- Gulf Coast Carbon Center, Bureau of Economic Geology, Jackson School of Geosciences, The University of Texas at Austin, Austin, TX, United States
This paper provides an overview of carbon dioxide enhanced oil recovery (CO2-EOR) and its ability to reduce greenhouse gas (GHG) emissions (even to the point of negative emissions), the role it needs to play in the challenge of decarbonization, and the need to scale up implementation and deployment in order to meet climate goals. Limitations in current legal and regulatory frameworks for CO2 injection are explored for both economic and environmental purposes, as well as the economic implications of combining CO2-EOR with geologic carbon storage. Results from a recent study, which demonstrate that all CO2-EOR operations produce negative emissions oil during the first several years of production, are analyzed in the context of the urgency of climate change mitigation. Acknowledging that fossil fuels currently provide the energy foundation upon which global societies function, and that a sudden shift in the composition of that foundation can potentially destabilize the global economy and key elements of modern society, we bring CO2-EOR to the fore as it can supply reduced carbon oil to support the current energy foundation as it steadily transitions toward decarbonization. In order to meet this urgent transition, greater fiscal, and regulatory incentives are needed to begin scaling CO2-EOR with storage around the globe. A viable and large-scale CO2-EOR/storage industry depends upon significant capital investments for CO2 capture and transportation infrastructure. Policy consistency and predictability, combined with targeted subsidies, will help to achieve this goal.
Introduction
Carbon capture and sequestration (CCS), a technology where carbon emissions are captured at a point source, transported, and injected deep underground into a safe, permitted geologic site for long term storage, was included in the portfolio of climate change mitigation options released in 2005 by the Intergovernmental Panel on Climate Change (IPCC) (IPCC, 2005). The technology continued to be acknowledged and strongly reiterated in successive reports by the International Energy Agency (IEA) (International Energy Agency, 2008a,b, 2011, 2013).
The goal of climate change mitigation is to hold the increase of global average temperature to well-below 2°C above pre-industrial levels (Article 2, United Nations, 2015). Although not explicitly mentioned in the Paris Agreement of November 4, 2016, it is clear that extensive investments in large-scale emission reduction technologies like CCS will be required, at least during a transitional stage (Article 4, United Nations, 2015).
Part of the continued discussion of whether CCS will contribute enough to meet climate targets is the discussion around the role of carbon dioxide enhanced oil recovery (CO2-EOR). Storing captured CO2 through CO2-EOR enters into a category called carbon capture, utilization and storage (CCUS), where the captured CO2 is utilized for a commercial activity, in this case EOR, as it becomes ultimately stored.
A few large-scale CCUS projects are operational today. The Weyburn-Midale Carbon Dioxide Project located in Midale, Saskatchewan, Canada, started CO2 injection in October 2000 and continues to produce oil from Weyburn and Midale oil fields at a rate of 14 thousand barrels of oil per day (Jensen, 2019). The CO2 injected is captured at a lignite-fired synfuels plant in Beulah, North Dakota, U.S., and transported to the fields via a 320 km long transnational pipeline. More recently, a couple of large-scale CCUS projects emerged in the U.S. The Air Products Capture Project injects CO2 captured at a hydrogen production facility in Port Arthur, Texas, into the West Hastings field for EOR, and the Petra Nova project injects CO2 captured at NRG's Parish Power Plant, southwest of Houston, into the nearby West Ranch oil field, also for EOR.
Many in the environmental community have argued that CO2-EOR only serves to prolong the use of fossil fuels and, as it produces carbon emitting oil, is not able to reduce emissions. However, a recent study by Núñez-López et al. (2019), shows that, depending on strategic operational choices, the incremental oil produced from CO2-EOR can achieve a net carbon negative status throughout most of the life of the operation (i.e., because a large percentage of the injected CO2 is unavoidably and permanently trapped in the subsurface, as discussed in section Associated Storage of CO2 through EOR). The study uses a novel dynamic lifecycle analysis (d-LCA) that includes greenhouse gas (GHG) emissions at the CO2-EOR site and downstream (including crude oil refining and refined product combustion), and is linked to an operational EOR performance model.
