- 1School of Environmental Sciences, University of East Anglia, Norwich, United Kingdom
- 2Sorbonne University, CNRS, Laboratoire d'Océanographie de Villefranche, Villefranche-sur-Mer, France
- 3Institute for Sustainable Development and International Relations, Sciences Po, Paris, France
- 4OACIS, Prince Albert 2 of Monaco Foundation, Monaco, Monaco
Mangrove forests, seagrass meadows and tidal saltmarshes are vegetated coastal ecosystems that accumulate and store large quantities of carbon in their sediments. Many recent studies and reviews have favorably identified the potential for such coastal “blue carbon” ecosystems to provide a natural climate solution in two ways: by conservation, reducing the greenhouse gas emissions arising from the loss and degradation of such habitats, and by restoration, to increase carbon dioxide drawdown and its long-term storage. The focus here is on the latter, assessing the feasibility of achieving quantified and secure carbon removal (negative emissions) through the restoration of coastal vegetation. Seven issues that affect the reliability of carbon accounting for this approach are considered: high variability in carbon burial rates; errors in determining carbon burial rates; lateral carbon transport; fluxes of methane and nitrous oxide; carbonate formation and dissolution; vulnerability to future climate change; and vulnerability to non-climatic factors. Information on restoration costs is also reviewed, with the conclusion that costs are highly uncertain, with lower-range estimates unrealistic for wider application. CO2 removal using coastal blue carbon restoration therefore has questionable cost-effectiveness when considered only as a climate mitigation action, either for carbon-offsetting or for inclusion in Nationally Determined Contributions. Many important issues relating to the measurement of carbon fluxes and storage have yet to be resolved, affecting certification and resulting in potential over-crediting. The restoration of coastal blue carbon ecosystems is nevertheless highly advantageous for climate adaptation, coastal protection, food provision and biodiversity conservation. Such action can therefore be societally justified in very many circumstances, based on the multiple benefits that such habitats provide at the local scale.
Introduction
The overall desirability of large-scale protection and restoration of coastal vegetated coastal ecosystems (primarily mangrove forests, saltmarshes, and seagrass meadows) and their carbon-rich sediments is now well-accepted. Although their global role in carbon storage has only relatively recently been identified (Duarte et al., 2005; Laffoley and Grimsditch, 2009; Nellemann et al., 2009), such “blue carbon” ecosystems have subsequently attracted considerable scientific attention, with >1,000 papers on their biogeochemical and socio-economic importance (2009–2021; Web of Science, with “blue carbon” as search term). The many services provided by coastal blue carbon ecosystems (hereafter CBCEs) include contributions to climate mitigation and adaptation (McLeod et al., 2011; Alongi, 2018; Bindoff et al., 2019; Windham-Myers et al., 2019), thereby providing a nature-based solution that could contribute to the Paris Agreement and the Glasgow Climate Pact (UNFCCC, 2015, 2021). The potential of those contributions is considered sufficiently large to justify the substantive use of CBCEs in climate policy by many studies and reviews (e.g., Herr and Landis, 2016; Crooks et al., 2018; Hoegh-Guldberg et al., 2019; Seddon et al., 2020; UNESCO, 2020; Hilmi et al., 2021; Macreadie et al., 2021; UNEP and IUCN, 2021), with the associated concepts of blue carbon farming (Duarte de Paula Costa et al., 2022) and blue carbon markets (Claes et al., 2022). The further development of such approaches has been prioritized for US research on CO2 removal (NASEM, 2019).
Until recently, coastal vegetation management has not been separately considered as a mitigation lever in IPCC climate assessments, being subsumed within afforestation and reforestation in Integrated Assessment Models (IPCC, 2018). It has also been omitted from several comparative assessments of negative emissions (e.g., Minx et al., 2018; GESAMP, 2019), or grouped with freshwater wetland and peatland restoration (McLaren, 2012; Royal Society Royal Academy of Engineering, 2018), or considered as a soil or land-based mitigation technique (Bossio et al., 2020; Roe et al., 2021). The IPCC AR6 cycle does, however, specifically discuss CBCEs in all three Working Group reports, with WG II coverage emphasizing their co-benefits (Parmesan et al., 2022) and vulnerability to climate change and direct anthropogenic impacts (Cooley et al., 2022). The AR6 WG III report considers “blue carbon management in coastal wetlands” and “peatland and coastal wetland restoration” as separate approaches in a NET summary table, assessing their status and role in mitigation pathways. However, costs (USD tCO removed) are not given in the table for these approaches, due to “insufficient data” (Babiker et al., 2022). In other syntheses, the restoration of coastal vegetation has been considered to have low effectiveness in reducing global warming and its impacts, providing a “low regret” or “no regrets” mitigation action (Gattuso et al., 2018, 2021; Bindoff et al., 2019).
To resolve the varying opinions on the usefulness of CBCEs in meeting climate policy goals, there is recognized need for “robust scientific evidence along with accountability of the contribution [of blue carbon options] to greenhouse gas mitigation, including meeting the requirements of additionality and permanence of this benefit” (Macreadie et al., 2021). Here we review the issues (primarily biogeochemical) affecting the reliability of carbon accounting for CBCE restoration, considered broadly, and the implications of these constraints, uncertainties and risks in determining climatic benefits—and hence the cost-effectiveness of this approach for CO2 removal, acknowledging that there are many non-climatic benefits. The scope for emission avoidance, by protection of coastal blue carbon stores, is not assessed (notwithstanding its importance), nor is any evaluation made of the considerable benefits that CBCE restoration can undoubtedly provide through improved climate adaptation (e.g., coastal protection) and through other ecosystem services (e.g., fisheries and biodiversity conservation). Comprehensive valuation estimates for the very many ecosystem services provided by CBCEs are given elsewhere (e.g., Vegh et al., 2019; Laffoley, 2020), with the wider socio-economic challenges associated with CBCE restoration reviewed by Thomas (2014), Abelson et al. (2020), and Macreadie et al. (2022).
Long-term carbon accumulation and storage primarily occurs in the sediment of CBCEs, rather than their above-ground biomass (that, once established, is not expected to significantly increase). CBCE restoration can involve actions of three kinds, each potentially providing negative emissions through increased carbon burial fluxes and storage. First, by resource management to improve local environmental conditions and promote natural functioning. For example, by restoring natural hydrodynamics to increase freshwater flows, tidal exchanges and sediment supply; by reducing pollution, particularly by nutrients; and by reinstating natural predatory control of bioturbators (Kroeger et al., 2017; Macreadie et al., 2017a). Second, by re-establishing such habitats where they had previously been lost as a result of land-use change or other coastal developments. This action involves planting seagrass or mangrove seedlings in subtidal or intertidal sediments; many such initiatives have already been carried out (van Katwijk et al., 2016; López-Portillo et al., 2017). Third, by the creation of entirely new habitats, such as purposeful coastal flooding to stimulate saltmarsh formation (Adam, 2019). This is not strictly a “restoration” process, but has been widely considered as such in terms of policy action.
In all cases, it is the combination of sustained photosynthesis and long term carbon storage that provides the climatic benefits of CBCE restoration. The aerial vegetation of mangroves and saltmarshes removes CO2 directly from the atmosphere; for continually-submerged seagrasses, the mitigation effect is indirect, mediated through seawater CO2 uptake (subsequently affecting air-sea CO2 fluxes).
Accounting Issues
For CBCE restoration to provide net carbon removal (negative emissions) that are valid contributions to national climate strategies (Herr and Landis, 2016; Hilmi et al., 2021) and/or used for carbon trading purposes (Ullman et al., 2013; Vanderklift et al., 2019), it is necessary for there to be additional carbon uptake and storage by such systems that is the unequivocal consequence of management action. It is also necessary for the expected magnitude of that climatic benefit to be forecast with reasonable confidence (preferably ± 10%), and for those benefits to be verifiable. Thus the projected extra CO2 removal from the atmosphere has to be initially estimated, together with any other associated climatic effects (e.g., changes in fluxes of non-CO2 greenhouse gases), and then reliably determined according to internationally-agreed standards (IPCC, 2014; Needelman et al., 2019; Eger et al., 2022). Furthermore, the additional carbon sequestered should be securely stored, and therefore monitored, for many decades, preferably “permanently” (CEC, 2014; Brander et al., 2021). From a climatic perspective, permanence is generally considered as >100 years (Fearnside, 2002). Whilst shorter storage may help meet near-term policy targets (Ruseva et al., 2020), it does not contribute to the UNFCCC goal of long-term climate stabilization and may actually be counter-productive (Kirschbaum, 2006).
These requirements are extremely challenging for CBCE restoration. To provide confidence that they are achievable, a Verified Carbon Standard (VCS) for Tidal Wetlands and Seagrass Restoration has been developed (Needelman et al., 2018; Verra, 2021), primarily in a US context. The VCS methodology is complex and technically demanding, with >30 parameters involved. To facilitate its operational application, a range of alternative sources can be used to obtain many of the basic datasets: proxies, published values, default factors, models, field measurements and historical (chrono-sequenced) data (Verra, 2021). These alternative data sources have different qualities and involve different assumptions; as a result, the intended standardization would seem compromised. The most reliable estimates for carbon removal are those directly derived from in situ measurements, ideally including comprehensive baseline data collected up to 4 years before the start of restoration (Verra, 2021). It may then take a further 10–20 years after the start of the restoration project before its carbon burial rates match those of adjacent mature ecosystems (Carnell et al., 2022), and the magnitude of sustained removal of additional carbon can then be determined.
The main unresolved uncertainties and risks that jeopardize the reliability of carbon accounting (and hence the associated climatic benefits of CBCE restoration) are discussed below. Although these issues are presented separately, there are many potential interactions between them. For example, much of the variability in reported carbon burial rates seems likely to be a consequence of the variability in sedimentation rates and the relative importance of bioturbation effects and of microbial decomposition. Similarly, the rate at which recalcitrant carbon increases in deeper sediments is a function of the composition (and decomposition) of recently-deposited organic carbon.
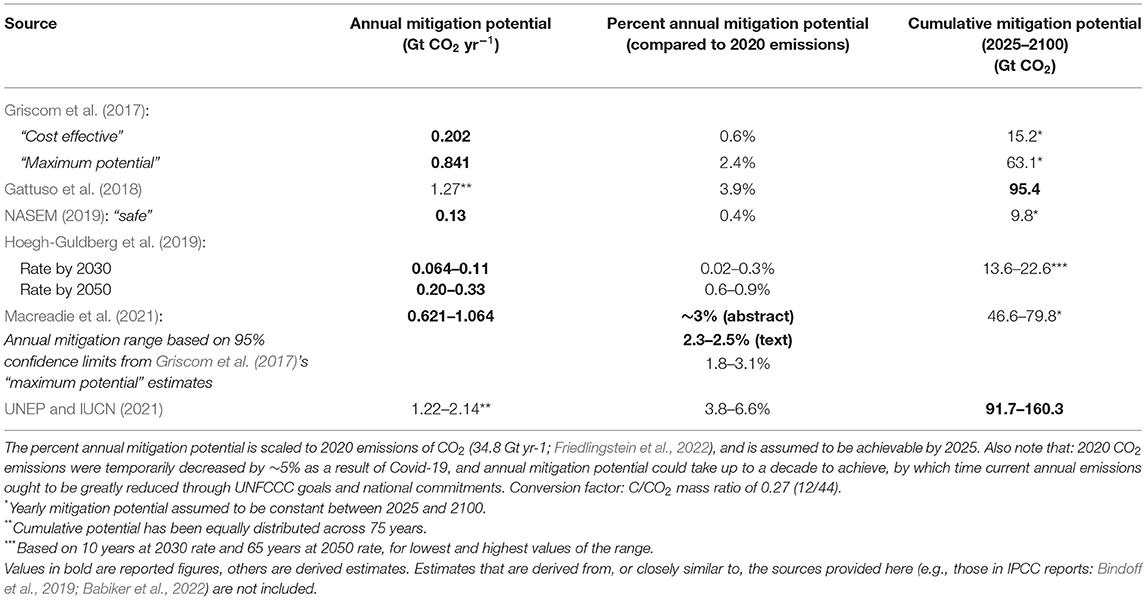
Table 1. Global mitigation potential estimates for CBCE restoration (excluding emission reductions through conservation).
