- 1California Department of Fish and Wildlife, Bodega Marine Laboratory, Bodega Bay, CA, United States
- 2Bodega Marine Laboratory, Coastal Marine Science Institute, University of California, Davis, Bodega Bay, CA, United States
- 3One Health Institute, University of California, Davis, Davis, CA, United States
- 4Washington State Department of Natural Resources, Olympia, WA, United States
The recent large-scale intensification of marine heatwaves, and other climate-related stressors, has dramatically impacted biogenic habitats around the globe, including marine ecosystems such as coral reefs, seagrasses, and kelp forests. While the impacts to foundation species may be of particular concern, these ecological catastrophes underscore the need to examine how whole systems respond to a suite of stressors. The recent climate-driven collapse of the bull kelp forest and recreational red abalone fishery in northern California provides an example of unanticipated ripple and lagged effects in the system, intensifying vulnerabilities and accelerating population and fishery collapse. For this case study, we examined 15 years (2003–2018) of biological survey data on the bull kelp forest ecosystem—before, during, and after an extreme climate event. We document the interactions and complexity of impacts over time, as well as the resulting increased vulnerability of red abalone to additional anthropogenic, biological, and environmental stressors. We observed progressively stronger population-level responses of the red abalone to the marine heatwave and the regional loss of kelp, driving the movement of adults and juveniles in search of food. As food remained scarce, we documented the loss of productivity with diminished gonad and body condition, the absence of larval or newly-settled abalone, mass mortalities, and shoreward shifts in depth distributions. With 40% of the population dead or dying, juvenile and trophy-sized abalone abandoning cryptic habitats, the shift in the distribution to shallower depths increased the vulnerability of red abalone to the fishery. Other anthropogenic, biological, and climate-related stressors that disproportionately impact shallow habitats are now a growing concern for the survivors. For red abalone, previously unanticipated cascading risks include increased wave energy, warming air temperatures, freshwater flooding, landslides, as well as possible oil spills and harmful algal blooms. Climate-driven changes in vulnerability to fishing and environmental stressors present significant challenges for sustainable natural resource management in dynamic stressed systems, and underscore the need for continued system-focused monitoring. We present a conceptual framework supporting similar ecosystem investigations of recent and future climate impacts to inform adaptive ecosystem-based management strategies.
Introduction
Climate change is dramatically shifting terrestrial and aquatic ecosystems (Scheffer et al., 2001), and these shifts are intensifying and creating novel stressors for populations. Many biological ecosystems are changing rapidly in dynamic, non-linear ways in response to stochastic environmental fluctuations with devastating consequences (Hsieh et al., 2005). Major perturbations of routine stochastic fluctuations are leading to trophic cascades, regime shifts/transitions (Biggs et al., 2015), ecological transformations (Schuurman et al., 2021), or even ecosystem collapse (Newbold et al., 2020), which can occur without warning (Hastings and Wysham, 2010), and may be difficult or infeasible to reverse (May, 1977; Scheffer et al., 2001). Examples of climate change driving major ecosystem disruptions or collapse have been documented in terrestrial forests (Ellison et al., 2005; Jönsson and Bärring, 2011; Mitton and Ferrenberg, 2012) as well as marine systems (Mumby et al., 2007), including nearshore kelp forests (Ling et al., 2015; Rogers-Bennett and Catton, 2019), and pelagic ecosystems (Kirby et al., 2009). Geographic shifts in the distribution of fisheries have also been documented (Perry et al., 2005). In warm years, the walleye Pollock and Pacific cod moved north, biasing stock assessment surveys from southern regions (Stevenson and Lauth, 2019), and necessitating the addition of environmental covariates to improve estimates of biomass (O'Leary et al., 2020). Such range shifts may result in altered ecosystem dynamics, fishery interactions and vulnerabilities, and fluctuations in environmental conditions.
When foundation species collapse (Dayton, 1972), there are broad ramifications for biodiversity (Hawkins et al., 2009; Hooper et al., 2012; Fraser et al., 2014), productivity, and ecosystem services (Parmesan et al., 2000). However, critical thresholds, vulnerabilities, synergies and novel stressors created by climate-driven impacts to populations and ecosystems are still poorly understood (Graham et al., 2003; Newbold et al., 2020). Predicting and managing transitions toward novel ecosystems in an area, and the potential biodiversity and productivity loss, is a particular challenge for natural resource managers (Keith, 2015).
There is a need to improve our understanding of both the impacts of climate change and what management actions may be beneficial for complex social-ecological systems (Pinsky and Mantua, 2014). Climate-ready or -resilient strategies will need to be developed to effectively prepare for and adapt to changing conditions. However, the challenge has been to understand interactions, which impacts to anticipate, on what timeframes, and over which spatial scales (Cassotta et al., 2022). These goals are further complicated by the effects of concurrent and interacting stressors, including anthropogenic, biological, and climate stressors. Cascading impacts and risks to ecosystems (Rocha et al., 2018) may accelerate the collapse of natural resources that are the foundations of societal cultures and economies.
In this paper, we present a conceptual framework (Figure 1A) for investigating and developing adaptive management strategies, to address these cascading risks of climate-driven ecosystem impacts. Investigating case studies of ecosystems that are sensitive to climate change will be the key to developing ecosystem-based conceptual models to inform management (Biggs et al., 2015). To illustrate an approach, we develop a model for the red abalone fishery in northern California following the 2014–2016 Marine Heatwave (MHW) (Primary Climate Stressor), and the resulting transition of the kelp forest ecosystem to an urchin barren (System Response). We assess multiple impacts to the abalone population (Resource Impacts), including abalone health and reproduction, shifts in habitat utilization patterns, population density, and mortality. Additional Stressors are identified that may interact with the impact to the abalone, and accelerate population and fishery collapse. The ramifications of mass mortalities, reductions in productivity and increased vulnerability to fishing and other stressors are discussed as an example of the challenges of fishery management in a warming ocean (Figure 1B). Additional potential Adaptive Management approaches are discussed, as outlined in the conceptual framework.
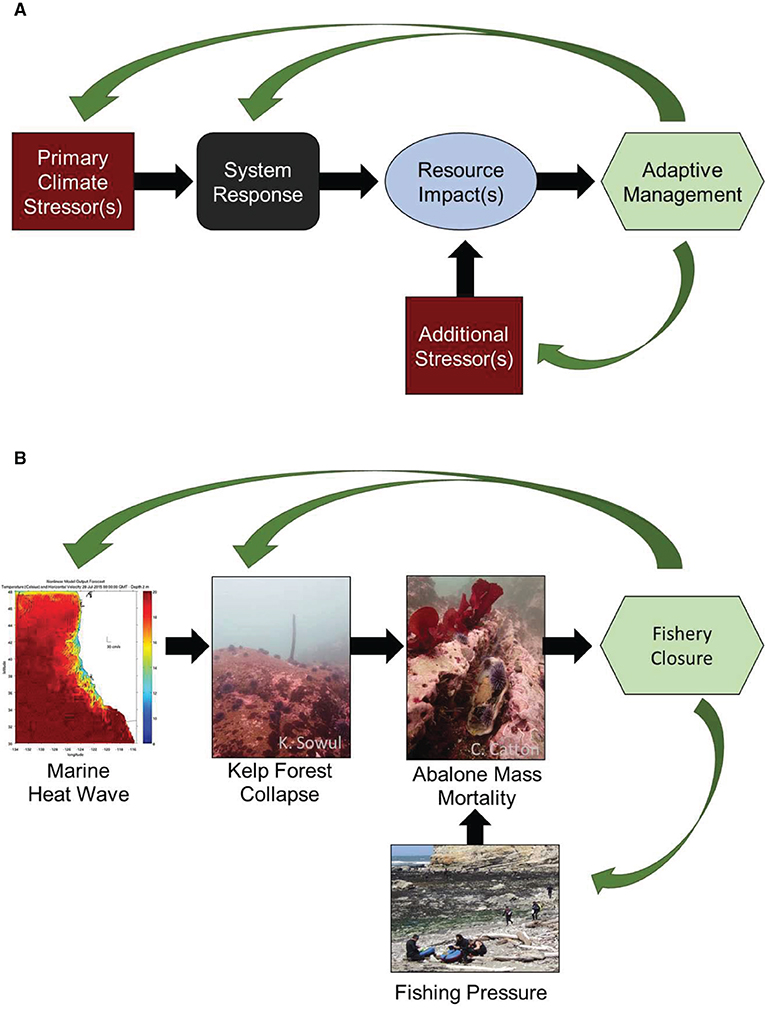
Figure 1. (A) Conceptual Framework for investigating cascading risks of climate change. (B) Conceptual Framework: Abalone case study (with details of “Resource Impact” and “Additional Stressors” explored in the manuscript).
Red abalone case study
Ocean warming is disproportionately impacting nearshore ecosystems (Barry et al., 1995; Thompson et al., 2002; Frölicher and Laufkötter, 2018), and MHW are increasing in both frequency and intensity now and into the future (Meehl and Tebaldi, 2004; Oliver, 2019). Gradual ocean warming (Hoegh-Guldberg et al., 2014) and episodic MHW impacts (Hobday et al., 2016) are leading to declines in the organisms that structure coral reefs (Hughes et al., 2017), seagrass meadows (Orth et al., 2006; Marbà and Duarte, 2010; Fraser et al., 2014), and kelp forest communities (Wernberg et al., 2013; Miller et al., 2018; Thomsen et al., 2019; Smale, 2020). These declines have resulted in dramatic decreases in biodiversity, population declines, and unprecedented decreases in ecosystem productivity, obfuscating resource management (Mills et al., 2013; Pershing et al., 2015). Further complicating management are interactions (synergies) between ocean warming and other stressors in complex and unexpected ways (Harley and Rogers-Bennett, 2004; Harley et al., 2006). These interactions are creating an intensification of climate-driven ecosystem declines, such as declines in salt marshes due to crab overgrazing exacerbated by sea level rise, which further softens the marshes and increases the vulnerability to crab overgrazing (Crotty et al., 2017). Ocean warming may unexpectedly magnify fishing pressures, heightening vulnerabilities and confounding management strategies (Bennett et al., 2004; Harley and Rogers-Bennett, 2004; Caputi et al., 2016). Therefore, it is imperative that we understand how climate change impacts resources directly and indirectly, interacts to magnify stressors, or creates novel stressors so that we can anticipate outcomes and craft adaptive climate-resilient management strategies.
