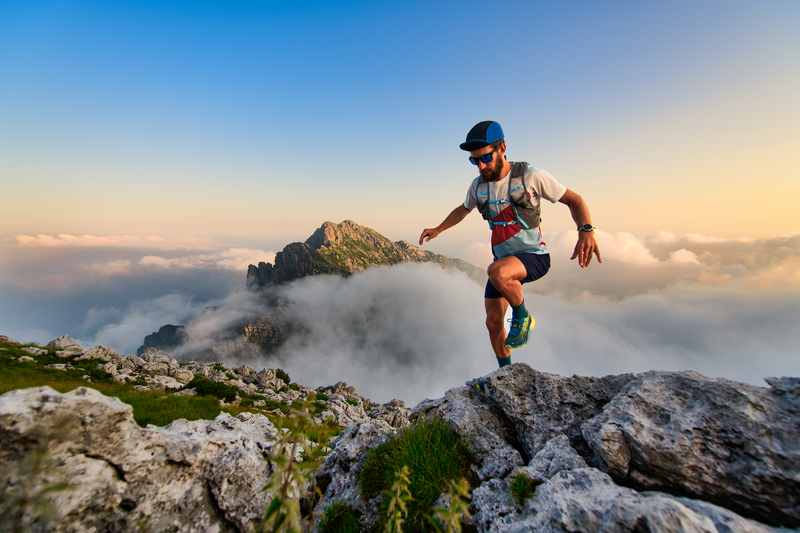
95% of researchers rate our articles as excellent or good
Learn more about the work of our research integrity team to safeguard the quality of each article we publish.
Find out more
ORIGINAL RESEARCH article
Front. Clim. , 20 May 2024
Sec. Carbon Dioxide Removal
Volume 6 - 2024 | https://doi.org/10.3389/fclim.2024.1338647
This article is part of the Research Topic Carbon dioxide removal: Perspectives from the social sciences and humanities View all 13 articles
Carbon dioxide removal (CDR) technologies, such as direct air carbon capture and storage (DACCS), will be critical in limiting the rise of the average global temperature over the next century. Scaling up DACCS technologies requires the support of a complex array of policies and infrastructure across multiple overlapping policy areas, such as climate, energy, technology innovation and resource management. While the literature on DACCS and other CDR technologies acknowledges the path-dependent nature of policy development, it has tended to focus on abstract policy prescriptions that are not rooted in the specific political, social and physical (infrastructural) context of the implementing state. To address this gap, this paper provides a country-level study of the emerging DACCS policy regime in Canada. Drawing on the existing literature that identifies idealized (acontextual) policy objectives that support DACCS development and effective regulation, we identify the actionable policy objectives across six issue domains: general climate mitigation strategies; energy and resource constraints; carbon storage and transport regulation and infrastructure; financing scale-up and supporting innovation; removal and capture technology availability and regulation; and addressing social acceptability and public interest. Using a database of Canadian climate policies (n = 457), we identify policies within the Canadian (federal and provincial) policy environment that map to the idealized policy objectives within each of these domains. This exercise allows us to analyze how key policy objectives for DACCS development are represented within the Canadian system, and enables us to identify potential niches, and landscape influences within the system, as well as gaps and potential barriers to the system transition process. This paper contributes to our understanding of national DACCS policy development by providing a framework for identifying components of the DAC system and linking those components to desired policy outcomes and may provide a basis for future cross-country comparisons of national-level DACCS policy.
As countries prepare to meet their Paris Agreement commitments, there is an increasing recognition that carbon dioxide removal technologies will play a central role in limiting the rise of average global temperatures over the next century. Among these technologies, direct air carbon capture and storage (DACCS)1 has the potential to make a significant contribution to state-level carbon management strategies. The scaling up of DACCS to meaningful levels will require states to develop a complex array of policies across multiple domains to support the infrastructural, technological, environmental and social demands of DACCS deployment. To date, much of the literature that seeks to examine these policy requirements has done so in an abstract manner, with less attention being paid to the existing policy frameworks with specific states. However, it is widely acknowledged that policies supportive of carbon dioxide removal (CDR) will be built on the foundations of existing policies and will be influenced by the structure and content of this policy landscape (Cox and Edwards, 2019; Creutzig et al., 2019; Fajardy et al., 2019; Schenuit et al., 2021; Craik et al., 2022). To address this deficit, this paper provides a country-level study – in this case Canada – that identifies and characterizes the existing policy foundations for developing and deploying DACCS. By mapping out the existing DACCS-relevant policy framework, we can identify policy gaps and better understand the potential trajectories or policy pathways that Canada can pursue in the context of its transition to net-zero emissions.
This study is underlain by several conditions that characterize the DACCS policy environment. Foremost, the policy environment is best understood as part of a complex, dynamic system. DACCS deployment relies on other large sub-systems, particularly non-emitting energy systems, but also large (gigatonne) scale storage, pipelines, and climate mitigation structures, such as carbon pricing (Minx et al., 2018; Erans et al., 2022). Since these systems interact and co-evolve with DACCS, DACCS development and deployment will be structured by policy decisions made in these domains and the relationships and barriers in current policies and infrastructure may enable or impede DACCS-related goals. The long lead times associated with DACCS deployment and related systems place pressure on existing policy frameworks since near-term decisions will have longer term, downstream implications. For example, decisions that Canada makes in the next 5 years regarding energy systems and geological storage may not foreground DACCS, but will nonetheless shape DACCS in the years and decades to come (O’Riordan, 2018; Craik et al., 2022). The embeddedness of DACCS extends to the social and political aspects of the system with implications on the acceptability of the technology, which may be influenced by perceptions of the distribution of future benefits and burdens from DACCS and associated sub-systems.
Since DACCS siting and scale-up are context-specific and have a high potential to be influenced by path dependencies within a system, it is important to conduct country-level case studies to assess the prospective technology development pathways, particularly as states begin to name negative emissions technologies (NETs) in their near and long-term climate mitigation policy plans (Schenuit et al., 2021; IEA, 2022). The existing physical infrastructure (pipeline networks, storage sites, energy, and DAC facilities) and policy infrastructure (regulations, carbon taxes, and other incentives and plans) will direct how states make policy and plans in light of path dependencies because they may impede the availability of alternative options and policy pathways in future decisions (Asayama, 2021).
Canada offers an informative case study for several reasons. Canada has one the highest per capita emissions rates globally and is already warming at twice the average global rate (Warren and Lulham, 2021; World Resources Institute, 2021). The Canadian government has set an ambitious mitigation target of reaching net-zero by 2050, and has established a robust policy framework, including an accelerating carbon price in support of this goal (Environment and Climate Change Canada, 2022). Canada, and certain Canadian provinces, have been active in the development and deployment of carbon capture and storage (CCS), and as such have advanced CCS regulatory and policy frameworks (Bankes, 2019). Canada also has a high potential for wealth loss associated with stranded fossil fuel assets over the course of the low carbon transition, which scaling up CCS infrastructure may help to reduce. According to a study by Marcucci et al. (2017), the higher marginal costs associated with producing oil and gas over the next few decades may eliminate the industry in countries like Canada and United States Canada not only needs to decarbonize for climate reasons, decarbonization is also an economic imperative. Finally, Canada has growing technological capacity to research and develop DAC technologies, which include innovation support and financial incentives to pursue DACCS, and has identified DACCS as an important element of future mitigation pathways (Canada Energy Regulator, 2023; Natural Resources Canada, 2023).
To address these conditions and conceptualize DACCS as a coordinated system of technologies and policies integrated within the larger socio-technical system, we adopt the Multi-Level Perspective (MLP) (Geels, 2002, 2004) as a framework for understanding the drivers at play within systems undergoing socio-technical transitions. The MLP is well-suited for the purposes of this study because it provides us with a basis to identify the different components of a socio-technical system and theorize how they interact with one another to shape economic, political, and social outcomes, particularly as they destabilize and restabilize the system in response to internal and external pressures during the transition process.
Our study followed a two-step approach. First, we identified actionable objectives for policy identified in the literature on DACCS and CDR. Then, we applied the objectives to the Canadian policy context by examining a database of 457 federal and provincial climate policies and mapped relevant policies onto 6 domains of policy relevant for supporting DACCS. The goal in this step was to identify where the idealized policy objectives are represented within the Canadian policy landscape. The results provide a picture of the existing DACCS policy environment in Canada, which we then interpret and analyze, drawing on insights from the MLP, but also drawing on the existing DACCS policy literature. In the second stage, our analysis centers on three dimensions of policy analysis: we identify policy gaps, areas where policy needs to be tailored to CDR or extended across the country, and interactions between regime policies and federal and provincial strategies.
The second section of this paper provides the context for the study. We describe the role that DACCS may play in future climate mitigation pathways, and the components of the technology and its system demands, its relationship to the wider physical, economic, social and political components of the socio-technical system in which DACCS is being developed in Canada. The third section of the paper describes the two-step methodology we employ to identify the components and structure of the existing policy framework for DACCS development and deployment in Canada. The results of this study are contained in section 4, which outlines the key findings from our analysis of the DACCS policy framework in Canada. In section five, we return to the MLP to structure our discussion of the broader structural implications of the study. Here we identify those elements of the system that are critical to the scaling-up of DACCS, and the extent to which DACCS has destabilizing and transformative potential. In the final section of the paper, we conclude with a discussion on the potential application of this study approach in other national contexts and situate the study within the existing descriptive and prescriptive literature on DACCS policy formation.
DACCS consists of a group of technologies that filter carbon dioxide from the ambient air through various chemical processes, after which captured carbon is either permanently stored in natural geological formations or sold for reuse in industrial activities. DAC, as a process, is energy-intensive, which means that, to be an efficient means of CDR, facilities should use a non-CO₂ emitting energy supply. There are variations of DAC technologies using different forms of chemical processes (solid sorbents and liquid solvents) that have different energy and heat input requirements (Barahimi et al., 2023). Other inputs into the capture process include heat, water and chemical materials (Realmonte et al., 2019). The other central components of the system consist of the geological storage sites or utilization processes and the means to transport the captured carbon to these sites (Nemet et al., 2018; Keleman et al., 2019). Since DACCS does not rely on point source emissions, there is greater flexibility in locating the capture facility close to renewable energy sources or storage sites, potentially reducing the need to transport energy supplies or the captured carbon, or both.
Along with other forms of CDR, the role of DACCS in achieving long-term climate objectives is to offset hard-to-abate emissions and to draw down atmospheric CO₂ to lower the overall concentration of CO2 to levels consistent with the Paris Agreement targets (IPCC, 2022). Some forms of CDR may act as a substitute for emissions reduction and may be preferred where the costs of CDR are lower than other forms of mitigation. The current estimated cost of DACCS ranges from 250 USD to upwards of 600 USD, though DACCS companies and the Sixth Assessment Report (AR6) expect costs to fall within the next decade (~100–300 USD) as new facilities are built and undertake large-scale removal (IPCC, 2022; Lebling et al., 2022). Relative to the high cost of DACCS, even high abatement costs or carbon prices may not be enough to incentivize purchases of CDR, therefore it is less likely that DACCS will play a significant role in the near term as a substitute to emissions reduction (Chen and Tavoni, 2013; McQueen et al., 2021; Erans et al., 2022). In the long-term CDR needs to be used to deliver net-negative emissions, not just as a means to offset high emissions industries. Despite the high relative costs, there are several characteristics of DACCS that provide advantages over other forms of CDR. Unlike forms of CDR that use biological sequestration, geological storage of CO2 is stable with high permanence and presents fewer accounting and verification challenges (Beuttler et al., 2019). DACCS has lower land requirements than other forms of CDR, such as bioenergy with carbon capture and storage (BECCS), and may, as a result, place less pressure on agricultural systems (Smith et al., 2016). Some DACCS technologies nevertheless have significant demand for water and the chemicals used in sorbent materials, but its greatest sustainability challenge is the energy required to remove CO2 from the air, in which the concentration of CO2 is relatively low (Realmonte et al., 2019).
