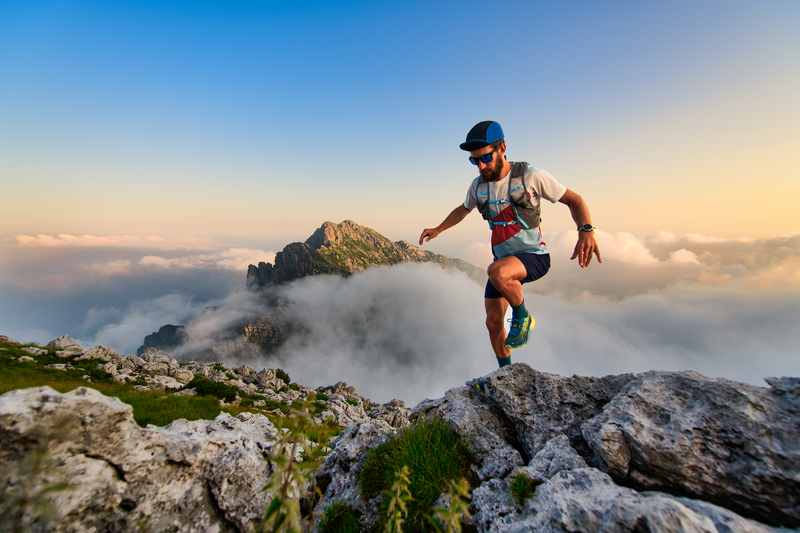
95% of researchers rate our articles as excellent or good
Learn more about the work of our research integrity team to safeguard the quality of each article we publish.
Find out more
ORIGINAL RESEARCH article
Front. Clim. , 22 September 2023
Sec. Carbon Dioxide Removal
Volume 5 - 2023 | https://doi.org/10.3389/fclim.2023.1207668
Carbon mineralization and storage in basaltic rock sequences is a developing technology but faces challenges with uptake and increases in scale. Northern Ireland (UK) is a useful analog for many parts of the world where thick basalt sequences could be used to aid in reaching carbon reduction and removal targets. Here I reanalyze and reinterpret available lithological, geochemical, and geophysical data to assess carbon storage potential. The physical and geochemical properties of the basalts are indistinguishable from those used for successful carbon sequestration in Iceland and Washington State (USA). Based on the thickness, composition, and potential permeability, I propose that this is a viable location for a series of small-volume stores (total volume ~9–12 MTCO2) suitable for capture at industrial point-sources or purpose-built CO2 “harvesting” facilities. The case for exploiting the CO2 storage potential in Northern Ireland is strengthened by (1) an increasingly urgent need to find socially and economically just decarbonization pathways needed to meet NI's targets, (2) increasing realization among policy experts that point-source CO2 capture and industrial decarbonization will be insufficient to meet those goals, due in part, to the size of the agricultural sector, and (3) the coincidence with plentiful renewable energy and geothermally-sourced industrial heat. These serendipitous relationships could be leveraged to develop CO2-“farms” where direct air capture operations are supplied by renewable energy (biomass and geothermal) and on-site geological storage. I envisage that these sites could be supplemented by CO2 from locally produced biomass as farmers are encouraged to transition away from raising livestock. Because CO2 can be captured directly from the atmosphere or via suitable biomass anywhere, NI's small size and position on the periphery of the UK and Europe need not be a disadvantage. Instead, NI's access to geological storage, renewable energy, and agricultural land may be a boon, and provide new opportunities to become a leader in carbon removal in basalt-covered regions.
The 2022 IPCC report stated explicitly that in addition to dramatic curbs on carbon dioxide (CO2) emissions, CO2 extraction from the atmosphere is now essential to limiting global climate change (Babiker et al., 2022). Direct-Air Capture with Carbon Storage (DACCS) and Bioenergy with Carbon Capture and Storage (BECCS) are two of the principal technologies expected to assist in removing atmospheric CO2 (e.g., Gough et al., 2018; Gambhir and Tavoni, 2019; Ozkan, 2021), and as such contribute toward United Nations Sustainable Development Goal 13—Climate Change (Mikunda et al., 2021). Rapid progress toward capturing atmospheric CO2 at gigaton-scale will necessitate a commensurate expansion of geological CO2 storage. Whereas, conventional geological CO2 storage is optimized for CO2 capture at industrial point-sources (e.g., refineries), direct-air capture (DAC) and bioenergy systems can be deployed almost anywhere, especially in areas with abundant renewable energy, as long as there is CO2 storage availability. Therefore, there is strong incentive to broaden the geological diversity and geographic range of CO2 storage options to support DAC and carbon-neutral bioenergy deployment (Sovacool et al., 2022), especially away from industrial clusters.
Moreover, although enormous volumetric potential of mafic rocks has been reported prominently (e.g., Goldberg et al., 2008; Goldberg and Slagle, 2009), commercial storage has only been pursued in Iceland. To aid expansion on unconventional storage, NI makes a useful case study for other regions underlain by thick basalt successions and without alternative geological carbon storage options [e.g., India, Kenya (Okoko and Olaka, 2021)] and many volcanic islands [e.g., Azores, Canary Islands, Cape Verde, Comoros, Hawaiian islands, Kerguelen (Goldberg et al., 2013), Reunion]. Developments of DACCS and BECCS in these locations will, in part, depend on the availability of CO2 storage.
This paper examines the potential for non-conventional geological storage of CO2 in basalt lavas in Northern Ireland (NI), using a combination of reanalysis and reinterpretation of legacy geoscience data and literature. Finally, it outlines one possible pilot program scenario.
There are several different geological CO2 storage approaches in use or in development worldwide (e.g., Martin-Roberts et al., 2021). Of these, only CO2 sequestration into (1) deep saline aquifers and depleted oil and gas reservoirs in sedimentary basins, and (2) shallow volcanic rocks are likely to be appropriate in NI (Bryden Centre, 2021) due to geological constraints. Sequestration into coalbeds, mine tailings, and industrial wastes is not considered herein.
Storage in sedimentary basins, especially those already characterized during oil and gas exploration, is attractive for several reasons (Benson and Cole, 2008):
• very large potential capacities (0.1–100 GtCO2 per basin);
• proven and available technologies to inject and monitor CO2 in the deep subsurface (e.g., Hannis et al., 2017);
• reliable and long-duration (>10,000-year) CO2 trapping mechanisms (e.g., Alcalde et al., 2018); and
• relatively well-constrained operational cost estimates (e.g., Benson and Deutch, 2018).
Therefore, this approach has been and continues to be studied intensively. However, there are several important disadvantages associated directly or indirectly with these approaches (e.g., Hannis et al., 2017). Primarily, the scale of new projects in non- or poorly-characterized sedimentary basins is daunting; requiring large investments in surveys, test drilling, and modeling of subsurface stratigraphy, structure, fluid flow, and volume estimates (e.g., Bachu et al., 2007). Injection into deep reservoirs requires high hydrostatic pressures to displace the in situ brines and this can, if the strength of the rocks is exceeded, fracture the reservoir or adjacent layers causing induced seismicity. The displaced brine must be recovered at the surface and disposed of safely, usually by reinjection into a different, under-saturated reservoir. These issues are most serious for deep saline aquifers where there is little or no data and infrastructure compared to oil and gas fields (Hannis et al., 2017).
There are additional disadvantages to sequestering CO2 into depleted oil and gas reservoirs like those proposed off the southern coast of the RoI (e.g., Kinsale gas field; Lewis et al., 2009). Foremost among these are the condition and pressure of the reservoir, and the age of infrastructure. Deep saline aquifers and other geological storage scenarios do not have this major limitation, but require sustained monitoring of the CO2 plume.
Mineral carbonation (Lackner et al., 1995; Oelkers et al., 2008) of mafic volcanic rocks like basalt in the subsurface was first proposed by McGrail et al. (2003, 2006) and Goldberg et al. (2008), and has subsequently been demonstrated to be an effective, efficient, and safe process at two pilot projects: CarbFix, Hellisheiði, Iceland, and Wallula, WA, U.S.A. (McGrail et al., 2011). Based on the CarbFix-Climeworks DACCS project at Hellisheiði, injection and in situ CO2 mineralization (i.e., not including the cost of CO2 capture) can be achieved at ~US$20−30/metric tonCO2, competitive with conventional geological storage (Keleman et al., 2019).
Carbonation in basalts takes advantage of pH-swings and the strong attraction between divalent cations (e.g., Mg2+) dissolved from the crystalline and amorphous (i.e., volcanic glass) rocks, and CO dissolved in water (CO2aq) to precipitate stable carbonate minerals (e.g., Oelkers et al., 2008). At Carbfix, CO2aq and water are combined to form carbonic acid (H2CO3) by the reactions:
Injected carbonic acid leaches silicate phases in the host basalt; releasing divalent cations that bind with carbonate anions (Equation 1c) and buffer the pH. These reactions occur readily in nature between CO2aq and mafic rocks (e.g., Dessert et al., 2003), and precipitate thermodynamically favorable carbonate mineral phases that are stable and solid over millions of years (Raza et al., 2022 and references therein).
Alternatively, injection of supercritical CO2 will also lead to mineral carbonation but much more slowly; the modes of CO2 introduction, and mineral alteration and precipitation are yet to be fully understood or optimized (Schaef et al., 2009, 2010, 2011). Understanding the rates of carbonation at an injection site is important for estimating the total CO2 volume potential as mineral carbonation adds ~20% over the volume stored in the available pore space alone.