Nearshore kelp forest ecosystems, and the fishery resources they support, are particularly vulnerable to ocean warming (Smale and Wernberg, 2013; Wernberg et al., 2016; Arafeh-Dalmau et al., 2019; Smale et al., 2019), making them model systems for examining non-linearity and unexpected consequences of climate change. Kelps are foundation or engineering species (Jones et al., 1994, 1997) that structure nearshore temperate kelp forests, and their loss (Coleman and Williams, 2002) can result in devastating ecosystem/regime shifts. Bull kelp, Nereocystis luetkeana, is an annual species requiring cool (<13C) nitrate-rich waters (Gerard, 1982; García-Reyes and Largier, 2010) growing >20m in the spring, and forming the lush species-rich kelp forest canopies in northern California. Cool water is also required for abalone (Haliotis spp.) health, growth, and reproduction (Vilchis et al., 2005; Rogers-Bennett et al., 2010), while warm water plus infection can trigger disease symptoms (Friedman et al., 1997; Moore et al., 2000), reproductive failure (Helmuth et al., 2006), cessation of growth (Rogers-Bennett et al., 2007), and mass mortality (Rogers-Bennett et al., 2019). As such, kelp forest ecosystems, and the abalone fisheries they support, have been particularly hard hit during MHWs around the world, leading to ecosystem shifts from kelp forests to sea urchin barrens (Johnson et al., 2011; Ling et al., 2015).
The historically-productive kelp forests in northern California (San Francisco to the Oregon border) were impacted by a series of MHWs from 2014 to 2016, including the NE Pacific MHW originating in the Bering Sea, Alaska (2014–2015) (Di Lorenzo and Mantua, 2016) and El Niño conditions (2015–2016) (Sanford et al., 2019). Thermal stress and nutrient limitation suppressed growth and spore production of the bull kelp during the summer of 2014 throughout the region (Rogers-Bennett and Catton, 2019; McPherson et al., 2021). Persistent warm-water conditions, combined with increases in sea urchin populations, led to the long-term collapse of the kelp forest ecosystem and the recreational red abalone fishery.
The recreational red abalone fishery had been the largest in the world averaging >300 MT per year (2002–2013), worth $44M (Reid et al., 2016) until its closure in 2018. Abalone landings, estimates of density and management measures were largely stable over time prior to the ecosystem transition/shift (Kashiwada and Taniguchi, 2007). The fishery was closely-managed using density surveys to guide management, which included a combination of strategies; size limits, protected areas, seasons, bag limits, as well as breath-hold diving only. The prohibition of scuba protected ~30% of the population in a deep water (<9 m) de facto reserve (Karpov et al., 1998). An estimated additional 7% of the stock was in abalone no-take marine protected areas. Reductions in the fishery take regulations were initiated in 2014 at the onset of the MHW due to prior impacts of a harmful algal bloom in the southern half of the fishery (Rogers-Bennett et al., 2012, 2019). Additional regulatory reductions were implemented in 2017 and the fishery was closed in 2018, due to ongoing severe starvation conditions and increased abalone mortalities.
Materials and methods
Bull kelp forest ecosystem monitoring
Kelp communities in northern California (San Francisco to the Oregon border) are on rocky reefs dominated by bull kelp, Nereocystis luetkeana. The understory is composed of short fleshy red and crustose coralline algae as well as subcanopy kelps, such as Pterygophora and Laminaria. These subtidal rocky reefs in northern California support a diverse assemblage of macroalgae and marine invertebrates. We investigated the changes to the red abalone population in northern California from 2003 to 2007 (baseline time period), 2014 to 2016 (early years of kelp forest collapse), and 2017 to 2018 (mass abalone mortality).
The bull kelp forest monitoring program, jointly led by the California Department of Fish and Wildlife and the University of California, Davis, has conducted subtidal nearshore surveys in northern California since 1999 (Rogers-Bennett and Catton, 2019). These surveys focus primarily on key abalone fishery sites, as well as Marine Protected Areas (MPA), in Sonoma and Mendocino counties in northern California. The coastline of these two counties was historically dominated by dense species-rich bull kelp (Nereocystis luetkeana) forests which supplied 96% of the historic catch in the recreational abalone fishery in northern California. Four sites in Sonoma County were surveyed regularly, including (from south to north): Fort Ross, Ocean Cove, Timber Cove, and Salt Point. In Mendocino County, five sites were surveyed regularly: Point Arena, Van Damme, Point Cabrillo (no-take reserve), Caspar Cove, and Todds Point (Figure 2). Surveys were conducted on subtidal rocky reefs by teams of scuba divers.
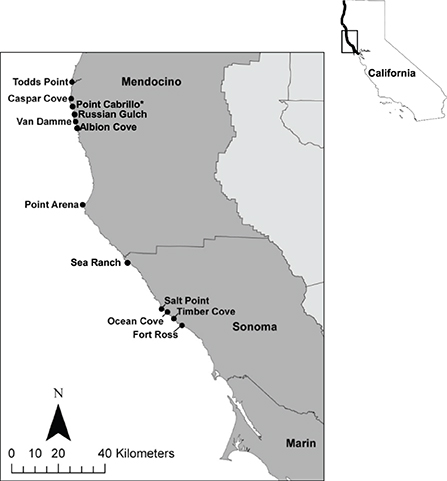
Figure 2. Scuba fishery index sites and *Marine Protected Area in northern California. The thick black coastline in the California inset map indicates the area dominated by bull kelp (San Francisco to the Oregon border).
A total of 25–55 transects were surveyed at each site, in areas with >50% rocky reef habitat. Each of the survey sites represented 2.4–3.2 km of coastline, extending to 18.2 m depth. Surveys were stratified by four depth zones. Depths included two shallow depth strata from 0 to 4.6 m (A) and from 4.7 to 9.1 m (B) as well as two deeper depths from 9.2 to 13.7 m (C) and 13.8 to 18.2 m (D). At each depth stratum, transects were randomly distributed, and divers enumerated abalone, and their size was recorded along the longest axis of the shell to the nearest mm using calipers. The surveys were non-invasive such that large boulders were not turned over to look for organisms.
Red abalone density estimates were made by averaging the densities of abalone counted in 30 × 2 m transects within each of the four depth strata and then calculating the average density across sites for a given depth stratum. The error bars represent standard error of the means. In 2017 and 2018, divers counted abalone as live, freshly dead (detached from the reef, and unresponsive), dying (detached from the reef, but somewhat responsive), and empty red abalone shells. In 2017, all nine sites were re-surveyed to record mortalities, and to assess the overall density decline from baseline. In 2018, six fishery sites were re-surveyed in order to assess continued mortality impacts.
Depth distribution analysis
Previous research has shown that red abalone are more abundant in the shallower depth strata, and that a minimum density of 0.2 abalone m−2 is an important reference point (MSD—minimum spawning density) for abalone population productivity (Karpov et al., 2000; Button, 2008). Because the red abalone fishery regulations were designed to protect a productive population beyond typical freediving depths (>9 m), a logistic regression was used to investigate shifts in the depth distribution of the red abalone with respect to the MSD reference point. Inputs to the generalized linear model in R were median transect Depth (D), Time period (T), and Site (S). A binomial MSD score (1 = transect density above MSD; 0 = below MSD) was input as the dependent variable. The Akaike Information Criterion (AIC) values of seven candidate models with increasing complexity were compared and weights calculated for each (Burnham and Anderson, 2002, 2004) (Supplementary Table S1). For each site and time period, the probability of transect densities greater than MSD was calculated for 9 m depth (shallowest depth of the intended depth refuge for the fishery).
Red abalone fishery trophies
An elite group of recreational abalone fishers is the trophy hunters, with expertise in catching the largest abalone. These divers and rock pickers measure their catch and keep meticulous records of size and date captured. Data for these record large abalone include: rank by size, abalone size, county where the abalone was taken, fisher's name, and capture date. Data from the largest red abalone are maintained on the California Department of Fish and Wildlife website of fishing records. The 20 largest abalone ever taken in the fishery are ranked on this unofficial list of record-breaking abalone. The largest red abalone ever taken in the fishery (313.4 mm) is recorded on this list and this is also the largest abalone in the world. These records have been collected for 34 years beginning in 1984. However, no abalone shells larger than these records have come forward from prior years. The length of the abalone is measured along the longest dimension of the shell and all of the record breaking red abalone are >288 mm. The procedure for submitting and verifying abalone for this list is shown here https://www.wildlife.ca.gov/fishing/records.
Note: in some cases there are more than one abalone of the same rank and size.