In AR6, the IPCC identifies the range of cumulative CDR deployment from 2020 to 2,100 within modeled pathways that limit warming to 1.5°C with no or limited overshoot from 20 to 660 GtCO₂ (IPCC, 2022). Within that bundle of CDR, the range of cumulative DACCS deployment for 1.5°C and 2°C is 0–310 GTCO2 and 0–250 GTCO2, respectively (IPCC, 2022). Motlaghzadeh et al. (2023), looking more closely at the scenarios within the AR6 database that include DACCS, indicate that annual DACCS deployment in the year 2,100 has significant variation among scenarios, ranging from 0 to 18.9 GTCO2/yr.
The significant uncertainty around the amount of DACCS at both global and national scales is driven by a variety of factors including assumptions regarding broader socio-economic pathways, future cost of DACCS, the degree of temperature overshoot (beyond 1.5° C and 2°C) that would be tolerated, and limits on competing forms of CDR, namely BECCS (Motlaghzadeh et al., 2023). Many national characteristics such as physical suitability (pore space access), availability of low carbon energy, financial and technological capacities and the degree of social and political acceptability of DACCS deployment are not represented in the IPCC scenarios but have been identified as important influences on DACCS development and deployment (Ocean Studies Board and National Research Council, 2015; Buck, 2016; Honegger et al., 2021; Erans et al., 2022; Satterfield et al., 2023).
Recognizing the importance of policy decisions and practices to shape these influences, the existing literature on DACCS policy requirements has focused on interventions that address these key infrastructural, environmental, economic and social conditions (Marcucci et al., 2017; Beuttler et al., 2019; Fajardy et al., 2019; Honegger et al., 2021; Meckling and Biber, 2021). In their article outlining the barriers to CDR, Honegger et al. (2021) claim that the ideal CDR policy mix for overcoming this gap should: “(i) clarify the intended role of CDR, (ii) accelerate innovation to reduce cost barriers, (iii) ensure public participation in the process of mobilizing NETs, (iv) promote long-term, rather than pilot projects for technologies, (v) have robust carbon reporting and accounting procedures, (vi) prevent side effects and maximize the co-benefits of carbon removal” (pp. 5–6). In effect, these recommendations seek to close the current incentive gap by encouraging policymakers to directly support innovation financially and to introduce measures to ensure transparency and diffusion of information on CDR throughout society, thereby reducing uncertainty in the system.
Sovacool et al. (2022), drawing on a large number (n = 125) of expert interviews identify a set of 10 DAC-specific policy recommendations. In line with earlier studies, Sovacool et al. emphasize the importance of using DACCS to lower the stock of atmospheric emissions, not as a substitute for reducing emissions and climate adaptation (see also Beuttler et al., 2019; McLaren et al., 2019; Erans et al., 2022), as well as the need to prioritize long term storage and to leverage emerging carbon capture and storage infrastructure. The social dimensions of DACCS have received increasing attention from both instrumental (acceptability) and non-instrumental (justice and legitimacy) perspectives, with recommendations directed toward procedural conditions, such as transparency and stakeholder participation, and substantive outcomes, such as the distribution of benefits and impacts of DACCS (Buck, 2016; Pozo et al., 2020; Sovacool et al., 2022; Satterfield et al., 2023). Overall, the literature underlines the importance of introducing policy instruments to create incentives for investing and scaling up DACCS, such as carbon pricing; decarbonization policy; CDR-specific targets and tax credits; research and development subsidies for NETs and carbon utilization; carbon offset and trading protocols; and clear regulations around carbon storage and transport (Creutzig et al., 2019; Fajardy et al., 2019; McLaren et al., 2019; Honegger et al., 2021; Meckling and Biber, 2021).
Of significance to the current study is that the DACCS policy literature identifies the embeddedness of DACCS systems within a wider system that is itself undergoing a significant low-carbon socio-technical transition. The key physical linkages are between DACCS and the energy system – specifically, the scale-up of non-emitting energy sources – and carbon storage and transportation systems. Likewise, much of the supporting policy environment, such as carbon pricing, innovation support and environmental information and public participation procedures, are part of an existing and evolving policy environment (Cox and Edwards, 2019). While the literature on recommended DACCS policy draws on examples of specific domestic policy instruments, there have been few studies that have sought to identify the elements and contours of domestic DACCS policy frameworks in a comprehensive fashion (but see Schenuit et al., 2021). General, idealized policy recommendations are an important and necessary step in understanding the requirements of the policy framework for DACCS development and deployment, but do not address the social, political and institutional conditions within a specific domestic context.
We share the view of Schenuit et al. (2021) that MLP provides a useful heuristic to structure and make sense of net-zero transitions and the role of CDR policy within those transitions. The MLP distinguishes the three ‘levels’ that make up the system and determine socio-technical pathways. The first is the existing regime, which encompasses the practices and associated rules that maintain the existing system (Geels, 2011). This includes culture and symbolic meaning, markets and user practice, techno-scientific knowledge, technology, infrastructure, industrial networks and strategic games, and sectoral policy (Geels, 2002). The existing system is subject to destabilization from exogenous “landscape” factors, such as “material and environmental conditions, external agents, [and the] larger socio-cultural context” (Geels, 2004, p. 908). In addition, niche innovations can gain traction and progressively shift, or else radically reconstruct, the architecture of the system, particularly where landscape conditions simultaneously destabilize a regime.
If we accept that DACCS systems will develop, not in isolation, but rather as part of an existing socio-technical system, then a key characteristic of such systems is that they will tend to exhibit high degrees of path dependence (Geels, 2019). Policies that are supportive of DACCS development will be influenced by the existing policy framework, which will reflect the preferences of dominant actors and institutions and will be responsive to the infrastructural and social conditions of the system (Cox and Edwards, 2019). These conditions will be context specific, which indicates the importance of understanding the policy framework at domestic levels since abstract policy recommendations may face “fit” issues within specific domestic contexts (Young, 2002).
In addition to identifying the path dependent nature of dominant technologies and the policies supporting those technologies, MLP provides a theory of change through the incorporation of insights from evolutionary economics that highlight the adaptive, progressive changes in material production and consumption that can align with or impose change on the dominant technological regime. In either case, evolutionary economics understands that successful niche technologies fulfill a functional role within the system by interacting and synergizing with existing technologies and institutions (Boulding, 1991; Geels, 2002; Cecere et al., 2014).
The interdependencies between economic, social and political forces are central to MLP dynamics, and lead to examinations of the way in which these often path dependent interactions result in “lock-in” and more specifically, “carbon lock-in” (Unruh, 2000; Cecere et al., 2014). In this regard, DACCS provides an interesting example, as aspects of DACCS, such as the development of a robust carbon storage and transport infrastructure or the potential role of DACCS as a substitute for emissions reduction, are viewed by many as being supportive of the dominant – fossil-fuel oriented – regime, and as such, may contribute to carbon lock-in (Markusson and Haszeldine, 2009; Shackley and Thompson, 2012; Cairns, 2014). However, DACCS is also a critical component of the transition to net zero (Asayama, 2021). Asayama argues, and we agree, that the dilemma that CDR poses in connection with carbon lock-in is not inherent to the technologies, and can, therefore be shaped through policy choices.
The complex-systems ontology that the MLP adopts is well-suited to an analysis of DACCS’ roles in the incumbent (fossil-fueled) or emergent (net-zero) regimes, since MLP favors non-linear, multi-factor causal explanations. DACCS development, because it is so deeply interconnected to other major systems, which interact with one another, is not likely going to be satisfactorily understood with reference to a select number of drivers. This does not, however, suggest that we cannot identify the principal sub-regimes or domains that are relevant to DACCS. Geels notes that “for sustainability transitions, it may be fruitful to pay more attention to multi-regime interactions” (2011, p. 32). In the case of DACCS, its development and deployment will be a product of interactions across energy, climate, and geological storage systems, each of which has constellations of policies that shape both the individual systems and their interactions with one another. In this study, we do not precisely specify the boundaries of the broader DACCS system in Canada, which are fluid and imprecise. Instead, we draw on the DACCS policy literature, which identifies a variety of policy domains and policy concerns, to identify the elements of the DACCS system. This approach differs from prior studies on CDR and DACCS policy frameworks, which have tended to look at policies that are explicitly directed to DACCS technologies, whereas our approach is more functionally driven looking at the system elements that will shape DACCS development and deployment, but may address broader system conditions.
Canada is certainly not the only country well-positioned to scale up CDR deployment, but its current policies and infrastructure (e.g., available government subsidies and existing carbon storage capacity and regulation) will likely allow it to become an early mover whose deployment strategy other countries may observe and emulate. There is already a maturing DACCS research and development presence in Canada, including an operating demonstration plant that captures 1 tCO2/day in British Columbia (Carbon Engineering, 2021). The Province of Alberta has several operating commercial-scale CCS projects which contribute a combined capacity of 3.0 MtCO2/year to dedicated storage. Alberta is actively considering proposals to increase its capacity to 56 MtCO2/year, which will make for more efficient use of the province’s existing carbon storage and transport infrastructure (Canada Energy Regulator, 2022a).
Canada’s emissions are high, both currently and historically. The country’s greenhouse gas (GHG) emissions were 670 MtCO₂eq in 2021, or approximately 1.5% of global annual emissions, though Canada accounts for only 0.5% of the global population. Canada’s existing nationally determined contribution (NDC) is to reduce its emissions 40–45% below 2005 levels by 2030 (to 438 to 401 MtCO2eq) and to achieve net zero emissions by 2050 (Government of Canada, 2021).
The government has acknowledged the importance of CDR in its long term low GHG emissions development strategy (Environment and Climate Change Canada, 2022). In a 2023 analysis by the CER, the (2050) net-zero scenario presented included approximately 150 MtCO2 of negative emissions, including 45 MtCO2 of DAC (Canada Energy Regulator, 2023). This analysis represents, of course, just one possible scenario for DACCS deployment. To provide a better sense of the potential scale for DACCS in Canada under different scenarios, we examined modeling studies that provide regional scale DACCS deployment pathways for a variety of socioeconomic pathways. As indicated in Table 1, derived from the integrated assessment modeling study conducted by Fuhrman et al. (2021), estimates of the cumulative deployment of direct air capture in Canada varies significantly based on scenarios defined by the Shared Socio-Economic Pathways (SSP) framework. This variation spans from 431 Mt. of CO2 removal in an SSP1 scenario to a substantial 11,775 Mt. of CO2 removal in an SSP5 world for scenarios aligning with the 2-degree target. The significant variation affirms the high levels of uncertainty at the national level, but suggests that from a planning perspective, there is a need for Canadian policymakers to consider and plan for the development and potential deployment of DACCS, perhaps at very large scales. This is complicated by the fact that DACCS deployment at any level may necessitate cautious or low regret policy steps until greater certainty can be achieved.
