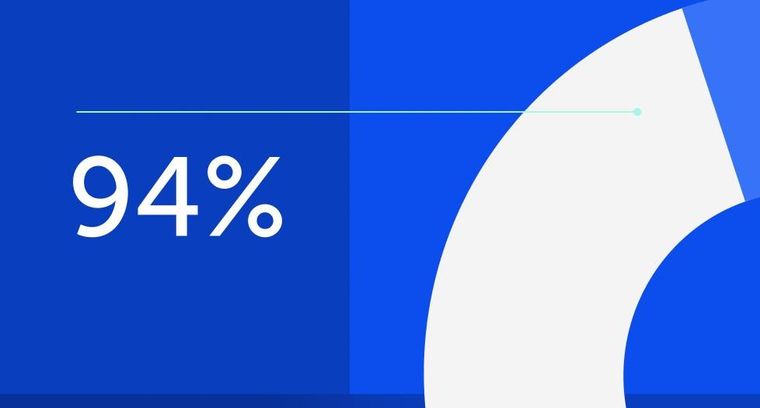
94% of researchers rate our articles as excellent or good
Learn more about the work of our research integrity team to safeguard the quality of each article we publish.
Find out more
REVIEW article
Front. Clim., 15 November 2019
Sec. Carbon Dioxide Removal
Volume 1 - 2019 | https://doi.org/10.3389/fclim.2019.00009
This article is part of the Research TopicThe Role of Negative Emission Technologies in Addressing Our Climate GoalsView all 10 articles
Since the Industrial Revolution, anthropogenic carbon dioxide (CO2) emissions have grown exponentially, accumulating in the atmosphere and leading to global warming. According to the IPCC (IPCC Special Report, 2018), atmospheric warming should be <2°C to avoid the most serious consequences associated with climate change. This goal may be achieved in part by reducing CO2 emissions, together with capturing and sequestering CO2 from point sources. The most mature storage technique is sequestration in deep saline aquifers. In addition, CO2 can be mineralized and sequestered in solid form by various techniques, i.e., ex-situ, surficial and in situ mineralization. Ex situ and surficial approaches may produce valuable products while mitigating environmental hazards. In-situ mineralization uses ultramafic and mafic geological formations for permanent, solid storage. In addition, the IPCC portfolio that limits warming to <2°C by 2100 includes avoiding CO2 emissions and removal of CO2 from air. Regardless of the specific mix of approaches, it will be essential to permanently sequester about 10 billion tons of CO2 per year by mid-century, and roughly twice that amount each year by 2100. Maximizing the potential of technologies for CO2 removal from air and CO2 storage will help to meet global climate goals. The research agenda published by National Academies of Sciences Engineering Medicine (2019) calls for roughly $1 billion over a 10–20 years time period to advance the deployment of CO2 sequestration in deep sedimentary reservoirs at the GtCO2/yr scale and develop CO2 mineralization at the MtCO2/yr scale. This would lead to a deeper understanding of the reservoir characteristics from the nano- to kilometer scale, some of which may include the distribution of the reaction products, the reaction rate of the minerals, the permeability evolution, the pressure build-up in the reservoir, the large-scale impact of chemicophysical processes leading to clogging or cracking, the effects of potential geochemical contamination, etc. This overview presents the advantages, drawbacks, costs, and CO2 storage potential of each technique, the current and future projects in this domain, and potential sequestration options in geologic formations around the world.
The cumulative release of anthropogenic carbon dioxide (CO2) into the atmosphere has been estimated at 2,035 ± 205 GtCO2 from 1870 to 2015 (Le Quéré et al., 2015). Today, emissions are about 40 GtCO2/yr (IPCC Special Report, 2018). Each year, roughly half of these emissions are removed naturally by uptake in the ocean and the terrestrial biosphere, while the remainder accumulates in the atmosphere and contributes to global warming. To avoid the worst impacts of global warming and subsequent climate change, the Paris agreement recommended limiting average warming of the atmosphere to <2°C, and preferably <1.5°C (UNFCCC, 2015).
Recently the IPCC published a report stating that, to reach the 1.5°C goal, negative emission technologies have to remove tens of Gt CO2 per year (IPCC Special Report, 2018), in addition to reducing emissions and capturing CO2 from point sources. Options for CO2 removal from air (CDR) include an increase of carbon storage in soils and biomass, but also Direct Air Capture using synthetic sorbents (DACSS) and carbon mineralization via enhanced weathering (DACEW). The likely scenario is that most of these options, operating in parallel, will be necessary to achieve the required level of global CDR, approximately 10 Gt/yr by 2050, and 20 Gt/yr by 2100 (United Nations Environment Programme (UNEP), 2017; National Academies of Sciences Engineering Medicine, 2019). While utilization of some captured CO2 may be attractive, to produce fuels with net-zero emissions for example, most of the captured CO2, from point sources and from air, must be permanently stored. Starting now, carbon capture and storage (CCS) methods must sequester about 125 Gt of CO2 by 2100 (National Academies of Sciences Engineering Medicine, 2019). Two possible methods for long-term CO2 storage are underground sequestration in sedimentary formations and carbon mineralization (National Academies of Sciences Engineering Medicine, 2019).
Underground CO2 sequestration in sedimentary formations is the most mature technique for storage of CO2 captured from point sources, and perhaps by DAC, as commercial-size CO2 injection projects are already operating today. This approach has been carried out over two decades globally, currently storing roughly 3.7–4.2 MtCO2/year, for a cumulative total of 30.4 MtCO2 at the end of 2017 (Global CCS Institute, 2019; National Academies of Sciences Engineering Medicine, 2019). Additionally, roughly 28 MtCO2/year of captured CO2 are injected into the ground for CO2-enhanced oil recovery (CO2-EOR) (Global CCS Institute, 2019). Approximately 85% of CO2-EOR projects use CO2 sourced from natural CO2 gas fields (DiPietro et al., 2012). Less common use of CO2 captured from smokestacks or removed from air leads to a lower carbon footprint of produced oil. More specifically, in the U.S., about 64 MtCO2/yr are used for CO2-EOR, from which 21 MtCO2/yr is from anthropogenic sources (U.S. Environmental Protection Agency (EPA), 2016). Conventional CO2-EOR today is optimized to produce as much oil as possible with the least amount of CO2 injection. In practice, about 0.3–0.6 tons of CO2 is injected for every barrel of oil produced—which compares to the 0.4 tons of CO2 emitted when the oil is burned (McGlade, 2019). During oil production, some of the CO2 is produced along with the oil. In many such cases, it is separated from the oil and reinjected underground. Larger amounts of CO2 could be sequestered in depleted oil reservoirs if advanced EOR+ or maximum storage EOR+, both which optimize the amount of CO2 sequestered, were used in place of conventional CO2-EOR. The combined underground storage capacity in saline aquifers and hydrocarbon reservoirs is estimated to range between 5,000 and 25,000 Gt CO2 (de Coninck and Benson, 2014), which is larger than necessary to limit warming to <1.5°C through 2100 (Rogelj et al., 2018).
Another storage option is carbon mineralization of mafic and ultramafic rocks. This has been implemented in the Carbfix Project in Iceland. There, more than 10,000 tons of CO2 captured from geothermal steam is injected into subsurface pore space in basaltic lavas, where it is mineralized within a year (Gislason et al., 2010; Aradóttir et al., 2011; Matter et al., 2011, 2016; Snæbjörnsdóttir et al., 2017; Gunnarsson et al., 2018). This approach is at a lower stage of technological readiness and is under continued investigation on the lab- and pilot-scales. Globally, carbon mineralization in these rock types has the potential of sequestering up to 60,000,000 GtCO2 if the resource is economically-accessible and ultimately fully carbonated.
Carbon mineralization was proposed 30 years ago as a strategy for CO2 removal from the atmosphere (Seifritz, 1990; Lackner et al., 1995). It is a long-term and non-toxic method of storing CO2 in solid form, that can also help in mitigating health and environmental hazards in specific contexts (National Academies of Sciences Engineering Medicine, 2019). This strategy of underground sequestration increases the uptake of CO2 in the reservoir through the interaction with rocks bearing magnesium or calcium ions (Gunter et al., 1993). Additionally, since it is converted to a stable carbonate form, this represents the safest storage mechanism with regard to minimizing leakage (Zhang and DePaolo, 2017).
Carbon mineralization involves the formation of solid carbonate minerals (calcite, magnesite, dolomite, and a variety of hydrated Mg-carbonate minerals such as nesquehonite) through reaction of CO2 (gas, liquid, dissolved in water, or supercritical) with rocks rich in calcium or magnesium. The best sources of Mg and Ca are mafic and ultramafic rocks (mantle peridotite, basaltic lava, and ultramafic plutons), mine tailings (mafic and ultramafic), and industrial byproducts (cement kiln dust, steel slag, and fly ash). The source of CO2 varies depending on the context and local availability, including ambient air (~0.04% CO2), flue gas from the power sector (~5–12% CO2), seawater or Mg-rich peridotite groundwater saturated in air (~0.01% CO2), fluids enriched in or saturated with CO2, or high purity CO2 gas and fluid (+99.5%). Direct Air Capture via Enhanced Weathering (DACEW) uses CO2 directly from air or surface water for the purpose of carbon mineralization, and can be combined with long-term solid storage (Kelemen et al., under review).
Various methods exist for carbon mineralization: (1) ex-situ, where the alkalinity source is transported to a site of CO2 capture, ground to a small particles, and combined with CO2 in a high temperature and pressure reaction vessel, (2) surficial, where dilute or concentrated CO2 is reacted with the alkalinity source on-site at the surface (e.g., mine tailings, smelter slag), and (3) in-situ, where CO2-bearing fluids circulate through subsurface porosity in geological formations. The rate and cost of carbon mineralization depends on available CO2 dissolved in solution, available alkalinity in the solution, and optimal reaction conditions (Kelemen et al., under review). Variations in pH are particularly important, as low pH favors mineral dissolution whereas high pH accelerates carbonate precipitation (Park and Fan, 2004; Pokrovsky and Schott, 2004).
Research results are difficult to compare because of the wide range of reagents and conditions used. Few comparisons of carbon mineralization kinetics exist as a function of temperature, CO2 partial pressure, and other variables (Matter and Kelemen, 2009; Kelemen et al., 2011; Gadikota et al., in preparation). Several processes have been considered. For instance, in two-step processes, the first step involves the dissolution of the alkalinity source at low pH, with precipitation of carbonates during a second step at high pH. In most cases, the dissolution of the alkalinity source is the rate-limiting step. Alternatively, dissolution of silicates and carbon mineralization may be combined into a single-step process. Experiments with olivine as the alkalinity source used NaHCO3 as a catalyst that buffers the pH (O'Connor et al., 2005; Chizmeshya et al., 2007; Gadikota et al., 2014). In the natural reactive flow of water through ultramafic rocks, a pH-swing occurs spontaneously making both dissolution of the alkalinity source and precipitation of carbonate possible (Bruni et al., 2002; Paukert et al., 2012; Canovas et al., 2017; Paukert Vankeuren et al., 2019; Leong and Shock, under review). Carbon mineralization rates of serpentine may be increased by heat treatment, which removes hydroxyl groups from the mineral and increases the rate of dissolution and carbon mineralization (e.g., O'Connor et al., 2005; Li et al., 2009; Larachi et al., 2012).
The mineral wollastonite (CaSiO3) has the highest reaction rate with CO2, in the range of 8.0·10−9-2.0·10−7 mol·m−2·s−1 at 25°C and in the range of 1.6·10−5-5.0·10−4. mol·m−2·s−1 at 180°C (Figure 1) (Palandri and Kharaka, 2004; O'Connor et al., 2005). However, the availability of wollastonite is low, with global reserves estimated at only 100M tons. Its high Ca content makes it a good analog for industrial waste byproducts (i.e., cement kiln dust, steel slag, and fly ash), that may have initial reaction rates similar to wollastonite, but they generally contain less Ca, and/or contain Ca-Al silicates that are slow to react [e.g., (Huijgen et al., 2007; Pan et al., 2012; Sanna et al., 2014; Gadikota et al., 2015; National Academies of Sciences Engineering Medicine, 2019)].
Figure 1. Dissolution rates of magnesium- and calcium-rich minerals at 25°C (blue) and 180°C (orange). The plagioclase feldspars are a solid solution between a sodium-end-member (albite NaAlSi3O8) and a calcium-end-member (anorthite CaAl2Si2O8) indicated as Na and Ca. The most common plagioclase feldspar in basalts is labradorite (labrad.). At high temperature, there is less variation of dissolution rates as a function of composition for plagioclase feldspars [Figure modified from National Academies of Sciences Engineering Medicine (2019), after data from Palandri and Kharaka (2004) and Thom et al. (2013)].
Olivine (Mg2SiO4) reacts with CO2-bearing aqueous fluids almost as fast as wollastonite (Figure 1) (Palandri and Kharaka, 2004; National Academies of Sciences Engineering Medicine, 2019). Additionally, as the major mineral of peridotite, which constitutes the Earth's upper mantle, olivine is highly abundant, making it a good candidate for carbon mineralization. Addition of NaHCO3 in single-step carbon mineralization experiments buffers the pH and helps for both dissolution and carbon mineralization reactions (O'Connor et al., 2005; Chizmeshya et al., 2007; Gadikota et al., 2015).
Plagioclase feldspars, one of the major constituents of basalt and gabbro (the major constituents of the oceanic crust), have lower reaction rates than wollastonite and olivine. At low temperature (25°C) the calcium-rich end-member (anorthite CaAl2Si2O6) dissolves faster than the sodium-rich end-member (albite NaAlSi3O6). In basalts, the plagioclase feldspar is usually labradorite (50–70% anorthite), which has reaction rates in the range of 1.3·10−11-2.0·10−10 mol·m−2·s−1 at 25°C, and 6.3·10−9-6.3·10−8 mol·m−2·s−1 at 180°C (Figure 1) (Palandri and Kharaka, 2004; Gadikota et al., in preparation; National Academies of Sciences Engineering Medicine, 2019). To the extent that steel slag and smelting waste contain Ca-Al silicate compounds similar to anorthite, formation of calcite (CaCO3) may be retarded by slow diffusion of Al.
The dissolution rate of serpentine (Mg3Si2O5(OH)4) is similar to the lower bound for plagioclase feldspars (Figure 1) (Thom et al., 2013; National Academies of Sciences Engineering Medicine, 2019). Serpentinite is formed by the alteration of peridotite, which is often partial. This results in a mineral association of serpentine, olivine, and brucite (Mg(OH)2), with olivine and brucite being among the most reactive minerals for carbon mineralization. Also, fibrous “asbestiform” chrysotile serpentine, composed of nano-scale cylinders, allows for rapid carbon mineralization due to its high surface-to-volume ratio. Consuming asbestiform serpentine also mitigates health hazards due to asbestos contained in mine tailings (National Academies of Sciences Engineering Medicine, 2019).
The low crystallinity of amorphous basaltic glass and some industrial byproducts may make them more reactive than crystalline minerals and rocks with the equivalent composition (Oelkers and Gislason, 2001; Gislason and Oelkers, 2003).
