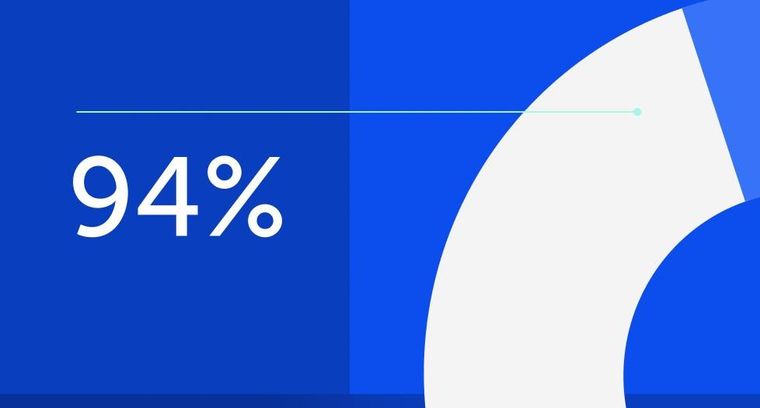
94% of researchers rate our articles as excellent or good
Learn more about the work of our research integrity team to safeguard the quality of each article we publish.
Find out more
ORIGINAL RESEARCH article
Front. Clim., 07 September 2021
Sec. Carbon Dioxide Removal
Volume 3 - 2021 | https://doi.org/10.3389/fclim.2021.728719
A rapid build-out of direct air capture (DAC), deployed in order to mitigate climate change, will require significant amounts of both low-carbon thermal and electrical energy. Firm low-carbon power resources, including nuclear, geothermal, or natural gas with carbon capture, which also will become more highly valued as variable renewable energy penetration increases, would be able to provide both heat and electricity for DAC. In this study, we examined the techno-economic synergy between a hypothetical DAC plant in the year 2030 and a nuclear small modular reactor, and determined two avenues for which this relationship could benefit the nuclear plant. First, we demonstrated that, under certain assumptions, selling a portion of its energy to a DAC facility allows the nuclear plant to take in 21% less revenue from selling electricity to wholesale markets than its projected levelized cost, and still break even. Second, after estimating a potential revenue stream, we showed that an integration with DAC allows for the nuclear plant's capital costs to be up to 35% higher than what would be required if only selling electricity to wholesale markets. This could enable the nuclear plant to operate economically even in the face of variable and decreasing wholesale electricity prices, and also could offer developers more financial certainty when planning a new project. Ultimately, this study shows that the need for low-carbon energy for DAC plants might incentivize the development of advanced nuclear plants and firm low-carbon resources more broadly.
Many changes to current energy systems are required to reach net-zero carbon emissions throughout the world, though the pathways will vary by sector. Electricity generation, for example, is one of the more straightforward sectors to decarbonize, yet with each increment of greenhouse-gas emissions abated, deeper decarbonization will become increasingly more expensive and difficult (Friedmann et al., 2020). Negative-emissions technologies (NETs) could reduce the cost of reaching net-zero emissions (Bistline and Blanford, 2021); the Intergovernmental Panel on Climate Change (IPCC) has stated that any chance to keep the global average temperature increase from pre-industrial levels under 1.5°C will require some utilization of NETs (IPCC, 2018).
Direct air capture (DAC) with permanent CO2 storage is one NET that could make a significant impact. DAC is a process that captures CO2 directly from the atmosphere via an engineered system. A typical DAC module pulls in ambient air and extracts the CO2 through a chemical reaction (Sanz-Pérez et al., 2016). The CO2 capture medium is regenerated to release a stream of nearly-pure CO2 that then can be reused or permanently sequestered. The treated air then is returned to the atmosphere. Despite its potential, commercializing and deploying DAC systems at a large scale may prove challenging (National Academies of Sciences Engineering Medicine, 2019).