We conducted an integrative review to identify articles related to our thematic areas of interest: CDR and DACCS systems and policy recommendations relevant to their scale up (Braun and Clarke, 2006; Snyder, 2019). Using Google Scholar and the snowballing method, we identified a group of 24 articles (published as of October 2022)2 published in English after 2015 (Wohlin, 2014). We initially searched for articles that contained the terms “direct air capture,” “carbon dioxide removal,” or “negative emissions technology” and “policy”. Based on this search, we eliminated articles that did not directly address policy and articles that focused on nature-based CDR or making recommendations specific to other NETs. Where possible, we included recommendations from articles that discussed Canada to some extent, though these did supply a more diverse selection of recommendations compared to others in the group. We only included articles that made actionable recommendations or outlined specific objectives of CDR or DAC-focused policy. In this context, “actionable” means that we did not include articles that made general observations about DAC pathways and CDR regime typologies, though many articles that did were still included in the general literature review; however, we did not require articles to identify specific policy instruments. We did not limit our selection to peer-reviewed academic articles because think tank and government reports have put forth substantial research at this intersection of CDR and policy. Gray literature was therefore included.3 Although the number of articles is smaller than the sample that might be used in a bibliometric or systematic review, many of the articles reviewed adjacent literature and identified similar objectives, therefore we did not restart or change the parameters of our search to add additional articles after reaching a thematic saturation point in the process of inductive coding (Saunders et al., 2018). Table 2 shows the articles and the criteria based on which they were selected. Manually coding these articles, we extracted 175 policy objectives; that is, specific recommendations regarding desirable policies or policy goals.
After developing the codes, we sorted them into six thematic domains: (1) general environmental policy goals and climate mitigation strategy; (2) energy policy and local resource constraints; (3) carbon transport and storage regulation and infrastructure; (4) financing scale-up and supporting innovation; (5) carbon capture and removal technology availability; and (6) social acceptability and public interest. Within each domain, the policy objectives were ordered in a hierarchical chart. The objectives hierarchy chart allowed us to classify the sub-objectives (or means objectives) that fulfill the more fundamental system objectives indicated by the core organizing domains (Clemens and Reilly, 2014). Subsequently, we removed redundant codes and used those that remained to produce a set of 116 assessment questions representing the objectives and sub-objectives.
The purpose of the second stage of the study was to determine the extent to which the identified policy objectives are represented in the current Canadian policy framework. We drew from the Canadian Climate Institute’s climate mitigation policy database, which includes descriptions of all relevant climate and related energy policies at the federal and provincial levels (see Bryan et al., 2022). Since the data collection for the study was completed in the latter half of 2022, there have since been changes in policy and government reports that reflect the pace of change in this arena. The original database itself has also been renamed and reorganized. Their database was suitable for the purposes of this research because it provides detailed descriptions and classifications of a broad spectrum of climate and associated policies at the federal and provincial levels. We collected additional information on non-climate environmental policies not included in the scope of the database, but relevant to the domains. Since our aim was not to conduct a document analysis, we used the descriptions provided within the Climate Institute’s database and located Supplementary material where necessary (such as specific policy details or quotations from government materials). The total sample size was 457. We conducted an initial analysis to identify those policies that were relevant to the DACCS policy objectives identified in Stage 1, reducing the sample size to 174 policies.
Using the assessment questions developed in Stage 1, we then examined the policy data set to determine whether the policy objectives were met, partially met, or absent (that is where we could not locate a policy that fulfilled the objective in question) within the Canadian policy regime. The policies that fulfilled the criteria were listed, with details of the policy purpose or mechanism where required. We did not place policy examples exclusively in one section or another. Instead, we highlighted the policy aspects that allowed it to meet each objective separately; some policies appear in multiple domains. We also detailed the “MLP level” (niche/regime/landscape) for each policy that fulfilled an objective to provide a more detailed picture of the policy’s systemic function within the context of a socio-technical transition. The coded matrix for all 116 questions is included in the Supplementary material.
In sections 4.1–4.6 below, we review and analyze the policy objectives that are present within the Canadian policy framework as well as the policy gaps revealed. We review each domain separately, with each section beginning with tables that summarize the findings under each domain (see Supplementary materials for disaggregated objectives). The first columns of the tables summarize the key objectives identified for each domain; the second columns identify specific policy instruments (from the Bryan et al., 2022 database); the third columns identify which and how objectives were met; and the fourth columns summarize key gaps in each domain. While the tables in sections 4.1–4.6 provide illustrative examples of the Canadian policy and deployment context, the examples provided in the tables are notexhaustive and do not reflect the complete array of policies analyzed (but which are found in the Supplementary material, along with the complete list of policy objectives and the corresponding policies identified as relevant to them under each domain). Each subsection will expand upon the policy gaps and tailoring needs in the Canadian context, as well as the socio-political dynamic that are not immediately evident in the tables.
A principal goal of this study is to assess the completeness of the objectives within the existing Canadian policy framework. Table 3 below provides a numerical overview of the total number of questions associated with each policy domain, and the policy objectives met, partially or absent for each domain. The results provide a sense of which parts of the DACCS system are more or less complete. Unsurprisingly, the two areas that appear to have policy objectives least represented in the existing policy framework are the two DACCS-specific policy domains addressing removal/capture technology and carbon storage and transportation. Here the policy objectives identified are specific to the technology itself and are less likely to build upon existing policy foundations. Within the storage domain, CCS regulations address many of the technical issues, but these only exist in one or two provincial jurisdictions, and the existing regulations still reflect the limited scale of current carbon storage activities. The more represented areas are those domains that rely more fully on general regulations and policies suitable for DACCS with limited need for adaptation. For example, general public participation processes will be suitable across multiple policy contexts. Table 3 must be read with caution, as a few absent policy objectives may still be highly consequential.
Whether the regime has a carbon pricing system is a critical policy for the sociotechnical regime in Canada as a whole, not just the DACCS system. Putting a price on emissions incentivizes abatement itself and the development of innovative technologies to assist abatement (Baranzini et al., 2017; Beuttler et al., 2019; Strefler et al., 2021). Table 4 lists several of the carbon pricing systems in place in Canada, including the federal benchmark that sets the minimum requirements across provinces. Multiple authors observe the centrality of maintaining a stable, long-term carbon pricing system, though most identify it as a necessary but not sufficient condition (Nemet et al., 2018; Cox and Edwards, 2019; Rueda et al., 2021). Although long-term plans for pricing increases have been well established and legislated across Canada, and as such are not identified as a gap in the system, it is important to note that such plans and regulations are politically vulnerable. Carbon pricing remains deeply contested within Canada, with the main opposition party promising to reverse carbon pricing policies (The Globe and Mail, 2023). The instability of a long term carbon price creates greater investment uncertainty for technologies like DACCS, where scaling relies on market-based choices. Without a carbon price, DACCS developers would need to rely solely on government procurement or deployment mandates – though estimates indicate that even the more cost effective end of the DACCS price range (per tonne) will not be comparable to a high national carbon price for at least a decade (Lebling et al., 2022). There are no procurement or deployment mandates for DACCS in Canada. Additionally, maintaining stability in a carbon pricing system is important as internalizing the cost of emissions shifts how society values carbon-intensive production compared to alternatives.
The federal output-based pricing system (OBPS) and the federal fuel charge that create the carbon pricing system in Canada do not apply to every province, as the federal scheme operates as a backstop, and allows provinces to develop equivalent schemes. Several provinces, including Alberta, Saskatchewan, and Ontario, have their own OBPS approved by the federal government, but still have to apply the federal fuel charge. Others, like British Columbia, Quebec, and most maritime provinces, have entirely independent systems; some are cap and trade, and others are tax-based. National policy coherence for climate governance is limited by the distribution of legislative powers between the federal government and provinces. This also results, for example, in inconsistencies between carbon reduction targets and strategies in different provinces. Federal commitments to reduce emissions do not necessarily yield equally robust plans across the country (Fertel et al., 2013).
Commentators have identified the need for government authorities to address removal targets separately from reductions targets within reporting structures (McLaren et al., 2019; Honegger et al., 2021; Schenuit et al., 2021). Currently, the distinction does not exist in Canadian policy, insofar as the regulatory structure treats removal credits in the same manner as reduction credits. Although Canada’s carbon offset credit protocol is incomplete under the Greenhouse Gas Offset Credit System, the government has indicated an intention to treat DACCS removals as creditable offsets. In the absence of distinct treatment in offset regimes, removal and reduction credits are effectively fungible, potentially affecting the ability to incentivize high cost carbon removal. It may, however, be desirable to provide further incentive for purchasing removals to enable a commercialization pathway (especially because, for hard-to-abate industries, removals will be more expensive than CCS-based reductions; Rickels et al., 2021). The distinction between types of credits is also necessary to establish standards and offset accounting protocols at the global level (Peters and Geden, 2017). Negative emissions will likely be provided by private firms on a transnational basis, which means that international cooperation will be necessary to avoid double-counting in trade and ensure consistency across national systems. This is a prevailing gap in Canada, but also within the broader system landscape.
Table 5 shows select energy decarbonization policies that exist or are in development in Canada. The policy framework for DACCS in Canada must address the strain that the energy and resource intensity of DACCS puts on the energy system, and the potential the DACCS energy demands may conflict with other priorities in the energy transition. Electricity in Canada is largely provided through low-carbon sources with 80% of electricity coming from non-emitting sources, such as hydro and nuclear (Canada Energy Regulator, 2022b). Canada does not have to replace large amounts of fossil fuel generated electricity; additional capacity could be directed to new sources of electricity demand, including DACCS (Wohland et al., 2018; Singh and Colosi, 2022). Since energy policies and infrastructure fall largely under provincial jurisdiction, provincial cooperation to expand non-emitting capacity or direct electricity to negative emission projects would be required. Energy policy planning that accounts for DACCS has been largely exploratory, without explicit policy direction, in part due to the uncertainty associated with DAC scaling. Nevertheless, energy systems will need to expand in lockstep with DACCS to achieve scale in the future (Fuss et al., 2018; Creutzig et al., 2019; Larsen et al., 2019; Lehtveer and Emanuelsson, 2021; Williams, 2022). At present, while the energy and resource demands of a technology like DACCS may be considered in impact assessment processes, such assessments are project-based and would not involve strategic or transition oriented assessment (Nwanekezie et al., 2022). In contrast, United States policy plans have identified the need to coordinate DACCS scale-up with domestic low carbon energy sources (U. S. Department of Energy, 2021). Canadian policy has not yet established guidelines for managing the trade-off between DACCS and the energy-efficiency goals of climate policy. The lack of long-term, coordinated energy planning is a significant deficit.