Reaction between the solid source of alkalinity and dissolved or supercritical CO2 can coat grains of solid reactants, thereby reducing the reactive surface area and limiting the extent of reaction (known as “passivation”). However, in most studies, there is no negative effect of passivation observed for olivine, for which the rate carbon mineralization is constant, in part due to reaction-driven cracking and formation of etch pits as the reaction proceeds (Gadikota et al., in preparation). This is not the case for many other reactants, for which the reaction rate decreases over time (perhaps due to passivation). This could be an issue for extensive carbonation of mafic intrusions and basalts rich in plagioclase feldspars and pyroxenes. Understanding the key parameters that favor reaction-driven cracking rather than surface passivation is essential for forecasting the extent of carbon mineralization reactions (National Academies of Sciences Engineering Medicine, 2019).
The most studied mineral for ex-situ carbon mineralization is olivine, as it is abundant, has a high reaction rate, and exhibits little to no passivation. The optimum temperature for carbon mineralization of olivine is ~185°C (O'Connor et al., 2005; Gadikota et al., 2014). Serpentine-rich mine tailings are an intriguing option as the carbonation of asbestiform chrysotile would mitigate health and environmental hazards, and products of rapid heat-treatment of serpentine-rich mine tailings could be used as a feedstock for carbon mineralization using concentrated sources of CO2 (McKelvy et al., 2004; Maroto-Valer et al., 2005; O'Connor et al., 2005; Li et al., 2009; Larachi et al., 2010, 2012; Balucan et al., 2011; Fedoročková et al., 2012; Balucan and Dlugogorski, 2013; Werner et al., 2013, 2014; Dlugogorski and Balucan, 2014; Ghoorah et al., 2014; Hariharan et al., 2014, 2016; Pasquier et al., 2014; Sanna et al., 2014; Hariharan and Mazzotti, 2017). However, these ex-situ methods are more expensive than the projected cost of direct air capture of CO2, and significantly more expensive than CO2 storage in subsurface pore space (see section Costs and Reservoir Capacities).
Another interesting source of alkalinity is waste byproducts (i.e., cement kiln dust, steel slag, smelter slag, and fly ash) (Huijgen et al., 2007; Pan et al., 2012; Sanna et al., 2014; Gadikota et al., 2015) because these are directly available for carbon mineralization. Using these materials for carbon mineralization also mitigates hazards due to chemical contamination associated with alkaline industrial wastes. However, it should be kept in mind that these contain variable, sometimes low concentrations of Ca and Mg, and that Ca may be “locked up” in slow-reacting Ca–Al silicate species. Ex-situ carbon mineralization using industrial waste, wollastonite, and olivine (excluding mine tailings) has the potential to carbonate many GtCO2/yr (Kelemen et al., 2011; Renforth et al., 2011; Sanna et al., 2014; Gadikota and Park, 2015; National Academies of Sciences Engineering Medicine, 2019). Nevertheless, costs remain high, and producing this volume of carbonated material raises additional questions regarding handling, transportation, and disposal.
The cost of ex-situ carbon mineralization is about 10 times higher than CO2 injection and sequestration into subsurface reservoirs (National Academies of Sciences Engineering Medicine, 2019). Ex-situ carbon mineralization could instead be used to create valuable products (Huijgen et al., 2007; Pan et al., 2012; Sanna et al., 2014; Gadikota et al., 2015), particularly for construction applications. If it were economically viable to use CO2-added aggregates in concrete, instead of currently-used materials, this could reduce the net emissions of the concrete industry. Replacing 10% of building material by material carbonated with anthropogenic CO2 could reduce CO2 emissions by up to 1.6 Gt/yr (Sridhar and Hill, 2011). Details about the mitigation capacity and the cost of various methods are provided in section Costs and Reservoir Capacities of this study.
Surficial carbon mineralization uses mafic and ultramafic mine tailings. At surface conditions most minerals in mafic and ultramafic rocks have relatively slow reaction rates, with the exception of brucite and asbestiform chrysotile (Power et al., 2011, 2013a; Harrison et al., 2013; Wilson et al., 2014). Mine tailings have a high surface area-to-volume ratio compared to subsurface geologic formations, resulting in increased reactivity. They are also low-cost sources provided that they are wastes of mafic and ultramafic rocks quarried or mined for other resources such as nickel, chromium, platinum-group elements, and diamonds. Mine tailings represent a small mitigation opportunity that would consume <36 MtCO2/yr and require potential transportation and large disposal areas (National Academies of Sciences Engineering Medicine, 2019). Still, mine tailings have a high uptake potential of carbon and mines that carbonate their tailings could offset on-site CO2-eq emissions (Harrison et al., 2013; Mervine et al., 2018).
Figure 2 summarizes data on the rate of carbon mineralization of mafic and ultramafic mine tailings in contact with air, and in contact with more CO2 rich gases and fluids. Carbon mineralization in mine tailings can be enhanced by several methods, such as sparging air, air-saturated water, or CO2-rich gas through the tailings (Assima et al., 2013, 2014; Harrison et al., 2013), stirring the tailings to have a turnover of reactive surfaces in contact with air (Kelemen et al., under review), or adding microorganisms that accelerate the formation of carbonates in disposal ponds (Power et al., 2011, 2013b). These methods can significantly increase the CO2 uptake rate of the tailings (Assima et al., 2013; Harrison et al., 2013). For instance, sparging CO2-rich gas through ultramafic tailings could accelerate the CO2 uptake rate up to a million times (Harrison et al., 2013; National Academies of Sciences Engineering Medicine, 2019). Though surficial mineral carbon mineralization methods could prove to be more expensive than the combination of Direct Air Capture using Synthetic Sorbents (DACSS) combined with geological sequestration, this method could provide local solutions where mine tailings are close to a local source of concentrated CO2. And, as noted above, mine tailings containing asbestos present health hazards that would be mitigated by carbon mineralization (National Academies of Sciences Engineering Medicine, 2019).
Figure 2. Reaction rate in mol·m−2 · s−1 of in-situ and surficial mineralization of CO2 using mine tailings or rocks as the source of alkalinity. Mine tailings are in gray, peridotite in dark green, basalt in purple, brucite in light green, and sedimentary rocks in orange. From National Academies of Sciences Engineering Medicine (2019) and references cited therein.
Another option for surficial carbon mineralization is mining of mafic and ultramafic rocks for the purpose of CO2 capture and storage, which might cost $55–500/tCO2 depending on the reactivity of specific tailings, the duration of their exposure to air, and the cost of “stirring” the tailings to prevent passivation (Kelemen et al., under review). This range of costs overlaps with the range of cost estimates for DACSS, combined with storage in geologic formations (National Academies of Sciences Engineering Medicine, 2019).
Recently, looping, or re-use, of Mg-rich mine tailings has been proposed (Kelemen et al., under review; McQueen et al., under review). The simplest version of this process would begin with magnesite (MgCO3). Heating of magnesite (“calcining”) produces a nearly pure stream of CO2 gas plus a solid residue of caustic magnesia (MgO). Subsequent weathering of caustic magnesia will rapidly remove CO2 from air, with nearly complete conversion to Mg-carbonates expected in about a year. Mg-carbonates can then be calcined again, and so on. Initial feedstock could also be ultramafic tailings, with the first step of calcining forming “heat-treated serpentine” and producing H2O + CO2 gas. After a few cycles of weathering, the solid residue of calcining is likely to be composed of caustic magnesia plus more or less crystalline SiO2 (opal, chalcedony, and quartz). The caustic magnesia can be used for many more cycles of weathering to remove CO2 from air. We believe that this process has costs and area requirements similar to, or less than, DACSS.
Grinding ultramafic and mafic rocks to sizes smaller than mine tailing sizes and spreading them over agricultural soil, forest soil, or along beaches is another possibility for CO2 removal (Figure 2) (e.g., Schuiling and Krijgsman, 2006; Renforth et al., 2015; Edwards et al., 2017; Kantola et al., 2017; Beerling et al., 2018). This process may be enhanced by microbial processes occurring in soils (Power et al., 2009, 2013b) and may be competitive with direct air capture using chemicals combined with geologic sequestration of CO2. However, mafic and ultramafic rocks contain nickel and chromium that, once oxidized, might present a significant health hazard if they accumulate in soils.
When CO2-bearing fluids or supercritical CO2 are injected at depth into geologic formations, the sequestration of CO2 in the subsurface porosity relies on the impermeability of the caprock of the reservoir. Suitable reservoirs are saline aquifers, depleted oil, and gas reservoirs (may or may not be associated with enhanced oil recovery, i.e., CO2-EOR), possibly hydraulically fractured shale formations, and thick formations of basalt and peridotite (Tao and Clarens, 2013; National Academies of Sciences Engineering Medicine, 2019). Ideal properties of saline formations for CO2 storage include high permeability to enable high rates of CO2 injection without large pressure buildup, thick formations with many interbedded low permeability barriers to use the pore space efficiently (Wen and Benson, 2019), and having salinity in excess of 10,000 ppm of total dissolved solids to comply with groundwater protection regulations. It is important to limit pore pressure buildup in order to avoid fracturing the caprock and inducing seismic events (National Academies of Sciences Engineering Medicine, 2019).
Secondary trapping mechanisms aim to improve CO2 storage and include solubility trapping by dissolution of the CO2 into aqueous pore fluid (Emami-Meybodi et al., 2015), residual gas trapping by capillary forces (Krevor et al., 2015), and mineralization by chemical interactions between the CO2, pore fluid, and rock (Figures 2, 3) (Zhang and DePaolo, 2017). The extent of secondary trapping mechanisms is highly site-specific, and depends on the geology, structure, and hydrology of each reservoir. For instance, increasing pore fluid salinity decreases CO2 solubility (Gunter et al., 1993). The purity of the CO2 also affects the storage capacity of the reservoir (Talman, 2015). Secondary trapping mechanisms in and of themselves may be sufficient for the mitigation of the risks of CO2 leakage (National Academies of Sciences Engineering Medicine, 2019), for example in basalt and peridotite formations where carbon mineralization rates are highest and 90% of the CO2 may be mineralized in a few months to decades (Figure 3, right panel) (National Academies of Sciences Engineering Medicine, 2019). The rate and capacity of mineral trapping are low in many sedimentary reservoirs due to the dominant quartz composition which has very limited reactivity with CO2 (Figure 3, left panel). In sedimentary settings, CO2 may remain mobile for centuries and trapping relies primarily on the impermeability of the caprock. This is observed for closed structural traps, in which fluids do not move far and both solubility and capillary trapping remain low. Conversely, large saline aquifers are hydrodynamic traps where CO2 moves rapidly through the pore space and interacts with a larger volume of the reservoir, increasing the extent of all secondary mechanisms (National Academies of Sciences Engineering Medicine, 2019).
Figure 3. Evolution of the extent of CO2 trapping mechanisms with time. The extent of each trapping mechanism is highly site specific and depends on several parameters including the type of rock: carbonatitic and siliciclastic rocks (left panel), or mafic and ultramafic rocks that have the ability to react much faster with CO2 to form carbonates (right panel) [from (National Academies of Sciences Engineering Medicine, 2019), Figure 6.7 and (Kelemen et al., under review), Figure 8, modified from (Benson et al., 2005); also see Figure 9 in (Snæbjörnsdóttir et al., 2017)].
The high porosity and permeability of basalt increases its reactivity with CO2 making it an ideal medium for CO2 injection and storage. Two pilot projects—Wallula in Washington state, USA and CarbFix in Iceland—demonstrate this high potential for CO2 storage in basalt. For both projects the composition, structure, and hydrology of the reservoir were studied, to assess the viability of the targeted reservoir for the injection and sequestration of CO2 in the rock pore space (National Academies of Sciences Engineering Medicine, 2019). The Wallula pilot plant successfully injected 977 tons of pure CO2 between 838 and 886 meters in depth over the course of 25 days during the summer of 2013 for the purpose of CO2 storage (McGrail et al., 2014, 2017a,b).
The aim of the CarbFix project is to dispose of H2S as well as CO2 derived from condensation of natural steam. In Phase I, ~200 tCO2 were injected at a depth of 500 m with a 100 m flow path through the basalt. New techniques were developed in both Phase I (Sigfusson et al., 2015) and Phase II (Gunnarsson et al., 2018) to inject water and CO2 separately with proportions insuring the complete dissolution of CO2 in water at depth. These techniques are very important, because they eliminate the need for an impermeable caprock and thereby greatly increases the number and extent of potential basalt storage reservoirs. Tracers including SF6 and 14C, were used to assess the fate of CO2 in the basalt-hosted aquifer. Rapid carbon mineralization removed dissolved CO2 from pore water within months. Phase II is more ambitious and continues to inject 10–20 ktCO2/yr and a roughly comparable mass of H2S at 1,500 m in depth, forecasting a 2,000 m flow path. Over 3 years, the carbon mineralization rate has been about 5·10−9 mol·m−2·s−1 (a value that can be compared with the summary of experimental data in Figure 2). This offers the potential to recycle pore water for continued co-injection with CO2, and addresses concerns about the extent of water consumption for solution trapping methods. Moreover, Climeworks has installed direct air capture unit on site, to investigate the potential for the project to be carbon negative (Gislason et al., 2010; Aradóttir et al., 2011; Matter et al., 2011, 2016; Snæbjörnsdóttir et al., 2017; Gunnarsson et al., 2018).
These pilot projects have demonstrated the potential for CO2 storage in basalt reservoirs (McGrail et al., 2014; Gíslason et al., 2018) and monitoring is ongoing for a better understanding of the process (Alfredsson et al., 2013; Matter et al., 2014, 2016). Basalts contain alteration minerals and glass that could be more reactive than the original, igneous minerals, which are generally more abundant (Oelkers and Gislason, 2001; Gislason and Oelkers, 2003; Wolff-Boenisch et al., 2006; Flaathen et al., 2010; Alfredsson et al., 2013). It is thus important to know the role and proportion of alteration minerals and glass at specific sites of carbon mineralization. Other concerns are potential formation of a passivation layer that may decrease the reactivity of the rock over time (Power et al., 2013b). Also, crystallization of reaction products in pore space may lead to clogging of pore space (Alfredsson et al., 2013) and subsequently, a decrease in permeability. Monitoring will quantify the evolution in space and time of a dissolution zone in acid waters close to the injection well, and a precipitation zone that will occur further away and pushed outwards as new carbonates are formed.
Storage of CO2 in basalts is estimated to cost $20–$30/tCO2, as compared to <$20, perhaps <$10, for storage in pore space in sedimentary reservoirs. Basalt storage is likely to be a preferred choice in volcanic provinces, and possibly additional gigantic near-shore reservoirs in the extensive basalt layer that comprises the upper few kilometers of oceanic crust, worldwide (Goldberg et al., 2008, 2010; Goldberg and Slagle, 2009). Because they cool very rapidly during submarine eruptions, basalts in oceanic crust may have higher proportions of basaltic glass that react much faster than crystalline basalt. Overall, abundant basalts, some close to populated areas, can potentially store thousands of gigatons of CO2.
