- Soil Chemistry and Chemical Soil Quality, Wageningen University and Research, Wageningen, Netherlands
Enhanced weathering is a negative emission technology that involves the spread of crushed silicate minerals and rocks on land and water. When applied to agricultural soils, the resulting increase in soil pH and release of nutrients may co-benefit plant productivity. Silicate minerals and rocks differ in their enhanced weathering potential, i.e., their potential for both carbon dioxide (CO2) sequestration and soil quality improvements. However, studies comparing silicate minerals and rocks for this dual potential are lacking. Therefore, we compared the enhanced weathering potential of olivine (Mg2SiO4), basalt, wollastonite (CaSiO3), and two minerals that are novel in this context, anorthite (CaAl2Si2O8) and albite (NaAlSi3O8). A down-flow soil column experiment was designed allowing for measurements on soils and leachate, and calculations of organic and inorganic carbon budgets. Our results showed comparatively high CO2 capture by enhanced wollastonite and olivine weathering. Furthermore, CO2 capture per m2 specific surface area indicated potential for enhanced anorthite and albite weathering. Calculated carbon budgets showed that most treatments produced net CO2 emissions from soils, likely related to the short duration of this experiment. All silicates generally improved soil quality, with soil nickel contents remaining below contamination limits. However, nickel concentrations in leachates from olivine-amended soils exceeded the groundwater threshold value, stressing the importance of monitoring nickel leaching. We found a relatively high enhanced weathering potential for wollastonite, while the potential for olivine may be constrained by nickel leaching. The promising results for anorthite and albite indicate the need to further quantify their enhanced weathering potential.
1. Introduction
Rising atmospheric carbon dioxide (CO2) concentrations resulting from fossil fuel combustion and land use change are causing climate change (IPCC, 2014). To limit global temperature rise well below 2°C, as agreed in the Paris Agreement, large scale application of Negative Emission Technologies (NETs) is needed (IPCC, 2018). Enhanced Weathering (EW) is a NET based on the natural silicate weathering cycle that stabilizes atmospheric CO2 concentrations and climate on geological time scales (Kump et al., 2000). When CO2 dissolves in water, carbonic acid is formed, which weathers silicate minerals. The resulting bicarbonate () and carbonate () ions may leach from soils and in addition contribute to counteracting ocean acidification (Hartmann et al., 2013; Bach et al., 2019). Weathering products may also precipitate as calcium and/or magnesium carbonates (CaCO3, MgCO3). Although carbonates form an important soil carbon (C) sink, carbonate precipitation releases part of the consumed CO2 back to the atmosphere, thereby limiting the overall efficiency of CO2 sequestration (Hartmann et al., 2013; Haque et al., 2019a). EW involves the artificial acceleration of the natural silicate weathering cycle through spreading crushed silicate minerals on land and water, aimed at CO2 sequestration at a rate significant on human time scales (Schuiling and Krijgsman, 2006; Moosdorf et al., 2014; Andrews and Taylor, 2019).
The application of crushed silicate minerals and rocks on agricultural soils may, besides capturing CO2, improve soil quality and consequently stimulate plant productivity (Ten Berge et al., 2012; Beerling et al., 2018; Haque et al., 2019b; Kelland et al., 2020). Silicate weathering releases nutrients, such as calcium (Ca2+), magnesium (Mg2+), and potassium (K+), depending on the type of silicate mineral or rock used (Harley and Gilkes, 2000; Lehmann and Possinger, 2020). Furthermore, the released silica can improve plant resistance to biotic and abiotic stresses (Beerling et al., 2018). In agriculture, carbonate minerals are often applied as liming agents, while the weathering of silicate minerals also increases soil pH (West and McBride, 2005; Dietzen et al., 2018). The increase in soil pH and formation of secondary (clay) minerals by incongruent silicate weathering may both generate negatively charged binding sites, resulting in a higher soil Cation Exchange Capacity (CEC) (Gillman, 1980). Therefore, EW can improve both the retention and availability of nutrients in soils (Gillman et al., 2002; Anda et al., 2015).
EW can be considered a long-term storage option for CO2 in the form of Soil Inorganic Carbon (SIC), since carbonate minerals and have a mean residence time in soils of 80.000–100.000 years (Andrews and Taylor, 2019). On generally shorter time scales, EW can affect Soil Organic Carbon (SOC) (Beerling et al., 2018; Andrews and Taylor, 2019; Lehmann and Possinger, 2020). The release of multivalent cations and formation of secondary minerals by EW may stabilize SOC both chemically through organo-mineral associations, and physically through aggregate formation (Paradelo et al., 2015; Beerling et al., 2018; Gao et al., 2020). Improved crop productivity due to EW may further enhance atmospheric CO2 uptake and belowground C inputs (Vicca et al., 2022). However, increasing soil pH can enhance microbial activity, and SOC bioavailability through deprotonation of functional groups (McBride, 1994; Leifeld et al., 2013). Similar to liming, EW may stimulate SOC decomposition, potentially reducing SOC (Paradelo et al., 2015). At the same time, chemical weathering rates, and consequently CO2 capture by EW, are generally reduced at higher pH values (Hartmann et al., 2013). Since abovementioned processes can exert opposite effects on soil C content, and considering that mining, crushing and spreading of silicates also produces CO2 emissions (Moosdorf et al., 2014), the net CO2 budget of EW is yet uncertain.
The main focus of EW research has so far been on olivine minerals, especially the Mg-endmember forsterite (Mg2SiO4), due to their potentially high weathering rates and CO2 consumption (Hartmann et al., 2013; Oelkers et al., 2018). However, olivine weathering also releases nickel cations (Ni2+). Depending on Ni concentrations and its speciation in soils, threshold values can be reached in soils, plants, and groundwater (Weng et al., 2004; Ten Berge et al., 2012; Renforth et al., 2015; Amann et al., 2020). Therefore, the use of basalt, an abundant silicate rock with lower Ni concentrations and a higher content of nutritional elements compared to olivine-rich rocks (Beerling et al., 2018), and wollastonite (CaSiO3), a fast weathering and abundant Ca-bearing silicate mineral (Haque et al., 2019b), have recently received more attention. However, other silicate minerals may also have potential for EW. For example, feldspar minerals such as anorthite (CaAl2Si2O8) and albite (NaAlSi3O8) are widely abundant (USGS, 2020). Anorthite minerals have high weathering rates (Palandri and Kharaka, 2004), and albite weathering does not result in carbonate precipitation thereby acting as a net sink for 100% of the consumed atmospheric CO2 (Hartmann et al., 2013). Despite their potential, these minerals have to our knowledge not yet been studied in the context of EW.
Different types of silicate minerals and rocks vary in what we define as their enhanced weathering potential, with respect to both improving agricultural soil quality through increasing soil pH and releasing nutrients, and supporting climate change mitigation by CO2 sequestration. However, a comparison between silicate minerals and rocks for this dual potential is lacking in current literature. Therefore, we aim to compare this enhanced weathering potential of five different silicates (olivine, basalt, wollastonite, anorthite, albite) using a down-flow soil column experiment. Hereby, this study supports the development of EW strategies on croplands that both improve agricultural soil quality and mitigate climate change.
2. Materials and methods
2.1. Characterization of the experimental soil and silicates
Soil samples were collected from the topsoil of a sandy grassland in Wageningen, the Netherlands (51°59'39.3”N 5°40'05.9”E) in November 2019. This field had not been used for livestock practices for 2 years and had not been fertilized since 1972. The soil was selected for its relatively low pH and geochemically reactive concentrations of base cations and Ni (Table 1). Soil samples were dried at 40°C for about 48 h, sieved over 2 mm, and homogenized.
Five types of silicates were used in this experiment. First, crushed olivine minerals were retrieved from the Aheim Gusdal Mine located in Norway, where forsterite is mined at Steinsvik (Kremer et al., 2019). Second, crushed basalt rocks were used from a mine located in between Nieder Ofleiden and Homburg, Germany (Actimin, 2019). Third, Canadian wollastonite was used, which is a crushed waste stream from mining operations at Saint Lawrence Wollastonite Deposit, Ontario, and therefore also contained some Mg impurities in the form of diopside (Haque et al., 2019b). Fourth, anorthite was retrieved from Grass Valley, California, United States. Finally, albite was obtained from a mine in Bancroft, Ontario, Canada. Anorthite and albite minerals were ordered from Ward's Science and were crushed using a jaw crusher. The silicates were characterized for their chemical composition using X-Ray Fluorescence (XRF) (ARLTM PERFORM'X wavelength-dispersive sequential XRF spectrometer, ThermoFisher Scientific), Specific Surface Area (SSA) by N2 adsorption using the Brunauer-Emmett-Teller (BET) method (Gemini model 2390p) (Table 2), and particle size distribution using laser diffraction (CoulterTM LS 230, fluid module) (Figure 1).