Other LCA studies, such as Aycaguer et al. (2001) and Fox (2009) conclude that GHG emissions generated by the combustion of the final product (e.g., gasoline) are offset by the mass of CO2 storage within the oil reservoir. Most LCA studies for CO2-EOR, however, are difficult to compare because the boundaries used for GHG emission accounting tend to be drawn differently across studies.
Carbon dioxide enhanced oil recovery (CO2-EOR) has historically used more captured CO2 than any other industrial process, and is the only commercially established carbon utilization option that provides large-scale permanent storage for captured CO2. As opposed to carbon storage generally being seen as a waste disposal activity when done in isolation of market activities, carbon storage paired with EOR can be a profitable activity that also reduces greenhouse gas emissions.
At this point in CCS technology development and deployment, much is still uncertain. Starting CCS projects at EOR sites, where oil profits offset the cost of deployment, is the most intuitive and economically justified action at the current stage of technology development. Many of the risks associated with CCS (carbon capture and storage in deep, brine-filled porous rocks referred to as saline aquifers) are reduced with EOR-associated storage. In CO2-EOR the (fluid) trapping and confining qualities of the deep subsurface container are well-demonstrated by the existence of the hydrocarbon accumulation. Furthermore, the historical records available to EOR projects contain valuable data that saline storage projects lack, as saline projects have not benefitted from decades of application. This data availability in EOR projects add value and accuracy to testing and monitoring results, which increases confidence in CCS. The only risk that is higher in EOR than in saline CCS is the larger density of well-penetrations, both from legacy operations and from the EOR activity. However, if operators take the necessary precautions, then the benefits are plentiful.
The long-established process of CO2-EOR has been overseen by existing law, regulations, and standards of the oil and gas industry. Yet, standardizations and framework for monitoring, quantifying and reporting CO2 retention within the reservoir throughout the EOR process have lagged behind. However, with emerging developments in this area and increased confidence that CO2-EOR can be used as a legitimate greenhouse gas emission reduction technology, we may see the adoption of laws, regulations, and standards that will lead to the proliferation of this resource to meet larger climate targets.
Fundamentals of CO2-EOR
Oil field development is carried out in two or three recovery stages. During primary recovery, oil is produced by natural drive mechanisms (dissolved gas expansion, gas cap expansion, saline water influx) supported by the reservoir's natural energy. As reservoir fluids are extracted, the reservoir pressure declines, and so do oil production rates. To prolong the duration of primary production, pressure maintenance and fluid lifting techniques are employed. During secondary recovery, a fluid, most commonly water, is injected (a.k.a. waterflooding) not only to maintain reservoir pressure but also to displace oil toward producing (i.e., extraction) wells. On average, only 30–50% of the oil is recovered after secondary recovery and 50–70% of the oil remains in the reservoir (Stalkup, 1984). Extracting the remaining oil requires more advanced and costly technologies; consequently, reservoirs were historically abandoned at this point.
Any technique applied after secondary recovery is considered tertiary recovery (Lake et al., 2014). EOR is often considered a tertiary phase of recovery for this reason, even though it can be applied at any stage of petroleum field development. In an EOR process, the oil is recovered by the injection of a material that is not originally present in the reservoir; in the case of CO2-EOR, carbon dioxide is the injected material.