Abalone health and reproduction
Abalone surveys were conducted at multiple sites throughout the fishery in Sonoma and Mendocino counties during spring low tides. Sites included (from south to north) in Sonoma County: Fort Ross, Salt Point, Sea Ranch; in Mendocino County: Moat Creek, Point Arena, Van Damme, South Coast Trail, Glass Beach, MacKerricher; and Hardy Creek. Van Damme was the most important fishery site and had the highest catches in the last years of the fishery (2014–2017). Data collected included the counts and lengths of abalone caught per fisher (rock-pickers and freedivers), as well as notes on any observations of abalone with a shrunken foot muscle (a symptom of starvation). In 2016 and 2017, samplers recorded a shrinkage score for all observed abalone at 10 sites in Sonoma and Mendocino counties due to strong concern over the potential impacts of starvation on the health of the abalone stock. The shrinkage score ranged from 0 (no shrinkage) to 3 (severe shrinkage), using a photographic guide to the visual scoring that is also used to assess shrinkage due to Withering Syndrome in abalone (Figure 3) (ranking developed by J. Moore). The disease agent for Withering Syndrome, which starves the abalone of nutrients due to a microbial infection in the gut, was not detected in any abalone in the study region (J. Moore pers. comm.). The abalone taken in the fishery were typically the largest and highest quality that the divers encountered, since the daily limit was three abalone.
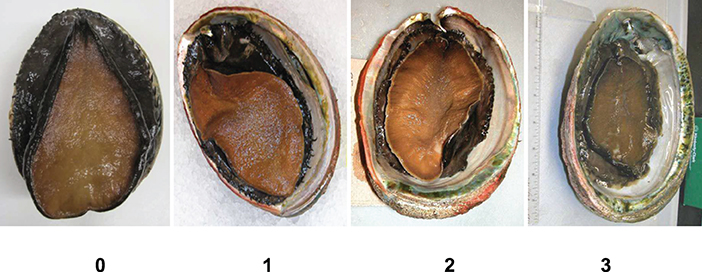
Figure 3. Abalone body shrinkage scores (0–3; increasing in shrinkage from no shrinkage 0 on the left to right severe shrinkage).
The relationships between the visual shrinkage score and other indices of abalone body condition and gonad development were assessed for legal-sized (>178 mm length) wild abalone samples from Van Damme State Park (2000–2009, 2016–2017) (see Methods in (Rogers-Bennett et al., 2021). All abalone sampled prior to 2016 were assigned a shrinkage score of zero because only visually healthy individuals were encountered in the surveys. By 2016–2017, some shrunken individuals were showing up in the fishery and so all sampled abalone were assigned a visual shrinkage score. Rogers-Bennett et al. (2021) reported gender-specific median body condition and gonad index values for abalone during the baseline period, and during 2016–2017. The median baseline body condition index was 0.136 for females and 0.153 for males. The median baseline gonad index was 292 for females and 194 for males. In 2016–2017, the gender difference was not significant, so the analysis of the body condition and gonad indices relative to the shrinkage score used all available data. A Kruskal-Wallis test was used to test for differences in body condition and gonad index across shrinkage scores recorded in 2016–2017.
The growing edges of the shells were also examined in a subset of the catch. In normal years at the growing margin of the shell there is a distinct band of red shell where new shell is deposited.
Larval and newly-settled abalone
To assess the recent evidence of abalone productivity in the area, larval and newly-settled abalone surveys were conducted from 2007 to 2018. Surveys were a snapshot in time conducted once per year (Rogers-Bennett et al., 2016). Plankton tows for abalone larvae were conducted in shallow 10–15 m (n = 3) and deep water 33–40 m (n = 3) habitats at Van Damme State Park in August. All abalone larvae observed in the plankton samples were of the size indicating that they were spawned within the previous 2 weeks. Samples were sorted under the microscope looking for larval abalone which were roughly 280 μm in length (see Section Methods in Rogers-Bennett et al., 2016). To quantify the number of newly settled abalone (<1 mm) on crustose coralline cobbles, cobbles (n = 80) were collected also at Van Damme State Park in August each year. Cobbles were collected by divers from a range of depths, rinsed, and the contents of the samples were sorted under a microscope looking for newly settled abalone (see Section Methods in Rogers-Bennett et al., 2016). All newly settled abalone <1 mm in shell length were enumerated.
Results
The impacts of the kelp forest collapse on the red abalone population following the onset of the MHW in 2014 progressively increased over time. We describe the results of the abalone surveys and abalone sampling efforts according to the sequence of observed impacts to the population.
Emergence of small and trophy size abalone (2014–2016)
The lengths of 3,348 red abalone were measured during subtidal scuba surveys during the early years of the kelp forest collapse (2014–2016) at six of the fishery index sites (Supplementary Table S2). A total of 6,496 red abalone were measured at those same sites during the baseline time period (2003–2007). A two-sample Kolmogorov Smirnov test showed that the two distributions were significantly different (D = 0.15954; p < <0.0001) (Figure 4). During the early years of the kelp forest collapse (2014–2016), a higher proportion of sub-legal abalone (<150 mm length), and three of the largest abalone ever observed (277, 285, and 296 mm length) during the surveys were recorded. Sub-legal abalone usually hide under rocks and in crevices in the rocky reef so they are typically less detectable than larger individuals. Likewise, trophy-sized abalone (>250 mm) were historically very rare to observe during the surveys. The red abalone that were observed during the kelp forest collapse were actively out of crevices searching for food and moving on rocky reef surfaces (personal observation). This unusual behavior could be contributing to the broader distribution of abalone lengths recorded during starvation and kelp forest collapse.
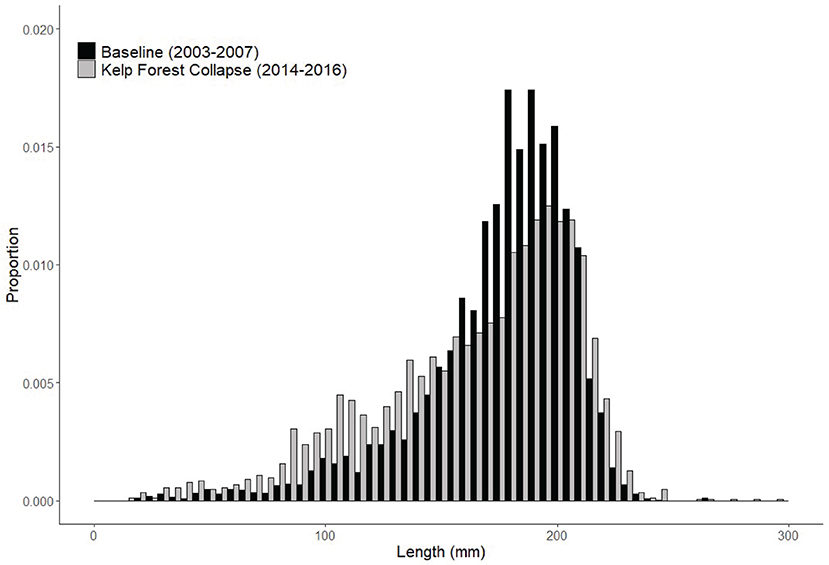
Figure 4. Size frequency distribution comparing abalone sizes in baseline years (2003–2007) with abundant kelp forest with abalone sizes after the kelp forest collapsed (2014–2016).
Fishery trophy records (2014–2016)
During the MHW (from 2014 to 2016), in just 3 out of a total of 34 years, 30% of the largest 20 record breaking red abalone (ranging in size from 295.0 to 289.0 mm) were captured in the fishery. One abalone was taken in 2014, two abalone in 2015 and then three record size red abalone were taken in 2016. The fishery was closed in 2018. In contrast, no record size abalone were taken in the previous decade in the fishery from 1998 to 2010. This large number of record size abalone were taken even though both catch and effort were lower in the MHW years (CDFW unpublished data). The largest abalone ever taken in the fishery was 313.4 mm and it was taken the year following a strong warm water El Niño event in 1993.
Body condition and gonad index (2016–2017)
Shrinkage scores were recorded for 11,818 red abalone at 9 fishery sites in 2016 (n = 5,108) and 10 fishery sites in 2017 (n = 6,710) (Supplementary Table S3). Visible body shrinkage was extensive across all of the fishery sites sampled. Shrunken abalone comprised 8–54% of the observations at a site (Figures 5A,B; Supplementary Table S3), with an average of 30 and 26% shrunken across all of the sites in 2016 and 2017, respectively. The highest percentage of shrunken abalone was observed at Fort Ross in 2017. Most of the shrunken abalone were scored with the first level of shrinkage (1: 17–21% average). One percent of the observed abalone were scored as severely shrunken (3). By the 2017 fishing season, fishers often shared how they looked for healthy abalone and avoided obviously shrunken abalone before selecting it for harvest. Divers observed abalone shells resting directly on the rocky reef where they resembled empty shells rather than being able to see the shell above the surface of the rock raised up by the thick mass of the abalone's healthy body. Divers noted that the shrunken abalone were weak as they were easily able to pick up by hand rather than prying the animal off the rock with an abalone iron. Further, healthy red abalone that are growing in size have a bright red shell margin which was absent in the vast majority of abalone examined during the body condition sampling in 2016–2017.
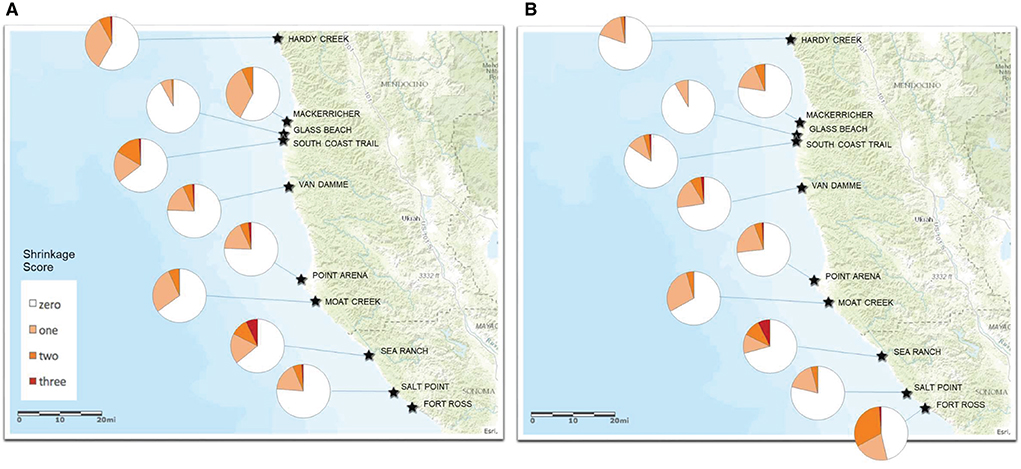
Figure 5. (A) Red abalone shrinkage score distribution at 9 creel survey sites in 2016. See Supplementary Table S2 for summary results. (B) Red abalone shrinkage score distribution at 11 creel survey sites in 2017. See Supplementary Table S2 for summary results.
