate the degree of onsite/in situ strong acid weathering and correct for cation supply by strong acids (Larkin et al., 2022). A recent study on EW with basalt powders in the midwest United States identified that less than 2% of the total cation flux was derived from nitric acids (Kantola et al., 2023), hinting that such losses may be minor. This estimate was made using the application rate of nitrogen fertilizer (liquid ammonium nitrate), however the approach does not capture the temporal dynamics of nitric acid weathering or the presence of other acids. The rate and amount of strong acid weathering must be characterized across a range of environments and agronomic settings. Moreover, nitrate, as a key nutrient, is short-lived in most natural environments (Meybeck, 1982) and so the long term fate of weathering products from nitric acid weathering is not known. Dietzen and Rosing (2023) suggest that the contribution of non-carbonic acids to soil pH can be accounted for using the difference between pH predicted by pCO2 alone and the true pH of the soil, potentially deriving a correction factor for mineral weathering due to non-carbonic acids. Importantly, their approach suggests that, depending on soil pCO2, below pH 4.5–5.5, more than 50% of weathering occurs due to strong acids. Only above a pH of 5.2–6 (again, depending on soil pCO2) can most of the weathering be assumed to derive from carbonic acid. This work, however, is based on soil pH only, and pore water pH can be closer to neutral even in acidic soils, and will rapidly increase with the addition of alkaline feedstocks (Bertagni and Porporato, 2022). Clearly, in such settings, accurate quantification of strong acid weathering is of prime importance for overall carbon budgets.
Even if estimates of non-carbonic acid weathering can be made, high baseline weathering rates, caused by extensive historical fertilizer use, can decrease the signal–baseline ratio making it difficult to resolve weathering changes as a result of EW activity (Larkin et al., 2022). Cation interactions with fertilizer components can add further complications to measuring protocols, for example the formation of hydroxyapatite minerals (Wood et al., 2023) that remove cations from solution but are robust enough to avoid extraction via leaching methods.
Ag-lime may act as a transient source or sink of CO2 depending on the relative contributions of acidity from strong acids versus carbonic acid as weathering proceeds (West and McBride, 2005; Hamilton et al., 2007). Further work is needed to understand the net carbon balance with the application of Ag-lime, however, providing additionality can be shown, it may hold promise as an alternative feedstock for EW due its fast dissolution kinetics (Knapp and Tipper, 2022).
In the case where soil (pedogenic) carbonates are a significant storage pool, strong acids present a risk of reversal (storage failure) through carbonate dissolution via strong acids. CO2 emission is also possible if the EW material itself contains any carbonate minerals, e.g., in concrete, or trace calcite in mafic rocks (Dietzen et al., 2018; Kemp et al., 2022; Larkin et al., 2022; Zhang et al., 2022; Skov et al., 2024). Ag-lime, as well as silicate minerals, may also potentially help offset excess acidity from non-carbonic acids, allowing further weathering by carbonate or silicate minerals to occur via carbonic acid instead (Hamilton et al., 2007).
With the supply of silica, aluminum, iron and cations from rock powder dissolution, the formation of secondary minerals is naturally enhanced. In addition to carbonate minerals, the most common secondary minerals are authigenic clays, amorphous silica, metal oxides and oxyhydroxides. Their formation is a function of weathering congruence, a term that describes the tendency for a mineral to dissolve completely and for the weathering products to be removed in solution, and is controlled by factors such as dissolution kinetics, ambient conditions (pH, eH), rainfall and especially porosity and permeability. Secondary minerals can form coatings on primary minerals, thereby isolating the mineral surface from reactive fluids and decreasing weathering rates, but the impact on dissolution rates is highly contextual (see Oelkers et al., 2019 for review).
By extension, it is not clear if CDR and its rate linearly increase with feedstock application, or whether high feedstock application rates promote supersaturation of secondary phases and surface passivation, which may act as a negative feedback on the CDR rate (Calabrese et al., 2022). Potentially, secondary mineral formation could increase reaction kinetics in some cases as high elemental saturation states may limit primary mineral dissolution (Köhler et al., 2010; Schuiling et al., 2011). Secondary precipitates can thereby decrease the fluid saturation state and potentially promote primary mineral dissolution (Harrington et al., 2023). From a monitoring perspective, secondary minerals increase the cation exchange capacity of the soil, which has implications for identifying weathering signals using liquids and total carbon export.
One further complication deriving from authigenic clay mineral formation (“reverse weathering”) is the potential for CO2 degassing (Fuhr et al., 2022) e.g.:
In marine settings, the importance of reverse weathering, and increased CO2 flux, is clear for global carbon cycling on geological time scales (Isson and Planavsky, 2018; Bayon et al., 2022). That said, the role of reserve weathering in terrestrial soils and EW applications is poorly understood (Renforth and Campbell, 2021).
Vegetation represents an immediate complication for CDR estimates based on liquids and exchangeable phase analysis, as they will selectively remove weathering products from solution. Thus, in order to complete the weathering mass balance, vegetation must be sampled to estimate cation loss from the system (Shao et al., 2015; Reershemius et al., 2023). Without accounting for this cation loss, there is a risk of underestimating the cation released by rock powder dissolution. That said, the effects are dependant on the cation species, and plant uptake has been shown to be relatively minor in comparison to cation release from basalt powder by weathering in EW field trials with corn/soy and miscanthus in the US Midwest (Kantola et al., 2023). Monitoring vegetation chemistry is also important for understanding the health risk of metals sourced from rock powders (Dupla et al., 2023), and must follow standardized agronomy practices that consider metal compartmentalization in plants and decrease exposure risk (Brune et al., 1995; Thomas and Reid, 2021). One of the biggest limitations caused by vegetation is simply the removal of liquids from the soil, which makes in-field liquids sampling difficult and contributes to uncertainty in flow rate calculations.
The impacts of cation removal by vegetation on wider system carbon cycling is currently understudied. For example, the vegetative removal of cations will disturb the charge balance of anions, including bicarbonate, in remaining pore waters (Britto and Kronzucker, 2008; Amann et al., 2022). The spatial and temporal aspects must also be considered, as, similar to organic carbon cycling, cation cycling in vegetation will be short term with the potential to return cations to the weathering system in cases where vegetation (or at least most of its biomass) is not removed from the system (e.g., harvested; Banwart et al., 2009).
While EW is a relatively new approach to CDR, the underlying science behind quantifying weathering rates in soils is well established and EW specific research is progressing rapidly in academia and the private sector. Moreover, while quantification of both weathering and subsequent carbonation rates should be advanced further through research, multiple methods exist today that are readily available to form the foundation of MRV approaches (Puro. earth, 2022; Sutherland et al., 2024), primarily through tracking mineral constituents or directly monitoring bicarbonate formation and export. The majority of measurement types can be made in different settings, including experiments that explore weathering fundamentals and dissolution kinetics, or field settings that monitor real world processes. This allows for a detailed determination of weathering parameters through the combination of several measurement types, and a more robust estimate of atmospheric CO2 removal. The efficacy of each approach in EW operations will vary with application material, soil conditions and soil management strategies that dictate the level of complexity of the system.
Considering the future scaling of the EW industry to reach the projected gigatonne removal potential, robust measurement campaigns can provide the foundation for geochemical modeling, including EW specific models (Kelland et al., 2020; Kanzaki et al., 2022; Vienne et al., 2022; Bertagni et al., 2024). However, a strong measurement component is urgently required to refine, calibrate and validate geochemical models. This is particularly relevant as current favored reaction transport or simplified dissolution models are described as more comparable to closed system batch reactors than natural field conditions, lacking real world processes such as wetting-drying cycles, spatial resolution of permeability and flow paths, secondary mineral formation (Kelland et al., 2020) or exchange processes (Beerling et al., 2020; Kantzas et al., 2022). Moreover, there is large uncertainty in core input parameters such as mineral dissolution kinetics (Calabrese et al., 2022) and models calibrated under certain experimental setups have varying success to predict empirical datasets in other applications (Vienne et al., 2022; Bertagni et al., 2024). The EW community would benefit from an intermodel comparison project, similar to those used for climate models (CMIP), especially when considering downstream processes.