Peridotite is the major constituent of Earth's upper mantle and is primarily composed of olivine, which reacts spontaneously with air or fluids containing CO2. The biggest massif of exposed peridotite is the Samail ophiolite in Oman and the United Arab Emirates. There are almost equally large massifs in New Caledonia and Papua New Guinea. The contiguous 48 US States contain relatively small bodies of peridotite that cumulatively have about the same mass as the Samail ophiolite (Krevor et al., 2009). The primary minerals in tectonically exposed mantle peridotite (mainly olivine, pyroxenes and spinel) transform partially or completely into mixtures of serpentine, brucite, Fe-oxides and -oxyhydroxides. Extensive carbon mineralization in the Samail ophiolite has been observed, forming carbonate veins in fractures, and travertine terraces around alkaline springs. The rate of natural carbon mineralization in the Samail ophiolite is on the order of 1,000 tCO2/km3/yr (Figure 2) (Kelemen and Matter, 2008; Kelemen et al., 2011; Streit et al., 2012; Mervine et al., 2014). The presence of listvenites—fully carbonated peridotites composed entirely of magnesite and dolomite (MgCO3, CaMg(CO3)2) + quartz (SiO2) + hematite and goethite (Fe2O3, FeO(OH)) + relict spinel and/or chromian mica—show that full carbonation of peridotites is possible at natural conditions (about 100°C) (e.g., Falk and Kelemen, 2015; Kelemen et al., 2018).
Peridotite reservoirs have the potential to mineralize and sequester 105-108 GtCO2 and are potentially the least expensive route for combined CO2 capture from air and storage. Because olivine, serpentine and brucite are far from CO2, H2, and O2 exchange equilibrium with air and surface water, peridotites are also an immense reservoir of chemical potential energy that could yield heat and work from weathering reactions. Nevertheless, compared to basalts, peridotites are less permeable, have less abundant outcrops, and are farther from population centers and sources of anthropogenic CO2.
As in any in situ carbon mineralization system, the extent of carbon mineralization could become limited through negative feedbacks, i.e., passivation of reactive surfaces and clogging of pore space causing a decrease in permeability. While basalt formations may be more vulnerable to passivation than peridotite, pore space clogging is less important in basalts because of their higher initial porosity and permeability (National Academies of Sciences Engineering Medicine, 2019). However, in peridotite formations, natural alkaline springs have persisted in the same locations for tens to hundreds of thousands of years without running out of reactive surface areas or destroying permeability (Kelley et al., 2001; Früh-Green et al., 2003; Ludwig et al., 2006, 2011; Kelemen and Matter, 2008; Kelemen et al., 2011, 2019). In some cases, every magnesium and calcium atom in peridotite has combined with CO2 to form listvenite (previous paragraph). This may be the result of positive feedback, in which reaction-driven volume changes cause increases in differential stress in the rock and generate fractures, maintaining or increasing the permeability of the rock and exposing fresh reactive surfaces (e.g., Jamtveit et al., 2008; Kelemen and Matter, 2008; Rudge et al., 2010; Kelemen and Hirth, 2012; Evans et al., 2018). Reaction-driven cracking is probably maximized when the rate of reaction is highest. During some experiments on carbon mineralization in peridotite, a reduction in permeability has been observed (e.g., Andreani et al., 2009; Hövelmann et al., 2012; Godard et al., 2013; van Noort et al., 2017), emphasizing the necessity to better understand of the parameters and mechanisms driving positive (cracking) or negative (clogging) feedbacks. The analog experiments of Zheng et al. (2018) illustrate the efficacy of reaction-driven cracking under the right conditions. In addition to their application to in situ carbon mineralization, a greater understanding of these mechanisms could be applied toward geothermal power generation, in-situ mining, extraction of oil and gas from tight reservoirs, and conversely, ensuring clogging to avoid failure of caprock and wellbore cement overlying CO2 storage reservoirs.
Geologic examples demonstrate that complete, in situ carbon mineralization of peridotite is possible at elevated temperature and relatively high partial pressures of CO2 (e.g., Falk and Kelemen, 2015; de Obeso et al., 2017). Thus, at about 185°C and more than ~70 bars PCO2, carbon mineralization may be implemented for solid storage of CO2 at a cost on the order of $10 per ton [(National Academies of Sciences Engineering Medicine, 2019), Figure 6.17]. Modeling has shown that injecting CO2 at depth in peridotite formations could increase the mineralization rate by 16,000× over natural systems, and the weight of CO2 sequestered per kilogram of peridotite could be 350× higher (Paukert et al., 2012). However, complete carbon mineralization is unlikely, and the cost of injecting fluid, per ton of CO2, becomes very high at temperatures lower than 50°C and/or CO2 partial pressure < ~1 bar.
As noted above, peridotite undergoing low temperature alteration and weathering produces C-depleted, Ca-rich alkaline waters, via precipitation of Mg-carbonates and dissolution of Ca-silicates along the subsurface reaction path. When these alkaline waters emerge at springs on the surface, they combine with atmospheric CO2 to form calcite (CaCO3) travertine deposits (e.g., Barnes and O'Neil, 1969; Bruni et al., 2002; Paukert et al., 2012). Thus, accelerated production of water from peridotite-hosted, alkaline aquifers represents a low-cost opportunity for CO2 removal from air coupled to solid storage. The size and recharge rate of such aquifers are unknown. Note that only very limited amounts of pumping could be employed. In near-surface peridotite catchments, natural water saturated in air contains ~40–400 ppm CO2 (calculated with all dissolved C as CO2, using C concentrations from Kelemen and Matter, 2008, Figure S2). Similarly, we calculate that alkaline water at the surface will consume about 400 ppm CO2 to form calcite (Vankeuren, personal communication). At these concentrations, every penny spent pumping a ton of water becomes $20–$200 per ton of CO2. Thus, to produce alkaline water, it is best to seek areas with artesian springs, relatively high subsurface permeability, and/or a significant thermal gradient promoting thermal convection of water in pore space. Recharge of alkaline, peridotite-hosted aquifers could be accelerated by recirculation of produced water into peridotite, with costs of $50–$100/tCO2 in formations with permeability ~10−12-10−13 m2 (1–0.1 Darcy), with each well having the capacity to capture and store a few thousand tCO2/yr (Kelemen et al., 2016; Kelemen et al., under review). Unfortunately, costs are strongly correlated with permeability, so that in low permeability formations (e.g., ≤10−14 m2), the costs would exceed $1,000/tCO2. On the other hand, costs are negatively correlated with the CO2 concentration in the circulated fluid. Initial investigations into relatively low-cost enrichment of CO2 from 0.04% in air to, e.g., 5% using direct air capture to increase the CO2 purity, followed by in situ carbon mineralization (Kelemen et al., under review) are ongoing.
In summary, near-surface peridotite formations have the capacity to store over 100,000 GtCO2 at relatively affordable costs that could be similar to CarbFix or injection in subsurface sedimentary reservoirs. Drilling to depths where temperature exceeds 100°C, and pumping of high PCO2 fluids, could yield efficient, permanent, solid storage of CO2 captured elsewhere. At lower temperature, production of alkaline water from peridotite-hosted aquifers could provide a route to CO2 removal from air, combined with solid storage that is cost-competitive with direct air capture using chemicals. A combination of peridotite carbonation and geothermal power generation could be a win-win situation, because the extraction and reinjection of geothermal fluid drives fluid flow through peridotite, necessary for extensive carbon mineralization, an exothermic reaction that could preserve the high temperature in the fluid reservoir, maintaining thermal buoyancy, and power generation.
Supercritical CO2 (density around 600 kg/m3) may be injected and stored in deep sedimentary formations. Ideal reservoirs for CO2 sequestration are thick reservoirs located below 1 km in depth with high porosity and high permeability ensuring a high storage capacity (i.e., 50–100 MtCO2 /project) at commercially meaningful injection rates (MtCO2/yr). Suitable reservoir rocks have high porosity and permeability such as sandstone, limestone, dolomite, basalt, or mixtures thereof, whereas a suitable caprock is made of shale, anhydrite, or low permeability carbonate rocks. Long-term CO2 sequestration and reservoir safety rely on the impermeability of the caprock and the extent of the secondary trapping mechanisms (section Storage in Subsurface Pore Space) (National Academies of Sciences Engineering Medicine, 2019).
Injections of CO2 into subsurface reservoirs began in the 1970s for enhanced oil recovery. Today CO2-EOR has the potential to sequester 30 GtCO2 if the CO2 used in oil recovery [assuming an industry standard of 3 bbl oil per tCO2 sequestered] is left in the ground after oil production (Kuuskraa, 2013; International Energy Agency, 2015). Advanced CO2-EOR methods, designed to co-optimize both oil recovery and CO2 sequestration, could sequester over 90 GtCO2 (National Academies of Sciences Engineering Medicine, 2019), and this could increase oil production compared to standard CO2-EOR methods (Benson and Deutch, 2018).
The Sleipner Saline Aquifer Storage Project is the first commercial-scale CO2 storage project. It commenced in 1996 and has injected CO2 into an offshore saline aquifer formation, comprised of permeable sandstone beneath a low permeability shale caprock for permanent disposal and climate change mitigation (Baklid et al., 1996). This project shows that CO2 storage in subsurface sedimentary reservoirs at a rate of 1 Mt/yr is possible and safe. In addition, the project helped increase our understanding of CO2 plume migration. The project led to the avoidance of a tax of roughly 320 NOK/tCO2 (36 USD at 9 NOK/USD) (Torp and Brown, 2005). Four commercial-scale projects globally are currently collectively sequestering 4.2 MtCO2/yr and a fifth pending project in Western Australia is aiming to sequester 3.4–4 MtCO2/yr. These projects store anthropogenic CO2 from natural gas processing, chemical production, and power generation (Table 1) (Global CCS Institute, 2019; National Academies of Sciences Engineering Medicine, 2019).
Table 1. Global CO2 sequestration projects for climate change mitigation (Rutqvist et al., 2010; Vasco et al., 2010; Eiken et al., 2011; Shi et al., 2013; McGrail et al., 2014; Gíslason et al., 2018; Marieni et al., 2018; Global CCS Institute, 2019; National Academies of Sciences Engineering Medicine, 2019).
Sedimentary basins around the world have the potential to store 8,200 GtCO2 in a conservative estimate and between 20,000 and 35,000 GtCO2 in high estimates (Benson et al., 2005, 2012; U.S. Geological Survey (USGS), 2013; de Coninck and Benson, 2014; U.S. Department of Energy (DOE), 2015; Global CCS Institute, 2019). A reasonable CO2 footprint for sequestration in sedimentary reservoirs lies between 0.5 and 5.0 tCO2/m2, and depends on the architecture of the reservoir and the caprock, the petrophysical properties of the rocks, the pressure and the temperature in the reservoir, and the extent of secondary trapping mechanisms (National Academies of Sciences Engineering Medicine, 2019).
True realization of a given capacity depends on a number of factors. More specifically, the uncertainties surrounding the pore space that is usable for CO2 storage include: the pressure buildup in the reservoir during injection; the quality of the seal, including the presence old boreholes that penetrate the seal; other uses of the formation; and other restrictions on above-ground or surface resources (Ehlig-Economides and Economides, 2010; Zoback and Gorelick, 2012; Bachu, 2015). The concept of dynamic capacity has been developed to address the issues surrounding pressure buildup and the maximum rate of CO2 injection. This parameter depends on other reservoir users and if active pressure management is implemented (e.g., brine removal) (Buscheck et al., 2012). Actual CO2 sequestration capacities could thus be in the lower range of assessed reservoir capacities (National Academies of Sciences Engineering Medicine, 2019). A more salient issue is the proximity of quality storage reservoirs to sources of CO2 emissions. While long-distance transport of CO2 is possible, it can add considerably to the cost of CCUS.
In general, even the conservative estimates for CO2 sequestration capacity far exceed the cumulative anthropogenic CO2 emissions since the Industrial Revolution (2,035 ± 205 GtCO2) (Le Quéré et al., 2015). Worldwide potential for sequestration of supercritical CO2 in sedimentary rock pore space is estimated to be between 8,200 GtCO2 and 34,700 GtCO2. Most of the possibilities are located in Asia (2,964–2,984 GtCO2), North America (2,600–21,770 GtCO2), and South America (~2,030 GtCO2) (Global CCS Institute, 2019). Given this geographic dispersion, highly concentrated CO2 sources and storage opportunities are often co-located, which minimizes CO2 transportation costs and associated emissions. If the strategy is to use the reservoirs with the highest capacities or the highest injection rates, CO2 may be provided by long distance transport or direct air capture depending on the relative costs of each (National Academies of Sciences Engineering Medicine, 2019).
Sequestration in sedimentary formations is the most mature technology for long-term storage of CO2 in subsurface pore space. The requirements for secure reservoirs are described in sections Storage in Subsurface Pore Space and Geological Sequestration in Saline Aquifers and Oil and Gas Depleted Reservoirs. Assurance of long-term storage security can be enhanced by secondary trapping mechanisms that immobilize the CO2, including dissolution, residual gas trapping, and mineralization. Prior to CO2 injection, targeted reservoirs are modeled using multiscale and multiphysics numerical simulations coupled to field observations in order to assess the ability of the reservoir to securely sequester CO2. During and after the injection it is necessary to monitor the evolution of CO2, the reservoir, and the caprock (Jenkins et al., 2015), in order to detect leakage from the reservoir. It is thus necessary to have suitable conditions for monitoring. In addition, underground sequestration of CO2 aims to avoid any competition with other vital resources, such as freshwater and land use (National Academies of Sciences Engineering Medicine, 2019).
Numerical modeling of the target reservoir helps to design and optimize CO2 injection and confirms the performance of the formation. The goal is to predict the evolution of the plume, its center and spread, as well as the CO2 phase distribution. Modeling outputs include CO2 plume migration, pressure buildup, geomechanical effects, and geochemical reactions (National Academies of Sciences Engineering Medicine, 2019). These phenomena are coupled and can be described by a set of nonlinear differential equations that model fluid thermodynamics and physical processes. Solving these set of equations requires using finite differences, finite volume, or finite element simulations (U.S. Department of Energy (DOE), 2017d). Mature codes using paralleled temporal and spatial discretization and related equations to model the fluid thermodynamics and physical processes provide similar results (Pruess et al., 2004; Class et al., 2009).
Accurate modeling of CO2 evolution in subsurface reservoirs faces several challenges: (1) the complexity of physical and chemical processes occurring, (2) spatial and temporal scales ranging over more than 12 orders of magnitude (from nm to km, and from ms to 1000s years, respectively), and (3) incomplete high-resolution knowledge of the subsurface geology that necessitates probabilistic modeling. Simultaneously addressing these challenges in a single simulation is too computationally intensive. Hence, modelers use reduced physics models (Nordbotten et al., 2012), upscaling, and empirical parametrization provided by lab experiments. However, in order to make the experiments feasible, experimentalists use small samples over short timescales, high flow rates, and other parameters that may not be representative of natural subsurface reservoirs like relative permeability. These parameters must be extrapolated in order to understand the natural reservoir dynamics. Modeling is carried out over specific time and space scales and relies on computationally-intensive simulations using advanced fluid mechanics in realistic pore networks (Raeini et al., 2018). Efforts are underway to properly bridge the gaps between the various scales (Abu-Al-Saud et al., 2017). Additional work is required for improved rate constant data of the various chemical processes (Zhang and DePaolo, 2017) and the parametrization of convective dissolution of CO2 in saline formations (Riaz et al., 2006). Additionally, a high-resolution description of the reservoir is only available at the wellbores. A description of the rest of the reservoir relies on indirect methods using geophysical imaging such as seismic surveys and probabilistic methods. The discrepancies on parameters and assumptions made from the various models result in differences in plume evolution and CO2 phases distribution, illustrating the need for improved modeling tools (Nordbotten et al., 2012).