Abalone sampled at Van Damme State Park in 2016 (n = 57) and 2017 (n = 64) were unevenly distributed across shrinkage scores (n0 = 34; n1 = 60; n2 = 21; n3 = 6). Median body condition index (BCI) and gonad index (GI) values decreased as the shrinkage score increased in severity (Figure 6). Kruskal-Wallis tests of the BCI (Chi2 = 38.997, df = 3, p < <0.0001) and the GI (Chi2 = 20.65, df = 3, p = 0.0001) both indicated significant differences across shrinkage score categories. The median BCI and GI was lower in 2016–2017 than the baseline index values (BCIbaseline = 0.136–0.153; GIbaseline = 194–394), regardless of shrinkage score (BCI0 = 0.121; BCI1 = 0.103; BCI2 = 0.086; BCI3 = 0.064; GI0 = 50.8; GI1 = 25.1; GI2 = 15.1; GI3 = 0). The low gonad index scores were associated with low numbers of eggs and few gonad with mature sperm present in histological samples of the gonad tissue (Rogers-Bennett et al., 2021).
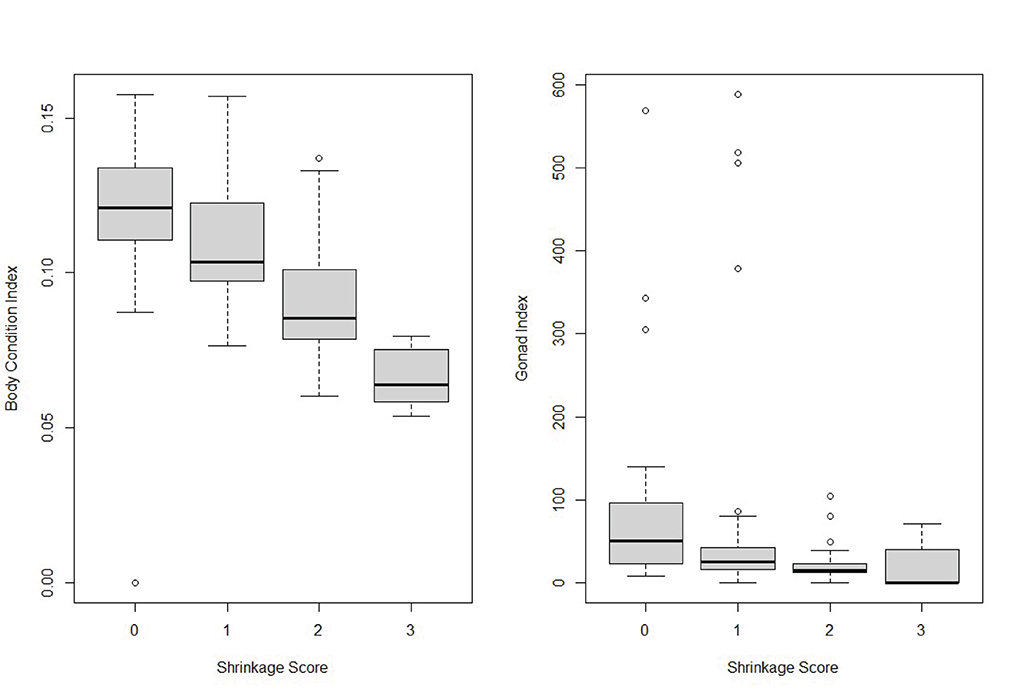
Figure 6. Boxplots of body condition index (left) and gonad index (right) by shrinkage score in 2016 and 2017 from Van Damme State Park (males and females combined). Baseline Body Index (2000–2009) = 0.144 (se = 0.001) Baseline Gonad Index (2000–2009) = 507 (se = 80.8).
Larval and newly-settled abalone (2014–2018)
Abalone productivity as seen by the production of abalone larvae and newly settled abalone was low during and after the MHW years (Figure 7). Prior to the warm water years larval abundance was patchy but in half the years larvae were seen in plankton tows conducted at Van Damme State Park in August from 2007 to 2012 (Rogers-Bennett et al., 2016). In 2013 and 2016, red abalone larvae were present in the plankton tows, but during the years encompassing the MHW (2014–2016) and the years that followed 2017–2019, no abalone larvae were encountered. Newly settled abalone were found on crustose coralline covered rocks from 2007 to 2013 (with the exception of 2012) (Rogers-Bennett et al., 2016). In 2014–2019, no newly-settled abalone were encountered on any of the rocks, although Hart et al. (2020) encountered some further south in kelp forests in the Monterey area during those years.
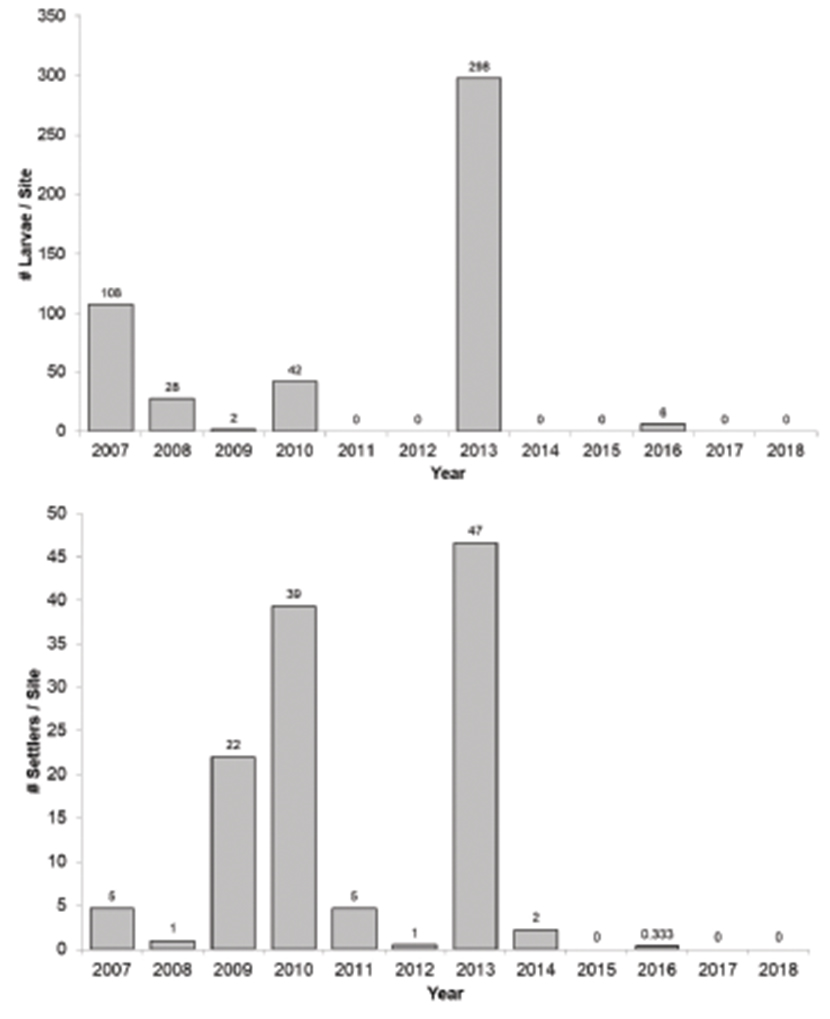
Figure 7. (Top) Number of larval abalone found in 6 plankton tows in August of each year at sites in northern California. (Bottom) The number of newly settled abalone found on crustose coralline covered rocks (N = 80) in August of each year at sites in northern California.
Mass mortality (2017–2018)
In 2017 and 2018, 36,240 m2 of subtidal rocky reef habitat were surveyed, and 5,493 live red abalone and 3,597 dead abalone (dead, dying, or empty shells) were quantified across all sites in both years (Table 1). The percentage of observed abalone that were dead at a site ranged from 22 to 84%, with the highest percentage reported at Caspar Cove in both 2017 (67%) and 2018 (84%). The percentage dead increased in 2018 at nearly all re-surveyed sites, with Fort Ross jumping from 23% in 2017 to 73% in 2018. The mortalities were also observed at the Point Cabrillo State Marine Reserve in 2017 (22%).
Depth distribution shift (2017)
Red abalone densities declined dramatically by 2017 due to mass mortalities (see Mass Mortality above) in all depths. Historically, subtidal abalone population densities were high (0.24–1.01 abalone m−2) during the years before the MHW and subsequent mass mortalities (Rogers-Bennett and Catton, 2019). Abalone population densities were also differentially impacted by depth, such that the three shallowest strata [A-C (<13.8 m depth)] experienced > 70% declines in density, and the deepest stratum experienced ~40% density decline overall (Figure 8). Although the historical pattern of higher densities in shallower habitats remained, the average density in the shallowest depth stratum [A (<4.9 m depth): 0.30 abalone m−2] was the only one above the MSD reference point of 0.20 abalone m−2.