The overwhelming majority of carbonate mineralization studies focus on the necessary geochemical processes and their rates (see Raza et al., 2022), while the geological and geophysical properties of the host basalts is much less thoroughly examined. Successful mineral carbonation depends on being able to inject CO2aq where it can reach and react with sufficient volumes of the host rock. This requires that the basalt formations are, at least locally, highly porous and permeable, and have large surface areas available for reactions. Basalt lava piles are widely recognized as voluminous aquifers in many regions, including western India (Limaye, 2010), southern Ethiopia (McKenzie et al., 2001), and southern Brazil (Navarro et al., 2020); however, flow properties (e.g., flow rate) and the spatial distribution of springs are often heterogeneous and unpredictable. A general model of basalt lava aquifers (e.g., Kulkarni et al., 2000) emphasizes the importance of highly permeable, horizontal joint systems within coherent lavas, and horizontal, inter-lava breccia layers in controlling the lateral transport of fluids. Transmission across otherwise low permeability coherent lavas is primarily along vertical or sub-vertical faults and fractures, including cooling joints.
Basalt rock is intrinsically variably permeable in proportion to the volume, size, and interconnectedness of the pores, whether they are intracrystalline (e.g., diktytaxitic texture) or contain vesicles (i.e., fossil bubbles). Porosity values measured in basalt samples typically range from 0 to ~85% (Navarro et al., 2020); with intracrystalline porosities of 0 to ~14% (e.g., Franzson et al., 2001). However, total porosity is moderated by the precipitation and dissolution (i.e., paragenesis) of secondary phases like zeolites and silica (Neuhoff et al., 1999). Permeability is estimated from measured porosity with the power-law Kozeny-Carman model (e.g., Navarro et al., 2020). Calculated bulk permeabilities vary over several orders of magnitude (100-104 mD; 10−10-10−17 m2; e.g., Navarro et al., 2020), with measured intrinsic permeability of avesicular basalt ranging from (100-101 mD; e.g., Franzson et al., 2001).
Most porosity and permeability estimates do not adequately account for fractures within the host rocks, sedimentary, igneous, or volcanic. For example, fractured, low porosity igneous rocks, are often very permeable (additional ≤ 103 mD from fractures) and productive, if heterogeneous, aquifers (e.g., Goldberg and Burgdorff, 2005) and are known to locally host significant volumes of hydrocarbons that have migrated into the fracture network (e.g., Rogers et al., 2006). Basalts are routinely highly fractured compared to many sedimentary reservoir rocks (e.g., Nara et al., 2011), by both sub-vertical (e.g., columnar joint), oblique, and sub-horizontal (sheet joint) sets, even in the absence of faulting. Fractures in basalt lavas are typically variably spaced and of variable length and connectivity, therefore, the fracture permeability is highly heterogeneous through a single lava. Fracture surfaces in basalt reservoirs are expected to be highly reactive to CO2 and therefore fractures are likely to seal rapidly and pose less risk of CO2 leakage than sedimentary reservoirs.
Carbon capture and storage is now widely accepted as a set of necessary carbon dioxide removal technologies (Kearns et al., 2021) to arrest global temperature rise and to maintain temperatures within tolerable limits by the end of this century (Galán-Martín et al., 2021; Babiker et al., 2022). The United Kingdom government's commitment to aggressive greenhouse gas (GHG) reductions to 78% of 1990 levels (806 MtCO2e) by 2035 requires a holistic approach to decarbonization of the whole economy, including carbon removal technologies like DACCS and BECCS (García-Freites et al., 2021). The UK's primary strategy is to develop four Carbon Capture Utilization and Storage (CCUS) “clusters” around existing concentrations of energy-intensive manufacturing, steel making, and petrochemical industries, often centered on large ports, by 2030 (BEIS, 2021). This strategy is projected to reduce total UK CO2 emissions to ~50 MtCO2e by 2050. Moreover, successful clustering of multiple CO2 sources may make transport to distal geological stores more economic (Bielicki, 2009). The alternative of a distributed CCUS network across the UK achieves the same target by 2050 with lower residual (i.e., unabated) CO2 emissions, but is considerably more expensive and challenging (BEIS, 2021). There is currently no tax incentive program (cf. 45Q) in the UK to encourage CCS.
As a constituent part of the UK, NI shares this responsibility but currently lags behind the rest of the UK and the neighboring Republic of Ireland (RoI). Furthermore, the rate of GHG and CO2 reduction is decreasing as the easiest abatements are implemented first, and the proportion of industries difficult to decarbonize increases. The NI Executive, through the Department of Agriculture, Environment, and Rural Affairs, has legislated very substantive cuts in GHG and specifically CO2 emissions in the 2020's and 2030's to achieve net-zero by 2050 (DAERA, 2022), and to do so through innovation and science-based decision-making in conjunction with the UK and RoI governments. The UK's Climate Change Committee (CCC) reported that to achieve net-zero by 2050, NI will have to exceed its “fair contribution” to the UK's Net Zero target of 83% reduction from 1990 levels (CCC, 2020, 2022). As of April 2023, NI was falling increasingly behind in reaching this ambitious target (CCC, 2023).
NI's GHG emissions in 2020 were 21 MtCO2e of which 68% (14.6 MtCO2) was CO2 (DAERA, 2023). Agriculture is the major contributor at 28% which is a far greater proportion than the UK average of 10% (1990–2020; CCC, 2023). In 2019, 31 CO2 point sources were recorded in NI in the National Atmospheric Emissions Inventory (Figure 1), ranging from 0.13 to 281 ktCO2/yr (total 0.95 MtCO2/yr; mean 30.8 ktCO2/yr; median 3.1 ktCO2/yr). Of these, only 5 sites exceeded 20 ktCO2/yr: 3 thermal power plants (Ballylumford, Coolkeeragh, Kilroot), 1 cement plant (Cookstown), and 1 chemical products plant (Maydown). The greatest proportion of the list is made up of food processing plants (n = 13) that total 39 ktCO2. It is unlikely that any of the emissions from these CO2 sources are being captured currently. The dispersed nature of relatively small-volume (<0.25 MtCO2/yr) point sources, as opposed to industrial clusters, poses a significant problem to effectively capturing, transporting, and storing CO2 in Northern Ireland and many less-intensively industrialized parts of Europe (Tamme and Beck, 2021). Conditions in NI are not addressed in the UK's industrial decarbonization strategy (BEIS, 2021), nor is it considered in depth in the UK CO2 Storage Appraisal.
Figure 1. Distributions of the 31 major CO2 emission point-sources and 91 bioenergy plants in Northern Ireland. CO2 emitters are color-coded by the industrial sector and scaled by 2019 emissions volumes (metric tones per year); the largest CO2 emitters are named. Bioenergy plants (anerobic digestion and biomass combined) are scaled by power production (kWe). Inset: location of Northern Ireland (NI) within the United Kingdom (UK) on the northwestern edge of Europe. UTM zone 29U; data from DAERA, OSNI Open Data, Irish Bioenergy Association, and NNFCC Consulting.
The RoI has a nearly identical CO2 emissions breakdown with transport dominating followed by more or less equal contributions from power generation, residential, land use, and business (SEAI, 2021). The RoI shares similar problems with the distribution of major CO2 emitters and a lack of well-characterized storage options (SEAI, 2022), but has similar potential for DAC (Casaban and Tsalaporta, 2022). Therefore, holistic assessments of CCUS potential for NI usually include an all-Ireland approach, following the examples of the all-Ireland electricity and natural gas networks, and future hydrogen networks (Van Rossum et al., 2022).
The absence of suitable CO2 storage and the expense of transportation to England or Scotland are recognized as serious impediments to carbon capture efforts in NI (CCC, 2020). However, as NI falls further behind its mandated net zero target, the need for expanded carbon capture increases, the alternative being punitive reductions in the agricultural sector. The situation is serious enough that the CCC has recently taken the unprecedented step of recommending DACCS and BECCS for NI and emphasizing the need for creative solutions to the local CO2 storage problem (CCC, 2023). NI's first DAC pilot (100 tonCO2/yr) was announced in January 2022 where SMART-DAC and B9 Energy will seek to utilize the CO2 rather than store it (BEIS, 2022).
CCUS policy is not devolved to the NI Assembly, which may impact on its development in the region. However, NI is included in two examinations of CCS adoption: one all-Ireland and one looking at NI only. The earliest assessment (Lewis et al., 2009) examined scenarios with integrated carbon capture and offshore geological storage. Scenario 3 focused on a new power plant at Kilroot (Figure 1), designed to capture CO2 and to then transport it by pipeline to a deep saline aquifer in the Sherwood Sandstone Group within the Portpatrick Basin (>37 MtCO2 capacity) between NI and Scotland. The report concluded that scenario 3 would be a very high-risk and expensive project because of the lack of detailed knowledge of the Portpatrick Basin, the relatively small size of the potentially suitable geological trapping structures, and the absence of existing infrastructure. Pre-development studies were estimated to be €100 million over 10 years. Lewis et al. (2009) concluded that an additional ~1.9 GtCO2 of plausible storage capacity was likely to exist in the Sherwood Sandstone Group beneath County Antrim.
The only comprehensive CCUS assessment for NI (Bryden Centre, 2021) determined that the lack of well-characterized geological CO2 storage was one of several major impediments, along with cost and the wide spatial distribution of small- and medium-sized point sources that would need to be linked together by a pipeline costing approximately £42 million. The report concluded that only the power generation sector is likely to be able to take advantage of carbon capture and geological storage, and even then, CO2 will likely have to be shipped to the North Sea or eastern Irish Sea, adding to the cost and embedded carbon footprint from shipping. However, this report, like others for the UK and RoI, emphasized offshore storage solutions based on known depleted oil and gas fields or deep saline aquifers. The Bryden Centre (2021) report acknowledged the alternative of CO2 storage by mineralization in volcanics rocks that are being exploited simultaneously for geothermal energy, using the Hellisheiði geothermal power plant and CarbFix project as an example (Matter et al., 2009, 2011; Gíslason et al., 2018). Geothermal potential exists in NI (Raine, 2021); however, the Bryden Centre's report concluded that until it was proven and advanced to project development, CCUS co-location would be delayed.