DAC systems have a substantial energy requirement of both heat and electricity. Electricity is required to run fans and other auxiliary equipment, and a significant amount of heat is needed to separate the CO2 from the capture medium. The two primary DAC technologies being commercialized today are high-temperature liquid solvent (HTLS) and low-temperature solid sorbent (LTSS) systems, although there are a number of newer concepts using alternative regeneration inputs such as moisture and electricity. HTLS systems utilize water solutions that contain hydroxide sorbents that have a strong affinity for CO2, while LTSS methods utilize amine materials that are bonded to a porous solid structure. The hydroxide solutions in HTLS DAC systems require high-temperature heat above 800°C to be regenerated, currently supplied by firing natural gas with oxygen (Keith et al., 2018). Amine adsorbents in LTSS DAC systems require heat at roughly 100°C, which allows for more flexibility in their energy source (Realmonte et al., 2019). The LTSS method may have a significant economic advantage over the HTLS method, as it can reduce its costs by taking advantage of low-grade heat from a co-located thermal power plant (McQueen et al., 2020). Although current costs for most of these systems are around 600 $/tCO2, estimates in the literature for possible costs range from below 100 $/tCO2 up to 1,000 $/tCO2 (National Academies of Sciences Engineering Medicine, 2019). However, once technology developers are able to scale up their manufacturing and operations, it is likely that costs will fall closer to the 100 $/tCO2 mark (Fasihi et al., 2019).
Due to its high energy requirements, any future scenario with large amounts of DAC systems may require a significant buildout of low-carbon thermal power generation resources. These resources, which can include nuclear, geothermal, and natural gas with carbon capture and sequestration (CCS), can be referred to as “firm” sources of power, since they are capable of running reliably and continuously over long durations. As decarbonization progresses, there likely will be increased demand for firm low-carbon power resources for three main reasons. First, increased electrification of end-use technologies in the transportation, residential, commercial, and industrial sectors will cause an increase in overall electricity demand (Mai et al., 2018). Second, as low-carbon energy becomes more highly valued, existing fossil-fuel generation will be replaced with clean energy resources. Third, as the power sector becomes further decarbonized, mostly through the increased penetration of variable renewable energy (VRE), firm sources of power likely will become more valuable for keeping system-wide costs of electricity lower (Sepulveda et al., 2018).
This study examines the techno-economic integration between one type of firm low-carbon resource, a nuclear small modular reactor (SMR), and a hypothetical LTSS DAC plant, and is based on a scenario in the year 2030. In the United States, VRE deployment has increased to provide roughly 40% of the power generation in many areas, resulting in a decrease in average wholesale electricity prices, but an increase in price volatility (Seel et al., 2018). Firm low-carbon power systems, such as nuclear SMRs, have proven technologically viable but do not yet contribute a significant amount of energy to the grid. In order to mitigate climate change, policies have been enacted that incentivize the deployment of DAC systems, which have become cost-competitive (Friedmann et al., 2020). Demand for additional DAC systems is high, but each new plant requires a substantial amount of energy. In addition, as VRE penetration increases, the need for more storage or transmission will increase system-wide costs of electricity (Sepulveda et al., 2018). Concerns about electricity price volatility and rising system-wide costs have created a desire to deploy more firm, low-carbon resources, but many of these options are not yet cost-competitive. Through this scenario, we attempt to determine the economic benefits that a nuclear SMR might realize from providing low-grade heat and electricity to a co-located DAC plant in addition to selling electricity to conventional wholesale markets.
We modeled a cogeneration facility that includes a DAC system and a nuclear SMR, and examined how this combination could improve the economics of the nuclear plant. First, we looked at the cost required to build a LTSS DAC plant when it buys power from wholesale markets for its electrical operations and for a heat pump to supply its thermal energy. Next, we considered a DAC plant that instead pays a co-located SMR for its required heat and electricity. This benefits the SMR because it would receive an additional source of revenue through the utilization of its low-grade heat, and it benefits the DAC plant because it would have a designated, reliable source of low-carbon energy nearby and would not require new transmission connections, which are expensive and time-consuming to construct (Eto, 2016).
The cost parameters of our hypothetical DAC plant were taken from Fasihi et al. (2019), which presents a detailed techno-economic assessment of DAC plants based on a literature review of DAC technologies and their possible costs.1 This plant, shown in Table 1, captures 360,000 tons of CO2 per year, has a lifetime of 25 years, and operates at a 91% capacity factor. It was assumed that a manufacturing learning rate, due to increased DAC development over the previous decade, has allowed for reduced capital expenses, which has enabled lower overall costs (Fasihi et al., 2019).
