- 1U.S. Geological Survey, Earth System Processes Division, Denver, CO, United States
- 2U.S. Geological Survey (Emeritus), Earth System Processes Division, Boulder, CO, United States
The spatial distribution and depth of permafrost are changing in response to warming and landscape disturbance across northern Arctic and boreal regions. This alters the infiltration, flow, surface and subsurface distribution, and hydrologic connectivity of inland waters. Such changes in the water cycle consequently alter the source, transport, and biogeochemical cycling of aquatic carbon (C), its role in the production and emission of greenhouse gases, and C delivery to inland waters and the Arctic Ocean. Responses to permafrost thaw across heterogeneous boreal landscapes will be neither spatially uniform nor synchronous, thus giving rise to expressions of low to medium confidence in predicting hydrologic and aquatic C response despite very high confidence in projections of widespread near-surface permafrost disappearance as described in the 2019 Intergovernmental Panel on Climate Change Special Report on the Ocean and Cryosphere in a Changing Climate: Polar Regions. Here, we describe the state of the science regarding mechanisms and factors that influence aquatic C and hydrologic responses to permafrost thaw. Through synthesis of recent topical field and modeling studies and evaluation of influential landscape characteristics, we present a framework for assessing vulnerabilities of northern permafrost landscapes to specific modes of thaw affecting local to regional hydrology and aquatic C biogeochemistry and transport. Lastly, we discuss scaling challenges relevant to model prediction of these impacts in heterogeneous permafrost landscapes.
Introduction
Permafrost, ground remaining below 0°C for more than 2 years, contains roughly a third of the world's soil organic carbon (Schuur et al., 2015; Meredith et al., 2019) and covers nearly one-fourth of the terrestrial Northern Hemisphere (Brown et al., 2002), including much of the boreal forest and tundra biomes (Figure 1). The state of permafrost and the corresponding sequestration of frozen soil organic carbon are not permanent. Disproportionally high rates of warming in northern latitudes increase thermal stress on permafrost, particularly warm permafrost near 0°C (James et al., 2013; Meredith et al., 2019). The fate of permafrost soil organic carbon has received attention in the past decade among the scientific community, greenhouse gas policy strategists, and the general public because its decomposition could result in enhanced emissions of carbon dioxide (CO2) and methane (CH4) to the atmosphere, promoting a positive feedback to atmospheric warming (Schuur et al., 2015). Large-scale model projections of future permafrost C release calculate CO2 and CH4 emissions that are spatially variable across landscapes (Grosse et al., 2016; Xia et al., 2017; McGuire et al., 2018). These projections are limited by several sources of uncertainty (i.e., vegetation shifts, biomass accumulation factors, organic carbon (OC) degradability, N availability, subsidence, and permafrost thaw rates) (Lawrence et al., 2015; Abbott et al., 2016; Schädel et al., 2018) with one of the largest being the response of the lateral hydrologic-C flux that is generally excluded or treated simplistically in large-scale models (Ala-aho et al., 2018; Hugelius et al., 2020; Tank et al., 2020). Although C export from some Arctic permafrost systems is frequently conceptualized as one-dimensional CO2 and CH4 emissions to the atmosphere, permafrost regions are also influenced by C lateral transport including above-ground pathways (e.g., streams) and below-ground pathways (e.g., groundwater) that transport particulate and dissolved inorganic and organic C (Stackpoole et al., 2017; Plaza et al., 2019; Wild et al., 2019). Potential ecosystem effects stimulated by lateral C transport include the release of colored terrestrial dissolved organic matter to the ocean, altering marine primary productivity (Spencer et al., 2009) and the release of stored contaminants associated with permafrost OC, such as mercury, to food webs (Schaefer et al., 2020; Zolkos et al., 2020a). The added complexity of lateral C transport requires comprehensive analyses that integrate hydrologic and biogeochemical considerations (Vonk et al., 2019; Tank et al., 2020). Unlike the atmospheric release pathway of permafrost C, in which high source permafrost C content generally corresponds to high potential C-gas emission, lateral hydrologic C transport potential is jointly influenced by C source and thaw-impacted surface and subsurface hydrologic flowpaths, which are largely unknown. Tank et al. (2020) describe a state factor approach for exploring aquatic biogeochemical responses to permafrost thaw evaluated through regional case studies across the Arctic. They note that challenges in implementing this approach arise in regions with spatial heterogeneity in state factors, particularly in discontinuous permafrost. We posit this is due to the complexities associated with changing surface and subsurface flowpaths, fluxes, and soil-water residence times in transitional continuous to discontinuous permafrost landscapes. This overview explores these complexities through synthesis of recent work in northern latitudes, particularly at the continuous (>90% coverage) to discontinuous (50–90% coverage) permafrost transition, where shifts from predominately shallow, warm-season flow processes toward deeper year-round flow processes are expected to impact hydrologic C lateral transport. Based on this synthesis, we offer a framework for assessing vulnerabilities of water and aquatic C cycles in unstudied Arctic-boreal areas by addressing landscape, subsurface hydrologic, and C source factors that influence coupled hydrologic and biogeochemical responses to five distinct modes of permafrost thaw. Vulnerability assessments that only account for C source and thaw rate miss the potential effects of hydrologic C lateral transport, a large uncertainty of growing relevance in thawing discontinuous permafrost landscapes (Vonk et al., 2019). Science gaps and research opportunities for advancing vulnerability assessments are highlighted.
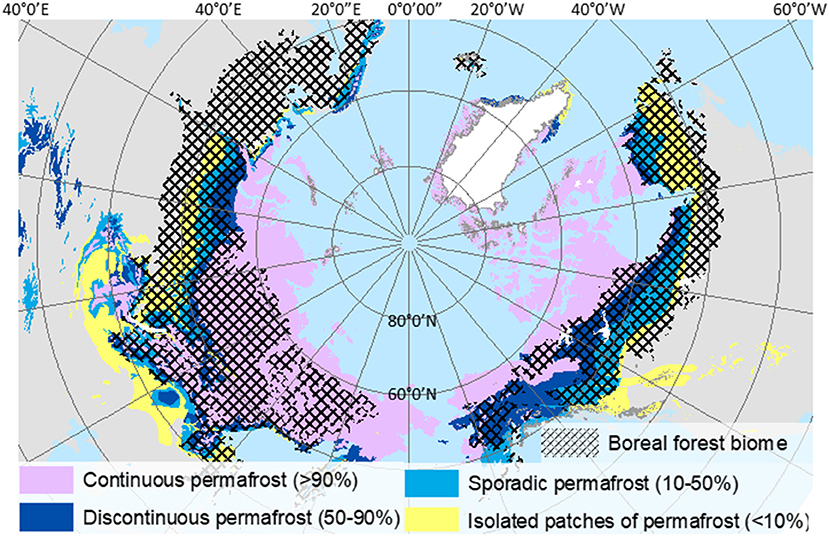
Figure 1. Permafrost coverage in the northern hemisphere (from Brown et al., 2002). Stipled pattern overlay represents the boreal forest biome coverage.
Factors Influencing Vulnerability to Permafrost Thaw
Large-scale losses in permafrost extent are expected by the end of this century (e.g., Zhang et al., 2014; Pastick et al., 2015; McGuire et al., 2018; Parazoo et al., 2018; Meredith et al., 2019). Although there are uncertainties in estimated rates and spatial patterns of permafrost loss, these losses are projected to have a major influence on the strength of boreal regions as net C sinks or sources (Grosse et al., 2011, 2016; Mishra et al., 2013; McGuire et al., 2018; Parazoo et al., 2018; Hugelius et al., 2020), and are highly likely to contribute to climate-related economic damages to infrastructure (Melvin et al., 2017). Ecosystem responses will depend on the mode of permafrost thaw and its associated physical, hydrologic, and biogeochemical impacts. It is therefore critical to distinguish among primary modes of permafrost thaw and identify landscape characteristics that influence susceptibility to each thaw mode.
Modes of Permafrost Thaw and Constraints on Detection
Active layer thickening is described by thaw occurring at the top of permafrost that effectively increases the thickness of the layer that seasonally oscillates in temperature above and below 0°C (Figure 2A). Active layer thickening tends to be observed as a gradual (press) response to northern latitude atmospheric warming trends and/or other near-surface perturbations with reported thaw rates generally not exceeding a few centimeters per year in areas of occurrence (Streletskiy et al., 2015). Repeat point measurements of the active layer over time can be made to determine thickening via manual frost probing, temperature monitoring, and geophysical techniques (Briggs et al., 2017; Douglas et al., 2020). Support for uniform active layer thickening is lacking due to sparse historical data and/or complex and potentially counterbalancing interactions among factors controlling near-surface thermal conditions such as soil properties, snow cover, moisture, and vegetation (Park et al., 2013; Clayton et al., 2021). The lack of a universal protocol that accounts for change in ground surface elevation datum in settings where ground subsidence and compaction accompany thaw also contributes to inconsistencies in active layer observations (Rodenhizer et al., 2020). Integrated modeling and analysis frameworks combining field and remote sensing observations, from both airborne radar and global satellite platforms, continue to improve estimates of active layer thickening (e.g., Yi et al., 2018; Chen et al., 2020).
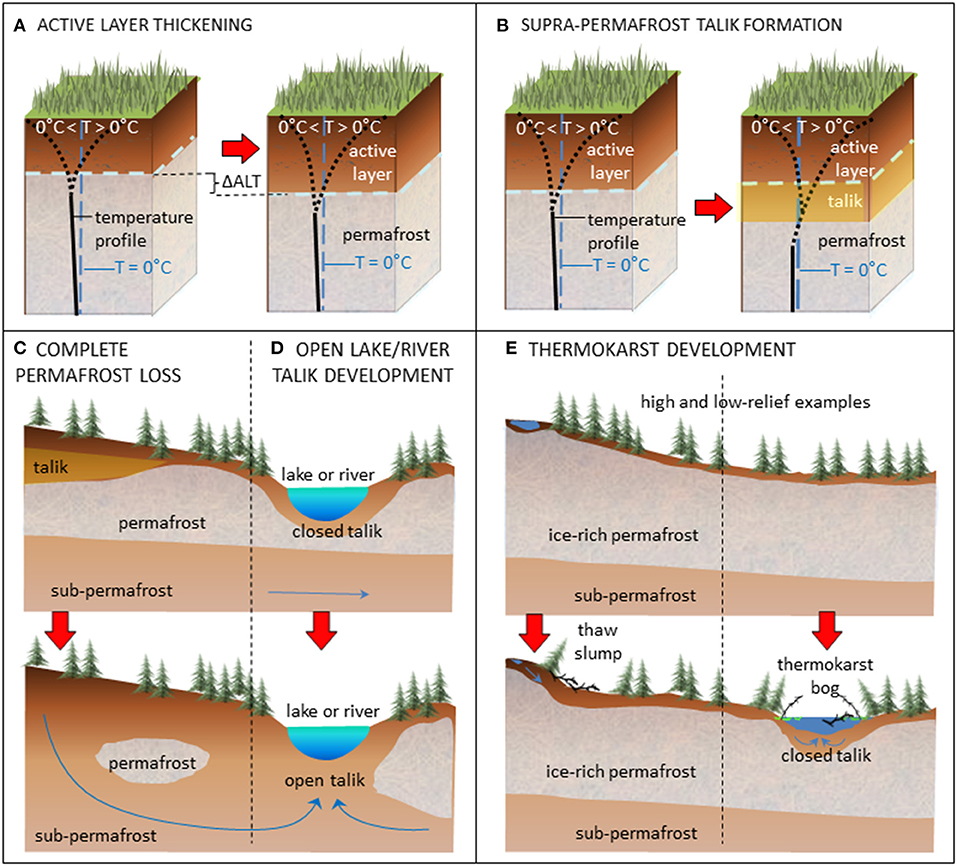
Figure 2. Five primary modes of permafrost thaw (A–E) modified from Kurylyk and Walvoord (2021). Red arrows point in the direction of thaw evolution from one state (left or above) to the next (right or below). ΔALT in (A) = change in active layer thickness. Blue arrows in (C–E) indicate the direction of subsurface water flow.