Several physical mechanisms enhance oil production when CO2 is introduced into the reservoir. If the technology is applied after waterflooding, the goal is to produce (extract) the mobile oil that was bypassed by water and the immobile residual oil trapped by capillary force. In the desirable case where the reservoir pressure is above the minimum miscibility pressure (MMP) and the injected CO2 and residual oil are miscible, the physical forces holding the two phases apart (interfacial tension) effectively disappears. This promotes a mass transfer (extraction/vaporization) of light and intermediate hydrocarbons, which reduces the residual immobile oil saturation. Additionally, the CO2 rich oil phase expands/swells regaining mobility. Mass transfer is improved at higher pressure, lower reservoir temperature and lighter oil. A reduction of mobile oil saturation can also be achieved through viscosity reduction, and pressure increase (Walsh and Lake, 1989).
Because CO2-EOR is a displacement process, CO2 is injected into the deep subsurface rock reservoir through an injection well to displace oil toward a production (extraction) well. CO2 is produced along with reservoir fluids, separated at the surface, and commonly, reinjected/recycled into the reservoir. The cycle repeats throughout the operation. Although CO2 is injected in supercritical (dense) phase, it remains significantly less viscous than reservoir fluids and thus highly mobile. When mobility contrast is high, an unstable displacement results in the form of viscous fingering (the uneven advance of CO2 -resembling fingers in a profile image- toward a producing well), which adversely impacts oil recovery (Juanes and Blunt, 2007).
To reduce the degree of fingering and stabilize the displacement front, water is injected in alternation with CO2 in a process called Water Alternating Gas (WAG; Caudle and Dyes, 1958). As viscous fingering is a common challenge in CO2-EOR, over 90% of CO2-EOR operations around the world have employed WAG techniques (Merchand, 2017). Figure 1 shows a cross-section illustrating the CO2-EOR displacement process, the mechanisms that enhance oil recovery, and the water-CO2 cycles.
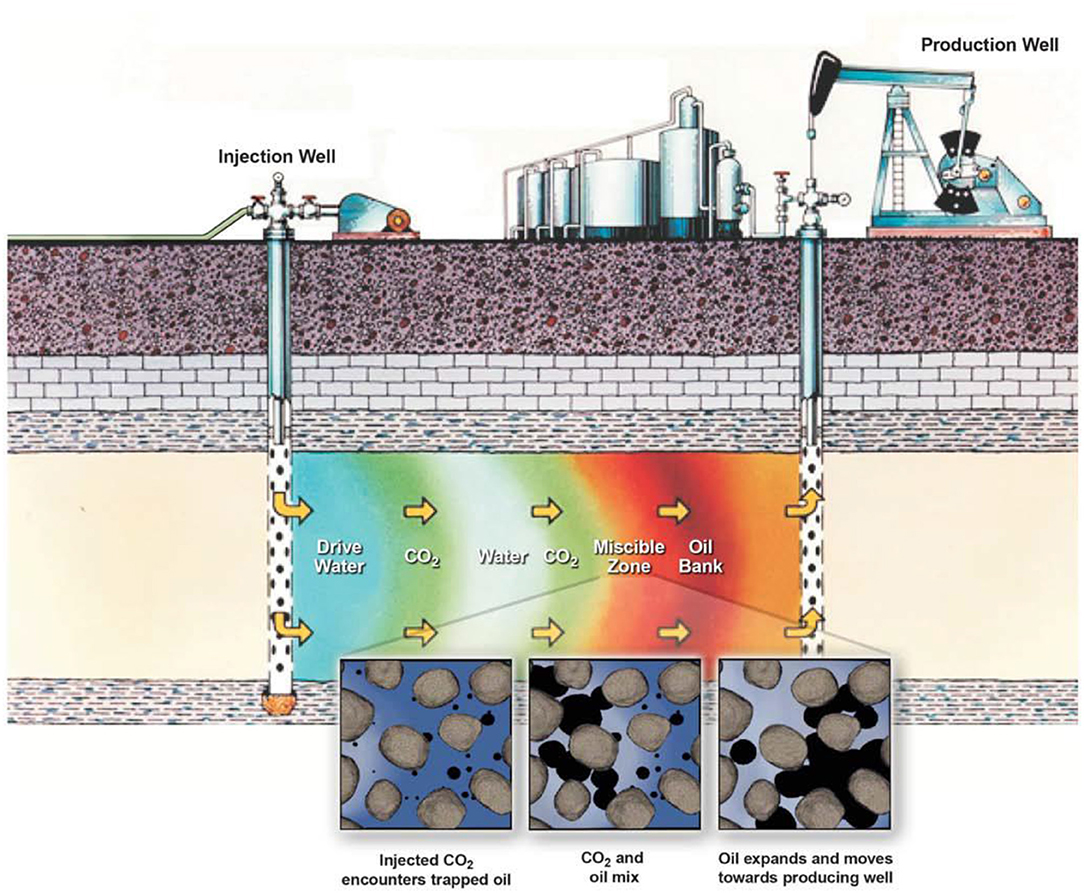
Figure 1. CO2-EOR displacement process (National Energy Technology Laboratory, 2010).