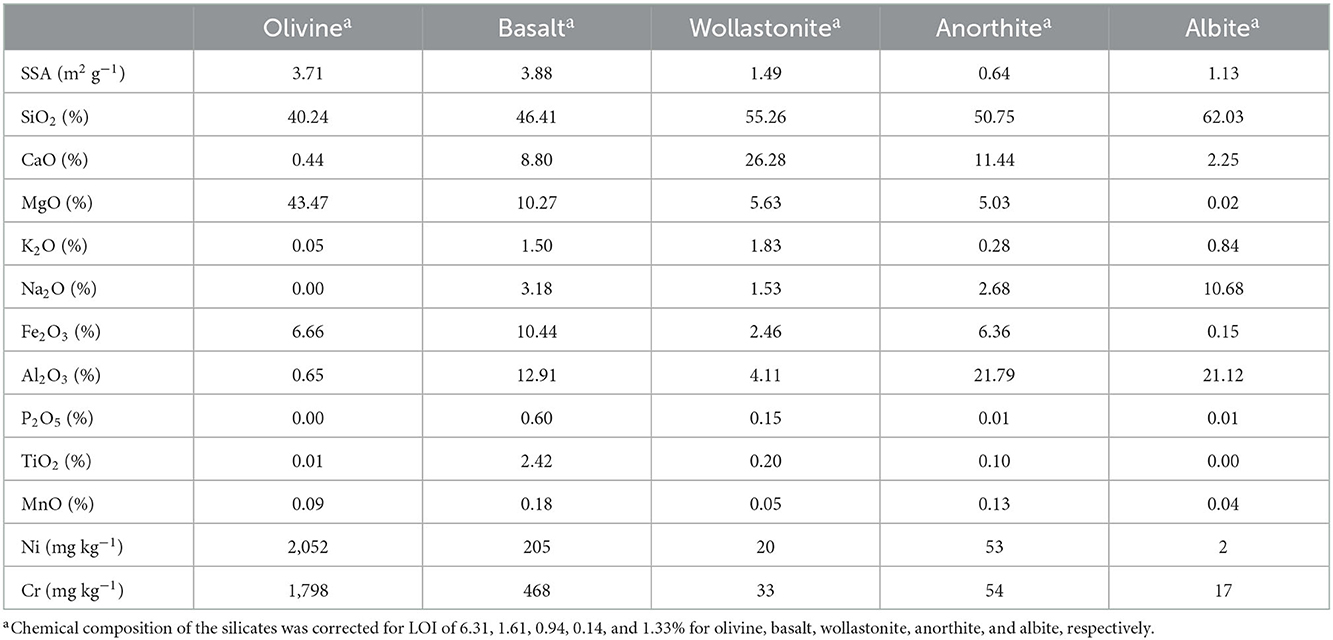
Table 2. Specific Surface Area (SSA) and chemical composition of the silicate minerals and basalt used in this experiment.
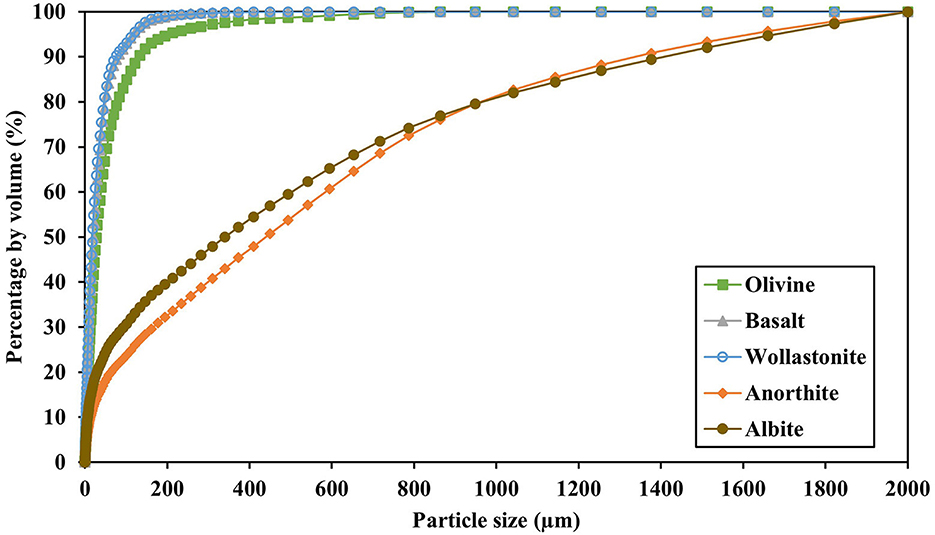
Figure 1. Particle size distribution of the five silicates used in this experiment, measured by laser diffraction (n = 1).
2.2. Experimental set-up
We designed a down-flow soil column experimental set-up that enabled controlling environmental conditions to ensure comparability among the different treatments, and measurements on both soil and leachate (Figure 2). Soil columns were made from polyethylene containers (180 ml) by drilling 30 (Ø2.5 mm) holes in the bottom of the containers. Each soil column was connected via a plastic funnel to a closed container, in which the leachate was collected and sampled every 2 to 7 days. A cellulose filter (12–25 μm pore size, Whatman 589/1) was placed at the bottom and on top of each column to prevent particle losses. Columns were filled with 150 g soil and 100 ml de-mineralised water following a five-step packing procedure in which each step consisted of one layer of 30 g soil and 20 ml de-mineralised water to reduce the chance of preferential flow paths (Gilbert et al., 2014; ISO, 2019). Aluminum foil was wrapped around the columns to prevent impact of incoming light on the soil. The experiment started after 2 days of pre-incubation, which allowed the soil to settle within the columns and redundant water to leach from the columns before the different treatments were applied, and lasted for 9 weeks (64 days). The experiment took place in a conditioned room of 20 ± 2°C.
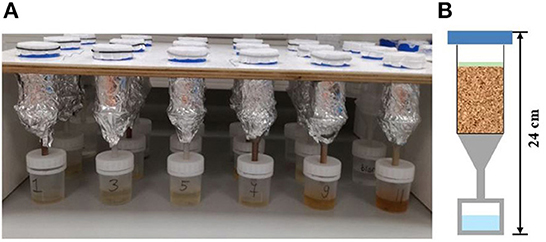
Figure 2. (A) Picture showing the experimental set-up. (B) Schematic overview of a single soil column.
On the 1st day of the experiment, 18.75 g (125 g kg−1 soil) of one of the five silicates was applied on top of each soil. This dose is in line with, though at the higher end of, the range found in current literature (Kelland et al., 2020). Each of the five treatments was studied in duplicate, being olivine (Ol1 and Ol2), basalt (Ba1 and Ba2), wollastonite (Wo1 and Wo2), anorthite (An1 and An2), and albite (Al1 and Al2). One control soil (Control) was used without silicate application. Every 2 to 4 days, 40 ml de-mineralised water was added on top of the soil columns. De-mineralised water has a pH similar to rainwater (about 5.7), but differs from rainwater in its ionic strength. Despite de-mineralised water not fully representing the effect of rainwater, the elemental concentrations measured in the leachate and soil of the treatments, corrected for those measured in the leachate and soil of the control, could be solely attributed to silicate weathering, thereby allowing mass balance calculations. Although temperature and precipitation conditions during the experiment were not representative for average weather conditions in the Netherlands (average annual temperature is about 10°C lower and average annual precipitation is about three times lower compared to experimental conditions), the use of controlled conditions allowed for a comparison between the silicates, and ensured enough leachate to conduct all measurements.
2.3. Chemical analyses of leachate samples
After each water addition, leachate was collected in the containers below the columns, from which samples were taken on day 1, 3, 5, 8, 12, 17, 24, 33, and 64 of the experiment. In the leachate samples, concentrations of Ca2+, Mg2+, K+, and sodium (Na+) were measured using Inductively Coupled Plasma Optical Emission Spectrometry (ICP-OES), and Ni2+ concentrations were measured using High-Resolution ICP Mass Spectrometry (HR-ICP-MS). Segmented Flow Analysis (SFA) was used to measure the dissolved total C and Dissolved Inorganic Carbon (DIC) concentrations in the leachate samples, from which Dissolved Organic Carbon (DOC) concentrations were calculated. More frequent leachate samples were taken (every 2 to 7 days) to measure pH and alkalinity. First, the pH of the leachate samples was measured using a PHM 92 Lab pH electrode (Radiometer Copenhagen). Then, total alkalinity was measured by titrating 7.5 ml leachate to a pH of 4.50 (±0.05) using a 0.02 M HCl solution.
2.4. Chemical analyses of soils
After day 64, the experiment was terminated. Visually detectable silicates on top of the soils were removed using a plastic knife to minimize the occurrence of mineral particles in the soil extractions and fractionations. The soils were separately dried at 40°C for about 4 days, sieved over 2 mm, and homogenized.
Soil CEC and base cation concentrations were measured using a 0.1 M BaCl2 extraction (ISO, 2018), followed by ICP-OES analysis. This data was used to calculate the base saturation, which represents the sum of Ca, Mg, K, and Na concentrations as a percentage of soil CEC. To calculate elemental mass balances and Ni release, soils were extracted with 0.43 M HNO3 (Groenenberg et al., 2017), followed by ICP-OES analysis of Ca, Mg, K, Na, and Ni concentrations. To quantify the SIC content, the Scheibler method was used (Allison, 1960; ISO, 1995). A LECO CN analyser was used to measure total C, from which the SIC content was subtracted to calculate the SOC content.