The progression from active layer thickening to supra-permafrost talik formation occurs when the depth to permafrost exceeds the depth of seasonal freezing. This perennially-thawed zone, or talik, typically develops (Figure 2B) over timescales of a few decades (press response) and can reach depths as great as several meters (Streletskiy et al., 2015; Connon et al., 2018). Perturbations, such as wildfire and flooding, can accelerate these processes, promoting talik development within a few years post disturbance (Grosse et al., 2011; Brown et al., 2015; Jepsen et al., 2016; Minsley et al., 2016). Taliks may be spatially isolated (e.g., Rey et al., 2020) or may be laterally extensive and hydrologically connective (Gibson et al., 2018; Terry et al., 2020; Ackley et al., 2021), a distinction that is critical in determining their hydrologic consequences. Taliks and their connectivity are difficult to characterize across broad areas because current permafrost mapping efforts and capabilities at large spatial scales tend to be restricted to near-surface conditions that are shallower than the depths of talik formation (O'Neill et al., 2020). Furthermore, shallow permafrost analysis using satellite and airborne remote sensing data typically employ the assumption that the seasonally-thawed active layer lies directly above permafrost with no perennially-thawed layer. Detection of aufeis, or icings, through remotely sensed optical and thermal imagery and their connection to groundwater upwelling provides a promising means of scoping taliks at large scales (Glass et al., 2020). Permafrost thermal modeling suggests that lateral taliks will become increasingly prevalent across Arctic and boreal regions in the coming decades (Parazoo et al., 2018) and serve as a precursor to accelerated (and irreversible) thaw (Devoie et al., 2019; Walvoord et al., 2019).
Complete permafrost loss refers to broad degradation in a terrestrial setting (Figure 2C). Open lake/river talik development also involves the elimination of permafrost at depth but is distinguished here as underlying a lake or river (Figure 2D). Detection and monitoring of complete permafrost loss and open taliks require deep-seeing subsurface characterization methods to confirm complete thaw and/or quantify rates of loss with time lapse information. The depth of interrogation required for such determination depends on the maximum depth of permafrost and can range from 100 to 103 m, limiting extensive characterization. Open taliks beneath lake and river corridors surrounded by thick permafrost have been inferred from airborne electromagnetic surveys (Minsley et al., 2012; Rey et al., 2019) and thermal modeling (Rowland et al., 2011; Wellman et al., 2013). Complete permafrost degradation over land or under water bodies tends to occur gradually (press response) over timescales of centuries or greater (McKenzie and Voss, 2013; Wellman et al., 2013) except in very thin, warm permafrost where complete loss can occur more rapidly (Quinton et al., 2011). Rates of open talik development beneath water bodies are typically greater than beneath terrestrial settings; both can be accelerated if heat transfer via groundwater flow contributes to thawing.
When ice-rich permafrost thaws, reduction of pore space associated with ice melt can cause deformation of the ground surface via notable subsidence and/or erosion, resulting in various forms of thermokarst development (Figure 2E) (Jorgenson and Osterkamp, 2005; Jorgenson, 2013). Though minor ground subsidence may occur upon permafrost thaw in areas of low permafrost ice content, high ice content (ice content in excess of thawed porosity) is a key element controlling thermokarst potential (Vonk et al., 2019; Saito et al., 2020). Thaw-driven subsidence in ice-rich permafrost landscapes produces distinct thermokarst features including thaw lakes, drained basins, water tracks, and bogs and gullies in low relief settings. In settings with moderate to high topographic relief, thaw slumps and active-layer detachment slides commonly occur and develop rapidly through a summer season (pulse response). The ability to remotely detect thermokarst features continues to improve with advances in sensor capability, multi-sensor data fusion, and data processing including synthetic aperture radar interferometry (Jorgenson and Grosse, 2016; Nitze et al., 2018, 2020; Abe et al., 2020).
Climatic and Landscape Characteristic Controls on Thaw Susceptibility and Rate
Permafrost distribution is primarily controlled by coupled climatic and terrain factors that influence heat exchange between the atmosphere and the ground surface (Jorgenson et al., 2010). Because paleoclimate and glacial history have a legacy effect, permafrost is not commonly in equilibrium with the current near-surface energy balance, thereby requiring considerations for mapping its distribution, thickness, and depth (Zhang et al., 2014). Large discrepancies of nearly five-fold in estimated current permafrost distribution account for some of the high uncertainties in projected permafrost loss reported by the 2019 IPCC report (Meredith et al., 2019). For instance, 30–99% (69 ± 20%; likely range) permafrost loss is projected under Representative Concentration Pathway (RCP) 8.5. The projected loss range and associated uncertainty is substantially reduced for all RCP scenarios for the subset of models that estimate similar current permafrost distributions. Modeling approaches that consider paleoclimate conditions with extended spin up durations and/or infusion of ground-truth data at multiple scales can effectively constrain initial permafrost conditions necessary for Earth System Model projections forced by Global Climate Model results for various emission scenarios, thus reducing the uncertainty in permafrost thaw predictions (McGuire et al., 2018).
Increasing air temperature translated to increasing ground temperature is the most noted and obvious driver of permafrost thaw. Changes in precipitation magnitude and seasonality can also influence ground temperatures (both positively and negatively), especially the timing and duration of snow cover that acts as an insulator to cold winter air temperatures (Jorgenson et al., 2010; Jafarov et al., 2018). Above-average summer precipitation can promote shallow thaw of warm permafrost as wet soils, with higher specific heat and bulk thermal conductivity, transfer thermal energy more effectively than dry soils. Douglas et al. (2020) report an average permafrost thaw rate of 0.7 ± 0.1 cm per cm increase in rainfall in boreal Alaska, with disturbed sites showing the greater thaw response to enhanced summer rain. Disturbance phenomena, such as fire (Brown et al., 2015; Minsley et al., 2016; Rey et al., 2020), flooding (Jepsen et al., 2016), vegetation change (Briggs et al., 2014), and infrastructure (Ghias et al., 2017) can further interact to modify the surface energy balance and shallow soil properties, which in turn influence permafrost dynamics, generally resulting in enhanced thaw rates in disturbed landscapes relative to their undisturbed counterparts. Wildfire is a prominent disturbance feature that shapes the carbon dynamics of northern ecosystems (Bond-Lamberty et al., 2007) by promoting permafrost thaw through the partial or complete combustion of the organic soil layer that provides insulation from warm summer temperatures as well as through the reduction in summer shading (Yoshikawa et al., 2002). Increases in wildfire activity and intensity have been documented and are projected to continue (Kasischke and Turetsky, 2006; Meredith et al., 2019), resulting in subsequent effects on permafrost thaw and lateral C export in both boreal and tundra regions (Gibson et al., 2018; Abbott et al., 2021; Ackley et al., 2021).
At the landscape scale, permafrost thaw responses to near-surface drivers typically display more spatial variability than that observed in the drivers themselves. This spatial mismatch in driver vs. response variability is due to prominent roles of landscape and subsurface characteristics, typically heterogeneous in nature, in mediating thaw rate and the coupled hydrologic and biogeochemical responses (Tank et al., 2020). Table 1 describes positive, neutral, and negative associations between thaw likelihood/rate and various landscape and subsurface characteristics based on observational and modeling studies. Primary landscape features that influence thaw can generally be mapped via remote sensing methods, although ground-based measurements are needed for calibration and validation. In contrast, many influential subsurface characteristics cannot be determined remotely with current technology and thus require ground-based techniques such as coring or excavation to produce point-scale measurements. Geophysical techniques offer intermediate-scale characterization that can help bridge major gaps between point and remotely-sensed measurements (Minsley et al., 2012; Briggs et al., 2017). Tradeoffs between resolution and spatial coverage as well as tradeoffs between resolution and depth of investigation can be balanced through multi-method geophysical and remote sensing approaches (Walvoord and Kurylyk, 2016).
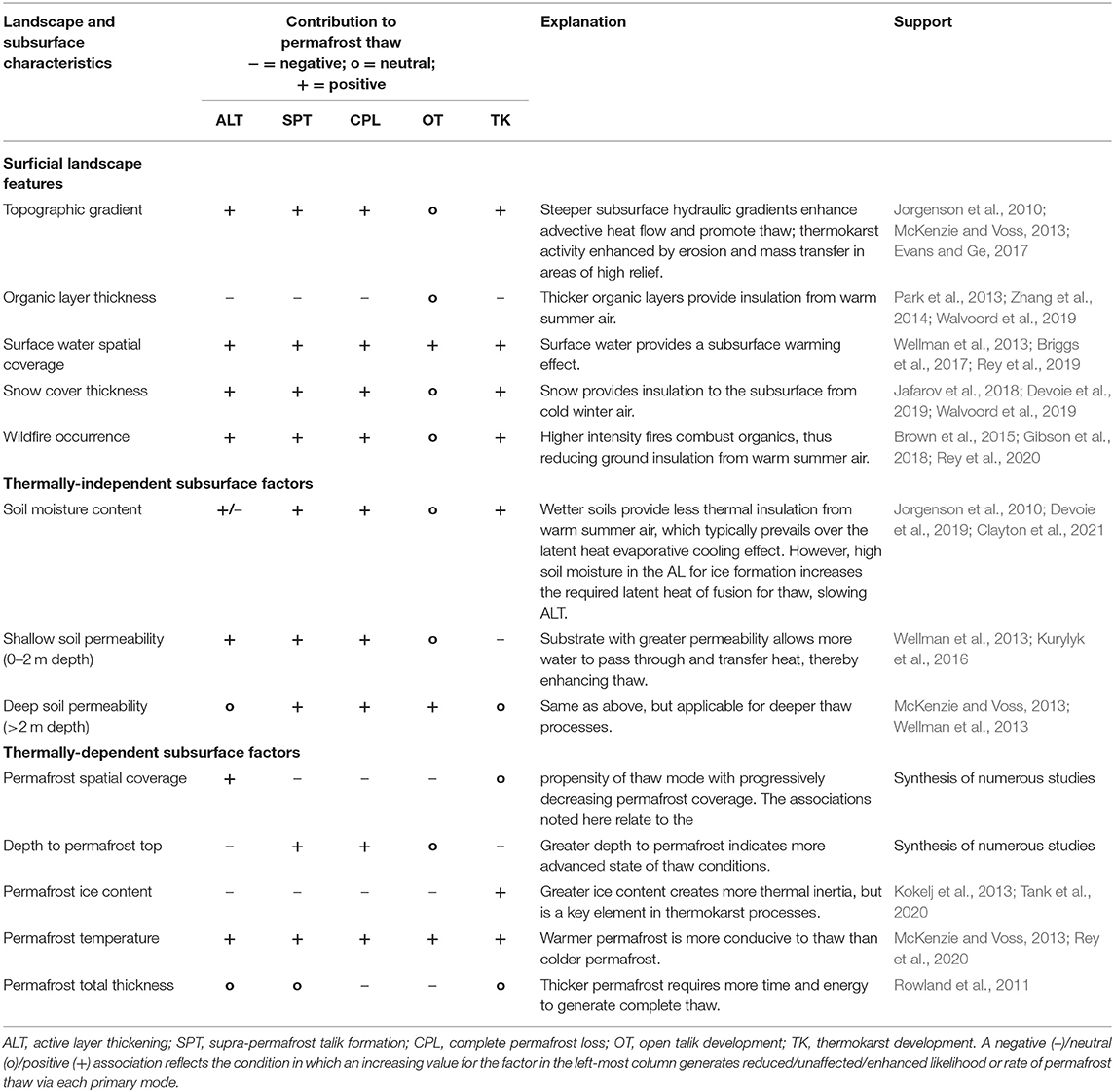
Table 1. Influence of landscape and subsurface characteristics on permafrost thaw vulnerability to each primary mode of thaw.