Volcanic rocks in NI may have potential for geological storage of CO2; specifically, the Antrim Lava Group basalts (ALG). The geology of the ALG is documented thoroughly in Mitchell (2004), and is only summarized briefly herein.
The ALG (61–58 Ma) is an erosional remnant of an originally much more extensive flood basalt succession within the Paleocene North Atlantic Igneous Province, centered in County Antrim and around Lough Neagh (Figure 2A), and is the largest contiguous basalt unit in northwestern Europe outside Iceland. The ALG overlies a regionally-extensive unconformity developed primarily in the Cretaceous Ulster White Limestone Formation. The ALG is buried by thick, Oligocene clay and silt deposits (Lough Neagh Group–LNG; Parnell et al., 1989), locally with lignite, under and around Lough Neagh, and everywhere by varying thicknesses (1–30 m) of Quaternary sediments, including glacial deposits (Mitchell, 2004).
Figure 2. (A) Bedrock geology map of the south Antrim—Lough Neagh area showing the extents of the Antrim Lava Group basalts (ALG; pinks) and overlying Lough Neagh Clays (LNC; beige). Minimum thicknesses of ALG (black-filled circles) come from named boreholes. Geological data from GSNI (2020) GeoIndex. (B) Measured stratigraphic sections from five boreholes around Lough Neagh (this study, Figure 3). Data compiled from Patterson et al. (1956), GSNI (1958), Thompson (1979), Wilson (1983), McCann (1991), Naylor et al. (2003), and Quinn (2006).
The ALG is divided into three major stratigraphic units (Figure 2B). The Lower Basalt Formation (LBF) is the most extensive unit within the ALG, and is overlain unconformably by the lateritic Interbasaltic Formation (IBF; 0–27 m-thick) everywhere except in north Antrim. The IBF is overlain by the laterally discontinuous Upper Basalt Formation (UBF) in eastern Londonderry, north and east Antrim (Figure 2A), and around Lough Neagh, including under the LNG. The LBF and UBF basalt lavas are petrographically and geochemically indistinct, and are similar in morphology and physical volcanology (e.g., Lyle, 1988; Lyle and Patton, 1989). Individual lavas are typically ~10 m-thick, laterally continuous over several kilometers, and composed of a columnar-jointed core of avesicular or low vesicularity basalt that grades upwards and downwards into moderately to highly vesicular basalt and basaltic breccia. The tops of individual lava flow-units are distinguished by red-weathering oxidation of the upper breccia, often together with red or orange lithomarge (syn. “bole”) where the original crystalline texture of the basalt has been totally replaced by clay minerals.
Published whole-rock data for ALG basalts show they are plagioclase-clinopyroxene-olivine tholeiites, with few basanites and picrites, with 4–22 wt. % MgO and 7.75–11.5 wt. % CaO (Figure 3), and 13–30 wt. % total MgO and CaO. There is no significant correlation between stratigraphic position and composition.
Figure 3. ALG basalts are intermediate between those of lavas at the Hellisheiði, Iceland (n = 18) and Wallula, WA, U.S.A. (n = 124) carbon storage sites. The composition of the Stapafell lava, Iceland, (Oelkers and Gíslason, 2001) is shown for reference.
What is known about the ALG in the subsurface comes from less than 30 exploration boreholes (e.g., Wilson, 1983), regional-scale geophysical surveys (e.g., Mitchell, 2004), and by extrapolation of outcroppings in large seacliffs and escarpments (e.g., Lyle, 1988). Based on the available geophysical and borehole data I have identified four areas of interest (AOIs) to concentrate upon: north, east, south, and west of Lough Neagh (Figure 4).
Figure 4. Geophysical data (GSNI, 2022). (A) Radiometric (natural gamma; NGR) survey showing K-U-Th-poor basalts in green, and K-U-Th-rich lithologies in yellows and reds. Areas of basalt buried beneath K-rich Lough Neagh Clay are characterized by green and yellow stippling. The large blue area is Lough Neagh. (B) Residual aeromagnetic data showing the strongly magnetic basalts characterized by a high-frequency (stippled) pattern. Older, non-magnetic rocks and the Lough Neagh Clays are characterized by very low frequency patterns.
Airborne geophysical surveys aid in constraining the buried margins of the ALG around Lough Neagh, and in estimating its thickness. Radiometric (natural gamma radiation; NGR) differentiates K-U-Th-poor rocks like basalt from other rock types (Figure 4A), especially K-rich clay-rich sediments like the LNC and evolved volcanic rocks like rhyolite. The ALG basalts in County Antrim define a uniform region of low NGR (greens and pale blues).
Whereas, small and thin LNC outliers immediately east of Lough Neagh are well-resolved (e.g., Langford Lodge; Figure 2A), the basalts and LNC are poorly defined south and west of Lough Neagh (Figure 4A). West of Lough Neagh, where boreholes demonstrate that the LNC is thick (>100 m), the NGR pattern is almost uniform, suggesting a uniformly thick LNG cover suppressing the ALG signature despite the basalts being >200 m-thick here (Figure 2B). South of Lough Neagh the NGR pattern is stippled with the presence of low NGR values similar to those of the unburied basalts suggesting that the LNG is thin here, possibly inconsistently so.
Tell us aeromagnetic data (residual field) distinguishes the ALG basalts from all other lithologies by a very high amplitude and high frequency (closely spaced red-blue-red) pattern (Figure 4B). The strong magnetic signature reflects the abundance of magnetite in the basalts. The ALG south of Lough Neagh is best defined by this method. The extent of the LNG south of and underneath Lough Neagh is defined by large, low amplitude (i.e., smooth) regions in red or blue, and small, moderate-amplitude areas in yellows and greens.
The only published estimates of the thickness of the ALG are Shelton's (1997) isopachs for the LBF that show it thickening toward Lough Neagh from the north and east (Figure 5), and outcrops around the northern and eastern margins. The total thickness of the ALG is only constrained in around Lough Neagh by four boreholes (Figure 2B). All contain different thicknesses of LBF (232–531 m) and penetrate into the underlying Ulster White Limestone Formation. The Langford Lodge (total ALG thickness 788 m) and Ballymacilroy (773 m) boreholes contain about one half to one third UBF (241–346 m), respectively. The Mire House (232 m) and Ballynamullan (463 m) boreholes do not contain any UBF; the LBF here is buried beneath 298 m of LNG sediments.
Figure 5. Isopachs (in meters) of the LBF adapted from Shelton (1997), the locations of boreholes that penetrate through the ALG, and the total ALG thickness in meters. Note the absence of data in the SE and NW to corroborate (Shelton, 1997).
The Ballymacilroy borehole (Figure 2) was chosen to reexamine and characterize a continuous, complete section through the ALG basalts so as to constrain the variation in composition and physical properties. The borehole was cored and logged between January and March 1979, with drill cuttings collected and analyzed in ~30 cm increments (Thompson, 1979). The borehole finished at 2,271.98 m below surface, of which the uppermost 773 m are ALG. Most other ALG penetrating boreholes do not have the same quality or continuity of data. To maintain consistency, all physical property values reported from hereon were measured in the Ballymacilroy borehole.
The ALG (Figure 6) at Ballymacilroy includes the Upper Basalt Formation (UBF; 346 m-thick), the Interbasaltic Formation (IBF; 6 m), and the Lower Basalt Formation (LBF; 422 m). There is a 37 m-thick zone of alternating basalt and rhyolite within the UBF (292–329 m below surface), and an 5 m-thick laterite and 8 m-thick rhyolite layer within the LBF (609–622 m below surface). The lithological boundaries are estimated from prominent breaks in physical properties, especially density and resistivity, and confirmed by identification of drill cuttings (Thompson, 1979).
Figure 6. New lithological and petrophysical logs generated from re-analysis of the Ballymacilroy borehole data. Left to right: (1) lithologies (colors from Figure 2) showing flow units identified in the petrophysical data. “C” indicates a compound flow unit. (2) Proportion of zeolites estimated from the core descriptions (this study). (3) Density; (4) resistivity; (5) NGR; (6) sonic velocity. (7) Black—measured neutron-porosity; red—porosity calculated from sonic velocity (this study). (8) Black—permeability calculated from measured neutron-porosity; red—permeability calculated from “sonic porosity” (this study). See text for details of the porosity and permeability calculations.
The UBF is characterized by strong, 10–20 m-thick, sawtooth patterns in density, resistivity, and sonic transit time logs (Figure 6), and uniformly low natural gamma radiation (NGR <15 API). The uppermost 30 m logged in the UBF shows anomalously high NGR and highly variable sonic velocity and resistivity, consistent with a zone of strong paleosurface weathering (e.g., Ruffell, 2016). The UBF rhyolite-basalt layer is not distinctive in the petrophysical data, and is defined by rhyolite cuttings only. Amygdule abundance in drill cuttings ranges from 0–20%. The IBF is characterized by low density (<2 g/cm3) and resistivity (~20 Ω.m2/m), and high NGR (50–75 API) and sonic transit time (~150 μs/ft). This is consistent with the kaolinite-rich and thorium-rich laterite horizons and uranium-rich iron crusts exposed throughout the IBF (Smith and McAlister, 1995; Ruffell, 2016).
The LBF is characterized by low NGR (<15–20 API) and moderate to high density (~2.2–2.8 g/cm3). The LBF forms three zones (Figure 6), from top down: (i) a ~70 m-thick (422–~490 m below surface) very high frequency and amplitude sawtooth-pattern zone; (ii) a ~90 m-thick (~490–609 m) zone similar to the UBF; and (iii) a ~111 m-thick (~633–774 m) zone with homogeneous density, anomalous resistivity and NGR, and a distinctly amygdule-rich (20–50%) upper half.