The success of EW as a CDR pathway will vary based on localized factors (e.g., climatic, geologic and agricultural; Cipolla et al., 2021, 2022), analogous to controls on natural weathering rates (West et al., 2005; Brantley et al., 2023), as well as rock type and reactivity (mineralogy, particle size and surface area; Taylor et al., 2016; Renforth, 2019). While rock powder dissolution in soil environments is highly complex at a local scale, leading to high spatial variability in measurements, this variability will become averaged out at larger spatial scales. As EW operations in a given catchment area expand, riverine monitoring may become the primary approach for quantification of carbon removed, rendering measurements at the soil level of secondary importance. To successfully identify enhanced weathering signals in rivers, however, requires that the signal is resolvable over baseline variability, which is challenging in environments of high baseline weathering activity, for example due to historic fertilizer use (Larkin et al., 2022; Mu et al., 2023). Innovative approaches to monitoring over larger spatial and temporal scales should be explored, as they may be required to complement local measurements and models for deployment at scales of megatonne or gigatonne CDR across wider geographies.
All measurement approaches require an accurate dynamic baseline via controlled trials (e.g., ‘untreated’ measurements) to quantify counterfactual weathering rates, that is, carbon capture that would have happened in the absence of application of EW feedstocks. This is particularly important to understand if feedstocks are replacing other agricultural amendments (such as Ag-lime or synthetic fertilizers) or in scenarios where rock powder application might already be practiced (albeit at lower application rates than are typical for EW; Swoboda et al., 2022). It is important that the baseline operations cover highly comparable geographic and climatic gradients as active EW applications.
It should also be stressed that CDR via EW is embedded in a complex network of organic and inorganic processes between multiple stocks of carbon involving fluxes of several greenhouse gasses, which have temporally variable responses. This complexity applies to agricultural systems in general, including traditional or sustainable land management practices with (no)tilling, fertilizers and AgLime (Golchin et al., 1995; Marland et al., 2003; West and McBride, 2005; Smith et al., 2010; Rousset et al., 2023). A holistic assessment of the CDR impact of EW must therefore account for changes in organic carbon reservoirs and resulting fluxes (Dietzen et al., 2018; Almaraz et al., 2022; Yan et al., 2023; Buss et al., 2024).
At this point in time, all EW operations, private sector or academic, are in a phase where data production is paramount to collectively solve the challenges of quantifying weathering activity, and current standards allow this research to occur in parallel to large scale deployments under crediting scenarios. Given the complexity of the soil system, and potential for competing processes to create uncertainty, we stress that multidimensional measurement campaigns should be undertaken and prioritized. Ultimately, scaling of EW will depend on public acceptance of this CDR approach; it is therefore in the interest of all stakeholders to be transparent in their measurement approaches and to provide a scientific basis and rationale for deployment of EW as a solution to address climate change.
MC: Conceptualization, Writing – original draft, Writing – review & editing. CL: Writing – original draft, Writing – review & editing. PS: Writing – review & editing, Writing – original draft. TR: Writing – review & editing, Writing – original draft. TS: Writing – review & editing, Writing – original draft. CM: Writing – review & editing, Writing – original draft. JC: Writing – review & editing.
The author(s) declare that financial support was received for the research, authorship, and/or publication of this article. JC was funded by the European Union’s Horizon 2020 Research and Innovation Program under grant 869357 (project OceanNETs: Ocean-based Negative Emission Technologies–analyzing the feasibility, risks, and co-benefits of ocean-based negative emission technologies for stabilizing the climate). TS is funded by the Swiss National Science Foundation (grant P500PN_210790). CM is funded through the Grantham Foundation for the Protection of the Environment.
We acknowledge and thank Elisabete T. Pedrosa for early discussions on some of the material in the manuscript.
MC, CL, and PS declare that they work for a for-profit company (InPlanet GmbH) deploying enhanced weathering for carbon dioxide removal. JC sits on the science advisory board for InPlanet.
The remaining authors declare that the research was conducted in the absence of any commercial or financial relationships that could be construed as a potential conflict of interest.
All claims expressed in this article are solely those of the authors and do not necessarily represent those of their affiliated organizations, or those of the publisher, the editors and the reviewers. Any product that may be evaluated in this article, or claim that may be made by its manufacturer, is not guaranteed or endorsed by the publisher.
The Supplementary material for this article can be found online at: https://www.frontiersin.org/articles/10.3389/fclim.2024.1345224/full#supplementary-material
Alley, W. M. (1984). On the treatment of evapotranspiration, soil moisture accounting, and aquifer recharge in monthly water balance models. Water Resour. Res. 20, 1137–1149. doi: 10.1029/WR020i008p01137
Alloway, B. J. (2013). Heavy metals in soils: Trace metals and metalloids in soils and their bioavailability. Dordrecht: Springer Netherlands.
Almaraz, M., Bingham, N. L., Holzer, I. O., Geoghegan, E. K., Goertzen, H., Sohng, J., et al. (2022). Methods for determining the CO2 removal capacity of enhanced weathering in agronomic settings. Front. Clim. 4:429. doi: 10.3389/fclim.2022.970429
Amann, T., and Hartmann, J. (2022). Carbon accounting for enhanced weathering. Front. Clim. 4:948. doi: 10.3389/fclim.2022.849948
Amann, T., Hartmann, J., Hellmann, R., Pedrosa, E. T., and Malik, A. (2022). Enhanced weathering potentials—the role of in situ CO2 and grain size distribution. Front. Clim. 4:268. doi: 10.3389/fclim.2022.929268
Amann, T., Hartmann, J., Struyf, E., De Oliveira Garcia, W., Fischer, E. K., Janssens, I., et al. (2020). Enhanced weathering and related element fluxes – a cropland mesocosm approach. Biogeosciences 17, 103–119. doi: 10.5194/bg-17-103-2020
Andrews, M. G., Jacobson, A. D., Lehn, G. O., Horton, T. W., and Craw, D. (2016). Radiogenic and stable Sr isotope ratios (87Sr/86Sr, δ88/86Sr) as tracers of riverine cation sources and biogeochemical cycling in the Milford sound region of Fiordland, New Zealand. Geochim. Cosmochim. Acta 173, 284–303. doi: 10.1016/j.gca.2015.10.005
Andrews, M. G., and Taylor, L. L. (2019). Combating climate change through enhanced weathering of agricultural soils. Elements 15, 253–258. doi: 10.2138/gselements.15.4.253
Aubert, D., Stille, P., and Probst, A. (2001). REE fractionation during granite weathering and removal by waters and suspended loads: Sr and Nd isotopic evidence. Geochim. Cosmochim. Acta 65, 387–406. doi: 10.1016/S0016-7037(00)00546-9
Baek, S. H., Kanzaki, Y., Lora, J. M., Planavsky, N., Reinhard, C. T., and Zhang, S. (2023). Impact of climate on the global capacity for enhanced rock weathering on croplands. Earths Future 11:e2023EF003698. doi: 10.1029/2023EF003698
Banwart, S. A., Berg, A., and Beerling, D. J. (2009). Process-based modeling of silicate mineral weathering responses to increasing atmospheric CO2 and climate change. Glob. Biogeochem. Cycles 23:243. doi: 10.1029/2008GB003243
Basak, B. B., Sarkar, B., Biswas, D. R., Sarkar, S., Sanderson, P., and Naidu, R. (2017). Bio-intervention of naturally occurring silicate minerals for alternative source of potassium: challenges and opportunities. Adv. Agron. 141, 115–145. doi: 10.1016/bs.agron.2016.10.016
Bayon, G., Bindeman, I. N., Trinquier, A., Retallack, G. J., and Bekker, A. (2022). Long-term evolution of terrestrial weathering and its link to Earth’s oxygenation. Earth Planet. Sci. Lett. 584:117490. doi: 10.1016/j.epsl.2022.117490
Beerling, D. J., Epihov, D. Z., Kantola, I. B., Masters, M. D., Reershemius, T., Planavsky, N. J., et al. (2023). Enhanced weathering in the U.S. Corn Belt delivers carbon removal with agronomic benefits. Proc Natl Acad Sci U S A 121:e2319436121. doi: 10.48550/arXiv.2307.05343
Beerling, D. J., Kantzas, E. P., Lomas, M. R., Wade, P., Eufrasio, R. M., Renforth, P., et al. (2020). Potential for large-scale CO2 removal via enhanced rock weathering with croplands. Nature 583, 242–248. doi: 10.1038/s41586-020-2448-9
Bergaya, F., Lagaly, G., and Vayer, M. (2006). “Chapter 12.10 cation and anion exchange” in Developments in clay science. eds. F. Bergaya, B. K. G. Theng, and G. Lagaly (Amsterdam, Netherlands: Elsevier), 979–1001.