The parameter used to evaluate the overall economics of the DAC systems is the levelized cost of DAC (LCOD). This can be calculated using Equation (1) above (Fasihi et al., 2019). The LCOD for the baseline DAC plant is 102 $/tCO2. A spreadsheet containing this calculation and other results can be found in the Supplementary Material.
Table 1. Cost parameters for the baseline DAC plant (Fasihi et al., 2019).
In this baseline scenario, illustrated in Figure 1, the DAC plant is assumed to purchase all of its energy in the form of electricity from the grid. Both the thermal and electrical requirements for the DAC system, shown in Table 2, were assumed to be the average of their range provided (National Academies of Sciences Engineering Medicine, 2019).
Table 2. Energy requirements for LTSS DAC systems (National Academies of Sciences Engineering Medicine, 2019).
The assumed cost of purchasing electricity for the baseline DAC plant is 25 $/MWhe, which was estimated by the average wholesale electricity prices across a range of scenarios for U.S. markets in 2030 with 40% VRE penetration as described in Seel et al. (2018). Its assumed cost of heat is 24.34 $/MWht, which was determined by the levelized cost of heat (LCOH) for a heat pump that is run at full capacity with electricity prices of 25 $/MWh (Dominković, 2015).
Next, we considered the scenario where this DAC plant is integrated alongside a nuclear SMR power plant, shown in Figure 2. This power plant was assumed to be sized to provide all of its low-grade heat to the DAC plant. In other words, for every MWh of electricity that the SMR produces, it also produces one MWh of steam at 100°C for the DAC plant. However, this extraction of heat causes a reduction in the potential amount of electricity that can be produced. In a power-plant steam cycle that consists of a high-pressure turbine, intermediate-pressure turbine, and low-pressure turbines, steam can be accessed and diverted to heat exchangers between the intermediate and low-pressure turbines at temperatures up to 159°C (Qvist, 2020). Extracting all available steam at a temperature of 100°C will result in a 15% decrease in electric output (Qvist, 2020). In addition, with the electricity requirement assumptions stated previously, 21% of the generated electricity from the SMR must be used to power the electrical equipment in the DAC plant. This leaves 64% of the power plant's available energy to be sold as electricity to wholesale markets, or as described in Table 3, there will be a ratio of 0.64 MWh of electricity sold to wholesale markets per MWh of electricity produced.
In order to determine the revenue the SMR would receive in return for providing the DAC facility with 36% of its energy, we assumed that the SMR and the DAC facility formed a bilateral agreement. Within this agreement the LCOD is recalculated with the energy costs as zero, at 69 $/tCO2 (LCODCogen). Then, instead of buying this electricity from wholesale markets, the DAC plant would pay the power plant a revenue that is equal to this difference in LCOD converted to be in terms of energy, as shown in Equation (2), which equals 30 $/MWh of energy produced.
The average LCOE of an advanced nuclear power plant is projected to be 60 $/MWhe (EIRP, 2017). This means that this power plant will need to receive an average of 60 $/MWhe in revenue over its lifetime in order to break even financially; developers will only build this plant if at least this amount of revenue can be projected.2 For a conventional power plant, this revenue largely would come from selling electricity to wholesale electricity markets, though some markets also offer capacity payments, which could range from 5 to 15 $/MWhe (Ingersoll et al., 2020). With an average wholesale electricity price of 25 $/MWhe and capacity payments of 15 $/MWhe, this nuclear plant would still be 20 $/MWhe away from being financially viable. Some of this shortfall could be made up through zero-emissions credits (ZECs), which some states have enacted in order to help subsidize clean energy. New York and Illinois both offer (ZECs) that had initial prices of 17.50 $/MWhe (Sciascia, 2017) and 16.50 $/MWhe (IPA, 2017), respectively. Additionally, it is possible a ZEC program will be implemented federally in the U.S. by 2030 (Stokes et al., 2021). However, it is still uncertain if ZECs would provide enough revenue to make these plants financially viable.
A co-located DAC plant would provide an additional revenue stream that could make up for this difference. Though the power plant receives a revenue of $30 from the DAC plant for every MWh of electricity it produces, it only has 64% of this energy left to sell to wholesale electricity markets. Factoring this in, as shown in Table 4, instead of needing an average of 60 $/MWhe in order to break even, the SMR now only needs an average of 47 $/MWhe. In other words, the SMR needs 21% less revenue from conventional energy and capacity markets in order to be economically viable.