The LBF upper zone is characterized by rapidly alternating (~10 m interval) highs (2.7–2.8 g/cm3, 1,000 Ω.m2/m, >100 μs/ft) and lows (2.1–2.4 g/cm3, 50–100 Ω.m2/m, 50–75 μs/ft) in density, resistivity, and sonic velocity. The lowermost zone is distinct with significantly lower resistivity (<100 Ω.m2/m) and slightly elevated natural gamma radiation (15–20 API). The upper half is very amygdule-rich, up to 50%, corresponding to higher sonic transit times and lower density and resistivity. The thin laterite and rhyolite lava within the middle zone of the LBF are not distinctive in the geophysical logs.
Porosity values vary from 0% to ~85% through the lavas in the Ballymacilroy borehole (Figure 7A). Neutron-porosity measurements range from 0% to 65% with a median of 33%. Porosity was calculated from the sonic transit-time data (“sonic porosity”) also, using the Raymer-Hunt-Gardner formula (Raymer et al., 1980):
where α is a fitting parameter of −0.8, tmat is a lithological constant of 80 μs/ft, and tlog is the measured sonic transit-time. The sonic porosity ranges from 0% to 84% with a median of 42%.
Figure 7. Ballymacilroy borehole petrophysical properties (n = 4,374; Thompson, 1979). Intracrystalline fields from the Öskjuhlið olivine tholeiite lava, Reykjavik, Iceland (Franzson et al., 2001). (A) Box and whisker plots of porosity measured by neutron logging and calculated from the sonic log (“sonic calc.”). (B) Plots of permeability calculated from neutron porosity (“neutron calc.”) and calculated from the calculated sonic porosity (“sonic calc.”). (C) Cross-plot of porosity and permeability.
I calculated the relationship between porosity and permeability as measured in scoriaceous basalts by Saar and Manga (1999). Rearranged, this allows for estimation of permeability from both the neutron-porosity and sonic porosity values, where:
Ballymacilroy lavas with >20% porosity have permeabilities of 101-104 mD (10−15-10−12 m2; Figure 7B). Below 10% porosity, the permeability is variable and typically very low (101 mD; Figure 7C; Saar and Manga, 1999).
The pre-requisite conditions for CO2 storage in basalts are: high porosity, high permeability, and abundant Ca2+ and Mg2+. To be feasible, a site must also have sufficient reservoir volume available for meaningful storage. Finally, the CO2 phase being injected influences the depth of storage and the abundance of water needed; however, this is largely dependent on engineering and economic concerns related to how CO2 is captured (e.g., purity) and transported on the surface. Supercritical CO2 (CO2SC) requires a higher pressure system and storage at greater depths (e.g., Wallula; >800 m depth) than a CO2aq system (e.g., Carbfix; >350 m depth; Gíslason et al., 2018).
Immediately north and east of Lough Neagh, and probably beneath much of Lough Neagh, the ALG is thick enough (500–800 m) to facilitate storage in the LBF (Figure 2) at depths equivalent to those successfully exploited in the Carbfix project with CO2aq but not with CO2SC injection as at Wallula. In addition, there is potential in the southwest of Lough Neagh (e.g., Washing Bay; Figure 2) where, although the ALG is thinner (>200 m) it is buried beneath 350 m of LNC sedimentary rocks. Therefore, at all potential sites the preferred injection mechanism is likely to be as CO2aq and an abundant water supply needs to be guaranteed. The hydrogeology of the deep aquifers within and beneath the ALG is very poorly constrained, largely due to the lack of deep water wells. However, the abundant rainfall in the region (~700–1,700 mm/yr) and the presence of Lough Neagh (~3.5 km3) should provide abundant surface water if access is granted.
The dissolution of basaltic glass and crystalline groundmass, and the mineralization of carbonate are rate-limited by the presence of clay minerals (e.g., Oelkers and Gíslason, 2001), and these are also likely to lower permeability. Clay minerals are known to occur in abundance in the IBL and the many red weathering horizons characteristic of the tops of individual lavas (e.g., Smith and McAlister, 1995). Petrographic descriptions of ALG basalts do not typically report significant clay abundances (Lyle and Patton, 1989).
Clay-rich lithologies are characterized by low resistivity. However, because saline fluids also reduce resistivity, care must be taken interpreting the most porous horizons. Where the porosity is predicted to be low, for example, in the core of a lava, the resistivity can be used more confidently to estimate clay content. The lowest resistivities in the Ballymacilroy borehole (Figure 6) are within the IBL (~20 Ω.m2/m) and the lowermost, ~150 m-thick composite flow unit (<100 Ω.m2/m). Illite (K-rich clay) content estimated from NGR is low (≤ 20 API) throughout.
Fresh ALG basalts, not surprisingly, have elevated CaO and MgO contents (Figure 3). Therefore, in the absence of a significant clay fraction, Ca2+ and Mg2+ are available to be carbonated throughout the ALG. Cores recovered from the mineralized zone in Wallula project exhibited calcite and aragonite (both CaCO3) and experiments crystallized ankerite [(Ca, Mg, Fe)CO3], including, in <100 μm pores within low vesicularity basalt (Xiong et al., 2018). The same experiments produced a carbon mineral trapping rate of 1.24 ± 0.52 kg of CO2/m3 of basalt per year, at 100°C and 0.1 kbar. With ~3.6% porosity, this yields a stored mass of 47 kg of CO2/m3 of basalt that is close to estimates from the much younger and fresher Hellisheiði [70–130 kg of CO2/m3 of basalt (Xiong et al., 2018)] rocks.
Petrophysical data values vary significantly between pairs of adjacent flow units, forming a high frequency sawtooth pattern (Figure 6). However, the range of values is generally consistent within groups of adjacent flow units, helping to define the three LBF zones identified in the Ballymacilroy borehole (Figure 6). The characteristic sawtooth patterns in the petrophysical data are typical of boreholes penetrating stacked basalt lavas (e.g., Millet et al., 2016), major aquifer-aquitard systems in basalts (e.g., Kulkarni et al., 2000; Navarro et al., 2020), and the CO2 storage sites at Wallula and Hellisheiði (e.g., McGrail et al., 2003). The sawtooth pattern is characteristic where (1) the core of each lava is high density (>2.5 g/cm3) and high resistivity (>500 Ω.m2/m), and (2) the intercalated breccias and rubbly lava at the boundary between two lavas is low density (1.8–2.5 g/cm3) and low resistivity (50–500 Ω.m2/m). Compound lavas formed of multiple, rapidly emplaced flow units, are inferred where the amplitude change is relatively small. Seventeen and Nineteen distinct lavas are identified in the UBF and LBF, respectively, of which 6 and 8 are compound lavas (Figure 6).
Neutron and sonic porosity trends are parallel downhole (Figure 6), ranging between 0 and 85%, medians of 32% and 42%, respectively (Figure 7A). The maximum porosity measured far exceeds the maxima reported from similar basalt lavas (e.g., ~22%; Neuhoff et al., 1999), in part, due to the difference in methodologies applied. Typically, direct measurements of porosity are made visually, or by pycnometry or porosimetry analyses of samples. In the absence of samples to analyze or systematic core descriptions, as is the case at Ballymacilroy, the neutron and sonic logging data must be used. Neutron log data usually exceed measured porosities (e.g., Zakharova et al., 2012) because they do not differentiate between fluid-filled void spaces: vesicles, intercrystalline pores, intergranular pores in brecciated zones, or fractures. Similarly, sonic porosity data are derived from bulk rock density, and do not differentiate between the different void space types. However, these techniques have the advantage of estimating the “bulk” porosity throughout all the lithologies present, especially those that are most brecciated or fractured. The semi-quantitative “amygdule content” (Figure 6) reported in Thompson (1979) ranges from 0 to 45%, but this only includes pale-colored mineral infilling and not open vesicles nor fractures. However, where the amygdule content is highest is also where the neutron and sonic porosity measurements are in best-agreement and high. Therefore, the logging data are probably best considered maxima for porosity where there is a bimodal distribution: ≤ 20% in the coherent lavas and 20–45% at the boundaries between lavas and where they are fractured strongly. These disagreements in porosity measurement, and therefore the calculated permeabilities, emphasize the need for direct rock properties measurements.
Saar and Manga (1999) report that vesicle size and bubble density are high enough in basalts with >10% porosity, that all pores were connected by narrow apertures that are ~10% the diameter of the vesicles, and thus, are very permeable. Below 10% porosity, the permeability of basalt lavas is much more variable, but typically very low (Saar and Manga, 1999). This is corroborated by analyses of basalts with intercrystalline porosity only (i.e., no macroscopic vesicles) with permeabilities of 100-101 mD (Franzson et al., 2001) and those with only isolated, large vesicles (<100 mD). Future, accurate measurement of permeability will require (1) direct measurements of porosity and permeability from core samples, and (2) characterization of the fractures (width, length, density, roughness, etc.) within the host basalt. Permeability calculated from the Ballymacilroy sonic log is lower and significantly more variable than from the neutron log (Figure 7B), however the median permeability values are within the same order of magnitude (103-104 mD). These data should be treated as preliminary, and rather than over-emphasizing specific values, it is useful to draw attention to the patterns within the data and ranges of values.
Porosity and permeability values only give a general sense of what the hydrogeological conditions may be within the ALG, and no flow-tests, tracer tests, or other hydrologeological measurements have been conducted within the basalts at depth appropriate for CO2 storage (>500 m). Falling-back on a comparable scenario, the first CarbFix injections demonstrated slow but consistent migration of CO2aq away from the injection well, and ~80% mineralization in 1 year at conditions similar to within the ALG (Gíslason and Oelkers, 2014). On the basis of the evidence available, there is no reason to assume that the ALG basalts are not suitable, although considerably more study is required to constrain their suitability for this form of COa storage.