2.5. Calculations and data analysis
Cumulative CO2 capture was calculated using two alternative methods. The first method was based on elemental mass balances (EMB-method), using Equation 1 to calculate the weathered fraction of the applied mineral, and Equation 2 to determine CO2 capture in this experiment. The element used to calculate the mass balance was based on the major element present in each of the silicates. Based on XRF analysis (Table 2), the Mg balance was used for olivine and basalt, the Ca balance for wollastonite and anorthite, and the Na balance for albite. Mg, Ca, and Na concentrations measured in the leachate using ICP-OES were linearly interpolated over time to calculate cumulative leaching. Soil Mg, Ca, and Na contents were measured by ICP-OES after extraction with 0.43 M HNO3. The Mg, Ca, and Na content measured in the initial soil was used as a correction to only take into account the Mg, Ca, and Na released by silicate weathering. This method was similar to the method used by Ten Berge et al. (2012), except that we used the 0.43 M HNO3 extraction instead of the 0.01 M CaCl2 extraction to include elements adsorbed onto reactive binding sites and avoid underestimation of the weathering rate as expected by these authors. The total amounts of applied Mg, Ca, and Na were calculated based on the mineral contents as determined by XRF analysis (Table 2).
In Equation 2, Fweathered was calculated with Equation 1, and the mineral dose was 18.75 g for all treatments. The term stoichiometry denoted CO2 capture assuming ideal stoichiometric ratios during mineral dissolution, being 1.25 g CO2 g−1 olivine weathered, 0.38 g CO2 g−1 basalt weathered, 0.76 g CO2 g−1 wollastonite weathered, 0.32 g CO2 g−1 anorthite weathered, and 0.17 g CO2 g−1 albite weathered (Supplementary Table S1). The results were divided by 0.15 to calculate the CO2 capture from 150 g soil to 1 kg soil.
For the second method (IC-method), Equation 3 was used to calculate cumulative CO2 capture based on alkalinity concentrations measured in the leachate and the soil carbonate content measured with the Scheibler method, representing the two pathways of IC sequestration by EW. Alkalinity concentrations were linearly interpolated to calculate cumulative alkalinity produced during this experiment, and the soil carbonate content was expressed as CaCO3 (both in moles). These results were multiplied by the molar mass of CO2 (44.0095 g mol−1) and divided by 0.15 to calculate the CO2 capture per kg soil. Finally, the carbonate content of the initial soil was subtracted to only take into account the carbonation resulting from EW.
Furthermore, inorganic and organic CO2 budgets were calculated and added to determine the net CO2 budget (Equation 4), in which ΔSIC and ΔSOC were relative to the concentrations measured in the initial soil.
Statistical analysis was conducted using R version 3.6.1 (R Core Team, 2020) and the package “car” (Fox and Weisberg, 2019). Data were checked for normality of residuals using the Shapiro Wilk Test, for homogeneity of variances using the Levence's Test, and for homoscedasticity of residuals by scatterplots. As the data did not meet the assumptions to allow the use of parametric tests, and as each treatment consisted of only two replicates, non-parametric Kruskal-Wallis tests were used to assess whether significant differences were found between the treatments for each variable. Since P-values are influenced by the number of replicates (n), we accepted a slightly lower confidence level than the most often used 95%, and considered differences between treatments significant if P ≤ 0.1. Moreover, we focused the data analysis and interpretation mostly on the raw values, because n was limited to two. In the Results section, instead of averages, the values for both replicates of each treatment are presented, with the first value always representing the first replicate and the second value the second replicate.
3. Results
3.1. Soil quality parameters
3.1.1. pH response
pH measured in the leachate increased from 5.16 (day 0) to 7.48–7.93 for wollastonite treatments, to 7.30–7.41 for olivine treatments, to 7.42–7.10 for albite treatments, to 6.55–7.58 for basalt treatments, and to 6.87–7.24 for anorthite treatments at the end of the experiment (Figure 3). Treatments did not differ significantly in their pH response (P = 0.387). The pH of the control fluctuated during most of the experiment between approximately 5.5 and 6.0, and increased to 6.71 at the end of the experiment.
3.1.2. Soil nutrient content and retention
Final soil base saturation differed significantly between treatments (P = 0.093; Figure 4A). All treatments increased the base saturation compared to the control (41.7%), being most pronounced for the olivine (78.9–89.0%) and wollastonite (79.8–81.9%) treatments, followed by basalt (73.9–63.9%) and albite (63.7–64.3%). The anorthite treatments showed the smallest increase in base saturation (44.8–44.8%). Furthermore, significant differences between treatments were observed for soil CEC (P = 0.089; Figure 4B). Like with base saturation, the olivine (39.5–47.2 mmol+ kg−1 soil), and wollastonite (40.5–34.1 mmol+ kg−1 soil) treatments strongly increased soil CEC compared to the control (22.4 mmol+ kg−1 soil). Soil CEC of the basalt (31.9–26.5 mmol+ kg−1 soil) and albite (25.6–27.7 mmol+ kg−1 soil) treatments showed slight increases, whereas the anorthite treatments had a soil CEC (21.8–22.2 mmol+ kg−1 soil) that was similar to the control.
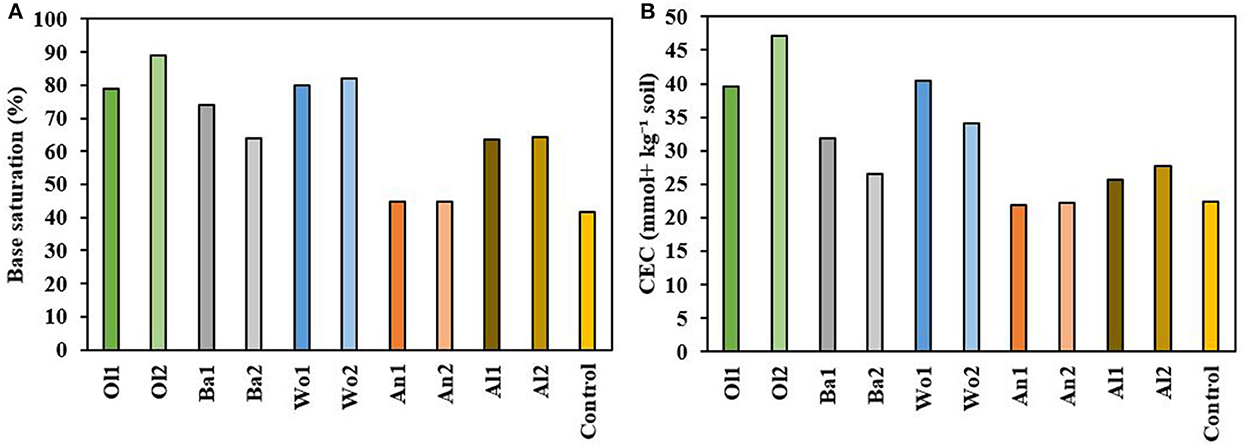
Figure 4. (A) Soil base saturation (%) measured at the end of the experiment. (B) Soil CEC (mmol+ kg−1 soil) measured at the end of the experiment.
3.1.3. Nickel concentrations in soil and leachate
The treatments differed significantly in their soil Ni content as extracted by 0.43 M HNO3 at the end of the experiment (P = 0.093; Figure 5). Olivine amendment resulted in the highest soil Ni concentrations (1.87–9.73 mg kg−1 soil), followed by basalt amendment (2.12–0.74 mg kg−1 soil). Wollastonite, anorthite, and albite amendment did not increase the geochemically reactive soil Ni content, as the measured concentrations were similar to those measured in the control soil (0.55 mg kg−1 soil). The differences in cumulative Ni leaching were also significant between treatments (P = 0.081), being highest for olivine (0.14–0.18 mg kg−1 soil), followed by wollastonite (0.11–0.10 mg kg−1 soil), and basalt (0.10–0.10 mg kg−1 soil). Ni leaching from soils amended with anorthite and albite was similar to the control (0.08 mg kg−1 soil). While none of the treatments produced soil Ni concentrations above the European threshold value for agricultural soils (50 mg Ni kg−1 soil; Tóth et al., 2016), all treatments and the control showed Ni2+ concentrations in individual leachate samples above the Dutch groundwater threshold value during the first 2 weeks of the experiment (Figure 6; 20 μg L−1; Besluit kwaliteitseisen en monitoring water, 2009). Afterwards, all treatments and the control had Ni2+ concentrations below this threshold value, except for soils amended with olivine.
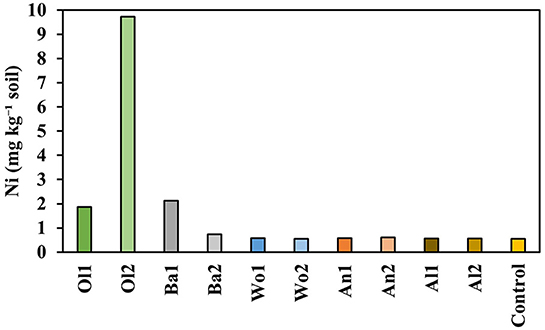
Figure 5. Geochemically reactive soil Ni concentrations at the end of the experiment, based on 0.43 M HNO3 extraction.