Since nuclear plants are capital-intensive projects, evidenced by recent high-profile failures in Vogtle, Georgia (Holt, 2018), the capital cost is a key consideration if determining whether or not to build a new nuclear power plant. Even though many next-generation nuclear reactors are being designed for modular manufacturing in order to reduce capital expenses, this still remains a primary concern. The SMR considered above with an LCOE of 60 $/MWhe, is consistent with a capital cost of 3,782 $/kW. However, there is a large uncertainty to capital costs for advanced nuclear plants, which could range from roughly 2,000 to 6,000 $/kW (EIRP, 2017).
The decision whether or not to build electricity generators depends on whether their capital costs allow the plant to be economically competitive based on current and anticipated market conditions. Table 5 shows a possible list of expected revenue sources, and the capital expenses that would be required, assuming the plant would receive these revenues on average over the course of its lifetime. Notably, most of the values in this table are reported in terms of $/MWhe produced by the SMR; the values in the second column are lower because the SMR can only sell 64% of its produced electricity to wholesale markets when also providing some energy to the DAC system. The SMR in our future baseline scenario that is built to only sell electricity can expect to receive an average revenue of 52 $/MWhe, and the plant would need to have a CAPEX3 of 3006 $/kW or lower to be economically viable. In comparison, the SMR that is built to sell electricity to a co-located DAC plant as well as to wholesale electricity markets can expect an average revenue of 65.45 $/MWhe, which would allow for a CAPEX up to 4,069 $/kW, or 35% greater, thus significantly increasing the chances it would be built.
One of the primary variables in determining our results is the average wholesale electricity price. This variable affects the LCOD of the DAC plant in the Baseline scenario, which then affects the difference in LCOD between the two scenarios, ultimately impacting the revenue that the SMR would receive from the DAC plant. The value used here, 25 $/MWh, is taken from Seel et al. (2018) and although there are many capacity expansion and production cost modeling reports to explore future electricity market scenarios, there are few instances in which the locational marginal price (LMP) of electricity is reported. Given the uncertainty around future electricity market conditions, we conducted a sensitivity analysis to examine how our results would change based on different average wholesale electricity prices.
For this sensitivity analysis, we chose a range of 15–60 $/MWh. Notably, varying this price also changes the LCOH for the heat pump, which provides heat to the Baseline scenario DAC plant. The new LCOH values were estimated by plotting a distribution of three heat pump scenarios given in Dominković (2015) and recalculating LCOH values based on its trendline. We then calculated new results for every 5 $/MWh change within this range and plotted the results, shown in Figures 3, 4 below.
Figure 3. Wholesale electricity price sensitivity for the reduction in conventional revenues (electricity and capacity revenue) an SMR must receive in order to break even, as a result of integration with a DAC system.
Figure 4. Wholesale electricity price sensitivity for the maximum allowable SMR CAPEX in order to break even.
In the first case of our results, we showed that, if co-locating a DAC plant, the SMR could take in 21% less revenue from conventional energy and capacity markets and still break even. Figure 3 shows how that percentage will change based on different wholesale electricity prices. As the wholesale electricity price increases, the LCOD for the baseline DAC plant will increase; thus receiving energy from an alternative source becomes increasingly valuable. This is reflected in the results by an increasing revenue that the SMR would receive from DAC for its energy as part of their bilateral agreement, as wholesale electricity prices increase. When able to rely on a higher revenue from the DAC plant, the SMR would then require an even lower price from selling the rest of its electricity to wholesale markets in order to breakeven. The graph shows this percent improvement from its LCOE of 60 $/MWh to the new price it would be required to sell electricity at to remain economically viable, which ranges from 56 to 18 $/MWh.
In the second case in our results, we showed that if the SMR can incorporate this revenue from the DAC plant, it would be acceptable for its CAPEX to be 35% higher than if it were only developed for selling electricity to wholesale markets, or 4,069 $/kW as opposed to 3,006 $/kW. In the baseline scenario, as the expected average electricity revenue increases, the maximum allowable CAPEX also increases, ranging from 2,037 to 6,401 $/kW for 15–60 $/MWh. This range of CAPEX estimates is consistent with the CAPEX range for advanced nuclear plants in EIRP (2017), as noted above. Incorporating both the changing expected average electricity revenue and DAC payments, in the co-generation scenario, the allowable CAPEX ranges from 2,915 to 8,107 $/kW. Figure 4 shows that as wholesale electricity prices increase, the relative difference between allowable CAPEX in our two scenarios also increases.
