- 1Center for Environmental Systems, Stevens Institute of Technology, Hoboken, NJ, United States
- 2Department of Civil, Environmental and Ocean Engineering, Stevens Institute of Technology, Hoboken, NJ, United States
- 3Department of Chemical Engineering and Materials Science, New Jersey Center for Microchemical Systems, Stevens Institute of Technology, Hoboken, NJ, United States
Microalgae cultivation is considered an attractive negative emission technology (NET) due to its ability to remove carbon dioxide (CO2) from the atmosphere as well as from flue gases. Moreover, some microalgae can uptake dissolved carbon in the form of bicarbonate (HCO) and grow well by removing nutrients from various wastewater effluents without competing with freshwater resources. Conventional carbon mineralization is another NET where carbon is fixed into carbonate minerals such as calcite (CaCO3), magnesite (MgCO3), nesquehonite (MgCO3-3H2O), and nahcolite (NaHCO3), which can be reused in various applications such as construction and cosmetics, and in this case, to supply carbon to microalgae. Previous initial laboratory studies demonstrated the possibility of using metastable carbonates as a source of carbon for biomass growth of the freshwater microalga Scenedesmus obliquus. In this study, we present results from an experimental and modeling work where 100 L open raceway reactors were used to grow an algae polyculture using industrial nitrogen-rich wastewater. In addition, carbonates such as MgCO3-3H2O and sodium carbonate (Na2CO3) were added to the ponds to primarily buffer the pH and maintain it within the acceptable algae physiological values and to provide extra carbon to the cultures. Cultures using BG-11 growth medium and without addition of carbonates were run as controls. Continuous online monitoring of pH, temperature, and dissolved oxygen was performed while nutrients content and dry weight of algae culture were measured offline daily. A mathematical model coupled with geochemistry was implemented to describe the overall process of algae growth, carbonate dissolution, and solution composition. Biomass harvested from the raceway reactors was concentrated and oil and fatty acid methyl esters (FAME) content were determined. Results showed that biomass and oil content obtained were comparable to controls, indicating a successful metabolic-based pH control by the addition of carbonates in the ammonium-based media. Furthermore, FAME analysis revealed different profiles depending on the source of carbon used. Overall, this study suggests that integrating both types of NET strategies can contribute to the reduction of carbon emissions while producing biomass that can be further processed to generate a variety of bioproducts.
Introduction
As a result of the growing world population, human and industrial activities, including mainly combustion of fossil fuels and deforestation, increases in carbon dioxide (CO2) and greenhouse gases (GHG) emissions are being observed. Since the Industrial Revolution, atmospheric CO2 concentrations have increased from 280 ppm in 1,750 to 421 ppm in 2022 (NOAA Global Monitoring Laboratory, USA) [National Oceanic and Atmospheric Administration (NOAA), 2021]. The changes in the ecosystems and the environment due to the increasing carbon footprint is actively being assessed and reported by the Intergovernmental Panel on Climate Change (IPCC). This panel, comprising a scientific community of 195 member countries, evaluates the implications and potential future risks, and suggests adaptation and mitigation options such as the implementation of negative emissions technologies (NETs) (Intergovernmental Panel on Climate Change, 2022).
On one hand, Carbon Capture Utilization and Storage (CCUS) techniques aim at mitigating CO2 emissions toward the atmosphere via geological storage, ocean storage, and mineral carbonation (Clarke and Jiang, 2014). Conventional carbon mineralization consists of fixing carbon into stable minerals that can be subsequently used for various industrial applications (IPCC, 2005; Glasser et al., 2016; Woodall et al., 2019). The formation of metastable carbonate phases is preferrable especially if the carbonate minerals are to be used for microalgae growth, as they can precipitate rapidly and dissolve quickly (Ye et al., 2019).
On the other hand, renewable energy sources such as solar, wind, geothermal, and biomass contribute to the reduction of CO2 emissions to the atmosphere. In this sense, photosynthetic microorganisms such as microalgae and cyanobacteria are considered an attractive NET due to their ability to sequester CO2 from the atmosphere as well as from industrial flue gases (Sayre, 2010; Razzak et al., 2013; Durán et al., 2018; Daneshvar et al., 2022). Furthermore, they grow in relatively short time by capturing the energy from the sun and using non-potable water resources, unlike other renewable feedstocks. Moreover, microalgal biomass have been assessed for a wide range of bioproducts, ranging from fuel, fertilizer, bioplastics, to food, cosmetics, and more; representing suitable biorefineries to replace fossil-based products (Khan et al., 2018; Mishra et al., 2019; Dolganyuk et al., 2020).
To date, the feasibility of large-scale algae biomass production and commercialization is still unfavorable partly due to the need for higher algal biomass productivity to reduce high capital and operating costs (Ummalyma et al., 2021). Remarkably, one of the major limiting factors is the carbon supply. In fact, the limitation is not the source of carbon dioxide, but its efficient delivery and utilization in a cost-effective manner. The low solubility of atmospheric CO2 in algal ponds sets a rather low limit to the carbon uptake by algae (de Godos et al., 2014; Chisti, 2016). Transporting pure CO2 (sometimes over long distances) or co-locating algal ponds and flue gas sources are economically unfavorable (Benemann, 2013; Somers and Quinn, 2019). In addition, CO2 supplied in a gaseous form easily escapes from the culture liquor when using the most common culturing systems like open ponds, instead of being captured and used by the algal cells, due to its poor mass transfer efficiency. In consequence, low CO2 fixation rates are obtained. Some studies have explored the utilization of membrane carbonation and carbonation columns and sumps to increase the carbon transfer efficiency into the water (Eustance et al., 2020; Lai et al., 2020). While others, such as Pancha et al. (2015), Najafabadi et al. (2015) and Singh et al. (2022) have studied the use of bicarbonates (HCO) to increase biomass productivity as well as the lipids content. The strategy of feeding HCO is feasible for all the microalgae and cyanobacteria that can successfully convert bicarbonates to carbon dioxide via enzymatic reaction mediated by carbonic anhydrase enzyme, known as a carbon concentrating mechanism (Wang et al., 2015).
Furthermore, the use of waste streams to produce biomass could help the economics of the process in addition to reducing the consumption of natural resources (Lammers et al., 2017). Ammonia/ammonium, one of the most common ions in all types of wastewaters Salbitani and Carfagna, 2021), is the preferred form of nitrogen utilized by microorganisms due to its direct assimilation and low energy requirements compared to other nitrogen forms. However, on one hand, its consumption decreases pond pH as a microalgae response to maintain a physiological pH environment inside the cell, resulting in lower productivity and growth inhibition (Eustance et al., 2013). On the other hand, increasing the pH to 9 can result in toxicity due to the conversion of nitrogen to ammonia. Therefore, the use of ammonia (as ammonium in solution) requires robust pH control.
Our hypothesis is that combining the low pH triggered by the ammonia consumption in the wastewater with metabolic-based pH control using metastable carbonates, which provide bicarbonate as carbon form able to remain in solution, will improve culture conditions and enhance biomass growth as well as carbon transfer and utilization efficiency. In other words, by integrating the fixation of CO2 via photosynthesis (biologically) and the formation of inorganic forms via mineral carbonation (chemically), we aim at increasing the carbon solubility and transfer in the ponds to obtain higher biomass and bioproducts production. Therefore, the objective of this study was to evaluate the growth of a photosynthetic freshwater polyculture using an industrial nitrogen-rich wastewater blend and adding carbonates as metastable magnesium carbonates (nesquehonite) and sodium carbonate as a source of carbon to (1) maintain pH within physiological optimum range and (2) promote healthy growth in comparison to a control (BG-11 culture medium). Moreover, the composition of the biomass by means of lipids content provides a glimpse of its potential for the generation of different bio-based products such as bioenergy. This study aims at integrating CO2 sequestration from different sources (e.g., CO2 capture as metastable minerals and algae fixation of atmospheric CO2 and HCO in solution) to reduce the carbon footprint while producing value-added products.