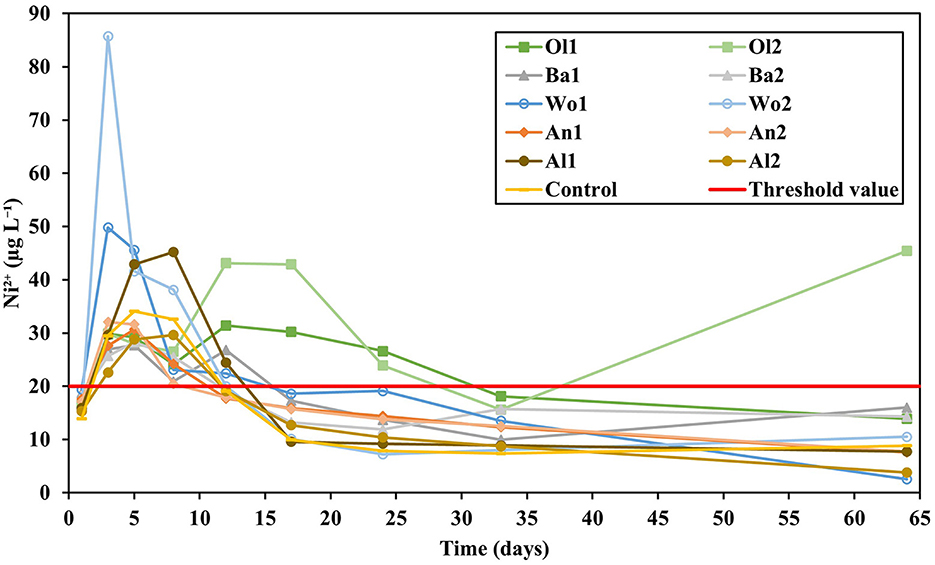
Figure 6. Ni2+ concentrations measured in the leachate over time. The red line represents the Dutch threshold value for Ni2+ concentrations in groundwater (Besluit kwaliteitseisen en monitoring water, 2009).
3.2. Soil CO2 sequestration parameters
3.2.1. CO2 capture by EW
Cumulative CO2 capture was calculated using elemental mass balances (EMB-method) and IC measurements (IC-method) (Figure 7A). Using the EMB-method, CO2 capture differed significantly between treatments (P = 0.081). Highest CO2 capture was observed for soils amended with olivine (1.82–4.45 g CO2 kg−1 soil), and wollastonite (2.95–1.91 g CO2 kg−1 soil), followed by basalt (1.61–0.41 g CO2 kg−1 soil), albite (0.31–0.39 g CO2 kg−1 soil), and anorthite (0.18–0.30 g CO2 kg−1 soil). The IC-method resulted in a lower calculated CO2 capture compared to the EMB-method, but similar trends were observed and treatments differed again significantly (P = 0.078). Using the IC-method, highest CO2 capture was observed for wollastonite (0.61–0.63 g CO2 kg−1 soil), and olivine (0.60–0.58 g CO2 kg−1 soil), followed by basalt (0.38–0.47 g CO2 kg−1 soil), albite (0.19–0.13 g CO2 kg−1 soil), and anorthite (0.15–0.09 g CO2 kg−1 soil).
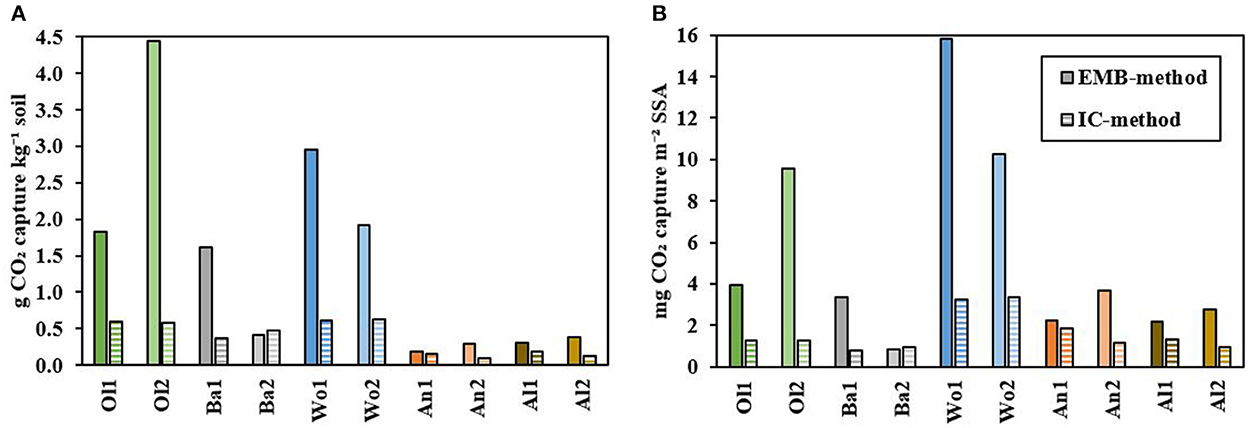
Figure 7. (A) Cumulative CO2 capture calculated with the EMB-method (Equations 1 and 2; filled bars) and IC-method (Equation 3; striped bars). (B) CO2 capture per m2 specific surface area (SSA) calculated with the EMB-method (filled bars) and IC-method (striped bars).
Figure 1 and Table 2 show however that the silicates differed in their particle size distribution, and consequently SSA, being highest for basalt and olivine, and lowest for albite and anorthite. Corrected for these differences in SSA (Figure 7B), wollastonite weathering resulted in the highest CO2 capture for both the EMB-method (15.8–10.3 mg CO2 m−2 SSA) and IC-method (3.25–3.37 mg CO2 m−2 SSA). Furthermore, Figure 7B shows a relatively higher potential for anorthite (2.22–3.70 and 1.87–1.13 mg CO2 m−2 SSA) and albite (2.17–2.75 and 1.34–0.93 mg CO2 m−2 SSA) using the EMB-method and IC-method, respectively, being similar to olivine weathering using the IC-method (1.28–1.25 mg CO2 m−2 SSA). For the latter, CO2 capture based on the EMB-method (3.93–9.58 mg CO2 m−2 SSA) remained substantially higher and second after wollastonite. Basalt weathering resulted on average in the lowest CO2 capture per m2 SSA for both the EMB-method (3.33–0.84 mg CO2 m−2 SSA) and IC-method (0.77–0.97 mg CO2 m−2 SSA). However, treatments did not differ significantly for SSA corrected CO2 capture (P = 0.120 and P = 0.169, EMB-method and IC-method, respectively).
3.2.2. SOC content
SOC contents measured at the end of the experiment did not differ significantly between treatments (P = 0.225; Figure 8). SOC values ranged from 18.4–17.9 g SOC kg−1 soil for the olivine treatments to 19.9–21.6 g SOC kg−1 soil for the wollastonite treatments, while the other treatments had values similar to the control (19.4 g SOC kg−1 soil).
3.2.3. Net CO2 budgets
To assess the net effect of EW in terms of CO2 sequestration or emissions, organic and inorganic carbon budgets were calculated, and added to determine the net CO2 budget (Equation 4; Figure 9). IC budgets resulted in positive values for all treatments, indicating CO2 sequestration. However, OC budgets showed generally negative values, indicating a loss of organic C as CO2 emissions (except Wo2). Overall, the net CO2 budget was negative for most treatments and the control, and no significant differences were observed between treatments (P = 0.496). These net CO2 budgets showed that most treatments produced more CO2 than they captured during this short-term experiment (filled bars in Figure 9), with Wo2 (3.10 g CO2 kg−1 soil) as a positive exception.
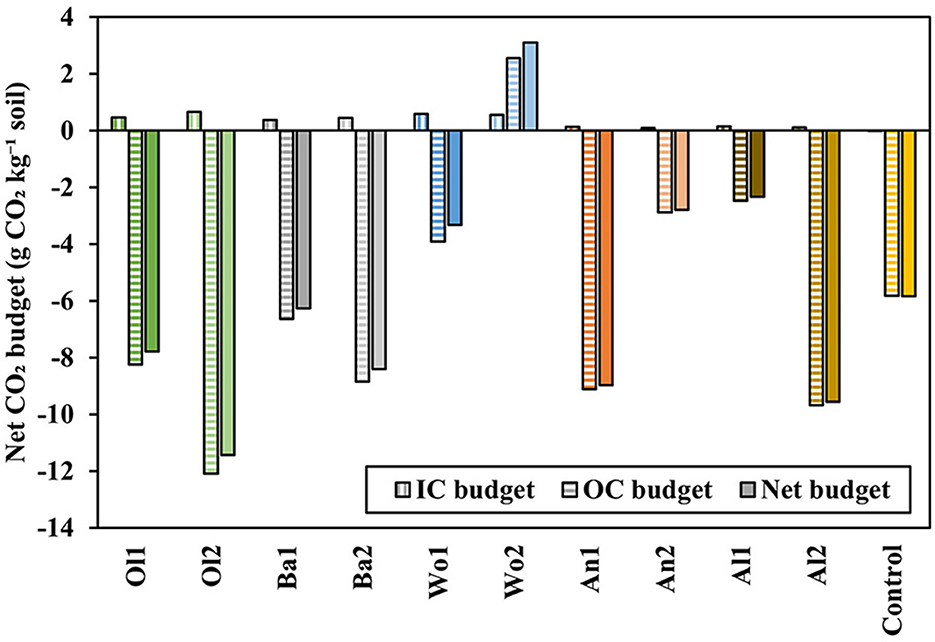
Figure 9. Net CO2 budgets (Equation 4). Positive values indicate CO2 sequestration. Negative values indicate CO2 emissions.