The analysis in this study estimated the potential benefits of integrating a nuclear SMR with a co-located DAC plant. It is more economically attractive for the nuclear plant to utilize and sell some low-grade heat along with some electricity than to only sell electricity. These results are notable because scaling of DAC will require a significant amount of low-carbon energy. Even though wholesale electricity prices are expected to decrease due to build-outs of VRE, it is expensive to convert electricity back into the heat needed for DAC. Advanced nuclear reactors and other firm low-carbon resources will be needed to help meet increases in electricity demand, replace fossil fuel generation, and help lower total costs of decarbonization. Importantly, these resources also will have the ability to meet the demand from DAC for low-carbon heat. The analysis in this study shows that if firm low-carbon resources can sell low-grade heat to DAC, in addition to selling electricity, this will increase their financial viability and create more opportunities for building new plants.
Our sensitivity analysis showed that if the future average price of wholesale electricity is higher than 25 $/MWh, DAC and SMR integration provides even more value to the SMR facility because the DAC facility would be willing to pay more to the nuclear plant in their bilateral agreement rather than buy more expensive electricity from the market. Conversely, if average electricity prices fall below 25 $/MWh, the benefits to the SMR facility are reduced, though still positive if the average electricity remains above ~10 $/MWh.
Throughout the results section we did not specify a size for the SMR. Instead, we assumed that for every MWh of electricity that the SMR produces, it also produces one MWh of steam at 100°C for the DAC plant. This was done in order to keep the size of the SMR dependent on the size and energy requirements of the DAC plant, and to not give preference to a specific advanced nuclear technology. In reality, this dependency likely would be reversed, as LTSS DAC plants currently being developed are much more modular at smaller scales than what would be possible for advanced nuclear plants. However, a literature review on DAC uncovered only one paper that provided a detailed enough techno-economic breakdown of a LTSS DAC plant that could be replicated (Fasihi et al., 2019). Thus, for the purposes of our analysis, the size and capital cost of the DAC plant was the main constraint.
Even though it was not mentioned in our results, this assumption allows for a determination of the size SMR required for our specific DAC plant. Based on its output and energy requirements, our DAC plant requires 51.25 MWt of thermal energy. Thus, the SMR must produce 51.25 MWt of 100°C steam. Considering that utilizing this amount of steam equates to a 15% reduction in electricity generated, the nameplate capacity of our SMR would be 60 MWe.
It is instructive to estimate the potential market for firm low-carbon power resources that a DAC industry might create. However, first, we must determine how much DAC could be feasibly built by the year 2030. Hanna et al. (2021) models the potential net removal from DAC if an international wartime-like deployment of DAC is enacted in response to climate change. With this type of concerted deployment effort, by 2030, DAC could reach removal rates of 0.03–0.07 GtCO2 (Hanna et al., 2021, p. 6). Assuming the average energy requirements shown in Table 2, this would create a demand for about 2–5 GW of new energy. This could represent a considerable market opportunity for SMRs, or other firm low-carbon power developers looking to deploy their products.
Since our analysis was focused on the economics of advanced nuclear, it also may be useful to compare this to alternatives. As mentioned earlier, the average LCOE for advanced nuclear is projected to be 60 $/MWh, with a range of 36–90 $/MWh. Their capital costs likely will range from 2,000 to 6,000 $/kW, with an average of 3,782 $/kW (EIRP, 2017). Two other generation sources that can offer firm low-carbon power are natural gas with CCS and geothermal. Even with CCS integrated, natural gas plants are simpler to develop than nuclear plants, but are much more reliant on fuel costs. The capital expenses for a natural gas plant with CCS are estimated to be much lower on average than nuclear, at 1,984 $/kW, but the LCOE estimation is higher, at 74 $/MWh (James et al., 2019). There is much more uncertainty around the costs of geothermal systems, as they will heavily depend on technology, location, and well-characteristics. Thus, estimates for geothermal capital expenses range from 3,000 $/kW at an absolute minimum to tens of thousands (NREL ATB, 2020), while LCOE for geothermal range from 69 to 112 $/MWh (Lazard, 2019).