The principles of reservoir volume characterization in basaltic lavas are not well established (Raza et al., 2022), and no detailed comparisons can be made with sedimentary basins. Information from the Wallula and Hellisheiði sites is limited to knowledge of the total volumes of CO2 injected to date.
Therefore, to estimate the magnitude of the potential volume available for sequestration in the ALG, the three AOIs inferred to host the thickest ALG (N, E, and W; Figure 4B) are analyzed. In each, the maximum ALG thickness is used to estimate the inferred thickness below a 500 m total depth threshold, based on the minimum depth of CO2aq injection at CarbFix with 30 bars partial pressure CO2 (Aradóttir et al., 2011) and 0.05 mole fraction. The volume modeled for CO2 storage is based on a range of total porosities 3% (Wallula; Xiong et al., 2018); 22% (Neuhoff et al., 1999); 32% (this study) in a rock-volume with a slab-like geometry below 500 m-depth. The volume in km3 (V) is calculated as:
where t is the “usable” thickness of the ALG below 500 m below surface, A is the area of the AOI, and ϕ is the porosity. The geometric model is necessarily simple, and considerably more data is needed to constrain the depth to the base of the basalts and their internal rock properties. Effective transmission of CO2aq and complete saturation are assumed.
The results show that only the N and E AOIs (Figure 4B) probably have storage potentials in the ~3–30 km3 and 2–20 km3 ranges, respectively (Table 1). The order of magnitude difference reflects the range of porosities possible, from 3% at Wallula to a maximum of 32% (this study). The lava flow-units in the ALG are typically much thinner than those in the Columbia River flood basalt and there are correspondingly more high-porosity inter-lava horizons in the ALG for a given thickness. The Ballymacilroy borehole between 495 and 610 m-depth records ~10 inter-lava horizons between ~1–10 m-thick (Figure 6), ~30% of the section. Assuming that these layers have the highest porosity (32%) and the lavas have the lowest (3%) gives a weighted average of ~10% (Table 1). Using a porosity of 10% yields maximum injected volumes of ~6 km3 and ~4 km3 for AOIs N and E, respectively, which equate to ~10 MtCO2 and ~7 MtCO2, assuming efficient mineralization. These volumes and masses of CO2 increase by ~20% when thorough mineral carbonation of the host basalt is included. This is a reasonable assumption because the limited depth range available calls for storage as CO2aq and the geothermal gradient is elevated (~40°C at 500 m-depth; Raine, 2021); therefore, mineral carbonation rates are enhanced (e.g., Oelkers and Gíslason, 2001; Potsma et al., 2022). For the N and E AOIs these increase to ~6 km3 and ~12 MtCO2, and ~4 km3 and ~9 MtCO2, respectively, for 10% porosity (Table 1). Put another way, a 1 km2 footprint in either N or E could sequester up to ~65 kTCO2 at 10% porosity.
Table 1. Data used to calculate the maximum potential CO2 reservoir volume at 3%, 22%, and 32% porosity.
The geological conditions exist within parts of the ALG to make carbon storage feasible. The data show that although the distribution of porosity and permeability is largely unknown, the weighted bulk average porosity (~10%) is encouraging and compare well with the successful injection site at Wallula. Finally, although the maximum sequestered CO2 volume in the ALG is very small (~10 MtCO2) compared to most sedimentary basins (0.1–100 GtCO2), it is significant at a local scale in NI where there are ~5 large-volume CO2 point-sources and no established alternative geological stores.
Table 2 compares a geological store in the ALG with planned or proposed sedimentary basin storage sites in the Irish Sea. The lack of knowledge about geological stores in or adjacent to NI is evident, especially when development costs are queried. The estimates for offshore storage are for initial exploration and assessment, and are very outdated and probably grossly underestimated. Development and operational costs are likely to exceed €1 billion. There is also the lack of infrastructure with none of the necessary point-source CO2 capture or CO2 pipelines in place. Onshore exploration, drilling, and operation in the oil and gas industries is typically 5–10 times lower cost; therefore, a site in the ALG may be expected to be significantly lower cost, albeit for a much smaller storage volume.
In contrast, the East Irish Sea Basin (1.1–1.7 GtCO2; Table 2) is currently under development as part of the HyNet H2 and CCUS cluster in NW England and NE Wales. A legacy of gas production means that there is abundant geological information and surface infrastructure in place. The CCUS portion of HyNet is considered viable because of (1) CO2 point-sources in existing industry and new green-hydrogen production, and (2) easy supply of imported CO2 by ship, and eventually by pipelines, from industrial clusters around the Irish Sea, including Belfast.
Given that HyNet is going ahead, it seems to be in pole position to be a store for any large volumes of CO2 captured at NI's power plants, if CO2 shipping proves to be economical. A geological store in the ALG is probably too small and not sufficiently developed to be viable to be a useful industrial CCS project.
Having demonstrated that there is useful potential for up to 10 MtCO2 geological storage in the ALG, albeit unlikely to be a viable store for large (>20 ktCO2/yr) industrial point-source CCS, I now explore some pathways by which a basalt-hosted storage project could still be developed and how it could fit into NI's net zero strategy.
Agriculture contributes 28% of NI's GHG emissions and is projected to grow to ~32% in 2031 as other sectors decarbonize faster (DAERA, 2023). NI's most aggressive GHG reduction models assume a major increase in biomass cultivation away from rearing livestock. Biomass combustion and anaerobic digestion to produce methane (CH4) are established, growing renewable energy technologies in NI and RoI (e.g., McGeever et al., 2019) producing both heat and electricity. Bioenergy plants are distributed across NI and ~80% of plants are <500 kWe (Figure 1). However, no Irish biogas facilities currently capture CO2 emissions, in part, due to the absence of available CO2 storage. However, developing geological storage would make BECCS more viable and could lead to an increase of the biomass market. Several anaerobic digestion plants are located within the N and E AOIs and more are expected.
DAC is widely considered to be an increasingly essential part of achieving rapid, near-term reductions in atmospheric CO2, despite it still being a set of nascent technologies. Impediments to DAC uptake are primarily the costs of the capture process itself (≤ $230/tonCO2; Bos et al., 2018; McQueen et al., 2021) and the costs of disposing of the captured CO2 ($20–30/tonCO2; Keleman et al., 2019). One of the chief attractions of DAC is that it can sited anywhere, ideally somewhere with abundant renewable energy (Singh and Colosi, 2022). However, this geographic advantage is curtailed by the need to be proximal to suitable geological storage if economic uses cannot be found for the captured CO2.
NI meets the criteria for DAC deployment through abundant renewable energy opportunities and the availability of geological storage in the ALG (this study).
Like geological carbon storage, geothermal heat extraction in NI is in its infancy, but it is recognized as a high priority as part of NI's energy transition. As of late 2022, there are a small number of deep geothermal projects in the planning stages and several installed or planned, geothermal heat pump systems. Very significant heat resources are thought to be present in Permian sandstones and the Triassic Sherwood Sandstone Group (Pasquali et al., 2010; Raine, 2021), with temperatures ranging from 70 to 90°C at 2–3 km-depth throughout the Larne Basin. The primary evidence for this comes from the same boreholes that provide constraints on the thickness of the ALG in the optimal CO2 storage AOIs (e.g., Langford Lodge, Ballymacilroy; Figure 2), implying that co-location and shared development are feasible. The groundwater within the ALG is very unlikely to be in hydrological contact with the main Larne Basin aquifer(s)/heat reservoir. ALG and the immediately underlying Ulster White Limestone are confined, low-yield aquifers (Robins et al., 2011). Therefore, injection into the ALG is unlikely to degrade the deeper geothermal heat resource.
Co-location of geological CO2 storage and geothermal heat extraction has co-benefits (e.g., Wu and Li, 2020). First, the costs of exploration and drilling can be shared, and risks spread. Second, the skills and surface infrastructure needed are similar. Third, and potentially the most interesting, many different DAC recycling processes will benefit from access to low-cost 70–90°C steam from geothermal sources, because energy load is the one of greatest challenges to DAC deployment (e.g., Ozkan et al., 2022). DAC systems typically require ~80% thermal energy and ~20% electric; this thermal energy could come directly from a geothermal heat supply. For example, the solid-state sorbent used in Climeworks' DAC technology is recycled at between 80°C and 120°C (McQueen et al., 2021). Many other solid-state sorbents are expected to be regenerated at ~90°C, achieving gross production costs of ≤ US$230/tCO2 (McQueen et al., 2021). Being able to co-locate industrial-grade heat with geological CO2 storage reduces the cost of DACCS by ~$5/tonCO2 (McQueen et al., 2020) and makes geothermal energy operations carbon-negative (e.g., Pilorgé et al., 2019).
One path of developing the potential outlined above is through a demonstrator or pilot project in one of the AOIs identified. Such a project could take the form of a co-located BECCS-DACCS-geothermal CO2 “farm,” here called the Antrim Carbon Development Centre (ACDC; Figure 8).
Figure 8. Conceptual diagram showing DACCS and BECCS operations around the co-located geological CO2 store and geothermal facility ACDC. Potential co-benefits are represented by colored connections. Sustainable natural gas is produced for sale. AD, anaerobic digestion; BC, biocombustion plant.