4. Discussion
The aim of this study was to compare five different silicates on their enhanced weathering potential, including effects on both soil quality and CO2 sequestration. Therefore, we designed a down-flow soil column experiment to allow measurements on soils and leachate. It must be noted that the water flow through some of the columns slowed down over time, resulting in a longer residence time of water in the soil. Upon dismantling the columns, dark coloring was observed suggesting reduced conditions. The release of dissolved iron (Fe2+) into soil solution, and subsequent contact with oxygen supplied through the funnel, resulted in visually noticeable precipitation of Fe-oxides in the outlet of these columns, thereby reducing the through-flow of water. These features occurred especially in Ol1, Ba2, and Wo2, and to a lesser extent in An1, An2, Al1, and Control. The reduced flow of water along the mineral particles may have limited the chemical weathering rates and release of elements, likely explaining the relatively large variation between duplicates for some parameters, for example in the soil Ni content of the olivine treatments (Figure 5). Nevertheless, this study generated valuable new insights into the effects of EW on several soil quality parameters, Ni accumulation and release, and net CO2 budgets.
4.1. Potential of EW to improve soil quality
4.1.1. Silicates as liming agents
The effects of EW on soil quality were determined through the pH response, base cation availability and retention, and Ni accumulation and release. During the first few days of the experiment, all treatments temporarily lowered pH as the cations released by EW exchanged with H+ on negatively charged binding sites in soils (Figure 3). This observation was most pronounced for the wollastonite and olivine treatments, as their weathering mainly released multivalent cations (Ca2+ and Mg2+), which are more strongly adsorbed onto exchange sites than K+ and Na+ (Supplementary Figure S1; Sparks, 2003). After about 10 days, pH started to gradually increase for all treatments as a result of proton consumption and alkalinity production by silicate weathering (Figure 3).
The optimum soil pH range for crop growth differs between species, but is generally between pH 6.0 and 8.0 (Fernández and Hoeft, 2009). Weathering of all five silicates raised pH within this optimum range without significant differences between the silicates, indicating their suitability as liming agents. Albite was the first mineral to raise pH above 6.0, within 12 days, which is likely due to its high alkalinity production (Supplementary Figure S2). Weathering of wollastonite, olivine, and basalt raised pH above 6.0 after 15 to 17 days. Anorthite weathering took 33 days to raise pH above 6.0, resulting in a longer period of soil acidity. However, the anorthite minerals used had the lowest SSA, likely explaining their lower weathering rates, and associated pH response and alkalinity production (Supplementary Figure S2). Wollastonite and olivine treatments showed the highest pH response, with individual measurements around pH 8.0 (Figure 3). These results do not necessarily indicate the occurrence of undesired pH increases in agricultural practice, as we applied a relatively high mineral dose. Furthermore, organic acids produced by plants lower pH, but these were absent in this experiment (Haque et al., 2019b; Vicca et al., 2022).
4.1.2. Silicates as fertilizers
The release of base cations by silicate weathering resulted in a significant increase in base saturation (Figure 4A). This increase was most pronounced for the wollastonite and olivine treatments, which mainly released Ca, Mg, and K into soil, three essential plant nutrients (Supplementary Figure S3; Schlesinger and Bernhardt, 2013). Relatively higher Na concentrations were measured in soils of the albite and basalt treatments, but these values are expected to have neither beneficial nor adverse effects on plant productivity (Kronzucker et al., 2013).
Olivine and wollastonite amendment almost doubled soil CEC (Figure 4B). Their strong increase in pH, and the consequent deprotonation of functional groups, likely increased soil negative charge (McBride, 1994; Anda et al., 2015), and thereby cation retention in soils. Furthermore, incongruent weathering may have resulted in the formation of secondary clay minerals that can increase soil CEC (Schlesinger and Bernhardt, 2013), but we do not have observations that confirm a significant occurrence of these processes. Basalt, anorthite, and albite treatments had only limited effect on soil CEC. For anorthite and albite these limited effects may be related to their relatively low SSA, and consequently lower weathering rates and effects on soil quality parameters measured in this study. However, previous studies applying basalt to highly weathered soils reported substantial increases in soil CEC (Anda et al., 2013, 2015) and therefore more research is suggested to understand the effects of EW application on soil CEC. If EW, in addition to releasing nutrients, also improves the retention of these and other nutrients that are not released by silicates such as nitrogen, EW could reduce the use and environmental impact of conventional fertilizers.
4.1.3. Nickel: Pollution or nutrition?
Wollastonite, anorthite, and albite amendment did not increase the Ni content of soils as extracted by 0.43 M HNO3 (Figure 5). Basalt and especially olivine amendment increased soil Ni content, but concentrations were well below the European threshold value of 50 mg Ni kg−1 soil (Tóth et al., 2016). In fact, all treatments increased Ni to concentrations at which it may act as an essential micronutrient. Pot experiments fertilizing soil with 0.5–10 mg Ni kg−1 soil, the range in which Ni concentrations were observed in this experiment (Figure 5), showed significant increases in the yields of barley and soy beans (Kumar et al., 2018; Siqueira Freitas et al., 2018). The effect of Ni as micronutrient can be indirect through enhancing the plant uptake of other micronutrients such as iron, copper, zinc, and manganese (Kumar et al., 2018), and direct through enhancing the enzymatic activity of urease and hydrogenase supporting photosynthetic activity and nitrogen assimilation, respectively (Kumar et al., 2018; Siqueira Freitas et al., 2018). Further research is recommended on these potential fertilization effects of Ni released by olivine and basalt weathering, as this may have implications for the application of silicates containing Ni in agricultural practice.
In contrast, Ni2+ concentrations measured in leachate samples of all treatments exceeded the Dutch threshold value for groundwater (20 μg L−1; Besluit kwaliteitseisen en monitoring water, 2009) during the first 2 weeks of the experiment (Figure 6). This exceedance is not solely an effect of silicate weathering as the control also exceeded the threshold value during this period. The initial reduction in pH (Figure 3) likely underlies this observation, as Ni mobility is higher at lower pH (Dijkstra et al., 2004). The pH was most strongly reduced in the wollastonite treatments, which presumably explains the substantially higher Ni2+ leachate concentrations compared the control. It is therefore likely that Ni was mainly mobilized from the soil itself and less by mineral weathering considering the comparably low Ni content of wollastonite (Table 2). However, this result shows that not only silicates with a high Ni content, but also fast weathering silicates with a low Ni content can induce undesired (short-term) environmental effects through pH alterations.
After 2 weeks, the control and all treatments showed Ni2+ concentrations below the threshold value, except the olivine treatments. While for Ol1 Ni mobility was likely reduced under anoxic conditions (Römkens et al., 2009), Ol2 increasingly exceeded the threshold value during the second half of the experiment. It should be noted here that the measured DOC concentrations were high (Supplementary Figure S4), possibly resulting from the additions of de-mineralised water that has a lower ionic strength than rainwater. DOC may have formed complexes with Ni, thereby increasing its mobility (Dijkstra et al., 2004). As a result, in natural soil environments with rain water additions, DOC and consequently Ni2+ concentrations may be lower than observed in our experiment. However, in a mesocosm experiment Amann et al. (2020) also measured Ni2+ concentrations in the surface layers of olivine-rich dunite amended soils that exceeded drinking water threshold values. Therefore, it remains unclear whether toxic Ni2+ concentrations will also be reached in field conditions.
Our results show that Ni release by EW is not adversely affecting soil quality, especially since at low concentrations Ni can serve as a micronutrient, and the increase in soil pH and CEC can immobilize higher Ni concentrations (Römkens et al., 2009). However, Ni2+ release by olivine amendment, and mobilization of native Ni by fast weathering minerals such as wollastonite, may adversely affect groundwater quality. Therefore, we encourage others not only to monitor Ni concentrations in soils and plants, but especially also Ni2+ leaching. In addition, further research may establish whether an increase in soil binding capacity induced by EW can mitigate Ni2+ leaching.