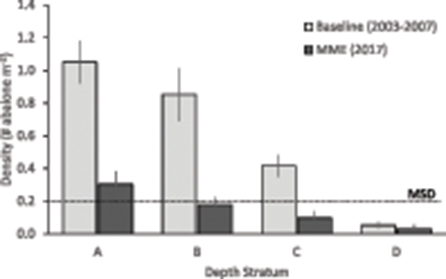
Figure 8. Comparison of average transect density across time periods, by depth stratum (shallow to deep) at the eight fishery index sites. Depth strata: (A) 0–4.6 m, (B) 4.7–9.1 m, (C) 9.2–13.7 m, (D) 13.8–18.2 m. Black dashed line represents the minimum spawning density (MSD) reference point used in the depth distribution analysis. “MME” is Mass Mortality Event.
The best fit models for estimating the probability of transect densities greater than MSD include all three factors (Depth, Time Period, and Site) and an interaction term (Table 2), indicating that the relationship between depth and the MSD probability differed across site and time period. During the baseline time period, the probability of densities >MSD was high (0.98 average) in the shallow depths, gradually decreasing from 0.9 probability in depths >3.5–7.0 m toward <0.1 in depths >15 m, depending on the site. The probability of densities >MSD at 9 m depth (the fishery refuge depth) ranged between 0.56 and 0.83 across the sites (average 0.71).
In 2017, the probability of densities >MSD in the shallowest depths were substantially reduced from baseline, and were highly variable across the sites (0.45–0.91; 0.72 average). The probabilities further declined with increased depth, such that the average probability of densities >MSD at 9 m depth averaged 0.14 (range: 0.05–0.29). Those estimated probabilities in 2017 are 0.51–0.67 lower than baseline estimates.
Discussion
Red abalone impacts
The progressive impacts on the abalone population (Resource Impacts) of the MHW (Primary Climate Stressor) and the kelp forest collapse (System Response) are outlined in the Conceptual Model case study detail in Figure 9. As a result of the Resource Impacts, a series of unanticipated anthropogenic, biological, and environmental stressors are identified that the population may be more vulnerable to in the impacted state (Table 3).
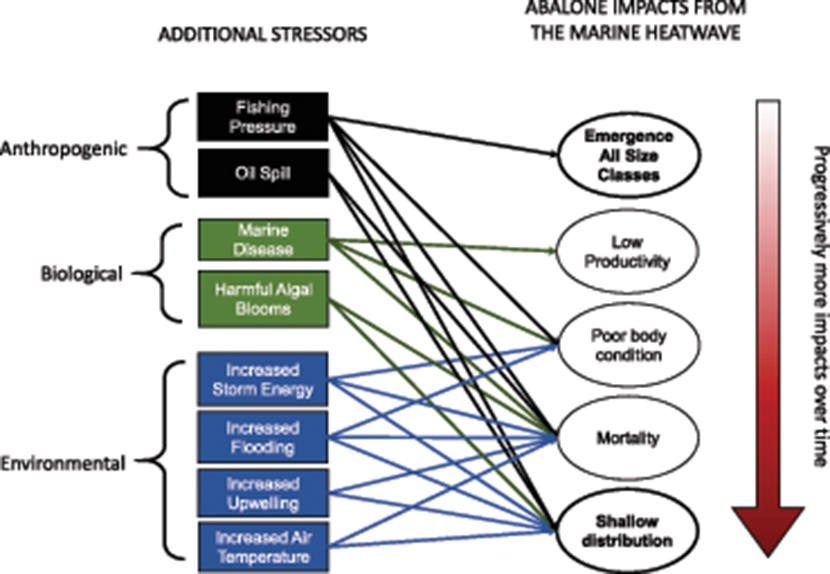
Figure 9. Conceptual Model (details of “resource impact” and “additional stressors” explored)—Abalone Example Interaction of additional stressors with consequences of MHW bold outline = unanticipated impacts.
In this red abalone case study, the impacts were cumulative and became increasingly more severe over time. Three of the impacts were expected, once food became extremely limited for abalone, and proceeded to occur in a predictable order based on previous research—low productivity, poor body condition, and mortality (Vilchis et al., 2005; Rogers-Bennett et al., 2010). Low quality or insufficient food availability reduces available energy for producing gametes. If low food conditions persist for an extended period, the abalone will cease to add new shell, which was observed in this case study as the absence of a bright red growing lip on the anterior margin of the shell. Prolonged starvation leads to poor body condition, and results in the weakening and shrinking of the foot muscle. The foot muscle is critical for adhesion, protecting the abalone from being dislodged by predators or large waves, and is used to capture drift algae for feeding. By the spring of 2016, visibly shrunken abalone were widely observed throughout the region (Figure 5), which corresponded to significantly reduced gonad and body condition (Figure 6). In the fall of 2016, freshly dead or dying abalone began to wash onto the shore in large numbers. Mortalities substantially increased in 2017 and 2018, as 40% of the abalone observed succumbed to the starvation conditions. Many abalone were seen upside down on the reef, having failed to adhere to the rock, and were vulnerable to predators and scavengers (Table 1).
The duration, geographic scale, and severity of these impacts were devastating to the productivity and recovery potential of the red abalone population in the region. The high mortality rates resulted in substantial declines in population densities throughout the depth ranges, across all of the sites (including inside a Marine Protected Area). Because abalone are broadcast-spawning and sedentary species, population densities need to be sufficiently high for successful fertilization to occur (Babcock and Keesing, 1999; Button, 2008). However, gamete production had also been severely impacted after 2015, and laboratory studies have shown that recovery from starvation may require more than a year for gamete production to resume, even with constant access to food (S. Boles, personal communication). Population recovery through natural reproduction and growth will take many years to decades to achieve even after the kelp forest may be restored, due to the ongoing declines in population densities below Minimum Spawning Density, and the recovery time needed for gamete production, and growth through early life history to maturity ~12 years (Rogers-Bennett et al., 2007).
Two additional impacts to the red abalone population were unanticipated—the emergence of size classes and the shift in the depth distribution to shallow habitats—both of which made the population more vulnerable to fishing (Table 3). These impacts were both driven, in part, by changes in abalone behavior in response to prolonged food limitation. Divers often noted that the abalone observed during the transect surveys were actively moving along the reef, presumably in search of food. This behavior is extremely unusual for abalone during the day, as they are usually sedentary or hidden in crevices. The emergence of sublegal and trophy-sized abalone increased the vulnerability of those size classes to predation and fishing. Thirty percent of the 34-year record of trophy-fished abalone were recorded since the onset of the MHW in 2014, suggesting that the MHW and kelp forest collapse increased the vulnerability of trophy-sized abalone to fishing.
This increased abalone mobility resulted in a shift in the depth distribution toward the intertidal, where a narrow band of fleshy algae persisted. Recreational abalone fishers frequently remarked on how many abalone they encountered high in the intertidal, and that the abalone were sometimes stacked on top of each other. These observations created a misleading impression that the abalone population was more abundant than in the past because they were in such high numbers where they were tidally-accessible. Many fishers did not believe the red abalone population was undergoing a mass mortality event since they saw more abalone in the shallowest habitats, further impeding fishery management efforts to protect the surviving stock.
Cascading risks and vulnerabilities
With each new impact to the abalone, the number and types of additional stressor vulnerabilities also increased. Many of the stressors listed in Table 3 had not been previously identified as high risks to red abalone population viability. Oil spills and increased flooding were considered to have only localized and infrequent impacts to the population, because abalone were previously distributed beyond (deeper than) the areas at risk. Severe wildfires in the region, such as the Tubbs Fire (2017) and the Glass Fire (2020) intensified stressors associated with flooding, such as high sediment loads from landslides and toxic pollutants from burned structures. Following a major freshwater flooding event in February 2019, abalone near river mouths were observed dead and washed up on the beaches.
Increased storm wave energy (Young et al., 2011), harmful algal blooms (Rogers-Bennett et al., 2012), and warming air temperatures have also been a growing concern as climate stressors that would have previously not had major impacts on subtidal abalone populations (Table 3). The wave energy from winter storms dislodged weakened abalone from the shallow rocky reefs and washed them onto local beaches in large numbers. Wave height data from the Coastal Data Information Program from the buoy at Cape Mendocino (station 094) for the years 1999–2021 showed that wave heights infrequently exceeded 9 m prior to 2015. During the MHW, and in subsequent years of the ongoing kelp forest collapse and mass abalone mortalities, anomalously large winter waves continued to impact the northern California shores. In November 2019, wave heights exceeded 12 m, the largest recorded waves in the record. These observations are consistent with the prediction that climate stressors are increasing in intensity and frequency, even as the fishery resources may be becoming more vulnerable to those and other stressors. As the depth distribution of the abalone population shifted dramatically up the shore, and the deep water population became scarce, these climate-related stressors pose greater risks to the overall population viability.
Adaptive management
As illustrated in the conceptual framework (Figure 1A), the goal of adaptive management will be to detect and respond to the Primary Climate Stressor(s), the System Response, and/or the Additional Stressors. For the abalone case study, the initial management response was focused solely on reducing the additional stressor associated with fishing pressure. In 2017 the fishing limits were reduced, and the following year the fishery was closed in 2018, due to ongoing mass mortalities and severe declines in population density (Rogers-Bennett et al., 2019) (Figure 1B). These decisions were informed by increased research efforts of the abalone and the ecosystem, starting in 2015. There was resistance from a small portion of the fishers who observed increased numbers of abalone in the very shallow waters, however most divers saw the massive large-scale mortality impacts. Given that major population-level impacts can arise in a short amount of time, the challenge to natural resource management will be to monitor the resource, and quickly impose restraints to extraction on environmentally-stressed populations. Adaptive management to the Primary Climate Stressor(s) must include both mitigation and adaptation strategies, to dampen or reverse the increasing trend in global temperature. Protecting existing kelp forests, and many other vulnerable foundational species (e.g., seagrasses and terrestrial forests), is critically important to maintain biodiversity and carbon sequestration.