Commercial Motivation for CO2-EOR
The U.S. has an oil resource base on the order of ~600 billion barrels of original oil in place. About one-third of this resource base, ~200 billion barrels, has been recovered after primary and secondary oil production. This means that a significant target of ~400 billion barrels of oil are still trapped in the subsurface (Kuuskraa et al., 2009). Not all of the stranded oil can be produced or placed into proved oil reserves, and not all is amenable for CO2-EOR technologies. The same report by Kuuskraa et al. (2009), estimates that from the ~400 billion barrels of oil remaining, ~84.8 billion barrels of oil are technically recoverable through CO2-EOR applications. However, the CO2-EOR market is still large and very attractive to developers. Several screening methodologies to identify candidate oil reservoirs for CO2-EOR exist in the literature, such as Kovscek (2002), Núñez-López et al. (2008), and Bachu (2016). Some methodologies lay out the conditions for miscible displacement (lighter hydrocarbons, higher pressures, lower temperatures), others rely on the estimation of MMP and screen for reservoirs that have pressures above MMP.
Carbon dioxide enhanced oil recovery (CO2-EOR) has been a successful commercial activity in the U.S. since 1972, when it first started in the Permian Basin, more specifically in SACROC Field, Texas. The Permian Basin, by far the most active CO2-EOR region in the world, is located in West Texas and southeastern New Mexico. This oil producing region, the third largest in the U.S., has produced over 30 billion barrels of oil, out of which 1.3 billion have been produced with CO2 (Merchand, 2017). Current EOR activities there produce on average 350,000 barrels of oil per day (BOPD) (the IEA estimates current world CO2-EOR production at 450,000 BOPD). The CO2, mostly naturally sourced, is transported to oil fields through a network of 4,500 miles of pipelines. The development of this vast CO2 supply infrastructure in the region has resulted from the increasing CO2 demand for EOR. CO2 supply has not always met the demand, restricting EOR production. The latter is starting to force a change in the low-cost natural CO2 supply trend, with forecasts including emerging industrial CO2 capture projects.
Associated Storage of CO2 Through EOR
A large fraction of the CO2 injected becomes trapped within the formation and cannot be produced back to the surface along with the produced reservoir fluids. In fact, the current operational experience is that 90–95% of the purchased CO2 remains geologically trapped (Melzer, 2012). The industry refers to this volume as CO2 retention and is considered a loss that needs to be replaced with new purchased CO2. In CCUS, the CO2 lost to the formation through the conventional process of EOR is referred to as associated storage.
The mechanisms that trapped oil within the reservoir also act to trap CO2. The fact that oil was trapped over geologic time provides confidence that CO2 will be safely trapped/stored for that long in four different forms: (1) structurally beneath an impermeable barrier, (2) residually as an immobile phase due to relative permeability and capillary curve hysteresis, (3) dissolved in reservoir brine and oil, and (4) mineralized over time (see Figure 2).