Materials and methods
Setup and operating conditions
This study was carried out indoors in lab-scale open raceway ponds setup purchased from MicroBio Engineering (California, USA) consisting of 4 reactors with 100 L of working capacity, 20 cm depth and an overall area of 0.5 m2 each. Figure 1 shows the configuration of the pilot system. The blend of wastewaters used as growth media consists of an industrial wastewater plant influent mixed with an ammonium nitrate (AN) solution and supplemented with the macro and micronutrients presented in BG-11 freshwater growth medium. Each of these wastewater streams was characterized and assessed as previously described in Abraham et al. (2018). The composition of the wastewater blend used in this study was 4 ± 0.5 mg total organic carbon /L, 34 ± 2 mg dissolved inorganic carbon (DIC)/L and 50 ± 5 mg total nitrogen/L. The recipe for the modified BG-11 medium used is described in the Supplementary Table 1. The reactors were inoculated with a freshwater photosynthetic polyculture of about 9 different species of loose and filamentous algae as well as cyanobacteria developed outdoors as described by Abraham et al. (2020). The polyculture consisted of Scenedesmus spp., Chlorella sp. Chlorococcum sp., Coelastrum sp. Dictyosphaerium sp., Ankistrodesmus sp., Ulothrix sp., Stigeoclonium sp. and Oscillatoria sp. In the current study, this consortium was cultured under the growth conditions described in Table 1. Each experiment was done at least in duplicate (n = 2), named experiments 1, 1r, 2, 2r, etc. The first 3 tests and their replicates were conducted under atmospheric CO2: experiments 1 and (1r) consist of a culture using BG-11 growth medium (nitrate-based medium, controls, no wastewater), 2 and (2r) correspond to wastewater blend and addition of a small dose of carbonates as sodium carbonate on day 2 and 3, and experiments 3 and (3r) similar to experiment 2 using metastable carbonates namely nesquehonite (MgCO3-3H2O) produced in our laboratory. Moreover, experiments 4 and 4r were performed by purging pure CO2 for 3 h per day into the system using microbubble diffusers (Pentair, USA) as well as adding carbonates when required to buffer the pH (in the same small doses as used in tests 2 and 3). A flowmeter was used to record the amount of CO2 injected into the system. The amount of C added was 6 g/day/reactor. For all three experiments and their replicates (2, 2r, 3, 3r, 4, and 4r), the addition of carbonates was in small doses, representing a total of 0.5 g C along the 7 days for each experiment. All experiments were performed with 3.2 mM of nitrogen (either as nitrate in controls, or a mixture of nitrate and ammonium, in the case of wastewater). The ratio N/P was the same for all tests (15:1). The recipe for the modified BG-11 medium used is described in the Supplementary Table 1. Continuous monitoring of pH, temperature, and dissolved oxygen (DO) was logged and stored using an APEX-Fusion controller (Neptune systems, CA, USA). Natural evaporation of water from the raceway reactors was made up with filtered water every other day. Biomass was harvested every week and the harvested volume was replaced with an equal volume of wastewater blend and nutrients.
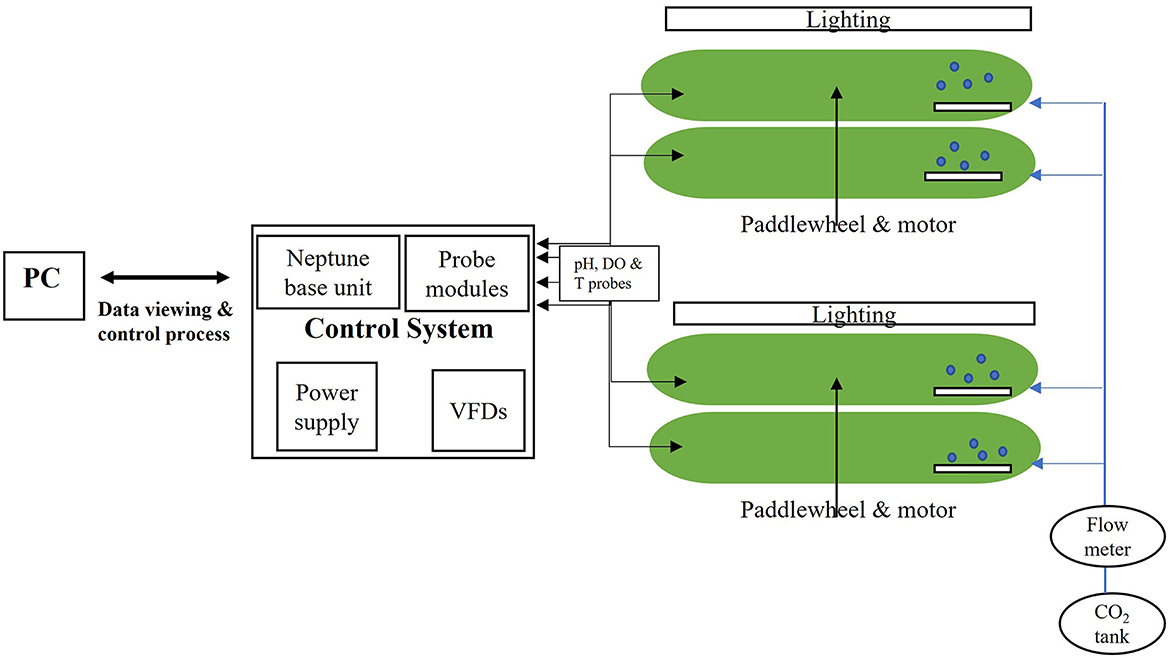
Figure 1. Pilot indoor setup consisting of four 100 L open raceway ponds equipped with T, pH and DO probes and, when needed, CO2 microbubble diffusers for each reactor.
Sample analysis
Dry biomass, expressed as ash free dry weight (AFDW), was determined daily by measuring the total suspended and volatile solids (TSS, and VSS, respectively, in g/L) following the standard method APHA-AWWA-WEF 2540 D (APHA-AWWA-WEF, 2005). Briefly, glass microfiber filter papers (Whatman 1821-055) were pre-ashed for 2 h at 550°C and then stored in a desiccator. Next, the pre-ashed filters were weighed and placed on a filtration apparatus, wetted with DI water and a determined volume of the algae culture was filtered. The filter was then dried for 2 h at 105°C, moved to a desiccator for cooling to room temperature and weighed to obtain the sample's dry weight. The filters were then again placed in the oven at 550°C for 2 h, removed to vacuum desiccator to cool down, and finally weighed to get their AFDW. Micrographs of biomass were captured using a digital microscope (Biotek, VT, USA) with brightfield and fluorescence (chlorophyll filter) modes. Carbon was measured as total carbon (TC) and TOC (total organic carbon) using a Teledyne Tekmar TOC Fusion instrument to assess the dissolved inorganic carbon (DIC) in the ponds. Ammonium, magnesium, sodium, nitrates, nitrites, phosphates, and sulfates were determined by ion chromatography (IC) using an IonPac® CS16 (4 × 250 mm, Dionex) and AS18 (2 × 250 mm, Dionex) columns for cation and anions, respectively. Areal biomass productivity (P) was calculated according to Knoshaug et al. (2018). Briefly, the harvest's yield areal productivity was calculated by considering the amount (mass) of algal biomass harvested from each of the two ponds (AFDW) minus the initial inoculum (which corresponds to the net growth, NG), multiplied by the volume harvested each time (60 L), divided by 0.5 m2 (the surface area of the ponds), and by the timeframe of culture (7 days). The biomass areal productivity for each pond was then averaged to arrive at the productivity number. Algae slurry samples from all reactors were concentrated by gravity settling and centrifugation as described in RoyChowdhury et al. (2019), and the total solid (TS) and volatile solids (VS) of each sample were measured. Elemental analysis of inoculum and biomass obtained was carried out by a CHN elemental analyzer.
Carbon utilization efficiency
For each experiment, the carbon utilization efficiency (CUE) was calculated. CUE is defined as the percentage of fixed carbon in biomass from the total input carbon added to the system. The carbon fixed in the biomass is determined by multiplying the net growth (NG) obtained by the carbon content in biomass (Cbio) as determined by elemental analysis. The total input carbon (TCin) was calculated as the sum of the various carbon forms added to the system in the different experiments such as atmospheric carbon, carbon from minerals and carbon from CO2 gas tank. The carbon dissolved in solution (from the atmosphere and the minerals) was measured by the TOC analyzer while the gaseous carbon injected (Exp#4) was measured by using the flowmeter.
Where AFDW corresponds to ash free dry weight (in g/L) at t time and at initial time or t0 (inoculum).
Carbonate minerals
Sodium carbonate (Na2CO3) was purchased from Fisher Scientific while nesquehonite (MgCO3-3H2O) was obtained in our laboratory as described in Ye et al. (2019). Briefly, in this system a solution of 1.25 M NaOH was purged with pure CO2 for 24 h and up to stabilization, followed by the addition of a solution of MgCl2. Then, the solution was mixed for up to 24 more h, and the precipitates were recovered, washed with ultrapure water, and dried at room temperature. The carbonate minerals obtained were characterized by means of a scanning electron microscope (SEM, Auriga 40, Zeiss) and X-ray diffraction analysis (XRD, Ultima IV, Rigaku). Figure 2 shows the characteristic of the crystals obtained and used in this study.
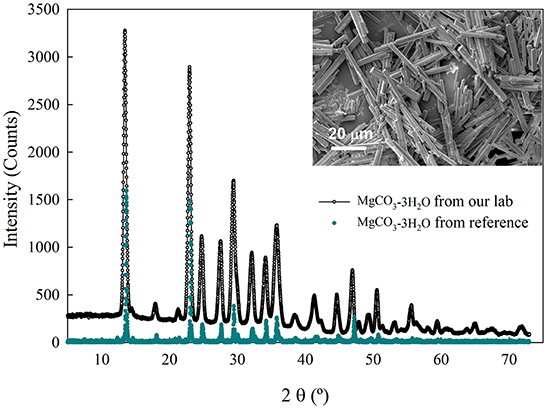
Figure 2. XRD pattern and SEM image in the inset for the carbonation product, MgCO3-3H2O, obtained at our laboratory. The reference for the XRD spectra is the Jade database (Database Administration Guide, 2016).