Despite precautionary management, a fishery management plan in action, and management regulations in place to protect a portion of the stock (e.g., minimum legal size limit, breath-hold diving, and MPAs), large-scale starvation conditions undermined those productivity protection and management measures. Gonad index values <30% of baseline, and the absence of larval and newly-settled abalone in surveys, signaled a potential problem with fishery recruitment in the future. The shift in the depth distribution, and high mortalities even in Marine Protected Areas, signaled the loss of the reserve populations as sources of productivity for the fishery. With ongoing population declines, and multiple indicators of long-term reproductive failure, any level of fishing was determined to be unsustainable. The fishery management strategies were designed for regular recruitment with constant natural mortality, which this case study shows is not consistent with climate change impacts to populations. Instead, fishery management plans will need to be redesigned to improve detection capabilities, responsiveness, and resilience to large-scale climate impacts and cascading risks. Without climate-ready management, fishing the survivors of unfavorable environmental conditions could greatly reduce the pace of future population recovery, or drive the stock to local extinction. A Draft Red Abalone Fishery Management Plan is being written in 2022, in response to the lessons learned from the kelp forest collapse, that expands the indicators guiding fishery management decisions to include environmental and biological indicators of ecosystem and abalone productivity (Fish and Game Commission March 2022; fgc.ca.gov).
Along with management efforts to stop fishing mortality, there is an urgent need to restore the kelp forest. Again, the urgency of the situation was misunderstood as many fishers, managers, and scientists thought the system would naturally rebound as it had in the past. With the wide-scale transition to sea urchin barrens (System Response), a quick recovery was not possible without intervention. In 2016, research was initiated to explore opportunities to support kelp forest recovery (System Response), through coordinated urchin harvest and kelp forest restoration approaches. Given that most kelp restoration projects are small in scale (<1 ha) and short in duration, the recommendation is for larger projects to enhance success. A collaborative kelp recovery plan for northern California was developed in 2019 to guide collective efforts by agencies, tribes, industry groups, universities, and non-profits (Hohman et al., 2019) yet the response has taken 8 years since the MHW and KFC for the state agencies to produce an action plan for restoring California's Kelp Forests (California Ocean Protection Council 2021). A review of possible policy responses suggest that resisting the decline and promoting kelp forest restoration will be key for the future of this ecosystem (Rogers-Bennett et al., 2022).
Climate change, and the resulting ecosystem impacts, are arguably some of the biggest challenges for natural resource science and management today. This work demonstrates the importance of tracking multiple metrics to develop an ecosystem-based conceptual model to inform management. The red abalone case study highlights the challenges with fixed management strategies, or strategies that do not explicitly incorporate environmental or ecosystem indicators. While adaptive management approaches are often designed to be reactive to changes in stock conditions, proactive management of additional potential stressor vulnerabilities may improve climate resilience of the ecosystem and the stock. Ecosystem-based management of critical habitats, as an adaptation strategy, may be improved by implementing environmental forecasting models, to inform understanding of climate drivers of ecosystem dynamics (McPherson et al., 2021). Identifying the likely resource responses to potential major ecosystem disruptions, and interactions with additional stressors, is the first step toward developing adaptive management strategies to protect resources in the face of climate-driven reductions in productivity and biodiversity. Continuing to examine outcomes from additional case studies will be critical to improving our understanding of the cascading impacts and risks of climate change.
Permissions for protected areas
Underwater surveys were conducted inside and outside Marine Protected Areas (MPA). Surveys in Van Damme State Park, and Salt Point State Park State Marine Conservation Areas and Point Cabrillo State Marine Reserve were conducted in collaboration with, and the permission of, the California Department of Fish and Wildlife who is the managing authority for MPAs in California.
Data availability statement
The raw data supporting the conclusions of this article will be made available by the authors, without undue reservation.
Author contributions
LR-B and CC wrote the paper, contributed to data collection, and performed the analyses. All authors contributed to the article and approved the submitted version.
Funding
This work was supported by the California Department of Fish and Wildlife and NSF OCE 2023664.
Acknowledgments
Thank you to patrol vessel captains A. Roberts, B. Bailie, and R. Puccinelli of the wildlife enforcement division and the diving safety officers D. Osorio and J. Herum who made this work possible. Thank you to S. Kawana and the many volunteer divers and boat operators who helped gather data on red abalone. J. Bray CSU COAST intern created figures of abalone condition. This is a publication of the University of California, Davis, Bodega Marine Laboratory and the Coastal Marine Science Institute.
Conflict of interest
The authors declare that the research was conducted in the absence of any commercial or financial relationships that could be construed as a potential conflict of interest.
Publisher's note
All claims expressed in this article are solely those of the authors and do not necessarily represent those of their affiliated organizations, or those of the publisher, the editors and the reviewers. Any product that may be evaluated in this article, or claim that may be made by its manufacturer, is not guaranteed or endorsed by the publisher.
Supplementary material
The Supplementary Material for this article can be found online at: https://www.frontiersin.org/articles/10.3389/fclim.2022.908708/full#supplementary-material
Supplementary Figure S1. Logistic regression model fit summary.
Supplementary Table S1. Sample sizes of red abalone lengths during scuba surveys—Baseline vs. during Kelp Forest Collapse.
Supplementary Table S2. Summary results of shrinkage scores of red abalone during 2016 and 2017 creel surveys.
Supplementary Table S3. Box plots of significant wave height in feet from the Mendocino offshore NOAA buoy station 094 by month from 2015 and 2019.
References
Arafeh-Dalmau, N., Montaño-Moctezuma, G., Martínez, J. A., Beas-Luna, R., Schoeman, D. S., Torres-Moye, G., et al. (2019). Extreme marine heatwaves alter kelp forest community near its equatorward distribution limit. Front. Marine Sci. 6, 499. doi: 10.3389/fmars.2019.00499
Babcock, R., and Keesing, J. (1999). Fertilization biology of the abalone Haliotis laevigata: laboratory and field studies. Can. J. Fish. Aquatic Sci. 56, 1668–1678. doi: 10.1139/f99-106
Barry, J. P., Baxter, C. H., Sagarin, R. D., and Gilman, S. E. (1995). Climate-related, long-term faunal changes in a California rocky intertidal community. Science 267, 672–675. doi: 10.1126/science.267.5198.672
Bennett, W. A., Roinestad, K., Rogers-Bennett, L., Kaufman, L., Wilson-Vandenberg, D., Heneman, B., et al. (2004). Inverse regional responses to climate change and fishing intensity by the recreational rockfish (Sebastes spp.) fishery in California. Can. J. Fish. Aquatic Sci. 61, 2499–2510. doi: 10.1139/f04-203
Biggs, R. O., Peterson, G., and Rocha, J. C. (2015). The regime shifts database: a framework for analyzing regime shifts in social-ecological systems. bioRxiv [Preprint]. doi: 10.5751/ES-10264-230309
Burnham, K. P., and Anderson, D. R. (2002). Model Selection and Multimodel Inference: A Practical Information-Theoretic Approach. New York, NY: Springer.
Burnham, K. P., and Anderson, D. R. (2004). Multimodel inference: understanding AIC and BIC in model selection. Sociol. Methods Res. 33, 261–304. doi: 10.1177/0049124104268644
Button, C. A. (2008). The influence of density-dependent aggregation characteristics on the population biology of benthic broadcast-spawning gastropods: Pink abalone (Haliotis corrugata), red abalone (Haliotis rufescens), and wavy turban snails (Megastraea undosa) (doctoral dissertation). University of California San Diego, La Jolla, CA, United States.
Caputi, N., Mervi, K., Ainslie, D., Ming, F., Alan, P., Yasha, H., et al. (2016). Management adaptation of invertebrate fisheries to an extreme marine heat wave event at a global warming hot spot. Ecol. Evol. 6, 3583–3593. doi: 10.1002/ece3.2137
Cassotta, S., Derksen, C., Ekaykin, A., Hollowed, A., Kofinas, G., MAckintosh, A., et al. (2022). “Polar Issues,” in The Ocean and Cryosphere in a Changing Climate: Special Report of the Intergovernmental Panel on Climate Change (Cambridge: Cambridge University Press). doi: 10.1017/9781009157964
Coleman, F. C., and Williams, S. L. (2002). Overexploiting marine ecosystem engineers: potential consequences for biodiversity. Trends Ecol. Evol. 17, 40–44. doi: 10.1016/S0169-5347(01)02330-8
Crotty, S. M., Angelini, C., and Bertness, M. D. (2017). Multiple stressors and the potential for synergistic loss of New England salt marshes. PLoS ONE 12, e0183058. doi: 10.1371/journal.pone.0183058
Dayton, P. K. (1972). “Toward an understanding of community resilience and the potential effects of enrichments to the benthos at McMurdo Sound, Antarctica,” in Proceedings of the colloquium on conservation problems in Antarctica (Blacksberg, VA), 81–96.
Di Lorenzo, E., and Mantua, N. (2016). Multi-year persistence of the 2014/15 North Pacific marine heatwave. Nat. Climate Change 6, 1042. doi: 10.1038/nclimate3082
Ellison, A. M., Bank, M. S., Clinton, B. D., Colburn, E. A., Elliott, K., Ford, C. R., et al. (2005). Loss of foundation species: consequences for the structure and dynamics of forested ecosystems. Front. Ecol. Environ. 3, 479–486. doi: 10.1890/1540-9295(2005)003[0479:LOFSCF]2.0.CO;2
Fraser, M. W., Kendrick, G. A., Statton, J., Hovey, R. K., Zavala-Perez, A., Walker, D. I., et al. (2014). Extreme climate events lower resilience of foundation seagrass at edge of biogeographical range. J. Ecol. 102, 1528–1536. doi: 10.1111/1365-2745.12300
Friedman, C. S., Thomson, M., Chun, C., Haaker, P. L., and Hedrick, R. P. (1997). Withering syndrome of the black abalone, Haliotis cracherodii (Leach): water temperature, food availability, and parasites as possible causes. J. Shellfish Res. 16, 403–411.