Active layer thickening, supra-permafrost talik formation, and complete permafrost loss generally show similar associations to thaw-influential factors shown in Table 1 because these modes represent a continuum. Deeper subsurface properties become increasingly more relevant in the progression from active layer thickening to complete permafrost loss. Permafrost ice content has a negative association to most modes of thaw because greater volumetric ice content requires more thermal energy to complete the phase change to liquid water. Though the same thermodynamics apply to thermokarst processes, by definition, the development of thermokarst landforms relies on the existence of ice volume that exceeds the soil matrix pore space allowing for ground subsidence and/or slumping upon thaw. Accordingly, permafrost ice content has a positive association with thermokarst formation. Permafrost coverage is an important factor in determining propensity to the primary permafrost thaw modes. Consistent with the thaw state progression from active layer thickening to supra-permafrost talik development to complete loss beneath both terrestrial and aquatic systems, the least advanced thaw stage is more likely to be associated with areas with high (continuous) permafrost coverage whereas the more advanced stages are more common in areas with progressively less (discontinuous to sporadic) permafrost coverage.
Hydrologic and Aquatic Carbon Responses to Permafrost Thaw
Hydrologic susceptibilities and effects on aquatic C transport strongly depend on the mode and occurrence of thaw with respect to permafrost coverage (Striegl et al., 2007; Pokrovsky et al., 2015). Aquatic carbon biogeochemistry, transport and storage are tightly linked with water availability, flowpath and residence time. Permafrost C includes: soil organic carbon as particulate organic carbon (POC) and dissolved organic carbon (DOC); carbonate minerals; and dissolved inorganic carbon (DIC). Soil organic carbon receives the most attention because of its large magnitude and potential to eventually mineralize to the greenhouse gases CO2 and CH4 (Hugelius et al., 2014, 2020; Schuur et al., 2015). In the context of the water cycle, POC and DOC thawed from permafrost have three general fates depending on form, chemical composition and decomposability: (1) mineralization in-place or at some downgradient location; (2) lateral transport downgradient by surface and/or subsurface water; and (3) storage in-place or downgradient. Permafrost and seasonally frozen ground are barriers to vertical and horizontal subsurface water flow, so subsurface decomposition and transport diminish, and storage prevails when soil organic carbon is frozen. When surface runoff occurs, organic carbon export commonly derives from current vegetation sources with limited permafrost contributions (Dean et al., 2020; Beel et al., 2021). Dissolved inorganic carbon, the largest component of aquatic carbon exported to oceans, derives from mineralization of modern and permafrost organic carbon, exchange of CO2 with the atmosphere, and dissolution (weathering) of carbonate minerals. DIC concentration is equal to the sum of the bicarbonate ion, carbonate ion, carbonic acid, plus dissolved CO2 [CO2(aq)] concentrations. For most streams, rivers, and lakes, bicarbonate is the prevalent species. Permafrost thaw makes frozen carbonates in permafrost available for downstream transport and/or weathering enhancing transport and downstream storage of particulate inorganic carbon (PIC) in stream and river channels and the production and export of DIC, primarily as bicarbonate. Mineralization to CO2 is also a potential fate for thawed carbonates. These processes are observed at the river basin scale across the circumboreal region (Striegl et al., 2005, 2007; Tank et al., 2012a,b; Tank et al., 2016) and locally, especially near slopes having retrogressive thaw slumps (Zolkos et al., 2018). Anticipated trajectories of hydrologic and aquatic carbon responses to specified modes of permafrost thaw are described below and are summarized in Table 2.
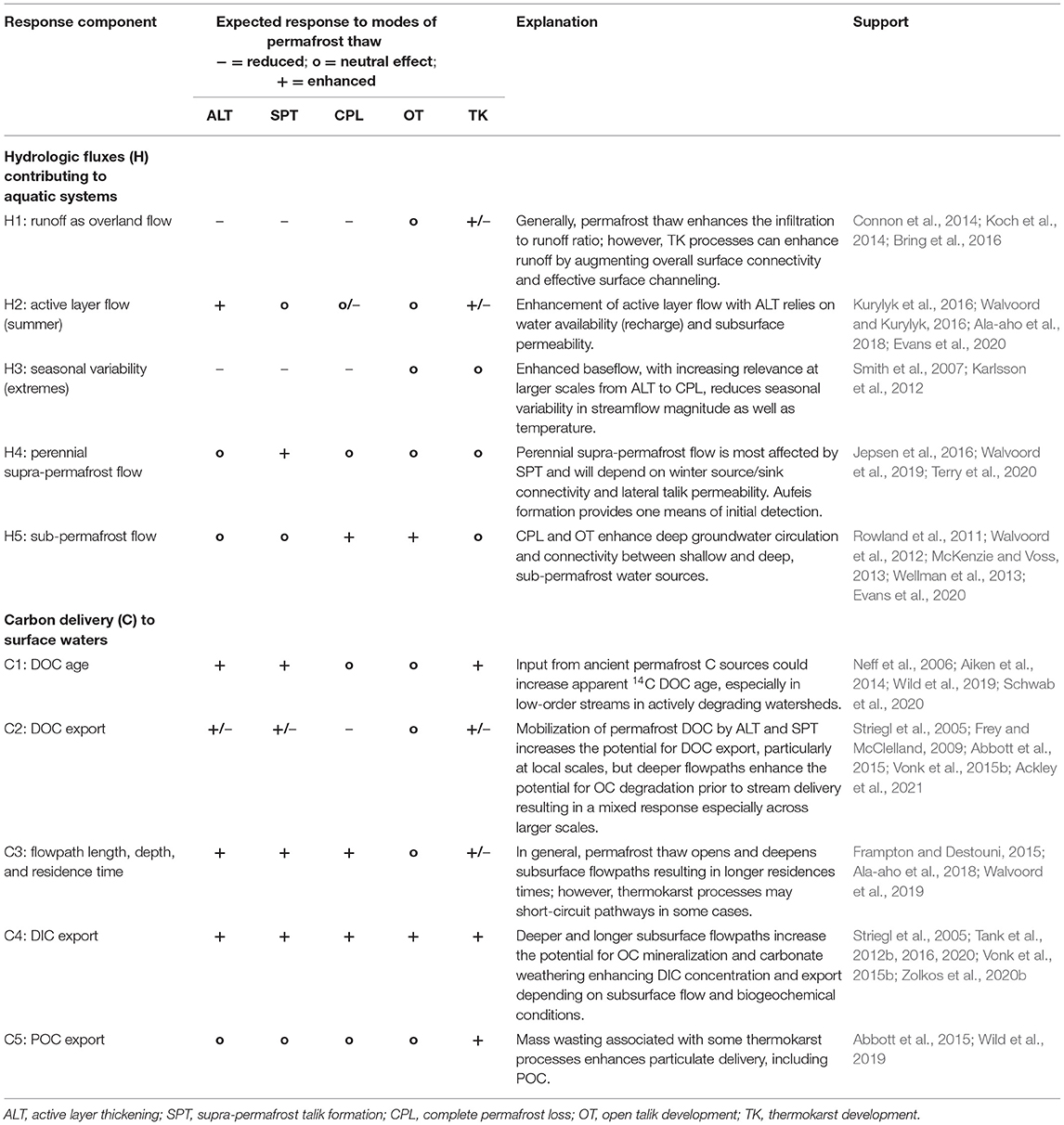
Table 2. Summary of expected hydrologic and aquatic carbon responses to specified modes of permafrost thaw.
Response to Active Layer Thickening
Thickening of the active layer increases potential shallow groundwater storage and promotes infiltration, thereby reducing runoff via overland flow and deepening subsurface flowpaths (Table 2, H1, H2). Collectively, these responses act to lengthen delivery times of water and solutes to stream and river networks (Ala-aho et al., 2018). This reduction in runoff mediated by top-down permafrost thaw, including active layer thickening, generally promotes a reduction in seasonal streamflow variability as the subsurface acts as a low-pass filter for water delivery to streams (Table 2, H3). An exception to the pattern of reduced runoff to active layer thickening can occur in low-lying landscapes where thaw is accompanied by ground subsidence and surface channeling that increases surface hydrologic connectivity (Connon et al., 2014) and reduces transit time. Lateral supra-permafrost flow increases with active layer thickening unless the change is accompanied by a water table decline that causes water movement downward through a newly thawed zone with reduced permeability relative to material above (Koch et al., 2014; Walvoord and Kurylyk, 2016). Hydrologic susceptibility to active layer thickening is greatest in regions where subsurface flow is predominantly through the active layer, such as in continuous permafrost or in high-altitude discontinuous permafrost having shallow unfractured bedrock (Bring et al., 2016). Regions where much subsurface flow occurs via deeper flowpaths (i.e., taliks and sub-permafrost aquifers) are less impacted by subtle changes in active layer flow conditions.
Active layer thickening makes the uppermost centimeters of permafrost OC available for decomposition or transport as DOC. There is evidence that ancient DOC decomposes quickly following thaw, so a large fraction of DOC released by active layer thickening may mineralize near the source (Drake et al., 2015; Vonk et al., 2015a). In the few instances where radiocarbon ages have been measured on surface water DOC exported from permafrost terrain, aged DOC is detectable, particularly late in the thaw season (Neff et al., 2006; Striegl et al., 2007) but the signal is small and likely to diminish quickly due to high biolability (Vonk et al., 2013; Wickland et al., 2018) and/or dilution by modern C sources (Aiken et al., 2014; Dean et al., 2020; Schwab et al., 2020; Table 2, C1). Studies in northern permafrost regions indicate a mixed response in DOC riverine export to active layer thickening (Vonk et al., 2015b; Table 2, C2). Coupled hydrologic and biogeochemical conditions favoring increased export include release of slow-reacting recalcitrant DOC and rapid transit times through lateral subsurface flowpaths. However, increased downward transport of DOC from terrestrial and permafrost sources and longer subsurface residence times typically associated with active layer thickening (Frampton and Destouni, 2015; Table 2, C3) tends to enhance DOC consumption or sorption resulting in a net reduction of DOC export, particularly at larger scales (Striegl et al., 2005). Thawed conditions promote longer subsurface residence time for microbial mineralization of DOC, increased respiration, and increased DIC production through mineral weathering (Table 2, C4). This balance between DOC consumption and DIC production is confounded by vegetation changes associated with climate warming resulting in both increased DOC production by new vegetation and increased consumption of that DOC in the subsurface that alter the hydrologic DOC and DIC balance (Dornblaser and Striegl, 2015).