In practice, subsurface reservoir modeling uses an iterative method that aims to match observations and simulations by refining and calibrating the numerical model with direct and indirect observations of the reservoir characteristics. This method is used today and is shown to work well for geological sequestration projects (National Academies of Sciences Engineering Medicine, 2019). A combination of rapid growth in computational power and algorithm evolution are promising for the emergence of more accurate forecasting of CO2 evolution in subsurface reservoirs, ultimately leading to the scale-up of geologic sequestration on the order of GtCO2/yr (National Academies of Sciences Engineering Medicine, 2019).
Major risks associated with the storage of CO2 in underground pore space include leakage from wellbores or non-sealed fractures in the caprock, pressure buildup in the reservoir that could result in caprock hydraulic fracturing, and contamination of drinking water. Prevention of these risks relies on the monitoring of CO2 plume migration, pressure in and above the reservoir, induced seismicity, extent of secondary trapping mechanisms, and the chemistry of freshwater aquifers close to the CO2 reservoir (National Academies of Sciences Engineering Medicine, 2019). Injection wells for CO2 sequestration are Class VI and in the U.S., guidelines exist that prescribe monitoring requirements throughout the lifecycle of a CO2 storage project (European Parliament the Council of the European Parliament, 2009; U.S. Environmental Protection Agency (EPA), 2010).
Pressure-variation monitoring is one of the best indicators of CO2 movement. Even if the horizontal displacement of the brine is small, the injection of CO2 can create a pressure buildup >100 km away from the injection zone (Birkholzer et al., 2009). Pressure measurements are performed with pressure sensors placed in injection and monitoring wells and in aquifers above the storage formation. Pressure buildup assists in tracking CO2 plume migration (Strandli and Benson, 2013; Strandli et al., 2014), but pressure changes in aquifers above the reservoir indicate leakage in the overlying aquifer (Meckel et al., 2013; Kim and Hosseini, 2014; Cameron et al., 2016). Interferometric synthetic aperture radar (InSAR) measurements of ground surface deformation have also proved to be efficient for estimation of large pressure buildup in the reservoir in regions with few seasonal variations (e.g., In Salah, Algeria) (Vasco et al., 2010). However, the most favored technique for CO2 plume monitoring is seismic imaging (Hovorka et al., 2006; Pevzner et al., 2011; Ivanova et al., 2012; Ajo-Franklin et al., 2013; White, 2013; Jenkins et al., 2015). Tracking the CO2 plume can be used to calibrate and validate reservoir models, detect leakage of CO2 into overlying aquifers, and indicate the “CO2 footprint” of the plume. It could also provide information about the interactions between several injection sites in the same formation (U.S. Environmental Protection Agency (EPA), 2013; National Academies of Sciences Engineering Medicine, 2019).
Injection of CO2 in subsurface reservoirs may create induced seismicity, likewise for injection of oilfield brine disposal or hydraulic fracturing for enhanced oil and gas recovery (Ellsworth, 2013; Walsh and Zoback, 2015; Langenbruch and Zoback, 2016). Induced earthquakes are mostly of magnitude 2 or below and are not felt at the surface and require sensitive geophone arrays to be recorded, identified, and located. Still, microseismicity monitoring at Decatur (USA) and In Salah (Algeria) sequestration sites have shown thousands of induced earthquakes per year (U.S. Department of Energy (DOE), 2017c). Most studies consider that induced earthquakes have a low risk of triggering fault displacements and endangering the security of the reservoir, but some authors have argued that even small- to moderate-magnitude earthquakes could damage the seal and compromise the integrity of sequestration reservoirs (Zoback and Gorelick, 2012). Experience to date has shown that the vast majority of micro-seismic events are not in the seal, instead, occurring in crystalline basement rocks far below the storage reservoir which may be due to a pre-existing geological structure—posing no risks to the storage integrity of the reservoir (Kaven et al., 2014).
The monitoring of CO2 reservoirs also includes checking for CO2 leaks at the surface or dissolved in groundwater. At the surface, CO2 is commonly detected using infrared detectors or techniques developed for studying the carbon cycle in the terrestrial environment (Fessenden et al., 2010; Krevor et al., 2010; Lewicki et al., 2010; Male et al., 2010; Rouse et al., 2010). In groundwater, the presence or an increase of CO2 can be deduced from reaction products (Hovorka et al., 2006; Jenkins et al., 2012; Romanak et al., 2012; Yang et al., 2013; Anderson et al., 2017), from analyses of pressurized samples representative of the subsurface conditions (Freifeld et al., 2005), and from tracers that help track waters containing dissolved CO2 (Kharaka et al., 2009; Ringrose et al., 2009; Würdemann et al., 2010; Matter et al., 2011). Probabilistic modeling of risks associated with leakage suggest that 98% of the CO2 will be retained in 10,000 years (Choi et al., 2013; Alcalde et al., 2018; Rogelj et al., 2018). An increase in CO2 in aquifers may mobilize lead and arsenic contained in rocks (Zheng et al., 2009) creating an environmental hazard if drinking water sources are affected. However, numerical simulations have shown that the migration of fluids between reservoirs is unlikely through low-permeability sealing units (Birkholzer et al., 2009).
In order to meet the 2°C target of the Paris agreement, 125 GtCO2 has to be removed from air and permanently stored by 2100 (National Academies of Sciences Engineering Medicine, 2019). The cheapest storage methods with large capacity cost roughly $7–$30 per ton of CO2 sequestered (storage costs only) and range from the injection of supercritical CO2 into subsurface sedimentary formations to in-situ carbon mineralization via injection of CO2-enriched fluids into on-land basalt and on-land peridotite formations (Figure 4). Storage of a total of 125 Gt CO2 would result in a capital input of approximately 1–4 trillion dollars in total and 10–50 billion dollars per year until 2100. For comparison, the total cost of the energy transition, i.e., decarbonizing energy through shifting to an energy infrastructure comprised primarily of renewables, required for <1.5°C of warming was estimated to be 1.6–3.8 trillion dollars per year until 2050 (McCollum et al., 2018; Rogelj et al., 2018).
Figure 4. Summary of the cost of CO2 stored (US$/tCO2) vs. storage potential of CO2 per year (GtCO2/yr). Red boxes illustrate costs and rates for ex-situ CO2 mineralization using heat and concentrated CO2. Yellow boxes are for surficial CO2 mineralization of mine tailings, of ground peridotite added to soils or beaches, and of peridotite mined and ground for the purpose of CO2 removal from air with solid storage. Green arrows are for in-situ carbon storage by injection of CO2-enriched fluids into mafic and ultramafic formations (e.g., CarbFix). Blue arrows are for in-situ carbon sequestration by circulating water saturated in air into peridotite formations, for CO2 removal from air with solid storage. Gray arrow is for in-situ carbon sequestration by injecting supercritical CO2 into subsurface sedimentary formations. Figure modified from (National Academies of Sciences Engineering Medicine, 2019), Figure 6.19, with data from references therein (Tables 6.1 and 6.2). Details on the capacity of industrial and municipal wastes are provided in Table 2.
Recently the IPCC published a report stating that, to reach the 1.5°C goal, negative emissions technologies must be implemented at the scale of tens of Gt by mid- century (IPCC Special Report, 2018). In other words, in addition to reducing emissions and capturing CO2 from point sources, CO2 removal from air (CDR) is required. The options for CDR include an increase of carbon storage in soils and biomass, but also Direct Air Capture using Synthetic Sorbents (DACSS) and carbon mineralization via enhanced weathering (DACEW). It is likely that all of these options, operating in parallel, will be necessary to achieve the required level of global CDR (United Nations Environment Programme (UNEP), 2017; National Academies of Sciences Engineering Medicine, 2019). Figure 4 summarizes the estimated cost vs. the annual capacity for several in-situ, surficial, and ex-situ techniques of CO2 storage, and for a smaller number of techniques combining CO2 removal from air with solid storage.
With regard to storage, the least expensive options with the greatest capacity involve injection of CO2 into subsurface pore space in sedimentary rock, basalt, or peridotite formations on land. Sequestration in on-land basalt is competitive with storage in sedimentary reservoirs, but costs rise for storage in seafloor basalts. Various low-cost methods (e.g., sparging of concentrated CO2, bioleaching, stirring, etc.) have been developed for CO2 storage in mine tailings at a cost <$30/tCO2. In Figure 4, unless otherwise specified, costs for sedimentary reservoirs, on-land and seafloor basalt, and on-land peridotite include only the costs for storage. The total cost of capture and storage of CO2 would include all costs incurred by the CO2 capture method used (e.g., DACSS, DACEW, point-source, etc.), compression, and transport (if appropriate) to the sequestration site.
One of the lower cost options proposed for combined CO2 removal from air and storage involves in situ circulation of water in equilibrium with air through peridotite, either in tailings or in the subsurface. Such methods may be optimized where they are co-located with geothermal power production. This technique does not require any CO2 capture equipment, though recirculation of water is required to maximize uptake, since the CO2 in air-saturated water is very dilute. With or without co-generation of geothermal power, configurations in which fluid circulation is driven by thermal convection or gravity could be competitive with DACSS coupled with storage in sedimentary reservoirs. However, where configurations require pumping water into low-permeability formations, costs rise to over $1,000/tCO2.
Another option for combined CO2 removal from air and solid storage is carbon mineralization in ultramafic mine tailings and alkaline industrial wastes, which spontaneously react with CO2 from air to form carbonate minerals during surficial weathering. In the case of in situ carbon mineralization in peridotite, mining and crushing peridotite for the purpose of CO2 removal from air may be cost competitive with DACSS. This is particularly true if tailings are weathered, calcined, and weathered again. In this case, CO2 produced during the calcining step could be stored, or sold for utilization. The storage potential of ultramafic tailings (about 0.2 GtCO2/yr) and industrial and municipal wastes (0.1–1.3 GtCO2/yr) depend on rates of mining and waste production, and are small compared to geological reservoirs. However, it may be that rocks can be mined and ground for the purpose of DACEW, again with costs and area requirements similar to, or less than, optimistic future cost estimates for DACSS. Moreover, surficial carbon mineralization can have other advantages: it can help industries lower their carbon footprint, and it may mitigate health and environmental hazards (e.g., from asbestos). Work has been done to create commodities from industrial waste (e.g., fabrication of CH4 or more complex hydrocarbons, and so on) with nearly-net-zero emissions. A few such applications (e.g., adding CO2 to aggregate for concrete) could provide CO2 storage as well as value-added products (Shao, 2014; Monkman et al., 2018).
The total capacities of reservoirs proposed for in-situ CO2 sequestration are 8.0·103-3.5·104 GtCO2 for sedimentary formations, 1.0·103-2.5·105 GtCO2 for on-land basalts, 8.0·102-1.0·105 GtCO2 for submarine basalts, 6.0·104-6.0·105 GtCO2 for on-land peridotite aquifers (National Academies of Sciences Engineering Medicine, 2019). There is perhaps another 6.0·107 GtCO2 of capacity in seafloor fractured peridotite aquifers. Thus, storage capacities are not a limitation for CO2 mitigation, given that current emissions are about 40 GtCO2/yr (Le Quéré et al., 2015; IPCC Special Report, 2018). An important limitation for CO2 sequestration in subsurface pore space is the maximum injectivity achievable in a given reservoir, which is 0.001–60 MtCO2/yr in the U.S. in sedimentary reservoirs (Baik et al., 2018). Such rates are compatible with the climate goal requirements of storing 125 GtCO2 by 2100 (National Academies of Sciences Engineering Medicine, 2019).
Ex-situ carbon mineralization is one of the most expensive techniques reviewed here, with costs generally estimated at hundreds of dollars per ton (Figure 4 and Table 2) depending on the reactant and the carbon mineralization technique. It is generally more expensive to carbonate serpentine-rich and olivine-rich tailings ex-situ than to carbonate basalt and peridotite in-situ, due to the price of heating and transportation of the tailings. Still, less costly opportunities may be found where a source of concentrated CO2 and a source of waste heat are close to a mine of mafic or ultramafic rock, or an industry producing waste byproducts with high alkalinity.
Table 2. Details of industrial and municipal wastes regarding the quantities produced per year, the potential CO2 uptake per year, and the cost of the method (National Academies of Sciences Engineering Medicine, 2019).
A large variety of sequestration options are available globally (Figure 5). The most effective for climate change mitigation is sequestration in geological formations, but locally—and where no geological formation is close to a source of concentrated CO2–other solutions such as surficial or ex-situ carbon mineralization may be cheaper or present other advantages. Large geological formations far from anthropogenic CO2 sources could be used to store CO2 concentrated by direct air capture, or via enhanced weathering processes that combine CO2 removal from air with solid storage.
Figure 5. Map of CO2 sequestration facilities, pilot projects, and long-term storage potential in geologic formations (Kelemen, 1998; Bradshaw and Dance, 2005; Oelkers et al., 2008; Krevor et al., 2009; Global CCS Institute, 2019; National Academies of Sciences Engineering Medicine, 2019).
Ex-situ and surficial carbon mineralization are currently under development, and surficial carbon mineralization is ready for kt to Mt CO2 field experiments. Research gaps remain in the area of carbon mineralization process improvement. One of the top priorities is to fill the gaps between datasets in order to compare different materials (rocks and industrial byproducts) at the same temperature, pressure, p(CO2), grain size, surface area, and fluid composition using the same units for comparison. Currently, research on ex-situ carbon mineralization focuses on the creation of usable products to offset high costs of this method relative to surficial and in situ carbon mineralization (for CDR and storage), and storage of supercritical CO2 in subsurface pore space.
Surficial carbon mineralization methods would benefit from the optimization of heat-pretreatment, grinding processes, mining, and the development of new techniques (e.g., microwave treatment and/or microbial acceleration). Current production of mine tailings (and alkaline industrial wastes) limits the capacity of these methods, but mining of peridotite at the Gt/year scale for the purpose of CO2 removal from air via carbon mineralization combined with solid storage (DACCM), may be cost competitive with the use of direct air capture with synthetic sorbents (DACSS) coupled to storage in subsurface pore space, particularly if the tailings are calcined and recycled. Some mine tailings sites are close to surface water and groundwater resources, requiring evaluation of the potential effect of geochemical contamination. More research is also needed to evaluate the impact of carbon mineralization on terrestrial, coastal, and marine environments. Current efforts to reduce the hazards of asbestos could be combined with carbon mineralization in tailings.
The CarbFix and Wallula pilot-scale CO2 storage projects have shown that in-situ carbon mineralization for storage in basalt formations is feasible and safe, and that an impermeable caprock is not always necessary when CO2 is dissolved in pore water at depth. Additional sites should be developed, ideally with high geothermal gradients to drive fluid flow and rapid reaction, readily available water to co-inject with CO2, and high proportions of basaltic glass for accelerated carbon mineralization. Research is needed on potential contamination of nearby aquifers, and on the time evolution of the carbonate-forming reaction front, to get feedback about reaction rates, the three-dimensional distribution of reaction products, and permeability evolution. Medium-size pilot projects to address these questions are estimated to cost tens of millions of dollars per year for several years (National Academies of Sciences Engineering Medicine, 2019).