4.2. EW as a climate change mitigation strategy
4.2.1. Comparing methods to calculate CO2 capture by EW
Since a standardized quantification method of CO2 capture by EW is yet to be developed, two methods based on different principles have been compared. The EMB-method was based on elemental mass balances using the concentrations of Mg for olivine and basalt, Ca for wollastonite and anorthite, and Na for albite, as measured in the leachate and extracted from soils (Equations 1 and 2; Figure 7A, filled bars). The 0.43 M HNO3 extraction allowed to measure both bioavailable elements and those absorbed onto soil particles, to avoid an underestimation of calculated weathering rates based on a 0.01 M CaCl2 extraction that only measures the bioavailable pool (Römkens et al., 2009; Ten Berge et al., 2012). However, the 0.43 M HNO3 extraction can also partly dissolve minerals (Rodrigues et al., 2010; Groenenberg et al., 2017). Although minerals on top of the soils were visually removed, some downward transported mineral particles may have remained in the soil and partly dissolved by the extraction, thereby causing a potential overestimation of the CO2 capture calculated with the EMB-method. To quantify the potential partial mineral dissolution, we conducted a 0.43 M HNO3 extraction on the five silicates studied (Supplementary Table S2). For olivine, basalt, anorthite, and albite similar dissolution rates were found, being 15.0% or lower (Mg-olivine: 15.0%, Mg-basalt: 14.7%, Ca-anorthite: 2.26%, Na-albite: 12.8%). For Ca contained in wollastonite we found a substantially higher dissolution rate, namely 46.6%. However, the differences in calculated CO2 capture between the EMB-method and IC-method are more than 15.0 and 46.6%, respectively. Mineral dissolution by the 0.43 M HNO3 extraction can therefore only partly explain the observed discrepancy between the two methods.
For the IC-method, the alkalinity concentrations measured in the leachate and the soil carbonate content measured with the Scheibler method were used, representing the two pathways in which captured CO2 can be stored (Equation 3; Figure 7A, striped bars). Alkalinity production and carbonate precipitation were highest for the olivine and wollastonite treatments (Supplementary Figures S2, S5), which is related to their high pH response (Figure 3) and DIC concentrations (Supplementary Figure S6). Albite weathering resulted in alkalinity concentrations similarly high as wollastonite weathering, while the soil carbonate contents of the five treatments was lowest for albite. This finding is consistent with albite releasing mainly Na+ in the leachate while Na2CO3 does not precipitate (Hartmann et al., 2013), thereby making conditions for carbonate precipitation, by which part of the captured CO2 is released, less favorable compared to the other treatments. As with the EMB-method, the IC-method contains some uncertainties. We assumed that the measured carbonates consisted of CaCO3, since CaCO3 is thermodynamically more stable than MgCO3 and most treatments showed relatively higher Ca2+ than Mg2+ release (Supplementary Figure S1). However, the exact carbonate mineral that was formed is unknown. Renforth et al. (2015) found in their soil column experiment using olivine that the formed carbonates consisted mainly of CaCO3 with only small amounts of MgCO3. Therefore, we do not expect our assumption to introduce a large error. Furthermore, alkalinity concentrations were assumed to fully consist of , which seems valid as the leachate samples all had a pH between 4.50 and 8.25 in which is the dominant DIC species (Verma, 2004). However, organic substances may have been present contributing to the measured alkalinity (Pearson, 1981), potentially causing an overestimation of the calculated CO2 capture using the IC-method. Different methodologies are used in literature for quantifying CO2 capture by EW, which are all based on different assumptions and uncertainties thereby hindering a comparison between studies. Therefore, we strongly encourage the use and comparison of multiple methodologies in future EW studies, as we have done here. Such a multiple approach can serve the development of a harmonized methodology to calculate CO2 capture by EW, which we consider an important target to enable a robust comparison of achieved CO2 capture in different EW studies.
To compare the silicates on CO2 capture, we need to consider the lower SSA of anorthite, albite, and wollastonite compared to olivine and basalt (Table 2). Based on BET measurements, CO2 capture per m2 SSA was calculated (Figure 7B). This calculation includes the assumption that the SSA does not change during our 64-day experiment. This assumption may not be fully valid, since secondary minerals may precipitate on reactive mineral surfaces, thereby reducing the SSA available for chemical weathering, and consequently CO2 capture over time (Huijgen et al., 2006; Amann et al., 2020). However, Scanning Electron Microscopy analysis of weathered relative to fresh mineral samples did not show visual evidence for secondary mineral precipitation on reactive surfaces (Supplementary Figure S7). Figure 7B shows that both methods indicate a high potential for CO2 capture by wollastonite weathering, and a low potential for basalt weathering. Furthermore, when CO2 capture is expressed per m2 SSA, a relatively higher potential for minerals that are novel in the context of EW, anorthite and albite, was observed. Since the low SSA of the used anorthite and albite minerals likely affected the measured weathering rates in our experiment, and thereby other results presented in this study, more research on the enhanced weathering potential of these and possibly other novel minerals in the EW field is encouraged.
4.2.2. Impact of EW on SOC content
All treatments showed non-significant changes in SOC content (Figure 8). Small reductions in SOC content compared to the control soil, as observed for especially olivine treatments, were expected for a short-term experiment in which pH strongly increased and OC inputs by plants were absent. Rising soil pH enhances microbial activity and consequently SOC decomposition, especially when pH is between 6.0 and 8.0 (Maier and Pepper, 2009; Leifeld et al., 2013; Paradelo et al., 2015). In addition, the absence of plants in our study played a role. Improved productivity resulting from EW is hypothesized to increase belowground plant OC inputs, which could (partly) counterbalance SOC losses (Vicca et al., 2022). Furthermore, results might have been different if a clayey soil was used instead of a sandy soil, as clayey soils generally have a higher amount of aggregates and reactive minerals that can stabilize SOC (Hassink et al., 1997). As SOC changes generally take place on a longer time scale, we recommend to include monitoring of SOC dynamics in long-term EW experiments.
4.2.3. EW: Net CO2 sequestration or net CO2 emissions?
The calculated net CO2 budgets show that for most treatments SIC sequestration by EW was counterbalanced by a loss of SOC as CO2 emissions (Figure 9). As indicated above, we show results for a short-term experiment (64 days) in which a strong increase in pH was observed. Therefore, the strong pH effect on SOC decomposition was likely dominant over the CO2 captured by EW. On the longer term, we hypothesize that SOC decomposition rates decrease, and CO2 sequestration can become the dominant process, yet long-term data of EW experiments are still limited. Since we observed substantial variation between duplicates, the positive budget, i.e., net CO2 sequestration, for Wo2 cannot be interpreted as significant. Nevertheless, these calculations demonstrate that, in addition to measuring CO2 capture by EW, calculating net organic and inorganic carbon budgets is a valuable addition to current EW research in the context of the global transition toward net negative emissions.
CO2 sequestration as SIC may support climate change mitigation beyond the human time scale (Andrews and Taylor, 2019; Haque et al., 2019a). However, especially when EW is applied to agricultural soils, land management strategies may control the stability of C. SIC is stable as long as land management, especially pH management, is maintained, i.e., frequent application of crushed silicates (Kantola et al., 2017; Haque et al., 2019b). Increasing soil pH favors carbonate precipitation and stability, but may have contradictory effects on SOC as outlined in the previous paragraph. Whether C storage is truly long term, i.e., beyond human time scales, is highly dependent on land management practices. Therefore, these net CO2 budgets are important information to land managers to maintain and enhance C storage in soils. In conclusion, we strongly encourage other authors to calculate organic and inorganic carbon budgets to allow an assessment of the net effects of EW on soil CO2 sequestration.
5. Conclusions and outlook
The ultimate aim of this study was to compare five different silicates on their enhanced weathering potential, which includes impacts on both soil quality and CO2 sequestration. A down-flow soil column experiment was used to measure effects on leachate and quantify organic and inorganic carbon budgets, both underrepresented topics in current EW literature. All silicate treatments generally improved soil quality, as indicated by their increases in pH, base saturation, and CEC. Olivine and wollastonite treatments showed the strongest increases in soil base saturation and CEC, and showed comparatively high CO2 capture. Based on the conditions and the two methodologies to calculate CO2 capture used in this study, application of olivine or wollastonite minerals on global cropland could capture 0.43–2.30 or 0.45–1.78 t CO2 ha−1, respectively. These values represent between 9 and 50% of global anthropogenic CO2 emissions in 2018 as reported by Jackson et al. (2019). We should note here that chemical weathering rates measured in laboratory experiments likely differ from those measured in field conditions, and differ per soil type and climatic region (Renforth et al., 2015; Beerling et al., 2020), and that the application of a single silicate on global cropland is rather unlikely given their estimated availability and distribution. Furthermore, calculated net CO2 budgets indicated that inorganic CO2 sequestration was, at least on the short term, counterbalanced by a loss of organic C, highlighting the importance of quantifying net CO2 budgets. Nevertheless, this simplified scenario shows the large potential of EW to contribute to the transition toward net negative emissions on a global scale.
We found a high enhanced weathering potential for wollastonite minerals, both in terms of CO2 sequestration and soil quality improvements, while the potential of olivine minerals may be constrained by Ni2+ leaching. Therefore, we recommend other authors to not only measure Ni concentrations in soils and plants, but also monitor Ni2+ leaching. While the use of basalt was recently suggested as an alternative to olivine due to potential Ni contamination by the latter (Beerling et al., 2018; Kelland et al., 2020), we suggest that the use of feldspar minerals should also be considered, since anorthite and albite released limited Ni into soils and leachate and showed potential for high CO2 capture. Albite treatments produced concentrations similarly high as wollastonite treatments, which is not only a long-term inorganic C sink, but also contributes to mitigation of ocean acidification, an important consequence of climate change (Hartmann et al., 2013; Andrews and Taylor, 2019; Bach et al., 2019). Our anorthite and albite treatments showed promising results, however these feldspar minerals deserve further investigation as their low SSA in our experiment not fully allowed us to quantify their enhanced weathering potential. Finally, we also strongly encourage other authors to include silicate minerals and rocks that are novel in the EW context in their experimental design to allow a wider exploration of the full potential of EW as a NET.