The ACDC is based on co-location of geological CO2 storage and geothermal heat mining, in and below the ALG, respectively. Having secured a CO2 store, local DACCS and BECCS projects (including from anaerobic digestion) become viable (e.g., McGeever et al., 2019). Moreover, industrial heat from the geothermal plant can improve anaerobic digestion performance significantly (e.g., Diamantis et al., 2016), as well as reduce the costs of DACCS operations. Anaerobic digestion supplemented by geothermal heat may produce ~23% more natural gas while reducing GHG emissions by ≤ 66% and capital costs by ~24% (Nazari et al., 2021). Finally, the ACDC is supplied by electricity from renewable sources, especially wind. There exists, therefore, a great potential for synergistic renewable energy and carbon capture and storage in NI, as long as a suitable CO2 store exists. This could be a successful model to develop and export to similar economies worldwide.
The regions east and north of Lough Neagh (AOIs E and N) are the most prospective locations for such a development. These have suitable geology as described, and benefit from the greatest proximity to major point-sources (Figure 1) and excellent transportation links. The regions are generally low-lying and relatively flat, and the dominant land-use is for agriculture.
Carbon storage in basalts is a developing technology but has only been used in two locations to date; NI could adapt this technology for much of its own CO2 storage needs and develop a test-bed and demonstrator facility. NI is a useful analog for many parts of the world where carbon reduction and removal targets and technologies are challenged by a lack of suitable geological CO2 storage. Although carbon capture and storage is at the forefront of UK carbon management, current policies do not support agriculture-dependent regions like NI strongly. NI's ambitious net zero targets may increasingly depend on successful geological CO2 storage in support of BECCS and DACCS.
The ALG basalts are likely to be a viable and useful geological site for mineral carbonation and permanent CO2 sequestration. The ALG is sufficiently thick east and north of Lough Neagh to provide voluminous storage at the porosities estimated from reanalysis of existing hydrocarbon exploration borehole data. The same data suggest that the ALG is typical of other basalt piles used as aquifers and CO2 stores, with a bimodal distribution of physical property values where the lava cores are dense, low porosity, and low permeability, and the intercalated layers are highly porous and permeable. The whole-rock compositions of the ALG basalts are not unusual, and they overlap with the CaO and MgO contents of basalts used for successful carbon mineralization elsewhere.
The case for exploiting the CO2 storage potential of the ALG is strengthened by (1) the coincidence with geothermal heat that can be mined from deeper, (2) a wealth of renewable energy, and (3) the increasing importance of BECCS and DACCS to achieving mandated net zero targets. These serendipitous relationships could be leveraged to develop a purpose-built, RandD facility to advance carbon removal and sustainable energy production in NI, the UK and RoI, and the EU, and help set NI on the path for net zero.
Publicly available datasets were analyzed in this study. This data can be found here: borehole log data are available by contacting the Geological Survey of Northern Ireland. Geochemical data from the PetDB online database and cited articles.
The author confirms being the sole contributor of this work and has approved it for publication.
Rob Raine (GSNI) kindly provided access to borehole logs and reports, and provided helpful comments on an early draft.
The author declares that the research was conducted in the absence of any commercial or financial relationships that could be construed as a potential conflict of interest.
All claims expressed in this article are solely those of the authors and do not necessarily represent those of their affiliated organizations, or those of the publisher, the editors and the reviewers. Any product that may be evaluated in this article, or claim that may be made by its manufacturer, is not guaranteed or endorsed by the publisher.
ACDC, Antrim Carbon Development Centre; ALG, Antrim Lava Group; AOI, area of interest; BECCS, bio-energy carbon capture and storage; CCC, Climate Change Commission; CCUS, carbon capture, utilization, and storage; DAC, direct air capture; DACCS, direct air capture and carbon storage; EOR, enhanced oil recovery; GHG, greenhouse gas; LBF, Lower Basalt Formation; LNG, Lough Neagh Group; NGR, natural gamma radiation; NI, Northern Ireland; RoI, Republic of Ireland; UBF, Upper Basalt Formation; UK, United Kingdom.
Alcalde, J., Flude, S., Wilkinson, M., Johnson, G., Edlmann, K., Bond, C. E., et al. (2018). Estimating geological CO2 storage security to deliver on climate mitigation. Nat. Commun. 9, 2201. doi: 10.1038./s41467-018-04423-1
Aradóttir, E. S. P., Sigurdardóttir, H., Sigfússon, B., and Gunnlaugsson, E. (2011). CarbFix: a CCS pilot project imitating and accelerating natural CO2 sequestration. Greenhouse Gas Sci. Technol. 1, 105–118. doi: 10.1002/ghg.18
Babiker, M., Berndes, G., Blok, K., Cohen, B., Cowie, A., Geden, O., et al. (2022). Cross-sectoral perspectives. In IPCC 2022: Climate Change 2022: Mitigation of Climate Change. Contribution of Working Group III to the Sixth Assessment Report of the Intergovernmental Panel on Climate Change eds P. R. Shukla, J. Skea, R. Slade, A. Al Khourdajie, R. van Diemen, D. McCollum, et al. (Cambridge University Press, Cambridge, UK and New York, NY, USA). doi: 10.1017/9781009157926.005
Bachu, S., Bonijoly, D., Bradshaw, J., Burruss, R., Holloway, S., Christensen, N. P, et al. (2007), CO2. storage capacity estimation: methodology and gaps. Int. J. Greenhouse Gas Cont. 1, 430–443. doi: 10.1016/S1750-5836(07)00086-2.
BEIS (2021). Industrial Decarbonisation Strategy. Westminster: Her Majesty's Stationery Office, p. 170.
BEIS (2022). Projects selected for Phase 1 of the Direct air capture and greenhouse gas removal programme. Available online at: https://www.gov.uk/government/publications/direct-air-capture-and-other-greenhouse-gas-removal-technologies-competition/projects-selected-for-phase-1-of-the-direct-air-capture-and-greenhouse-gas-removal-programme (accessed March 24, 2022).
Benson, S. M., and Cole, D. R. (2008). CO2 sequestration in deep sedimentary formations. Elements 4, 325–331. doi: 10.2113/gselements.4.5.325
Benson, S. M., and Deutch, J. (2018). Advancing enhanced oil recovery as a sequestration asset. Joule 2, 1386–1389. doi: 10.1016/j.joule.2018.07.026
Bielicki, J. M. (2009). (2009). Spatial clustering and carbon capture and storage deployment. Energy Proced. 1, 1691–1698. doi: 10.1016/j.egypro.01221
Bos, M. J., Kroeze, V., Sutanto, S., and Brilman, D. (2018). Evaluating regeneration options of solid amine sorbent for CO2 removal. Ind. Engin. Chem. Res. 57, 11141–11153. doi: 10.1021/acs.iecr.8b00768
Bryden Centre (2021). Carbon Capture, Storage and Utilization Potential in Northern Ireland. Belfast: Bryden Centre. P113.
Casaban, D., and Tsalaporta, E. (2022). Direct air capture of CO2 in the Republic of Ireland. Is it necessary? Energy Reports, 8, 10449–10463. doi: 10.1016/j.egyr.08194
CCC (2020). Letter to DAERA Minister. Climate Change Committee, London, UK, 9th December 2020. Available online at: https://www.theccc.org.uk/wp-content/uploads/2020/12/Lord-Deben-CCC-Letter-to-DAERA-Minister.pdf (accessed March 24, 2022).
CCC (2022). Letter to DAERA Minister. Climate Change Committee, London, UK. Available online at: https://www.theccc.org.uk/publication/letter-northern-irelands-climate-change-bill (accessed March 24, 2022).
CCC (2023). Advice report: The path to a Net Zero Northern Ireland. Climate Change Committee, London, UK. Available online at: https://www.theccc.org.uk/publication/advice-report-the-path-to-a-net-zero-northern-ireland/
DAERA (2022). Green Growth Climate Change Summary. Available online at: https://www.daera-ni.gov.uk/sites/default/files/publications/daera/Green%20Growth%20Climate%20Bill%20Summary.pdf (accessed 20 June, 2022).
DAERA (2023). Northern Ireland greenhouse gas inventory projections update. Available online at: https://www.daera-ni.gov.uk/sites/default/files/publications/daera/NI%20Greenhouse%20Gas%20projections%20based%20on%20the%202020%20GHG%20inventory.PDF (accessed February 9, 2023).
Dessert, C., Dupré, B., Gaillardet, J., Francois, L. M., and Allègre, C. J. (2003). Basalt weathering laws and the impact of basalt weathering on the global carbon cycle. Chem. Geol. 202, 257–273. doi: 10.1016/j.chemgeo.10001
Diamantis, V., Tataki, V., Eftaxias, A., Iliadis, G., and Aivasidis, A. (2016). Geothermal energy valorisation for enhanced biogas production from agro-industrial residues. Environ. Process. 3, 81–90. doi: 10.1007/s40710-016-0182-y
Franzson, H., Guðlaugsson, S. Þ., and Friðleifsson, G. O. (2001). Petrophysical properties of icelandic rocks. Proceedings of the 6th Nordic Symposium on Petrophysics, Trondheim, Norway, May 15-16, 14.