In-situ carbon mineralization in peridotite formations should be implemented on a pilot-scale, with particular emphasis on the maintenance or enhancement of permeability, perhaps via reaction-driven cracking as described in section Carbon Mineralization in Peridotites. It is essential to understand the chemicophysical parameters that lead to cracking vs. clogging, which can then be applied to favor cracking in reservoirs and clogging in caprocks. Currently, the size, injectivity, permeability, geomechanics, and microstructure of peridotite reservoirs are relatively unknown. Understanding nano-scale rock behavior is essential to understanding the macro-evolution of the reservoir, and more research is needed in this field. In order to assess the possibility of CO2 storage in peridotite formations, two to three small-to medium-scale pilot projects are needed. This will lead to a greater understanding of the behavior of hierarchical fracture networks, which are difficult to simulate in laboratories. These pilot projects would cost about $10M−20M/yr based on Phase I of the CarbFix project and would test the efficiency of CO2 mineralization when circulating surface water or CO2-enriched water into peridotite (National Academies of Sciences Engineering Medicine, 2019).
Sequestration of CO2 in sedimentary formations already stores about 4.2 MtCO2/year. Current challenges are associated with upscaling more than 100 times in order to reach the GtCO2/year scale and beyond. This requires field experimentation and testing, and sequestration of CO2 in regions lacking major emission sources. Currently, it would cost $5M per well for supercritical CO2 injection in sedimentary formations. Major research concerns are related to site selection, reservoir performance (e.g., increasing the speed of secondary trapping mechanisms, co-optimizing CO2-EOR and sequestration, improving models), improving monitoring and reducing its costs, assessing risks (e.g., induced seismicity, groundwater contamination) and managing risks in compromised sequestration systems, as well as improving public confidence in secondary trapping mechanisms. Development of negative emission technologies also relies on the engagement of the community and on the favorable opinion of the general public that should be informed about the needs, opportunities, risks, and benefits of CO2 sequestration in geologic formations.
Regulation already exists in the U.S. regarding fluid injection in subsurface pore space, due to the routine injection of wastewater into deep saline aquifers. Best practice manuals have been developed by the Department of Energy (DOE) for safe injection and sequestration of CO2 in sedimentary formations (U.S. Department of Energy (DOE), 2010a,b, 2017a,b,c,d,e). Still, some problems remain to be resolved, e.g., the financial responsibility for long-term liability, pore space ownership, and regulatory impediments. Indeed, CO2 injected into pore space in depleted oil reservoirs and deep saline aquifers can remain mobile during hundreds of years. Even if secondary mechanisms increase reservoir safety over time, regulations are needed to determine who is responsible for monitoring and remediation after injection projects shut down. Sedimentary units extend over tens or hundreds of km2, and thus are shared by hundreds of owners, potentially restricting the scope of CO2 sequestration projects. These two issues are easier to solve in countries where underground resources are owned by the government, which can act as a deciding body for project realization and be responsible for long-term management. Currently, the requirements for monitoring are significant and costly for operators. More flexibility adapted to each situation would still ensure safety and reduce the costs, making more projects affordable thereby facilitating the required deployment at the gigaton scale.
In the U.S., most research on CO2 subsurface injection and carbon mineralization at the laboratory- and pilot-scale would be financed mainly by the DOE and the National Science Foundation (NSF), with support from the Environment Protection Agency (EPA), the US Geological Survey (USGS) and the Bureau of Land Management (BLM). The NSF would fund more fundamental research in hydrology, geochemistry, geophysics, biochemistry, and social sciences, whereas the DOE usually funds applied research on topics such as site characterization, modeling, optimization of trapping mechanisms, co-optimized carbon capture and oil production, monitoring, induced seismicity, ex-situ and surficial carbon mineralization, mitigation of environmental hazards, and pilot-plant projects in basalt and ultramafic formations. The EPA oversees regulatory aspects regarding site selection, operation, and monitoring, and aims to assess, minimize, and monitor risks of ground water contamination and mitigate environmental hazards. The USGS is in charge of mapping the geology of the U.S. and has already assessed sites suitable for CO2 sequestration in sedimentary reservoirs and could help with site selection in mafic and ultramafic formations. Scaling up CO2 sequestration in sedimentary reservoirs to GtCO2/yr, would require using federal lands, and BLM could help investigate possibilities for CO2 sequestration in these locations. The 10-year research agenda established in the 2019 National Academies of Sciences report calls for $250/yr to realize the MtCO2 to GtCO2 upscaling for sedimentary reservoirs and for $700M to expand pilot-scale studies in mafic and ultramafic formations (National Academies of Sciences Engineering Medicine, 2019).
Successful and rapid development of CO2 sequestration and mineralization projects depends on data sharing, community coordination and funding. Research topics being shared between several institutions and partnerships would facilitate research and development. Partnerships are also essential between universities or government agencies and industry. Such partnerships already exist for some projects on carbon mineralization in mine tailings, and for the CarbFix project. Oil and gas industries have expertise in drilling, injection of fluids, and monitoring reservoir evolution. The oil industry currently injects megatons of CO2 per year for CO2-EOR, and their input in CO2 sequestration projects would be valued, even if the quantity required to be injected for CO2 sequestration is much larger. Some academic and government labs have expertise in laboratory analyses, computational modeling, monitoring, and practical experience with sequestration, that could result in a three-way transfer of knowledge. The federal tax credit 45Q is designed to support CO2 injection in subsurface pore space. The credit increases progressively to $35/tCO2 in the case of EOR and up to $50/tCO2 for storage in deep saline aquifers.
Sequestration of CO2 in the amount needed for climate change mitigation requires scaling CO2 sequestration to the industrial scale. Public acceptance is essential. Thus, it is important to involve local communities and the general public in project realization. Education, and the demonstration of safe storage of CO2 with successful pilot projects, would help in gaining public support.
Sequestration of CO2 has to increase to the GtCO2/yr scale to play a significant role in mitigation of climate change. Many options have to be considered to ensure fast development of some or all of the methods described in this paper. The research agenda outlined here, and described in more detail in National Academies of Sciences Engineering Medicine (2019), includes priorities ranging from fundamental research on kinetics, rock mechanics, numerical simulation, and database creation to small and medium-scale pilot projects for surficial and in situ carbon mineralization to assess the efficiency of reservoirs and to reduce associated risks. Supercritical CO2 injection is the most mature storage method. Research is needed to improve actual techniques and reduce risks concomitant with injection and leakage. The 10-year research agenda requires $250M to lead this field from the current, MtCO2/yr scale to the GtCO2/yr scale required for climate change mitigation. Carbon mineralization methods are at an earlier stage of development but include a set of reactants and techniques that are promising in terms of CO2 uptake and safety of CO2 storage. The research agenda aims to expand pilot-scale studies, with a total of $700 M needed to reach this goal. These approaches complement each other as carbon mineralization is the ultimate step for permanent CO2 storage, while injection of CO2 into sedimentary reservoirs at the Gt/year scale leads the way in terms of technique, regulation, and public acceptance.
All authors listed have made a substantial, direct and intellectual contribution to the work, and approved it for publication.
PK's effort on this paper and the underlying research were supported in part by National Science Foundation Research Grants EAR-1520732 and EAR-1516300, Sloan Foundation Grant 2014-3-01, and the International Continental Scientific Drilling Program (ICDP) Oman Drilling Project. JW and HP acknowledge ClimateWorks Foundation for partial support for their efforts on the project.
The authors declare that the research was conducted in the absence of any commercial or financial relationships that could be construed as a potential conflict of interest.
We also acknowledge the committee and staff from National Academies of Sciences, Engineering, and Medicine report, Negative Emissions Technologies and Reliable Sequestration: A Research Agenda, which served as a key reference for this overview study.
Abu-Al-Saud, M. O., Riaz, A., and Tchelepi, H. A. (2017). Multiscale level-set method for accurate modeling of immiscible two-phase flow with deposited thin films on solid surfaces. J. Comput. Phys. 333, 297–320. doi: 10.1016/j.jcp.2016.12.038
Ajo-Franklin, J. B., Peterson, J., Doetsch, J., and Daley, T. M. (2013). High-resolution characterization of a CO2 plume using crosswell seismic tomography: Cranfield, MS, USA. Int. J. Greenhouse Gas Control 18, 497–509. doi: 10.1016/j.ijggc.2012.12.018
Alcalde, J., Flude, S., Wilkinson, M., Johnson, G., Edlmann, K., Bond, C. E., et al. (2018). Estimating geological CO2 storage security to deliver on climate mitigation. Nat. Commun. 9, 1–13. doi: 10.1038/s41467-018-04423-1
Alfredsson, H. A., Oelkers, E. H., Hardarsson, B. S., Franzson, H., Gunnlaugsson, E., and Gislason, S. R. (2013). The geology and water chemistry of the Hellisheidi, SW-Iceland carbon storage site. Int. J. Greenhouse Gas Control 12, 399–418. doi: 10.1016/j.ijggc.2012.11.019
Anderson, J. S., Romanak, K. D., Yang, C., Lu, J., Hovorka, S. D., and Young, M. H. (2017). Gas source attribution techniques for assessing leakage at geologic CO2 storage sites: Evaluating a CO2 and CH4 soil gas anomaly at the Cranfield CO2-EOR site. Chem. Geol. 454, 93–104. doi: 10.1016/j.chemgeo.2017.02.024
Andreani, M, Luquot, L, Gouze, P, Godard, M, Hoisé, E, and Gibert, B. (2009). Experimental study of carbon sequestration reactions controlled by the percolation of CO2-rich brine through peridotites. Environ. Sci. Technol. 43, 1226–1231. doi: 10.1021/es8018429
Aradóttir, E. S. P., Sigurdardóttir, H., Sigfússon, B., and Gunnlaugsson, E. (2011). CarbFix: a CCS pilot project imitating and accelerating natural CO2 sequestration. Greenhouse Gases Sci. Technol. 1, 105–118. doi: 10.1002/ghg.18
Assima, G. P., Larachi, F., Beaudoin, G., and Molson, J. (2013). Dynamics of carbon dioxide uptake in chrysotile mining residues - effect of mineralogy and liquid saturation. Int. J. Greenhouse Gas Control 12, 124–135. doi: 10.1016/j.ijggc.2012.10.001
Assima, G. P., Larachi, F., Molson, J., and Beaudoin, G. (2014). New tools for stimulating dissolution and carbonation of ultramafic mining residues. Canadian J. Chem. Eng. 92, 2029–2038. doi: 10.1002/cjce.22066
Bachu, S. (2015). Review of CO2 storage efficiency in deep saline aquifers. Int. J. Greenhouse Gas Control 40, 188–202. doi: 10.1016/j.ijggc.2015.01.007
Baik, E., Sanchez, D. L., Turner, P. A., Mach, K. J., Field, C. B., and Benson, S. M. (2018). Geospatial analysis of near-term potential for carbon-negative bioenergy in the United States. Proc. Natl. Acad. Sci. U.S.A. 115, 3290–3295. doi: 10.1073/pnas.1720338115
Baklid, A., Korbøl, R., STATOIL, Owren, G., and SINTEF (1996). “Sleipner vest CO2 disposal, CO2 injection into a shallow underground aquifer,” in SPE Annual Technical Conference and Exhibition (Denver, CO: Society of Petroleum Engineers), 269–277.
Balucan, R. D., and Dlugogorski, B. Z. (2013). Thermal activation of antigorite for mineralization of CO2. Environ. Sci. Technol. 47, 182–190. doi: 10.1021/es303566z
Balucan, R. D., Kennedy, E. M., Mackie, J. F., and Dlugogorski, B. Z. (2011). Optimization of antigorite heat pre-treatment via kinetic modeling of the dehydroxylation reaction for CO2 mineralization. Greenhouse Gases Sci. Technol. 1, 294–304. doi: 10.1002/ghg.33
Barnes, I., and O'Neil, J. R. (1969). The relationship between fluids in some fresh alpine-type ultramafics and possible modern serpentinization, Western United States. Geol. Soc. Am. Bull. 80, 1947–1960. doi: 10.1130/0016-7606(1969)801947:TRBFIS2.0.CO;2
Beerling, D. J., Leake, J. R., Long, S. P., Scholes, J. D., Ton, J., Nelson, P. N., et al. (2018). Farming with crops and rocks to address global climate, food and soil security. Nature Plants 4, 138–147. doi: 10.1038/s41477-018-0108-y
Benson, S. M., Bennaceur, K., Cook, P., Davison, J., de Coninck, H., Farhat, K.. (eds.). (2012). “Chapter 13: carbon capture and storage,” in Global Energy Assessment - Toward a Sustainable Future (Cambridge; New York, NY; Laxenburg: Cambridge University Press; The International Institute for Applied Systems Analysis), 993–1068.
Benson, S. M., Cook, P. J., Anderson, J. S., Bachu, S., Nimir, H. B., Basu, B., et al. (2005). “Underground geological storage,” in IPCC Special Report on Carbon Dioxide Capture and Storage, eds B. Metz, O. Davidson, H. de Coninck, M. Loos, and L. Meyer (Cambridge: Cambridge University Press, 179–194.
Benson, S. M., and Deutch, J. (2018). Advancing enhanced oil recovery as a sequestration asset. Joule 2, 1386–1389. doi: 10.1016/j.joule.2018.07.026
Birkholzer, J. T., Zhou, Q., and Tsang, C. F. (2009). Large-scale impact of CO2 storage in deep saline aquifers: a sensitivity study on pressure response in stratified systems. Int. J. Greenhouse Gas Control 3, 181–194. doi: 10.1016/j.ijggc.2008.08.002
Bradshaw, J., and Dance, T. (2005). Mapping geological storage prospectivity of CO2 for the world's sedimentary basins and regional source to sink matching. Greenhouse Gas Control Technol. 7, 583–591. doi: 10.1016/B978-008044704-9/50059-8
Bruni, J., Canepa, M., Chiodini, G., Cioni, R., Cipolli, F., Longinelli, A., et al. (2002). Irreversible water-rock mass transfer accompanying the generation of the neutral, Mg-HCO3 and high-pH, Ca-OC spring waters of the Genova province, Italy. Appl. Geochem. 17, 455–474. doi: 10.1016/S0883-2927(01)00113-5
Buscheck, T. A., Sun, Y., Chen, M., Hao, Y., Wolery, T. J., Bourcier, W. L., et al. (2012). Active CO2 reservoir management for carbon storage: analysis of operational strategies to relieve pressure buildup and improve injectivity. Int. J. Greenhouse Gas Control 6, 230–245. doi: 10.1016/j.ijggc.2011.11.007
Cameron, D. A., Durlofsky, L. J., and Benson, S. M. (2016). Use of above-zone pressure data to locate and quantify leaks during carbon storage operations. Int. J. Greenhouse Gas Control 52, 32–43. doi: 10.1016/j.ijggc.2016.06.014
Canovas, P. A., Hoehler, T., and Shock, E. L. (2017). Geochemical bioenergetics during low-temperature serpentinization: An example from the Samail ophiolite, Sultanate of Oman. J. Geophys. Res. Biogeosci. 122, 1821–1847. doi: 10.1002/2017JG003825
Chizmeshya, A. V. G., McKelvy, M. J., Squires, K., Carpenter, R. W., and Béarat, H. (2007). A Novel Approach to Mineral Carbonation: Enhancing Carbonaton While Avoiding Pretreatment Process Cost. Tempe, AZ.