Many of the policy examples in Table 5 show how governments are targeting emission reduction via electrification and total energy system decarbonization as a regime objective. However, the regional and sectoral distribution of emissions is uneven, with high concentrations of emissions coming from western Canada, as a result of high GHG emissions associated with the oil and gas industry. Table 5 does not reflect the fact that some provinces are further along than others. For example, through the Renewable Electricity Act, Alberta will require 30% of electricity in the province to come from renewables by 2030, in addition to the government’s commitment to phase out coal-powered electricity by 2023 and the Oil Sands Emissions Limit Act restriction of GHG emissions above 100 MtCOeq/year. Simultaneously, Alberta has the most fossil fuel intensive electricity production in the country at 90% (54% Natural Gas and 36% Coal and Coke) and the oil and gas sector is a major part of the province’s economy, which makes decarbonization a politically contentious prerogative (Canada Energy Regulator, 2022b). Energy infrastructure remains largely a provincial matter, which creates a disjuncture between national climate goals, including DACCS development and jurisdictional responsibilities. The federal government may influence policy direction through fiscal tools, such as subsidies and tax credits, but these may increasingly be at odds with some provincial policies and regulations that are directed toward optimizing fossil fuel extraction.
The geology of a region is a landscape condition that, in part, determines where projects can be sited. However, transport infrastructure, technology, and regulations enable particular types of storage. Table 6 shows illustrative provincial and federal carbon storage and transport regulations. Canada has large amounts of accessible geological storage that would be suitable for CO₂ storage, though this potential varies from province to province based on geology (Hares et al., 2022). Alberta, where pore space is abundant relative to most other provinces, has established a clear regulatory regime for CCS (see Bankes, 2008, 2019), and has built supporting transportation and injection infrastructure that optimizes CCS (but not necessarily DACCS) using a hub-based model (Government of Alberta, 2023). As of 2021, Alberta is injecting and storing 3.17 MT CO2/yr. (CER, 2022). In addition to the Western Canada sedimentary basins, there is further potential CO2 storage capacity in offshore areas in Atlantic Canada, and transboundary opportunities to transport CO2 in sedimentary basins located in the Appalachian region of the United States (CCS Knowledge Centre, 2021).
Table 6. Summary of policy and objectives in carbon transport and storage regulation and infrastructure.
The federal government is in the early stages of clarifying what kinds of storage sites and existing infrastructure can be used to support the scale-up of CDR in the system. There is not enough publicly available information about the federal government’s plans to determine what objectives they fulfill – which would enable us to analyze which features may need further improvements to benefit a DACCS system rather than a CCS system. The absence of federal guidelines and incentives to coordinate transport, prioritize secure and permanent storage, and co-locate DAC projects accordingly may inhibit the development of large-scale CDR storage projects (Cox and Edwards, 2019; Craik et al., 2022; Erans et al., 2022). The federal government retains some environmental authority around the regulation of carbon (e.g., via the Canadian Environmental Protection Act [CEPA]), but the regulation of pore space itself falls under provincial jurisdiction. The regulation of geological storage is uneven across regions, with existing frameworks being found in jurisdictions with emerging CCS industries. Other areas with storage potential, notably Atlantic Canada, have not yet taken any concrete actions to develop storage opportunities. The current approach to storage policy reflects the prior interests of oil and gas and other subsurface rights holders, and is rooted in sectoral policy in these areas (Bankes, 2019) – a trajectory that may be followed in other jurisdictions, if and when storage opportunities unfold. The legal issue that arises is how to apply existing laws, where to introduce new amendments and new policies, and whose interests will prevail in the development and allocation of storage opportunities (Cox and Edwards, 2019; Craik et al., 2022). In this latter regard, there is potential for different user groups (EOR, CCS and DACCS) to compete for storage and transport space, but there are no policies in place to address allocation.
The policy objectives identified in this domain emphasized the need for regulations and incentives that support and prioritize the scale-up of CO₂ storage over CO2 utilization, considering current forms of utilization are generally less permanent or result in rerelease of CO2 (e.g., beverage carbonation, EOR) (Cox and Edwards, 2019; Fajardy et al., 2019; Meckling and Biber, 2021; Erans et al., 2022). Nevertheless, utilization is an important element of NET developer business models and the commercialization of CO2 utilization niche innovations will play an important role in securing financing within the system (Mac Dowell et al., 2017; Haszeldine et al., 2018; Nemet et al., 2018). The planned federal investment tax credit for carbon capture and storage will likely introduce some incentives that are directed toward utilization activities, such as using carbon in cementitious material (Department of Finance, 2022).
Niche technologies can radically change a system and stabilize it amidst landscape changes (Geels, 2004). Moreover, providing financial incentives for investing in and directly funding a niche technology creates a protected market that allows the technology to mature and become more efficient and cheap, grow less dependent on subsidies, and more effectively integrate into the system (Geels and Schot, 2007; Schot and Geels, 2008; Cecere et al., 2014). The government’s deployment strategy for CDR seems to focus on creating an environment to foster niche development through subsidies and coordinating infrastructure. Funds for such projects are currently available through a variety of government programs, as listed in Table 7. The Federal Government has recently introduced new funds and incentives to support NETs, CCS, and carbon pipeline infrastructure across the country. Of note, is an investment tax credit (ITC) for CCS development, with a specific DACCS component. The tax credit resembles similar support provided by the United States government for DACCS, although the Canadian amounts provide a lower subsidy (IEA, 2022). The structure of the ITC includes a phase out from 2030 to 2040, indicating an expectation that DACCS would be commercially competitive with other forms of removal within that time frame (Department of Finance, 2022). Through the Energy Innovation Program, the federal government will also invest $319 million over 7 years to the research and development of CCS technologies to lower technology costs. Such funds will also increase the availability and commercial development of CO2 utilization niches, which would help develop a market for captured carbon. The scale of support in Canada is significantly lower than under recent U.S. legislation, raising potential competitiveness concerns (U. S. Department of Energy, 2021).
Table 7. Summary of policy and objectives in policy for financing scale-up and supporting innovation.
There is no indication that the government intends to pay for DACCS directly or act as a primary market by purchasing DACCS credits to offset their emissions, which is a model that some authors explore (Buck, 2019; Honegger et al., 2021; Hodgson and Hodgson, 2022). It is important to clarify whether the government intends to foster the creation of carbon removal markets or treat CDR as a public good because uncertainty in the system may undermine other market signals and incentives that would otherwise aid CDR scale-up. The government’s support determines how niche technology developers shape their business models.
Finally, the optimal order and strategy for rolling out financing and innovation policies are still unclear. Meckling and Biber (2021) suggest an “incentives + mandates” policy strategy: mandates because of their lower political cost and incentives due to their demonstrated effectiveness in scaling up other low carbon technologies. Feed-in tariffs, new emissions standards, reverse auctions, and carbon pricing are all options that are likely to help incentivize DACCS (Lackner and Azarabadi, 2021). Subsidies can be controversial, as evidenced by the national backlash against the CCUS ITC (Tuttle, 2022). Subsidies, at least in the near term, are a necessary part of the strategy for CDR at scale because they support the development of niche innovations. The planned reduction of the ITC after 2030 is a reflection of the government’s intention for financial support to create a viable niche market that will be able to sustain itself once technologies have reached scale. However, the timeline for reducing high removal costs is a major uncertainty in the current regime.
There is overlap between the policy objectives in this section and domains addressing innovation financing and broader market structures. Table 8 shows a gap in the policy framework related to DAC specific regulation and overall CDR scale-up (Lomax et al., 2015; Marcucci et al., 2017; Fuss et al., 2018; Haszeldine et al., 2018; Larsen et al., 2019; Honegger et al., 2021). For example, technology mandates in other sectors are a part of the government’s climate mitigation strategy at the federal level, both for the market and for the technology the government itself uses (e.g., the Zero Emission Vehicle Mandate and the Low-Carbon Fuel Procurement Program). A DACCS deployment mandate for the government or the private sector has some analogous programs to build upon, recognizing that DACCS presents some unique challenges (Larsen et al., 2019; Honegger et al., 2021; Erans et al., 2022; Hodgson and Hodgson, 2022; Williams, 2022). Many objectives in this section included longer-term considerations compared to questions about what kind of near-term support the government should provide. This mainly involves introducing new, CDR-specific policy not addressed in other domains (i.e., storage-related tailoring). Canadian policy makers have not identified specific targets for CDR or which types of CDR may play an important role in achieving its net-zero objective. The role that the government needs to take in managing and providing CDR will depend on the degree to which new market mechanisms or mandates related to CDR can provide sufficient social benefit, which are not necessarily reflected in the historical market failures that allowed climate change to progress. It is premature to assess the state of CDR-specific policy in Canada, but the current pattern, as exhibited in CCS regulation and emerging CDR regulation, is for specific regulations to develop in a more reactionary mode, as commercial scale deployment begins. There is little evidence of anticipatory regulation intended to shape technological or development outcomes.
Table 8. Summary of policy and objectives in removal & capture technology availability and regulation.
Table 9 shows select policies related to social acceptability in Canada. Recent literature suggests that perceptions of risks and climate benefits associated with storage, rather than the capture process alone, are a key criterion for acceptance, as well as the use of renewables within DACCS systems (Arning et al., 2019; Cox et al., 2020; Satterfield et al., 2023). In particular, a recent study by Satterfield et al. (2023) surveyed Canadian and US residents in the vicinity of proposed pilot sites of DAC paired with sub-sea floor CO2 storage and found that participants were concerned with the impacts and risks of the whole DACCS system after being informed of the components of the process, including energy sources. These results suggest that public engagement efforts should be holistic, “fine-grained, and sensitive to public knowledge of NETs,” as community values and perceived urgency of climate change will vary based on socio-political and environmental contexts (Satterfield et al., 2023, p. 13). Although public acceptability tends to be higher for nature-based CDR than for NETs, it also largely depends on how the risks and benefits of new infrastructure and technology projects are framed in public discourse; stakeholders may be more open to engineered solutions with gigaton-scale removal potential if they receive adequate information during the engagement process (Buck, 2016; Bellamy, 2022; Cooley et al., 2023; Satterfield et al., 2023). In turn, this depends on the dynamics and social relations in a particular social context, as well as effective government communications, which makes this policy dimension especially important in the early stages of the transition and DACCS scale-up. Canadian policy discourses on just transitions have encompassed the potential transitional role of CCS, providing a foundation for similar discussions in relation to DACCS.
Although recent government publications and plans have signaled that the Government of Canada intends to integrate CDR, CCS, and DACCS into the national climate strategy, there is generally insufficient public communication about the technology to accompany this signal for scale-up (Government of Canada, 2021; Environment and Climate Change Canada, 2022). Thus, the main policy concerns for this domain are tailoring and general improvements to public engagement processes. Existing processes for public consultation, such as environmental impact assessment, are project driven, which provide an opportunity for public understanding of localized, environmental impacts. There is, however, a significant gap in opportunities for higher order, strategic assessments, involving public awareness and consultations that would look at the long-term strategy associated with scaling up DACCS. The 2023 Carbon Management Strategy, which outlines long-term strategies for meeting Canada’s net-zero commitments through CCUS and DACCS describes a public consultation process, but in doing so focuses exclusively on actions in support of the approach. Among the key policy tools identified in the strategy is the investment tax credit for CCS and DACCS, but when this instrument was released it was subject to trenchant opposition from some quarters. The close association in Canada between CCS and DACCS presents a risk that concerns over the ability of CCS to further entrench fossil fuel interests will spill over to DACCS (Anderson, 2022). Another potential mechanism for public awareness, the Canada Net-Zero Emission Accountability Act, which includes an advisory board that produces reports to guide the (federal) government’s pathway to net-zero has not engaged in any significant analysis of the role of DACCS in transition plans.