Galán-Martín, A., Vásquez, D., Cobo, S., Mac Dowell, N., Caballero, J. A., Guillén-Gosálbez, G., et al. (2021). Delaying carbon dioxide removal in the European Union puts climate targets at risk. Nature Communications 12, 6490. doi: 10.1038/s41467-021-26680-3
Gambhir, A., and Tavoni, M. (2019). Direct air carbon capture and sequestration: how it works and how it could contribute to climate-change mitigation. One Earth 1, 405–409. doi: 10.1016/j.oneear.11006
García-Freites, S., Gough, C., and Röder, M. (2021). The greenhouse gas removal potential of bioenergy with carbon capture and storage (BECCS) to support the UK's net-zero emission target. Biomass and Bioen. 151, 106164. doi: 10.1016/j.biombioe.2021.106164
Gíslason, S. R., and Oelkers, E. H. (2014). Carbon storage in basalt. Science 344, 373–374. doi: 10.1126/science.1250828
Gíslason, S. R., Sigurdardóttir, H., Aradóttir, E. S., and Oelkers, E. H. (2018). A brief history of CarbFix: challenges and victories of the project's pilot phase. Energy Proced. 146, 103–114. doi: 10.1016/j.egypro.07014
Goldberg, D., and Burgdorff, K. (2005). “Natural fracturing and petrophysical properties of the Palisades dolerite sill,” in Petrophysical Properties of Crystalline Rocks eds P. K. Harvey, T. S. Brewer, P. A. Pezard, and P. A. Petrov (Geological Society of London Special Publication), 25–36. doi: 10.1144/GSL.SP.240.01.03
Goldberg, D., and Slagle, A. L. (2009). A global assessment of deep-sea basalt sites for carbon sequestration. Energy Proced. 1, 3675–3682. doi: 10.1016/j.egypro.2009.02.165
Goldberg, D., Takahashi, T., and Slagle, A. L. (2008). Carbon dioxide sequestration in deep-sea basalt. Proceed. Nat. Acad. Sci. 105, 9920–9925. doi: 10.1073/pnas.0804397105
Goldberg, D. S., Lackner, K. S., Han, P., Slagle, A. L., and Wang, T. (2013). Co-location of air capture, subseafloor CO2 sequestration, and energy production on the kerguelen plateau. Environ. Sci. Technol. 47, 7521–7529. doi: 10.1021/es401531y
Gough, C., Garcia-Freites, S., Jones, C., Mander, S., Moore, B., Pereira, C., et al. (2018). Challenges to the use of BECCS as a keystone technology in pursuit of 1.5°C. Global Sustain. 1, E5. doi: 10.1017/sus.2018.3
GSNI (2020). GeoIndex. Available online at: http://mapapps2.bgs.ac.uk/GSNI_Geoindex/home.html (accessed April 3, 2022).
GSNI (2022). Tellus Data Downloads. Available online at: https://www2.bgs.ac.uk/gsni/tellus/data_licensing/index.html (accessed April 3, 2022).
Hannis, S., Lu, J., Chadwick, A., Hovorka, S., Kirk, K., Romanak, K., et al. (2017). CO2 storage in depleted or depleting oil and gas fields: what can we learn from existing projects? Energy Proced. 114, 5680–5690. doi: 10.1016/j.egypro.031707
Jones, G. L., Goodman, R., Pasquali, R., Kelly, J. G., O'Neill, N., Slowey, E., et al. (2007). The Status of Geothermal Resource Development in Ireland. Proceedings European Geothermal Congress 2007, Unterhaching, Germany, 30 May-1 June 2007, 3.
Kearns, D., Lui, H., and Consoli, C. (2021). Technology Readiness and Costs of CCS. Global CCS Institute, p50. Available online at: www.globalccsinstitute.com/wp-content/uploads/2021/03/Technology-Readiness-and-Costs-for-CCS-2021-1.pdf (accessed March 24, 2022).
Keleman, P., Benson, S. M., Pilorgé, H., Psarras, P., and Wilcox, J. (2019). An overview of the status and challenges of CO2 storage in minerals and geological formations. Front. Clim. 1, 9. doi: 10.3389/fclim.2019.00009
Kulkarni, H., Deolanker, S. B., Lalwani, A., Joseph, B., and Pawar, S. (2000). Hydrogeological framework of the Deccan basalt groundwater systems, west-central India. Hydrogeol. J. 8, 368–378. doi: 10.1007/s100400000079
Lackner, K. S., Wendt, C. H., Butt, D. P., Joyce Jr, E. L., and Sharp, D. H. (1995). Carbon dioxide disposal in carbonate minerals. Energy 20, 1153–1170. doi: 10.1016/0360-5442(95)00071-N
Lewis, D., Bentham, M., Cleary, T., Vernon, R., O'Neill, N., Kirk, K., et al. (2009). (2009). Assessment of the potential for geological storage of carbon dioxide in Ireland and Northern Ireland. Energy Proced. 1, 2655–2662. doi: 10.1016/j.egypro.02033
Limaye, S. D. (2010). Groundwater development and management in the Deccan Traps (basalts) of western India. Hydrogeol. J. 18, 543–558. doi: 10.1007/s10040-009-0566-4
Lyle, P. (1988). The Geochemistry, Petrology and Volcanology of the Tertiary Lava Succession of the Binevenagh-Benbraddagh Area of County Londonderry. Irish J. Earth Sci. 9, 141–151.
Lyle, P., and Patton, D. J. S. (1989). The petrography and geochemistry of the Upper Basalt Formation of the Antrim Lava Group in northeast Ireland. Irish J. Earth Sci. 10, 33–41.
Mallon, W., Buit, L., van Wingerden, J., Lemmens, H., and Eldrup, N. H. (2013). Costs of CO2 transportation infrastructures. Energy Proced. 37, 2969–2980. doi: 10.1016/j.egypro.06183
Martin-Roberts, E., Scott, V., Flude, S., Johnson, G., Haszeldine, S., Gilfillan, S., et al. (2021). Carbon capture and storage at the end of a lost decade. One Earth 4, 1569–1584. doi: 10.1016/j.oneear.10002
Matter, J. M., Broecker, W. S., Gíslason, S. R., Oelkers, E. H., Stute, M., Sigurdardóttir, H., et al. (2011). The CarbFix Pilot Project—Storing carbon dioxide in basalt. Energy Procedia 4, 5579–5585. doi: 10.1016/j.egypro.02546
Matter, J. M., Broecker, W. S., Stute, M., Gíslason, S. R., Oelkers, E. H., Stefánsson, A., et al. (2009). Permanent carbon dioxide storage into basalt: the CarbFix pilot project, Iceland. Energy Proced. 1, 3641–3646. doi: 10.1016/j.egypro.02160
McCann, N. (1991). Subsurface Geology of the Lough Neagh—Larne Basin, Northern Ireland. Irish J. Earth Sci. 11, 53–64.
McGeever, A. H., Price, P., McMullin, B., and Jones, M. B. (2019). Assessing the terrestrial capacity for negative emission technologies in Ireland. Carbon Manag. 10, 1–10. doi: 10.1080/17583004.2018.1537516
McGrail, B. P., Ho, A. M., Reidel, S. P., and Schaef, H. T. (2003). Use and Features of Basalt Formations for Geologic Sequestration. Greenhouse Gas Control Technologies: Proceedings of the 6th International Conference on Greenhouse Gas Control Technologies, Kyoto, Japan, 1–4 October, 2, 1637–1640. doi: 10.1016/B978-008044276-1/50264-6
McGrail, B. P., Schaef, H. T., Ho, A. M., Chien, Y. J., Dooley, J. J., Davidson, C. L., et al. (2006). Potential for carbon dioxide sequestration in flood basalts. J. Geophy. Res. 111, B12201. doi: 10.1029/2005JB004169
McGrail, B. P., Spane, F. E., Sullivan, E. C., Bacon, D. H., and Hund, G. (2011). (2011). The wallula basalt sequestration pilot project. Energy Proced. 4, 5653–5660. doi: 10.1016/j.egypro.02557
McKenzie, J. M., Siegel, D. I., Patterson, W., and McKenzie, D. J. (2001). A geochemical survey of spring water from the main Ethiopian rift valley, southern Ethiopia: implications for well-head protection. Hydrogeology J. 9, 265–272. doi: 10.1007./s100400100134
McQueen, N., Gomes, K. V., McCormick, C., Blumanthal, K., Pisciotta, M., Wilcox, J., et al. (2021). A review of direct air capture (DAC): scaling up commercial technologies and innovating for the future. Prog. Energy 3, 0310. doi: 10.1088/2516-1083/abf1ce
McQueen, N., Psarras, P., Pilorgé, H., Liguori, S., He, J., Yuan, M., et al. (2020). Cost analysis of direct air capture and sequestration coupled to low-carbon thermal energy in the United States. Environ. Sci. Technol. 54, 7542–7551. doi: 10.1021/acs.est.0c00476
Mikunda, T., Brunner, L., Skylogianni, E., Monteiro, J., and Rycroft, L. (2021). Carbon capture and storage and the sustainable development goals. Int. J. Greenhouse Gas Control 108, 103318. doi: 10.1016/j.ijggc.2021.103318
Millet, J. M., Hole, M. J., Jolley, D. W., Schofield, N., and Campbell, E. (2016). Frontier exploration and the North Atlantic Igneous Province: new insights from a 2.6km offshore volcanic sequence in the NE Faroe–Shetland Basin. J. Geol. Soc. 173, 320–336. doi: 10.1144/jgs2015-069
Mitchell, W. I. (2004). The Geology of Northern Ireland—Our Natural Foundation. Belfast: Geological Survey of Northern Ireland.