Extraction and quantitative analysis of algal oil
A detailed description of the oil extraction apparatus can be found in RoyChowdhury et al. (2019) and Abimbola et al. (2021). For this study, a constant amount of ethanol (100 g solvent), 15 g of algal paste at 7.5 wt.% TS, and an extraction time of 3 h were used. Subsequently, recovered lipids were transesterified using the method presented in Section Fatty acid methyl esters (FAMEs).
Fatty acid methyl esters (FAMEs)
FAMEs analysis was performed as described by Abimbola et al. (2021) following two steps. The first one corresponds to the transesterification of the lipids performed at 100°C for 90 min, and the second step corresponds to the identification and quantification of FAME using a gas chromatograph (GC). In the first step, about 35 mg of algal oil was derivatized by adding a mixture of acetyl chloride and acidified methanol (1:20 by volume) to react with the oil exothermically at 100°C for 90 minutes. The reaction was quenched with water and subsequently, the FAMEs were selectively extracted from the derivatized crude oil into the heptane layer that resulted after centrifuging the heptane-derivatized oil mixture at 2,700 rpm for 15 minutes. Afterwards, a sample of the neat heptane layer containing the FAME was injected into the GC.
The FAMEs were quantified using an Agilent GC-FID 122-2361 equipped with a DB-23 column (60 m × 250 × 0.15 μm) and Helium as carrier gas. About 1 μL of neat FAME samples were introduced into the GC using an autosampler in the split-splitless mode, a split ratio of 50 and a split flow of 50 mL/min. The resolution of the peaks separated best at an oven temperature program initially at 50°C for 1 minute, temperature ramp of 25°C/min to 175°C and a hold time of 1 minute, 4°C/minutes ramp to 230°C at a hold time of 5 minutes. The FID detector was set at 280°C while the airflow, Hydrogen flow and makeup flow of Helium were set at 450, 40, and 30 mL/minutes, respectively.
Modeling
In this paper, a model describing the kinetics of algae growth and carbonate dissolution coupled with a geochemical model was implemented. The model describes the evolution of the solution and suspension composition, and it can be used to predict the behavior of the CO2-microalgae-brine system under conditions which are not explored in the lab. The model is an extension of those published in our earlier works (Prigiobbe, 2018; Ye et al., 2019) and describes the concentration evolution of the algae (X, mg/L), and substrates such as carbon (Sc, mg/L), and cations (ci, mg/L),
Where t is time (day), cc,CO2 is the carbon concentration in equivalents of aqueous CO2 calculated using Henry's law
With KH the Henry's constant equal to 1,470 mg/(L·atm) at 25°C, PCO2 the partial pressure of CO2, taken equal to atmospheric, i.e., 3.9 × 10−4 atm; PAC and PMCO2 are the atomic weight and the molecular weight of the carbon and CO2, respectively. A first-order kinetics for CO2 dissolution into the solution was considered, and kCO2 is the dissolution rate constant (1/day). The term cc, HCO3 is the concentration of bicarbonates (HCO) in carbon equivalents, mg/L, released upon carbonate dissolution assuming local equilibrium.
The subscript i in Equation 3 refers to either Mg2+ or Na+ and a is the stochiometric coefficient of dissolution reactions (i) and (ii) which is equal to 1 and 2, respectively.
Moreover, Ks, Kd, μmax, and Y are Monod half-saturation constant (mg/L), endogenous decay coefficient (1/day), maximum specific growth rate of microalgae (1/day), and yield (–), respectively; α and β are model parameters and Si is the supersaturation with respect to the carbonates either MgCO3-3H2O or Na2CO3 respectively,
Where Ksp is the solubility of nesquehonite/sodium carbonates at the experimental temperature, cH is the proton concentration, and cHCO3 is the concentration of the bicarbonates.
Equation 1 through 4 are coupled through the geochemical model and they were discretized with a finite difference scheme forward in time and solved iteratively in MATLAB (MATLAB, 2021). The model parameters were estimated through the inversion of the model on the experimental data using the function lsqnonlin.
Results and discussion
Carbon utilization and biomass production
Ye et al. (2019) demonstrated that metastable carbonates such as nesquehonite (MgCO3.3H2O) and nahcolite (NaHCO3) are a potential alternative carbon source for algal growth in closed photobioreactors at laboratory scale. In addition, the dissolution of these minerals increased the pH drastically and proportional to their concentration. Higher concentrations than solubility levels of nesquehonite triggered again the precipitation of solid phases, limiting the nutrient availability to microorganisms. In the current study, MgCO3.3H2O and sodium carbonate (Na2CO3) crystals were added in lower concentrations (under solubility values) for buffering the microalgal culture media consisting of a wastewater blend with ammonium-N at 100 L open raceway pond reactors.
Preliminary tests performed at laboratory scale (150 mL working volume, Supplementary Figure 1) showed that these crystals can buffer well the medium containing ammonium and maintain growth within optimum physiological pH levels. Figure 3 shows the process parameters along each experiment and replicates tested at 100 L (experiments 1, 1r, 2, 2r, 3, and 3r). Figure 3 (first row) shows the water temperature inside the ponds throughout this study, which ranged between 13.5 and 19°C with an average temperature of 16°C. These temperatures correspond to the laboratory values and the lower range of optimal values for algal growth (Zhao and Su, 2014). In Figure 3 (second and last row), pH and DO trends can also be observed. The pH and DO online measurements are essential parameters to follow the biomass growth and the performance of the system in study. Figure 3 second row shows the pH fluctuations between day and night cycles. In addition to this pattern, for experiment 1, the pH increased over time as algae grew and consumed nitrate in BG-11 medium. In contrast, the pH trend in experiments 2 and 3 decreased due to the consumption of ammonium present in the wastewater blend. These pH variations are related to physiological factors during algae growth and the relative uptake of either nitrate or ammonium by the algae as shown in the following equations:
Both stoichiometric reactions represent the photosynthesis process or biosynthesis in photoautotrophic systems. The formula between brackets corresponds to algae biomass. Nitrate uptake results in a pH rise due to the generation of alkalinity while the uptake of ammonium results in a pH decrease due to the consumption of alkalinity (Ebeling et al., 2006; Eustance et al., 2013; Chisti, 2016). Therefore, in the current study, carbonates were added on days 2 and 3 (indicated by arrows in the respective plots of Figure 3) to maintain the pH and avoid algae crashes due to pH reductions. The same amount of carbon (5 mgC/L) was added from each carbonate source used, and the results showed that both salts successfully increased the pH of the culture and maintained it above neutrality up to day 5. This is of particular importance since the alkalinity consumed by the uptake of ammonium can be reconstituted by adding mineral carbonates and, in consequence, maintain the pH under physiological levels. Both minerals can contribute with substantial buffer capacity. After day 5, Na2CO3 seems to have better pH buffering capacity periods for the culture media. Consequently, if using metastable carbonates, carbon addition should be performed at longer time interval and in accordance with the harvesting frequency.