Response to Supra-Permafrost Talik Development
Hydrologic and biogeochemical susceptibilities for supra-permafrost talik development are similar to active layer thickening but include seasonal extension and moderation of flow variability (Table 2, H1–4 and C1–4), due to the potential year-round persistence of supra-permafrost flow even as winter ice forms above in the active layer. This allows for perennial reactivity and transport of C in the shallow subsurface through progressively deeper flowpaths (Walvoord et al., 2019). Increased seasonal flow duration can result in increased supra-permafrost delivery of DOC, DIC, CO2(aq) and CH4(aq) to inland waters, while relatively slow water movement in the subsurface also increases residence time for DOC mineralization and production of DIC and C-gases. The amounts of water and dissolved C that can be laterally transported during winter by supra-permafrost taliks depend strongly on talik permeability and the capacity of the system to maintain hydraulic gradients and subsurface connectivity to drive flow, conditions that are challenging to both characterize in the field and to represent in large-scale models. The presence of aufeis, or icings, can be useful in detecting substantial perennial supra-permafrost talik flow (Glass et al., 2020; Terry et al., 2020). The importance of supra-permafrost flow and the concomitant delivery or subsurface biogeochemical processing of permafrost C resulting from supra-permafrost taliks is a research area of required attention (Commane et al., 2017; Vonk et al., 2019). Plaza et al. (2019) directly measured carbon loss in permafrost soils over a 5-year warming experiment and pointed to lateral hydrologic export as a pathway potentially accounting for more than half of the large losses measured. Dissolved C transport through lateral taliks could help explain the large observed soil carbon losses. Likewise, Schwab et al. (2020) attribute a pulse of aged DOC, determined by radiocarbon activity in DOC, in the northern Mackenzie River basin of Canada, to lateral transport of thawed permafrost C through newly developed supra-permafrost taliks following several warm summer and winter seasons. The coupled hydrologic and biogeochemical role of lateral taliks remains poorly quantified and is likely to increase in importance with time and continued subsurface warming (Vonk et al., 2019; Walvoord et al., 2019).
Response to Complete Permafrost Loss
Areas of warm, thin, discontinuous permafrost are most likely to experience complete permafrost degradation. From an ecological perspective, complete loss of near-surface (~3–4 m below the surface) permafrost is most pertinent, whereas from a hydrogeological perspective, complete permafrost loss (typically > 4 m) that establishes subsurface connectivity is most relevant. Complete permafrost loss has the potential to influence subsurface fluxes and flowpaths at intermediate to basin-wide scales by altering the hydrogeologic framework that controls groundwater flow, groundwater storage, and hydrologic connectivity among inland waters, shallow groundwater, and deep sub-permafrost groundwater. Widespread increases in streamflow minimums and river baseflow across the Arctic-boreal region suggest a multi-decadal shift from dominance of surface-water input toward increased groundwater input from a combination of connective supra-permafrost flow and sub-permafrost flow through open taliks (Smith et al., 2007; Walvoord and Striegl, 2007; Evans et al., 2020). This is consistent with the paradigm that proportionally more water is being recharged, stored, and circulated through deep subsurface pathways opened by extensive permafrost loss (Walvoord et al., 2012; Kurylyk and Walvoord, 2021). This conceptual model does not require enhanced precipitation or additional recharge from meltwater sourced by thawing permafrost or glaciers, but simply involves the rerouting of some surface water through deeper subsurface flowpaths, enhancing baseflow and reducing seasonal streamflow variability (Table 2, H1–5). Determination of the hydrologic impacts of complete permafrost loss requires site-specific consideration due to the strong influence of the underlying (unfrozen) hydrogeologic structure. For example, transitions from frozen to unfrozen coarse-grained sediment with high thawed permeability will invoke greater hydrogeologic changes than transitions from frozen to unfrozen bedrock with low thawed permeability. Anticipating broad changes in groundwater contributions to inland waters via permafrost loss and corresponding changes in flowpaths and water residence times will necessitate improved baseline hydrogeologic characterization of permafrost-impacted basins (i.e., airborne geophysics; Minsley et al., 2012) integrated with coupled permafrost and groundwater modeling. Some permafrost-impacted regions may demonstrate enhanced potential for groundwater resource development upon continued permafrost thaw (i.e., Lemieux et al., 2016).
Permafrost degradation over long timescales will affect aquatic C dynamics at all scales as hydrologic conditions shift from surface-water dominated to more groundwater influenced and residence times increase (Frey and McClelland, 2009; O'Donnell et al., 2012). Long-term consequences of permafrost thaw include increased downward movement of DOC from surface, shallow subsurface, and permafrost sources. This results in increased subsurface OC mineralization, rock weathering and bicarbonate production, enhancing DIC export to oceans (Striegl et al., 2005; Tank et al., 2012b; Table 2, C2, C4). Increased thaw and warmer soil conditions will likely also result in vegetation shifts and increased DOC production and export from non-permafrost sources (Tank et al., 2012a, 2016; Dornblaser and Striegl, 2015).
Response to Open Talik Development Beneath Water Bodies
Open taliks beneath large lake and river corridors within continuous and discontinuous permafrost regions can enhance surface water and sub-permafrost groundwater exchange (Wellman et al., 2013; Table 2, H5) and promote baseflow in large rivers throughout the winter. The direction of the exchange (drainage out or seepage in) depends on regional hydraulic gradients. For example, lakes that are topographically high on the landscape tend to be subjected to downward gradients and thus drain to the deeper groundwater system through open talik development, whereas topographically low lakes tend toward upward gradients that support seepage into the lakes through open talik development. However, local and regional gradients influenced by permafrost distribution are not well known without detailed field investigation, and hydraulic gradients can change in response to permafrost thaw. This complicates the expected hydrologic response associated with open talik processes.
Talik formation below water bodies exposes permafrost OC to decomposition and likely has consequences on lake CO2 and CH4 production and DOC composition. High proportions of anaerobic C mineralization near the thaw front of lake taliks have been observed (e.g., Heslop et al., 2020), with up to 80% of CH4 lake emissions being associated with recent (decadal-scale) permafrost thaw (Walter Anthony et al., 2021). Lakes overlying thawed permafrost are known to have high CH4 emission, largely by ebullition (Walter Anthony et al., 2021) but other aqueous C consequences are not well characterized. Lake hydrological models suggest that increased subsurface connectivity among lakes will also lead to increased similarity in lake solute chemistry (Jepsen et al., 2016). Similar to other permafrost modes, open talik formation generally promotes deeper and longer subsurface flowpaths with increased time for OC mineralization and carbonate weathering, leading to enhanced DIC export (Table 2, C2–4).
Response to Thermokarst Formation
The numerous types of thermokarst development and complex geomorphic changes provide considerable challenge for the prediction of hydrologic responses. Thermokarst processes alter topography and erosional potential in moderate to steeply sloping watersheds and may lead to large mass wasting and dramatic increases in sediment and organic particulate transport to streams and rivers (Figures 3A,B). In relatively flat regions, localized thermal erosion (Figure 3C) and subsidence from thermokarst development may result in increases in surface water area (bog, pond, and lake development) (Figure 3D) (Walter Anthony et al., 2021) or loss of net surface water area (Nitze et al., 2018) due to increases in surface water drainage as thermokarst development evolves and the surface water connectivity increases (Connon et al., 2014). Episodic thermokarst events can result in the formation of new lakes or loss of old lakes in days to weeks, dramatically altering the ecosystem services associated with those waterbodies (Nitze et al., 2020).
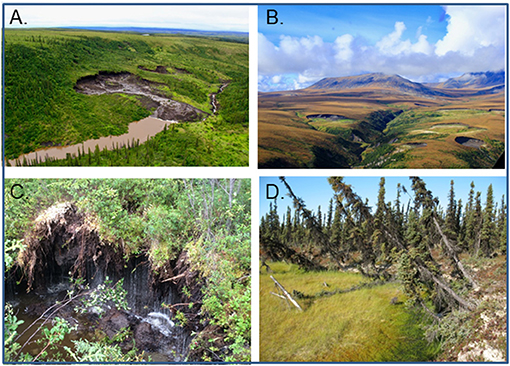
Figure 3. Thermokarst processes affecting the storage and delivery of water and carbon across boreal landscapes. These include: (A) thaw slumps and enhanced particulate matter to stream input and (B) collapse and drainage features on the Peel Plateau, Northwest Territories, Canada, (C) active thermally-driven erosion pervasive in the Erickson Creek watershed, Alaska, USA, and (D) subsidence and bog formation in Innoko Flats, Alaska, USA. Photo credits: Scott Zolkos, Woodwell Climate Research Center (A and B); Kim Wickland, U.S. Geological Survey (D).
Thermokarst development can have a dramatic and visual effect on aquatic C dynamics. Mass wasting of hillslopes and shorelines contributes large amounts of POC, aged DOC, nutrients and sediment with short residence times into lakes and streams (Figure 3A; Abbott et al., 2015; Wild et al., 2019; Table 2 C1–C3, C5). These inputs tend to be biogeochemically reactive on short time scales affecting aquatic ecosystem respiration, primary production, mineral weathering, greenhouse gas emissions and other processes (Vonk et al., 2015a,b; Walter Anthony et al., 2021). Subsurface organic C buried and frozen for centuries to millennia can be respired quicky to enhance stream CO2 emissions when exposed by retrogressive thaw slumps (Zolkos et al., 2019). Countering this process, carbonic acid weathering of carbonate minerals exposed by thermokarst and erosion decreases stream and lake CO2 emissions while increasing bicarbonate production and transport (Tank et al., 2016; Zolkos et al., 2018, 2020b; Table 2, C4). This pattern is complicated in regions where sulfuric acid weathering of carbonates produces CO2 (Zolkos et al., 2018), emphasizing the need to understand local geological and geochemical conditions when assessing the role of permafrost thaw on aquatic carbon cycling. Carbon storage is a component of POC delivery to inland waters via thermokarst processes. POC released by thermokarst can be transported downstream to coastal regions, lakes, or wetlands where duration of storage is a function of POC biodegradability and oxygen conditions in bottom sediments at the relocation sites (Beel et al., 2020).