The current, modest levels of CDR activity in Canada have not been without controversy. The ITC, which covers CCS and DACCS, was opposed by a number of environmental groups and academics in Canada on the basis that it was a further form of subsidization to the fossil fuel industry and would delay more meaningful decarbonization efforts (Anderson, 2022). DACCS-related infrastructure, particularly at large scales, may give rise to public and Indigenous opposition similar to what has been seen with pipeline and other large infrastructure projects in Canada (BBC World News, 2020). The dynamics of social opposition and acceptance will influence net-zero pathways at a systemic level. An important aspect of public consultation in Canada is the constitutional duty on the government to consult Indigenous groups where government decisions have the potential to impact Aboriginal and treaty rights under 35 of the Constitution Act (1982). Insufficient Indigenous consultation has had a major impact on numerous infrastructure projects in Canada, such as pipelines and dams, and has increasingly turned to discussions on benefit sharing arrangements between resource developers and Indigenous groups (Exner-Pirot and Ignasiak, 2023). There is little evidence of Indigenous engagement on the scale-up of CCS and DACCS in Canada, notwithstanding that storage activities occur in areas that are subject to Aboriginal and Treaty rights, and a rising recognition of the role of benefit sharing in carbon management activities (Government of Canada, 2022). Beyond the Canadian government’s duty to consult Indigenous groups, these gaps and tailoring needs for policy extend to other countries planning to implement large-scale CDR-related infrastructure projects. Specifically, in preserving Indigenous peoples’ right to free, prior and informed consent in countries that have ratified the United Nations Declaration on the Rights of Indigenous Peoples. In the context of a sociotechnical transition, infrastructure projects and land use changes must be designed to address rather than exacerbate the vulnerabilities of local communities by creating low-carbon job opportunities and providing benefits to aid sectors in transition. Improving the public’s awareness of these technologies is important in the transition process. The socio-technical regime, according to Geels (2002), includes cultural and symbolic meaning. Context-appropriate communications about NETs at national, provincial, and local levels impact the symbolic meaning of the technologies in society, potentially reducing their perceived risks through techno-scientific knowledge sharing. This, in turn, influences user preferences and markets within the regime, helping project developers secure a social license to operate and avoid delays caused by societal backlash if a project is perceived as illegitimate or harmful. On the other hand, failures to address legitimacy concerns associated with technologies, or aspects of the transition can erode social acceptance.
The MLP allows us to situate DACCS in relation to the key components of the existing system and the processes of change within the system. There are clear examples of niche development initiatives oriented toward the development and scaling of carbon capture technologies. These mostly include financial support for research and commercialization of the capture technologies and linked support for utilization markets, such as using carbon in cementitious material or in synthetic fuels. By introducing new ‘rules’ to the system through incentives and regulations, regulators can help niche technologies both enter the regime and fulfill a necessary role in the transition. Other technological niches will also help to leverage change and successfully implement DACCS while maximizing the efficiency of project sites (e.g., via utilization).
Our assessment has revealed that the current regime largely lacks sectoral policies specific to DACCS and CDR, which are necessary to establish a functional DACCS system. The absence of policy is obvious enough, given the newness of DACCS and other NETs. However, this analysis has also highlighted the importance of identifying the policies and infrastructure that are precursors to more specific policies and niche development. Coordinated regime changes will work to adapt infrastructure and attitudes around these technologies to weaken path-dependencies and diffuse the cost of restructuring the system and introducing new network technologies. Policies that support niche carbon utilization opportunities in the near term, for example, will influence the availability of mature utilization technologies by mid-to-late century, therefore affecting the commercial viability of DAC and opportunities to reduce removal costs further once the technologies have begun to scale. The development of a carbon utilization market will, depending on transport and energy infrastructure, also eventually influence where DACCS and CCS projects are sited, as well as more targeted regime policies on regional and national levels (Valiaho, 2020).
The scale-up of DACCS will occur in the context of regime change and a low-carbon system transition. DACCS will, in other words, become one of many technologies within Canada’s climate mitigation toolkit and within the socio-technical system, rather than simply a technology introduced mid-century to mitigate overshoot. From an MLP perspective, the embeddedness of DACCS with other systems points to the importance of incremental shifts within the existing regime as an important determinant of future pathways. If energy infrastructure planning decisions remain separate from discussions of where to site DAC and coordinate carbon transport and storage networks, then the risk of technology lock-in in Canada will increase, since pipelines and energy infrastructure are capital-intensive, long-term infrastructures. This, in turn, factors into a firm’s selection criteria when deciding where to site their facilities; in energy-intensive industries, for example, firms would be most willing to site facilities where they could access a cheap supply of energy.
Unlike many historical cases of technology transitions that have featured the substitution of one technology by another, DAC is not replacing a previous technology and taking on its role in the regime. Rather, DACCS is a technology that fulfills a service that was not previously necessary to maintain a stable regime but is increasingly viewed as fulfilling novel functions that are necessary due to landscape conditions. As Geels (2011) notes, sustainability transitions are purposive, in contrast to transitions driven by market structures. An important question in the Canadian context is who is shaping these purposes and the function of DACCS within the emerging net-zero transition. In this regard, DACCS has a complicated relationship to the existing regime. The dominant regime includes the infrastructure and technologies of a fossil fuel-intensive economy. Replacing this economy and repurposing existing infrastructure will be an important part of managing the transition. The process of developing a DACCS sub-system within the larger Canadian socio-technical system is largely congruent with that objective because, for the purposes of carbon storage and transport, DACCS and other NETs can make use of some existing pipelines and depleted oil wells. The storage dimensions of DACCS are similarly aligned with the dominant regime as they have potential to share transportation and storage infrastructure with CCS.
DACCS is portrayed as simply part of a larger carbon management plan that includes point source capture and carbon utilization (Natural Resources Canada, 2023), meaning the development of DACCS and CCS draw on common policy instruments and infrastructure. DACCS does not directly threaten the fossil fuel based political economy since it is not intended to replace existing energy sources. Nonetheless, DACCS may be a significant driver in future energy decisions, as its efficiency as a carbon removal process is strongly dependent on the scale of non-emitting energy.
Geels and Schot (2007) note that different configurations of landscape pressures and the extent to which niche innovations are competitive or symbiotic with the existing regime will lead to different transition pathways. At this early stage, the transition pathway for DACCS in Canada has some characteristics that are consistent with a “reconfiguration” pathway, where the niche innovations have symbiotic relations with the existing regime and are adopted as add-ons, which may be adapted over time and reconfigured in response to landscape pressures. What is unclear at this stage is whether DACCS is a means of catalyzing a shift within the broader system or if it will further entrench path-dependencies in the currently dominant regime. In exploring this tension that DACCS creates between transformation and stasis, Asayama (2021) sees some potential for policy design to limit the extent to which DACCS acts as a reinforcing technology. In our examination of the Canadian policy framework, we saw no indication of policies that would have as their goal the realignment of DACCS away from fossil fuel industry interests.
A key finding of this study is that many of the types of policy actions that the government should undertake do not belong to a single domain or sector. Rather, the objectives that DACCS system policies must fulfill extend to multiple parts of the system, which requires cooperation between different actors and coordination across government institutions. For example, clarifying carbon storage regulations not only enables developers to design transport and storage infrastructure, it also reduces uncertainty for investors, which helps DAC projects secure funding; with effective public communication, these clarifications can also promote social acceptability in Canada.
The interdependencies within the system are a defining characteristic of complex systems generally and suggest the presence of leverage points – points of intervention within the system that will most effectively transition the system (Meadows, 2008). Our study was not directed toward establishing leverage points, but we can observe a number of potential candidates that deserve further attention. For example, CCS development provides a commercially viable pathway to scale up storage sites and build related infrastructure and expertise. Carbon from CCS is often used for enhanced oil recovery (EOR) where it is injected to access an otherwise inaccessible output from depleted oil wells, which furthers its commercial value. While carbon from CDR may be used for EOR, many of the articles we selected cautioned against this business model due to its potential to detract from mitigation. However, other research has found EOR yields a net reduction in the carbon intensity of oil since injected carbon is stored permanently, which makes it a marginally more sustainable alternative to oil extracted through conventional methods (Sminchak et al., 2020; Clean Air Task Force, 2021). The CCS system is likely to influence the shape of a DACCS system that builds upon this foundation. The potentiality of CCS and EOR operating simultaneously suggests a need for policy to promote permanent carbon storage and utilization above other uses that entail reemission (or at least include a strategy for transitioning away from point-source CCS and EOR once fossil fuel production declines). In Canada, CCS is mostly being bought and paid for by the oil and gas industry, who are paying because they want to preserve the long-term viability of their industry, not just prolong its life by a decade. The Canadian ITC will not credit EOR uses of carbon (crediting only predefined “permanent” uses and storage) and has defined end-date, unlike the tax credit policy in the U.S which provides credits for CCS projects that use EOR; this difference may reduce lock-in risk by comparison (U. S. Department of Energy, 2021; Department of Finance, 2022), but may also place Canadian developers of DACCS at a competitive disadvantage.
Energy policy – specifically, the ability to provide large amounts of non-emitting power – drives the overall viability of a DACCS system and will drive siting decisions, which technology developers will need to leverage to support energy-intensive niches. A third potential leverage point is the presence of a robust carbon pricing system, which provides a commercialization pathway for DACCS. Carbon pricing alone will not be enough to incentivize uptake, but it does help to reorient the objectives of the system and support the viability of niche technologies during and after they ‘incubate’ with the support of subsidies, private investment, and regulatory support. The federal benchmark for carbon pricing in Canada will rise to 170 CAD by 2030; if policy support can help CDR and DACCS technologies scale and lower their prices by the end of the decade, removals could be cheaper than the carbon tax (Environment and Climate Change Canada, 2022). Both Climeworks and Carbon Engineering expect their technology’s removal cost to fall below 100 USD per tonne in this approximate time frame, although other estimates are less optimistic (McQueen et al., 2021). Though the cost of nature-based removals and abatement are lower than both in the near term, their marginal costs will also rise as land becomes scarcer and firms exhaust lower-cost abatement options (Fuss et al., 2018; Gillingham and Stock, 2018). The policy framework in Canada is oriented toward a market structure for DACCS, as evidenced by the phase-out timeframe of the investment tax credit, which suggests an intention to limit subsidies over time, and an expectation that DACCS will transition to a self-supporting commercial model. We note that the alternative is a procurement or mandate model, but there are no indications within the policy framework that this is an anticipated orientation. A final potential leverage point is the degree of social acceptance of DACCS and its components. Social acceptance will influence the physical siting challenges, which will be shaped by risk perceptions. Acceptability may affect the ability of governments to subsidize DACCS development over an extended period and at what may be high levels. Social acceptance will also operate on a more diffuse level, affecting whether DACCS is viewed as a constructive element of the net zero transition or as a dangerous distraction. There is evidence of these opposing framings shaping the Canadian discourse around government support for DACCS.