Lastly, in our analysis we assume that DAC plants will be economically viable by 2030, and that they at least will earn more revenue for the captured CO2 than their levelized cost. However, concerted government policies or drastic market developments are needed in order for this to become a reality. Policies or programs that provide payment for the captured CO2 may come in the form of carbon pricing, such as a carbon tax or cap-and-trade system, which could allow DAC to receive a direct payment at the set carbon price; tax credits, whether performance-based that provide revenue per captured ton of CO2, or investment-based that lower the capital costs required to build; or low-carbon fuel standards, which would allow DAC to receive offset credits. In addition, revenue for DAC could come from selling captured CO2 to various markets that would utilize the CO2 for other purposes, such as enhanced oil recovery (EOR), polymers, synthetic fuels, chemicals such as ammonia, urea, or methanol, and concrete production (Hepburn et al., 2019).
This study sought to highlight the potential synergies between a DAC plant and firm low-carbon power plants. However, a number of assumptions and simplifications had to be incorporated in our analysis, which leaves room for future research endeavors. This might include a similar techno-economic comparison between a DAC system and other types of firm low-carbon resources, a focus on a specific SMR design and its optimal economic integration along with varying sizes of DAC systems, or an analysis utilizing detailed capacity-expansion modeling that would provide a more detailed look at potential future energy costs and revenues, which could then facilitate an analysis of the economic feasibility of the firm low-carbon power resource.
Two key technologies that can help to mitigate climate change are firm low-carbon power and DAC. Firm low-carbon resources can help decarbonize the power sector by replacing fossil fuel resources, working to meet increasing electricity demand, and reducing potential costs associated with the integration of VREs. DAC also will be needed to help offset emissions from sectors that may be too difficult or expensive to decarbonize, and to help reduce the vast sums of carbon that have already been emitted into the atmosphere. DAC systems will require a large amount of energy, most of it heat, but this creates another opportunity for firm low-carbon power.
This study aimed to delve deeper into this opportunity and demonstrate how it might incentivize building more firm low-carbon power. We examined the economics of a hypothetical DAC plant in the year 2030 and determined a potential revenue amount its owners would pay for energy from a co-located nuclear SMR. We then suggested two examples of how this additional revenue could benefit the SMR. It could allow the SMR to take in revenue from selling electricity to wholesale markets that is about 21% less than its projected levelized cost, and still break even financially. This would enable the SMR to operate economically even in the face of variable and decreasing wholesale electricity prices. In addition, after estimating a potential external revenue stream, we show that additional revenue from DAC could allow for capital costs of the same SMR up to 35% higher than what would be required if only selling electricity to wholesale markets. For an energy resource that has been expensive to build, revenue from DAC could offer developers more financial certainty when planning a new project.
We illustrated the potential for synergy between advanced nuclear plants and DAC systems, while also noting that this benefit could extend to other firm low-carbon resources. The findings from this study can be used to inform policy-makers, technology developers, or investors as they plan how to provide low-carbon electricity and heat to new DAC facilities.
The original contributions presented in the study are included in the article/Supplementary Material, further inquiries can be directed to the corresponding author/s.
DS formulated the main idea for the paper and performed the bulk of the analysis and writing. SL helped shape the direction of the paper, advised heavily on the analysis, and performed much of the editing. All authors contributed to the article and approved the submitted version.
DS performed this analysis as part of a capstone Master's project at Johns Hopkins University. Open access fees are to be paid by the Advanced Research Projects Agency-Energy.
The authors declare that the research was conducted in the absence of any commercial or financial relationships that could be construed as a potential conflict of interest.
All claims expressed in this article are solely those of the authors and do not necessarily represent those of their affiliated organizations, or those of the publisher, the editors and the reviewers. Any product that may be evaluated in this article, or claim that may be made by its manufacturer, is not guaranteed or endorsed by the publisher.
We would like to thank Malcolm Handley for feedback with the analysis and Daniel Zachary for guidance throughout the writing process.
The Supplementary Material for this article can be found online at: https://www.frontiersin.org/articles/10.3389/fclim.2021.728719/full#supplementary-material
1. ^Fasihi et al. (2019) reports all costs in Euros. The exchange rate used is 1.33 USD/EUR.