Accounting for Competing Influences and Heterogeneity in Permafrost C Response to Thaw
Because of the importance of changing hydrologic conditions in thawing permafrost landscapes as described in this section, the primary pathways of permafrost carbon and trajectories of C export (Table 2) depend on joint consideration of factors influencing subsurface water flux (permeability, gradient, hydrologic connectivity, and sustained water source) (Figure 4A) and carbon source (chemical composition, amount, and OC degradability) (Figure 4B), in addition to factors that control modes and rates of permafrost thaw, described in section Factors Influencing Vulnerability to Permafrost Thaw (Table 1, Figures 4C,D). Challenges in assessing permafrost C pathways (Figure 4E) arise because landscape and subsurface characteristics that influence subsurface water flux, carbon source, pulse and press thaw (Figures 4A–D) are not commonly aligned. For example, as illustrated in Figure 4, systems that have among the greatest potential carbon source characteristics, such as low-lying thick peatlands and silty uplands and lowlands (Vonk et al., 2015a,b; Wickland et al., 2018), have limited potential for lateral subsurface water flux upon thaw due to low hydraulic gradient or low thawed permeability (Kurylyk et al., 2016; Ebel et al., 2019). Similarly, rocky or sandy uplands, with the greatest potential for lateral subsurface hydrologic flux upon thaw (Evans and Ge, 2017; Ebel et al., 2019), have limited carbon source potential (Saito et al., 2020). Incorporation of joint potentials is thus required for estimating the magnitude of the pathways for permafrost carbon fate via lateral dissolved or particulate pathways or release to the atmosphere as gas (Figure 4E, Table 2). Evidence for enhanced subsurface connectivity and lateral flowpath development accompanying thaw in discontinuous permafrost landscapes continues to mount (Connon et al., 2018; Parazoo et al., 2018; Rey et al., 2019) suggesting an increasing potential for lateral hydrologic C transport. Models that do not consider lateral hydrologic C flux may underestimate dissolved C delivery to inland waters in response to permafrost thaw and overestimate C gas released to the atmosphere.
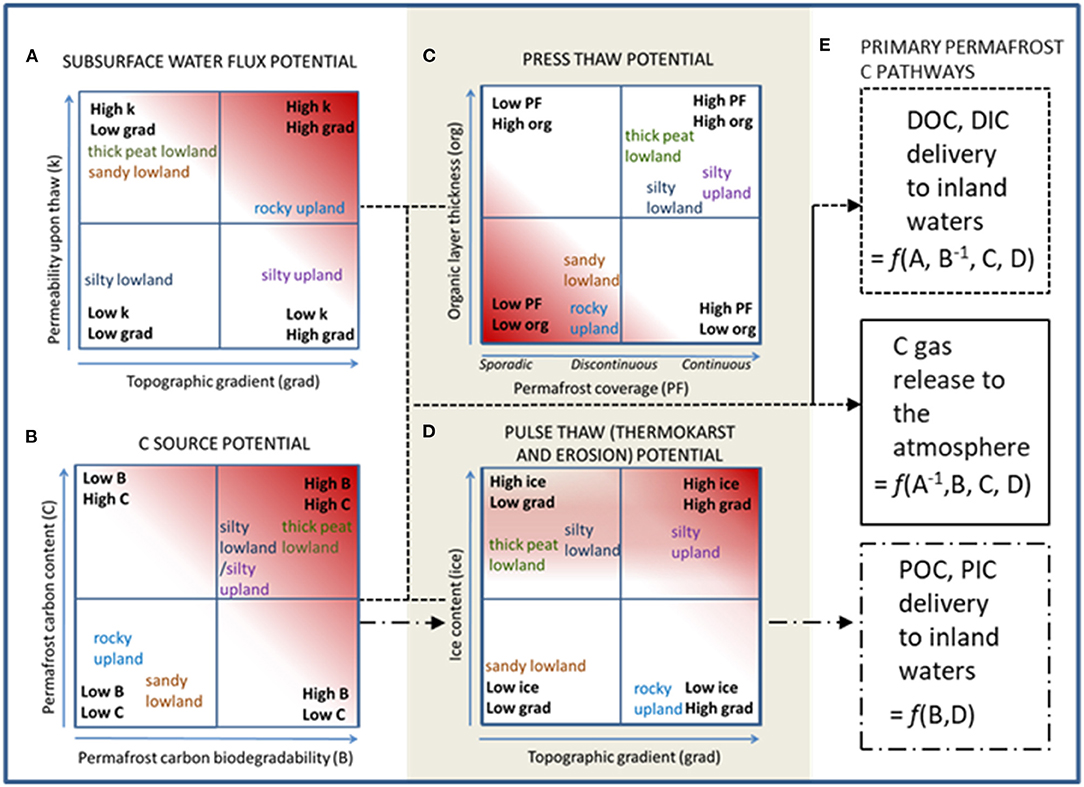
Figure 4. Framework depicting primary characteristics influencing (A) subsurface hydrology (B) permafrost carbon source strength, and (C,D) permafrost thaw, and the collective effect on (E) the fate of permafrost carbon delivered to coastal and inland waters where it is mineralized and/or stored in sediments. Red shading represents conditions with the highest potential for (A) lateral hydrologic flux, (B) carbon source strength, (C) press thaw, and (D) pulse thaw. Representative, generalized hydrogeologic landscapes (thick peat lowland, sandy lowland, silty upland, silty lowland, rocky upland) are noted in colored text and are provided to demonstrate the non-alignment of hydrologic, biogeochemical, and thaw potentials.
Additional challenges toward the goal of projecting hydrologic and biogeochemical change in northern latitudes arise from the substantial degree of spatial heterogeneity in landscape and subsurface characteristics that give rise to the discontinuous permafrost distribution typical of these regions. Consequently, aquatic C and hydrologic responses to climate change in discontinuous permafrost also tend to display a high degree of heterogeneity (Tank et al., 2020). Furthermore, these regions are subject to all five primary modes of thaw, thus elevating the complexity of conceptual model representation. The nature of discontinuous permafrost and thaw evolution in these systems heightens the impact of subsurface flow and C transport relative to continuous permafrost where water movement and carbon cycling are mainly confined to the active layer. The impact of continued thaw toward sporadic and isolated permafrost coverage lessens as the capacity to affect hydrologic and biogeochemical processes and pathways becomes increasingly diminished. Thaw occurring in regions near the continuous-discontinuous permafrost transition is increasingly recognized as having the highest potential for hydrogeologic impact as subsurface connectivity evolves non-uniformly (Rey et al., 2019). Computational limitations and current characterization gaps have justified major simplification of subsurface lateral flow processes in large-scale Earth System Models (ESMs) used to predict changes in water and carbon dynamics of thawing permafrost regions. Major advances must overcome these challenges. Establishing guidelines for the required spatial and vertical resolution of influential landscape and subsurface characteristics controlling thaw and its hydrologic and biogeochemical impact in diverse Arctic and boreal regions is an important step in this direction.
Conclusions
Carbon cycling is largely driven by water availability, phase and movement. As permafrost thaws, water and carbon become available for increased biogeochemical processing and transport via deeper subsurface pathways. Locations, landscape and subsurface factors, and modes of thaw will determine the ultimate consequences of thaw with complexities arising in part because characteristics influencing thaw are not necessarily in line with characteristics influencing coupled hydrologic and biogeochemical responses. Shallow permafrost thaw processes (active layer thickening and thermokarst formation) have proportionally large hydrologic and C-cycle impacts in systems with continuous permafrost coverage, whereas systems having discontinuous to sporadic permafrost coverage tend to be progressively more impacted by deeper permafrost thaw processes, including supra-permafrost talik development and complete permafrost loss. A major challenge for projecting the fate and transport of northern permafrost C in the twenty-first century and beyond is addressing change resulting from permafrost thaw in water flowpaths, fluxes, and availability. Vulnerability depends not only on carbon source (composition, abundance, reactivity) and likelihood of permafrost thaw, but also on factors and drivers sensitive to subsurface water movement, hydrologic connectivity, and the availability of water to drive and sustain flow and the transport of dissolved and particulate C. Improving projections of permafrost C release for future planning in northern boreal regions requires strong, general understanding of the timescales associated with various modes of permafrost thaw, a framework describing observable factors in the context of permafrost thaw likelihood (Table 1), joint hydrologic and biogeochemical considerations (Figure 4), and understanding of the expected trajectories of hydrologic and aquatic C responses to thaw (Table 2). Yet, critical research questions remain in determining spatial and vertical resolution thresholds for landscape and subsurface characterization (Table 1) required to adequately represent critical thermal, hydrologic, and biogeochemical processes in models designed to assess and predict permafrost thaw and its impacts. Rapid advances in remote sensing are being made to improve relevant near-surface characterization. Deeper subsurface characterization over large scales is a critical research need and current data gap. It follows that predictive large-scale permafrost models that focus on near-surface permafrost most directly apply to modes of thaw occurring in the shallow subsurface (active layer thickening and thermokarst formation). Incorporation of deeper thaw processes in large-scale predictive models to represent supra-permafrost and open talik development are needed for comprehensive evaluation of coupled surface water and groundwater resources in transitional permafrost environments (McKenzie et al., 2021). Comprehensive evaluation of the fate of circumboreal permafrost carbon and associated climate feedbacks in Earth System Models requires the incorporation of permafrost-hydrologic interactions that influence terrestrial and aquatic C cycles and lateral C transport to inland waters and coastal oceans in addition to land and water surface exchange of C with the atmosphere.
Permafrost thaw and its impacts to the water and carbon cycles are of interest not only to earth scientists, local communities, and land managers, but also to economists, military advisors, and policy makers due to the important role of northern high latitudes in the future global economy and international relations. Improved understanding and prediction of permafrost thaw impacts to hydrology will help mitigate economic losses derived from climate change (Melvin et al., 2017; Yumashev et al., 2019). Cost-effective development of infrastructure and groundwater resources in thawing permafrost regions also rely on a solid understanding of plausible futures.
This synthesis broadly applies to northern permafrost regions and provides a framework for discussing the implications of climate change and permafrost thaw on hydrologic and aquatic C dynamics across diverse landscapes. Understanding the underlying complexities that shape the hydrologic and aquatic C responses to various modes of permafrost thaw will elevate confidence in their prediction from the low to medium levels noted in the IPCC 2019 report.
Author Contributions
MW led the manuscript writing with significant contributions from RS. Both authors contributed to the article and approved the submitted version.
Funding
We gratefully acknowledge support from the U.S. Geological Survey's Water Availability & Use Science Program and the Biological Carbon Sequestration Program (LandCarbon) and funding provided by the Arctic Boreal Vulnerability Experiment (ABoVE), a NASA Terrestrial Ecology project, under award 14-TE14-0012.
Conflict of Interest
The authors declare that the research was conducted in the absence of any commercial or financial relationships that could be construed as a potential conflict of interest.
Publisher's Note
All claims expressed in this article are solely those of the authors and do not necessarily represent those of their affiliated organizations, or those of the publisher, the editors and the reviewers. Any product that may be evaluated in this article, or claim that may be made by its manufacturer, is not guaranteed or endorsed by the publisher.
Acknowledgments
We appreciate suggestions from the Reviewers that helped improve the paper. We also thank Dr. Suzanne Tank for her constructive comments on an early version of the manuscript.
References
Abbott, B. W., Jones, J. B., Godsey, S. E., Larouche, J. R., and Bowden, W. B. (2015). Patterns and persistence of hydrologic carbon and nutrient export from collapsing upland permafrost. Biogeoscience 12, 3725–3740. doi: 10.5194/bg-12-3725-2015
Abbott, B. W., Jones, J. B., Schuur, E. A. G., Chapin, F. S., Bowden, W. B., Bret-Harte, M. S., et al. (2016). Biomass offsets little or none of permafrost carbon release from soils, streams, and wildfire: an expert assessment. Environ. Res. Lett. 11:034014.