Large-scale CDR and DACCS deployment will be highly context-specific, as will the long-term approaches for managing the technologies. This research was motivated by a recognition of the need to investigate the structure and policy content of national-level policy frameworks that will support and shape DACCS development and scale-up. The importance of national contexts for CDR and DACCS development pre-existed this study, but our study builds on the literature by providing a first in-depth analysis of a nascent national-level DACCS system and the findings provide empirical support for this position.
An important contribution of this study is the methodological approach we developed to map the contours of the DACCS policy framework in national settings. A key advantage of our approach is that by starting with broader policy objectives and matching those objectives to a database of existing policies, we were able to identify the policy framework in a more comprehensive fashion, then analyzing policies that are framed as explicitly directed toward CDR or DACCS. We believe that the approach is replicable in other jurisdictions, and there would be benefit in further country-level studies that share a common approach to support inter-country comparability.
The policy objectives identified in the CDR and DACCS literature are represented within a complex array of pre-existing policy instruments and programs within Canada. These policies are in many cases not oriented toward DACCS, and as such will be subject to a variety of political and economic influences. The system may be characterized as having pockets of DACCS specific policies, which operate in a larger system that is not intentionally directed toward DACCS or CDR. This finding aligns with the MLP in that change within the system will be a function of both the pre-existing stricture as well as the exercise of agency within the system.
This points to a more overarching gap that is evident within the DACCS policy framework. Many of the existing policy processes drawn upon respond to local and often shorter-term priorities, at the expense of more strategic-level guidance. This, in our view, is most evident in the complex interplay between CCS and DACCS in Canada, where resource interests are able to shape the emerging CCS system and draw on the promise of DACCS to move the system toward net zero. However, there is little evidence of how the transition from a CCS-dominated system to DACCS would be managed. Existing policies will need to be tailored to enable a transition between the two and ensure CDR-specific strategies at all levels of government, including a robust MRV process, an overarching deployment plan, and cohesion across different policy domains.
Many of the policy domains for DACCS deployment, such as geological storage regulation and energy policy, are under the jurisdiction of provincial governments, which creates a potential disjuncture between the federal government’s ambitions and long-term strategies for DACCS and the policy authority to influence those outcomes. Since DACCS deployment will depend on provincial involvement, federal/provincial cooperation appears to be a key structural variable within the system. Different provinces are likely to hold divergent interests in climate solutions that will shape the regime structures within their respective provinces. This is already manifested in the regionalized approach to CCS, which is focused in Alberta and directed toward the maintenance of oil and gas exploitation within that province. The federal government has its own levers, such as tax policy and innovation support, but climate and energy policy are deeply politicized in Canada, with implications for the exercise of power by both federal and provincial governments (Fertel et al., 2013; MacDonald, 2020).
The specificity involved in coordinating action and supporting CDR scale-up points to a further potential advantage of national-level policy studies for DACCS. Identifying the policy framework and mapping out the interconnections between policies provides a foundation to examine the political economy of DACCS. Political power and the ability to influence how DACCS is framed, is an important element that will impact the acceptability of DACCS (Buck, 2016; Bellamy, 2022). For example, the alignment of DACCS with existing resource structures provides a potential explanatory basis for the current government support of DACCS that requires further attention. The demand for CDR amidst the climate crisis will grow over the coming decades, along with the demand for NET-focused policy research. Thus, investigating the impact of DACCS politics on the transition process will be especially salient as the levels of technology deployment and CDR capacity rise.
The original contributions presented in the study are included in the article/Supplementary material, further inquiries can be directed to the corresponding author.
SC: Conceptualization, Formal analysis, Methodology, Visualization, Writing – original draft, Writing – review & editing. NC: Conceptualization, Supervision, Writing – original draft, Writing – review & editing. JM-C: Supervision, Writing – review & editing. KM: Writing – original draft. VS: Funding acquisition, Supervision, Writing – review & editing.
The author(s) declare that financial support was received for the research, authorship, and/or publication of this article. This project was funded in part by the Government of Canada. The Government of Canada’s Environmental Damages Fund provided funding for this project under its Climate Action and Awareness Fund. SC is supported in part by funding from the Social Sciences and Humanities Research Council. JM-C acknowledges support by the Alfred P. Sloan Foundation grant #2020-14025.
The authors declare that the research was conducted in the absence of any commercial or financial relationships that could be construed as a potential conflict of interest.
All claims expressed in this article are solely those of the authors and do not necessarily represent those of their affiliated organizations, or those of the publisher, the editors and the reviewers. Any product that may be evaluated in this article, or claim that may be made by its manufacturer, is not guaranteed or endorsed by the publisher.
The Supplementary material for this article can be found online at: https://www.frontiersin.org/articles/10.3389/fclim.2024.1338647/full#supplementary-material
1. ^In this paper we use the acronym “DACCS” to describe the system components as a whole (direct air capture and the transportation and storage of carbon). We use the acronym “DAC” to describe the direct air capture component alone.
2. ^Several relevant articles have also been published since we completed data collection, which may be useful in future studies of this kind.
3. ^Though number of citations on articles was included in early selection, it was not ultimately relevant because many gray literature sources lacked such numbers and because of the recency of some of the articles (e.g., at the time it was selected, Erans et al., 2022 had 34 citations and now has 182).
Anderson, D. (2022). How Canada's new carbon capture tax credit aligns (or doesn't) with the latest climate science. The Narwhal. Available at: https://thenarwhal.ca/carbon-capture-credit-ipcc/.
Arning, K., Offermann-van Heek, J., Linzenich, A., Kätelhön, A., Sternberg, A., Bardow, A., et al. (2019). Same or different? Insights on public perception and acceptance of carbon capture and storage or utilization in Germany. Energy Policy 125, 235–249. doi: 10.1016/j.enpol.2018.10.039
Asayama, S. (2021). The oxymoron of carbon dioxide removal: escaping carbon lock-in and yet perpetuating the fossil status quo? Front. Clim. 3:515. doi: 10.3389/fclim.2021.673515
Bankes, N. (2008). Legal issues associated with the adoption of commercial scale CCS projects. In Carbon Capture and Storage Forum, A Pembina-ISEEE Thought Leaders Forum.
Bankes, N. (2019). Alberta’s approach to the transfer of liability for carbon capture and storage projects. Int. J. Risk Assess. Manag. 22, 311–323. doi: 10.1504/IJRAM.2019.103331
Barahimi, V., Ho, M., and Croiset, E. (2023). From lab to fab: development and deployment of direct air capture of CO₂. Energies 16:6385. doi: 10.3390/en16176385
Baranzini, A., Van den Bergh, J. C., Carattini, S., Howarth, R. B., Padilla, E., and Roca, J. (2017). Carbon pricing in climate policy: seven reasons, complementary instruments, and political economy considerations. Wiley Interdiscip. Rev. Clim. Chang. 8:e462. doi: 10.1002/wcc.462
BBC World News (2020). Indigenous pipeline blockades spark Canada-wide protests. BBC. Available at: https://www.bbc.com/news/world-us-canada-51452217.
Bellamy, R. (2018). Incentivize negative emissions responsibly. Nature. Energy 3, 532–534. doi: 10.1038/s41560-018-0156-6
Bellamy, R. (2022). Mapping public appraisals of carbon dioxide removal. Glob. Environ. Chang. 76:102593. doi: 10.1016/j.gloenvcha.2022.102593
Bernstein, S., and Hoffmann, M. (2019). Climate politics, metaphors and the fractal carbon trap. Nat. Clim. Chang. 9, 919–925. doi: 10.1038/s41558-019-0618-2
Beuttler, C., Charles, L., and Wurzbacher, J. (2019). The role of direct air capture in mitigation of anthropogenic greenhouse gas emissions. Front. Clim. 1:10,
Boulding, K. E. (1991). What is evolutionary economics? J. Evol. Econ. 1, 9–17. doi: 10.1007/BF01202334
Braun, V., and Clarke, V. (2006). Using thematic analysis in psychology. Qual. Res. Psychol. 3, 77–101. doi: 10.1191/1478088706qp063oa
Bryan, D., Forg, F., Chambers, H., Thompson, K., and Bluschke, R. (2022). Climate mitigation policy tracker. Navius Research. Canadian Climate Institute. Available at: https://440megatonnes.ca/policy-tracker/.
Buck, H. J. (2016). Rapid scale-up of negative emissions technologies: social barriers and social implications. Clim. Chang. 139, 155–167. doi: 10.1007/s10584-016-1770-6
Buck, H. J. (2019). After geoengineering: Climate tragedy, repair, and restoration. New York: Verso Books.
Buylova, A., Fridahl, M., Nasiritousi, N., and Reischl, G. (2021). Cancel (out) emissions? The envisaged role of carbon dioxide removal Technologies in Long-Term National Climate Strategies. Front. Clim. 3:63. doi: 10.3389/fclim.2021.675499
Cairns, R. (2014). Climate geoengineering: issues of path-dependence and socio-technical lock-in. Wiley Interdiscip. Rev. Clim. Chang. 5, 649–661. doi: 10.1002/wcc.296
Canada Energy Regulator (2022a). Market snapshot: new projects in Alberta could add significant carbon storage capacity by 2030. Available at: https://www.cer-rec.gc.ca/en/data-analysis/energy-markets/market-snapshots/2022/market-snapshot-new-projects-alberta-could-add-significant-carbon-storage-capacity-2030.html.
Canada Energy Regulator (2022b). Provincial and territorial energy profiles. Available at: https://www.cer-rec.gc.ca/en/data-analysis/energy-markets/provincial-territorial-energy-profiles/provincial-territorial-energy-profiles-alberta.html
Canada Energy Regulator (2023). Canada’s Energy Future 2023. Available at: https://www.cer-rec.gc.ca/en/data-analysis/canada-energy-future/2023/canada-energy-futures-2023.pdf.
Carbon Engineering (2021). Direct air capture of CO2. Available at: https://carbonengineering.com/.
CCS Knowledge Centre (2021). Canada’s CO₂ landscape: a guided map for sources and sinks. Canada: CCS Knowledge Centre.
Cecere, G., Corrocher, N., Gossart, C., and Ozman, M. (2014). Lock-in and path dependence: an evolutionary approach to eco-innovations. J. Evol. Econ. 24, 1037–1065. doi: 10.1007/s00191-014-0381-5
Chen, C., and Tavoni, M. (2013). Direct air capture of CO2 and climate stabilization: a model based assessment. Clim. Chang. 118, 59–72. doi: 10.1007/s10584-013-0714-7
Clean Air Task Force (2021). Fact sheet: CO₂ EOR yields a 37% reduction in CO2 emitted per barrel of oil produced. Available at: https://www.catf.us/resource/co2-eor-emission-reduction/.
Clemens, R. T., and Reilly, T. (2014). Making hard decisions with decision tools. Boston, MA: Cengage Learning.
Climeworks (2022). Achieve net zero targets with Climeworks direct air capture. Available at: https://climeworks.com/.