2. ^While in reality, a detailed pro forma cost breakdown that calculates estimated net present value would be the appropriate financial input in determining whether or not a new power plant should be built, for our purposes the LCOE gives a close-enough approximation.
3. ^The CAPEX is calculated based on average values taken from EIRP (2017). The OPEX is assumed to be 21 $/MWh and the capacity factor 95%. The capital recovery factor (crf) is calculated with an interest rate of 7% over 25 years.
Bistline, J. E. T., and Blanford, G. J. (2021). Impact of carbon dioxide removal technologies on deep decarbonization of the electric power sector. Nat. Commun. 12:3732. doi: 10.1038/s41467-021-23554-6
Dominković, D. (2015). The Role of Large Scale Heat Pumps in Future Energy Systems. (MEng Thesis), Technical University of Denmark, Kongens Lyngby, Denmark. Available online at: https://www.researchgate.net/publication/305073631_The_Role_of_Large_Scale_Heat_Pumps_in_Future_Energy_Systems (accessed June 14, 2021).
EIRP (2017). What Will Advanced Nuclear Power Plants Cost. Available online at: https://www.innovationreform.org/wp-content/uploads/2018/01/Advanced-Nuclear-Reactors-Cost-Study.pdf (accessed June 14, 2021).
Eto, J. (2016). Building Electric Transmission Lines: A Review of Recent Transmission Projects. Available online at: https://emp.lbl.gov/publications/building-electric-transmission-lines (accessed June 14, 2021).
Fasihi, M., Efimova, O., and Breyer, C. (2019). Techno-economic assessment of CO2 direct air capture plants J. Clean. Prod. 224, 957–980. doi: 10.1016/j.jclepro.2019.03.086
Friedmann, J., Fan, Z., Ochu, E., Sheeazi, H., Byrum, Z., and Bhardwaj, A. (2020). Levelized Cost of Carbon Abatement. Available online at: https://www.energypolicy.columbia.edu/research/report/levelized-cost-carbon-abatement-improved-cost-assessment-methodology-net-zero-emissions-world (accessed June 14, 2021).
Hanna, R., Abdulla, A., Xu, Y., and Victor, D. (2021). Emergency Deployment of Direct Air Capture as a Response to the Climate Crisis. Available online at: https://www.nature.com/articles/s41467-020-20437-0 (accessed June 14, 2021).
Hepburn, C., Adlen, E., Beddington, J., Carter, E., Fuss, S., MacDowell, N., et al. (2019). The technological and economic prospects for CO2 utilization and removal Nature 575, 87–97. doi: 10.1038/s41586-019-1681-6
Holt, M. (2018). Nuclear Energy: Overview of Congressional Issues. Available online at: https://crsreports.congress.gov/product/pdf/R/R42853 (accessed June 14, 2021).
Ingersoll, E., Gogan, K., Herter, J., and Foss, A. (2020). Cost and Performance Requirements for Flexible Advanced Nuclear Plants in Future U.S. Power Markets. Available online at: https://www.lucidcatalyst.com/arpa-e-report-nuclear-costs. doi: 10.2172/1646858 (accessed June 14, 2021).
IPA (2017). Zero Emission Standard Procurement Plan. Available online at: https://www2.illinois.gov/sites/ipa/Documents/2018ProcurementPlan/Zero-Emission-Standard-Procurement-Plan-ICC-Filing.pdf (accessed June 14, 2021).
IPCC (2018). Global Warming of 1.5°C. Available online at: https://report.ipcc.ch/sr15/pdf/sr15_spm_final.pdf (accessed June 14, 2021).
James, R., Zoelle, A., Keairns, D., Turner, M., Woods, M., and Kuehn, N. (2019). Cost and Performance Baseline for Fossil Energy Plants Volume 1. Available online at: https://netl.doe.gov/projects/files/ (accessed June 14, 2021).
Keith, D., Holmes, G., Angelo, D., and Heidel, K. (2018). A process for capturing CO2 from the atmosphere. Joule 8, 1573–1594. doi: 10.1016/j.joule.2018.05.006
Lazard (2019). Lazard's Levelized Cost of Energy Analysis - Version 13.0. Available online at: https://www.lazard.com/media/451086/lazards-levelized-cost-of-energy-version-130-vf.pdf (accessed June 14, 2021).