Abbott, B. W., Rocha, A. V., Shogren, A., Zarnetske, J. P., Iannucci, F., Bowden, W. B., et al. (2021). Tundra wildfire triggers sustained lateral nutrient loss in Alaskan Arctic. Glob. Change Biol. 27, 1408–1430. doi: 10.1111/gcb.15507
Abe, T., Iwahana, G., Efremov, P. V., Desyatkin, A. R., Kawamura, T., Fedorov, A., et al. (2020). Surface displacement revealed by L-band InSAR analysis in the Mayya area, Central Yakutia, underlain by continuous permafrost. Earth Planets Space 72:138. doi: 10.1186/s40623-020-01266-3
Ackley, C., Tank, S. E., Haynes, K. M., Rezaneshad, F., McCarter, C., and Quinton, W. L. (2021). Coupled hydrological and geochemical impacts of wildfire in peatland-dominated regions of discontinuous permafrost. Sci. Total Environ. 782:146841. doi: 10.1016/j.scitotenv.2021.146841
Aiken, G. R., Spencer, R. G., Striegl, R. G., Schuster, P. F., and Raymond, P. A. (2014). Influences of glacier melt and permafrost thaw on the age of dissolved organic carbon in the Yukon River basin. Glob. Biogeochem. Cycles 28, 525–537. doi: 10.1002/2013GB004764
Ala-aho, P., Soulsby, C., Pokrovsky, O. S., Kirpotin, S. N., Karlsson, J., Serikova, S., et al. (2018). Permafrost and lakes control river isotope composition across a boreal Arctic transect in the Western Siberian lowlands. Environ. Res. Lett. 13:034028. doi: 10.1088/1748-9326/aaa4fe
Beel, C. R., Heslop, J. K., Orwin, J. F., Pope, M. A., Schevers, J., Hung, J. K. Y., et al. (2021). Emerging dominance of summer rainfall driving High Arctic terrestrial-aquatic connectivity. Nat. Commun. 12:1448. doi: 10.1038/s41467-021-21759-3
Beel, C. R., Lamoureux, S. F., Orwin, J. F., Pope, M. A., Lafrenière, M. J., and Scott, N. A., et al. (2020). Differential impact of thermal and physical permafrost disturbances on High Arctic dissolved and particulate fluvial fluxes. Sci. Rep. 10:11836. doi: 10.1038/s41598-020-68824-3
Bond-Lamberty, B., Peckham, S., Ahl, D., and Gower, S. T. (2007). Fire as the dominant driver of central Canadian boreal forest carbon balance. Nature 450, 89–92. doi: 10.1038/nature06272
Briggs, M. A., Campbell, S., Nolan, J., Walvoord, M. A., Ntarlagiannis, D., Day-Lewis, F. D., et al. (2017). Surface geophysical methods for characterising frozen ground in transitional permafrost landscapes. Permafrost Periglac. Process. 28, 52–65. doi: 10.1002/ppp.1893
Briggs, M. A., Walvoord, J. M., McKenzie, C. I., Voss, F. D., Day-Lewis, D., and Lane, J. W. (2014). New permafrost is forming around shrinking arctic lakes, but will it last? Geophys. Res. Lett. 4, 1585–1592. doi: 10.1002/2014GL059251
Bring, A., Fedorova, I., Dibike, Y., Hinzman, L., Mård, J., Mernild, S. H., et al. (2016). Arctic terrestrial hydrology: a synthesis of processes, regional effects, and research challenges. J. Geophys. Res. Biogeosci. 121, 621–649. doi: 10.1002/2015JG003131
Brown, D. R. N., Jorgenson, M. T., Douglas, T. A., Romanovsky, V. E., Kielland, K., Hiemstra, C., et al. (2015). Interactive effects of wildfire and climate on permafrost degradation in Alaskan lowland forests. J Geophys. Res. Biogeosci. 120, 1619–1637. doi: 10.1002/2015JG003033
Brown, J., Ferrians, O., Heginbottom, J. A., and Melnikov, E. (2002). Circum-Arctic Map of Permafrost and Ground-Ice Conditions, Version 2. [Permafrost Extent]. Boulder, Colorado: NSIDC: National Snow and Ice Data Center (accessed July 6, 2017).
Chen, J., Wu, Y., O'Connor, M., Cardenas, M. B., Schafer, K., Michaelides, R., et al. (2020). Active layer freeze-thaw and water storage dynamics in permafrost environments inferred from InSAR. Remote Sens. Environ. 248:112007. doi: 10.1016/j.rse.2020.112007
Clayton, L. K., Schaefer, K., Battaglia, M. J., Bourgeau-Chavez, L., Chen, J., Chen, R. H., et al. (2021). Active layer thickness as a function of soil water content. Environ. Res. Lett. 16:055028. doi: 10.1088/1748-9326/abfa4c
Commane, R., Lindaas, J., Benmergui, J., Luus, K. A., Change, R. Y.-W., Daube, B. C., et al. (2017). Carbon dioxide sources from Alaska driven by increasing early winter respiration from Arctic tundra. Proc. Natl. Acad. Sci. 14, 5361–5366.
Connon, R., Devoie, E., Hayashi, M., Veness, T., and Quinton, W. (2018). The influence of shallow taliks on permafrost thaw and active layer dynamics in subarctic Canada. J. Geophys. Res. Earth Surf. 123, 281–297. doi: 10.1002/2017JF004469
Connon, R. F., Quinton, W. L., Craig, J. R., and Hayashi, M. (2014). Changing hydrologic connectivity due to permafrost thaw in the lower Liard River valley, NWT, Canada. Hydrol. Process. 28, 4163–4178. doi: 10.1002/hyp.10206
Dean, J. F., Meisel, O. H., Martyn Rosco, M., Belelli Marchesini, L., Garner, M. H., Lenderink, H., et al. (2020). East Siberian Arctic inland waters emit mostly contemporary carbon. Nat. Commun. 11:1627. doi: 10.1038/s41467-020-15511-6
Devoie, E. G., Craig, J. R., Connon, R. F., and Quinton, W. L. (2019). Taliks: a tipping point in discontinuous permafrost degradation in peatlands. Water Resour. Res. 55, 9838–9857. doi: 10.1029/2018WR024488
Dornblaser, M. M., and Striegl, R. G. (2015). Switching predominance of organic versus inorganic carbon exports from an intermediate-size subarctic watershed. Geophys. Res. Lett. 42, 386–394. doi: 10.1002/2014GL062349
Douglas, T. A., Turetsky, M. R., and Koven, C. D. (2020). Increased rainfall stimulates permafrost thaw across a variety of Interior Alaskan boreal ecosystems. npj Clim. Atmos. Sci. 3:28. doi: 10.1038/s41612-020-00155-6
Drake, T. W., Wickland, K. P., Spencer, R. G. M., McKnight, D. M., and Striegl, R. G. (2015). Ancient low-molecular-weight organic acids in permafrost fuel rapid carbon dioxide production upon thaw. Proceed. Natl. Acad. Sci. 112, 13946–13951. doi: 10.1073/pnas.1511705112
Ebel, B. A., Koch, J. C., and Walvoord, M. A. (2019). Soil physical, hydraulic, and thermal properties in interior Alaska, USA: implications for hydrologic response to thawing permafrost conditions. Water Resour. Res. 55, 4427–4447. doi: 10.1029/2018WR023673
Evans, S. G., and Ge, S. (2017). Contrasting hydrogeologic responses to warming in permafrost and seasonally frozen ground hillslopes. Geophys. Res. Lett. 44, 1803–1813. doi: 10.1002/2016GL072009
Evans, S. G., Yokeley, B., Stephens, C., and Brewer, B. (2020). Potential mechanistic causes of increased baseflow across northern Eurasia catchments underlain by permafrost. Hydrol. Proc. 34, 2676–2690. doi: 10.1002/hyp.13759
Frampton, A., and Destouni, G. (2015). Impact of degrading permafrost on subsurface solute transport pathways and travel times. Water Resour. Res. 51, 7680–7701. doi: 10.1002/2014WR016689
Frey, K. E., and McClelland, J. W. (2009). Impacts of permafrost degradation on arctic river biogeochemistry. Hydrol. Proc. 23, 169–182. doi: 10.1002/hyp.7196
Ghias, M. S., Therrien, R., Molson, J., and Lemieux, J.-M. (2017). Controls on permafrost thaw in a coupled groundwater-flow and heat-transport system: Iqaluit Airport, Nunavut, Canada. Hydrol. J. 25, 657–673. doi: 10.1007/s10040-016-1515-7
Gibson, C. M., Chasmer, L. E., Thompson, D. K., Quinton, W. L., Flannigan, M. D., and Olefeldt, D. (2018). Wildfire as a major driver of recent permafrost thaw in boreal peatlands. Nat. Commun. 9:3041. doi: 10.1038/s41467-018-05457-1
Glass, B. K., Rudolph, D. L., Duguay, C., and Wicke, A. (2020). Identifying groundwater discharge zones in the Central Mackenzie Valley using remotely sensed optical and thermal imagery. Canad. J. Earth Sci. 58, 105–121. doi: 10.1139/cjes-2019-0169
Grosse, G., Goetz, S., McGuire, A. D., Romanovsky, V. E., and Schuur, E. A. G. (2016). Changing permafrost in a warming world and feedbacks to the Earth system. Environ. Res. Lett. 11:040201. doi: 10.1088/1748-9326/11/4/040201
Grosse, G., Harden, J., Turetsky, M., McGuire, A. D., Camill, P., Tarnocai, C., et al. (2011). Vulnerability of high-latitude soil organic carbon in North America to disturbance. J. Geophys. Res. 116:G00K06. doi: 10.1029/2010JG001507
Heslop, J., Walter Anthony, K. M., Winkel, M., Sepulveda-Jauregui, A., Martinez-Cruz, K., Bondurant, A., et al. (2020). A synthesis of methane dynamics in thermokarst lake environments. Earth-Sci. Rev. 210:103365. doi: 10.1016/j.earscirev.2020.103365
Hugelius, G., Loisel, J., Chadburn, S., Jackson, R. B., Miriam, J., MacDonald, G., Marushchak, M., Olefeldt, D., Packalen, M., Siewert, M. B., Treat, C. et al. (2020). Large stocks of peatland carbon and nitrogen are vulnerable to permafrost thaw. Proc. Natl. Acad. Sci. 34, 20438–20446, doi: 10.1073/pnas.1916387117
Hugelius, G., Strauss, J., Zubrzycki, S., Harden, J. W., Schuur, E. A. G., Ping, C.-L., et al. (2014). Estimated stocks of circumpolar permafrost carbon with quantified uncertainty ranges and identified data gaps. Biogeosci. 11, 6573–6593. doi: 10.5194/bg-11-6573-2014
Jafarov, E. E., Coon, E. T., Harp, D. R., Wilson, C. J., Painter, S. L., Atchley, A. L., et al. (2018). Modeling the role of preferential snow accumulation in through talik development and hillslope groundwater flow in a transitional permafrost landscape. Environ. Res. Lett. 13:105006. doi: 10.1088/1748-9326/aadd30
James, M., Lewkowicz, A., Smith, S., and Miceli, C. M. (2013). Multidecadal degradation and persistence of permafrost in the Alaska Highway corridor, northwest Canada. Environ. Res. Lett. 8:045013. doi: 10.1088/1748-9326/8/4/045013
Jepsen, S. M., Walvoord, M. A., Voss, C. I., and Rover, J. (2016). Effect of permafrost thaw on the dynamics of lakes recharged by ice-jam floods: case study of Yukon Flats, Alaska. Hydrol Proc 30, 1782–1795. doi: 10.1002/hyp.10756
Jorgenson, M. T. (2013). “Thermokarst terrains,” in Treatise on Geomorphology, eds Shroder JF (Editor-in-chief), Giardino, R. and Harbor, J. (Volume Editors) (San Diego: Academic Press), 313–332.