High Variability in Carbon Burial Rates
Multiple biological, chemical and physical factors strongly influence CBCE carbon burial rates through their interacting effects on primary production, sedimentation, decomposition and preservation (McLeod et al., 2011; Macreadie et al., 2019; NASEM, 2019). As a result, there is very high variability in site-specific estimates of natural carbon burial rates: for saltmarshes, there is a 600-fold range between lowest and highest reported burial rates (7.7–4,693 mg C m−2 day−1); for seagrasses, the range between lowest and highest is 76-fold (6.9–521 mg C m−2 day−1), and for mangroves, 19-fold (155–2,940 mg C m−2 day−1) (Rosentreter et al., 2021, updated to include Hatje et al., 2021). Ranges rather than means and their confidence limits are given here because of unresolved statistical problems relating to data distributions; see Box 1.
Box 1. Low confidence in blue carbon confidence limits?
The literature review by McLeod et al. (2011) calculated global mean carbon burial rates and their standard errors to be 226 ± 39 gC m−2 yr−1 for mangroves, 218 ± 24 gC m−2 yr−1 for saltmarshes and 138 ± 38 for seagrasses, based on 34, 96 and 123 sites respectively. This study has been highly influential (~2,400 citations) and was used by Griscom et al. (2017) for their coastal wetland synthesis, including associated confidence limits. Breithaupt's et al. (2012) used a closely similar primary dataset for mangrove carbon burial, and found a closely similar arithmetic mean: 231 gC m−2 yr−1. However, their uncertainty (standard error) estimate was considerably higher, at ± 209, as a result of high skewness (a long right tail of extreme values) in the dataset; i.e., a non-normal distribution. When recalculated from log-transformed values, Breithaupt et al.'s geometric mean for carbon burial was ~30% lower, at 163 gC m−2 yr−1, with 95% confidence range of 131–203 gC m−2 yr−1.
The frequency distribution of Breithaupt's et al. (2012) dataset and its basic statistics are shown in Figure 1. Whilst we are unaware of equivalent statistical analyses for saltmarsh and seagrass data, or of more up-to-date compilations for mangroves, the problem of non-normal distributions in carbon burial rates was recognized relatively early (Duarte et al., 2005) and seems deep-seated, strongly favoring the use of geometric means or medians to determine the central tendency. This data skewness may genuinely reflect the global reality; however, the occurrence of “anomalous” high values could also arise from unrepresentative geographical sampling (Breithaupt's et al., 2012), and/or from methodological errors, potentially of more than an order of magnitude (Johannessen and Macdonald, 2016). Such issues contribute to the high variability, and possible overestimation, of the global mitigation potential of CBCE restoration (see Table 1), frequently calculated using the arithmetic mean values of McLeod et al. (2011).
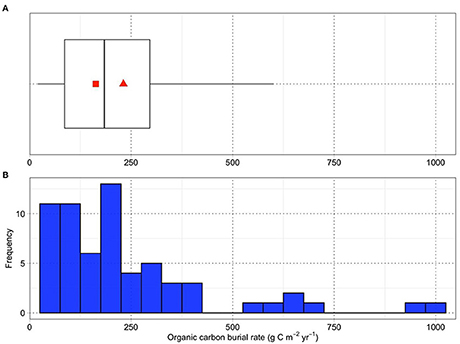
Figure 1. (A) Box-and-whisker plot of 65 reported estimates of the burial rates of organic carbon in mangroves compiled by Breithaupt's et al. (2012). The box represents the middle 50% of the data, between the 1st and 3rd quartiles. The median, represented by the vertical line across the box, is the middle value of the data set; half the values are greater than the median and half are less. The whiskers represent the “minimum” and “maximum” values, based on the inter-quartile range and excluding reported data considered as outliers. The red triangle and square represent the arithmetic and geometric means respectively, for all the data. (B) Histogram of the frequency distribution summarized in (A).
Much effort is currently being made to account for this high variability in carbon burial through process-based research and multi-factorial modeling (e.g., Morris and Callaway, 2019; Kim et al., 2022), with increasing focus on rates and time-scales rather than standing stocks—as discussed in greater detail below. However, comprehensive understanding of its causality has yet to be achieved, and progress on this front is likely to require the collection of much more site-specific environmental data, with significant cost implications for CBCE restoration projects. Until then, mean or median values derived from the global literature provide a highly uncertain default estimate for the projected outcome of any new restoration initiative. Whilst national or regional data would seem inherently more acceptable (by limiting the influence of large-scale environmental and biogeographic factors), local variability in carbon burial rates can also be very high. For example, Hatje et al. (2021) found that the variability in measured burial rates for six intertidal transects of mangrove forest in a single Brazilian estuary was as great as the global range.
Errors in Determining Carbon Burial Rates
Most estimates of carbon burial rates in CBCEs to date have been indirect, based on the sediment carbon inventory (soil carbon stock) in near-surface layers, typically the top 1 m, rather than using direct flux measurements. However, inventory-based approaches can result in order-of-magnitude errors in carbon burial rates (Johannessen and Macdonald, 2016), unless there is also reliable information on sediment accumulation rates, derived directly or using isotopic tracers (Arias-Ortiz et al., 2018a; Callaway, 2019; Jennerjahn, 2020). Such accretion rates may have changed markedly in recent decades due to anthropogenic and climatic influences on local water flows and sediment delivery (Ladd et al., 2019). In estuaries, these parameters will be strongly influenced by upstream land-use and water quality changes at catchment-wide scale. Since restoration projects are almost always located at sites that have been subject to substantive anthropogenic disturbance, interpretation of their sediment core data (and also from nearby “control” sites) requires particular care.
Bioturbation (sediment mixing by burrowing fauna) can significantly compromise tracer-based measurement of sediment accumulation, and hence carbon burial rates, resulting in the linked over-estimation of these parameters (Silverberg et al., 1986). Microbial decomposition of organic carbon can also continue, albeit slowly, in more recently-deposited sediment layers. In seagrass sediments, both these processes can occur to ~40 cm depth. If not measured or otherwise allowed for (Gardner et al., 1987), each effect can result in an over-estimation of carbon burial rates by 50–100% (Johannessen and Macdonald, 2016).
When restoration is carried out, initial measurements of organic carbon in sediment profiles will primarily provide information on carbon deposition, rather than long-term carbon burial. This effect is similar to the achievement of steady state conditions for above-ground biomass; both processes may take several years, if not decades, to (re-)establish the sustained carbon dynamics of the mature system. The time-dependence of such processes should, ideally, be determined on a site-specific basis (Carnell et al., 2022).
Lateral Carbon Transport
For CBCE-based climate mitigation, it is necessary to know the source of the carbon buried in coastal sediments, with subsequent exclusion of most, if not all, of the allochthonous (non-local) carbon originating from terrestrial or atmospheric sources, or other marine ecosystems. That is because the CBCE did not remove allochthonous carbon from the atmosphere (neither directly nor indirectly), and its long-term storage may have occurred anyway. The contributions of different organic materials to long-term carbon burial in a CBCE restoration site can be estimated by identifying their taxonomic origins using “fingerprinting” techniques (Geraldi et al., 2019), thereby determining the proportion of the total that can be ascribed to the restoration action. The criteria for inclusion can be wider than just material arising from the dominant, habitat-structuring species; for example, benthic microalgae can be considered as part of a mangrove ecosystem, and therefore their contribution to carbon storage is autochthonous rather than allochthonous. Even for non-local carbon, full exclusion may not be necessary if it can be shown that its decomposition has been significantly slowed or prevented by the anoxic conditions of CBCE sediments. However, this factor introduces additional site-specific uncertainties that cannot easily be resolved.
The proportion of carbon buried in CBCE sediments that is allochthonous is highly variable. For mangroves, allochthonous carbon has been estimated as 24–55% of the total for a range of mangrove sites in Vietnam (Hieu et al., 2017), 3–73% in China (Xiong et al., 2018), and 59–79% in Ecuador (Suello et al., 2022). For seagrasses, the allochthonous contribution to carbon burial can be as high as 70–90% in Australian estuaries (Ricart et al., 2020). For saltmarshes, allochthonous sources may also dominate (Saintilan et al., 2013; Van de Broek et al., 2018), although again with high variability. For example, the relatively low proportions of 12 and 39% were estimated for two saltmarsh sites in Germany (Mueller et al., 2019), and of 11–27% in undisturbed and 58–68% in restored US saltmarsh sites (Drexler et al., 2020).
Recalcitrant black carbon, including soot from burning biomass or fossil fuels, can be an important component of allochthonous carbon, comprising 9–25% of total carbon burial in a range of deltaic CBCEs in China (Li et al., 2021) and up to 43% in sandy seagrass environments in Australia (Chew and Gallagher, 2018).
Most of the above values are depth-averaged, including near-surface layers. Such measurements are, however, likely to underestimate the contribution of allochthonous carbon to long-term carbon storage (and hence further overestimate the climatic effectiveness of CBCE restoration). That is because the locally-derived organic carbon can be expected to be fresher and more labile, decomposing more rapidly than material from elsewhere, likely to be mostly land-derived (at least in estuaries) and either recalcitrant or already partly re-mineralized (Van de Broek et al., 2018). Thus a typical 50:50 ratio of allochthonous to autochthonous carbon in near-surface saltmarsh deposits may change to a 80:20 ratio for more deeply-buried buried carbon (Leorri et al., 2018; Van de Broek et al., 2018). Similar effects can be expected for mangrove and seagrass sediments, but have not to our knowledge been investigated. The rate at which allochthonous carbon proportionally increases with sediment depth can be expected to vary between sites, since it will affected by site-specific sedimentation rates and bioturbation processes.
A counteracting consequence of lateral carbon transport (that would result in an underestimation of climatic benefits) is that there is also likely to be significant carbon export from CBCEs, a proportion of which may be subject to long-term storage, either as dissolved inorganic or organic carbon in deep ocean water, or as particulates that are buried in other depositional systems. The scale of these export processes may be as great as, or exceed, direct carbon burial (Maher et al., 2018; Santos et al., 2019, 2021). However, export cannot be directly equated with long-term sequestration, and the decomposition rates and fate (on decadal time-scales) of carbon transported from the coast to very large areas of the open ocean is poorly constrained, spatially heterogeneous and difficult to quantify (Legge et al., 2020). Furthermore, it is relevant to note that the initial identification of the importance of CBCEs in the global carbon cycle was based on the estimate that around 50% of carbon burial in the global ocean occurred in their sediments (Duarte et al., 2005).
Cost considerations mean that it is very unlikely that the scale of the potential benefits arising from carbon export and subsequent long-term sequestration from restored CBCEs could be reliably determined through their routine monitoring.