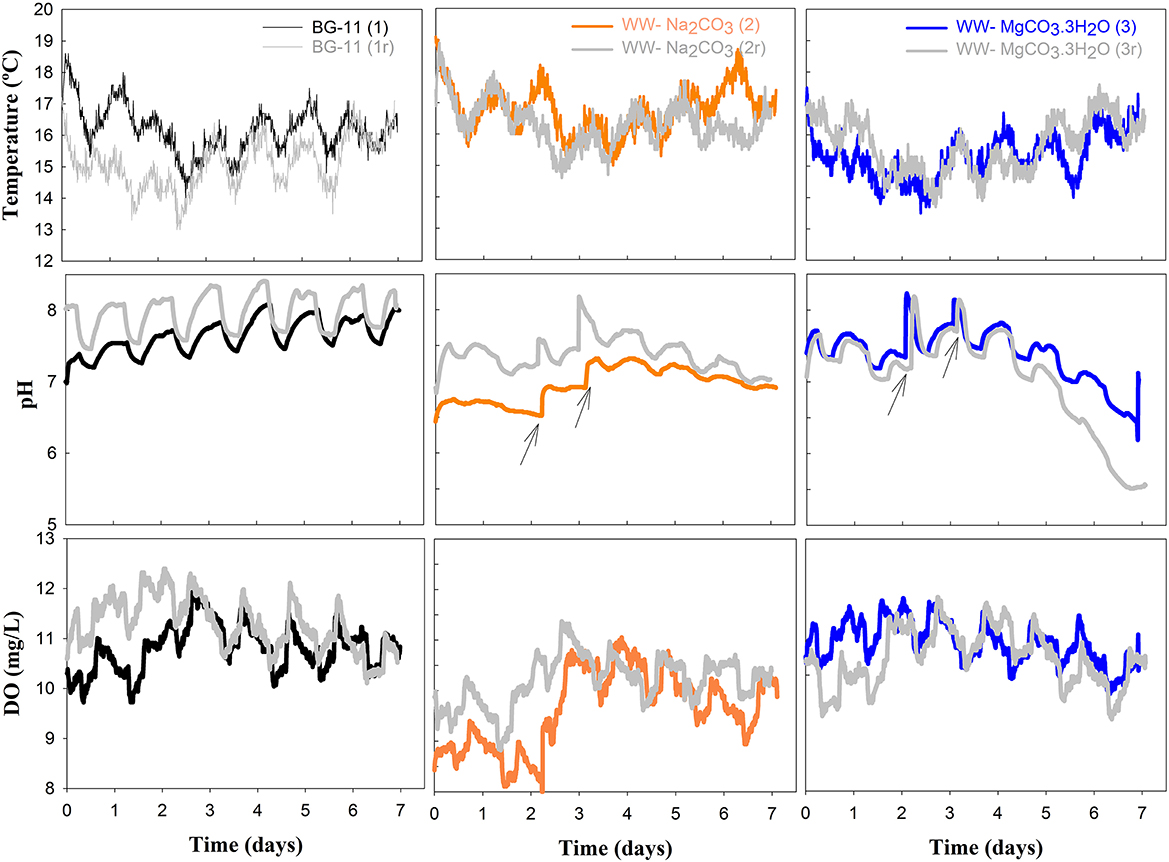
Figure 3. Change over time of temperature, pH and dissolve oxygen (DO), respectively from top to bottom for two replicates of experiments: 1 and (1r) nitrate-based medium (BG-11)—left; 2 and (2r) ammonium-based wastewater with sodium carbonates -middle; and 3 and (3r) ammonium-based wastewater with nesquehonite—right. Arrows indicate the time of mineral addition.
The last row in Figure 3 shows dissolved oxygen (DO) values, one of the products of photosynthesis, along with biomass. Oxygen dissolves into water from both the atmosphere and microalgae. Like the pH, DO values fluctuate between day and night cycles, showing production of oxygen during the day because of photosynthesis, and reduction of oxygen during night due to photorespiration or the release of oxygen from pond water to the atmosphere. These DO values are quite similar for all the duplicates performed. The DO varies from 9 to 12.5 mg/L, and slightly higher values were obtained for the polyculture growing in BG-11 than in the wastewater mixture. In all cases the DO produced indicated photosynthetic activity higher than photorespiration. DO in aqueous solutions at this temperature range is between 8 and 9 mg/L.
Moreover, AFDW measurements were carried out daily for all the experiments to evaluate algae growth. Table 2 summarizes the net growth and the biomass productivity observed for the 3 experiments performed. The net growth (calculated as biomass at day 7 -AFDWt- minus biomass inoculated at initial time or after restarting the culture from harvesting -AFDWt0) for the nitrate-based media (experiments 1 and 1r) is similar to those of the ammonium-based ones (wastewater blend, experiments 2, 2r, 3, and 3r) buffered with small doses of either sodium or magnesium mineral carbonates. Consequently, the biomass productivity obtained is equivalent for all tests as well. These values are in accordance with literature values for algae growth experiments performed using open ponds (Narala et al., 2016; McGowen et al., 2017).

Table 2. Biomass net growth and areal productivity values observed for the different approaches tested.
Overall, it seems that the photosynthetic consortium (Figure 4) can grow well in the wastewater mixture buffered with carbonates without addition of extra N; hence, reducing the logistics and cost of the process. Moreover, the synergy of the species in the consortium could be playing a role on capturing carbon, where the suspended algae of the consortium growing in the bulk liquor (Figures 4C,D) can capture both carbon dioxide and bicarbonates dissolved, and the filamentous algae and cyanobacteria growing on the surface of the pond like a floating mat (Figures 4A,B,E) can capture the gaseous carbon dioxide directly from the atmosphere. In addition, this consortium appears to be resilient to predators and environmental conditions avoiding pond crashes. The cultures maintained a healthy green color along all experimental runs with no brownish or yellowish color development (Figure 4A). Likewise, several studies have shown that using a community of species provides higher biomass stability and better tolerance to changing environmental conditions along the year. For instance, Stockenreiter et al. (2016) studied a multispecies community grown in municipal wastewater obtaining higher growth and nutrient depletion when increasing the number of species in the community. Moreover, the biomass growth values were equivalent to the monoculture ones. Corcoran and Boeing (2012) as well as Lammers et al. (2017) showed that algal polycultures are more beneficial and stable compared to algal monocultures, due to their resistance to predators and environmental conditions.
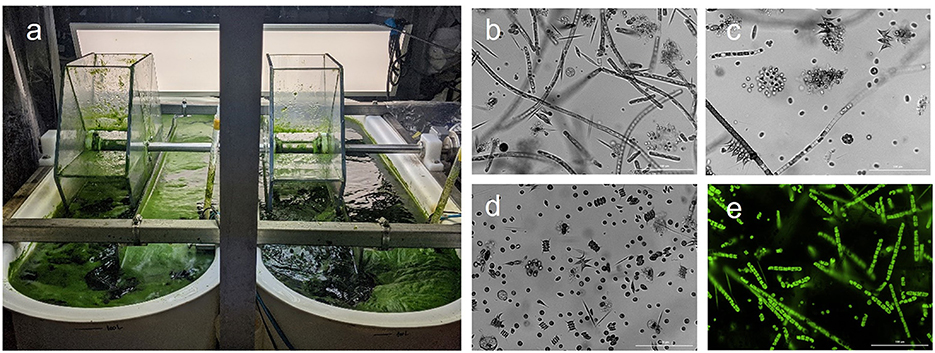
Figure 4. Appearance of the polyculture under (a) naked eye, (b-d) brightfield and (e) fluorescence microscope modes.
After completion of this first set of experiments, another test was performed to evaluate the increase of biomass productivity in carbonate-buffered ammonium systems. For this purpose, carbon dioxide from a pure source (100% CO2) was injected into the solution to increase the carbon concentration in the liquors (experiment 4 and 4r). Increasing the partial pressure of CO2 in the liquor allows better mass transfer, and, in consequence, a higher concentration of CO2 is available for photosynthesis (Singh and Singh, 2014; Zhao and Su, 2014). At the pH of the liquors (7–8) the majority of CO2 is found as bicarbonates. Figure 5 shows the pH and DO for this test. This time, the results are presented as a continuous culture with harvesting time at day 7 and 14 instead of replicates (as in Figure 3). As previously described for the ammonium medium, the pH decreased while ammonium was being consumed due to the consumption of alkalinity. Addition of carbonates (with the same small C dose as used before) elevated the pH and kept it under neutral or even alkaline conditions, even when CO2 was purged into the system. Consequently, the pH of the system was always maintained neutral or higher as controlled by the dissolution of carbonates into water. This pH rise and the addition of carbon favored algal growth as is observed in Figure 5, where by the time the pH is buffered, the DO increased. Table 2 summarizes the growth rate, net growth and productivity values obtained for this experiment. As expected, the biomass growth and productivity are higher than previous experiments due to the addition of carbon.
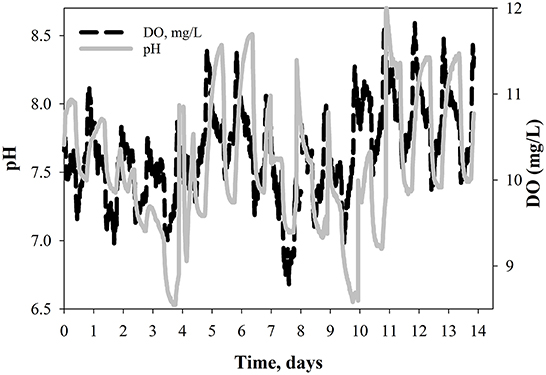
Figure 5. Change over time of pH and dissolve oxygen (DO) for experiment 4 (ammonium-based wastewater + Na2CO3 + CO2p). Solid line represents the harvesting time.
Modeling results
A modified Monod model was used for estimating the growth of microalgae in open ponds. It was assumed abundance of nutrients except for carbon, which was obtained from the atmosphere and via addition of carbonate minerals. The model is reported together with the experimental data of experiments # 1, 2, and 3. Figures 6–8 show the algae and substrate concentrations evolution. Figures 7, 8 also include the evolution of Na+ and Mg2+ concentrations as Na2CO3 and MgCO3-3H2O were supplied.