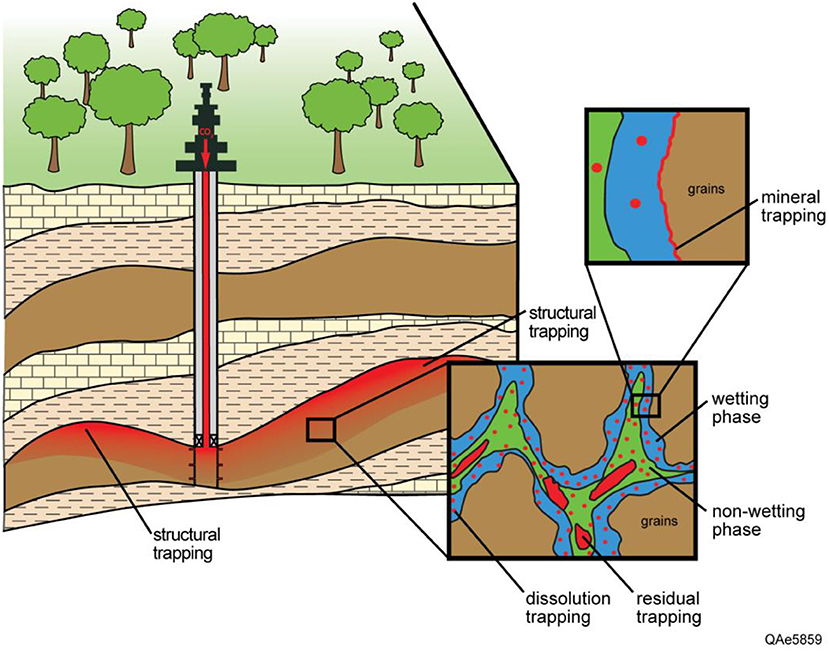
Figure 2. CO2 trapping mechanisms (Hosseininoosheri et al., 2018).
As CO2 is considered a commodity within an economic activity, the primary goal of conventional CO2-EOR is to produce more oil with less purchased CO2. If an incentive to store CO2 exists, through a tax credit or a carbon market, the operator can adjust its practice to achieve a second goal of storing CO2.
The first adjustment is to switch the source of the CO2 from natural to captured from industrial emitters. The second adjustment is in regard to operational changes needed to confirm and account for CO2 storage, and possibly increase the amount of CO2 use. The IEA developed three models that combine oil production with CO2 storage: (1) Conventional EOR+: a conventional practice that maximizes oil production and minimizes CO2 use, but uses additional monitoring and verification practices; (2) Advanced EOR+: the co-exploitation of oil recovery and CO2 storage with larger amounts of CO2 use under Conventional EOR+; and (3) Maximum Storage EOR+: a model focused on maximizing long-term storage of CO2 while achieving the same level of production as Advanced EOR+ (International Energy Agency, 2015).
The use of captured CO2 for EOR dates back to the origins of the EOR industry in the early 1970s with CO2 captured from natural gas production (Marston, 2017). However, the use of natural CO2 (i.e., non-anthropogenic CO2 from the Earth's subsurface) has always been dominant. As of the mid-2010s, over three-quarters of the approximately 60 million tons of CO2 (Godec et al., 2013) used in North American EOR operations came from a few naturally-occurring CO2 reservoirs (Marston, 2018). With recent oil prices, the price paid today for CO2 injection for CO2-EOR averages $40-per-ton (Middleton, 2013; Martin et al., 2017). With prices still running about breakeven, industry does not have enough incentive for a major sourcing push from natural reservoirs to anthropogenic sources, which would increase the cost (Massachusetts Institute of Technology, 2010). Oil fields that are farther away from natural CO2 source reservoirs have more of an incentive to use anthropogenic sources of CO2 (Kuuskraa et al., 2009). Identifying these hot spot areas for development would further progress a likelihood that more anthropogenic-sourced and greenhouse gas-offsetting EOR projects come online.