Nara, Y., Meredith, P. G., Yoneda, T., and Kaneko, K. (2011). Influence of macro-fractures and micro-fractures on permeability and elastic wave velocities in basalt at elevated pressure. Tectonophysics 503, 52–69. doi: 10.1016/j.tecto.09027
Navarro, J., Teramoto, E. H., Engelbrecht, B. Z., and Kiang, C. H. (2020). Assessing hydrofacies and hydraulic properties of basaltic aquifers derived from geophysical logging. Braz. J. Geol. 50, e20200013. doi: 10.1590/2317-4889202020200013
Naylor, D., Philcox, M. E., and Clayton, G. (2003). Annaghmore-1 and Ballynamullan-1 Wells, Larne–Lough Neagh Basin, Northern Ireland. Irish J. Earth Sci. 21, 47–69. doi: 10.1353/ijes.2003.a810062
Nazari, A., Soltani, M., Hosseinpour, M., Alharbi, W., and Raahemifar, K. (2021). Integrated anaerobic co-digestion of municipal organic waste to biogas using geothermal and CHP plants: a comprehensive analysis. Ren. Sustain. Energy Rev. 152, 111709. doi: 10.1016/j.rser.2021.111709
Neuhoff, P. S., Fridriksson, T., Arnorsson, S., and Bird, D. K. (1999). Porosity evolution and mineral paragenesis during low-grade metamorphism of basaltic lavas at Teigarhorn, Eastern Iceland. Am. J. Sci. 299, 467–501. doi: 10.2475/ajs.299.6.467
Oelkers, E., Gíslason, S. R., and Matter, J. (2008). Mineral carbonation of CO2. Elements 4, 333–337. doi: 10.2113/gselements.4.5.333
Oelkers, E. H., and Gíslason, S. R. (2001). The mechanism, rates and consequences of basaltic glass dissolution: I. An experimental study of the dissolution rates of basaltic glass as a function of aqueous Al, Si and oxalic acid concentration at 25°C and pH 3 and 11. Geochimica et Cosmochimica Acta 65, 3671–3681. doi: 10.1016/S0016-7037(01)00664-0
Okoko, G. O., and Olaka, L. A. (2021). Can East African rift basalts sequester CO2? Case study of the Kenya rift. Sci. African 13, e00924. doi: 10.1016/j.sciaf.2021.e00924
Ozkan, M. (2021). Direct air capture of CO2: a response to meet the global climate targets. MRS Ene. Sustain. 8, 51–56. doi: 10.1557/s43581-021-00005-9
Ozkan, M., Nayak, S. P., Ruiz, A. D., and Jiang, W. (2022). Current status and pillars of direct air capture technologies. iScience 25, 103990. doi: 10.1016/j.isci.2022.103990
Parnell, J., Shukla, B., and Meighan, I. G. (1989). The lignite and associated sediments of the tertiary lough neagh basin. Irish J. Earth Sci. 10, 67–88.
Pasquali, R., O'Neill, N., Reay, D., and Waugh, T. (2010). The Geothermal Potential of Northern Ireland. Proceedings World Geothermal Congress 2010, (Bali), 5.
Patterson, E. M., Mitchell, W. A., and Swaine, D. J. (1956). The tertiary volcanic succession in the western part of the antrim plateau. Proceedings of the Royal Irish Academy. Section B: Biological, Geological, and Chemical Science, 57, 155–178.
Pilorgé, H., Psarras, P., He, J., and Wilcox, J. L. (2019). Combining geothermal potential and direct air capture for negative emission power generation in California. In Geothermal: Green Energy for the Long Run–Geothermal Resources Council 2019 Annual Meeting, GRC 2019, 462–468.
Potsma, T. J. W., Bandilla, K. W., Peters, C. A., and Celia, M. A. (2022). Field-scale modeling of CO2 mineral trapping in reactive rocks: a vertically integrated approach. Water Reso. Res. 58, e2021WR.030626. doi: 10.1029/2021WR030626
Quinn, M. F. (2006). Lough Neagh: the site of a Cenozoic pull-apart basin. Scottish J. Geol. 42, 101–112. doi: 10.1144/sjg42020101
Raine, R. (2021). Geothermal Energy Potential in Northern Ireland: Summary and Recommendations for the Geothermal Advisory Committee. Belfast: GSNI Technical Report 2021/EM/01.
Raymer, L. L., Hunt, E. R., and Gardner, J. S. (1980). An Improved Sonic Transit Time-to-Porosity Transform. Society of Petrophysicists and Well Log Analysts 21st Annual Logging Symposium, Lafayette, Louisiana, July 8–11, 1–12.
Raza, A., Glatz, G., Gholami, R., Mahmoud, M., and Alafnan, S. (2022). Carbon mineralization and geological storage of CO2 in basalt: mechanisms and technical challenges. Earth-Sci. Rev. 229, 104036. doi: 10.1016/j.earscirev.2022.104036
Robins, N. S., McConvey, P. J., and McLorinan, D. (2011). Groundwater flow in the Antrim Lava Group and Ulster White Limestone Formation, Northern Ireland. Quart. J. Engin. Geol. Hydrogeol. 44, 63–73. doi: 10.1144/1470-9236/08-059
Rogers, K. L., Neuhoff, P. S., Pedersen, A. K., and Bird, D. K. (2006). CO2 metasomatism in a basalt-hosted petroleum reservoir, Nuussuaq, West Greenland. Lithos 92, 55–82. doi: 10.1016/j.lithos.04002
Ruffell, A. (2016). Do spectral gamma ray data really reflect humid–arid palaeoclimates? A test from palaeogene interbasaltic weathered horizons at the Giant's causeway, N. Ireland. Proceed. Geol. Assoc. 127, 18–28. doi: 10.1016/j.pgeola.02003
Saar, M. O., and Manga, M. (1999). Permeability-porosity relationship in vesicular basalts. Geophys. Res. Lett, 26, 111–114. doi: 10.1029/1998GL900256
Schaef, H. T., McGrail, B. P., and Owen, A. T. (2009). Basalt-CO2-H2O interactions and variability in carbonate mineralization rates. Energy Proc 1, 4899–4906. doi: 10.1016/j.egypro.02320
Schaef, H. T., McGrail, B. P., and Owen, A. T. (2010). Carbonate mineralization of volcanic province basalts. Int. J. Greenhouse Gas Cont. 4, 249–261. doi: 10.1016/j.ijggc.10009
Schaef, H. T., McGrail, B. P., and Owen, A. T. (2011). Basalt reactivity variability with reservoir depth in supercritical CO2 and aqueous phases. Energy Proced. 4, 4977–4984. doi: 10.1016/j.egypro.02468
SEAI (2021). Energy-Related CO2 Emissions in Ireland 2020. Companion Note to the 2020 National Energy Balance. SEAI, Dublin, Government Publications Office.
SEAI (2022). Carbon Capture Utilisation and Storage (CCUS): Suitability, Costs and Deployment: Options in Ireland. Report 6 of the National Heat Study. February 2022, 39.
Shelton, R. (1997). “Tectonic evolution of the Larne Basin,” in Petroleum Geology of the Irish Sea and Adjacent Areas, Vol. 124, eds N. S. Meadows, S. P. Trueblood, M. Hardman, and G. Cowan (London: Geological Society, Special Publications), 113–133. doi: 10.1144/gsl.sp.1997.124.01.08
Singh, U., and Colosi, L. M. (2022). Capture or curtail: the potential and performance of direct air capture powered through excess renewable electricity. Energy Conv. Manag. 15, 100230. doi: 10.1016/j.ecmx.2022.100230
Smith, B. J., and McAlister, J. J. (1995). Mineralogy, chemistry and palaeoenvironmental significance of an Early Tertiary Terra Rossa from Northern Ireland: a preliminary review. Geomorphology 12, 63–73. doi: 10.1016/0169-555X(94)00077-5
Sovacool, B. K., Baum, C. H., Low, S., Roberts, C., and Steinhauser, J. (2022). Climate policy for a net-zero future: ten recommendations for direct air capture. Environ. Res. Lett. 17, 074014. doi: 10.1088/1748-9326/ac77a4
Tamme, E., and Beck, L. L. (2021). European carbon dioxide removal policy: current status and future opportunities. Front. Clim. 3, doi: 10.3389/fclim.2021.682882
Thompson, S. J. (1979). Preliminary report on the ballymacilroy No 1 Borehole, Ahoghill, Co. Antrim. Geol. Survey Northern Ireland Open File Report 63, 20.
Van Rossum, R., Jens, J., La Guardia, G., Wang, A., Kuhnen, L., Overgaag, M., et al. (2022). A European Hydrogen Infrastructure Vision Covering 28 Countries. European Hydrogen Backbone. Utrecht, Guidehouse. April 2022, 18.
Wu, Y., and Li, P. (2020). The potential of coupled carbon storage and geothermal extraction in a CO2-enhanced geothermal system: a review. Geothermal Ene. 8, 19. doi: 10.1186/s40517-020-00173-w
Xiong, W., Wells, R. K., Horner, J. A., Schaef, H. T., Skemer, P. A., Giammar, D. E., et al. (2018). CO2 mineral sequestration in naturally porous basalt. Environ. Sci. Technol. Lett. 5, 142–147. doi: 10.1021/acs.estlett.8b00047
Keywords: carbon mineralization and storage, basalt, carbon capture and sequestration (CCS), CarbFix, Northern Ireland (NI)
Citation: Andrews GDM (2023) Geological carbon storage in northern Irish basalts: prospectivity and potential. Front. Clim. 5:1207668. doi: 10.3389/fclim.2023.1207668
Received: 17 April 2023; Accepted: 01 August 2023;
Published: 22 September 2023.
Edited by:
Stuart Gilfillan, University of Edinburgh, United KingdomReviewed by:
Michael Bizimis, University of South Carolina, United StatesCopyright © 2023 Andrews. This is an open-access article distributed under the terms of the Creative Commons Attribution License (CC BY). The use, distribution or reproduction in other forums is permitted, provided the original author(s) and the copyright owner(s) are credited and that the original publication in this journal is cited, in accordance with accepted academic practice. No use, distribution or reproduction is permitted which does not comply with these terms.
*Correspondence: Graham D. M. Andrews, Z2RtYTE5NzdAZ21haWwuY29t
†Present address: Graham D. M. Andrews, School of Environmental Sciences, University of Hull, Hull, United Kingdom
Disclaimer: All claims expressed in this article are solely those of the authors and do not necessarily represent those of their affiliated organizations, or those of the publisher, the editors and the reviewers. Any product that may be evaluated in this article or claim that may be made by its manufacturer is not guaranteed or endorsed by the publisher.
Research integrity at Frontiers
Learn more about the work of our research integrity team to safeguard the quality of each article we publish.