Constitution Act (1982). Canadian charter of rights and freedoms, part 1 of the constitution act, 1982, being schedule B to the Canada act 1982, p. 35.
Cooley, S. R., Klinsky, S., Morrow, D. R., and Satterfield, T. (2023). Sociotechnical considerations about ocean carbon dioxide removal. Annu. Rev. Mar. Sci. 15, 41–66. doi: 10.1146/annurev-marine-032122-113850
Cox, E., and Edwards, N. R. (2019). Beyond carbon pricing: policy levers for negative emissions technologies. Clim. Pol. 19, 1144–1156. doi: 10.1080/14693062.2019.1634509
Cox, E., Spence, E., and Pidgeon, N. (2020). Public perceptions of carbon dioxide removal in the United States and the United Kingdom. Nat. Clim. Chang. 10, 744–749. doi: 10.1038/s41558-020-0823-z
Craik, N., Hubert, A. M., and Daku, C. (2022). The legal framework for carbon dioxide removal in Canada. Alta. Law Rev. 59, 833–870. doi: 10.29173/alr2699
Creutzig, F., Breyer, C., Hilaire, J., Minx, J., Peters, G. P., and Socolow, R. (2019). The mutual dependence of negative emission technologies and energy systems. Energy Environ. Sci. 12, 1805–1817. doi: 10.1039/C8EE03682A
Department of Finance (2022). Budget 2022: A plan to grow our economy and make life more affordable. Government of Canada. Available at: https://www.budget.canada.ca/2022/report-rapport/toc-tdm-en.html.
Dion, J. (2021). Policy implementation will be tricky on carbon capture and storage. Policy Options. Available at: https://policyoptions.irpp.org/magazines/june-2021/policy-implementation-will-be-tricky-on-carbon-capture-and-storage/.
Environment and Climate Change Canada (2022). 2030 emissions reduction plan: Canada’s next steps for clean air and a strong economy. Canada: Environment and Climate Change Canada.
Erans, M., Sanz-Pérez, E. S., Hanak, D. P., Clulow, Z., Reiner, D. M., and Mutch, G. A. (2022). Direct air capture: process technology, techno-economic and socio-political challenges. Energy Environ. Sci. 15, 1360–1405. doi: 10.1039/D1EE03523A
Exner-Pirot, H., and Ignasiak, M. (2023), From shield to sword: The evolution of indigenous economic rights in Canada: MacDonald-Laurier Institute. Macdonald-Laurier Institute. Available at: https://macdonaldlaurier.ca/from-shield-to-sword-the-evolution-of-indigenous-economic-rights-in-canada/.
Fajardy, M., Patrizio, P., Daggash, H. A., and mac Dowell, N. (2019). Negative emissions: priorities for research and policy design. Front. Clim. 1:6. doi: 10.3389/fclim.2019.00006
Fertel, C., Bahn, O., Vaillancourt, K., and Waaub, J. P. (2013). Canadian energy and climate policies: a SWOT analysis in search of federal/provincial coherence. Energy Policy 63, 1139–1150. doi: 10.1016/j.enpol.2013.09.057
Fuhrman, J., Clarens, A., Calvin, K., Doney, S. C., Edmonds, J. A., O’Rourke, P., et al. (2021). The role of direct air capture and negative emissions technologies in the shared socioeconomic pathways towards+ 1.5° C and+ 2° C futures. Environ. Res. Lett. 16:114012. doi: 10.1088/1748-9326/ac2db0
Fuss, S., Lamb, W. F., Callaghan, M. W., Hilaire, J., Creutzig, F., Amann, T., et al. (2018). Negative emissions–part 2: costs, potentials and side effects. Environ. Res. Lett. 13:063002. doi: 10.1088/1748-9326/aabf9f
Gasser, T., Guivarch, C., Tachiiri, K., Jones, C. D., and Ciais, P. (2015). Negative emissions physically needed to keep global warming below 2 C. Nat. Commun. 6, 1–7. doi: 10.1038/ncomms8958
Geels, F. W. (2002). Technological transitions as evolutionary reconfiguration processes: a multi-level perspective and a case-study. Res. Policy 31, 1257–1274. doi: 10.1016/S0048-7333(02)00062-8
Geels, F. W. (2004). From sectoral systems of innovation to socio-technical systems: insights about dynamics and change from sociology and institutional theory. Res. Policy 33, 897–920. doi: 10.1016/j.respol.2004.01.015
Geels, F. W. (2011). The multi-level perspective on sustainability transitions: responses to seven criticisms. Environ. Innov. Soc. Trans. 1, 24–40,
Geels, F. W. (2019). Socio-technical transitions to sustainability: a review of criticisms and elaborations of the multi-level perspective. Curr. Opin. Environ. Sustain. 39, 187–201. doi: 10.1016/j.cosust.2019.06.009
Geels, F. W., and Schot, J. (2007). Typology of sociotechnical transition pathways. Res. Policy 36, 399–417. doi: 10.1016/j.respol.2007.01.003
Geels, F. W., Sovacool, B. K., Schwanen, T., and Sorrell, S. (2017). The socio-technical dynamics of low-carbon transitions. Joule 1, 463–479. doi: 10.1016/j.joule.2017.09.018
Gillingham, K., and Stock, J. H. (2018). The cost of reducing greenhouse gas emissions. J. Econ. Perspect. 32, 53–72. doi: 10.1257/jep.32.4.53
Government of Alberta (2023). Carbon Capture, Utilization and Storage: Developing Storage Hubs to Meet Growing Demand. Available at: https://www.alberta.ca/system/files/custom_downloaded_images/energy-fact-sheet-storage-hub-development.pdf.
Government of Canada (2021). Canada’s enhanced nationally determined contribution. Available at: https://www.canada.ca/en/environment-climate-change/news/2021/04/canadas-enhanced-nationally-determined-contribution.html.
Government of Canada (2022). Canada launches greenhouse gas offset credit system to support a clean, green economy. Available at: https://www.canada.ca/en/environment-climate-change/news/2022/06/canada-launches-greenhouse-gas-offset-credit-system-to-support-a-clean-green-economy.html.
Haley, B. (2011). From staples trap to carbon trap: Canada’s peculiar form of carbon lock-in. Stud. Political Econ. 88, 97–132. doi: 10.1080/19187033.2011.11675011
Hares, R., McCoy, S., and Layzell, D. B. (2022). Review of carbon-dioxide storage potential in Western Canada: blue hydrogen roadmap to 2050. Transit. Accelerator Rep. 4, 1–42,
Haszeldine, R. S., Flude, S., Johnson, G., and Scott, V. (2018). Negative emissions technologies and carbon capture and storage to achieve the Paris agreement commitments. Philos. Trans. R. Soc. A Math. Phys. Eng. Sci. 376:20160447. doi: 10.1098/rsta.2016.0447
Hirschhausen, C. V., Herold, J., and Oei, P. Y. (2012). How a “low carbon” innovation can fail—tales from a “lost decade” for carbon capture, transport, and sequestration (CCTS). Econ. Energy Environ. Policy 1, 115–124. doi: 10.5547/2160-5890.1.2.8
Hodgson, G., and Hodgson, D. (2022). Federal Purchases of direct air capture would help build a viable market. C.D. Howe Institute. Available at: https://www.cdhowe.org/intelligence-memos/hodgson-hodgson-federal-purchases-direct-air-capture-would-help-build-viable.
Honegger, M., Poralla, M., Michaelowa, A., and Ahonen, H.-M. (2021). Who is paying for carbon dioxide removal? Designing policy instruments for mobilizing negative emissions technologies. Front. Clim. 3:996. doi: 10.3389/fclim.2021.672996
IEA (2022). Carbon capture, utilisation and storage. IEA. Available at: https://www.iea.org/reports/carbon-capture-utilisation-and-storage-2 (Accessed August 18, 2022).
IPCC (2022). “Summary for policymakers” in Climate change 2022: Impacts, adaptation, and vulnerability. Contribution of working group II to the sixth assessment report of the intergovernmental panel on climate change. eds. H.-O. Pörtner, D. C. Roberts, E. S. Poloczanska, K. Mintenbeck, M. Tignor, and A. Alegría (Cambridge, UK: Cambridge University Press).
Jebari, J., Táíwò, O. O., Andrews, T. M., Aquila, V., Beckage, B., Belaia, M., et al. (2021). From moral hazard to risk-response feedback. Clim. Risk Manag. 33:100324. doi: 10.1016/j.crm.2021.100324
Keleman, P., Benson, S. M., Pilorgé, H., Psarras, P., and Wilcox, J. (2019). An overview of the status and challenges of CO₂ storage in minerals and geological formations. Front. Clim. 1:9. doi: 10.3389/fclim.2019.00009
Lackner, K. S., and Azarabadi, H. (2021). Buying down the cost of direct air capture. Ind. Eng. Chem. Res. 60, 8196–8208. doi: 10.1021/acs.iecr.0c04839
Larsen, J., Herndon, W., Grant, M., and Marsters, P. (2019). Capturing leadership: Policies for the US to advance direct air capture technology. Rhodium Group. Available at: https://rhg.com/wp-content/uploads/2019/05/Rhodium_CapturingLeadership_May2019-1.pdf.
Lehtveer, M., and Emanuelsson, A. (2021). BECCS and DACCS as negative emission providers in an intermittent electricity system: why levelized cost of carbon may be a misleading measure for policy decisions. Front. Clim. 3:647276. doi: 10.3389/fclim.2021.647276
Lomax, G., Workman, M., Lenton, T., and Shah, N. (2015). Reframing the policy approach to greenhouse gas removal technologies. Energy Policy 78, 125–136. doi: 10.1016/j.enpol.2014.10.002
Mac Dowell, N., Fennell, P. S., Shah, N., and Maitland, G. C. (2017). The role of CO₂ capture and utilization in mitigating climate change. Nat. Clim. Chang. 7, 243–249. doi: 10.1038/nclimate3231
Marcucci, A., Kypreos, S., and Panos, E. (2017). The road to achieving the long-term Paris targets: energy transition and the role of direct air capture. Clim. Chang. 144, 181–193. doi: 10.1007/s10584-017-2051-8
Markusson, N., and Haszeldine, S. (2009). ‘Capture readiness’–lock-in problems for CCS governance. Energy Procedia 1, 4625–4632. doi: 10.1016/j.egypro.2009.02.284
McLaren, D. P., Tyfield, D. P., Willis, R., Szerszynski, B., and Markusson, N. O. (2019). Beyond “net-zero”: a case for separate targets for emissions reduction and negative emissions. Front. Clim. 1:4. doi: 10.3389/fclim.2019.00004
McQueen, N., Gomes, K. V., McCormick, C., Blumanthal, K., Pisciotta, M., and Wilcox, J. (2021). A review of direct air capture (DAC): scaling up commercial technologies and innovating for the future. Prog. Energy 3:032001. doi: 10.1088/2516-1083/abf1ce
Meckling, J., and Biber, E. (2021). A policy roadmap for negative emissions using direct air capture. Nat. Commun. 12, 1–6. doi: 10.1038/s41467-021-22347-1
Minx, J. C., Lamb, W. F., Callaghan, M. W., Fuss, S., Hilaire, J., Creutzig, F., et al. (2018). Negative emissions—part 1: research landscape and synthesis. Environ. Res. Lett. 13:063001. doi: 10.1088/1748-9326/aabf9b
Motlaghzadeh, K., Schweizer, V., Craik, N., and Moreno-Cruz, J. (2023). Key uncertainties behind global projections of direct air capture deployment. Appl. Energy 348:121485. doi: 10.1016/j.apenergy.2023.121485
Muraca, B., and Neuber, F. (2017). Viable and convivial technologies: considerations on climate engineering from a degrowth perspective. J. Clean. Prod. 197, 1810–1822. doi: 10.1016/j.jclepro.2017.04.159
Natural Resources Canada (2023). Canada’s Carbon Management Strategy. Available at: https://natural-resources.canada.ca/climate-change/canadas-green-future/capturing-the-opportunity-carbon-management-strategy-for-canada/canadas-carbon-management-strategy/25337.