Carbon Lifecycle Analysis of CCUS Systems
Even though large amounts of CO2 are geologically stored through EOR, the extent to which the technology can reduce greenhouse gas emissions, if any at all, has been assessed by many (Jaramillo et al., 2009; Stewart and Haszeldine, 2014; Cooney, 2015). Carbon lifecycle analysis (LCA) is a systematic process, standardized in ISO 14044:2006, used to assess the environmental impact of a product system throughout the product's lifecycle, from raw materials acquisition through production, use, final treatment, recycle, and disposal. LCA applied to CO2-EOR answers the question of whether CO2 emissions resulting from the EOR energy consumption and, more significantly, from the combustion of the incremental oil produced, are offset by the mass of anthropogenic CO2 stored (Figure 3).
The definition of boundaries in LCA studies is very important. Interpretations and comparison of results among different existing LCA studies of CCUS systems is difficult because different boundaries are used. For example, Jaramillo et al. (2009) assessed the net lifecycle emissions of CO2-EOR in a full CCUS system boundary (cradle-to-grave), from coal mining to product combustion, and concluded that CO2-EOR projects have historically emitted more CO2 than they have removed through geologic storage. On the other hand, Aycaguer et al. (2001) assessed CO2-EOR emissions at the EOR site in a gate-to-gate boundary and concluded that CO2-EOR effectively results in net CO2 emission reduction. Figure 4 shows the general components of CCUS systems and the lifecycle boundaries most commonly used.
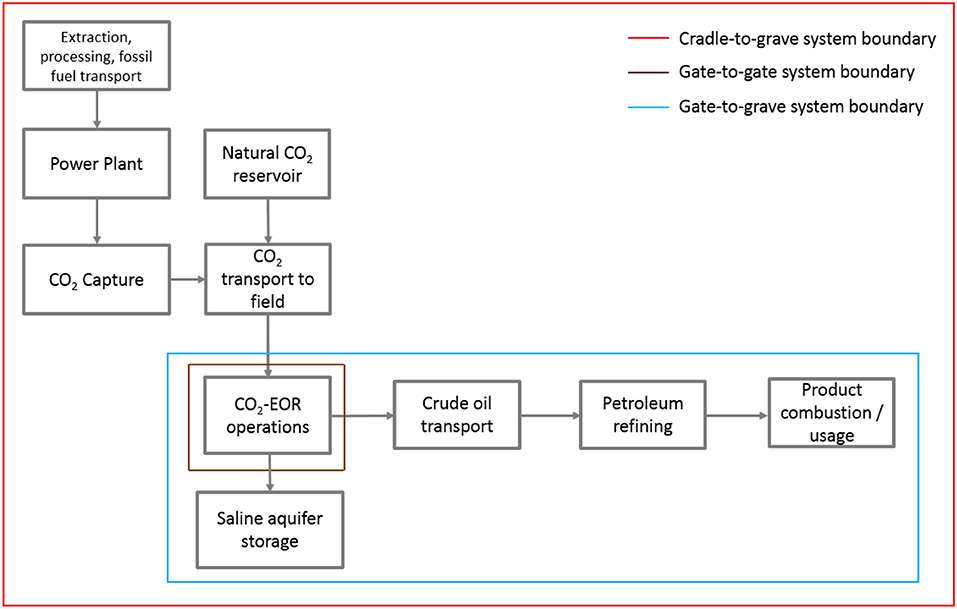
Figure 4. Lifecycle analysis CCUS boundaries. Adapted from Núñez-López et al. (2019).
Núñez-López, first author of this paper, conducted a study that analyzed the dynamic potential of CO2-EOR to reduce greenhouse gas emissions using a novel dynamic LCA (d-LCA). It was determined that a dynamic approach was needed, given that the rate of crude oil production varies significantly with time. The d-LCA linked instant energy demand and associated greenhouse gas (GHG) emissions to instant carbon storage mass, and provided a better understanding of the evolution of the environmental impact (CO2 emissions) and mitigation (geologic CO2 storage) associated with an expanded carbon CCUS system, from start to closure of operations.