Choi, Y. S., Young, D., Nešić, S., and Gray, L. G. S. (2013). Wellbore integrity and corrosion of carbon steel in CO2 geologic storage environments: a literature review. Int. J. Greenhouse Gas Control 16S, S70–77. doi: 10.1016/j.ijggc.2012.12.028
Class, H., Ebigbo, A., Helmig, R., Dahle, H. K., Nordbotten, J. M., Celia, M. A., et al. (2009). A benchmark study on problems related to CO2 storage in geologic formations. Comput. Geosci. 13, 409–434. doi: 10.1007/s10596-009-9146-x
de Coninck, H., and Benson, S. M. (2014). Carbon dioxide capture and storage: issues and prospects. Annu. Rev. Environ. Resour. 39, 243–270. doi: 10.1146/annurev-environ-032112-095222
de Obeso, J. C., Kelemen, P. B., Manning, C. E., Michibayashi, K., and Harris, M. (2017). “Listvenite formation from peridotite: insights from oman drilling project hole BT1B and preliminary reaction path model approach,” in Fall Meeting Abstracts (New Orleans, LA: American Geophysical Union).
DiPietro, P., Balash, P., and Wallace, M. (2012). A note on sources of CO2 supply for enhanced-oil-recovery opperations. SPE Econ. Manag. 4, 69–74. Available online at: https://energywv.org/assets/files/Energy-Summit-Presentations/2012/Carbon-Dioxide-Enhanced-Oil-Recovery-DiPietro.pdf
Dlugogorski, B. Z., and Balucan, R. D. (2014). Dehydroxylation of serpentine minerals: implications for mineral carbonation. Renew. Sustain. Energy Rev. 31, 353–367. doi: 10.1016/j.rser.2013.11.002
Edwards, D. P., Lim, F., James, R. H., Pearce, C. R., Scholes, J., Freckleton, R. P., et al. (2017). Climate change mitigation: potential benefits and pitfalls of enhanced rock weathering in tropical agriculture. Biol. Lett. 13, 1–7. doi: 10.1098/rsbl.2016.0715
Ehlig-Economides, C., and Economides, M. J. (2010). Sequestering carbon dioxide in a closed underground volume. J. Petrol. Sci. Eng. 70, 123–130. doi: 10.1016/j.petrol.2009.11.002
Eiken, O., Ringrose, P., Hermanrud, C., Nazarian, B., Torp, T. A., and Høier, L. (2011). Lessons learned from 14 years of CCS operations: sleipner, In Salah and Snøhvit. Energy Procedia 4, 5541–5548. doi: 10.1016/j.egypro.2011.02.541
Ellsworth, W. L. (2013). Injection-induced earthquakes. Science 341, 1–7. doi: 10.1126/science.1225942
Emami-Meybodi, H., Hassanzadeh, H., Green, C. P., and Ennis-King, J. (2015). Convective dissolution of CO2 in saline aquifers: progress in modeling and experiments. Int. J. Greenhouse Gas Control 40, 238–266. doi: 10.1016/j.ijggc.2015.04.003
European Parliament and the Council of the European Parliament (2009). Directive 2009/31/EC of the European Parliament and of the Council of 23 April 2009 on the Geological Storage of Carbon Dioxide and Amending Council Directive 85/337/EEC, European Parliament and Council Directives 2000/60/EC, 2001/80/EC, 2004/35/EC, 2006/12/EC, 2008/1/EC and Regulation (EC) No 1013/2006. Off. J. Eur. Union L140/114 52, 115–137. doi: 10.3000/17252555.L_2009.140.eng
Evans, O., Spiegelman, M. W., and Kelemen, P. B. (2018). Reactive-brittle dynamics in peridotite alteration. J. Geophys. Res. 123, 8653–8675. doi: 10.1029/2017JB015214
Falk, E. S., and Kelemen, P. B. (2015). Geochemistry and petrology of listvenite in the Samail ophiolite, Sultanate of Oman: complete carbonation of peridotite during ophiolite emplacement. Geochim. Geochim. Cosmochim. Acta 160, 70–90. doi: 10.1016/j.gca.2015.03.014
Fedoročková, A., Hreus, M., Raschman, P., and Sučik, G. (2012). Dissolution of magnesium from calcined serpentinite in hydrochloric acid. Miner. Eng. 32, 1–4. doi: 10.1016/j.mineng.2012.03.006
Fessenden, J. E., Clegg, S. M., Rahn, T. A., Humphries, S. D., and Baldridge, W. S. (2010). Novel MVA tools to track CO2 seepage, tested at the ZERT controlled release site in Bozeman, MT. Environ. Earth Sci. 60, 325–334. doi: 10.1007/s12665-010-0489-3
Flaathen, T. K., Gislason, S. R., and Oelkers, E. H. (2010). The effect of aqueous sulphate on basaltic glass dissolution rates. Chem. Geol. 277, 345–354. doi: 10.1016/j.chemgeo.2010.08.018
Freifeld, B. M., Trautz, R. C., Kharaka, Y. K, Phelps, T. J., Myer, L. R., Hovorka, S., et al. (2005). The U-tube: A novel system for acquiring borehole fluid samples from a deep geologic CO2 sequestration experiment. J. Geophys. Res. Solid Earth 110, 1–10. doi: 10.1029/2005JB003735
Früh-Green, G. L., Kelley, D. S., Bernasconi, S. M., Karson, J. A., Ludwig, K. A., Butterfield, D. A., et al. (2003). 30,000 years of hydrothermal activity at the lost city vent field. Science 301, 495–498. doi: 10.1126/science.1085582
Gadikota, G., Fricker, K., Jang, S.-H., and Park, A.-H. A. (2015). “Carbonation of silicate minerals and industrial wastes and their potential use as sustainable construction materials,” in Advances in CO2 Capture, Sequestration, and Conversion, eds F. Jin, L.-N. He, and Y. H. Hu (Washington, DC: American Chemical Society), 295–322.
Gadikota, G., Matter, J., Kelemen, P., and Park, A. H. A. (2014). Chemical and morphological changes during olivine carbonation for CO2 storage in the presence of NaCl and NaHCO3. Phys. Chem. Chem. Phys. 16, 4679–4693. doi: 10.1039/c3cp54903h
Gadikota, G., and Park, A.-H. A. (2015). “Chapter 8 - accelerated carbonation of Ca- and Mg-bearing minerals and industrial wastes using CO2,” in Carbon Dioxide Utilisation, eds P. Styring, E. A. Quadrelli, and K. Armstrong (Amsterdam: Elsevier)
Ghoorah, M., Dlugogorski, B. Z., Oskierski, H. C., and Kennedy, E. M. (2014). Study of thermally conditioned and weak acid-treated serpentinites for mineralisation of carbon dioxide. Miner. Eng. 59, 17–30. doi: 10.1016/j.mineng.2014.02.005
Gislason, S. R., and Oelkers, E. H. (2003). Mechanism, rates, and consequences of basaltic glass dissolution: II. An experimental study of the dissolution rates of basaltic glass as a function of pH and temperature. Geochim. Cosmochim. Acta 67, 3817–3832. doi: 10.1016/S0016-7037(03)00176-5
Gíslason, S. R., Sigurdardóttir, H., Aradóttir, E. S., and Oelkers, E. H. (2018). A brief history of CarbFix: challenges and victories of the project's pilot phase. Energy Procedia 146, 103–114. doi: 10.1016/j.egypro.2018.07.014
Gislason, S. R., Wolff-Boenisch, D., Stefansson, A., Oelkers, E. H., Gunnlaugsson, E., Sigurdardottir, H., et al. (2010). Mineral sequestration of carbon dioxide in basalt: a pre-injection overview of the CarbFix project. Int. J. Greenhouse Gas Control 4, 537–545. doi: 10.1016/j.ijggc.2009.11.013
Global CCS Institute (2019). CO2RE Database. Available online at: https://co2re.co
Godard, M., Luquot, L., Andreani, M., and Gouze, P. (2013). Incipient hydration of mantle lithosphere at ridges: a reactive-percolation experiment. Earth Planet. Sci. Lett. 371–372, 92–102. doi: 10.1016/j.epsl.2013.03.052
Goldberg, D., and Slagle, A. L. (2009). A global assessment of deep-sea basalt sites for carbon sequestration. Energy Procedia 1, 3675–3682. doi: 10.1016/j.egypro.2009.02.165
Goldberg, D. S., Kent, D. V., and Olsen, P. E. (2010). Potential on-shore and off-shore reservoirs for CO2 sequestration in Central Atlantic magmatic province basalts. Proc. Natl. Acad. Sci. U.S.A. 107, 1327–1332. doi: 10.1073/pnas.0913721107
Goldberg, D. S., Takahashi, T., and Slagle, A. L. (2008). Carbon dioxide sequestration in deep-sea basalt. Proc. Natl. Acad. Sci. U.S.A. 105, 9920–9925. doi: 10.1073/pnas.0804397105
Gunnarsson, I., Aradóttir, E. S., Oelkers, E. H., Clark, D. E., Arnarson, M. Þ*., Sigfússon, B., et al. (2018). The rapid and cost-effective capture and subsurface mineral storage of carbon and sulfur at the CarbFix2 site. Int. J. Greenhouse Gas Control 79, 117–126. doi: 10.1016/j.ijggc.2018.08.014
Gunter, W. D., Perkins, E. H., and McCann, T. J. (1993). Aquifer disposal of CO2-rich gases: reaction design for added capacity. Energy Convers. Manage. 34, 941–948. doi: 10.1016/0196-8904(93)90040-H
Hariharan, S., and Mazzotti, M. (2017). Kinetics of flue gas CO2 mineralization processes using partially dehydroxylated lizardite. Chem. Eng. J. 324, 397–413. doi: 10.1016/j.cej.2017.05.040
Hariharan, S., Repmann-Werner, M., and Mazzotti, M. (2016). Dissolution of dehydroxylated lizardite at flue gas conditions: III. Near-equilibrium kinetics. Chem. Eng. J. 298, 44–54. doi: 10.1016/j.cej.2016.03.144
Hariharan, S., Werner, M., Hänchen, M., and Mazzotti, M. (2014). Dissolution of dehydroxylated lizardite at flue gas conditions: II. Kinetic modeling. Chem. Eng. J. 241, 314–326. doi: 10.1016/j.cej.2013.12.056
Harrison, A. L., Power, I. M., and Dipple, G. M. (2013). Accelerated carbonation of brucite in mine tailings for carbon sequestration. Environ. Sci. Technol. 47, 126–134. doi: 10.1021/es3012854
Hövelmann, J., Austrheim, H., and Jamtveit, B. (2012). Microstructure and porosity evolution during experimental carbonation of a natural peridotite. Chem. Geol. 334, 254–265. doi: 10.1016/j.chemgeo.2012.10.025
Hovorka, S. D., Benson, S. M., Doughty, C., Freifield, B. M., Sakurai, S., Daley, T. M., et al. (2006). Measuring permanence of CO2 storage in saline formations: the Frio experiment. Environ. Geosci. 13, 105–121. doi: 10.1306/eg.11210505011
Huijgen, W. J. J., Comans, R. N. J., and Witkamp, G. J. (2007). Cost evaluation of CO2 sequestration by aqueous mineral carbonation. Energy Convers. Manage. 48, 1923–1935. doi: 10.1016/j.enconman.2007.01.035
International Energy Agency (2015). Storing CO2 Through Enhanced Oil Recovery: Combining EOR With CO2 Storage (EOR+) for Profit. Paris. Available online at: https://webstore.iea.org/insights-series-2015-storing-co2-through-enhanced-oil-recovery
IPCC Special Report (2018). Global Warming of 1.5 °C. Available online at: https://www.ipcc.ch/sr15/
Ivanova, A., Kashubin, A., Juhojuntti, N., Kummerow, J., Henninges, J., Juhlin, C., et al. (2012). Monitoring and volumetric estimation of injected CO2 using 4D seismic, petrophysical data, core measurements and well logging: a case study at Ketzin, Germany. Geophys. Prospect. 60, 957–973. doi: 10.1111/j.1365-2478.2012.01045.x
Jamtveit, B., Malthe-Sørenssen, A., and Kostenko, O. (2008). Reaction enhanced permeability during retrogressive metamorphism. Earth Planet. Sci. Lett. 267, 620–627. doi: 10.1016/j.epsl.2007.12.016
Jenkins, C., Chadwick, A., and Hovorka, S. D. (2015). The state of the art in monitoring and verification - ten years on. Int. J. Greenhouse Gas Control 40, 312–349. doi: 10.1016/j.ijggc.2015.05.009
Jenkins, C. R., Cook, P. J., Ennis-King, J., Undershultz, J., Boreham, C., Dance, T., et al. (2012). Safe storage and effective monitoring of CO2 in depleted gas fields. Proc. Natl. Acad. Sci. U.S.A. 109, E35–41. doi: 10.1073/pnas.1107255108
Kantola, I. B., Masters, M. D., Beerling, D. J., Long, S. P., and DeLucia, E. H. (2017). Potential of global croplands and bioenergy crops for climate change mitigation through deployment for enhanced weathering. Biol. Lett. 13, 1–7. doi: 10.1098/rsbl.2016.0714
Kaven, J. O., Hickman, S. H., McGarr, A. F., Walter, S., and Ellsworth, W. L. (2014). Seismic monitoring at the Decatur, IL, CO2 sequestration demonstration site. Energy Procedia 63, 4264–4272. doi: 10.1016/j.egypro.2014.11.461
Kelemen, P., de Obeso, J. C., Manning, C. E., Godard, M., Bach, W., Cai, M., et al. (2019). “Peridotite alteration in OmanDP cores,” in Geophysical Research Abstracts 21 : EGU2019-17259 (Vienna).
Kelemen, P. B., Aines, R., Bennett, E., Benson, S. M., Carter, E., Coggon, J. A., et al. (2018). In situ carbon mineralization in ultramafic rocks: natural processes and possible engineered methods. Energy Procedia 146, 92–102. doi: 10.1016/j.egypro.2018.07.013
Kelemen, P. B., Brandt, A. R., and Benson, S. M. (2016). “Carbon dioxide removal from air using seafloor peridotite,” in AGU Fall Meeting Abstract GC21J-07 (San Francisco, CA).