Berner, E. K., and Berner, R. A. (2012). “Global environment: water, air, and geochemical cycles - second edition” in Global Environment. ed. E. K. Berner (Princeton, NJ: Princeton University Press).
Berner, R. A., Lasaga, A. C., and Garrels, R. M. (1983). Carbonate-silicate geochemical cycle and its effect on atmospheric carbon dioxide over the past 100 million years. Am. J. Sci. U. S. 283, 641–683. doi: 10.2475/ajs.283.7.641
Bertagni, M. B., Calabrese, S., Cipolla, G., Noto, L. V., and Porporato, A. M. (2024). Advancing enhanced weathering modeling in soils: systematic comparison and validation with experimental data. Preprints 720:183720. doi: 10.22541/essoar.170559500.09183720/v1
Bertagni, M. B., and Porporato, A. (2022). The carbon-capture efficiency of natural water Alkalinization: implications for enhanced weathering. Sci. Total Environ. 838:156524. doi: 10.1016/j.scitotenv.2022.156524
Bhatia, M. P., Kujawinski, E. B., Das, S. B., Breier, C. F., Henderson, P. B., and Charette, M. A. (2013). Greenland meltwater as a significant and potentially bioavailable source of iron to the ocean. Nat. Geosci. 6, 274–278. doi: 10.1038/ngeo1746
Blum, J. D., and Erel, Y. (1995). A silicate weathering mechanism linking increases in marine 87Sr/86Sr with global glaciation. Nature 373, 415–418. doi: 10.1038/373415a0
Blum, J. D., and Erel, Y. (2003). “5.12- radiogenic isotopes in weathering and hydrology” in Treatise on geochemistry. eds. H. D. Holland and K. K. Turekian (Oxford: Pergamon), 365–392.
Bolland, M., and Baker, M. J. (2000). Powdered granite is not an effective fertilizer for clover and wheat in sandy soils from Western Australia. Nutr. Cycl. Agroecosyst. 56, 59–68. doi: 10.1023/A:1009757525421
Brady, N. C., and Weil, R. R. (2008). The nature and properties of soils. Hoboken, NJ: Pearson Prentice Hall.
Brander, M., Ascui, F., Scott, V., and Tett, S. (2021). Carbon accounting for negative emissions technologies. Clim. Pol. 21, 699–717. doi: 10.1080/14693062.2021.1878009
Brantley, S. L., Shaughnessy, A., Lebedeva, M. I., and Balashov, V. N. (2023). How temperature-dependent silicate weathering acts as Earth’s geological thermostat. Science 379, 382–389. doi: 10.1126/science.add2922
Brimhall, G. H., and Dietrich, W. E. (1987). Constitutive mass balance relations between chemical composition, volume, density, porosity, and strain in metasomatic hydrochemical systems: results on weathering and pedogenesis. Geochim. Cosmochim. Acta 51, 567–587. doi: 10.1016/0016-7037(87)90070-6
Britto, D. T., and Kronzucker, H. J. (2008). Cellular mechanisms of potassium transport in plants. Physiol. Plant. 133, 637–650. doi: 10.1111/j.1399-3054.2008.01067.x
Brown, I. C. (1943). A rapid method of determining exchangeable hydrogen and total exchangeable bases of soils. Soil Sci. 56, 353–358. doi: 10.1097/00010694-194311000-00004
Brune, A., Urbach, W., and Dietz, K.-J. (1995). Differential toxicity of heavy metals is partly related to a loss of preferential extraplasmic compartmentation: a comparison of cd-, Mo-, Ni-and Zn-stress. New Phytol. 129, 403–409. doi: 10.1111/j.1469-8137.1995.tb04310.x
Busato, J. G., Dos Santos, L. F., Monteiro de Paula, A., Fabriz Sodré, F., de Oliveira, A. L., Dobbss, L. B., et al. (2022). Can co-application of silicate rock powder and humic-like acids increase nutrient uptake and plant growth in weathered tropical soil? Acta Agric. Scand. Sect. B Soil Plant Sci. 72, 761–774. doi: 10.1080/09064710.2022.2078222
Buss, W., Hasemer, H., Ferguson, S., and Borevitz, J. (2024). Stabilisation of soil organic matter with rock dust partially counteracted by plants. Glob. Change Biol. 30:e17052. doi: 10.1111/gcb.17052
Calabrese, S., Parolari, A. J., and Porporato, A. (2017). Hydrologic transport of dissolved inorganic carbon and its control on chemical weathering. J. Geophys. Res. Earth Surf. 122, 2016–2032. doi: 10.1002/2017JF004346
Calabrese, S., Wild, B., Bertagni, M. B., Bourg, I. C., White, C., Aburto, F., et al. (2022). Nano-to global-scale uncertainties in terrestrial enhanced weathering. Environ. Sci. Technol. 56, 15261–15272. doi: 10.1021/acs.est.2c03163
Campbell, J., Bastianini, L., Buckman, J., Bullock, L., Foteinis, S., Furey, V., et al. (2023). Measurements in geochemical carbon dioxide removal. Heriot Watt Univ. 2023:8. doi: 10.17861/2GE7-RE08
Campbell, J. S., Foteinis, S., Furey, V., Hawrot, O., Pike, D., Aeschlimann, S., et al. (2022). Geochemical negative emissions technologies: part I. Review. Front. Clim. 4:133. doi: 10.3389/fclim.2022.879133
Canadian Wollastonite . (2017). Canadian Wollastonite. History Available at: https://www.canadianwollastonite.com/about/history/ (Accessed April 18, 2024).
Carbon Standards International . (2022). Global Rock C-Sink. Available at: https://www.european-biochar.org/media/doc/139/rock-c-guidelines_0_9.pdf (Accessed October 26, 2023).
Cerling, T. E. (1984). The stable isotopic composition of modern soil carbonate and its relationship to climate. Earth Planet. Sci. Lett. 71, 229–240. doi: 10.1016/0012-821X(84)90089-X
Chiaravalloti, I., Theunissen, N., Zhang, S., Wang, J., Sun, F., Ahmed, A. A., et al. (2023). Mitigation of soil nitrous oxide emissions during maize production with basalt amendments. Front. Clim. 5:3043. doi: 10.3389/fclim.2023.1203043
Chittenden, E. T., Stanton, D. J., Watson, J., and Dodson, K. J. (1967). Serpentine and dunite as magnesium fertiliser. N. Z. J. Agric. Res. 10, 160–171. doi: 10.1080/00288233.1967.10423088
Cipolla, G., Calabrese, S., Noto, L. V., and Porporato, A. (2021). The role of hydrology on enhanced weathering for carbon sequestration II. From hydroclimatic scenarios to carbon-sequestration efficiencies. Adv. Water Resour. 154:103949. doi: 10.1016/j.advwatres.2021.103949
Cipolla, G., Calabrese, S., Porporato, A., and Noto, L. V. (2022). Effects of precipitation seasonality, irrigation, vegetation cycle and soil type on enhanced weathering – modeling of cropland case studies across four sites. Biogeosciences 19, 3877–3896. doi: 10.5194/bg-19-3877-2022
Conceição, L. T., Silva, G. N., Holsback, H. M. S., De Oliveira, C., Marcante, N. C., Martins, É. S., et al. (2022). Potential of basalt dust to improve soil fertility and crop nutrition. J. Agric. Food Res. 10:100443. doi: 10.1016/j.jafr.2022.100443
Deng, H., Sonnenthal, E., Arora, B., Breunig, H., Brodie, E., Kleber, M., et al. (2023). The environmental controls on efficiency of enhanced rock weathering in soils. Sci. Rep. 13:9765. doi: 10.1038/s41598-023-36113-4
Dietzen, C., Harrison, R., and Michelsen-Correa, S. (2018). Effectiveness of enhanced mineral weathering as a carbon sequestration tool and alternative to agricultural lime: an incubation experiment. Int. J. Greenh. Gas Control 74, 251–258. doi: 10.1016/j.ijggc.2018.05.007
Dietzen, C., and Rosing, M. T. (2023). Quantification of CO2 uptake by enhanced weathering of silicate minerals applied to acidic soils. Int. J. Greenh. Gas Control 125:103872. doi: 10.1016/j.ijggc.2023.103872
Dudhaiya, A., Haque, F., Fantucci, H., and Santos, R. M. (2019). Characterization of physically fractionated wollastonite-amended agricultural soils. Fortschr. Mineral. 9:635. doi: 10.3390/min9100635
Dupla, X., Möller, B., Baveye, P. C., and Grand, S. (2023). Potential accumulation of toxic trace elements in soils during enhanced rock weathering. Eur. J. Soil Sci. 74:e13343. doi: 10.1111/ejss.13343
Eufrasio, R. M., Kantzas, E. P., Edwards, N. R., Holden, P. B., Pollitt, H., Mercure, J.-F., et al. (2022). Environmental and health impacts of atmospheric CO2 removal by enhanced rock weathering depend on nations’ energy mix. Commun. Earth Environ. 3:106. doi: 10.1038/s43247-022-00436-3
Faure, G., and Mensing, T. M. (2005). Principles and applications. New York: John Wiley and Sons, Inc.