Fluxes of Methane and Nitrous Oxide
The anaerobic conditions in CBCE sediments that are responsible for long-term carbon storage also favor the production and emissions of two potent greenhouse gases of increasing climatic concern: methane (CH4) and nitrous oxide (N2O; Rosentreter et al., 2021). Contrary to previous indications (Poffenbarger et al., 2011) and assumptions (IPCC, 2014), saline conditions do not fully inhibit CH4 production in coastal sediments (Conrad, 2020; Marchand et al., 2022). Such emissions can show high temporal variability (Roth et al., 2022); furthermore, biogenic structures such as bioturbation burrows can enhance CH4 fluxes by an order of magnitude (Kristensen et al., 2022), and CH4 can be released directly to the air from plant stems (Zhang et al., 2022).
Total global CH4 emissions from CBCEs have been estimated at >5 million tons yr−1 (Al-Haj and Fulweiler, 2020). This total has the potential to fully counteract the climatic benefits of carbon burial by these ecosystems. However, statistical methods affect these estimates and comparisons, with lower global values for CH4 emissions obtained when based on the median, rather than the arithmetic mean, of site-specific studies (Rosentreter and Williamson, 2020). As discussed in Box 1, the arithmetic mean is best avoided for highly skewed distributions.
Further methodological uncertainties relate to the timescales and assumptions used to estimate the global warming potential (GWP) of CH4, for comparison of its warming effects to those of CO2. Because of the relatively short atmospheric lifetime of CH4, higher GWP values apply when considering medium-term mitigation targets (e.g., Nationally Determined Contributions by 2030, or net zero by 2050) rather than global radiative flux budgets in 2100. For long-established natural CBCEs, their CH4 emissions (even if high) can have neutral radiative balance, since such emissions are counteracted by steady-state atmospheric removal processes, i.e., they do not result in any further atmospheric accumulation (Neubauer and Verhoeven, 2019).
Key issues for CBCE restoration is therefore whether that action increases CH4 and N2O emissions (likely from new habitat formation, e.g., saltmarsh creation) or decreases them, and the magnitude of those changes. Decreases could occur when pre-restoration conditions are strongly methanogenic, e.g., rice paddies, shrimp ponds or wet pastures (Chauhan et al., 2017; Yang et al., 2017; Iram et al., 2021) or might be achieved if restoration involves increasing water flows to existing habitat (Kroeger et at., 2017). Nevertheless, it is invalid to assume that restoration will necessarily reduce CH4 emissions, or to assume there will be no effect, since there is also potential for increased CH4 emissions to significantly offset future climate benefits (Rosentreter et al., 2021).
Emissions of N2O from CBCEs seem climatically less important than CH4, and uptake can also occur as a result of denitrification when nitrate availability is low (Foster and Fulweiler, 2016). However, the net global N2O efflux from CBCEs is not well-constrained (Wilson et al., 2020; Rosentreter et al., 2021), with high spatial and temporal variability resulting in a 40-fold range between upper and lower quartiles for estimated global values for the three ecosystems combined (Murray et al., 2015). Although N2O and CH4 have similar radiative forcing effects on a weight-for-weight basis, N2O has a much longer atmospheric lifetime, resulting in a 100 year GWP (for a pulse emission, with feedbacks) around 9 times higher than CH4, and nearly 300 times higher than CO2 (Myhre et al., 2013). As a result, relatively small changes in N2O emissions can significantly affect the climatic benefits of CBCE restoration.
Long-term site-specific monitoring of both CH4 and N2O fluxes would therefore seem necessary for CBCE restoration for climate mitigation purposes, with sufficient baseline data to determine the changes arising from restoration. Site-specific knowledge of previous land-use changes (i.e., when the historical habitat degradation or loss occurred) is also relevant to CH4 emissions, since associated “switchover times” (Neubauer and Verhoeven, 2019) affect the scale of net radiative warming or cooling prior to the restoration, and hence the magnitude and direction of the change arising from management action. Proxy measurements (salinity, hydrology, and plant community composition) have been proposed as alternatives to direct measurements of CH4 and N2O fluxes at restoration sites to reduce costs (Derby et al., 2022); their reliability and wider effectiveness have yet to be demonstrated.
Carbonate Formation and Dissolution
Calcium carbonate (CaCO3) precipitation (including the biological process of calcification by corals, many other benthic invertebrates and coralline algae) releases CO2, whilst its dissolution has the opposite effect. Both these processes can occur in CBCEs (Macreadie et al., 2017b; Howard et al., 2018; Kennedy et al., 2019), and there is ongoing debate regarding the overall direction and magnitude (and hence climatic consequences) of such effects.
Uncertainties are greatest for mangrove and seagrass habitats that accumulate relatively high levels of biogenic CaCO3 in their sediments. If the initial stage of calcium carbonate formation occurred centuries earlier or took place elsewhere, e.g., adjacent coral reefs, the CO2 emissions associated with calcification cannot be ascribed to the CBCE, whether natural or restored. Under those circumstances, calcium carbonate dissolution occurring within CBCE sediments has the potential to significantly enhance their climate mitigation role (Saderne et al., 2019). In Red Sea mangroves with relatively low organic carbon burial rates, such mitigation enhancement is estimated to be as much as 23-fold (Saderne et al., 2021). The opposite effect has been reported for one of the largest seagrass-dominated ecosystems in the world, in Florida Bay, USA. At that site, local calcification apparently exceeds dissolution; as a result, CO2 emissions are estimated to be around three times >CO2 removal through the burial of organic carbon (Van Dam et al., 2021). This unexpected outcome was shown by an atmospheric eddy covariance technique that directly measures air-water CO2 exchanges; it contradicts earlier evidence for net carbonate dissolution, based on water sampling and benthic chambers, with less extensive spatial and temporal coverage (Van Dam et al., 2019). However, there may be a contribution to CO2 emissions from high-CO2 groundwater discharge, that has been shown to be important elsewhere (Sadat-Noori et al., 2016).
Whilst both the Red Sea and Florida sites may be considered untypical, for different reasons, they show that the calcium carbonate dynamics can potentially override the climatic role of organic carbon burial in CBCEs. Such effects have been neglected to date by standard blue carbon accounting, such as the VCS methodology (Verra, 2021). There is now urgent need to determine their wider applicability and implications for CBCE restoration.
Vulnerability to Future Climate Change
The effects on CBCEs of future warming (including marine heatwaves), sea level rise, increased storminess and ocean acidification have been assessed by IPCC (Bindoff et al., 2019; Cooley et al., 2022) and elsewhere (Macreadie et al., 2019; Lovelock and Reef, 2020; Williamson and Guinder, 2021). Overall effects are considered to be damaging, threatening the continued viability of such ecosystems (Bindoff et al., 2019). Nevertheless, Macreadie et al. (2019) identified 11 potentially positive climate change effects for mangrove, saltmarsh and seagrass ecosystems considered separately, in addition to 11 potentially negative effects and 11 effects where the outcome was uncertain. The main factor that could result in beneficial effects is landward range expansion, with a potential increase of 1.5 GtC in net global carbon storage by 2100 (Lovelock and Reef, 2020) for the high emissions scenario, RCP 8.5. If such landward movement is not possible, losses of 3.4 GtC by 2100 were estimated as the worst-case scenario.
Seagrasses are considered the CBCE that is most sensitive to higher temperatures (Nguyen et al., 2021), particularly episodic extremes: their ecological structure and functioning are already subject to moderate impacts, attributed to warming with medium confidence (Bindoff et al., 2019). High impacts for seagrasses are projected if global mean surface temperatures increase by > ~2.3°C relative to pre-industrial, likely to involve the loss of their carbon stores (Arias-Ortiz et al., 2018b; Chefaoui et al., 2018). For saltmarshes, the thresholds for moderate and high impacts are estimated to be global temperature increases of ~1.2 and 3.1°C, respectively; for mangroves, ~2.0 and 3.7°C (Bindoff et al., 2019). Although mangroves therefore seem relatively resilient, severe and widespread mortalities have already occurred in Australia, linked to drought conditions, unprecedented high temperatures and a temporary drop in sea level (Duke et al., 2017).
The possibility of range expansion provides a counteracting effect, with expansion of mangroves into saltmarsh habitat already occurring on several continents (Saintilan et al., 2014; Cavanaugh et al., 2019). For CBCE restoration sites, the consequences of climate-driven species replacements are difficult to predict. Whilst they might be either increase or decrease carbon burial rates, the latter would seem inherently more likely. Future warming impacts for restored CBCEs could however be minimized, although not completely averted, if restoration effort is focused on the higher latitude (i.e., cooler) parts of the distributional ranges of the species that structure such ecosystems.
The implications of future changes in sea level rise, storm events, wave energy, and sea level rise for CBCEs are not well-understood, and are likely to show greater local and regional variability. Thus, vulnerability—and resilience—will also be affected by sediment erodibility and sediment resupply, as well as by vegetation type, the frequency of extreme events, the rate of local sea level rise, and whether there is space for landward relocation (Schuerch et al., 2018; Valiela et al., 2018; Rogers et al., 2019; Hanley et al., 2020). The possibility of landward migration would seem inapplicable to most CBCE restoration projects, unless land suitable for such re-location is either initially included or can be added later; either option would have significant cost implications.
Vulnerability to Non-climatic Factors
Assuming (optimistically) that climate change impacts can be minimized by limiting global warming to ~1.5°C, then would still be many non-climatic factors that might adversely affect the viability and long-term survival of restored CBCEs. The success of such restoration projects has been variable to date (Cunha et al., 2012; van Katwijk et al., 2016; Zhao et al., 2016; Kodikara et al., 2017; Duarte et al., 2020). A key issue is whether the (human) factors causing original loss/degradation or land use conversion have been properly addressed. The concept of social-ecological restoration (Abelson et al., 2020) is applicable here, taking account of all relevant anthropogenic drivers and decision-making processes, from local to international level. These include “opportunity costs” (not usually included in the restoration costs discussed below); i.e., the potential economic benefits that are lost when CBCE restoration excludes other uses of the coastal land, for agriculture, aquaculture, industry, or settlement (Stewart et al., 2003; Herr et al., 2019; Zeng et al., 2020).
Other causes for CBCE restoration failures include poor site selection or poor choice of species introduced, particularly for saltmarshes (Konisky and Burdick, 2004; Berck and Gustafson, 2012). Larger-scale plantings, natural regeneration, and strong local stakeholder engagement all increase the likelihood of long-term survival for the restored system (Bayraktarov et al., 2016; van Katwijk et al., 2016). Additional “lessons learnt” from greater implementation of CBCE restoration projects can be expected to increase their future success (Wylie et al., 2016). There is also evidence for natural recovery of CBCEs due to reduced anthropogenic pressures, at least at the local and regional scale (Almeida et al., 2014; Jia et al., 2018; de los Santos et al., 2019; Duarte et al., 2020). Nevertheless, adequate resourcing for long-term monitoring and strong protection of restored CBCE habitats will be needed, to ensure that they function as intended, for long enough to deliver their expected climate mitigation benefits.
Climatic Cost-Effectiveness and Scalability
The cost-effectiveness of using CBCEs for climate mitigation through carbon removal is considered here in terms of US$ per ton of CO2e removed from the atmosphere as a result of restoration action carried out for climate mitigation purposes, either as an integral part of national climate policy (Gallo et al., 2017; Kelleway et al., 2020) or primarily for carbon trading purposes (Vanderklift et al., 2019; Sapkota and White, 2020). The cost-effectiveness for CBCE restoration can also be determined more holistically, based on both climatic and non-climatic benefits; that wider context is briefly considered in Discussion and Conclusions below. Whichever approach is taken, many uncertainties affect the determination of cost-effectiveness, limiting the usefulness of CBCE restoration as a reliable and significant component of climate policy.