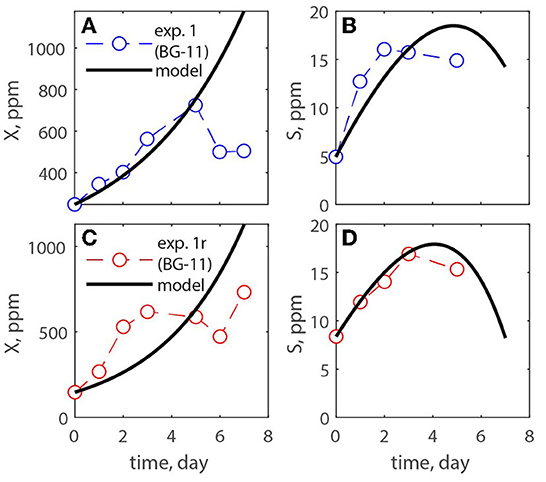
Figure 6. (A–D) Measurements of exp. 1 and exp. 1r where a nitrate-based media was used, and carbon was provided by atmospheric CO2.
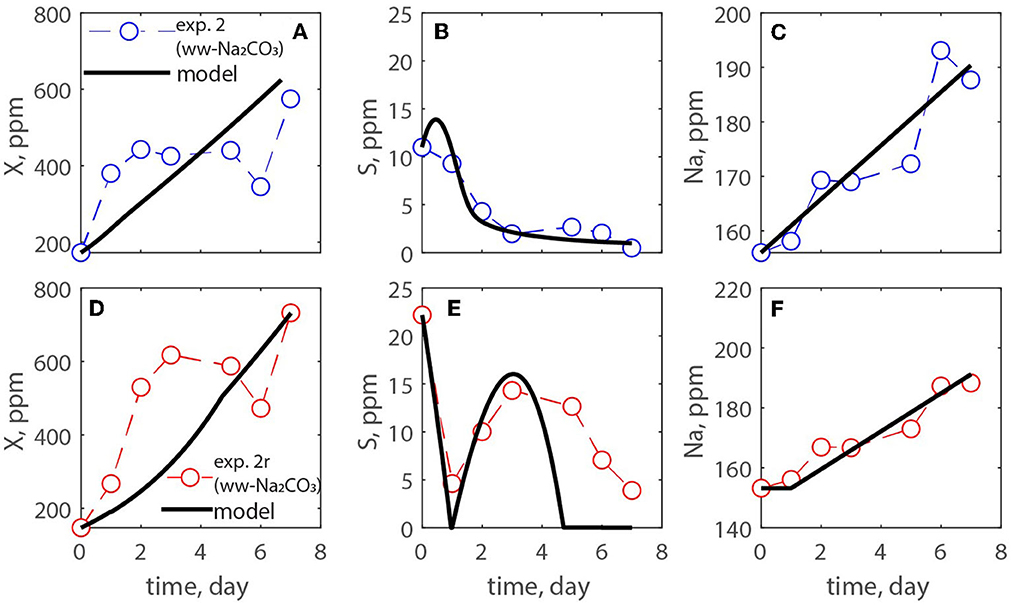
Figure 7. (A–F) Measurements of exp. 2 and exp. 2r where an ammonium-based media was used, and carbon was provided by atmospheric CO2 while Na2CO3 was added on days 2 and 3 of culturing.
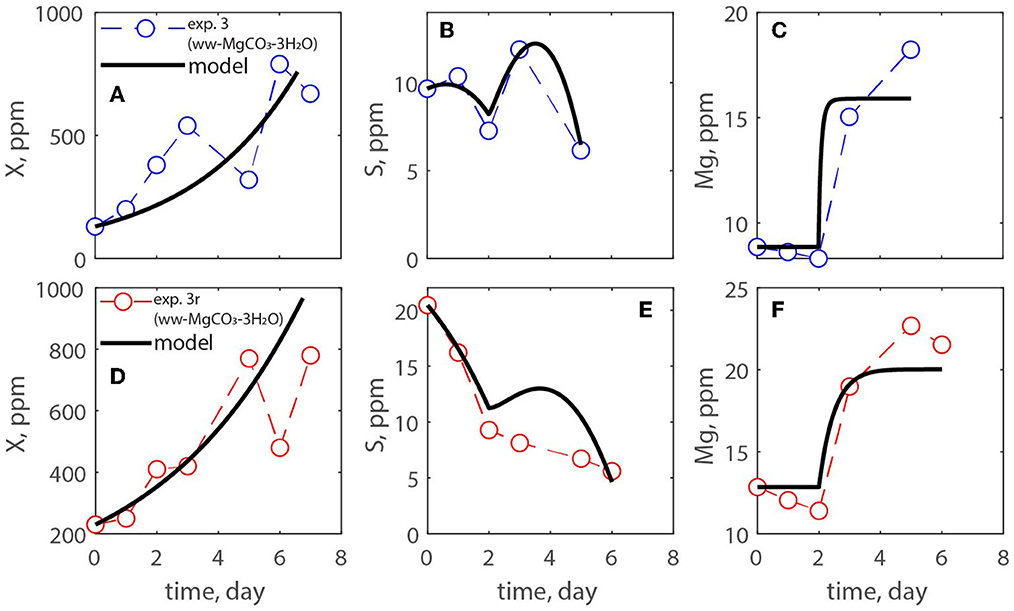
Figure 8. (A–F) Measurements of exp. 3 and exp. 3r where an ammonium-based media was used, and carbon was provided by atmospheric CO2 while MgCO3-3H2O was added on days 2 and 3 of culturing.
Table 3 reported the estimated values of the model parameters. The value of the decay rate was not estimated as the decay process was not observed over the span of the culturing time, and it was out of the scope of this study. The values in the table show similar maximum specific growth rate of microalgae (μmax) for all three experiments. Moreover, the rate of CO2 dissolution (kCO2) decreases from exp. 1 to 2, and 3; this could be due to the concentration of carbon in solution and/or the pH of the medium that reduces the driving force of CO2 dissolution into the solution. It is important to point out that in this paper the yield coefficient (Y) is expressed as g biomass/g C. To compare its values with the literature the inverse of Y should be calculated. The values of 1/Y for exp. 1, 2, 3, and its replicates are, respectively, 0.043, 0.5, 0.089 g C/g biomass, which agree with the recent work by Zurano et al. (2021), where algae growth kinetic parameters were estimated. The parameters α and β increase from exp. 2 to exp. 3 showing that the dissolution kinetics of the produced MgCO3-3H2O is faster than that of the commercial Na2CO3.
Overall, the model describes well the measurements in particular considering the complexity of the investigated dynamic system, and thus it has a potential to be used in optimizing growth kinetics in open pond reactors when using mineral carbonates as a C source. However, a dataset with higher temporal resolution will allow us to perform a further refinement of the model to ensure statistical significance as well as explore system dynamics and the response of the systems to changes.
Biomass carbon and potential products
Table 4 summarizes the elemental carbon composition found for each treatment. As evident from the data, the carbon contents in the biomass obtained using different treatment approaches are close. In other words, the carbon assimilation was similar for the cultures grown under nitrate-based media (1), ammonia-based media buffered with carbonates (2, 3), and with carbonates and CO2 microbubble diffusion (4). The effect of the different treatment approaches tested on the oil content of harvested algae is also similar to the observations made on the carbon content. From the third column of Table 4, it can be observed that the oil contents were experimentally close, considering the error deviations of each experiment. This indicates that the approach of adding carbonate to buffer the algal ammonium-based culture is satisfactory, while adding gaseous CO2 increases the biomass production without significant impact on the oil content. Accordingly, the oil productivity was also higher for experiment #4 (Table 4). These oil content values are in accordance with literature (Yoo et al., 2010), and can be enhanced by modifying culture conditions in the ponds i.e., by adding more carbon to the system while reducing the N content as described by Lohman et al. (2015), Najafabadi et al. (2015) and Pancha et al. (2015).
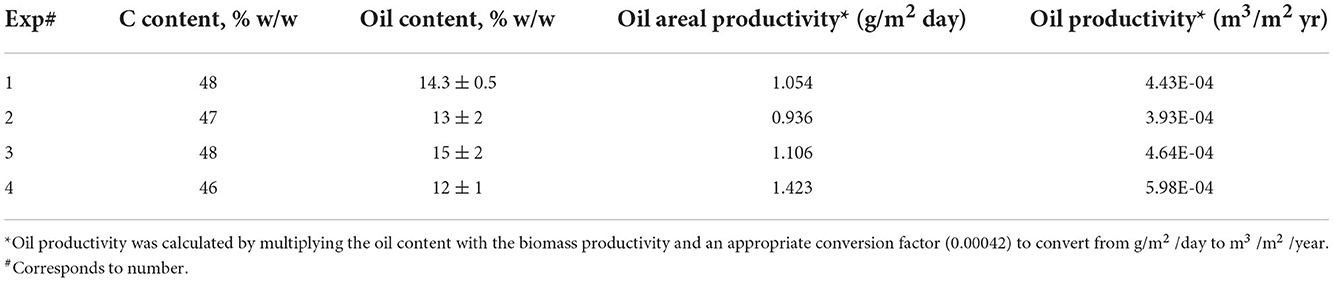
Table 4. Elemental carbon, oil content and productivity observed for the different approaches tested.