Nemet, G. F., Callaghan, M. W., Creutzig, F., Fuss, S., Hartmann, J., Hilaire, J., et al. (2018). Negative emissions–part 3: innovation and upscaling. Environ. Res. Lett. 13:063003. doi: 10.1088/1748-9326/aabff4
Nwanekezie, K., Noble, B., and Poelzer, G. (2022). Strategic assessment for energy transitions: a case study of renewable energy development in Saskatchewan, Canada. Environ. Impact Assess. Rev. 92:106688. doi: 10.1016/j.eiar.2021.106688
Ocean Studies Board and National Research Council (2015). Climate intervention: Carbon dioxide removal and reliable sequestration. Washington, DC: National Academies Press.
O’Riordan, J. (2018). The challenges at the Nexus of Canada's energy and climate change policies. Environ. Sci. Policy Sustain. Dev. 60, 4–17,
Peters, G. P., and Geden, O. (2017). Catalysing a political shift from low to negative carbon. Nat. Clim. Chang. 7, 619–621. doi: 10.1038/nclimate3369
Pozo, C., Galán-Martín, Á., Reiner, D. M., Mac Dowell, N., and Guillén-Gosálbez, G. (2020). Equity in allocating carbon dioxide removal quotas. Nat. Clim. Chang. 10, 640–646. doi: 10.1038/s41558-020-0802-4
Realmonte, G., Drouet, L., Gambhir, A., Glynn, J., Hawkes, A., Köberle, A. C., et al. (2019). An inter-model assessment of the role of direct air capture in deep mitigation pathways. Nat. Commun. 10, 1–12. doi: 10.1038/s41467-019-10842-5
Rickels, W., Proelß, A., Geden, O., Burhenne, J., and Fridahl, M. (2021). Integrating carbon dioxide removal into European emissions trading. Front. Clim. 3:23. doi: 10.3389/fclim.2021.690023
Ritchie, H., and Roser, M. (2020) CO2 emissions. Our World in Data. Available at: https://ourworldindata.org/CO2-emissions.
Roberts, C., Geels, F. W., Lockwood, M., Newell, P., Schmitz, H., Turnheim, B., et al. (2018). The politics of accelerating low-carbon transitions: towards a new research agenda. Energy Res. Soc. Sci. 44, 304–311. doi: 10.1016/j.erss.2018.06.001
Rueda, O., Mogollón, J. M., Tukker, A., and Scherer, L. (2021). Negative-emissions technology portfolios to meet the 1.5° C target. Glob. Environ. Chang. 67:102238. doi: 10.1016/j.gloenvcha.2021.102238
Satterfield, T., Nawaz, S., and St-Laurent, G. P. (2023). Exploring public acceptability of direct air carbon capture with storage: climate urgency, moral hazards and perceptions of the ‘whole versus the parts’. Clim. Chang. 176:14. doi: 10.1007/s10584-023-03483-7
Saunders, B., Sim, J., Kingstone, T., Baker, S., Waterfield, J., Bartlam, B., et al. (2018). Saturation in qualitative research: exploring its conceptualization and operationalization. Qual. Quant. 52, 1893–1907. doi: 10.1007/s11135-017-0574-8
Schenuit, F., Colvin, R., Fridahl, M., McMullin, B., Reisinger, A., Sanchez, D. L., et al. (2021). Carbon dioxide removal policy in the making: assessing developments in 9 OECD cases. Front. Clim. 3:805. doi: 10.3389/fclim.2021.638805
Schot, J., and Geels, F. W. (2008). Strategic niche management and sustainable innovation journeys: theory, findings, research agenda, and policy. Tech. Anal. Strat. Manag. 20, 537–554. doi: 10.1080/09537320802292651
Shackley, S., and Thompson, M. (2012). Lost in the mix: will the technologies of carbon dioxide capture and storage provide us with a breathing space as we strive to make the transition from fossil fuels to renewables? Clim. Chang. 110, 101–121. doi: 10.1007/s10584-011-0071-3
Singh, U., and Colosi, L. M. (2022). Capture or curtail: the potential and performance of direct air capture powered through excess renewable electricity. Energy Convers. Manag. 15:100230. doi: 10.1016/j.ecmx.2022.100230
Slawinski, N., Pinkse, J., Busch, T., and Banerjee, S. B. (2017). The role of short-termism and uncertainty avoidance in organizational inaction on climate change: a multi-level framework. Bus. Soc. 56, 253–282. doi: 10.1177/0007650315576136
Slesinski, D., and Litzelman, S. (2021). How low-carbon heat requirements for direct air capture of CO₂ can enable the expansion of firm low-carbon electricity generation resources. Front. Clim. 3:719. doi: 10.3389/fclim.2021.728719
Sminchak, J. R., Mawalkar, S., and Gupta, N. (2020). Large CO2 storage volumes result in net negative emissions for greenhouse gas life cycle analysis based on records from 22 years of CO2-enhanced oil recovery operations. Energy Fuel 34, 3566–3577. doi: 10.1021/acs.energyfuels.9b04540
Smith, P., Davis, S. J., Creutzig, F., Fuss, S., Minx, J., Gabrielle, B., et al. (2016). Biophysical and economic limits to negative CO₂ emissions. Nat. Clim. Chang. 6, 42–50. doi: 10.1038/nclimate2870
Snyder, H. (2019). Literature review as a research methodology: an overview and guidelines. J. Bus. Res. 104, 333–339. doi: 10.1016/j.jbusres.2019.07.039
Sovacool, B. K., Baum, C. M., and Low, S. (2022). Determining our climate policy future: expert opinions about negative emissions and solar radiation management pathways. Mitig. Adapt. Strateg. Glob. Chang. 27:58. doi: 10.1007/s11027-022-10030-9
Strefler, J., Bauer, N., Humpenöder, F., Klein, D., Popp, A., and Kriegler, E. (2021). Carbon dioxide removal technologies are not born equal. Environ. Res. Lett. 16:074021. doi: 10.1088/1748-9326/ac0a11
Temple, J. (2023). The US just invested more than $1 billion in carbon removal. MIT Technology Review. Available at: https://www.technologyreview.com/2023/08/11/1077756/the-us-just-invested-more-than-1-billion-into-carbon-removal/.
The Globe and Mail . (2023). Globe editorial: Pierre Poilievre’s conservatives still don’t have a viable climate plan. The Globe and Mail. Available at: https://www.theglobeandmail.com/opinion/editorials/article-pierre-poilievres-conservatives-still-dont-have-a-viable-climate-plan/.
Tuttle, R. (2022). More than 400 academics urge Canada to ditch carbon capture tax credit. Financial Post. Available at: https://financialpost.com/commodities/energy/academics-urge-canada-to-ditch-carbon-capture-tax-credit-letter.
U. S. Department of Energy (2021). DOE announces $14.5 million supporting direct air capture and storage coupled to low-carbon energy sources. Available at: https://www.energy.gov/articles/doe-announces-145-million-supporting-direct-air-capture-and-storage-coupled-low-carbon.
Unruh, G. C. (2000). “Understanding carbon lock” in Energy policy, vol. 28 (Amsterdam, Netherlands: Elsevier), 817–830.
Valiaho, B. H. (2020). Importance of CCS hubs. International CCS Knowledge Centre. Available at: https://ccsknowledge.com/blog/importance-of-ccs-hubs.
Van der Vleuten, E. (2006). “Understanding network societies: two decades of large technical system studies” in Networking Europe Transnational infrastructures and the shaping of Europe (Cambridge, England: Science History Publications), 279–314.
Warren, F., and Lulham, N. (2021). Canada in a changing climate: National issues report. Ottawa, ON: Government of Canada.
Webb, R., and Gerrard, M. (2021). The legal framework for offshore carbon capture and storage in Canada. Sabin Center for Climate Change law. Columbia Law School: Columbia Public Law Research Paper Forthcoming.
Weber, B. (2022). Alberta first nations seek consultation, benefits from Oilsands carbon storage plans CBC news. CBC News. Available at: https://www.cbc.ca/news/canada/edmonton/alberta-first-nations-seek-consultation-benefits-from-oilsands-carbon-storage-plans-1.6481711.
Williams, E. (2022). The economics of direct air capture and storage. Melbourne: Global CCS Institute.
Wohland, J., Witthaut, D., and Schleussner, C. F. (2018). Negative emission potential of direct air capture powered by renewable excess electricity in Europe. Earth's Future 6, 1380–1384. doi: 10.1029/2018EF000954
Wohlin, C. (2014). Guidelines for snowballing in systematic literature studies and a replication in software engineering. In: Proceedings of the 18th international conference on evaluation and assessment in software engineering, pp. 1–10.
World Resources Institute (2021). Climate watch historical GHG emissions. Washington, DC: World Resources Institute. Available at: https://www.climatewatchdata.org/ghg-emissions.
Keywords: carbon dioxide removal, direct air capture, negative emissions, climate policy, low-carbon transition, socio-technical system, multi-level perspective
Citation: Cortinovis SR, Craik N, Moreno-Cruz J, Motlaghzadeh K and Schweizer V (2024) Scaling carbon removal systems: deploying direct air capture amidst Canada’s low-carbon transition. Front. Clim. 6:1338647. doi: 10.3389/fclim.2024.1338647
Received: 15 November 2023; Accepted: 24 April 2024;
Published: 20 May 2024.
Edited by:
Shinichiro Asayama, National Institute for Environmental Studies, JapanReviewed by:
Wil Burns, American University, United StatesCopyright © 2024 Cortinovis, Craik, Moreno-Cruz, Motlaghzadeh and Schweizer. This is an open-access article distributed under the terms of the Creative Commons Attribution License (CC BY). The use, distribution or reproduction in other forums is permitted, provided the original author(s) and the copyright owner(s) are credited and that the original publication in this journal is cited, in accordance with accepted academic practice. No use, distribution or reproduction is permitted which does not comply with these terms.
*Correspondence: Stephanie Rose Cortinovis, c3Jjb3J0aW5AdXdhdGVybG9vLmNh
Disclaimer: All claims expressed in this article are solely those of the authors and do not necessarily represent those of their affiliated organizations, or those of the publisher, the editors and the reviewers. Any product that may be evaluated in this article or claim that may be made by its manufacturer is not guaranteed or endorsed by the publisher.
Research integrity at Frontiers
Learn more about the work of our research integrity team to safeguard the quality of each article we publish.