Fritz, S. J. (1994). A survey of charge-balance errors on published analyses of potable ground and surface waters. Groundwater 32, 539–546. doi: 10.1111/j.1745-6584.1994.tb00888.x
Fuhr, M., Geilert, S., Schmidt, M., Liebetrau, V., Vogt, C., Ledwig, B., et al. (2022). Kinetics of olivine weathering in seawater: an experimental study. Front. Clim. 4:1587. doi: 10.3389/fclim.2022.831587
Fuss, S., Lamb, W. F., Callaghan, M. W., Hilaire, J., Creutzig, F., Amann, T., et al. (2018). Negative emissions–part 2: costs, potentials and side effects. Environ. Res. Lett. 13:063002. doi: 10.1088/1748-9326/aabf9f
Gaillardet, J., Dupré, B., and Allègre, C. J. (1999a). Geochemistry of large river suspended sediments: silicate weathering or recycling tracer? Geochim. Cosmochim. Acta 63, 4037–4051. doi: 10.1016/S0016-7037(99)00307-5
Gaillardet, J., Dupré, B., Louvat, P., and Allègre, C. J. (1999b). Global silicate weathering and CO2 consumption rates deduced from the chemistry of large rivers. Chem. Geol. 159, 3–30. doi: 10.1016/S0009-2541(99)00031-5
Galy, A., and France-Lanord, C. (1999). Weathering processes in the Ganges–Brahmaputra basin and the riverine alkalinity budget. Chem. Geol. 159, 31–60. doi: 10.1016/S0009-2541(99)00033-9
Georg, R. B., Reynolds, B. C., West, A. J., Burton, K. W., and Halliday, A. N. (2007). Silicon isotope variations accompanying basalt weathering in Iceland. Earth Planet. Sci. Lett. 261, 476–490. doi: 10.1016/j.epsl.2007.07.004
Golchin, A., Clarke, P., Oades, J. M., and Skjemstad, J. O. (1995). The effects of cultivation on the composition of organic-matter and structural stability of soils. Soil Res. 33, 975–993. doi: 10.1071/SR9950975
Goldschmidt, V. M. (1922). Oversikgtskart over utbredelsen av de forskjellige kalimineraler I norsk fjeldgrund. Nor. Landmansblad 41, 268–269.
Goll, D. S., Ciais, P., Amann, T., Buermann, W., Chang, J., Eker, S., et al. (2021). Potential CO2 removal from enhanced weathering by ecosystem responses to powdered rock. Nat. Geosci. 14, 545–549. doi: 10.1038/s41561-021-00798-x
Gran, G. (1952). Determination of the equivalence point in potentiometric titrations. Part II. Analyst 77, 661–671. doi: 10.1039/AN9527700661
Groenenberg, J. E., Römkens, P. F., Zomeren, A. V., Rodrigues, S. M., and Comans, R. N. (2017). Evaluation of the single dilute (0.43 M) nitric acid extraction to determine geochemically reactive elements in soil. Environ. Sci. Technol. 51, 2246–2253. doi: 10.1021/acs.est.6b05151
Guo, F., Wang, Y., Zhu, H., Zhang, C., Sun, H., Fang, Z., et al. (2023). Crop productivity and soil inorganic carbon change mediated by enhanced rock weathering in farmland: a comparative field analysis of multi-agroclimatic regions in Central China. Agric. Syst. 210:103691. doi: 10.1016/j.agsy.2023.103691
Hamilton, S. K., Kurzman, A. L., Arango, C., Jin, L., and Robertson, G. P. (2007). Evidence for carbon sequestration by agricultural liming. Glob. Biogeochem. Cycles 21:2738. doi: 10.1029/2006GB002738
Haque, F., Khalidy, R., Chiang, Y. W., and Santos, R. M. (2023). Constraining the capacity of global croplands to CO2 drawdown via mineral weathering. ACS Earth Space Chem. 7, 1294–1305. doi: 10.1021/acsearthspacechem.2c00374
Haque, F., Santos, R. M., and Chiang, Y. W. (2020). CO2 sequestration by wollastonite-amended agricultural soils – an Ontario field study. Int. J. Greenh. Gas Control 97:103017. doi: 10.1016/j.ijggc.2020.103017
Haque, F., Santos, R. M., Dutta, A., Thimmanagari, M., and Chiang, Y. W. (2019). Co-benefits of Wollastonite weathering in agriculture: CO2 sequestration and promoted plant growth. ACS Omega 4, 1425–1433. doi: 10.1021/acsomega.8b02477
Harrington, K. J., Hilton, R. G., and Henderson, G. M. (2023). Implications of the riverine response to enhanced weathering for CO2 removal in the UK. Appl. Geochem. 152:105643. doi: 10.1016/j.apgeochem.2023.105643
Harris, N., Bickle, M., Chapman, H., Fairchild, I., and Bunbury, J. (1998). The significance of Himalayan rivers for silicate weathering rates: evidence from the Bhote Kosi tributary. Chem. Geol. 144, 205–220. doi: 10.1016/S0009-2541(97)00132-0
Hartmann, J., Lauerwald, R., and Moosdorf, N. (2014). A brief overview of the GLObal RIver chemistry database, GLORICH. Proc. Earth Planet. Sci. 10, 23–27. doi: 10.1016/j.proeps.2014.08.005
Hartmann, J., West, A. J., Renforth, P., Köhler, P., De La Rocha, C. L., Wolf-Gladrow, D. A., et al. (2013). Enhanced chemical weathering as a geoengineering strategy to reduce atmospheric carbon dioxide, supply nutrients, and mitigate ocean acidification. Rev. Geophys. 51, 113–149. doi: 10.1002/rog.20004
Hilton, R. G., and West, A. J. (2020). Mountains, erosion and the carbon cycle. Nat. Rev. Earth Environ. 1, 284–299. doi: 10.1038/s43017-020-0058-6
Holzer, I. O., Nocco, M. A., and Houlton, B. Z. (2023a). Direct evidence for atmospheric carbon dioxide removal via enhanced weathering in cropland soil. Environ. Res. Commun. 5:101004. doi: 10.1088/2515-7620/acfd89
Holzer, I., Sokol, N., Slessarev, E., Martin, K., and Chay, F. (2023b). Quantifying enhanced weathering – CarbonPlan. Available at: https://carbonplan.org/research/ew-quantification-explainer (Accessed October 16, 2023).
Horan, K., Hilton, R. G., Dellinger, M., Tipper, E., Galy, V., Calmels, D., et al. (2019). Carbon dioxide emissions by rock organic carbon oxidation and the net geochemical carbon budget of the Mackenzie River basin. Am. J. Sci. 319, 473–499. doi: 10.2475/06.2019.02
Ilchenko, W. (1955). Os tufos da Mata da Corda e seu emprego na Agricultura. Dep. Prod. Veg. Belo Horiz. Bol. Agric. 1, 9–10.