Figure 9 shows the fatty acid methyl esters (FAME) analysis performed for experiment #1 (nitrate-based medium) and experiment #3 (ammonia-based medium buffered with MgCO3-3H2O). This analysis provides data on FAMEs composition of the extracted oil from algae. FAME analysis was performed to evaluate the biodiesel potential of the extracted oil. In addition, FAMEs are known to comprise a wide range of bio-functional materials such as antimicrobials, dietary supplements, and nutraceuticals amongst others (Lu et al., 2015; Stokes et al., 2020). Overall, the results show that most of the FAMEs are saturated fatty acids and it revealed slightly different profiles depending on the source of carbon used. This opens the possibility to design and tune the carbon source to make algae synthesize any of the desired bio-product.
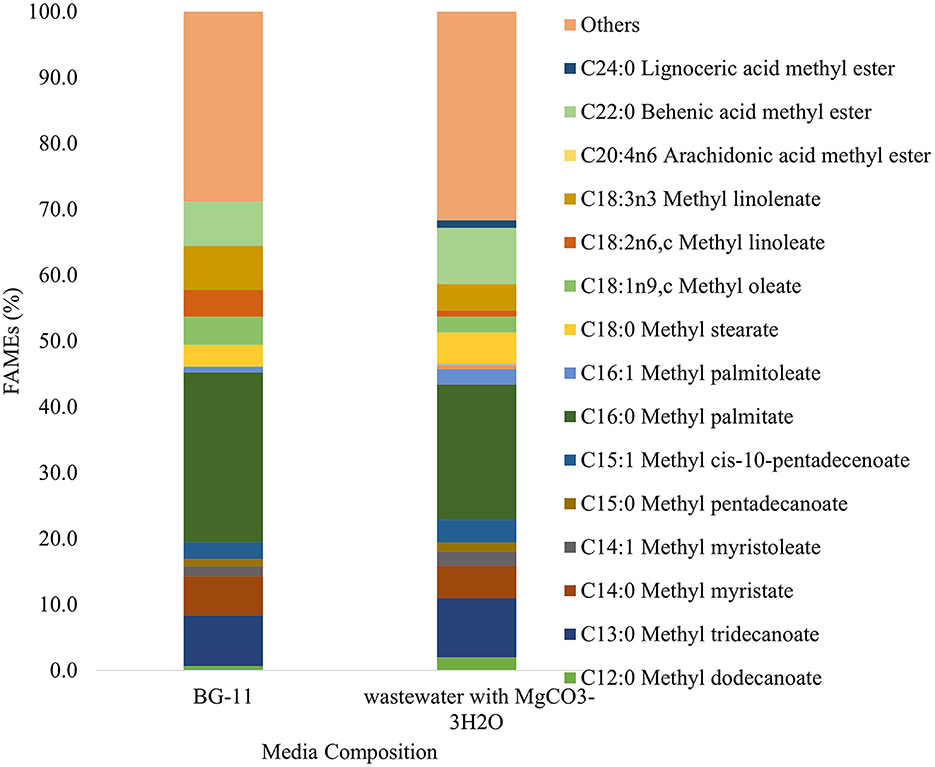
Figure 9. Fatty acid methyl esters (FAMEs) composition in biomass grown under atmospheric conditions in nitrate-based medium -BG-11(exp 1) and ammonium-based wastewater buffered with MgCO3-3H2O (exp 3).
From Figure 9, the FAMEs in the range of C16–C18 encompass up to 40–45% of the FAME content. According to Song et al. (2014) and Deshmukh et al. (2019), the FAMEs existing within this range are the most suitable feedstock for biodiesel production. Moreover, considering the total amount of FAMEs derived, both samples have about 50 and 10% of saturated fatty acids (SFAs) and monounsaturated fatty acids (MUFAs), respectively. Contrary to sample #1, sample #3 has less polyunsaturated fatty acids (PUFAs). According to Francisco et al. (2010) and Manzoor et al. (2022), the degree of unsaturation affects the oxidative stability of biodiesel. In other words, SFAs and MUFAs improve the stability of biodiesel. Thus, because of less PUFA content in the sample from experiment #3, it seems biomass generated from the ammonium-based medium buffered with MgCO3-3H2O can generate FAMEs with higher quality for biodiesel.
The most abundant FAME in both samples was C16:0 (Methyl palmitate). This compound has also been found in the oil of the microalgae S. intermedius by Davoodbasha et al. (2018), which showed strong antimicrobial and antioxidant activities.
From the FAMEs reported, about 25–30% of the FAMEs were not identified, and therefore expressed as “Others” in Figure 9. Further analysis should be performed for a complete interpretation of the results. The FAMEs are products of transesterification reactions on the extracted oil. Thus, based on the amount of extracted oil converted to FAMEs in samples #1 and #3, only 20, and 15% of the reported oil content was transformed to FAMEs, respectively. However, several studies on catalytic hydroprocessing of crude algal oils demonstrated the upgrade of the microalgae-extracted oils (Yang et al., 2016). For example, studies performed by Zhou and Lawal (2016), demonstrated that extracted oil can be transformed to green diesel with about 76.5% conversion via hydrodeoxygenation utilizing 1% Pt catalyst. Hence, although the yield of the FAMEs is low, adopting the hydrodeoxygenation process after oil extraction can be a promising method to derive biofuel from the harvested algae in this work.
Carbon utilization efficiency
The carbon utilization efficiency (CUE) calculated for the first 3 experiments was comparable (21, 16, and 19% for experiment #1, 2, and 3, respectively). In these cases, the CUE is low due to the low algal biomass growth obtained and the low carbon content in solution. The low CO2 concentrations in the atmosphere compared to other gases (e.g., 0.04% CO2, 21% O2 and 75% N2), along with the low solubility of CO2 in the liquor (as described by Henry's law) are the result for low photosynthetic activity. Furthermore, the enzyme Ribulose-1,5-bisphosphate carboxylase-oxygenase (RuBisCo) is the one responsibleof the photosynthetic activity, fixing CO2 into a molecule of organic carbon (e.g., glucose) via the Calvin-Benson cycle, and its low specificity is regulated by the ratio of CO2: O2 concentrations (Zheng et al., 2018).
In the experiments performed under atmospheric carbon dioxide concentrations, the amount of carbon in solution (determined by TC-TOC) was derived from the amount of CO2 dissolved in solution along with the pH of the medium, according to its composition (e.g., nitrates or ammonium), which generates or consumes alkalinity, respectively. The fact that the 3 experiments reached similar CUE and biomass amount indicates a successful metabolic-based pH control by the addition of carbonates in the ammonium-based media. Under atmospheric conditions, nitrate-based media exhibited an increase in pH due to growth and this reflects on the DIC in solution, whereas growth in ammonium-based media decrease the pH, which was adjusted by the addition of carbonates (Figures 3, 6B,D, 7B,E, 8B,E).
Moreover, CUE was increased by 4-fold (96%) when injecting microbubbles of CO2 while buffering the pH with carbonates. Nevertheless, a more cost-effective addition of carbon should be considered over injecting gaseous CO2. Consequently, the next challenge to investigate will be to assess the use of only carbonate minerals (always under their solubility levels) to buffer the solution and increase the carbon availability for algae growth, eliminating the addition of gaseous CO2.
Conclusions
Overall, carbonate species are a promising alternative to combine, capture, and reuse of CO2 for microalgae growth. Both carbon capture processes, chemical (via mineral carbonation) and biological fixation (via photosynthesis), can be combined successfully, and they seem to be a suitable strategy to use waste materials to produce algal biomass that can be used to displace petroleum-based products. The addition of carbonates allows the use of ammonia-rich wastewater streams, one of the most common ions in wastewater and the most favorable nutrient for fast growth of algae due to its lower requirements of energy. Severe pH fluctuations can be successfully buffered using metastable carbonates generated from industrial flue gases or waste CO2 emissions from a stationary fermentation point. Moreover, the use of carbonates instead of a gaseous form of carbon has the potential to increase the carbon utilization efficiency by avoiding losses to the atmosphere. This combined strategy could be useful to decouple geographically and temporally gaseous waste CO2 sources from industrial/municipal plants and algae production farms. Moreover, this approach would promote the reduction of the carbon footprint. Further investigation should be performed to increase biomass productivity by sole use of carbonates as C sources.
Data availability statement
The original contributions presented in the study are included in the article/Supplementary material, further inquiries can be directed to the corresponding author.