These uncertainties not only relate to the accounting issues discussed above, but also due to the very high variability in restoration costs, both between and within the three main vegetation types. Based on 91 studies of CBCE restoration (environmental improvements, re-planting and new habitat creation, as considered here), Bayraktarov et al. (2016) estimated that median total restoration costs for mangroves were US$ 2,500 ha−1; for saltmarsh, US$ 151,100 ha−1 and seagrasses, US$ 383,700 ha−1 (2010 prices). The wide range of costs within each CBCE is shown by their arithmetic mean values being 2–7 times higher than their medians, at US$ 15,000 ha−1, US$ 1,042,100 ha−1, and US$ 699,500 ha−1, respectively. Causes for cost variability included: whether or not monitoring costs were included; the differences in labor costs between developed and developing countries (responsible for a ~30-fold difference in median values for mangroves); and the planting method (responsible for an 8-fold difference for saltmarshes).
Taillardat et al. (2020) combined data on CBCE restoration costs (expressed annually) with estimated CO2 removal and CH4 emission rates: they estimated global median costs of US$ 469,100 t−1 CO2e for climate mitigation using saltmarsh restoration and US$ 560 t−1 CO2e for mangrove restoration. There was insufficient data to estimate equivalent values for seagrasses. Very large differences in median costs were confirmed for mangrove restoration between developing and developed nations (at US$ 990 ha−1 yr−1 and US$ 100,860 ha−1 yr−1, respectively); global cost estimates should therefore not be used as site-specific default values.
Griscom et al. (2017) applied a different approach for their cost-effectiveness analysis, using a Marginal Abatement Cost (MAC) curve to estimate that 24% of lost or degraded coastal wetlands could be restored at a target cost of less than US$ 100 t−1 CO2 (quoted as CO2e to indicate C to CO2 conversion, but without allowing for CH4 emissions). Their Supplementary Data showed that this proportion was based on 30% of “maximum restoration with safeguards” for the area of lost mangrove habitat and 60% of the area of lost saltmarsh, but no seagrass restoration (since the MAC-assessed cost of seagrass restoration was unable to meet the < US$ 100 criterion). There could, however, be bias in Griscom et al.'s estimates, that are based on the reported cost range of CBCE restoration projects to date. These initiatives are highly likely to have favored relatively straightforward and successful situations (Bayraktarov et al., 2016), thus their costs are likely to be unrepresentative (and lower) than average for the total restorable area where CBCE losses have occurred. The “safeguards” used by Griscom et al. (2017) did not seem to address this issue: their exclusions for cropland and timber production are generally inapplicable to CBCEs, where habitat losses are frequently due to mariculture, major hydrological changes, pollution, the construction of hard sea defenses and coastal development related to tourism, port infrastructure and human settlement. For most coastal development, restoration costs are prohibitively high; as a result, such data are not included in the reported cost range.
In South East Asia, the region often considered as a coastal blue carbon “hotspot” with high restoration potential for mangroves (Thorhaug et al., 2020), a combination of financial, land-use and operational factors were estimated to limit reforestation (of all kinds) to 0.3–18% of the maximum theoretically possible (Zeng et al., 2020). Macreadie et al. (2021) recognized this scalability problem: “The scope for global-scale coastal wetland restoration is constrained by multiple socio-economic considerations. This constraint is especially important in countries where a large proportion of the restorable habitat is on small agricultural land holdings, where restoration efforts could conflict with livelihoods and food security of local communities.” Nevertheless, Macreadie et al. (2021) used Griscom et al.'s (2017) values for maximum restoration, without any cost considerations, as the basis for their estimates of the potential climatic benefits of CBCE restoration (see Table 1).
Discussion and Conclusion
All IPCC pathways that limit global warming to 1.5°C with limited or no overshoot use negative emissions with a cumulative total of 100–1,000 Gt CO2 over the twenty-first century (IPCC, 2018). Coastal and ocean-based CO2 removal has attracted attention because there are multiple feasibility and sustainability constraints for land-based mitigation (Williamson, 2016), and the ocean has a very large potential for carbon uptake and storage due to its huge surface area and volume as well as distinct chemical characteristics.
Nature based solutions involving marine processes, such as CBCE restoration, are attractive not only for climate mitigation but also in the context of their other benefits, that include improved food security, reduced coastal erosion, and rebuilding marine biodiversity. They also enjoy stronger public support than ocean-based technological measures regarded as geoengineering (Bertram and Merk, 2020), although the natural/artificial distinction is arguably over-simplistic (Osaka et al., 2021). Table 1 gives estimates by different studies of the potential climatic effectiveness of CBCE restoration, considered as the additional CO2 that might be removed and stored per year with a ramp-up time of a few years. The range of these estimates is large, from 0.06 to 2.1 Gt CO2 per year, equivalent to 0.02 to 6.6% of 2020 CO2 emissions (Friedlingstein et al., 2022), reflecting the key uncertainties reviewed above.
Several of the effectiveness estimates are upper theoretical limits that are unrealistic for policy planning and implementation. For example, the relatively high estimate of Gattuso et al. (2018) assumes that historic losses of blue carbon ecosystems (Waycott et al., 2009; McLeod et al., 2011; estimated as 50% for mangroves since the 1940's, 29% for seagrass since 1879, 25% for saltmarsh since the 1800's) can all be restored or compensated for elsewhere, and uses arithmetic mean values for global carbon burial rates (McLeod et al., 2011). The former assumption is not feasible, since a large fraction of surface area of lost CBCEs, particularly mangrove and saltmarsh habitats, has been developed in ways that would make restoration prohibitively expensive and/or societally unacceptable (e.g., for settlement, port facilities or tourism infrastructure). Furthermore, as discussed above, carbon burial rates seem likely to be overestimated; fluxes of other greenhouse gases and carbonate dynamics are not taken into account; and the long-term security of storage may be jeopardized by future climate change or direct anthropogenic impacts.
Table 2 summarizes the specific uncertainties discussed in this paper that affect carbon accounting and wider effectiveness of using CBCE restoration for climate mitigation. On the basis of the groupings given, most effects are directional: six decrease and two increase the potential for CBCE-based climate mitigation, whilst three could have effects in either direction.
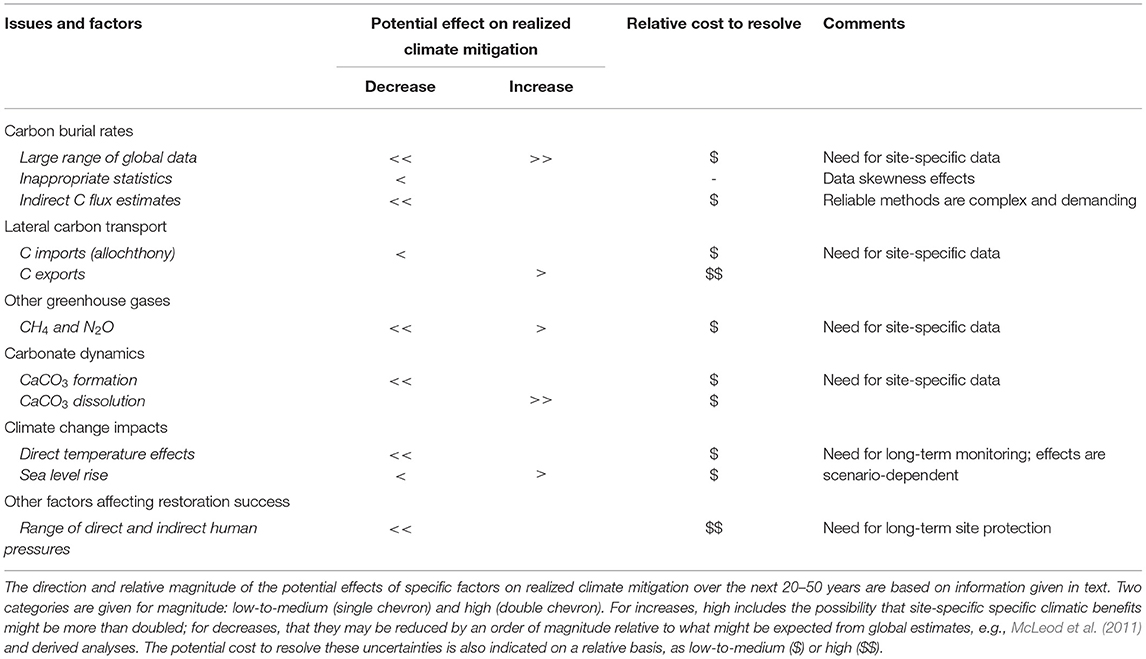
Table 2. Summary of biogeochemical issues affecting the reliability of using CBCE restoration for climate mitigation.
The above concerns do not mean that CBCE restoration cannot be worthwhile, and do not contradict Gattuso et al.'s (2018, 2021) assessments that CBCE restoration is a “low regret” measure. The underlying rationale of that conclusion is that, despite low effectiveness in increasing carbon removal (cumulative removal of, at most, 2–3 years of current CO2 emissions by 2100; Table 1), CBCE restoration has a high level of technological readiness, high governability at the local scale, high-to-very-high levels of co-benefits, and a low-to-very-low level of disbenefits (Gattuso et al., 2021; Babiker et al., 2022). Measures from the “low regret” cluster were therefore recommended by Gattuso et al. (2021) as high priority for implementation, recognizing that they may prove to be more advantageous at the national, rather than global, level (Herr et al., 2018; Taillardat et al., 2018).
Yet caution is clearly needed, even at the national level (Box 2). The many uncertainties associated with using CBCE restoration for carbon removal (Table 2) mean that there is a very real risk of non-delivery of the expected climatic benefits. For that reason, and because of the well-recognized seriousness of failure to meet national and global mitigation targets in the near future, we consider that it is premature to “operationalize” marketable blue carbon (Macreadie et al., 2022) with associated effort to include CBCE restoration in carbon offset trading (Claes et al., 2022). CBCE restoration should therefore be in addition to, not as a substitute for, near-total emission reductions. Where CBCE restoration projects are carried out primarily for their intended climatic benefits, they need to include comprehensive long-term monitoring and protection, involving additional costs.
Box 2. Caution needed for blue carbon comparisons.
Comparisons of CBCE carbon stocks (that are relatively easy to measure) with their carbon burial rates (that are not) can be misleading (Jennerjahn, 2020). An inadvertent example is provided by Macreadie et al. (2021), where the accumulation of sediment carbon resulting from widespread mangrove restoration in the Mekong Delta is stated as being climatically equivalent to three times Vietnam's 2013 greenhouse gas emissions. That claim is based on Dung et al. (2016), who measured depth-profiled carbon stock in 2.5 m sediment cores at a single cluster of sites in 2013.
However, sediment accretion rates were not determined, and the “accumulation” value would only be valid if there was zero carbon before restoration began (in 1978). Initial sediment carbon stocks do not seem to have been measured, but nearby unforested mudflats might reasonably be considered to provide “control” values for comparative purposes. On that basis, the carbon increase, and associated climate benefit, would be reduced to 7.1% of claimed values over the 35 years of the restoration project—hence 0.20% when expressed as an annual rate. Further scaling-down would seem necessary due to: the presence of non-local (allochthonous) carbon in the sediment; the non-permanent storage of organic carbon in upper layers; and the possibility of enhanced CH4 and N2O emissions. Taking account of just the first factor could result in the actual annual benefit being 0.12% of the amount claimed (assuming the proportion of allochthonous carbon is 0.4, a mid-range value as measured at another Vietnamese mangrove restoration site; Hieu et al., 2017). Overall, these considerations could therefore reduce the climatic benefits of the mangrove restoration by as much as two orders of magnitude, from three times Vietnam's 2013 emissions to around 0.3% of those emissions, when both rates are expressed annually (although subject to several unresolved uncertainties).
Nevertheless, wetland habitat restoration in the Mekong Delta, and its long-term protection, can be considered worthwhile for a wide range of other reasons.