Mai, T., Jadun, P., Logan, J., McMillan, C., Muratori, M., Steinberg, D., et al. (2018). Electrification Futures Study: Scenarios of Electric Technology Adoption and Power Consumption for the United States. Available online at: https://www.nrel.gov/docs/fy18osti/71500.pdf (accessed June 14, 2021).
McQueen, N., Psarras, P., Pilorgé, H., Liguori, S., He, J., Yuan, M., et al. (2020). Cost analysis of direct air capture and sequestration coupled to low-carbon thermal energy in the United States. Environ. Sci. Technol. 54, 7542–7551. doi: 10.1021/acs.est.0c00476
National Academies of Sciences Engineering Medicine (2019). Negative Emissions Technologies and Reliable Sequestration: A Research Agenda. Available online at: https://www.nap.edu/catalog/25259/negative-emissions-technologies-and-reliable-sequestration-a-research-agenda (accessed June 14, 2021).
NREL ATB (2020). Annual Technology Baseline Geothermal. Available online at: https://atb.nrel.gov/electricity/2020/index.php?t=gt (accessed June 14, 2021).
Realmonte, G., Drouet, L., Gambhir, A., Glynn, J., Hawkes, A., Köberle, A. C., et al. (2019). An inter-model assessment of the role of direct air capture in deep mitigation pathways. Nat. Commun. 10:3277. doi: 10.1038/s41467-019-10842-5
Sanz-Pérez, E., Murdock, C., Didas, S., and Jones, C. (2016). Direct capture of CO2 from ambient air. Chem. Rev. 116, 11840–11876. doi: 10.1021/acs.chemrev.6b00173
Sciascia, M. (2017). New York State's Zero Emission Credits: Exploring the Drivers and Significance of Nuclear Energy Subsidization in the Empire State. Available online at: https://digitalworks.union.edu/cgi/viewcontent.cgi?article=1081&context=theses (accessed June 14, 2021).
Seel, J., Mills, A., and Wiser, R. (2018). Impacts of High Variable Renewable Energy Futures on Wholesale Electricity Prices, and on Electric-Sector Decision Making. Available online at: https://emp.lbl.gov/publications/impacts-high-variable-renewable (accessed June 14, 2021).
Sepulveda, N., Jenkins, J., de Sisternes, F., and Lester, R. (2018). The role of firm low-carbon electricity resources in deep decarbonization of power generation. Joule 2, 2403–2420. doi: 10.1016/j.joule.2018.08.006
Stokes, L., Ricketts, S., Quinn, O., Subramanian, N., and Hendricks, B. (2021). A Roadmap to 100% Clean Electricity by 2035. Available online at: https://www.evergreenaction.com/policy-hub/evergreen-ces-report.pdf (accessed June 14, 2021).
Keywords: climate change, direct air capture, firm low-carbon power, nuclear, small modular reactor
Citation: Slesinski D and Litzelman S (2021) How Low-Carbon Heat Requirements for Direct Air Capture of CO2 Can Enable the Expansion of Firm Low-Carbon Electricity Generation Resources. Front. Clim. 3:728719. doi: 10.3389/fclim.2021.728719
Received: 21 June 2021; Accepted: 09 August 2021;
Published: 07 September 2021.
Edited by:
Mijndert Van Der Spek, Heriot-Watt University, United KingdomReviewed by:
Shareq Mohd Nazir, KTH Royal Institute of Technology, SwedenCopyright © 2021 Slesinski and Litzelman. This is an open-access article distributed under the terms of the Creative Commons Attribution License (CC BY). The use, distribution or reproduction in other forums is permitted, provided the original author(s) and the copyright owner(s) are credited and that the original publication in this journal is cited, in accordance with accepted academic practice. No use, distribution or reproduction is permitted which does not comply with these terms.
*Correspondence: Scott Litzelman, c2NvdHQubGl0emVsbWFuQGhxLmRvZS5nb3Y=
Disclaimer: All claims expressed in this article are solely those of the authors and do not necessarily represent those of their affiliated organizations, or those of the publisher, the editors and the reviewers. Any product that may be evaluated in this article or claim that may be made by its manufacturer is not guaranteed or endorsed by the publisher.
Research integrity at Frontiers
Learn more about the work of our research integrity team to safeguard the quality of each article we publish.