IPCC (2022). “Climate change 2022: Impacts, adaptation and vulnerability” in Contribution of working group III to the sixth assessment report of the intergovernmental panel on climate change. eds. P. R. Shukla, J. Skea, R. Slade, A. Al Khourdajie, R. Van Diemen, and D. McCollum (Cambridge: Cambridge University Press).
Isson, T. T., and Planavsky, N. J. (2018). Reverse weathering as a long-term stabilizer of marine pH and planetary climate. Nature 560, 471–475. doi: 10.1038/s41586-018-0408-4
Jacobson, A. D., and Blum, J. D. (2003). Relationship between mechanical erosion and atmospheric CO2 consumption in the New Zealand southern Alps. Geology 31, 865–868. doi: 10.1130/G19662.1
Jacobson, A. D., Blum, J. D., and Walter, L. M. (2002). Reconciling the elemental and Sr isotope composition of Himalayan weathering fluxes: insights from the carbonate geochemistry of stream waters. Geochim. Cosmochim. Acta 66, 3417–3429. doi: 10.1016/S0016-7037(02)00951-1
Jerden, J., Mejbel, M., Filho, A. N. Z., Carroll, M., and Campe, J. (2024). The impact of geochemical and life-cycle variables on carbon dioxide removal by enhanced rock weathering: development and application of the Stella ERW model. Appl. Geochem. 167:106002. doi: 10.1016/j.apgeochem.2024.106002
Kantola, I. B., Blanc-Betes, E., Masters, M. D., Chang, E., Marklein, A., Moore, C. E., et al. (2023). Improved net carbon budgets in the US Midwest through direct measured impacts of enhanced weathering. Glob. Change Biol. 29, 7012–7028. doi: 10.1111/gcb.16903
Kantzas, E. P., Val Martin, M., Lomas, M. R., Eufrasio, R. M., Renforth, P., Lewis, A. L., et al. (2022). Substantial carbon drawdown potential from enhanced rock weathering in the United Kingdom. Nat. Geosci. 15, 382–389. doi: 10.1038/s41561-022-00925-2
Kanzaki, Y., Planavsky, N. J., and Reinhard, C. T. (2023). New estimates of the storage permanence and ocean co-benefits of enhanced rock weathering. PNAS Nexus 2:pgad059. doi: 10.1093/pnasnexus/pgad059
Kanzaki, Y., Zhang, S., Planavsky, N. J., and Reinhard, C. T. (2022). Soil cycles of elements simulator for predicting TERrestrial regulation of greenhouse gases: SCEPTER v0.9. Geosci. Model Dev. 15, 4959–4990. doi: 10.5194/gmd-15-4959-2022
Kelland, M. E., Wade, P. W., Lewis, A. L., Taylor, L. L., Sarkar, B., Andrews, M. G., et al. (2020). Increased yield and CO2 sequestration potential with the C4 cereal Sorghum bicolor cultivated in basaltic rock dust-amended agricultural soil. Glob. Change Biol. 26, 3658–3676. doi: 10.1111/gcb.15089
Kemp, S. J., Lewis, A. L., and Rushton, J. C. (2022). Detection and quantification of low levels of carbonate mineral species using thermogravimetric-mass spectrometry to validate CO2 drawdown via enhanced rock weathering. Appl. Geochem. 146:105465. doi: 10.1016/j.apgeochem.2022.105465
Kerr, D. E., Brown, P. J., Grey, A., and Kelleher, B. P. (2021). The influence of organic alkalinity on the carbonate system in coastal waters. Mar. Chem. 237:104050. doi: 10.1016/j.marchem.2021.104050
Khalidy, R., Haque, F., Chiang, Y. W., and Santos, R. M. (2021). Monitoring Pedogenic inorganic carbon accumulation due to weathering of amended silicate minerals in agricultural soils. J. Vis. Exp. 2021:1996. doi: 10.3791/61996
Knapp, W. J., Stevenson, E. I., Renforth, P., Ascough, P. L., Knight, A. C. G., Bridgestock, L., et al. (2023). Quantifying CO2 removal at enhanced weathering sites: a multiproxy approach. Environ. Sci. Technol. 57, 9854–9864. doi: 10.1021/acs.est.3c03757
Knapp, W. J., and Tipper, E. T. (2022). The efficacy of enhancing carbonate weathering for carbon dioxide sequestration. Front. Clim. 4:215. doi: 10.3389/fclim.2022.928215
Köhler, P., Hartmann, J., and Wolf-Gladrow, D. A. (2010). Geoengineering potential of artificially enhanced silicate weathering of olivine. Proc. Natl. Acad. Sci. 107, 20228–20233. doi: 10.1073/pnas.1000545107
Larkin, C. S., Andrews, M. G., Pearce, C. R., Yeong, K. L., Beerling, D. J., Bellamy, J., et al. (2022). Quantification of CO2 removal in a large-scale enhanced weathering field trial on an oil palm plantation in Sabah, Malaysia. Front. Clim. 4:9229. doi: 10.3389/fclim.2022.959229
Larkin, C. S., Piotrowski, A. M., Hindshaw, R. S., Bayon, G., Hilton, R. G., Baronas, J. J., et al. (2021). Constraints on the source of reactive phases in sediment from a major Arctic river using neodymium isotopes. Earth Planet. Sci. Lett. 565:116933. doi: 10.1016/j.epsl.2021.116933
Lefebvre, D., Goglio, P., Williams, A., Manning, D. A., De Azevedo, A. C., Bergmann, M., et al. (2019). Assessing the potential of soil carbonation and enhanced weathering through life cycle assessment: a case study for São Paulo state, Brazil. J. Clean. Prod. 233, 468–481. doi: 10.1016/j.jclepro.2019.06.099
Leonardos, O. H., Theodoro, S. H., and Assad, M. L. (2000). Remineralization for sustainable agriculture: a tropical perspective from a Brazilian viewpoint. Nutr. Cycl. Agroecosyst. 56, 3–9. doi: 10.1023/A:1009855409700
Lerman, A., and Wu, L. (2006). CO2 and sulfuric acid controls of weathering and river water composition. J. Geochem. Explor. 88, 427–430. doi: 10.1016/j.gexplo.2005.08.100
Lewis, A. L., Sarkar, B., Wade, P., Kemp, S. J., Hodson, M. E., Taylor, L. L., et al. (2021). Effects of mineralogy, chemistry and physical properties of basalts on carbon capture potential and plant-nutrient element release via enhanced weathering. Appl. Geochem. 132:105023. doi: 10.1016/j.apgeochem.2021.105023
Liu, F., and Wang, D. (2022). Dissolved organic carbon concentration and biodegradability across the global rivers: A meta-analysis. Sci. Total Environ. 818:151828. doi: 10.1016/j.scitotenv.2021.151828
Lockhart, S. R. A., Keller, C. K., Evans, R. D., Carpenter-Boggs, L. A., and Huggins, D. R. (2023). Soil CO2 in organic and no-till agroecosystems. Agric. Ecosyst. Environ. 349:108442. doi: 10.1016/j.agee.2023.108442
Maesano, C. N., Campbell, J. S., Foteinis, S., Furey, V., Hawrot, O., Pike, D., et al. (2022). Geochemical negative emissions technologies: part II. Roadmap. Front. Clim. 4:5332. doi: 10.3389/fclim.2022.945332
Manning, D. A. C., Renforth, P., Lopez-Capel, E., Robertson, S., and Ghazireh, N. (2013). Carbonate precipitation in artificial soils produced from basaltic quarry fines and composts: an opportunity for passive carbon sequestration. Int. J. Greenh. Gas Control 17, 309–317. doi: 10.1016/j.ijggc.2013.05.012
Manning, D. A. C., and Theodoro, S. H. (2020). Enabling food security through use of local rocks and minerals. Extr. Ind. Soc. 7, 480–487. doi: 10.1016/j.exis.2018.11.002
Marland, G., West, T. O., Schlamadinger, B., and Canella, L. (2003). Managing soil organic carbon in agriculture: the net effect on greenhouse gas emissions. Tellus Ser. B Chem. Phys. Meteorol. 55, 613–621. doi: 10.3402/tellusb.v55i2.17042
McCabe, G. J., and Markstrom, S. L. (2007). A monthly water-balance model driven by a graphical user interface. United States: Geological Survey.