Jorgenson, M. T., and Grosse, G. (2016). Remote sensing of landscape change in permafrost regions. Permafrost. Periglac. Proc. 27, 324–338. doi: 10.1002/ppp.1914
Jorgenson, M. T., and Osterkamp, T. E. (2005). Response of boreal ecosystems to varying modes of permafrost degradation. Can. J. For. Res. 35, 2100–2111 doi: 10.1139/x05-153
Jorgenson, M. T., Romanovsky, V., Harden, J., Shur, Y., O'Donnell, J., Schuur, E. A. G., et al. (2010). Resilience and vulnerability of permafrost to climate change. Can. J. For. Res. 40, 1219–1236. doi: 10.1139/X10-060
Karlsson, J. M., Lyon, S. W., and Destouni, G. (2012). Thermokarst lake, hydrological flow and water balance indicators of permafrost change in Western Siberia. J. Hydrol. 464, 459-466. doi: 10.1016/j.jhydrol.2012.07.037
Kasischke, E. S., and Turetsky, M. R. (2006). Recent changes in the fire regime across the North American boreal region - spatial and temporal patterns of burning across Canada and Alaska. Geophys. Res. Lett. 33:L09703. doi: 10.1029/2006GL025677
Koch, J. C., Kikuchi, K. P., Wickland, K. P., and Schuster, P. (2014). Runoff sources and flow paths in a partially burned, upland boreal catchment underlain by permafrost. Water Resour. Res. 50, 8141–8158. doi: 10.1002/2014WR015586
Kokelj, S. V., Lacelle, D., Lantz, T. C., Tunnicliffe, J., Malone, L., Clark, I. D., et al. (2013). Thawing of massive ground ice in mega slumps drives increases in stream sediment and solute flux across a range of watershed scales. J. Geophys. Res. -Earth 118, 681–692. doi: 10.1002/jgrf.20063
Kurylyk, B. L., Hayashi, M., Quinton, W. L., McKenzie, J. M., and Voss, C. I. (2016). Influence of vertical and lateral heat transfer on permafrost thaw, peatland landscape transition, and groundwater flow. Water Resour. Res. 52, 1286–1305. doi: 10.1002/2015WR018057
Kurylyk, B. L., and Walvoord, M. A. (2021). “Permafrost hydrogeology,” in Arctic Hydrology, Permafrost and Ecosystems, eds D. Yang, D. Kane (Springer), 493–523.
Lawrence, D. M., Koven, C. D., Swenson, S. C., Riley, W. J., and Slater, A. G. (2015). Permafrost thaw and resulting soil moisture changes regulate projected high-latitude CO2 and CH4 emissions. Environ. Res. Lett. 10:094011. doi: 10.1088/1748-9326/10/9/094011
Lemieux, J.-M., Fortier, R., Talbot-Poulin, M.-C., Molson, J., Therrien, R., Ouellet, M., et al. (2016). Groundwater occurrence in cold environments: examples from Nunavik, Canada. Hydrogeol. J. 24, 1497–1513. doi: 10.1007/s10040-016-1411-1
McGuire, A. D., Lawrence, D. M., Koven, C., Clein, J. S., Burke, E., Chen, G., et al. (2018). Dependence of the evolution of carbon dynamics in the northern permafrost region on the trajectory of climate change. Proc. Natl. Acad. Sci. 115, 3882–3887. doi: 10.1073/pnas.1719903115
McKenzie, J. M., Kurylyk, B. L., Walvoord, M. A., Bense, V. F., Fortier, D., Spence, C., et al. (2021). Invited perspective: what lies beneath a changing arctic? Cryosphere 15, 479–484. doi: 10.5194/tc-15-479-2021
McKenzie, J. M., and Voss, C. I. (2013). Permafrost thaw in a nested groundwater-flow system. Hydrogeol. J. 21:299. doi: 10.1007/s10040-012-0942-3
Melvin, A. M., Larsen, P., Boehlert, B., Neumann, J. E., Chinowsky, P., Espinet, X., et al. (2017). Climate change damages to Alaska public infrastructure and the economics of proactive adaption. Proc. Natl. Acad. Sci. 114, E122–E131. doi: 10.1073/pnas.1611056113
Meredith, M., Sommerkorn, M., Cassota, S., Derksen, C., Ekaykin, A., Hollowed, A., et al. (2019). Polar Regions, IPCC Special Report on the Ocean and Cryosphere in a Changing Climate, IPCC, WMO, UNEP, H-O Portner, DC Roberts, V Masson-Delmotte, P Zhai, M Tignor, E Poloczanska, K Mintenbeck (ed), 1–173. [Research Book Chapter]
Minsley, B. J., Abraham, J. D., Smith, B. D., Cannia, J. C., Voss, C. I., Jorgenson, M. T., et al. (2012). Airborne electromagnetic imaging of discontinuous permafrost. Geophys. Res. Lett. 39:L02503. doi: 10.1029/2011GL050079
Minsley, B. J., Pastick, N. J., Wylie, B. K., Brown, D. R. N., and Kass, M. A. (2016). Evidence for non-uniform permafrost degradation after fire in boreal landscapes. J. Geophys. Res. Earth Surf. 121, 320–333. doi: 10.1002/2015JF003781
Mishra, U., Jastrow, J. D., Matamala, R., Hugelius, G., Koven, C. D., Harden, J. W., et al. (2013). Empirical estimates to reduce modeling uncertainties of soil organic carbon in permafrost regions: a review of recent progress and remaining challenges. Environ. Res. Lett. 8:035020. doi: 10.1088/1748-9326/8/3/035020
Neff, J. C., Finlay, J. C., Zimov, S. A., Davydov, S. P., Carrasco, J. J., Schuur, E. A. G., et al. (2006). Seasonal changes in the age and structure of dissolved organic carbon in Siberian rivers and streams. Geophys. Res. Lett. 33:L23401. doi: 10.1029/2006GL028222
Nitze, I., Cooley, S. W., Duguay, C. R., Jones, B. M., and Grosse, G. (2020). The catastrophic thermokarst lake drainage events of 2018 in northwestern Alaska: fast-forward into the future. Cryosphere 14, 4279–4429. doi: 10.5194/tc-14-4279-2020
Nitze, I., Grosse, G., Jones, B. M., Romanovsky, V. E., and Boike, J. (2018). Remote sensing quantifies widespread abundance of permafrost region disturbances across the Arctic and Subarctic. Nat. Commun. 9:5423. doi: 10.1038/s41467-018-07663-3
O'Donnell, J. A., Aiken, G. R., Walvoord, M. A., and Butler, K. D. (2012). Dissolved organic matter composition of winter stream flow in the Yukon River basin: implications of permafrost thaw and increased groundwater discharge. Global Biogeochem. Cycles 26:GB0E06. doi: 10.1029/2012GB004341
O'Neill, H. B., Roy-Leveillee, P., Lebedeva, L., and Ling, F. (2020). Recent advances (2010–2019) in the study of taliks. Permafrost Periglac Process. 31, 346–357. doi: 10.1002/ppp.2050
Parazoo, N. C., Koven, C. D., Lawrence, D. M., Romanovsky, V., and Miller, C. E. (2018). Detecting the permafrost carbon feedback: talik formation and increased cold-season respiration as precursors to sink-to-source transitions. Cryosphere 12, 123–144. doi: 10.5194/tc-12-123-2018
Park, H., Walsh, J., Fedorov, A. N., Sherstiukov, A. B., Iijima, Y., and Ohata, T. (2013). The influence of climate and hydrological variables on opposite anomaly in active-layer thickness between Eurasian and North American watersheds. Cryosphere 7, 631–645. doi: 10.5194/tc-7-631-2013
Pastick, N. J., Jorgenson, M. T., Wylie, B. K., Nield, S. J., Johnson, K. D., and Finley, A. O. (2015). Distribution of near-surface permafrost in Alaska: estimates of present and future conditions. Remote Sens. Environ. 168, 301–315. doi: 10.1016/j.rse.2015.07.019
Plaza, C., Pegoraro, E., Bracho, R., Celis, G., Crummer, K. G., Hutchings, J. A., et al. (2019). Direct observation of permafrost degradation and rapid soil carbon loss in tundra. Nat. Geosci. 12, 627–631. doi: 10.1038/s41561-019-0387-6
Pokrovsky, O. S., Manasypov, R. M., Loiko, S., Shirokova, L. S., Krickov, I. A., Pokrovsky, B. G., et al. (2015). Permafrost coverage, watershed area and season control of dissolved carbon and major elements in western Siberian rivers. Biogeosci 12, 6301–6320. doi: 10.5194/bg-12-6301-2015
Quinton, W. L., Hayashi, M., and Chasmer, L. E. (2011). Permafrost-thaw-induced land-cover change in the Canadian subarctic: implications for water resources. Hydrol. Proc. 25, 152–158. doi: 10.1002/hyp.7894
Rey, D., Walvoord, M., Ebel, B., Minsley, B., Voss, C., and Singha, K. (2020). Wildfire-initiated talik development exceeds current thaw projections: observations and models from Alaska's continuous permafrost zone. Geophys. Res. Lett. 47:e2020GL087565. doi: 10.1029/2020GL087565
Rey, D., Walvoord, M., Minsley, B., Rover, J., and Singha, K. (2019). Investigating lake-area dynamics across a permafrost-thaw spectrum using airborne electromagnetic surveys and remote sensing time-series data in Yukon Flats, Alaska. Environ. Res. Lett. 14:025001. doi: 10.1088/1748-9326/aaf06f
Rodenhizer, H., Ledman, J., Mauritz, M., Natali, S. M., Pegoraro, E., Plaza, C., et al. (2020). Carbon thaw rate doubles when accounting for subsidence in a permafrost warming experiment. J. Geophys. Res. Biogeosci. 125:e2019JG005528. doi: 10.1029/2019JG005528
Rowland, J. C., Travis, B. J., and Wilson, C. J. (2011). The role of advective heat transport in talik development beneath lakes and ponds in discontinuous permafrost. Geophys. Res. Lett. 38:17504. doi: 10.1029/2011GL048497
Saito, K., Machiya, H., Iwahana, G., Ohno, H., and Yokohata, T. (2020). Mapping simulated circum-Arctic organic carbon, ground ice, and vulnerability of ice-rich permafrost to degradation. Prog. Earth Planet Sci. 7:31. doi: 10.1186/s40645-020-00345-z
Schädel, C., Koven, C. D., Lawrence, D. M., Celis, G., Garnello, A. J., Hutchings, J., et al. (2018). Divergent patterns of experimental and model-derived permafrost ecosystem carbon dynamics in response to Arctic warming. Environ. Res. Lett. 13:105002.