Frölicher, T. L., and Laufkötter, C. (2018). Emerging risks from marine heat waves. Nat. Commun. 9, 650. doi: 10.1038/s41467-018-03163-6
García-Reyes, M., and Largier, J. (2010). Observations of increased wind-driven coastal upwelling off central California. J. Geophys. Res. Oceans 115, 576. doi: 10.1029/2009JC005576
Gerard, V. A. (1982). In situ rates of nitrate uptake by giant kelp, Macrocystis pyrifera (L.) C. Agardh: tissue differences, environmental effects, and predictions of nitrogen-limited growth. J. Exp. Marine Biol. Ecol. 62, 211–224. doi: 10.1016/0022-0981(82)90202-7
Graham, M. H., Dayton, P. K., and Erlandson, J. M. (2003). Ice ages and ecological transitions on temperate coasts. Trends Ecol. Evol. 18, 33–40. doi: 10.1016/S0169-5347(02)00006-X
Harley, C. D., Randall Hughes, A., Hultgren, K. M., Miner, B. G., Sorte, C. J., Thornber, C. S., et al. (2006). The impacts of climate change in coastal marine systems. Ecol. Lett. 9, 228–241. doi: 10.1111/j.1461-0248.2005.00871.x
Harley, C. D. G., and Rogers-Bennett, L. (2004). The potential synergistic effects of climate change and fishing pressure on exploited invertebrates on rocky intertidal shores. Calif. Coop. Oceanic Fish. Investig. Rep. 45, 98–110. Available online at: https://www.researchgate.net/profile/Christopher-Harley-2/publication/230636020_The_potential_synergistic_effects_of_climate_change_and_fishing_pressure_on_exploited_invertebrates_on_rocky_intertidal_shores/links/09e415023fa348d98f000000/The-potential-synergistic-effects-of-climate-change-and-fishing-pressure-on-exploited-invertebrates-on-rocky-intertidal-shores.pdf
Hart, L. C., Goodman, M. C., Walter, R. K., Rogers-Bennett, L., Shum, P., Garrett, A. D., et al. (2020). Abalone recruitment in low-density and aggregated populations facing climatic stress. J. Shellfish Res. 39, 359–373. doi: 10.2983/035.039.0218
Hastings, A., and Wysham, D. B. (2010). Regime shifts in ecological systems can occur with no warning. Ecology Letters 13, 464–472. doi: 10.1111/j.1461-0248.2010.01439.x
Hawkins, S., Sugden, H., Mieszkowska, N., Moore, P., Poloczanska, E., Leaper, R., et al. (2009). Consequences of climate-driven biodiversity changes for ecosystem functioning of North European rocky shores. Marine Ecol. Prog. Series 396, 245–259. doi: 10.3354/meps08378
Helmuth, B., Broitman, B. R., Blanchette, C. A., Gilman, S., Halpin, P., Harley, C. D. G., et al. (2006). Mosaic patterns of thermal stress in the rocky intertidal zone: implications for climate change. Ecol. Monogr. 76, 461–479. doi: 10.1890/0012-9615(2006)076[0461:MPOTSI]2.0.CO;2
Hobday, A. J., Alexander, L. V., Perkins, S. E., Smale, D. A., Straub, S. C., Oliver, E. C. J., et al. (2016). A hierarchical approach to defining marine heatwaves. Prog. Oceanogr. 141, 227–238. doi: 10.1016/j.pocean.2015.12.014
Hoegh-Guldberg, O., Cai, R., Poloczanska, E. S., Brewer, P. G., Sundby, S., Hilmi, K., et al. (2014). “The ocean,” in Climate Change 2014: Impacts, Adaptation, and Vulnerability. Part B: Regional Aspects. Contribution of Working Group II to the Fifth Assessment Report of the Intergovernmental Panel of Climate Change, eds V. R. Barros, C. B. Field, D. J. Dokken, M. D. Mastrandrea, K. J. Mach, T. E. Bilir, M. Chatterjee, K. L. Ebi, Y. O. Estrada, R. C. Genova, B. Girma, E. S. Kissel, A. N. Levy, S. MacCracken, P. R. Mastrandrea, and L. L. White (Cambridge University Press), 1655–1731.
Hohman, R., Hutto, S. V., Catton, C., and Koe, F. (2019). Sonoma-Mendocino bull kelp recovery plan. Plan for the Greater Farallones National Marine Sanctuary and the California Department of Fish and Wildlife. San Francisco, CA: Federal Information and News Dispatch, LLC.
Hooper, D. U., Adair, E. C., Cardinale, B. J., Byrnes, J. E., Hungate, B. A., Matulich, K. L., et al. (2012). A global synthesis reveals biodiversity loss as a major driver of ecosystem change. Nature 486, 105–108. doi: 10.1038/nature11118
Hsieh, C.-h., Glaser, S. M., Lucas, A. J., and Sugihara, G. (2005). Distinguishing random environmental fluctuations from ecological catastrophes for the North Pacific Ocean. Nature 435, 336–340. doi: 10.1038/nature03553
Hughes, T. P., Kerry, J. T., Álvarez-Noriega, M., Álvarez-Romero, J. G., Anderson, K. D., Baird, A. H., et al. (2017). Global warming and recurrent mass bleaching of corals. Nature 543, 373. doi: 10.1038/nature21707
Johnson, C. R., Banks, S. C., Barrett, N. S., Cazassus, F., Dunstan, P. K., Edgar, G. J., et al. (2011). Climate change cascades: shifts in oceanography, species' ranges and subtidal marine community dynamics in eastern Tasmania. J. Exp. Marine Biol. Ecol. 400, 17–32. doi: 10.1016/j.jembe.2011.02.032
Jones, C. G., Lawton, J. H., and Shachak, M. (1994). “Organisms as ecosystem engineers,” in Ecosystem Management (New York, NY: Springer), 130–147.
Jones, C. G., Lawton, J. H., and Shachak, M. (1997). Positive and negative effects of organisms as physical ecosystem engineers. Ecology 78, 1946–1957. doi: 10.1890/0012-9658(1997)078[1946:PANEOO]2.0.CO;2
Jönsson, A. M., and Bärring, L. (2011). Ensemble analysis of frost damage on vegetation caused by spring backlashes in a warmer Europe. Nat. Hazards Earth Syst. Sci. 11, 401–418. doi: 10.5194/nhess-11-401-2011
Karpov, K. A., Haaker, P. L., Albin, D., Taniguchi, I. K., and Kushner, D. (1998). The red abalone, Haliotis rufescens, in California: importance of depth refuge to abalone management. J. Shellfish Res. 17, 863–870.
Karpov, K. A., Haaker, P. L., Taniguchi, I. K., and Rogers-Bennett, L. (2000). Serial depletion and the collapse of the California abalone (Haliotis spp.) fishery. Can. Special Publ. Fish. Aquatic Sci. 130, 11–24.
Kashiwada, J. V., and Taniguchi, I. K. (2007). Application of recent red abalone Haliotis rufescens surveys to management decisions outlined in the California Abalone Recovery and Management Plan. J. Shellfish Res. 26, 713–717. doi: 10.2983/0730-8000(2007)26[713:AORRAH]2.0.CO;2
Keith, D. A. (2015). Assessing and managing risks to ecosystem biodiversity. Austr. Ecol. 40, 337–346. doi: 10.1111/aec.12249
Kirby, R. R., Beaugrand, G., and Lindley, J. A. (2009). Synergistic effects of climate and fishing in a marine ecosystem. Ecosystems 12, 548–561. doi: 10.1007/s10021-009-9241-9
Ling, S., Scheibling, R., Rassweiler, A., Johnson, C., Shears, N., Connell, S., et al. (2015). Global regime shift dynamics of catastrophic sea urchin overgrazing. Philos. Trans. Royal Soc. B Biol. Sci. 370, 20130269. doi: 10.1098/rstb.2013.0269
Marbà, N., and Duarte, C. M. (2010). Mediterranean warming triggers seagrass (Posidonia oceanica) shoot mortality. Global Change Biol. 16, 2366–2375. doi: 10.1111/j.1365-2486.2009.02130.x
May, R. M. (1977). Thresholds and breakpoints in ecosystems with a multiplicity of stable states. Nature 269, 471–477. doi: 10.1038/269471a0
McPherson, M. L., Finger, D. J., Houskeeper, H. F., Bell, T. W., Carr, M. H., Rogers-Bennett, L., et al. (2021). Large-scale shift in the structure of a kelp forest ecosystem co-occurs with an epizootic and marine heatwave. Commun. Biol. 4, 1–9. doi: 10.1038/s42003-021-01827-6
Meehl, G. A., and Tebaldi, C. (2004). More intense, more frequent, and longer lasting heat waves in the 21st century. Science 305, 994–997. doi: 10.1126/science.1098704
Miller, R. J., Lafferty, K. D., Lamy, T., Kui, L., Rassweiler, A., Reed, D. C., et al. (2018). Giant kelp, Macrocystis pyrifera, increases faunal diversity through physical engineering. Proc. R. Soc. B Biol. Sci. 285, 20172571. doi: 10.1098/rspb.2017.2571
Mills, K. E., Pershing, A. J., Brown, C. J., Chen, Y., Chiang, F.-S., Holland, D. S., et al. (2013). Fisheries management in a changing climate: lessons from the 2012 ocean heat wave in the Northwest Atlantic. Oceanography 26, 191–195. doi: 10.5670/oceanog.2013.27
Mitton, J. B., and Ferrenberg, S. M. (2012). Mountain pine beetle develops an unprecedented summer generation in response to climate warming. Am. Natur. 179, E163–E171. doi: 10.1086/665007
Moore, J. D., Robbins, T. T., and Friedman, C. S. (2000). Withering syndrome in farmed red abalone Haliotis rufescens: thermal induction and association with a gastrointestinal Rickettsiales-like prokaryote. J. Aquatic Anim. Health 12, 26–34. doi: 10.1577/1548-8667(2000)012<0026:WSIFRA>2.0.CO;2
Mumby, P. J., Harborne, A. R., Williams, J., Kappel, C. V., Brumbaugh, D. R., Micheli, F., et al. (2007). Trophic cascade facilitates coral recruitment in a marine reserve. Proc. Natl. Acad. Sci. 104, 8362–8367. doi: 10.1073/pnas.0702602104
Newbold, T., Tittensor, D. P., Harfoot, M. B. J., Scharlemann, J. P. W., and Purves, D. W. (2020). Non-linear changes in modelled terrestrial ecosystems subjected to perturbations. Sci. Rep. 10, 14051. doi: 10.1038/s41598-020-70960-9
O'Leary, C. A., Thorson, J. T., Ianelli, J. N., and Kotwicki, S. (2020). Adapting to climate-driven distribution shifts using model-based indices and age composition from multiple surveys in the walleye pollock (Gadus chalcogrammus) stock assessment. Fish. Oceanogr. 29, 541–557. doi: 10.1111/fog.12494
Oliver, E. C. (2019). Mean warming not variability drives marine heatwave trends. Clim. Dynam. 53, 1653–1659. doi: 10.1007/s00382-019-04707-2
Orth, R. J., Carruthers, T. J., Dennison, W. C., Duarte, C. M., Fourqurean, J. W., Heck, K. L., et al. (2006). A global crisis for seagrass ecosystems. Bioscience 56, 987–996. doi: 10.1641/0006-3568(2006)56[987:AGCFSE]2.0.CO;2
Parmesan, C., Root, T. L., and Willig, M. R. (2000). Impacts of extreme weather and climate on terrestrial biota. Bull. Am. Meteorol. Soc. 81, 443–450.