Author contributions
JA: conceptualization, investigation-data collection, visualization, formal analysis, writing-original draft, and review and editing. VP: production of carbonates used in this study, methodology-modeling, formal analysis-modeling, and writing-review and editing. TA: investigation-data collection—FAME, formal analysis-oil and FAME, and writing-original draft. CC: funding acquisition, supervision, and writing—review and editing. All authors contributed to the article and approved the submitted version.
Funding
This work was supported by LEIDOS contract 2009-372/P010205636.
Conflict of interest
The authors declare that the research was conducted in the absence of any commercial or financial relationships that could be construed as a potential conflict of interest.
Publisher's note
All claims expressed in this article are solely those of the authors and do not necessarily represent those of their affiliated organizations, or those of the publisher, the editors and the reviewers. Any product that may be evaluated in this article, or claim that may be made by its manufacturer, is not guaranteed or endorsed by the publisher.
Supplementary material
The Supplementary Material for this article can be found online at: https://www.frontiersin.org/articles/10.3389/fclim.2022.949411/full#supplementary-material
References
Abimbola, T., Christodoulatos, C., and Lawal, A. (2021). Performance and optimization studies of oil extraction from Nannochloropsis spp. and Scenedesmus obliquus. J. Clean. Prod. 311. doi: 10.1016/j.jclepro.2021.127295
Abraham, J., Abimbola, T., RoyChowdhury, A., Su, T., Christodoulatos, C., Koutsospyros, A., et al. (2020). “On-site freshwater culture using untreated industrial energetic-laden wastewater at pilot scale,” in XV International Conference on Protection and Restoration of the Environment, eds P. C. Yannopoulos et al. (Patras), 521–530.
Abraham, J., Lin, Y., RoyChowdhury, A., Christodoulatos, C., Arienti, P., Smolinski, B., et al. (2018). Algae toxicological assessment and valorization of energetic-laden wastewater streams using Scenedesmus obliquus. J. Clean. Prod. 202, 838–845. doi: 10.1016/j.jclepro.2018.08.148
APHA-AWWA-WEF (2005). Standard methods for the examination of water and wastewater,”. in Total Solids Suspended, Method, 21th edn (New York, NW: APHA-AWWA-WEF). 2540 D, 2-55 a 2-59.
Benemann, J. (2013). Microalgae for biofuels and animal feeds. Energies 6, 5869–5886. doi: 10.3390/en6115869
Chisti, Y. (2016). “Large-scale production of algal biomass: Raceway ponds,” in Algae Biotechnology. Green Energy and Technology, eds F. Bux and Y. Chisti (Cham: Springer). doi: 10.1007/978-3-319-12334-9_2
Clarke, L., and Jiang, K. (2014). “Assessing transformation pathways climate change 2014: mitigation of climate change,” in IPCC14: Contribution of Working Group III to the Fifth Assessment Report of the Intergovernmental Panel on Climate Change (Cambridge: Cambridge University Press).
Corcoran, A. A., and Boeing, W. J. (2012). Biodiversity increases the productivity and stability of phytoplankton communities. PLoS ONE 7, e49397. doi: 10.1371/journal.pone.0049397
Daneshvar, E., Wicker, R. J., Show, P. L., and Bhatnagar, A. (2022). Biologically-mediated carbon capture and utilization by microalgae towards sustainable CO2 biofixation and biomass valorization —a review. Chem. Eng. J. 427, 130884. doi: 10.1016/j.cej.2021.130884
Davoodbasha, M., Edachery, B., Nooruddin, T., Lee, S. Y., and Kim, J. W. (2018). An evidence of C16 fatty acid methyl esters extracted from microalga for effective antimicrobial and antioxidant property. Microb. Pathog. 115, 233–238doi: 10.1016/j.micpath.2017.12.049
de Godos, I., Mendoza, J. L., Acién, F. G., Molina, E., Banks, C. J., Heaven, S., et al. (2014). Evaluation of carbon dioxide mass transfer in raceway reactors for microalgae culture using flue gases. Bioresourc. Technol. 153, 307–314. doi: 10.1016/j.biortech.2013.11.087
Deshmukh, S., Kumar, R., and Bala, K. (2019). Microalgae biodiesel: a review on oil extraction, fatty acid composition, properties and effect on engine performance and emissions. Fuel Process. Technol. 191, 232–247. doi: 10.1016/j.fuproc.2019.03.013
Dolganyuk, V., Belova, D., Babich, O., Prosekov, A., Ivanova, S., Katserov, D., et al. (2020). Microalgae: a promising source of valuable bioproducts. Biomolecules 10, 1153. doi: 10.3390/biom10081153
Durán, I., Rubiera, F., and Pevida, C. (2018). Microalgae: potential precursors of CO2 adsorbents. J. CO2 Util. 26, 454–464. doi: 10.1016/j.jcou.2018.06.001
Ebeling, J. M., Timmons, M. B., and Bisogni, J. J. (2006). Engineering analysis of the stoichiometry of photoautotrophic, autotrophic, and heterotrophic removal of ammonia–nitrogen in aquaculture systems. Aquac. 257, 346–358. doi: 10.1016/j.aquaculture.2006.03.019
Eustance, E., Gardner, R. D, Moll, K. M., Menicucci, J., Gerlach, R., and Peyton, B. M. (2013). Growth, nitrogen utilization and biodiesel potential for two chlorophytes grown on ammonium, nitrate or urea. J. Appl. Phycol. 25, 1663–1677. doi: 10.1007/s10811-013-0008-5
Eustance, E., Lai, Y. S., Shesh, T., and Rittmann, B. E. (2020). Improved CO2 utilization efficiency using membrane carbonation in outdoor raceways. Algal Res. 51, 102070. doi: 10.1016/j.algal.2020.102070
Francisco, É. C., Neves, D. B., Jacob-Lopes, E., and Franco, T. T. (2010). Microalgae as feedstock for biodiesel production: Carbon dioxide sequestration, lipid production and biofuel quality. J. Chem. Technol. Biotechnol. 85, 395–403. doi: 10.1002/jctb.2338
Glasser, F. P., Jauffret, G., Morrison, J., Galvez-Martos, J.-L., Patterson, N., and Imbabi, M. S.-E. (2016). Sequestering CO2 by mineralization into useful nesquehonite-based products. Front. Energy Res. 4, 3. doi: 10.3389/fenrg.2016.00003
Intergovernmental Panel on Climate Change, IPCC. (2022). “Climate change 2022: impacts, adaptation, and vulnerability,” in Contribution of Working Group II to the Sixth Assessment Report of the Intergovernmental Panel on Climate Change, eds H. -O. Pörtner, D. C. Roberts, M. Tignor, E. S. Poloczanska, K. Mintenbeck, A. Alegría, et al. (Cambridge,NY: Cambridge University Press).
IPCC (2005). “IPCC special report on carbon dioxide capture and storage,” in Prepared by Working Group III of the Intergovernmental Panel on Climate Change, eds B. Metz, O. Davidson, H. C. de Coninck, M. Loos, and L. A. Meyer (Cambridge, NY: Cambridge University Press). 442 pp.
Khan, M. I., Shin, J. H., and Kim, J. D. (2018). The promising future of microalgae: current status, challenges, and optimization of a sustainable and renewable industry for biofuels, feed, and other products. Microb. Cell. Fact. 17, 36. doi: 10.1186/s12934-018-0879-x
Knoshaug, E., Laurens, L., Kinchin, C., and Davi, R. (2018). “Use of cultivation data from the algae testbed public private partnership as utilized in NREL's Algae State of technology assessments,” in Technical Report(National Renewable Energy Laboratory. Golden, Colorado). NREL/TP-5100–67289.