Medeiros, F.De, Carvalho, A. M. X., Gindri Ramos, C., Dotto, G. L., Cardoso, I. M., and Theodoro, S. H. (2024). Rock powder enhances soil nutrition and coffee quality in agroforestry systems. Sustain. For. 16,:354. doi: 10.3390/su16010354
Meybeck, M. (1982). Carbon, nitrogen, and phosphorus transport by world rivers. Am. J. Sci. U. S. 282, 401–450. doi: 10.2475/ajs.282.4.401
Meybeck, M. (2003). “5.08 - Global occurrence of major elements in rivers,” in Treatise on Geochemistry, eds. H. D. Holland and K. K. Turekian (Oxford: Pergamon), 207–223.
Moatar, F., and Meybeck, M. (2005). Compared performances of different algorithms for estimating annual nutrient loads discharged by the eutrophic river Loire. Hydrol. Process. 19, 429–444. doi: 10.1002/hyp.5541
Moon, S., Chamberlain, C. P., and Hilley, G. E. (2014). New estimates of silicate weathering rates and their uncertainties in global rivers. Geochim. Cosmochim. Acta 134, 257–274. doi: 10.1016/j.gca.2014.02.033
Mu, L., Palter, J. B., and Wang, H. (2023). Considerations for hypothetical carbon dioxide removal via alkalinity addition in the Amazon River watershed. Biogeosciences 20, 1963–1977. doi: 10.5194/bg-20-1963-2023
Négrel, P., Allègre, C. J., Dupré, B., and Lewin, E. (1993). Erosion sources determined by inversion of major and trace element ratios and strontium isotopic ratios in river water: the Congo Basin case. Earth Planet. Sci. Lett. 120, 59–76. doi: 10.1016/0012-821X(93)90023-3
Nelson, C. J., Jacobson, A. D., and Weisenberger, T. B. (2022). Controls on riverine calcium isotope ratios during basalt weathering in the Skagafjörður watershed, Iceland. Geochim. Cosmochim. Acta 333, 216–241. doi: 10.1016/j.gca.2022.07.002
Nunes, J. M. G., Kautzmann, R. M., and Oliveira, C. (2014). Evaluation of the natural fertilizing potential of basalt dust wastes from the mining district of Nova Prata (Brazil). J. Clean. Prod. 84, 649–656. doi: 10.1016/j.jclepro.2014.04.032
Oelkers, E. H., Pogge von Strandmann, P. A. E., and Mavromatis, V. (2019). The rapid resetting of the ca isotopic signatures of calcite at ambient temperature during its congruent dissolution, precipitation, and at equilibrium. Chem. Geol. 512, 1–10. doi: 10.1016/j.chemgeo.2019.02.035
Opfergelt, S., and Delmelle, P. (2012). Silicon isotopes and continental weathering processes: assessing controls on Si transfer to the ocean. Comptes Rendus Geosci. 344, 723–738. doi: 10.1016/j.crte.2012.09.006
Parkhurst, D. L. (1995). User’s guide to PHREEQC: A computer program for speciation, reaction-path, advective-transport, and inverse geochemical calculations. US Geological Survey: US Department of the Interior.
Penniston-Dorland, S., Liu, X.-M., and Rudnick, R. L. (2017). Lithium isotope geochemistry. Rev. Mineral. Geochem. 82, 165–217. doi: 10.2138/rmg.2017.82.6
Perrin, A.-S., Probst, A., and Probst, J.-L. (2008). Impact of nitrogenous fertilizers on carbonate dissolution in small agricultural catchments: implications for weathering CO2 uptake at regional and global scales. Geochim. Cosmochim. Acta 72, 3105–3123. doi: 10.1016/j.gca.2008.04.011
Peters, S. C., Blum, J. D., Driscoll, C. T., and Likens, G. E. (2004). Dissolution of wollastonite during the experimental manipulation of Hubbard brook watershed 1. Biogeochemistry 67, 309–329. doi: 10.1023/B:BIOG.0000015787.44175.3f
Pistiner, J. S., and Henderson, G. M. (2003). Lithium-isotope fractionation during continental weathering processes. Earth Planet. Sci. Lett. 214, 327–339. doi: 10.1016/S0012-821X(03)00348-0
Pogge von Strandmann, P. A. E., Fraser, W. T., Hammond, S. J., Tarbuck, G., Wood, I. G., Oelkers, E. H., et al. (2019). Experimental determination of Li isotope behaviour during basalt weathering. Chem. Geol. 517, 34–43. doi: 10.1016/j.chemgeo.2019.04.020
Pogge von Strandmann, P. A. E., Renforth, P., West, A. J., Murphy, M. J., Luu, T.-H., and Henderson, G. M. (2021). The lithium and magnesium isotope signature of olivine dissolution in soil experiments. Chem. Geol. 560:120008. doi: 10.1016/j.chemgeo.2020.120008
Puro. earth . (2022). Enhanced Rock Weathering Methodology. Available at: https://carbon.puro.earth/enhanced-rock-weathering.
Puro.earth . (2022). Enhanced Rock Weathering Methodology. Available at: https://7518557.fs1.hubspotusercontent-na1.net/hubfs/7518557/Supplier%20Documents/ERW%20methodology.pdf (Accessed October 26, 2023).
Ramos, C. G., Hower, J. C., Blanco, E., Oliveira, M. L. S., and Theodoro, S. H. (2022). Possibilities of using silicate rock powder: an overview. Geosci. Front. 13:101185. doi: 10.1016/j.gsf.2021.101185
Ramos, E. J., Larsen, W. J., Hou, Y., Muñoz, S., Kemeny, P. C., Scheingross, J. S., et al. (2024). Competition or collaboration: clay formation sets the relationship between silicate weathering and organic carbon burial in soil. Earth Planet. Sci. Lett. 628:118584. doi: 10.1016/j.epsl.2024.118584
Rausis, K., Stubbs, A. R., Power, I. M., and Paulo, C. (2022). Rates of atmospheric CO2 capture using magnesium oxide powder. Int. J. Greenh. Gas Control 119:103701. doi: 10.1016/j.ijggc.2022.103701
Raymond, P. A., Oh, N.-H., Turner, R. E., and Broussard, W. (2008). Anthropogenically enhanced fluxes of water and carbon from the Mississippi River. Nature 451, 449–452. doi: 10.1038/nature06505
Reershemius, T., Kelland, M. E., Jordan, J. S., Davis, I. R., D’Ascanio, R., Kalderon-Asael, B., et al. (2023). Initial validation of a soil-based mass-balance approach for empirical monitoring of enhanced rock weathering rates. Environ. Sci. Technol. 57, 19497–19507. doi: 10.1021/acs.est.3c03609
Reershemius, T., and Suhrhoff, T. J. (2024). On error, uncertainty, and assumptions in calculating carbon dioxide removal rates by enhanced rock weathering in Kantola et al., 2023. Glob. Change Biol. Bioenergy. 30:e17025. doi: 10.1111/gcb.17025
Reiman, J. H., and Xu, Y. J. (2019). Dissolved carbon export and CO2 outgassing from the lower Mississippi River–implications of future river carbon fluxes. J. Hydrol. 578:124093. doi: 10.1016/j.jhydrol.2019.124093
Relph, K. E., Stevenson, E. I., Turchyn, A. V., Antler, G., Bickle, M. J., Baronas, J. J., et al. (2021). Partitioning riverine sulfate sources using oxygen and sulfur isotopes: implications for carbon budgets of large rivers. Earth Planet. Sci. Lett. 567:116957. doi: 10.1016/j.epsl.2021.116957
Renforth, P. (2012). The potential of enhanced weathering in the UK. Int. J. Greenh. Gas Control 10, 229–243. doi: 10.1016/j.ijggc.2012.06.011
Renforth, P. (2019). The negative emission potential of alkaline materials. Nat. Commun. 10:1401. doi: 10.1038/s41467-019-09475-5
Renforth, P., and Campbell, J. S. (2021). The role of soils in the regulation of ocean acidification. Philos. Trans. R. Soc. B Biol. Sci. 376:20200174. doi: 10.1098/rstb.2020.0174
Renforth, P., and Henderson, G. (2017). Assessing Ocean alkalinity for carbon sequestration. Rev. Geophys. 55, 636–674. doi: 10.1002/2016RG000533