Data availability statement
The original contributions presented in the study are included in the article/Supplementary material. Further inquiries can be directed to the corresponding author.
Author contributions
EP conceptualized the research and designed the experiment under the supervision of MH and RC. EP conducted the experiment and performed the chemical analyses in the laboratory, analyzed, visualized, and interpreted the data, and wrote the manuscript. MH and RC supervised the project, data analysis, writing of the manuscript, and revised and improved the manuscript. All authors approved the submitted version of the manuscript.
Funding
This work was supported through internal funding.
Acknowledgments
We thank Pol Knops for providing the olivine minerals, Huig Bergsma for providing the basalt, and Rafael Mattos Dos Santos for providing the wollastonite minerals for this experiment. Furthermore, we thank the laboratory staff, Gerlinde Vink, Miranda Vlag, Erna van den Hengel-Voskuilen, Peter Nobels, Andre van Leeuwen, Wobbe Schuurmans, Wim Pape, and Johan Uijtenbroek, for supporting the laboratory experiment and chemical analyses. We also thank Henk-Jan Koudijs for constructing the set-up of the down-flow soil column experiment. Finally, we thank the reviewers for their constructive comments that improved the quality of this manuscript.
Conflict of interest
The authors declare that the research was conducted in the absence of any commercial or financial relationships that could be construed as a potential conflict of interest.
Publisher's note
All claims expressed in this article are solely those of the authors and do not necessarily represent those of their affiliated organizations, or those of the publisher, the editors and the reviewers. Any product that may be evaluated in this article, or claim that may be made by its manufacturer, is not guaranteed or endorsed by the publisher.
Supplementary material
The Supplementary Material for this article can be found online at: https://www.frontiersin.org/articles/10.3389/fclim.2022.954064/full#supplementary-material
References
Actimin. (2019). Composition stonemeal Actimin-BT. Available online at: www.actimin.nl/analyse/ (accessed October 4, 2019).
Allison, L. (1960). Wet-combustion apparatus and procedure for organic and inorganic carbon in soil. Soil Sci. Soc. Am. J. 24, 36–40. doi: 10.2136/sssaj1960.03615995002400010018x
Amann, T., Hartmann, J., Struyf, E., Oliveira Garcia, d. e., Fischer, W., Janssens, E. K., et al. (2020). Enhanced Weathering and related element fluxes–a cropland mesocosm approach. Biogeosciences 17, 103–119. doi: 10.5194/bg-17-103-2020
Anda, M., Shamshuddin, J., and Fauziah, C. (2013). Increasing negative charge and nutrient contents of a highly weathered soil using basalt and rice husk to promote cocoa growth under field conditions. Soil Tillage Res. 132, 1–11. doi: 10.1016/j.still.2013.04.005
Anda, M., Shamshuddin, J., and Fauziah, C. (2015). Improving chemical properties of a highly weathered soil using finely ground basalt rocks. Catena 124, 147–161. doi: 10.1016/j.catena.2014.09.012
Andrews, M. G., and Taylor, L. L. (2019). Combating climate change through enhanced weathering of agricultural soils. Elem. Int. Mag. Mineral. Geochem. Petrol. 15, 253–258. doi: 10.2138/gselements.15.4.253
Bach, L. T., Gill, S., Rickaby, R., Gore, S., and Renforth, P. (2019), CO2. removal with enhanced weathering ocean alkalinity enhancement: Potential risks co-benefits for marine pelagic ecosystems. Front. Clim. 1, 7. doi: 10.3389/fclim.2019.00007
Beerling, D. J., Kantzas, E. P., Lomas, M. R., Wade, P., Eufrasio, R. M., Renforth, P., et al. (2020). Potential for large-scale CO2 removal via enhanced rock weathering with croplands. Nature 583, 242–248. doi: 10.1038/s41586-020-2448-9
Beerling, D. J., Leake, J. R., Long, S. P., Scholes, J. D., Ton, J., Nelson, P. N., et al. (2018). Farming with crops and rocks to address global climate, food and soil security. Nature Plants 4, 392–392. doi: 10.1038/s41477-018-0162-5
Besluit kwaliteitseisen en monitoring water. (2009). (Decree on quality requirements and monitoring water). Available online at: https://wetten.overheid.nl/BWBR0027061/2017-01-01 (accessed September 16, 2022).
Dietzen, C., Harrison, R., and Michelsen-Correa, S. (2018). Effectiveness of enhanced mineral weathering as a carbon sequestration tool and alternative to agricultural lime: an incubation experiment. Int. J. Greenhouse Gas Control 74, 251–258. doi: 10.1016/j.ijggc.2018.05.007
Dijkstra, J. J., Meeussen, J. C., and Comans, R. N. (2004). Leaching of heavy metals from contaminated soils: an experimental and modeling study. Environ. Sci. Technol. 38, 4390–4395. doi: 10.1021/es049885v
Fernández, F. G., and Hoeft, R. G. (2009). Managing soil pH and crop nutrients. Illinois Agron. Handbook 24, 91–112.
Fox, J., and Weisberg, S. (2019). An R Companion to Applied Regression (third edition). California: Sage.
Gao, J., Mikutta, R., Jansen, B., Guggenberger, G., Vogel, C., Kalbitz, K., et al. (2020). The multilayer model of soil mineral–organic interfaces—a review. J. Plant Nutri. Soil Sci. 183, 27–41. doi: 10.1002/jpln.201900530
Gilbert, O., Hernández, M., Vilanova, E., and Cornellà, O. (2014). Guidelining protocol for soil-column experiments assessing fate and transport of trace organics. Demeau, Brussels, Belgium 3, 54.
Gillman, G. (1980). The effect of crushed basalt scoria on the cation exchange properties of a highly weathered soil. Soil Sci. Soc. Am. J. 44, 465–468. doi: 10.2136/sssaj1980.03615995004400030005x
Gillman, G., Burkett, D., and Coventry, R. (2002). Amending highly weathered soils with finely ground basalt rock. Appl. Geochem. 17, 987–1001. doi: 10.1016/S0883-2927(02)00078-1
Groenenberg, J. E., Römkens, P. F., Zomeren, A. V., Rodrigues, S. M., and Comans, R. N. (2017). Evaluation of the single dilute (0.43 M) nitric acid extraction to determine geochemically reactive elements in soil. Environ. Sci. Technol. 51, 2246–2253. doi: 10.1021/acs.est.6b05151
Haque, F., Chiang, Y. W., and Santos, R. M. (2019a). Alkaline mineral soil amendment: a climate change ‘stabilization wedge'? Energies 12, 2299. doi: 10.3390/en12122299
Haque, F., Santos, R. M., Dutta, A., Thimmanagari, M., and Chiang, Y. W. (2019b). Co-benefits of wollastonite weathering in agriculture: CO2 sequestration and promoted plant growth. ACS Omega 4, 1425–1433. doi: 10.1021/acsomega.8b02477
Harley, A., and Gilkes, R. (2000). Factors influencing the release of plant nutrient elements from silicate rock powders: a geochemical overview. Nut. Cycl. Agroecosys. 56, 11–36. doi: 10.1023/A:1009859309453
Hartmann, J., West, A. J., Renforth, P., Köhler, P., La Rocha, D. e. C. L., Wolf-Gladrow, D. A., et al. (2013). Enhanced chemical weathering as a geoengineering strategy to reduce atmospheric carbon dioxide, supply nutrients, and mitigate ocean acidification. Rev. Geophy. 51, 113–149. doi: 10.1002/rog.20004
Hassink, J., Whitmore, A. P., and Kubát, J. (1997). Size and density fractionation of soil organic matter and the physical capacity of soils to protect organic matter. Eur. J. Agron. 7, 189–199. doi: 10.1016/S1161-0301(97)00045-2
Huijgen, W. J., Witkamp, G-. J., and Comans, R. N. (2006). Mechanisms of aqueous wollastonite carbonation as a possible CO2 sequestration process. Chem. Eng. Sci. 61, 4242–4251. doi: 10.1016/j.ces.2006.01.048
IPCC. (2014). Climate Change 2014: Synthesis Report. Fifth Assessment Report of the Intergovernmental Panel on Climate Change. In. Geneva, Switzerland.
IPCC. (2018). Global Warming of 1, 5.°C. Special Report of the Intergovernmental Panel on Climate Change. In: Incheon, South Korea.
ISO 10693. (1995). Soil Quality - Determination of Carbonate Content - Volumetric Method. International Organization for Standardization.
ISO 11260. (2018). Soil Quality - Determination of Effective Cation Exchange Capacity and Base Saturation Level Using Barium Chloride Solution. International Organization for Standardization.