Lai, Y. S., Eustance, E., Shesh, T., and Rittmann, B. E. (2020). Enhanced carbon-transfer and—utilization efficiencies achieved using membrane carbonation with gas sources having a range of CO2 concentrations. Algal Res. 52, 102098. doi: 10.1016/j.algal.2020.102098
Lammers, P. J., Huesemann, M., Boeing, W., Anderson, D. B., Arnold, R. G., Bai, X., et al. (2017). Review of the cultivation program within the National alliance for advanced biofuels and bioproducts. Algal Res. 22, 166–186. doi: 10.1016/j.algal.2016.11.021
Lohman, E. J., Gardner, R. D., Pedersen, T., Peyton, B. P., Cooksey, K. E., and Gerlach, R. (2015). Optimized inorganic carbon regime for enhanced growth and lipid accumulation in Chlorella vulgaris. Biotechnol. Biofuels. 8, 82. doi: 10.1186/s13068-015-0265-4
Lu, Y., Levine, R. B., and Savage, P. E. (2015). Fatty acids for nutraceuticals and biofuels from hydrothermal carbonization of microalgae. Ind. Eng. Chem. Res. 54, 4066–4071. doi: 10.1021/ie503448u
Manzoor, M., Hussain, A., Ahmad, Q. -A, Chaudhary, A., Schenk, P. M., et al. (2022). Biodiesel quality assessment of microalgae cultivated mixotrophically on sugarcane bagasse. Sustain. Energy Technol. Assess. 53, 102359. doi: 10.1016/j.seta.2022.102359
McGowen, J., Knoshaug, E. P., Lauren, L. M. L., Dempster, T. A., Pienkos, P. T., Wolfrum, E., et al. (2017). The algae testbed public-private partnership (ATP3) framework; establishment of a national network of testbed sites to support sustainable algae production. Algal Res. 25,168–177. doi: 10.1016/j.algal.2017.05.017
Mishra, S., Roy, M., and Mohanty, K. (2019). Microalgal bioenergy production under zero-waste biorefinery approach: recent advances and future perspectives. Bioresour. Technol. 292, 122008. doi: 10.1016/j.biortech.2019.122008
Najafabadi, H. A., Malekzadeh, M., Farhad Jalilian, F., Vossoughi, M., and Pazuki, G. (2015). Effect of various carbon sources on biomass and lipid production of Chlorella vulgaris during nutrient sufficient and nitrogen starvation conditions. Bioresour. Technol. 180, 311–317. doi: 10.1016/j.biortech.2014.12.076
Narala, R. R., Garg, S., Sharma, K. K., Thomas-Hall, S. R., Deme, M., Li, Y., et al. (2016). Comparison of microalgae cultivation in photobioreactor, open raceway pond, and a two-stage hybrid system. Front. Energy Res. 4:29. doi: 10.3389/fenrg.2016.00029
National Oceanic Atmospheric Administration (NOAA). (2021). Global Monitoring Laboratory, Mauna Loa, USA. Availble online at: https://gml.noaa.gov/ccgg/trends/monthly.html
Pancha, I., Chokshi, K., Ghosh, T., Paliwal, C., Maurya, R, and Mishra, S. (2015). Bicarbonate supplementation enhanced biofuel production potential as well as nutritional stress mitigation in the microalgae Scenedesmus sp. CCNM 1077. Bioresour. Technol. 193, 315–323. doi: 10.1016/j.biortech.2015.06.107
Prigiobbe, V. (2018). Estimation of nucleation and growth parameters from in situ Raman spectroscopy in carbonate systems. J. Environ. Chem. Eng. 6, 930–936. doi: 10.1016/j.jece.2017.12.024
Razzak, S. A., Hossain, M. M., Lucky, R. A., Bassi, A. S., and de Lasa, H. (2013). Integrated CO2 capture, wastewater treatment and biofuel production by microalgae culturing—a review. Renew. Sust. Energ. Rev. 27, 622–653. doi: 10.1016/j.rser.2013.05.063
RoyChowdhury, A., Abraham, J., Abimbola, T, Lin, Y., Christodoulatos, C., Lawal, A., et al. (2019). Assessing oil content of microalgae grown in industrial energetic-laden wastewater. Environ. Process
Salbitani, G., and Carfagna, S. (2021). Ammonium utilization in microalgae: a sustainable method for wastewater treatment. Sustainability 13, 956. doi: 10.3390/su13020956
Sayre, R. (2010). Microalgae: the potential for carbon capture. BioScience 60, 722–727. doi: 10.1525/bio.2010.60.9.9
Singh, R. P., Yadav, P., Kumar, A., Hashem, A., Al-Arjani, A.-B. F., AbdAllah, E. F., et al. (2022). Physiological and biochemical responses of bicarbonate supplementation on biomass and lipid content of green algae Scenedesmus sp. BHU1 isolated from wastewater for renewable biofuel feedstock. Front. Microbiol. 13, 839800. doi: 10.3389/fmicb.2022.839800
Singh, S. P., and Singh, P. (2014). Effect of CO2 concentration on algal growth: a review. Renew. Sust. Energ. Rev. 38, 172–179. doi: 10.1016/j.rser.2014.05.043
Somers, M. D., and Quinn, J. C. (2019). Sustainability of carbon delivery to an algal biorefinery: a techno-economic and life-cycle assessment. J. CO2 Util. 30,193–204. doi: 10.1016/j.jcou.2019.01.007
Song, M., Pei, H., Hu, W., Zhang, S., Ma, G., Han, L., et al. (2014). Identification and characterization of a freshwater microalga Scenedesmus SDEC-8 for nutrient removal and biodiesel production. Bioresour. Technol. 162, 129–135. doi: 10.1016/j.biortech.2014.03.135
Stockenreiter, M., Haupt, F., Seppäl,ä, J., Tamminen, T., and Spilling, K. (2016). Nutrient uptake and lipid yield in diverse microalgal communities grown in wastewater. Algal Res. 15, 77–82. doi: 10.1016/j.algal.2016.02.013
Stokes, J., Tu, R., Peters, M., Yadav, G., Fabiano, L. A., and Seider, W. D. (2020). Omega-3 fatty acids from algae produced biodiesel. Algal Res. 51, 102047. doi: 10.1016/j.algal.2020.102047
Ummalyma, S. B., Sahoo, D., and Pandey, A. (2021). Resource recovery through bioremediation of wastewaters and waste carbon by microalgae: a circular bioeconomy approach. Environ. Sci. Pollut. Res. 28, 58837–58856. doi: 10.1007/s11356-020-11645-8
Wang, Y., Stessman, D. J., and Spalding, M. H. (2015). The CO2 concentrating mechanism and photosynthetic carbon assimilation in limiting CO2: how Chlamydomonas works against the gradient. Plant J. 82, 429–448. doi: 10.1111/tpj.12829
Woodall, C. M., McQueen, N., Pilorg,é, H., and Wilcox, J. (2019). Utilization of mineral carbonation products: current state and potential. Greenhouse Gas Sci. Technol. 9:1096–1113. doi: 10.1002/ghg.1940
Yang, C., Li, R., Cui, C., Liu, S., Qiu, Q., Ding, Y., et al. (2016). Catalytic hydroprocessing of microalgae-derived biofuels: a review. Green Chem. 18, 3684–3699. doi: 10.1039/C6GC01239F
Ye, Z., Abraham, J., Christodoulatos, C., and Prigiobbe, V. (2019). Mineral carbonation for carbon utilization in microalgae culture. Energy Fuels, 33, 8843. doi: 10.1021/acs.energyfuels.9b01232
Yoo, C., Jun, C.-,w., Lee, J.-Y., Ahn, C.-Y., and Oh, H.-M. (2010). Selection of microalgae for lipid production under high levels carbon dioxide. Bioresour. Technol. 101, S71–S74. doi: 10.1016/j.biortech.2009.03.030
Zhao, B., and Su, Y. (2014). Process effect of microalgal-carbon dioxide fixation and biomass production: a review. Renew. Sust. Energ. Rev. 31, 121–132. doi: 10.1016/j.rser.2013.11.054
Zheng, Q., Xu, X., Martin, G. J. O., and Kentish, S. E. (2018). Critical review of strategies for CO2 delivery to large-scale microalgae cultures. Chin. J. Chem. Eng. 26, 2219–2228. doi: 10.1016/j.cjche.2018.07.013
Zhou, L., and Lawal, A. (2016). Hydrodeoxygenation of microalgae oil to green diesel over Pt, Rh and presulfided NiMo catalysts. Catal. Sci. Technol. 6, 1442. doi: 10.1039/C5CY01307K
Zurano, A. S., Serrano, C. G., Acién-Fernández, F. G., Fernández-Sevilla, J. M., and Molina-Grima, E. (2021). Modelling of photosynthesis, respiration, and nutrient yield coefficients in Scenedemus almeriensis culture as a function of nitrogen and phosphorus. Appl. Microbiol. Biotechnol. 105, 7487–7503. doi: 10.1007/s00253-021-11484-8
Keywords: freshwater microalgae cultivation, industrial wastewater valorization, CO2 mineralization, metastable carbonates, nesquehonite, algal oil, renewable energy, bioproducts
Citation: Abraham J, Prigiobbe V, Abimbola T and Christodoulatos C (2023) Integrating biological and chemical CO2 sequestration using green microalgae for bioproducts generation. Front. Clim. 4:949411. doi: 10.3389/fclim.2022.949411
Received: 20 May 2022; Accepted: 16 November 2022;
Published: 04 January 2023.
Edited by:
S. Venkata Mohan, Indian Institute of Chemical Technology (CSIR), IndiaReviewed by:
Annie Modestra J., Luleå University of Technology, SwedenKit Wayne Chew, Xiamen University, Malaysia
Imran Pancha, SRM University, India
Copyright © 2023 Abraham, Prigiobbe, Abimbola and Christodoulatos. This is an open-access article distributed under the terms of the Creative Commons Attribution License (CC BY). The use, distribution or reproduction in other forums is permitted, provided the original author(s) and the copyright owner(s) are credited and that the original publication in this journal is cited, in accordance with accepted academic practice. No use, distribution or reproduction is permitted which does not comply with these terms.
*Correspondence: Juliana Abraham, jabraham@stevens.edu