Renforth, P., Manning, D. A. C., and Lopez-Capel, E. (2009). Carbonate precipitation in artificial soils as a sink for atmospheric carbon dioxide. Appl. Geochem. 24, 1757–1764. doi: 10.1016/j.apgeochem.2009.05.005
Renforth, P., Pogge von Strandmann, P. A. E., and Henderson, G. M. (2015). The dissolution of olivine added to soil: implications for enhanced weathering. Appl. Geochem. 61, 109–118. doi: 10.1016/j.apgeochem.2015.05.016
Rieder, L., Amann, T., and Hartmann, J. (2024). Soil electrical conductivity as a proxy for enhanced weathering in soils. Front. Clim. 5:107. doi: 10.3389/fclim.2023.1283107
Rousset, C., Brefort, H., Arkoun, M., Mathieu, O., and Hénault, C. (2023). The reducing effect of aglime on N2O and CO2 emissions balance from an acidic soil: a study on intact soil cores. Eur. J. Soil Sci. 74:e13367. doi: 10.1111/ejss.13367
Santos, R. M., Araujo, F., Jariwala, H., Khalidy, R., Haque, F., and Chiang, Y. W. (2023). Pathways, roundabouts, roadblocks, and shortcuts to safe and sustainable deployment of enhanced rock weathering in agriculture. Front. Earth Sci. 11:15930. doi: 10.3389/feart.2023.1215930
Sanz Scovino, J. I., and Rowell, D. L. (1988). The use of feldspars as potassium fertilizers in the savannah of Colombia. Fertil. Res. 17, 71–83. doi: 10.1007/BF01050458
Schuiling, R. D., and Krijgsman, P. (2006). Enhanced weathering: an effective and cheap tool to sequester Co2. Clim. Chang. 74, 349–354. doi: 10.1007/s10584-005-3485-y
Schuiling, R. D., Wilson, S., and Power, L. M. (2011). Enhanced silicate weathering is not limited by silicic acid saturation. Proc. Natl. Acad. Sci. 108:E41. doi: 10.1073/pnas.1019024108
Schulte, P., Van Geldern, R., Freitag, H., Karim, A., Négrel, P., Petelet-Giraud, E., et al. (2011). Applications of stable water and carbon isotopes in watershed research: weathering, carbon cycling, and water balances. Earth-Sci. Rev. 109, 20–31. doi: 10.1016/j.earscirev.2011.07.003
Shao, S., Driscoll, C. T., Johnson, C. E., Fahey, T. J., Battles, J. J., and Blum, J. D. (2015). Long-term responses in soil solution and stream-water chemistry at Hubbard brook after experimental addition of wollastonite. Environ. Chem. 13, 528–540. doi: 10.1071/EN15113
Skov, K., Wardman, J., Healey, M., McBride, A., Bierowiec, T., Cooper, J., et al. (2024). Initial agronomic benefits of enhanced weathering using basalt: a study of spring oat in a temperate climate. PLoS One 19:e0295031. doi: 10.1371/journal.pone.0295031
Slessarev, E. W., Chadwick, O. A., Sokol, N. W., Nuccio, E. E., and Pett-Ridge, J. (2022). Rock weathering controls the potential for soil carbon storage at a continental scale. Biogeochemistry 157, 1–13. doi: 10.1007/s10533-021-00859-8
Smith, S. M., Geden, O., Nemet, G. F., Gidden, M. J., Lamb, W. F., Powis, C., et al. (2023). The state of carbon dioxide removal. The State of Carbon Dioxide Removal. 1st Edn.
Smith, P., Lanigan, G., Kutsch, W. L., Buchmann, N., Eugster, W., Aubinet, M., et al. (2010). Measurements necessary for assessing the net ecosystem carbon budget of croplands. Agric. Ecosyst. Environ. 139, 302–315. doi: 10.1016/j.agee.2010.04.004
Steinour, H. H. (1959). Some effects of carbon dioxide on mortars and concrete-discussion. J. Am. Concr. Inst 30, 905–907.
Stets, E. G., Kelly, V. J., and Crawford, C. G. (2014). Long-term trends in alkalinity in large rivers of the conterminous US in relation to acidification, agriculture, and hydrologic modification. Sci. Total Environ. 488–489, 280–289. doi: 10.1016/j.scitotenv.2014.04.054
Stubbs, A. R., Paulo, C., Power, I. M., Wang, B., Zeyen, N., and Wilson, S. (2022). Direct measurement of CO2 drawdown in mine wastes and rock powders: implications for enhanced rock weathering. Int. J. Greenh. Gas Control 113:103554. doi: 10.1016/j.ijggc.2021.103554
Stubbs, A. R., Power, I. M., Paulo, C., Wang, B., Zeyen, N., Wilson, S., et al. (2023). Impact of wet-dry cycles on enhanced rock weathering of brucite, wollastonite, serpentinite and kimberlite: implications for carbon verification. Chem. Geol. 637:121674. doi: 10.1016/j.chemgeo.2023.121674
Stumm, W., and Morgan, J. J. (1996). Aquatic chemistry: Chemical equilibria and rates in natural waters. 3rd Edn. New York: Wiley.
Suhrhoff, T. J., Reershemius, T., Wang, J., Jordan, J. S., Reinhard, C. T., and Planavsky, N. J. (2024). A tool for assessing the sensitivity of soil-based approaches for quantifying enhanced weathering: a US case study. Front. Clim. 6:1346117. doi: 10.3389/fclim.2024.1346117
Suhrhoff, T. J., Rickli, J., Christl, M., Vologina, E. G., Pham, V., Belhadj, M., et al. (2022). Source to sink analysis of weathering fluxes in Lake Baikal and its watershed based on riverine fluxes, elemental lake budgets, REE patterns, and radiogenic (Nd, Sr) and 10Be/9Be isotopes. Geochim. Cosmochim. Acta 321, 133–154. doi: 10.1016/j.gca.2022.01.007
Sutherland, K., Holme, E., Savage, R., Gill, S., Matlin-Wainer, M., He, J., et al. (2024). Enhanced weathering in agriculture v1.0–isometric. Available at: https://registry.isometric.com/protocol/enhanced-weathering-agriculture (Accessed April 18, 2024).
Swoboda, P., Döring, T. F., and Hamer, M. (2022). Remineralizing soils? The agricultural usage of silicate rock powders: a review. Sci. Total Environ. 807:150976. doi: 10.1016/j.scitotenv.2021.150976
Swoboda, P., Hamer, M., Stotter, M., Döring, T. F., and Trimborn, M. (2021). Effects of rock powder additions to cattle slurry on Ammonia and greenhouse gas emissions. Atmos. 12:1652. doi: 10.3390/atmos12121652
Taylor, L. L., Driscoll, C. T., Groffman, P. M., Rau, G. H., Blum, J. D., and Beerling, D. J. (2021). Increased carbon capture by a silicate-treated forested watershed affected by acid deposition. Biogeosciences 18, 169–188. doi: 10.5194/bg-18-169-2021
Taylor, L. L., Quirk, J., Thorley, R. M. S., Kharecha, P. A., Hansen, J., Ridgwell, A., et al. (2016). Enhanced weathering strategies for stabilizing climate and averting ocean acidification. Nat. Clim. Chang. 6, 402–406. doi: 10.1038/nclimate2882
Te Pas, E. E. E., Hagens, M., and Comans, R. N. J. (2023). Assessment of the enhanced weathering potential of different silicate minerals to improve soil quality and sequester CO2. Front. Clim. 4:4064. doi: 10.3389/fclim.2022.954064
Teng, F.-Z., Dauphas, N., and Watkins, J. M. (2017). Non-traditional stable isotopes: retrospective and prospective. Rev. Mineral. Geochem. 82, 1–26. doi: 10.2138/rmg.2017.82.1
Tessier, A., Campbell, P. G., and Bisson, M. (1979). Sequential extraction procedure for the speciation of particulate trace metals. Anal. Chem. 51, 844–851. doi: 10.1021/ac50043a017
Thomas, M., and Reid, R. (2021). Vacuolar compartmentalisation and efflux of cadmium in barley. Botany 99, 1–8. doi: 10.1139/cjb-2020-0080
USGS . (2019). National Field Manual for the collection of water-quality data (NFM) US. Geological Survey. Available at: https://www.usgs.gov/mission-areas/water-resources/science/national-field-manual-collection-water-quality-data-nfm (Accessed October 27, 2023).