Kelemen, P. B., and Hirth, G. (2012). Reaction-driven cracking during retrograde metamorphism: olivine hydration and carbonation. Earth Planet. Sci. Lett. 345–348, 81–89. doi: 10.1016/j.epsl.2012.06.018
Kelemen, P. B., and Matter, J. (2008). In situ carbonation of peridotite for CO2 storage. Proc. Natl. Acad. Sci. U.S.A. 105, 17295–17300. doi: 10.1073/pnas.0805794105
Kelemen, P. B., Matter, J., Streit, E. E., Rudge, J. F., Curry, W. B., and Blusztajn, J. (2011). Rates and mechanisms of mineral carbonation in peridotite: natural processes and recipes for enhanced, in situ CO2 capture and storage. Annu. Rev. Earth Planet. Sci. 39, 545–576. doi: 10.1146/annurev-earth-092010-152509
Kelley, D. S., Karson, J. A., Blackman, D. K., Fruh-Green, G. L., Butterfield, D. A., Lilley, M. D., et al. (2001). An off-axis hydrothermal vent field near the Mid-Atlantic Ridge at 30 degrees N. Nature 412, 145–149. doi: 10.1038/35084000
Kharaka, Y. K., Thordsen, J. J., Hovorka, S. D., Nance, H. S., Cole, D. R., Phelps, T. J., et al. (2009). Potential environmental issues of CO2 storage in deep saline aquifers: Geochemical results from the Frio-I Brine Pilot test, Texas, USA. Appl. Geochem. 24, 1106–1112. doi: 10.1016/j.apgeochem.2009.02.010
Kim, S., and Hosseini, S. A. (2014). Above-zone pressure monitoring and geomechanical analyses for a field-scale CO2 injection project in Cranfield, MS. Greenhouse Gases Sci. Technol. 4, 81–98. doi: 10.1002/ghg.1388
Krevor, S., Blunt, M. J., Benson, S. M., Pentland, C. H., Reynolds, C., Al-Menhali, A., et al. (2015). Capillary trapping for geologic carbon dioxide storage - from pore scale physics to field scale implications. Int. J. Greenhouse Gas Control 40, 221–237. doi: 10.1016/j.ijggc.2015.04.006
Krevor, S., Perrin, J.-C., Esposito, A., Rella, C., and Benson, S. (2010). Rapid detection and characterization of surface CO2 leakage through the real-time measurement of δ13C signatures in CO2 flux from the ground. Int. J. Greenhouse Gas Control 4, 811–815. doi: 10.1016/j.ijggc.2010.05.002
Krevor, S. C., Graves, C. R., Van Gosen, B. S., and McCafferty, A. E. (2009). Mapping the Mineral Resource Base for Mineral Carbon-Dioxide Sequestration in the Conterminous United States: U.S. Geological Survey Digital Data Series 414. doi: 10.3133/ds414
Kuuskraa, V. (2013). The role of enhanced oil recovery for carbon capture, use, and storage. Greenhouse Gases Sci. Technol. 3, 3–4. doi: 10.1002/ghg.1334
Lackner, K. S., Wendt, C. H., Butt, D. P., Joyce, E. L., and Sharp, D. H. (1995). Carbon dioxide disposal in carbonate minerals. Energy 20, 1153–1170. doi: 10.1016/0360-5442(95)00071-N
Langenbruch, C., and Zoback, M. D. (2016). How will induced seismicity in Oklahoma respond to decreased saltwater injection rates? Sci. Adv. 2, 1–9. doi: 10.1126/sciadv.1601542
Larachi, F., Daldoul, I., and Beaudoin, G. (2010). Fixation of CO2 by chrysotile in low-pressure dry and moist carbonation: Ex-situ and in-situ characterizations. Geochim. Cosmochim. Acta 74, 3051–3075. doi: 10.1016/j.gca.2010.03.007
Larachi, F., Gravel, J. P., Grandjean, B. P. A., and Beaudoin, G. (2012). Role of steam, hydrogen and pretreatment in chrysotile gas-solid carbonation: opportunities for pre-combustion CO2 capture. Int. J. Greenhouse Gas Control 6, 69–76. doi: 10.1016/j.ijggc.2011.10.010
Le Quéré, C., Moriarty, R., Andrew, R. M., Canadell, J. G., Sitch, S., Korsbakken, J. I., et al. (2015). Global carbon budget 2015. Earth Syst. Sci. Data 7, 349–396. doi: 10.5194/essd-7-349-2015
Lewicki, J. L., Hilley, G. E., Dobeck, L., and Spangler, L. (2010). Dynamics of CO2 fluxes and concentrations during a shallow subsurface CO2 release. Environ. Earth Sci. 60, 285–297. doi: 10.1007/s12665-009-0396-7
Li, W., Li, W., Li, B., and Bai, Z. (2009). Electrolysis and heat pretreatment methods to promote CO2 sequestration by mineral carbonation. Chem. Eng. Res. Design 87, 210–215. doi: 10.1016/j.cherd.2008.08.001
Ludwig, K. A., Kelley, D. S., Butterfield, D. A., Nelson, B. K., and Früh-Green, G. (2006). Formation and evolution of carbonate chimneys at the Lost City Hydrothermal Field. Geochim. Cosmochim. Acta 70, 3625–3645. doi: 10.1016/j.gca.2006.04.016
Ludwig, K. A., Shen, C.-C., Kelley, D. S., Cheng, H., and Edwards, R. L. (2011). U-Th systematics and 230Th ages of carbonate chimneys at the Lost City Hydrothermal Field. Geochim. Cosmochim. Acta 75, 1869–1888. doi: 10.1016/j.gca.2011.01.008
Male, E. J., Pickles, W. L., Silver, E. A., Hoffmann, G. D., Lewicki, J. L., Apple, M., et al. (2010). Using hyperspectral plant signatures for CO2 leak detection during the 2008 ZERT CO2 sequestration field experiment in Bozeman, Montana. Environ. Earth Sci. 60, 251–261. doi: 10.1007/s12665-009-0372-2
Marieni, C., Prikryl, J., Aradóttir, E. S., Gunnarsson, I., and Stefánsson, A. (2018). Towards ‘green' geothermal energy: Co-mineralization of carbon and sulfur in geothermal reservoirs. Int. J. Greenhouse Gas Control 77, 96–105. doi: 10.1016/j.ijggc.2018.07.011
Maroto-Valer, M. M., Fauth, D. J., Kuchta, M. E., Zhang, Y., and Andrésen, J. M. (2005). Activation of magnesium rich minerals as carbonation feedstock materials for CO2 sequestration. Fuel Process. Technol. 86, 1627–1645. doi: 10.1016/j.fuproc.2005.01.017
Matter, J. M., Broecker, W. S., Gislason, S. R., Gunnlaugsson, E., Oelkers, E. H., Stute, M., et al. (2011). The CarbFix pilot project - storing carbon dioxide in basalt. Energy Procedia 4, 5579–5585. doi: 10.1016/j.egypro.2011.02.546
Matter, J. M., and Kelemen, P. B. (2009). Permanent storage of carbon dioxide in geological reservoirs by mineral carbonation. Nat. Geosci. 2, 837–841. doi: 10.1038/ngeo683
Matter, J. M., Stute, M., Hall, J., Mesfin, K., Snæbjörnsdóttir, S., Gislason, S. R., et al. (2014). Monitoring permanent CO2 storage by in situ mineral carbonation using a reactive tracer technique. Energy Procedia 63, 4180–4185. doi: 10.1016/j.egypro.2014.11.450
Matter, J. M., Stute, M., Snæbjörnsdottir, S. Ó., Oelkers, E. H., Gislason, S. R., Aradottir, E. S., et al. (2016). Rapid carbon mineralization for permanent disposal of anthropogenic carbon dioxide emissions. Science 352, 1312–1314. doi: 10.1126/science.aad8132
McCollum, D. L., Zhou, W., Bertram, C., de Boer, H.-S., Bosetti, V., Busch, S., et al. (2018). Energy investment needs for fulfilling the Paris Agreement and achieving the sustainable development goals. Nat. Energy 3, 589–599. doi: 10.1038/s41560-018-0179-z
McGlade, C. (2019). Commentary: Can CO2-EOR Really Provide Carbon-Capture Oil? International Energy Agency (IEA). Available online at: https://www.iea.org/newsroom/news/2019/april/can-co2-eor-really-provide-carbon-negativeoil.html
McGrail, B. P., Schaef, H. T., Spane, F. A, Horner, J. A., Owen, A. T., et al. (2017b). Wallula Basalt pilot demonstration project: post-injection results and conclusions. Energy Procedia 114, 5783–5790. doi: 10.1016/j.egypro.2017.03.1716
McGrail, B. P., Schaef, H. T., Spane, F. A., Cliff, J. B., Qafoku, O., Horner, J. A., et al. (2017a). Field validation of supercritical CO2 reactivity with basalts. Environ. Sci. Technol. Lett. 4, 6–10. doi: 10.1021/acs.estlett.6b00387
McGrail, B. P., Spane, F. A., Amonette, J. E., Thompson, C. R., and Brown, C. F. (2014). Injection and monitoring at the wallula basalt pilot project. Energy Procedia 63, 2939–2948. doi: 10.1016/j.egypro.2014.11.316
McKelvy, M. J., Chizmeshya, A. V. G., Diefenbacher, J., Béarat, H., and Wolf, G. (2004). Exploration of the role of heat activation in enhancing serpentine carbon sequestration reactions. Environ. Sci. Technol. 38, 6897–6903. doi: 10.1021/es049473m
Meckel, T. A., Zeidouni, M., Hovorka, S. D., and Hosseini, S. A. (2013). Assessing sensitivity to well leakage from three years of continuous reservoir pressure monitoring during CO2 injection at Cranfield, MS, USA. Int. J. Greenhouse Gas Control 18, 439–448. doi: 10.1016/j.ijggc.2013.01.019
Mervine, E. M., Humphris, S. E., Sims, K. W. W., Kelemen, P. B., and Jenkins, W. J. (2014). Carbonation rates of peridotite in the Samail Ophiolite, Sultanate of Oman, constrained through 14C dating and stable isotopes. Geochim. Cosmochim. Acta 126, 371–397. doi: 10.1016/j.gca.2013.11.007
Mervine, E. M., Wilson, S. A., Power, I. M., Dippler, G. M., Turvey, C. C., Hamilton, J. L., et al. (2018). Potential for offsetting diamond mine carbon emissions through mineral carbonation of processed kimberlite: an assessment of De Beers mine sites in South Africa and Canada. Mineral. Petrol. 12, S755–S765. doi: 10.1007/s00710-018-0589-4
Monkman, S., Kenward, P. A., Dipple, G., MacDonald, M., and Raudsepp, M. (2018). Activation of cement hydration with carbon dioxide. J. Sustain. Cement Based Mater. 7, 160–181. doi: 10.1080/21650373.2018.1443854
National Academies of Sciences Engineering Medicine (2019). Negative Emissions Technologies and Reliable Sequestration: A Research Agenda. Washington, DC: The National Academies Press. Available online at: https://www.nap.edu/catalog/25259/negative-emissions-technologies-and-reliable-sequestration-a-research-agenda
Nordbotten, J. M., Flemisch, B., Gasda, S. E., Nilsen, H. M., Fan, Y., Pickup, G. E., et al. (2012). Uncertainties in practical simulation of CO2 storage. Int. J. Greenhouse Gas Control 9, 234–242. doi: 10.1016/j.ijggc.2012.03.007
O'Connor, W. K., Dahlin, D. C., Rush, G. E., Gerdemann, S. J., Penner, L. R., and Nilsen, D. N. (2005). Aqueous Mineral Carbonation: Mineral Availability, Pretreatment, Reaction Parametrics, and Process Studies. Doe/Arc-Tr-04-002. Department of Energy.
Oelkers, E. H., and Gislason, S. R. (2001). The mechanism, rates and consequences of basaltic glass dissolution: I. An experimental study of the dissolution rates of basaltic glass as a function of aqueous Al, Si and oxalic acid concentration at 25°C and pH = 3 and 11. Geochim. Cosmochim. Acta 65, 3671–3681. doi: 10.1016/S0016-7037(01)00664-0
Oelkers, E. H., Gislason, S. R., and Matter, J. (2008). Mineral carbonation of CO2. Elements 4, 333–337. doi: 10.2113/gselements.4.5.333
Palandri, J. L., and Kharaka, Y. K. (2004). “A compilation of rate parameters of water-mineral interaction kinetics for application to geochemical modeling,” in U.S. Geological Survey 2004–1068 (Menlo Park, CA), 71. doi: 10.3133/ofr20041068
Pan, S. Y., Chang, E. E., and Chiang, P. C. (2012). CO2 capture by accelerated carbonation of alkaline wastes: a review on its principles and applications. Aerosol Air Qual. Res. 12, 770–791. doi: 10.4209/aaqr.2012.06.0149
Park, A.-H. A., and Fan, L.-S. (2004). CO2 mineral sequestration: Physically activated dissolution of serpentine and pH swing process. Chem. Eng. Sci. 59, 5241–5247. doi: 10.1016/j.ces.2004.09.008
Pasquier, L. C., Mercier, G., Blais, J. F., Cecchi, E., and Kentish, S. (2014). Reaction mechanism for the aqueous-phase mineral carbonation of heat-activated serpentine at low temperatures and pressures in flue gas conditions. Environ. Sci. Technol. 48, 5163–5170. doi: 10.1021/es405449v
Paukert Vankeuren, A. N., Matter, J. M., Stute, M., and Kelemen, P. B. (2019). Multitracer determination of apparent groundwater ages in peridotite aquifers within the Samail ophiolite, Sultanate of Oman. Earth Planet. Sci. Lett. 516, 37–48. doi: 10.1016/j.epsl.2019.03.007
Paukert, A. N., Matter, J. M., Kelemen, P. B., Shock, E. L., and Havig, J. R. (2012). Reaction path modeling of enhanced in situ CO2 mineralization for carbon sequestration in the peridotite of the Samail Ophiolite, Sultanate of Oman. Chem. Geol. 330–331, 86–100. doi: 10.1016/j.chemgeo.2012.08.013
Pevzner, R., Shulakova, V., Kepic, A., and Urosevic, M. (2011). Repeatability analysis of land time-lapse seismic data: CO2CRC Otway pilot project case study. Geophys. Prospect. 59, 66–77. doi: 10.1111/j.1365-2478.2010.00907.x
Pokrovsky, O. S., and Schott, J. (2004). Experimental study of brucite dissolution and precipitation in aqueous solutions: Surface speciation and chemical affinity control. Geochim. Cosmochim. Acta 68, 31–45. doi: 10.1016/S0016-7037(03)00238-2
Power, I. M., Harrison, A. L., Dipple, G. M., Wilson, S. A., Kelemen, P. B., Hitch, M., et al. (2013a). Carbon mineralization: from natural analogues to engineered systems. Rev. Mineral. Geochem. 77, 305–360. doi: 10.2138/rmg.2013.77.9
Power, I. M., Wilson, S. A., and Dipple, G. M. (2013b). Serpentinite carbonation for CO2 sequestration. Elements 9, 115–121. doi: 10.2113/gselements.9.2.115
Power, I. M., Wilson, S. A., Small, D. P., Dipple, G. M., Wan, W., and Southam, G. (2011). Microbially mediated mineral carbonation: roles of phototrophy and heterotrophy. Environ. Sci. Technol. 45, 9061–9068. doi: 10.1021/es201648g
Power, I. M., Wilson, S. A., Thom, J. M., Dipple, G. M., Gabites, J. E., and Southam, G. (2009). The hydromagnesite playas of Atlin, British Columbia, Canada: a biogeochemical model for CO2 sequestration. Chem. Geol. 260, 286–300. doi: 10.1016/j.chemgeo.2009.01.012
Pruess, K., García, J., Kovscek, T., Oldenburg, C., Rutqvist, J., Steefel, C., et al. (2004). Code intercomparison builds confidence in numerical simulation models for geologic disposal of CO2. Energy 29, 1431–1444. doi: 10.1016/j.energy.2004.03.077
Raeini, A. Q., Bijeljic, B., and Blunt, M. J. (2018). Generalized network modeling of capillary-dominated two-phase flow. Phys. Rev. E97, 1–20. doi: 10.1103/PhysRevE.97.023308
Renforth, P., Pogge von Strandmann, P. A. E., and Henderson, G. M. (2015). The dissolution of olivine added to soil: Implications for enhanced weathering. Appl. Geochem. 61, 109–118. doi: 10.1016/j.apgeochem.2015.05.016
Renforth, P., Washbourne, C. L., Taylder, J., and Manning, D. A. C. (2011). Silicate production and availability for mineral carbonation. Environ. Sci. Technol. 45, 2035–2041. doi: 10.1021/es103241w