Additional governance precautions are also likely to be necessary (Jakovac et al., 2020). Such precautions include the avoidance of loopholes, mis-reporting and perverse incentives (Climate Analytics, 2017), as have widely occurred when complex financial incentives have been used as part of UNFCCC's REDD+ mechanism under the Kyoto Protocol (Correa et al., 2019; Badgley et al., 2021) with its goal of reducing emissions from deforestation and forest degradation in developing countries.
Author Contributions
PW and J-PG drafted and finalized the content of this paper. All authors contributed to the article and approved the submitted version.
Funding
The Ocean Solutions Initiative was financially supported by the Prince Albert II of Monaco Foundation, the Veolia Foundation, the IAEA Ocean Acidification International Coordination Center, and the French Facility for Global Environment.
Conflict of Interest
The authors declare that the research was conducted in the absence of any commercial or financial relationships that could be construed as a potential conflict of interest.
Publisher's Note
All claims expressed in this article are solely those of the authors and do not necessarily represent those of their affiliated organizations, or those of the publisher, the editors and the reviewers. Any product that may be evaluated in this article, or claim that may be made by its manufacturer, is not guaranteed or endorsed by the publisher.
Acknowledgments
We thank the reviewers for their constructive comments, also Carlos Duarte and Judith Rosentreter for comments that helped improve the text (without necessarily endorsing all our conclusions) and Josh Breithaupt for providing the data used for Figure 1. This work is a product of the Ocean Solutions Initiative (https://bit.ly/3sCzEpc).
References
Abelson, A., Reed, D. C., Edgar, G. J., Smith, C. S., Kendrick, G. A., Orth, R. J., et al. (2020). Challenges for the restoration of coastal marine ecosystems in the Anthropocene. Front. Mar. Sci. 7:544105. doi: 10.3389/fmars.2020.544105
Adam, P. (2019). “Salt marsh restoration,” in Coastal Wetlands, eds G. M. E. Perillo, E. Wolanski, D. R. Cahoon and C. S. Hopkinson (Amsterdam: Elsevier), 817–861. doi: 10.1016/B978-0-444-63893-9.00023-X
Al-Haj, A. N., and Fulweiler, R. W. (2020). A synthesis of methane emissions from shallow vegetated coastal ecosystems. Glob. Change Biol. 26, 2988–3005. doi: 10.1111/gcb.15046
Almeida, D., Neto, C., Esteves, L. S., and Costa, J. C. (2014). The impacts of land-use change on the recovery of saltmarshes in Portugal. Ocean Coast. Manage. 92, 40–49. doi: 10.1016/j.ocecoaman.2014.02.008
Alongi, D. M. (2018). Blue Carbon. Coastal Sequestration for Climate Change Mitigation. Cham: Springer. doi: 10.1007/978-3-319-91698-9
Arias-Ortiz, A., Masqué, P., Garcia-Orellana, J., Serrano, O., Mazarrasa, I., Marbàn, A., et al. (2018a). Reviews and syntheses: 210Pb-derived sediment and carbon accumulation rates in vegetated coastal ecosystems – setting the record straight. Biogeosci. 15, 6791–6818. doi: 10.5194/bg-15-6791-2018
Arias-Ortiz, A., Serrano, O., Masqué, P., Lavery, P. S., Mueller, U., Kendrick, G. A., et al. (2018b). A marine heatwave drives massive losses from the world's largest seagrass carbon stocks. Nat. Clim. Change 8, 338–344. doi: 10.1038/s41558-018-0096-y
Babiker, M., Berndes, G., Blok, K., Cohen, B., Cowie, A., Geden, O., et al. (2022). “Cross sectoral perspectives,” in: Climate Change 2022: Mitigation of Climate Change. Contribution of Working Group III to the Sixth Assessment Report of the Intergovernmental Panel on Climate Change. Cambridge: Cambridge University Press.
Badgley, G., Freeman, J., Hamman, J. J., Haya, B., Trugman, A. T., Anderegg, W. R. L., et al. (2021). Systematic over-crediting in California's forest carbon offsets program. Glob. Change Biol. 28:441870. doi: 10.1101/2021.04.28.441870
Bayraktarov, E., Saunders, M. I., Abdullah, S., Mills, M., Beher, J., Possingham, H. P., et al. (2016). The cost and feasibility of marine coastal restoration. Ecol. Applic. 26, 1055–1074. doi: 10.1890/15-1077
Berck, J., and Gustafson, D. J. (2012). Plant source influence on Spartina alterniflora survival and growth in restored South Carolina salt marshes. Southeast. Naturalist. 11, 747–754. doi: 10.1656/058.011.0412
Bertram, C., and Merk, C. (2020). Public perceptions of ocean-based carbon dioxide removal: the nature-engineering divide? Front. Clim. 2:594194. doi: 10.3389/fclim.2020.594194
Bindoff, N. L., Cheung, W. W. L., Kairo, J. G., Arístegui, J., Guinder, V. A., Hallberg, R., et al. (2019). “Changing ocean, marine ecosystems, and dependent communities,” in IPCC Special Report on the Ocean and Cryosphere in a Changing Climate, eds H. O. Pörtner, D. C. Roberts, V. Masson-Delmotte, P. Zhai, M. Tignor, E. Poloczanska, et al. (Geneva: Intergovernmental Panel on Climate Change), 447–587.
Bossio, D. A., Cook-Patton, S. C., Ellis, P. W., Fargione, J., Sanderman, J., Smith, P., et al. (2020). The role of soil carbon in natural climate solutions. Nat. Sustain. 3, 391–398. doi: 10.1038/s41893-020-0491-z
Brander, M., Ascui, F., Scott, V., and Tett, S. (2021). Carbon accounting for negative emissions technologies. Clim. Policy 21, 699–717. doi: 10.1080/14693062.2021.1878009
Breithaupt, J. L., Smoak, J. M., Smith, I. I. I. T. J, Sanders, C. J., and Hoare, A. (2012). Organic carbon burial rates in mangrove sediments: strengthening the global budget. Glob. Biogeochem. Cycles 26:GB3011. doi: 10.1029/2012GB004375
Callaway, J. C. (2019). “Accretion: measurement and interpretation of wetland sediments,” in A Blue Carbon Primer: The State of Coastal Wetland Carbon Science, Practice and Policy, eds L. Windham-Myers, S. Crooks and T.G. Troxler (Boca Raton, FL: CRC Press, Boca Raton), 81–92. doi: 10.1201/9780429435362-7
Carnell, P. E., Palacios, M. M., Waryszak, P., Trevathan-Tackett, S. M., Masqué, P., and Macreadie, P. I. (2022). Blue carbon drawdown by restored mangrove forests improves with age. J. Environ. Manage. 306:114301. doi: 10.1016/j.jenvman.2021.114301
Cavanaugh, K. C., Dangemond, E. M., Doughty, C. L., Williams, A. P., Parker, J. D., Hayes, M. A., et al. (2019). Climate-driven regime shifts in a mangrove-salt marsh ecotone over the past 250 years. Proc. Natl. Acad. Sci. U. S. A. 116, 21602–21608. doi: 10.1073/pnas.1902181116
CEC (2014). Greenhouse Gas Offset Methodology Criteria for Tidal Wetland Conservation. Montreal: Commission for Environmental Cooperation. Available online at: http://www.cec.org/publications/greenhouse-gas-offset-methodology-criteria-for-tidal-wetland-conservation (accessed December 15, 2021).
Chauhan, R., Datta, A., Ramathan, A. L., and Adhya, T. K. (2017). Whether conversion of mangrove forests to rice cropland is environmentally and economically viable? Agric. Ecosyst. Environ. 246, 38–47. doi: 10.1016/j.agee.2017.05.010
Chefaoui, R. M., Duarte, C. M., and Serrão, E. A. (2018). Dramatic loss of seagrass habitat under projected climate change in the Mediterranean Sea. Glob. Change Biol. 24,4919–4928. doi: 10.1111/gcb.14401
Chew, S. T., and Gallagher, J. B. (2018). Accounting for black carbon lowers estimates of blue carbon storage services. Sci. Rep. 8:2553. doi: 10.1038/s41598-018-20644-2
Claes, J., Hopman, D., Jaeger, G., and Rogers, M. (2022). Blue Carbon: The Potential of Coastal and Oceanic Climate Action, 32. McKinsey & Company. Available online at: https://www.mckinsey.com/business-functions/sustainability/our-insights/blue-carbon-the-potential-of-coastal-and-oceanic-climate-action (accessed June 8, 2022).
Climate Analytics (2017). The Dangers of Blue Carbon Offsets: From Hot Air to Hot Water? 13. Berlin: Climate Analytics. Available online at: https://climateanalytics.org/publications/2017/the-dangers-of-blue-carbon-offsets-from-hot-air-to-hot-water (accessed January 11, 2022).
Conrad, R. (2020). Importance of hydrogenotrophic, aceticlastic and methylotrophic methanogenesis for methane production in terrestrial, aquatic and other anoxic environments: a mini review. Pedosphere 30, 25–39. doi: 10.1016/S1002-0160(18)60052-9
Cooley, S., Schoeman, D., Bopp, L., Boyd, P., Donner, S., Ito, S.-I., et al. (2022). “Oceans and Coastal Ecosystems and their Services,” in Climate Change 2022: Impacts, Adaptation and Vulnerability. Contribution of Working Group II to the Sixth Assessment Report of the Intergovernmental Panel on Climate Change. Cambridge: Cambridge University Press.