Schaefer, K., Elshorbany, Y., Jafarov, E., et al. (2020). Potential impacts of mercury released from thawing permafrost. Nat. Commun. 11:4650. doi: 10.1038/s41467-020-18398-5
Schuur, E. A. G., McGuire, A. D., Schädel, C., Grosse, G., Harden, J. W., Hayes, D. J., et al. (2015). Climate change and the permafrost carbon feedback. Nature 520, 171–179. doi: 10.1038/nature14338
Schwab, M. S., Hilton, R. G., Raymond, P. A., Haghipour, N., Amos, E., Tank, S. E., et al. (2020). An abrupt aging of dissolved organic carbon in large Arctic rivers. Geophys. Res. Lett. 47:e2020GL088823. doi: 10.1029/2020GL088823
Smith, L. C., Pavelsky, T. M., MacDonald, G. M., Shiklomanov, A. I., and Lammers, R. B. (2007). Rising minimum daily flows in northern Eurasian rivers: a growing influence of groundwater in the high-latitude hydrologic cycle. J. Geophys. Res. 112:G04S47. doi: 10.1029/2006JG000327
Spencer, R. G. M., Aiken, G. R., Butler, K. D., Dornblaser, M. M., Striegl, R. G., and Hernes, P. J. (2009). Utilizing chromophoric dissolved organic matter measurements to derive export and reactivity of dissolved organic carbon exported to the Arctic Ocean: a case study of the Yukon River, Alaska. Geophys. Res. Lett. 36:L06401. doi: 10.1029/2008GL036831
Stackpoole, S. M., Butman, D. E., Clow, D. W., Verdin, K. L., Gaglioti, B. V., Genet, H., et al. (2017). Inland waters and their role in the carbon cycle of Alaska. Ecol. Appl. 27, 1403–1420. doi: 10.1002/eap.1552
Streletskiy, D. A., Sherstiukov, A. B., Frauenfeld, O. W., and Nelson, F. E. (2015). Changes in the 1963–2013 shallow ground thermal regime in Russian permafrost regions. Env. Res. Lett. 10:125005. doi: 10.1088/1748-9326/10/12/125005
Striegl, R. G., Aiken, G. R., Dornblaser, M. M., Raymond, P. A., and Wickland, K. P. (2005). A decrease in discharge-normalized DOC export by the Yukon River during summer through autumn. Geophys. Res. Lett. 32:L21413. doi: 10.1029/2005GL024413
Striegl, R. G., Dornblaser, M. M., Aiken, G. R., Wickland, K. P., and Raymond, P. A. (2007). Carbon export and cycling by the Yukon, Tanana, and Porcupine rivers, Alaska, 2001–2005. Water Resour. Res. 43:W02411. doi: 10.1029/2006WR005201
Tank, S. E., Frey, K. E., Striegl, R. G., Raymond, P. A., Holmes, R. M., McClelland, J. W., et al. (2012a). Landscape-level controls on dissolved carbon flux from diverse catchments of the circumboreal. Glob. Biogeochem. Cycles 26:GB0E02. doi: 10.1029/2012GB004299
Tank, S. E., Raymond, P. A., Striegl, R. G., McClelland, J. W., Holmes, R. M., Fiske, G. J., et al. (2012b). A land-to-ocean perspective on the magnitude, source and implication of DIC flux from major Arctic rivers to the Arctic Ocean. Global Biogeochem. Cycles 26:GB4018. doi: 10.1029/2011GB004192
Tank, S. E., Striegl, R. G., McClelland, J. W., and Kokelj, S. V. (2016). Multi-decadal increases in dissolved organic carbon and alkalinity flux from the Mackenzie drainage basin to the Arctic Ocean. Environ. Res. Lett. 11:054015. doi: 10.1088/1748-9326/11/5/054015
Tank, S. E., Vonk, J. E., Walvoord, M. A., McClelland, J. W., Laurion, I., and Abbott, B. W. (2020). Landscape matters: Predicting the biogeochemical effects of permafrost thaw on aquatic networks with a state factor approach. Permafrost Periglac. Process. 31, 358–370. doi: 10.1002/ppp.2057
Terry, N., Grunewald, E., Briggs, M., Gooseff, M., Huryn, A. D., Kass, M. A., et al. (2020). Seasonal subsurface thaw dynamics of an aufeis feature inferred from geophysical methods. J. Geophys. Res. Earth Surf. 125:e2019JF005345. doi: 10.1029/2019JF005345
Vonk, J. E., Mann, P. J., Davydov, S., Davydova, A., Spencer, R. G. M., Schade, J., et al. (2013). High biolability of ancient permafrost carbon upon thaw. Geophys. Res. Lett. 40, 2689–2693. doi: 10.1002/grl.50348
Vonk, J. E., Tank, S. E., Bowden, W. B., Laurion, I., Vincent, W. F., Alekseychik, P., et al. (2015b). Reviews and syntheses: effects of permafrost thaw on Arctic aquatic ecosystems. Biogeosci. 12, 7129–7167. doi: 10.5194/bg-12-7129-2015
Vonk, J. E., Tank, S. E., Mann, P. J., Spencer, R. G. M., Treat, C. C., Striegl, R. G., et al. (2015a). Biodegradability of dissolved organic carbon in permafrost soils and aquatic systems: a meta-analysis. Biogeoscience 12, 6915–6930. doi: 10.5194/bg-12-6915-2015
Vonk, J. E., Tank, S. E., and Walvoord, M. A. (2019). Integrating hydrology and biogeochemistry across frozen landscapes. Nat. Commun. 10:5377.
Walter Anthony, K. M., Lindgren, P., Hanke, P., Engram, M., Anthony, P., Daanen, R. P., et al. (2021). Decadal-scale hotspot methane ebullition within lakes following abrupt permafrost thaw. Environ. Res. Lett. 16:035010. doi: 10.1088/1748-9326/abc848
Walvoord, M. A., and Kurylyk, B. L. (2016). Hydrologic impacts of thawing permafrost—a review. Vadose Zone J. 15:10. doi: 10.2136/vzj2016.01.0010
Walvoord, M. A., and Striegl, R. G. (2007). Increased groundwater to stream discharge from permafrost thawing in the Yukon River basin: potential impacts on lateral export of carbon and nitrogen. Geophys. Res. Lett. 34:L12402. doi: 10.1029/2007GL030216
Walvoord, M. A., Voss, C. I., Ebel, B. A., and Minsley, B. J. (2019). Development of perennial thaw zones in boreal hillslopes enhances potential mobilization of permafrost carbon. Envion. Res. Lett. 14:015003. doi: 10.1088/1748-9326/aaf0cc
Walvoord, M. A., Voss, C. I., and Wellman, T. P. (2012). Influence of permafrost distribution on groundwater flow in the context of climate-driven permafrost thaw: example from Yukon Flats Basin, Alaska, USA. Water Resour. Res. 48:W07524. doi: 10.1029/2011WR011595
Wellman, T. P., Voss, C. I., and Walvoord, M. (2013). Impacts of climate, lake size, and supra- and sub-permafrost groundwater flow on lake-talik evolution Yukon Flats, Alaska, USA. Hydrogeol. J. 21, 281–298. doi: 10.1007/s10040-012-0941-4
Wickland, K. W., Waldrop, M., Aiken, G. R., Koch, J. C., and Jorgenson, M. T. (2018). Dissolved organic carbon and nitrogen release from boreal Holocene permafrost and seasonally frozen soils of Alaska. Environ. Res. Lett. 13:065011. doi: 10.1088/1748-9326/aac4ad
Wild, B., Andersson, A., Bröder, L., Vonk, J., McClelland, J. W., Song, W., et al. (2019). Rivers across the Siberian Arctic unearth the patterns of carbon release from thawing permafrost. Proc. Natl. Acad. Sci. 116, 10280–10285. doi: 10.1073/pnas.1811797116
Xia, J., McGuire, D. A., Lawrence, D., Burke, E., Chen, G., Chen, X., et al. (2017). Terrestrial ecosystem model performance in simulating productivity and its vulnerability to climate change in the northern permafrost region. J. Geophys. Res. Biogeosci. 122, 430–446. doi: 10.1002/2016JG003384
Yi, Y., Kimball, J. S., Chen, R. H., Moghaddam, M., Reichle, R. H., Mishra, U., et al. (2018). Characterizing permafrost active layer dynamics and sensitivity to landscape spatial heterogeneity in Alaska. Cryosphere 12, 145–161. doi: 10.5194/tc-12-145-2018
Yoshikawa, K., Bolton, W. R., Romanovsky, V. E., Fukuda, M., and Hinzman, L. D. (2002). Impacts of wildfire on the permafrost in the boreal forests of Interior Alaska. J. Geophys. Res. 108:8148. doi: 10.1029/2001JD000438
Yumashev, D., Hope, C., Shaefer, K., Riemann-Campe, K., Iglesias-Suarez, F., Jafarov, E., et al. (2019). Climate policy implications of nonlinear decline of Arctic land permafrost and other cryosphere elements. Nature Comm. 10:1900. doi: 10.1038/s41467-019-09863-x
Zhang, Y., Olthof, I., Fraser, R., and Wolfe, S. A. (2014). A new approach to mapping permafrost and change incorporating uncertainties in ground conditions and climate projections. Cryosphere 8, 2177–2194. doi: 10.5194/tc-8-2177-2014
Zolkos, S., Krabbenhoft, D., Suslova, A., Tank, S. E., McClelland, J. W., Spencer, R. G. M., et al. (2020a). Mercury export from Arctic Great Rivers. Environ. Sci. Technol. 54, 4140–4148. doi: 10.1021/acs.est.9b07145
Zolkos, S., Tank, S. E., and Kokelj, S. V. (2018). Mineral weathering and the permafrost carbon-climate feedback. Geophys. Res. Lett. 45, 9623–9632. doi: 10.1029/2018GL078748
Zolkos, S., Tank, S. E., Striegl, R. G., and Kokelj, S. V. (2019). Thermokarst effects on carbon dioxide and methane fluxes in streams on the Peel Plateau (NWT, Canada). J. Geophys. Res. Biogeosci. 124, 1781–1798. doi: 10.1029/2019JG005038
Keywords: permafrost, boreal, carbon, hydrology, climate change
Citation: Walvoord MA and Striegl RG (2021) Complex Vulnerabilities of the Water and Aquatic Carbon Cycles to Permafrost Thaw. Front. Clim. 3:730402. doi: 10.3389/fclim.2021.730402
Received: 24 June 2021; Accepted: 26 August 2021;
Published: 04 October 2021.
Edited by:
Chris Derksen, Environment and Climate Change, CanadaReviewed by:
Ted Schuur, Northern Arizona University, United StatesAllison Kelley, Northern Arizona University, Flagstaff, United States in collaboration with reviewer TS
Joanne K. Heslop, German Research Center for Geosciences, Germany
Copyright © 2021 Walvoord and Striegl. This is an open-access article distributed under the terms of the Creative Commons Attribution License (CC BY). The use, distribution or reproduction in other forums is permitted, provided the original author(s) and the copyright owner(s) are credited and that the original publication in this journal is cited, in accordance with accepted academic practice. No use, distribution or reproduction is permitted which does not comply with these terms.
*Correspondence: Michelle A. Walvoord, d2Fsdm9vcmRAdXNncy5nb3Y=