Perry, A. L., Low, P. J., Ellis, J. R., and Reynolds, J. D. (2005). Climate change and distribution shifts in marine fishes. Science 308, 1912–1915. doi: 10.1126/science.1111322
Pershing, A. J., Alexander, M. A., Hernandez, C. M., Kerr, L. A., Le Bris, A., Mills, K. E., et al. (2015). Slow adaptation in the face of rapid warming leads to collapse of the Gulf of Maine cod fishery. Science 350, 809–812. doi: 10.1126/science.aac9819
Pinsky, M. L., and Mantua, N. J. (2014). Emerging adaptation approaches for climate-ready fisheries management. Oceanography 27, 146–159. doi: 10.5670/oceanog.2014.93
Reid, J., Rogers-Bennett, L., Lavín, F., Pace, M., Catton, C., and Taniguchi, I. (2016). The economic value of the recreational red abalone fishery in northern California. California Fish Game. 102, 119–130.
Rocha, J. C., Peterson, G., Bodin, O., and Levin, S. (2018). Cascading regime shifts within and across scales. Science 362, 1379–1383. doi: 10.1126/science.aat7850
Rogers-Bennett, L., and Catton, C. A. (2019). Marine heat wave and multiple stressors tip bull kelp forest to sea urchin barrens. Sci. Rep. 9, 15050. doi: 10.1038/s41598-019-51114-y
Rogers-Bennett, L., Dondanville, R. F., Catton, C. A., Juhasz, C. I., Horii, T., Hamaguchi, M., et al. (2016). Tracking larval, newly settled, and juvenile red abalone (Haliotis rufescens) recruitment in Northern California. J. Shellfish Res. 35, 601–609. doi: 10.2983/035.035.0305
Rogers-Bennett, L., Dondanville, R. F., Moore, J. D., and Vilchis, L. I. (2010). Response of red abalone reproduction to warm water, starvation, and disease stressors: Implications of ocean warming. J. Shellf. Res. 29, 599–611. doi: 10.2983/035.029.0308
Rogers-Bennett, L., Kashiwada, J., Taniguchi, I., Kawana, S., and Catton, C. (2019). Using density-based fishery management strategies to respond to mass mortality events. J. Shellf. Res. 38, 485. doi: 10.2983/035.038.0232
Rogers-Bennett, L., Klamt, R., and Catton, C. A. (2021). Survivors of climate driven abalone mass mortality exhibit declines in health and reproduction following kelp forest collapse. Front. Marine Sci. 1071, 725134. doi: 10.3389/fmars.2021.725134
Rogers-Bennett, L., Kudela, R., Nielsen, K., Paquin, A., O'Kelley, C., Langlois, G. W., et al. (2012). Dinoflagellate bloom coincides with marine invertebrate mortalities in northern California. Harmful Algae News 46, 10–11. Available online at: https://citeseerx.ist.psu.edu/viewdoc/download?doi=10.1.1.354.54&rep=rep1&type=pdf
Rogers-Bennett, L., Rogers, D. W., and Schultz, S. A. (2007). Modeling growth and mortality of red abalone (Haliotis rufescens) in Northern California. J. Shellf. Res. 26, 719–727. doi: 10.2983/0730-8000(2007)26[719:MGAMOR]2.0.CO;2
Rogers-Bennett, L., Yang, G., and Mann, J. D. (2022). Using the Resist-Accept-Direct management framework to respond to climate-driven transformations in marine ecosystems. Fish. Manag. Ecol. 29, 409–422. doi: 10.1111/fme.12539
Sanford, E., Sones, J. L., García-Reyes, M., Goddard, J. H., and Largier, J. L. (2019). Widespread shifts in the coastal biota of northern California during the 2014–2016 marine heatwaves. Sci. Rep. 9, 1–14. doi: 10.1038/s41598-019-40784-3
Scheffer, M., Carpenter, S., Foley, J. A., Folke, C., and Walker, B. (2001). Catastrophic shifts in ecosystems. Nature 413, 591. doi: 10.1038/35098000
Schuurman, G. W., Cole, D. N., Cravens, A. E., Covington, S., Crausbay, S. D., Hoffman, C. H., et al. (2021). Navigating ecological transformation: resist–accept–direct as a path to a new resource management paradigm. Bioscience 72, 16–29. doi: 10.1093/biosci/biab067
Smale, D. A. (2020). Impacts of ocean warming on kelp forest ecosystems. New Phytol. 225, 1447–1454. doi: 10.1111/nph.16107
Smale, D. A., and Wernberg, T. (2013). Extreme climatic event drives range contraction of a habitat-forming species. Proc. Biol. Sci. 280, 20122829. doi: 10.1098/rspb.2012.2829
Smale, D. A., Wernberg, T., Oliver, E. C., Thomsen, M., Harvey, B. P., Straub, S. C., et al. (2019). Marine heatwaves threaten global biodiversity and the provision of ecosystem services. Nat. Clim. Change 9, 306–312. doi: 10.1038/s41558-019-0412-1
Stevenson, D. E., and Lauth, R. R. (2019). Bottom trawl surveys in the northern Bering Sea indicate recent shifts in the distribution of marine species. Polar Biol. 42, 407–421. doi: 10.1007/s00300-018-2431-1
Thompson, R., Crowe, T., and Hawkins, S. (2002). Rocky intertidal communities: past environmental changes, present status and predictions for the next 25 years. Environ. Conserv. 29, 168–191. doi: 10.1017/S0376892902000115
Thomsen, M. S., Mondardini, L., Alestra, T., Gerrity, S., Tait, L., South, P. M., et al. (2019). Local extinction of bull kelp (Durvillaea spp.) due to a marine heatwave. Front. Marine Sci. 6, 84. doi: 10.3389/fmars.2019.00084
Vilchis, L. I., Tegner, M. J., Moore, J. D., Friedman, C. S., Riser, K. L., Robbins, T. T., et al. (2005). Ocean warming effects on growth, reproduction, and survivorship of Southern California abalone. Ecol. Appl. 15, 469–480. doi: 10.1890/03-5326
Wernberg, T., Bennett, S., Babcock, R. C., de Bettignies, T., Cure, K., Depczynski, M., et al. (2016). Climate-driven regime shift of a temperate marine ecosystem. Science 353, 169–172. doi: 10.1126/science.aad8745
Wernberg, T., Smale, D. A., Tuya, F., Thomsen, M. S., Langlois, T. J., De Bettignies, T., et al. (2013). An extreme climatic event alters marine ecosystem structure in a global biodiversity hotspot. Nat. Climate Change 3, 78. doi: 10.1038/nclimate1627
Keywords: climate change, ecosystem shift, fisheries management, Haliotis spp., kelp deforestation, marine heatwave, conceptual framework
Citation: Rogers-Bennett L and Catton CA (2022) Cascading impacts of a climate-driven ecosystem transition intensifies population vulnerabilities and fishery collapse. Front. Clim. 4:908708. doi: 10.3389/fclim.2022.908708
Received: 30 March 2022; Accepted: 25 July 2022;
Published: 18 August 2022.
Edited by:
Thomas Wernberg, University of Western Australia, AustraliaReviewed by:
Keryn Gedan, George Washington University, United StatesRobert V. Rohli, Louisiana State University, United States
Jose M. Farina, Pontificia Universidad Católica de Chile, Chile
Copyright © 2022 Rogers-Bennett and Catton. This is an open-access article distributed under the terms of the Creative Commons Attribution License (CC BY). The use, distribution or reproduction in other forums is permitted, provided the original author(s) and the copyright owner(s) are credited and that the original publication in this journal is cited, in accordance with accepted academic practice. No use, distribution or reproduction is permitted which does not comply with these terms.
*Correspondence: Laura Rogers-Bennett, cm9nZXJzYmVubmV0dCYjeDAwMDQwO3VjZGF2aXMuZWR1