Correa, J., van der Hoff, R., and Rajão, R. (2019). Amazon Fund 10 years later: lessons from the world's largest REDD+ program. Forests 10:272. doi: 10.3390/f10030272
Crooks, S., Sutton-Grier, A. E., Troxler, T. G., Herold, N., Bernal, B., Schile-Beers, L., et al. (2018). Coastal wetland management as a contribution to the US National Greenhouse Gas Inventory. Nat. Clim. Change 8, 1109–1112. doi: 10.1038/s41558-018-0345-0
Cunha, A. H., Marb,à, N. N., van Katwijk, M. M., Pickerell, C., Henriques, M., Bernard, G., et al. (2012). Changing paradigms in seagrass restoration. Restor. Ecol. 20, 427–430. doi: 10.1111/j.1526-100X.2012.00878.x
de los Santos, C., Krause-Jensen, D., Alcoverro, T., Marb,à, N., Duarte, C. M., van Katwijk, M. M., et al. (2019). Recent trend reversal for declining European seagrass meadows. Nat. Comms. 10:3356. doi: 10.1038/s41467-019-11340-4
Derby, R. K., Needelman, B. A., Roden, A. A., and Megonigal, J. P. (2022). Vegetation and hydrology stratification as proxies to estimate methane emissions from tidal marshes. Biogeochem. 157, 227–243. doi: 10.1007/s10533-021-00870-z
Drexler, J. Z., Davis, M. J., Woo, I., and De La Cruz, S. (2020). Carbon sources in the sediments of a restoring vs. historically unaltered salt marsh. Est. Coasts 43, 1345–1360. doi: 10.1007/s12237-020-00748-7
Duarte de Paula Costa, M., Lovelock, C. E., Waltham, N. J., Moritsch, M. M., Butler, D., Power, T., et al. (2022). Modelling blue carbon farming opportunities at different spatial scales. J. Environ. Manage. 301:113813. doi: 10.1016/j.jenvman.2021.113813
Duarte, C. M., Agusti, S., Barbier, E., Britten, G. L., Castilla, J. C., Gattuso, J.-P., et al. (2020). Rebuilding marine life. Nature 580, 39–51. doi: 10.1038/s41586-020-2146-7
Duarte, C. M., Middelburg, J. J., and Caraco, N. (2005). Major role of marine vegetation on the oceanic carbon cycle. Biogeosci. 2, 1–8. doi: 10.5194/bg-2-1-2005
Duke, N. C., Kovacs, J. M., Griffiths, A. D., Preece, L., Hill, D. J., Van Oosterzee, P., et al. (2017). Large-scale dieback of mangroves in Australia's Gulf of Carpentaria: a severe ecosystem response, coincidental with an unusually extreme weather event. Mar. Freshwater Res. 68, 1816–1829. doi: 10.1071/MF16322
Dung, L. V., Tue, N. T., Nhuan, M. T., and Omori, K. (2016). Carbon storage in a restored mangrove forest in Can Gio Mangrove Forest Park, Mekong Delta, Vietnam. Forest Ecol. Manag. 380, 31–40. doi: 10.1016/j.foreco.2016.08.032
Eger, A. M., Earp, H. S., Friedman, K., Gatt, Y., Hagger, V., Hancock, B., et al. (2022). The need, opportunities, and challenges for creating a standardized framework for marine restoration monitoring and reporting. Biol. Cons. 266:109429. doi: 10.1016/j.biocon.2021.109429
Fearnside, P. M. (2002). Why a 100-year time horizon should be used for global warming mitigation calculations. Mitig. Adapt. Strat. Glob. Change 7, 19–30. doi: 10.1023/A:1015885027530
Foster, S. Q., and Fulweiler, R. W. (2016). Sediment nitrous oxide fluxes are dominated by uptake in a temperate estuary. Front. Mar. Sci. 3, 1–13. doi: 10.3389/fmars.2016.00040
Friedlingstein, P., Jones, M. W., O'Sullivan, M., Andrew, R. M., Bakker, D. C., Hauck, J., et al. (2022). Global carbon budget 2021. Earth Syst. Sci. Data 14, 1917–2005. doi: 10.5194/essd-14-1917-2022
Gallo, N. D., Victor, D. G., and Levin, L. A. (2017). Ocean commitments under the Paris Agreement. Nat. Clim. Change 7. 833–838. doi: 10.1038/nclimate3422
Gardner, L. R., Sharma, P., and Moore, W. S. (1987). A regeneration model for the effect of bioturbation by fiddler crabs on 210Pb profiles in salt marsh sediments. J. Environ. Radioactivity 5, 25–36. doi: 10.1016/0265-931X(87)90042-7
Gattuso, J.-P., Magnan, A., Bopp, L., Cheung, W., Duarte, C., Hinkel, J., et al. (2018). Ocean solutions to address climate change and its effects on marine ecosystems. Front. Mar. Sci. 5:337. doi: 10.3389/fmars.2018.00337
Gattuso, J.-P., Williamson, P., Duarte, C. M., and Magnan, A. K. (2021). The potential for ocean-based climate action: negative emissions technologies and beyond. Front. Clim. 2:575716. doi: 10.3389/fclim.2020.575716
Geraldi, N. R., Ortega, A., Serrano, O., Macreadie, P. I., Lovelock, C. E., Krause-Jensen, D., et al. (2019). Fingerprinting blue carbon: rationale and tools to determine the source of organic carbon in marine depositional environments. Front. Mar. Sci. 6:263. doi: 10.3389/fmars.2019.00263
GESAMP (2019). “High level review of a wide range of proposed marine geoengineering techniques,” in (IMO/FAO/UNESCO-IOC/UNIDO/WMO/IAEA/UN/UN Environment/ UNDP/ISA Joint Group of Experts on the Scientific Aspects of Marine Environmental Protection). Rep. Stud. GESAMP No. 98, eds P. W. Boyd and C.M.G. Vivian (London: International Maritime Organization), 2019.
Griscom, B. W., Adams, J., Ellis, P. W., Houghton, R. A., Lomax, G., et al. (2017). Natural climate solutions. Proc. Natl. Acad. Sci. U. S. A. 114, 11645–11650. doi: 10.1073/pnas.1710465114
Hanley, M. E., Bouma, T. J., and Mossman, H. L. (2020). The gathering storm: optimizing management of coastal ecosystems in the face of a climate-driven threat. Ann. Bot. 125, 197–212. doi: 10.1093/aob/mcz204
Hatje, V., Masqué, P., Patire, V. F., Dórea, A., and Barros, F. (2021). Blue carbon stocks, accumulation rates, and associated spatial variability in Brazilian mangroves. Limnol. Oceanogr. 66, 321–324. doi: 10.1002/lno.11607
Herr, D., Blum, J., Himes-Cornell, A., and Sutton-Grier, A. (2019). An analysis of the potential positive and negative livelihood impacts of coastal carbon offset projects. J. Environ. Manag. 235, 463–479. doi: 10.1016/j.jenvman.2019.01.067
Herr, D., Chagas, T., Krämer, N., Conway, D. V. P., and Streck, C. (2018). Coastal Blue Carbon and Article 6: Implications and Opportunities, 26. Amsterdam: Climate Focus.
Herr, D., and Landis, E. (2016). Coastal Blue Carbon Ecosystems. Opportunities for Nationally Determined Contributions. Policy Brief. Gland: International Union for the Conservation of Nature.
Hieu, P. V., Dung, L. V., Tue, N. T., and Omori, K. (2017). Will restored mangrove forests enhance sediment organic carbon and ecosystem carbon storage? Reg. Stud. Mar. Sci. 14, 43–52. doi: 10.1016/j.rsma.2017.05.003
Hilmi, N., Chami, R., Sutherland, M. D., Hall-Spencer, J. M., Leblue, L., Benetiz, M. B., et al. (2021). The role of blue carbon in climate change mitigation and carbon stock conservation. Front. Clim. 3:710546. doi: 10.3389/fclim.2021.710546
Hoegh-Guldberg, O., Caldeira, K., Chopin, T., Gaines, S., Haugan, P., Hemer, M., et al. (2019). The Ocean as a Solution to Climate Change: Five Opportunities for Action. Washington, DC: World Resources Institute.
Howard, J. L., Creed, J. C., Aguiar, M. V. P., and Fourqurean, J. W. (2018). CO2 released by carbonate sediment production in some coastal areas may offset the benefits of seagrass “Blue Carbon” storage. Limnol. Oceanogr. 63, 160–172. doi: 10.1002/lno.10621
IPCC (2014). “2013 supplement to the 2006 IPCC guidelines for national greenhouse gas inventories: wetlands,” in eds T. Hiraishi, T. Krug, K. Tanabe, N. Srivastava, N. Baasansuren, M. Fukuda et al. (Geneva; IPCC).
IPCC (2018). “Summary for policymakers,” in Global Warming of 1.5°C. An IPCC Special Report on the Impacts of Global Warming of 1.5°C above Pre-industrial Levels and Related Global Greenhouse Gas Emission Pathways, in the Context of Strengthening the Global Response to the Threat of Climate Change, Sustainable Development, and Efforts to Eradicate Poverty, eds V. Masson-Delmotte, P. Zhai, H.-O. Pörtner, D. Roberts, J. Skea, P. R. Shukla, et al. (Geneva: Intergovernmental Panel on Climate Change), 3–24.
Iram, N., Kavehei, E., Maher, D. T., Bunn, S. E., Rashti, M. R., Farahani, B. S., et al. (2021). Soil greenhouse gas fluxes from tropical coastal wetlands and alternative agricultural land uses. Biogeosci. 18, 5085–5096. doi: 10.5194/bg-18-5085-2021
Jakovac, C. C., Latawiec, A. E., Lacerda, E., Lucas, I. L., Korys, K. A., Irribarrem, A., et al. (2020). Costs and carbon benefits of mangrove conservation and restoration: a global analysis. Ecol. Econ. 176:106758. doi: 10.1016/j.ecolecon.2020.106758
Jennerjahn, T. C. (2020). Relevance and magnitude of ‘Blue Carbon' storage in mangrove sediments: carbon accumulation rates vs. stocks, sources vs. sinks. Est. Coast. Shelf Sci. 247:107027. doi: 10.1016/j.ecss.2020.107027
Jia, M., Wang, Z., Zhang, Y., Mao, D., and Wang, C. (2018). Monitoring loss and recovery of mangrove forests during 42 years: the achievements of mangrove conservation in China. Int. J. Appl. Earth Obs. Geoinform. 73, 535–545. doi: 10.1016/j.jag.2018.07.025
Johannessen, S. C., and Macdonald, R. W. (2016). Geoengineering with seagrasses: is credit due where credit is given? Environ. Res. Lett. 11:113001. doi: 10.1088/1748-9326/11/11/113001
Kelleway, J. J., Serrano, O., Baldock, J. A., Burgess, R., Cannard, T., et al. (2020). A national approach to greenhouse gas abatement through blue carbon management. Glob. Environ. Change 63:102083. doi: 10.1016/j.gloenvcha.2020.102083
Kennedy, H. A., Fourqurean, J. W., and Papadimitriou, S. (2019). “The calcium carbonate cycle in seagrass ecosystems,” in A Blue Carbon Primer: The State of Coastal Wetland Carbon Science, Practice and Policy, eds L. Windham-Myers, S. Crooks and T.G. Troxler (Boca Raton, FL: CRC Press, Boca Raton), 107–119. doi: 10.1201/9780429435362-9
Kim, S. H., Suonan, Z., Qin, L.-Z., Kim, H., Park, J.-I., Kim, Y. K., et al. (2022). Variability in blue carbon storage related to biogeochemical factors in seagrass meadows off the coast of the Korean peninsula. Sci. Tot. Environ. 813:152680. doi: 10.1016/j.scitotenv.2021.152680
Kirschbaum, M. U. F. (2006). Temporary carbon sequestration cannot prevent climate change. Mitig. Adapt. Strat. Glob. Change 11, 1151–1164. doi: 10.1007/s11027-006-9027-8
Kodikara, K. A. S., Mukherjee, N., Jayatissa, L. P., Dahdouh-Guebas, F., and Koedam, N. (2017). Have mangrove restoration projects worked? An in depth-study in Sri Lanka. Restor. Ecol. 25, 705–716. doi: 10.1111/rec.12492
Konisky, R. A., and Burdick, D. M. (2004). Effects of stressors on invasive and halophytic plants of New England salt marshes: a framework for predicting response to tidal restoration. Wetlands 24, 434–447. doi: 10.672/0277-5212(2004)024[0434:EOSOIA]2.0.CO;2
Kristensen, E., Quintana, C. O., and Petersen, S. G. G. (2022). “The role of biogenic structures for greenhouse gas balance in vegetated tidal wetlands,” in Carbon Mineralization in Coastal Wetlands, from Litter Decomposition to Greenhouse Gas Dynamics, eds X. Ouyang, S. Y. Lee, D.Y. F. Lai and C. Marchand (Amsterdam: Elsevier), 233–267. doi: 10.1016/B978-0-12-819220-7.00001-7
Kroeger, K. D., Crooks, S., Moseman-Valtierra, S., and Tang, J. (2017). Restoring tides to reduce methane emissions in impounded wetlands. Sci. Rep. 7:11914. doi: 10.1038/s41598-017-12138-4
Ladd, C. J. T., Duggan-Edwards, M. F., Bouma, T. J., Pagès, J. F., and Skov, M. W. (2019). Sediment supply explains long-term and large-scale patterns in salt marsh lateral expansion and erosion. Geophys. Res. Lett. 46, 11178–11187. doi: 10.1029/2019GL083315
Laffoley, D. (2020). Protecting and Effectively Managing Blue Carbon Ecosystems to Realise the Full Value to Society- a Sea of Opportunities. Opinion piece for WWF-UK, Woking, 2020. Available online at: https://www.wwf.org.uk/sites/default/files/2020-11/WWF_blue_carbon020.pdf (accessed December 14, 2021).