The d-LCA was applied to an ongoing CO2-EOR operation in Cranfield field, a 3,000 m. deep clastic reservoir in southwestern Mississippi, U.S. A scenario analysis captured oil production, CO2 storage, and CO2 utilization curves for four common and recent CO2 injection strategies used in the USA: (1) Continuous gas injection (CGI), where CO2 is injected continuously into the oil bearing formation; (2) WAG, where CO2 slugs and brine slugs are injected in an alternating fashion to improve flood conformance and economics; (3) Water curtain injection (WCI), a continuous gas injection with peripheral water injection (commonly along the oil-water contact); and (4) Hybrid WAG + WCI (see Figures 5, 6).
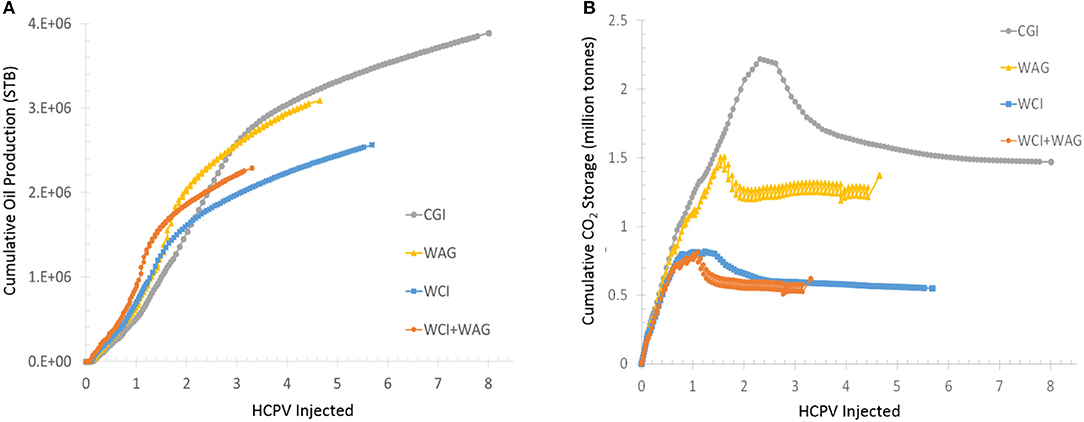
Figure 5. (A) Cumulative oil production as a function of CO2 volumes injected. (B) CO2 storage as a function of CO2 volumes injected. HCPV, hydrocarbon pore volume [intergranular pore volume occupied by hydrocarbons]. Núñez-López et al. (2019).
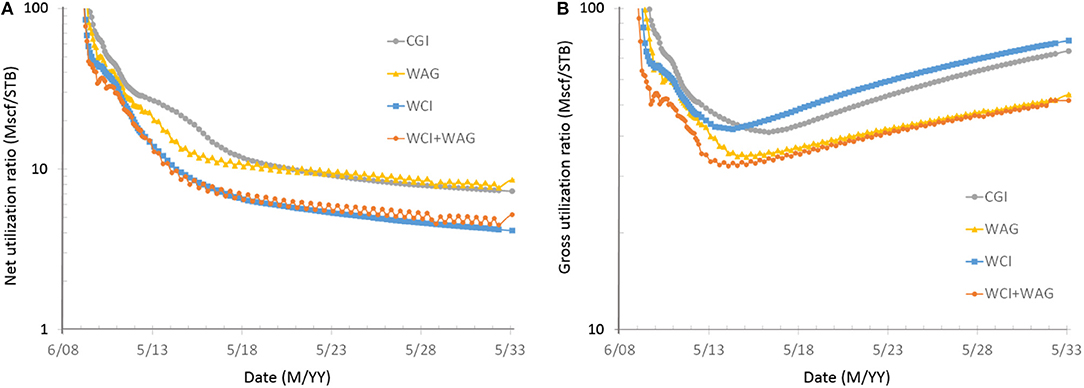
Figure 6. (A) Net utilization ratio (purchased CO2 needed to produce one barrel of oil). (B) Gross CO2 utilization ratio (purchased plus recycled CO2 needed to produce one barrel of oil). Mscf/STB, thousand standard cubic feet per standard barrel. Núñez-López et al. (2019).