Riaz, A., Hesse, M., Tchelepi, H. A., and Orr, F. M. (2006). Onset of convection in a gravitationally unstable diffusive boundary layer in porous media. J. Fluid Mech. 548, 87–111. doi: 10.1017/S0022112005007494
Ringrose, P., Atbi, M., Mason, D. C., Espinassous, M., Myhrer, Ø., Iding, M., et al. (2009). Plume development around well KB-502 at the in Salah CO2 storage site. First Break 27, 85–89. Available online at: http://www.earthdoc.org/publication/publicationdetails/?publication=28744
Rogelj, J., Shindell, D., Jiang, K., Fifita, S., Forster, P., Ginzburg, V., et al. (2018). “Mitigation pathways compatible with 1.5°C in the context of sustainable development,” in Global Warming of 1.5°C. An IPCC Special Report on the Impacts of Global Warming of 1.5°C above Pre-Industrial Levels and Related Global Greenhouse Gas Emission Pathways, in the Context of Strengthening the Global Response to the Threat of Climate Change, eds V. Masson-Delmotte, P. Zhai, H.-O. Pörtner, D. Roberts, J. Skea, P. R. Shukla, et al., 1–82. Available online at: https://www.ipcc.ch/sr15/chapter/2-0/
Romanak, K. D., Smyth, R. C., Yang, C., Hovorka, S. D., Rearick, M., and Lu, J. (2012). Sensitivity of groundwater systems to CO2: application of a site-specific analysis of carbonate monitoring parameters at the SACROC CO2-enhanced oil field. Int. J. Greenhouse Gas Control 6, 142–152. doi: 10.1016/j.ijggc.2011.10.011
Rouse, J. H., Shaw, J. A., Lawrence, R. L., Lewicki, J. L., Dobeck, L. M., Repasky, K. S., et al. (2010). Multi-spectral imaging of vegetation for detecting CO2 leaking from underground. Environ. Earth Sci. 60, 313–323. doi: 10.1007/s12665-010-0483-9
Rudge, J. F., Kelemen, P. B., and Spiegelman, M. (2010). A simple model of reaction-induced cracking applied to serpentinization and carbonation of peridotite. Earth Planet. Sci. Lett. 291, 215–227. doi: 10.1016/j.epsl.2010.01.016
Rutqvist, J., Vasco, D. W., and Myer, L. (2010). Coupled reservoir-geomechanical analysis of CO2 injection and ground deformations at In Salah, Algeria. Int. J. Greenhouse Gas Control 4, 225–230. doi: 10.1016/j.ijggc.2009.10.017
Sanna, A., Uibu, M., Caramanna, G., Kuusik, R., and Maroto-Valer, M. M. (2014). A review of mineral carbonation technologies to sequester CO2. Chem. Soc. Rev. 43, 8049–8080. doi: 10.1039/C4CS00035H
Schuiling, R. D., and Krijgsman, P. (2006). Enhanced weathering: an effective and cheap tool to sequester CO2. Clim. Change 74, 349–354. doi: 10.1007/s10584-005-3485-y
Shao, Y. (2014). Beneficial Use of Carbon Dioxide in Precast Concrete Production. Montreal, QC: McGill University. Available online at: https://www.osti.gov/servlets/purl/1155035
Shi, J. Q., Imrie, C., Sinayuc, C., Durucan, S., Korre, A., and Eiken, O. (2013). Snøhvit CO2 storage project: assessment of CO2 injection performance through history matching of the injection well pressure over a 32-months period. Energy Procedia 37, 3267–3274. doi: 10.1016/j.egypro.2013.06.214
Sigfusson, B., Gislason, S. R., Matter, J. M., Stute, M., Gunnlaugsson, E., Gunnarsson, I., et al. (2015). Solving the carbon-dioxide buoyancy challenge: The design and field testing of a dissolved CO2 injection system. Int. J. Greenhouse Gas Control 37, 213–219. doi: 10.1016/j.ijggc.2015.02.022
Snæbjörnsdóttir, S., Oelkers, E. H., Mesfin, K., Aradóttir, E. S., Dideriksen, K., Gunnarsson, I., et al. (2017). The chemistry and saturation states of subsurface fluids during the in situ mineralisation of CO2 and H2S at the CarbFix site in SW-Iceland. Int. J. Greenhouse Gas Control 58, 87–102. doi: 10.1016/j.ijggc.2017.01.007
Sridhar, N., and Hill, D. (2011). “Carbon dioxide utilization: electrochemical conversion of CO2 - opportunities and challenges,” in Research and Innovation, no. Position Paper 07-2011. Available online at: https://issuu.com/dnv.com/docs/dnv-position_paper_co2_utilization
Strandli, C. W., and Benson, S. M. (2013). Identifying diagnostics for reservoir structure and CO2 plume migration from multilevel pressure measurements. Water Resour. Res. 49, 3462–3475. doi: 10.1002/wrcr.20285
Strandli, C. W., Mehnert, E., and Benson, S. M. (2014). CO2 plume tracking and history matching using multilevel pressure monitoring at the Illinois basin - Decatur project. Energy Procedia 63, 4473–4484. doi: 10.1016/j.egypro.2014.11.483
Streit, E., Kelemen, P., and Eiler, J. (2012). Coexisting serpentine and quartz from carbonate-bearing serpentinized peridotite in the Samail Ophiolite, Oman. Contribut. Mineral. Petrol. 164, 821–837. doi: 10.1007/s00410-012-0775-z
Talman, S. (2015). Subsurface geochemical fate and effects of impurities contained in a CO2 stream injected into a deep saline aquifer: what is known. Int. J. Greenhouse Gas Control 40, 267–291. doi: 10.1016/j.ijggc.2015.04.019
Tao, Z., and Clarens, A. (2013). Estimating the carbon sequestration capacity of shale formations using methane production rates. Environ. Sci. Technol. 47, 10318–10325. doi: 10.1021/es401221j
Thom, J. G. M., Dipple, G. M., Power, I. M., and Harrison, A. L. (2013). Chrysotile dissolution rates: implications for carbon sequestration. Appl. Geochem. 35, 244–254. doi: 10.1016/j.apgeochem.2013.04.016
Torp, T. A., and Brown, K. R. (2005). CO2 underground storage costs as experienced at Sleipner and Weyburn. Greenhouse Gas Control Technol. 7, 531–538. doi: 10.1016/B978-008044704-9/50054-9
U.S. Department of Energy (DOE) (2010a). Best Practices For: Terrestrial Sequestration of Carbon Dioxide. Available online at: https://https://www.netl.doe.gov/sites/default/files/2018-10/BPM_Terrestrial.pdf
U.S. Department of Energy (DOE) (2010b). Geologic Storage Formation Classification: Understanding Its Importance and Impacts on CCS Opportunities in the United States. DOE/NETL-2010/1420. Washington, DC. Available online at: https://www.netl.doe.gov/sites/default/files/2019-01/BPM_GeologicStorageClassification.pdf
U.S. Department of Energy (DOE) (2015). Developing a Research Agenda for Carbon Dioxide Removal and Reliable Sequestration, 5th Edn. Washington, DC: U.S. Department of Energy.
U.S. Department of Energy (DOE) (2017a). Best practices : Monitoring, Verification, and Accounting (MVA) for Geologic Storage Projects. DOE/NETL-2017/1847. Washington, DC. Available online at: https://www.netl.doe.gov/sites/default/files/2018-10/BPM-MVA-2012.pdf
U.S. Department of Energy (DOE) (2017b). Best Practices: Operations for Geologic Storage Projects. DOE/NETL-2017/1848. Washington, DC. Available online at: https://www.netl.doe.gov/sites/default/files/2019-01/BPM_GeologicStorageClassification.pdf
U.S. Department of Energy (DOE) (2017c). Best Practices: Public Outreach and Education for Geologic Storage Projects. DOE/NETL-2017/1845. Washington, DC. Available online at: https://www.netl.doe.gov/sites/default/files/2018-10BPM_PublicOutreach.pdf
U.S. Department of Energy (DOE) (2017d). Best Practices: Risk Management and Simulation for Geologic Storage Projects. DOE/NETL-2017/1846. Washington, DC. Available online at: https://www.netl.doe.gov/sites/default/files/2018-10/BPM_RiskAnalysisSimulation.pdf
U.S. Department of Energy (DOE) (2017e). Best Practices: Site Screening, Site Selection, and Site Characterization for Geologic Storage Projects. DOE/NETL-2017/1844. Washington, DC. Available online at: https://www.netl.doe.gov/sites/default/files/2018-10/BPM-SiteScreening.pdf
U.S. Environmental Protection Agency (EPA) (2010). Federal requirements under the underground injection control (UIC) program for carbon dioxide (CO2) geologic sequestration (GS) wells final rule. Federal Reg. 75, 77230–77303. Available online at: https://www.federalregister.gov/documents/2010/12/10/2010-29954/federal-requirements-under-the-undergroundinjection-control-uic-program-for-carbon-dioxide-co2
U.S. Environmental Protection Agency (EPA) (2013). Geologic Sequestration of Carbon Dioxide: Underground Injection Control (UIC) Program Class VI Well Area of Review Evaluation and Corrective Action Guidance. EPA 816-R-13-005. Washington, DC. Available online at: https://www.epa.gov/sites/production/files/2015-07/documents/epa816r13005.pdf
U.S. Environmental Protection Agency (EPA) (2016). Capture, Supply, and Underground Injection of Carbon Dioxide. Available online at: https://www.epa.gov/ghgreporting/capture-supply-and-underground-injection-carbon-dioxide
U.S. Geological Survey (USGS) (2013). National Assessment of Geologic Carbon Dioxide Storage Resources. Available online at: https://pubs.usgs.gov/ds/774/
United Nations Environment Programme (UNEP) (2017). The Emissions Gap Report 2017 A UN Environment Synthesis Report. Nairobi. Available online at: https://wedocs.unep.org/bitstream/handle/20.500.11822/22070/EGR_2017.pdf
van Noort, R., Wolterbeek, T., Drury, M., Kandianis, M., and Spiers, C. (2017). The force of crystallization and fracture propagation during in-situ carbonation of peridotite. Minerals 7, 1–31. doi: 10.3390/min7100190
Vasco, D. W., Rucci, A., Ferretti, A., Novali, F., Bissell, R. C., Ringrose, P. S., et al. (2010). Satellite-based measurements of surface deformation reveal fluid flow associated with the geological storage of carbon dioxide. Geophys. Res. Lett. 37, 1–5. doi: 10.1029/2009GL041544
Walsh, F. R., and Zoback, M. D. (2015). Oklahoma's recent earthquakes and saltwater disposal. Sci. Adv. 1, 1–9. doi: 10.1126/sciadv.1500195
Wen, G., and Benson, S. M. (2019). CO2 plume migration and dissolution in layered reservoirs. Int. J. Greenhouse Gas Control 87, 66–79. doi: 10.1016/j.ijggc.2019.05.012
Werner, M., Hariharan, S., Zingaretti, D., Baciocchi, R., and Mazzotti, M. (2014). Dissolution of dehydroxylated lizardite at flue gas conditions: I. Experimental study. Chem. Eng. J. 241, 301–313. doi: 10.1016/j.cej.2013.12.057
Werner, M., Hariharan, S. B., Bortolan, A. V., Zingaretti, D., Baciocchi, R., and Mazzotti, M. (2013). Carbonation of activated serpentine for direct flue gas mineralization. Energy Procedia 37, 5929–5937. doi: 10.1016/j.egypro.2013.06.519
White, D. (2013). Seismic characterization and time-lapse imaging during seven years of CO2 flood in the weyburn field, saskatchewan, canada. Int. J. Greenhouse Gas Control 16S, S78–94. doi: 10.1016/j.ijggc.2013.02.006
Wilson, S. A., Harrison, A. L., Dipple, G. M., Power, I. M., Barker, S. L. L., Ulrich Mayer, K., et al. (2014). Offsetting of CO2 emissions by air capture in mine tailings at the Mount Keith Nickel Mine, Western Australia: rates, controls and prospects for carbon neutral mining. Int. J. Greenhouse Gas Control 25, 121–140. doi: 10.1016/j.ijggc.2014.04.002
Wolff-Boenisch, D., Gislason, S. R., and Oelkers, E. H. (2006). The effect of crystallinity on dissolution rates and CO2 consumption capacity of silicates. Geochim. Cosmochim. Acta 70, 858–870. doi: 10.1016/j.gca.2005.10.016
Würdemann, H., Möller, F., Kühn, M., Heidug, W., Christensen, N. P., Borm, G., et al. (2010). CO2SINK - from site characterisation and risk assessment to monitoring and verification: one year of operational experience with the field laboratory for CO2 storage at Ketzin, Germany. Int. J. Greenhouse Gas Control 4, 938–951. doi: 10.1016/j.ijggc.2010.08.010
Yang, C., Mickler, P. J., Reedy, R., Scanlon, B. R., Romanak, K. D., Nicot, J.-P., et al. (2013). Single-well push-pull test for assessing potential impacts of CO2 leakage on groundwater quality in a shallow Gulf Coast aquifer in Cranfield, Mississippi. Int. J. Greenhouse Gas Control 18, 375–387. doi: 10.1016/j.ijggc.2012.12.030
Zhang, S., and DePaolo, D. J. (2017). Rates of CO2 mineralization in geological carbon storage. Acc. Chem. Res. 50, 2075–2084. doi: 10.1021/acs.accounts.7b00334
Zheng, L., Apps, J. A., Zhang, Y., Xu, T., and Birkholzer, J. T. (2009). On mobilization of lead and arsenic in groundwater in response to CO2 leakage from deep geological storage. Chem. Geol. 268, 281–297. doi: 10.1016/j.chemgeo.2009.09.007
Zheng, X., Cordonnier, B., Zhu, W., Renard, F., and Jamtveit, B. (2018). Effects of confinement on reaction-induced fracturing during hydration of periclase. Geochem. Geophys. Geosyst. 19, 2661–2672. doi: 10.1029/2017GC007322
Keywords: geologic storage, mineral carbonation, mineralization, carbon sequestration, negative emissions, carbon capture and storage
Citation: Kelemen P, Benson SM, Pilorgé H, Psarras P and Wilcox J (2019) An Overview of the Status and Challenges of CO2 Storage in Minerals and Geological Formations. Front. Clim. 1:9. doi: 10.3389/fclim.2019.00009
Received: 02 July 2019; Accepted: 25 October 2019;
Published: 15 November 2019.
Edited by:
Phil Renforth, Heriot-Watt University, United KingdomReviewed by:
Andres Fernando Clarens, University of Virginia, United StatesCopyright © 2019 Kelemen, Benson, Pilorgé, Psarras and Wilcox. This is an open-access article distributed under the terms of the Creative Commons Attribution License (CC BY). The use, distribution or reproduction in other forums is permitted, provided the original author(s) and the copyright owner(s) are credited and that the original publication in this journal is cited, in accordance with accepted academic practice. No use, distribution or reproduction is permitted which does not comply with these terms.
*Correspondence: Jennifer Wilcox, amx3aWxjb3hAd3BpLmVkdQ==
Disclaimer: All claims expressed in this article are solely those of the authors and do not necessarily represent those of their affiliated organizations, or those of the publisher, the editors and the reviewers. Any product that may be evaluated in this article or claim that may be made by its manufacturer is not guaranteed or endorsed by the publisher.
Research integrity at Frontiers
Learn more about the work of our research integrity team to safeguard the quality of each article we publish.