Laffoley, D. d'A., and Grimsditch, G. (2009). The Management of Natural Coastal Carbon Sinks. Gland: International Union for the Conservation of Nature.
Legge, O., Johnson, M., Hicks, N., Jickells, T., Diesing, D., Aldridge, J., et al. (2020). Carbon on the northwest European shelf: contemporary budget and future influences. Front. Mar. Sci. 7:143. doi: 10.3389/fmars.2020.00143
Leorri, E., Zimmerman, A. R., Mitra, S., Christian, R. R., Fatela, F., and Mallinson, D. J. (2018). Refractory organic matter in coastal saltmarshes – effect on C sequestration calculations. Sci. Total Env. 633, 391–398. doi: 10.1016/j.scitotenv.2018.03.120
Li, Y., Fu, C., Zeng, L., Zhou, Q., Zhang, H., Tu, C., et al. (2021). Black carbon contributes substantially to allochthonous carbon storage in deltaic vegetated coastal habitats. Env. Sci. Technol. 55, 6495–6504. doi: 10.1021/acs.est.1c00636
López-Portillo, J., Lewis, R. R., Saenger, P., Rovai, A., Koedam, N., Dahdouh-Guebas, F., et al. (2017). “Mangrove forest restoration and rehabilitation,” in Mangrove Ecosystems: A Global Biogeographic Perspective, eds V. H. Rivera-Monroy, S. Y. Lee, E. Kristensen and R. R. Twilley (Cham: Springer), 301–345. doi: 10.1007/978-3-319-62206-4_10
Lovelock, C. E., and Reef, R. (2020). Variable impacts of climate change on blue carbon. One Earth 3, 195–211. doi: 10.1016/j.oneear.2020.07.010
Macreadie, P I., Nielsen, D. A., Kelleway, J. J., Atwood, T. B., Seymour, J. R., Petrou, K., et al. (2017a). Can we manage coastal ecosystems to sequester more blue carbon?. Front. Ecol. Environ. 15, 206–213. doi: 10.1002/fee.1484
Macreadie, P. I., Anton, A., Raven, J. A., Beaumont, N., Connolly, R. M., Friess, D. A., et al. (2019). The future of blue carbon science. Nat. Comms. 10:3998. doi: 10.1038/s41467-019-11693-w
Macreadie, P. I., Costa, M. D. P., Atwood, T. B., Friess, D. A., Kelleway, J. J., Kennedy, H., et al. (2021). Blue carbon as a natural climate solution. Nat. Rev. Earth Environ. 2, 826–839. doi: 10.1038/s43017-021-00224-1
Macreadie, P. I., Robertson, A. I., Spinks, B., Adams, M. P., Atchison, J. M., Bell-James, J., et al. (2022). Operationalizing marketable blue carbon. One Earth 5, 485–492. doi: 10.1016/j.oneear.2022.04.005
Macreadie, P. I., Serrano, O., Maher, D. T., Duarte, C. M., and Beardall, J. (2017b). Addressing calcium carbonate cycling in blue carbon accounting. Limnol. Oceanogr. Lett. 2, 195–201. doi: 10.1002/lol2.10052
Maher, D. T., Call, M., Santos, I. R., and Sanders, C. J. (2018). Beyond burial: lateral exchange is a significant atmospheric carbon sink in mangrove forests. Biol. Lett. 14:20180200. doi: 10.1098/rsbl.2018.0200
Marchand, C., David, F., Jacotot, A., Leopold, A., and Ouyang, X. (2022). “CO2 and CH4 emissions from coastal wetland soils,” in Carbon Mineralization in Coastal Wetlands, from Litter Decomposition to Greenhouse Gas Dynamics, eds X. Ouyang, S. Y. Lee, D.Y. F. Lai and C. Marchand (Amsterdam: Elsevier), 55–91. doi: 10.1016/B978-0-12-819220-7.00006-6
McLaren, D. (2012). A comparative global assessment of potential negative emissions technologies. Proc. Safety Env. Prot. 90, 489–500, doi: 10.1016/j.psep.2012.10.005
McLeod, E., Chmura, G. L., Bouillon, S., Salm, R., Björk, M., et al. (2011). A blueprint for blue carbon: toward an improved understanding of the role of vegetated coastal habitats in sequestering CO2. Ecol. Environ. 9, 552–560. doi: 10.1890/110004
Minx, J. C., Lamb, W. F., Callaghan, M. W., Fuss, S., Hilaire, J., Creutzig, F., et al. (2018). Negative emissions—Part 1: research landscape and synthesis. Environ. Res. Lett. 13:063001. doi: 10.1088/1748-9326/aabf9b
Morris, J. T., and Callaway, J. C. (2019). “Physical and biological regulation of carbon sequestration in tidal marshes,” in A Blue Carbon Primer: The State of Coastal Wetland Carbon Science, Practice and Policy, eds L. Windham-Myers, S. Crooks and T. G. Troxler (Boca Raton, FL: CRC Press), 67–79. doi: 10.1201/9780429435362-6
Mueller, P., Do, H. T., Jensen, K., and Nolte, S. (2019). Origin of organic carbon in the topsoil of Wadden Sea salt marshes. Mar. Ecol. Prog. Ser. 624, 39–50. doi: 10.3354/meps13009
Murray, R. H., Erler, D. V., and Eyre, B. D. (2015). Nitrous oxide fluxes in estuarine environments: response to global change. Glob. Change Biol. 21, 3219–3245. doi: 10.1111/gcb.12923
Myhre, G., Shindell, D., Bréon, F.-M., Collins, W., Fuglestvedt, J., Huang, J., et al. (2013). “Anthropogenic and Natural Radiative Forcing,” in Climate Change 2013: The Physical Science Basis. Contribution of Working Group 1 to the Fifth Assessment Report of the Intergovernmental Panel on Climate Change, eds T. F. Stocker, D. Qin, G.-K. Plattner, M. Tignor, S. K. Allen, J. Boschung, et al. (Cambridge: Cambridge University Press), 659–740. doi: 10.1017/CBO9781107415324.018
NASEM (2019). Negative Emissions Technologies and Reliable Sequestration: A Research Agenda. Washington, DC: The National Academies Press.
Needelman, B. A., Emmer, I. M., Emmett-Mattox, S., Crooks, S., Megonigal, J. P., Myers, D., et al. (2018). The science and policy of the Verified Carbon Standard Methodology for Tidal Wetland and Seagrass Restoration. Est. Coasts 41, 2159–2171. doi: 10.1007/s12237-018-0429-0
Needelman, B. A., Emmer, I. M., Oreska, M. P. J., and Megonigal, J. P. (2019). “Blue carbon accounting for carbon markets,” in A Blue Carbon Primer: The State of Coastal Wetland Carbon Science, Practice and Policy, eds L. Windham-Myers, S. Crooks and T. G. Troxler (Boca Raton, FL: CRC Press), 283–292. doi: 10.1201/9780429435362-20
Nellemann, C., Corcoran, E., Duarte, C. M., Valdes, L., DeYoung, C., et al. (2009). Blue Carbon. A Rapid Response Assessment. GRID-Arendal. Available online at: https://www.grida.no/publications/145 (accessed January 4, 2022).
Neubauer, S. C., and Verhoeven, J. T. A. (2019). “Wetland effects on global climate: mechanisms, impacts and management recommendations,” in Wetlands: Ecosystem Services, Restoration and Wise Use, eds S. An and J. T. A. Voerhoven (Cham: Springer), 39–42. doi: 10.1007/978-3-030-14861-4_3
Nguyen, H. M., Ralph, P. J., Marín-Guirao, L., Pernice, M., and Procaccini, G. (2021). Seagrasses in an era of ocean warming. Biol. Rev. 96, 2009–2030. doi: 10.1111/brv.12736
Osaka, S., Bellamy, R., and Castree, N. (2021). Framing “nature-based” solutions to climate change. WIREs Clim. Change 12:e729. doi: 10.1002/wcc.729
Parmesan, C., Morecroft, M. D., Trisurat, Y., Adrian, R., Anshari, G. Z., Arneth, A., et al. (2022). “Terrestrial and freshwater ecosystems and their services,” in Climate Change 2022: Impacts, Adaptation and Vulnerability. Contribution of Working Group II to the Sixth Assessment Report of the Intergovernmental Panel on Climate Change. Cambridge: Cambridge University Press.
Poffenbarger, H. J., Needelman, B. A., and Megonigal, J. P. (2011). Salinity influence on methane emissions from tidal marshes. Wetlands 31, 831–842. doi: 10.1007/s13157-011-0197-0
Ricart, A. M., York, P. H., Bryant, C. V., Rasheed, M. A., Ierodiaconou, D., and Macreadie, P. I. (2020). High variability of blue carbon storage in seagrass meadows at the estuary scale. Sci. Rep. 10, 5865. doi: 10.1038/s41598-020-62639-y
Roe, S., Streck, C., Beach, R., Busch, J., Chapman, M., Daioglou, V., et al. (2021). Land-based measures to mitigate climate change: potential and feasibility by country. Glob. Change Biol. 27, 6025–6058. doi: 10.1111/gcb.15873
Rogers, K., Kelleway, J. J., Saintilan, N., Megonigal, J. P., Adams, J. B., Holmquist, J. R., et al. (2019). Wetland carbon storage controlled by millennial-scale variation in relative sea-level rise. Nature 567, 91–95. doi: 10.1038/s41586-019-0951-7
Rosentreter, J. A., Al-Haj, A. N., Fulweiler, R. W., and Williamson, P. (2021). Methane and nitrous oxide emissions complicate blue carbon assessments. Glob. Biogeochem. Cycles 35:e2020GB006858. doi: 10.1029/2020GB006858
Rosentreter, J. A., and Williamson, P. (2020). Concerns and uncertainties relating to methane emissions synthesis for vegetated coastal ecosystems. Glob. Change Biol. 26, 5351–5352. doi: 10.1111/gcb.15201
Roth, F., Sun, X., Geibel, M. C., Prytherch, J., Brüchert, V., Bonaglia, S., et al. (2022). High spatiotemporal variability in methane concentrations challenges estimates of emissions across vegetated coastal ecosystems, Glob. Change Biol. 2022:gcb.16177. doi: 10.1111/gcb.16177
Royal Society and Royal Academy of Engineering (2018). Greenhouse Gas Removal. London: Royal Society.