Van Straaten, P. (2007). Agrogeology: the use of rocks for crops. Available at: https://agris.fao.org/search/en/providers/122621/records/6473965f68b4c299a3fb47e6 (Accessed March 11, 2024).
Vandeginste, V., Lim, C., and Ji, Y. (2024). Exploratory review on environmental aspects of enhanced weathering as a carbon dioxide removal method. Fortschr. Mineral. 14:75. doi: 10.3390/min14010075
Vicca, S., Goll, D. S., Hagens, M., Hartmann, J., Janssens, I. A., Neubeck, A., et al. (2022). Is the climate change mitigation effect of enhanced silicate weathering governed by biological processes? Glob. Change Biol. 28, 711–726. doi: 10.1111/gcb.15993
Vienne, A., Frings, P., Poblador, S., Steinwidder, L., Rijnders, J., Schoelynck, J., et al. (2023). Soil carbon sequestration and the role of earthworms in an enhanced weathering Mesocosm experiment, No. 4449286.
Vienne, A., Poblador, S., Portillo-Estrada, M., Hartmann, J., Ijiehon, S., Wade, P., et al. (2022). Enhanced weathering using basalt rock powder: carbon sequestration, co-benefits and risks in a Mesocosm study with Solanum tuberosum. Front. Clim. 4:869456. doi: 10.3389/fclim.2022.869456
Viers, J., Oliva, P., Dandurand, J.-L., Dupré, B., and Gaillardet, J. (2007). “5.20 - chemical weathering rates, CO2 consumption, and control parameters deduced from the chemical composition of Rivers” in Treatise on geochemistry. eds. H. D. Holland and K. K. Turekian (Oxford: Pergamon), 1–25.
Vink, J. P. M., Giesen, D., and Ahlrichs, E. (2022). Olivine weathering in field trials effect of natural environmental conditions on mineral dissolution and the potential toxicity of nickel.
Vogel, J. C. (1993). “4 - variability of carbon isotope fractionation during photosynthesis” in Stable isotopes and plant carbon-water relations. eds. J. R. Ehleringer, A. E. Hall, and G. D. Farquhar (San Diego: Academic Press), 29–46.
Walker, J. C. G., Hays, P. B., and Kasting, J. F. (1981). A negative feedback mechanism for the long-term stabilization of Earth’s surface temperature. J. Geophys. Res. Oceans 86, 9776–9782. doi: 10.1029/JC086iC10p09776
Wang, F., Zhu, F., Liu, D., Qu, Y., Liu, D., Xie, J., et al. (2024). Wollastonite powder application increases rice yield and CO2 sequestration in a paddy field in Northeast China. Plant Soil 2024:6570. doi: 10.1007/s11104-024-06570-5
Weil, R., and Brady, N. (2017). The nature and properties of soils. 15th Edn. Upper Saddle River NJ: Pearson Press.
Wen, H., Sullivan, P. L., Billings, S. A., Ajami, H., Cueva, A., Flores, A., et al. (2022). From soils to streams: connecting terrestrial carbon transformation, chemical weathering, and solute export across hydrological regimes. Water Resour. Res. 58:e2022WR032314. doi: 10.1029/2022WR032314
West, A. J., Galy, A., and Bickle, M. (2005). Tectonic and climatic controls on silicate weathering. Earth Planet. Sci. Lett. 235, 211–228. doi: 10.1016/j.epsl.2005.03.020
West, T. O., and McBride, A. C. (2005). The contribution of agricultural lime to carbon dioxide emissions in the United States: dissolution, transport, and net emissions. Agric. Ecosyst. Environ. 108, 145–154. doi: 10.1016/j.agee.2005.01.002
Whitworth, T. M. (1998). “Clay minerals: ion exchangeIon exchange” in Geochemistry. ed. T. M. Whitworth (Dordrecht: Springer Netherlands), 85–87.
Wolf, A., Chang, E., Kantola, I. B., Blanc-Betes, E., Masters, M. D., Marklein, A., et al. (2024). Validating assumptions in calculating carbon dioxide removal by enhanced rock weathering in Kantola et al., 2023. Glob. Chang. Biol. 30:e17031. doi: 10.1111/gcb.17031
Wolf, A., Chang, E., and Tank, A. (2023). Verification methods and agronomic enhancements for carbon removal based on enhanced rock weathering. Available at: https://image-ppubs.uspto.gov/dirsearch-public/print/downloadPdf/11644454 (Accessed October 23, 2023).
Wolf-Gladrow, D. A., Zeebe, R. E., Klaas, C., Körtzinger, A., and Dickson, A. G. (2007). Total alkalinity: the explicit conservative expression and its application to biogeochemical processes. Mar. Chem. 106, 287–300. doi: 10.1016/j.marchem.2007.01.006
Wood, C., Harrison, A. L., and Power, I. M. (2023). Impacts of dissolved phosphorus and soil-mineral-fluid interactions on CO2 removal through enhanced weathering of wollastonite in soils. Appl. Geochem. 148:105511. doi: 10.1016/j.apgeochem.2022.105511
Yan, Y., Dong, X., Li, R., Zhang, Y., Yan, S., Guan, X., et al. (2023). Wollastonite addition stimulates soil organic carbon mineralization: evidences from 12 land-use types in subtropical China. Catena 225:107031. doi: 10.1016/j.catena.2023.107031
Zamanian, K., Pustovoytov, K., and Kuzyakov, Y. (2016). Pedogenic carbonates: forms and formation processes. Earth-Sci. Rev. 157, 1–17. doi: 10.1016/j.earscirev.2016.03.003
Zhang, B., Kroeger, J., Planavsky, N., and Yao, Y. (2023). Techno-economic and life cycle assessment of enhanced rock weathering: a case study from the Midwestern United States. Environ. Sci. Technol. 57, 13828–13837. doi: 10.1021/acs.est.3c01658
Zhang, S., Planavsky, N. J., Katchinoff, J., Raymond, P. A., Kanzaki, Y., Reershemius, T., et al. (2022). River chemistry constraints on the carbon capture potential of surficial enhanced rock weathering. Limnol. Oceanogr. 67, S148–S157. doi: 10.1002/lno.12244
Keywords: enhanced silicate weathering, negative emissions technologies, climate change mitigation, carbon mineralization, MRV
Citation: Clarkson MO, Larkin CS, Swoboda P, Reershemius T, Suhrhoff TJ, Maesano CN and Campbell JS (2024) A review of measurement for quantification of carbon dioxide removal by enhanced weathering in soil. Front. Clim. 6:1345224. doi: 10.3389/fclim.2024.1345224
Received: 27 November 2023; Accepted: 27 May 2024;
Published: 19 June 2024.
Edited by:
Wil Burns, Institute for Carbon Removal Law and Policy, American University, United StatesReviewed by:
Andrew Jacobson, Northwestern University, United StatesCopyright © 2024 Clarkson, Larkin, Swoboda, Reershemius, Suhrhoff, Maesano and Campbell. This is an open-access article distributed under the terms of the Creative Commons Attribution License (CC BY). The use, distribution or reproduction in other forums is permitted, provided the original author(s) and the copyright owner(s) are credited and that the original publication in this journal is cited, in accordance with accepted academic practice. No use, distribution or reproduction is permitted which does not comply with these terms.
*Correspondence: Matthew O. Clarkson, bWF0dGhldy5jbGFya3NvbkBpbnBsYW5ldC5lYXJ0aA==
Disclaimer: All claims expressed in this article are solely those of the authors and do not necessarily represent those of their affiliated organizations, or those of the publisher, the editors and the reviewers. Any product that may be evaluated in this article or claim that may be made by its manufacturer is not guaranteed or endorsed by the publisher.
Research integrity at Frontiers
Learn more about the work of our research integrity team to safeguard the quality of each article we publish.