ISO 21268-3. (2019). Soil Quality - Leaching Procedures for Subsequent Chemical and Ecotoxicological Testing of Soil and Soil-Like Materials - Part 3: Up-Flow Percolation Test. International Organization for Standardization.
Jackson, R., Friedlingstein, P., Andrew, R., Canadell, J., Le Quéré, C., Peters, G., et al. (2019). Persistent fossil fuel growth threatens the Paris agreement and planetary health. Environ. Res. Lett. 14, 121001. doi: 10.1088/1748-9326/ab57b3
Kantola, I. B., Masters, M. D., Beerling, D. J., Long, S. P., and DeLucia, E. H. (2017). Potential of global croplands and bioenergy crops for climate change mitigation through deployment for enhanced weathering. Biol. Lett. 13, 20160714. doi: 10.1098/rsbl.2016.0714
Kelland, M. E., Wade, P. W., Lewis, A. L., Taylor, L. L., Sarkar, B., Andrews, M. G., et al. (2020). Increased yield and CO2 sequestration potential with the C4 cereal Sorghum bicolor cultivated in basaltic rock dust-amended agricultural soil. Glob. Chang. Biol. 26, 3658–3676. doi: 10.1111/gcb.15089
Kremer, D., Etzold, S., Boldt, J., Blaum, P., Hahn, K. M., Wotruba, H., et al. (2019). Geological mapping and characterization of possible primary input materials for the mineral sequestration of carbon dioxide in Europe. Minerals 9, 485. doi: 10.3390/min9080485
Kronzucker, H. J., Coskun, D., Schulze, L. M., Wong, J. R., and Britto, D. T. (2013). Sodium as nutrient and toxicant. Plant Soil 369, 1–23. doi: 10.1007/s11104-013-1801-2
Kumar, O., Singh, S., Singh, A., Yadav, S., and Latare, A. (2018). Effect of soil application of nickel on growth, micronutrient concentration and uptake in barley (Hordeum vulgare L.) grown in Inceptisols of Varanasi. J. Plant Nutr. 41, 50–66. doi: 10.1080/01904167.2017.1381724
Kump, L. R., Brantley, S. L., and Arthur, M. A. (2000). Chemical weathering, atmospheric CO2, and climate. Annu. Rev. Earth Planet. Sci. 28, 611–667. doi: 10.1146/annurev.earth.28.1.611
Lehmann, J., and Possinger, A. (2020). Removal of atmospheric CO2 by rock weathering holds promise for mitigating climate change. Nature. 583, 204–205. doi: 10.1038/d41586-020-01965-7
Leifeld, J., Bassin, S., Conen, F., Hajdas, I., Egli, M., Fuhrer, J., et al. (2013). Control of soil pH on turnover of belowground organic matter in subalpine grassland. Biogeochemistry 112, 59–69. doi: 10.1007/s10533-011-9689-5
Maier, R. M., and Pepper, I. L. (2009). Earth environments. In Environmental microbiology: Elsevier (pp. 57-82). doi: 10.1016/B978-0-12-370519-8.00004-3
Moosdorf, N., Renforth, P., and Hartmann, J. (2014). Carbon dioxide efficiency of terrestrial enhanced weathering. Environ. Sci. Technol. 48, 4809–4816. doi: 10.1021/es4052022
NEN (2018). Soil - Determination of Clay Content and Particle Size Distribution in Soil and Sediment by Sieve and Pipet.
Oelkers, E. H., Declercq, J., Saldi, G. D., Gislason, S. R., and Schott, J. (2018). Olivine dissolution rates: a critical review. Chem. Geol. 500, 1–19. doi: 10.1016/j.chemgeo.2018.10.008
Palandri, J. L., and Kharaka, Y. K. (2004). A Compilation of Rate Parameters of Water-Mineral Interaction Kinetics for Application to Geochemical Modeling. California: U.S. Geological Survey. doi: 10.3133/ofr20041068
Paradelo, R., Virto, I., and Chenu, C. (2015). Net effect of liming on soil organic carbon stocks: a review. Agric. Ecosyst. Environ. 202, 98–107. doi: 10.1016/j.agee.2015.01.005
Pearson, F. (1981). Fixed endpoint alkalinity determination. Water Pollution Control Federation 53, 1243–1252.
R Core Team. (2020). R: A Language and Environment for Statistical Computing. Vienna: R Foundation for Statistical Computing. Available online at: https://www.R-project.org/
Renforth, P., von Strandmann, P. P., and Henderson, G. (2015). The dissolution of olivine added to soil: implications for enhanced weathering. Ap. Geochem. 61, 109–118. doi: 10.1016/j.apgeochem.2015.05.016
Rodrigues, S., Henriques, B., Silva, d. a., Pereira, E. F., Duarte, M. A., and Römkens, P. (2010). Evaluation of an approach for the characterization of reactive and available pools of twenty potentially toxic elements in soils: Part I–The role of key soil properties in the variation of contaminants' reactivity. Chemosphere 81, 1549–1559. doi: 10.1016/j.chemosphere.2010.07.026
Römkens, P. F., Guo, H-. Y., Chu, C. L., Liu, T. S., Chiang, C. F., Koopmans, G. F., et al. (2009). Characterization of soil heavy metal pools in paddy fields in Taiwan: chemical extraction and solid-solution partitioning. J. Soils Sediments 9, 216–228. doi: 10.1007/s11368-009-0075-z
Schlesinger, W., and Bernhardt, E. (2013). Biogeochemistry: An Analysis of Global Change (third edition). Oxford: Academic Press.
Schuiling, R., and Krijgsman, P. (2006). Enhanced weathering: an effective and cheap tool to sequester CO2. Clim. Change 74, 349–354. doi: 10.1007/s10584-005-3485-y
Siqueira Freitas, D., Wurr Rodak, B., Rodrigues dos Reis, A., de Barros Reis, F., Soares de Carvalho, T., Schulze, J., et al. (2018). Hidden nickel deficiency? Nickel fertilization via soil improves nitrogen metabolism and grain yield in soybean genotypes. Front. Plant Sci. 9, 614. doi: 10.3389/fpls.2018.00614
Sparks, D. L. (2003). Environmental Soil Chemistry (Second edition ed.). California: Elsevier Academic Press. doi: 10.1016/B978-012656446-4/50001-3
Ten Berge, H. F., Van der Meer, H. G., Steenhuizen, J. W., Goedhart, P. W., Knops, P., Verhagen, J., et al. (2012). Olivine weathering in soil, and its effects on growth and nutrient uptake in ryegrass (Lolium perenne L.): a pot experiment. PLoS ONE 7, e42098. doi: 10.1371/journal.pone.0042098
Tóth, G., Hermann, T., Silva, D. A., and Montanarella, M. (2016). Heavy metals in agricultural soils of the European Union with implications for food safety. Environ. Int. 88, 299–309. doi: 10.1016/j.envint.2015.12.017
USGS. (2020). Mineral Commodity Summaries 2020. U.S. Geological Survey. Available online at: www.usgs.gov/centers/nmic/mineral-commodity-summaries (accessed June 8, 2021).
Verma, M. P. (2004). A revised analytical method for HCO3-and CO32-determinations in geothermal waters: an assessment of IAGC and IAEA interlaboratory comparisons. Geostandards and Geoanal. Res. 28, 391–409. doi: 10.1111/j.1751-908X.2004.tb00758.x
Vicca, S., Goll, D. S., Hagens, M., Hartmann, J., Janssens, I. A., Neubeck, A., et al. (2022). Is the climate change mitigation effect of enhanced silicate weathering governed by biological processes? Glob. Chang. Biol. 28, 711–726. doi: 10.1111/gcb.15993
Weng, L. P., Wolthoorn, A., Lexmond, T. M., Temminghoff, E. J., and van Riemsdijk, W. H. (2004). Understanding the effects of soil characteristics on phytotoxicity and bioavailability of nickel using speciation models. Environ. Sci. Technol. 38, 156–162. doi: 10.1021/es030053r
Keywords: enhanced weathering potential, silicate minerals and rocks, net CO2 sequestration, agricultural soil quality, nickel, soil column experiment
Citation: te Pas EEEM, Hagens M and Comans RNJ (2023) Assessment of the enhanced weathering potential of different silicate minerals to improve soil quality and sequester CO2. Front. Clim. 4:954064. doi: 10.3389/fclim.2022.954064
Received: 26 May 2022; Accepted: 02 December 2022;
Published: 10 January 2023.
Edited by:
Vikram Vishal, Indian Institute of Technology Bombay, IndiaReviewed by:
Rafael Mattos Dos Santos, University of Guelph, CanadaTamara Jane Zelikova, Colorado State University, United States
Copyright © 2023 te Pas, Hagens and Comans. This is an open-access article distributed under the terms of the Creative Commons Attribution License (CC BY). The use, distribution or reproduction in other forums is permitted, provided the original author(s) and the copyright owner(s) are credited and that the original publication in this journal is cited, in accordance with accepted academic practice. No use, distribution or reproduction is permitted which does not comply with these terms.
*Correspondence: Emily E. E. M. te Pas, ZW1pbHkudGVwYXMmI3gwMDA0MDt3dXIubmw=