Ruseva, T., Hedrick, J., Marland, G., Tover, H., Sabou, C., and Besombes, E. (2020). Rethinking standards of permanence for terrestrial and coastal carbon: implications for governance and sustainability. Curr. Op. Environ. Sust. 45, 69–77. doi: 10.1016/j.cosust.2020.09.009
Sadat-Noori, M., Maher, D. T., and Santos, I. R. (2016). Groundwater discharge as a source of dissolved carbon and greenhouse gases in a subtropical estuary. Est Coasts 39, 639–656. doi: 10.1007/s12237-015-0042-4
Saderne, V., Fusi, M, Thomson, T., Dunne, A., Mahmud, F., Roth, F., et al. (2021). Total alkalinity production in a mangrove system reveals an overlooked Blue Carbon component. Limnol. Oceanogr. Lett. 6, 61–67. doi: 10.1002/lol2.10170
Saderne, V., Geraldi, N. R., Macreadie, P. I., Maher, D. T., Middelburg, J. J., Serrano, O., et al. (2019). Role of carbonate burial in Blue Carbon budgets. Nat. Comms. 10:1106. doi: 10.1038/s41467-019-08842-6
Saintilan, N., Rogers, K., Mazumder, D., and Woodroffe, C. (2013). Allochthonous and autochthonous contributions to carbon accumulations and carbon store in southeastern Australian coastal wetlands. Est. Coast. Shelf Sci. 128, 84–92. doi: 10.1016/j.ecss.2013.05.010
Saintilan, N., Wilson, N. C., Rogers, K., Rajkaran, A., and Krauss, K. W. (2014). Mangrove expansion and salt marsh decline at mangrove poleward limits. Glob. Change Biol. 20, 147–157. doi: 10.1111/gcb.12341
Santos, I. R., Burdige, D. J., Jennerjahn, T. C., Bouillon, S., Cabral, A., Serrano, O., et al. (2021). The renaissance of Odum's outwelling hypothesis in ‘Blue Carbon' science. Est. Coastal Shelf Sci. 255:107361. doi: 10.1016/j.ecss.2021.107361
Santos, I. R., Maher, D. T., Larkin, R., Webb, J. R., and Sanders, C. J. (2019). Carbon outwelling and outgassing vs. burial in an estuarine tidal creek surrounded by mangrove and saltmarsh wetlands. Limnol. Oceanogr. 64, 996–1013. doi: 10.1002/lno.11090
Sapkota, Y., and White, J. R. (2020). Carbon offset market methodologies applicable for coastal wetland restoration and conservation in the United States: a review. Sci. Tot. Env. 701:134497. doi: 10.1016/j.scitotenv.2019.134497
Schuerch, M., Spencer, T., Temmerman, S., Kirwan, M. L., Wolff, C., Lincke, D., et al. (2018). Future response of global coastal wetlands to sea-level rise. Nature 561, 231–234. doi: 10.1038/s41586-018-0476-5
Seddon, N., Daniels, E., Davis, R., Chausson, A., Harris, R., Hou-Jones, X., et al. (2020). Global recognition of the importance of nature-based solutions to the impacts of climate change. Glob. Sustain. 3:e15. doi: 10.1017/sus.2020.8
Silverberg, N., Nguyen, H. V., Delibrias, G., Koide, M., Sundby, B., Yokoyama, Y., et al. (1986). Radionuclide profiles, sedimentation rates, and bioturbation in modern sediment of the Laurentian Trough, Gulf of St Lawrence. Oceanol. Acta 9, 285–290.
Stewart, R. R., Noyce, T., and Possingham, H. P. (2003). Opportunity cost of an ad hoc marine reserve design decisions: an example from South Australia. Mar. Ecol. Prog. Ser. 253, 25–38. doi: 10.3354/meps253025
Suello, R. H., Hernandez, S. L., Bouillon, S., Belliard, J.-P., Dominguez-Granda, L., Van de Broek, M., et al. (2022). Mangrove sediment organic carbon storage and sources in relation to forest age and position along a deltaic salinity gradient. Biogeosci. 19, 1571–1585. doi: 10.5194/bg-19-1571-2022
Taillardat, P., Friess, D. A., and Lupascu, M. (2018). Mangrove blue carbon strategies for climate change mitigation are most effective at the national scale. Biol. Lett. 14:20180251. doi: 10.1098/rsbl.2018.0251
Taillardat, P., Thompson, B. S., Garneau, M., Trottier, K., and Friess, D. A. (2020). Climate change mitigation potential of wetlands and the cost-effectiveness of their restoration. Interface Focus 10:20190129. doi: 10.1098/rsfs.2019.0129
Thomas, S. (2014). Blue carbon: knowledge gaps, critical issues, and novel approaches. Ecol Econ. 107, 22–38. doi: 10.1016/j.ecolecon.2014.07.028
Thorhaug, A., Gallagher, J. B., Kiswara, W., Prathep, A., Huang, X., et al. (2020). Coastal and estuarine blue carbon stocks in the greater Southeast Asia region: seagrasses and mangroves per nation and sum of total. Mar. Poll. Bull. 160:111168. doi: 10.1016/j.marpolbul.2020.111168
Ullman, R., Bilbao-Bastida, V., and Grimsditch, G. (2013). Including blue carbon in climate market mechanisms. Ocean Coastal Manage. 83, 15–18. doi: 10.1016/j.ocecoaman.2012.02.009
UNEP and IUCN (2021). Nature-Based Solutions for Climate Change Mitigation, 35. Nairobi; Gland: United Nations Environment Programme and International Union for the Conservation of Nature.
UNESCO (2020). UNESCO Marine World Heritage: Custodians of the Globe's Blue Carbon assets. Paris: United Nations Educational, Scientific and Cultural Organization.
UNFCCC (2015). Adoption of The Paris Agreement FCCC/CP/2015/L.9/Rev.1. United Nations Framework Convention on Climate Change. Available online at: http://unfccc.int/resource/docs/2015/cop21/eng/l09r01.pdf (accessed December 14, 2021).
UNFCCC (2021). Glasgow Climate Pact. Decision -/CP.26. United Nations Framework. Available online at: https://unfccc.int/sites/default/files/resource/cop26_auv_2f_cover_decision.pdf (accessed December 14, 2021).
Valiela, I., Lloret, J., Bowyer, T., Miner, S., Remsen, D., Elmstrom, E., et al. (2018). Transient coastal landscapes: rising sea level threatens saltmarshes. Sci. Tot. Env. 640, 1148–1156. doi: 10.1016/j.scitotenv.2018.05.235
Van Dam, B., Lopes, C., Osburn, C. L., and Forqurean, J. W. (2019). Net heterotrophy and carbonate dissolution in two subtropical seagrass meadows. Biogeosci. 16, 4411–4428. doi: 10.5194/bg-16-4411-2019
Van Dam, B., Zeller, M. A., Lopes, C., Smyth, A. R., Böttcher, M. E., Osburn, C., et al. (2021). Calcification-driven CO2 emissions exceed “Blue Carbon” sequestration in a carbonate seagrass meadow. Sci. Adv. 7:eabj1372. doi: 10.1126/sciadv.abj1372
Van de Broek, M., Vandendriessche, C., Poppelmonde, D., Merckx, R., Temmerman, S., et al. (2018). Long-term organic carbon sequestration in tidal marsh sediments is dominated by old-aged allochthonous inputs in a macro-tidal estuary. Glob. Change Biol. 24, 2498–2512. doi: 10.1111/gcb.14089
van Katwijk, M. M., Thorhaug, A., Marb,à, N., Orth, R. J., Duarte, C. M., Kendrick, G. A., et al. (2016). Global analysis of seagrass restoration: the importance of large-scale planting. J. Appl. Ecol. 53, 567–578 doi: 10.1111/1365-2664.12562
Vanderklift, M. A., Marcos-Martinez, R., Butler, J. R. A., Coleman, M., Lawrence, A., Prislan, H., et al. (2019). Constraints and opportunities for market-based finance for the restoration and protection of blue carbon ecosystems. Mar. Pol. 107:103429. doi: 10.1016/j.marpol.2019.02.001
Vegh, T., Pendleton, L., Murray, B., Troxler, T., Zhang, K., Castañeda-Moya, E., et al. (2019). “Ecosystem services and economic valuation: co-benefits of coastal wetlands,” in A Blue Carbon Primer: The State of Coastal Wetland Carbon Science, Practice and Policy, eds L. Windham-Myers, S. Crooks and T. G. Troxler (Boca Raton, FL: CRC Press), 249–266. doi: 10.1201/9780429435362-18
Verra (2021). VM0033 Methodology for Tidal Wetland and Seagrass Restoration. Verified Carbon Standard. Available online at: https://verra.org/methodology/vm0033-methodology-for-tidal-wetland-and-seagrass-restoration-v2-0/ (accessed December 15, 2021).
Waycott, M., Duarte, C. M., Carruthers, T. J. B., Orth, R. J., Dennison, W. C., Olyarnik, S., et al. (2009). Accelerating loss of seagrass across the globe threatens coastal ecosystems. Proc. Natl. Acad. Sci. USA 106, 12377–12381. doi: 10.1073/pnas.0905620106
Williamson, P. (2016). Emissions reduction: scrutinize CO2 removal methods. Nature 530, 153–155. doi: 10.1038/530153a
Williamson, P., and Guinder, V. A. (2021). “Effects of climate change on marine ecosystems,” in The Impacts of Climate Change: A Comprehensive Study of Physical, Biophysical, Social and Political Issues, ed T. M. Letcher (Amsterdam: Elsevier), 115–176. doi: 10.1016/B978-0-12-822373-4.00024-0
Wilson, S. T., Al-Haj, A. N., Bourbonnais, A., Frey, C., Fulweiler, R. W., Kessler, J. D., et al. (2020). A strategic assessment of methane and nitrous oxide measurements in the marine environment. Biogeosciences 17, 5809–5828. doi: 10.5194/bg-17-5809-2020
Windham-Myers, L, Crooks, S., and Troxler, T. G. (2019). A Blue Carbon Primer: The State of Coastal Wetland Carbon Science, Practice and Policy. Boca Raton, FL: CRC Press. doi: 10.1201/9780429435362
Wylie, L., Sutton-Grier, A. E., and Moore, A. (2016). Keys to successful blue carbon projects: lessons learned from global case studies. Mar. Pol. 65, 76–84. doi: 10.1016/j.marpol.2015.12.020
Xiong, Y., Liao, B., and Wang, F. (2018), Mangrove vegetation enhances soil carbon storage primarily through in situ inputs rather than increasing allochthonous sediments. Mar. Poll. Bull.131, 378–385. doi: 10.1016/j.marpolbul.2018.04.043
Yang, P., Bastviken, D., Lai, D. Y. F., Jin, B. S., Mou, X. J., Tong, C., et al. (2017). Effects of coastal marsh conversion to shrimp aquaculture ponds on CH4 and N2O emissions. Estuar. Coast Shelf Sci. 199, 125–131. doi: 10.1016/j.ecss.2017.09.023
Zeng, Y., Sarira, T. V., Carrasco, L. R., Chong, K. Y., Friess, D. A., Lee, J. S. H., et al. (2020). Economic and social constraints on reforestation for climate mitigation in Southeast Asia. Nat. Clim. Change. 19, 842–844. doi: 10.1038/s41558-020-0856-3
Zhang, C., Zhang, Y., Luo, M., Tan, J., Chen, X., Ten, F., et al. (2022). Massive methane emissions from tree stems and pneumatophores in a subtropical mangrove wetland. Plant Soil 22:5300. doi: 10.1007/s11104-022-05300-z
Keywords: negative emissions, carbon dioxide removal, blue carbon, mangroves, saltmarsh, seagrass, restoration, carbon offset
Citation: Williamson P and Gattuso J-P (2022) Carbon Removal Using Coastal Blue Carbon Ecosystems Is Uncertain and Unreliable, With Questionable Climatic Cost-Effectiveness. Front. Clim. 4:853666. doi: 10.3389/fclim.2022.853666
Received: 12 January 2022; Accepted: 24 June 2022;
Published: 28 July 2022.
Edited by:
Phil Renforth, Heriot-Watt University, United KingdomReviewed by:
Christopher Robert Pearce, University of Southampton, United KingdomAndreas Oschlies, Helmholtz Association of German Research Centres (HZ), Germany
Copyright © 2022 Williamson and Gattuso. This is an open-access article distributed under the terms of the Creative Commons Attribution License (CC BY). The use, distribution or reproduction in other forums is permitted, provided the original author(s) and the copyright owner(s) are credited and that the original publication in this journal is cited, in accordance with accepted academic practice. No use, distribution or reproduction is permitted which does not comply with these terms.
*Correspondence: Phillip Williamson, cC53aWxsaWFtc29uQHVlYS5hYy51aw==