Results of the gate-to-grave CCUS system, in a CO2-EOR scheme analogous to IEA's Conventional EOR+ model, indicate that all four CO2 injection scenarios start operating with a negative carbon footprint and, after years of operation transition into operating with a positive carbon footprint (Figure 7). For WAG, the period of negative emissions is longest, from 14 to 18 years depending on the process technology used to separate the CO2 from other reservoir gases at the surface before re-injection. Gas separation technologies (e.g., Ryan-Holmes, membrane, fractionation, and refrigeration) were included as variables as they are carbon intensive.
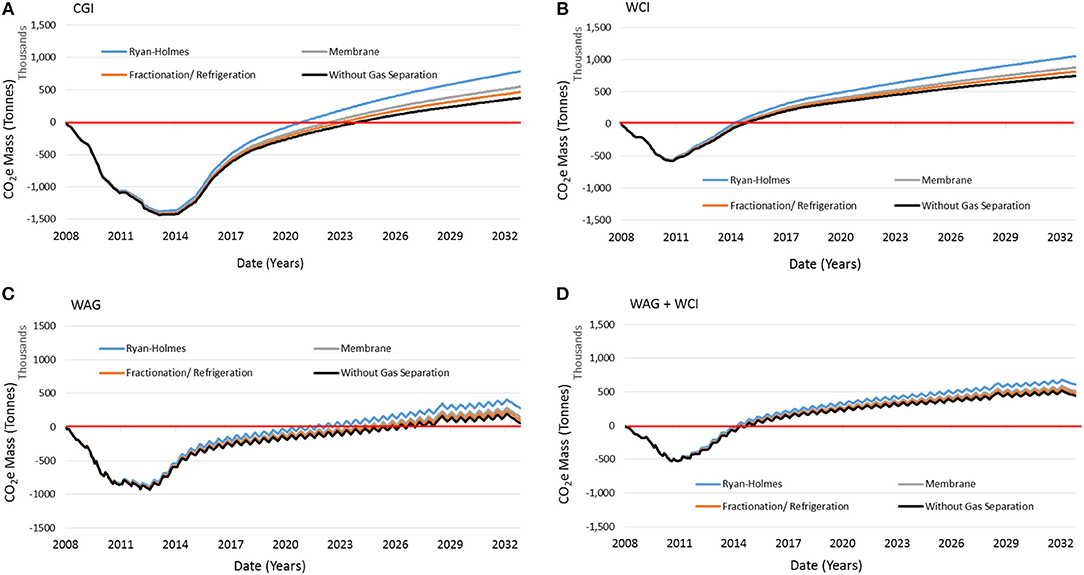
Figure 7. Carbon balance (CO2e emissions minus CO2 storage) of the gate-to-grave CCUS system for: (A) continuous gas injection, (B) water curtain injection, (C) water alternating gas, and (D) Hybrid WAG + WCI. Núñez-López et al. (2019).
The study included a scenario analogous to IEA's Maximum Storage EOR+, where excess CO2 from the recycling facility is injected into an underlying saline aquifer in a stacked storage fashion for long term CO2 offtake and storage. This scenario demonstrates significant potential for improving environmental performance while providing a better understanding of how EOR and saline storage can be co-managed as a CCUS project matures. Results are illustrated in Figure 8, where the negative emissions period is extended considerably in all scenarios. In the WAG scenario, oil can be produced with negative emissions from 18 years to potentially the entire life of the EOR project if gases are not separated at the surface.
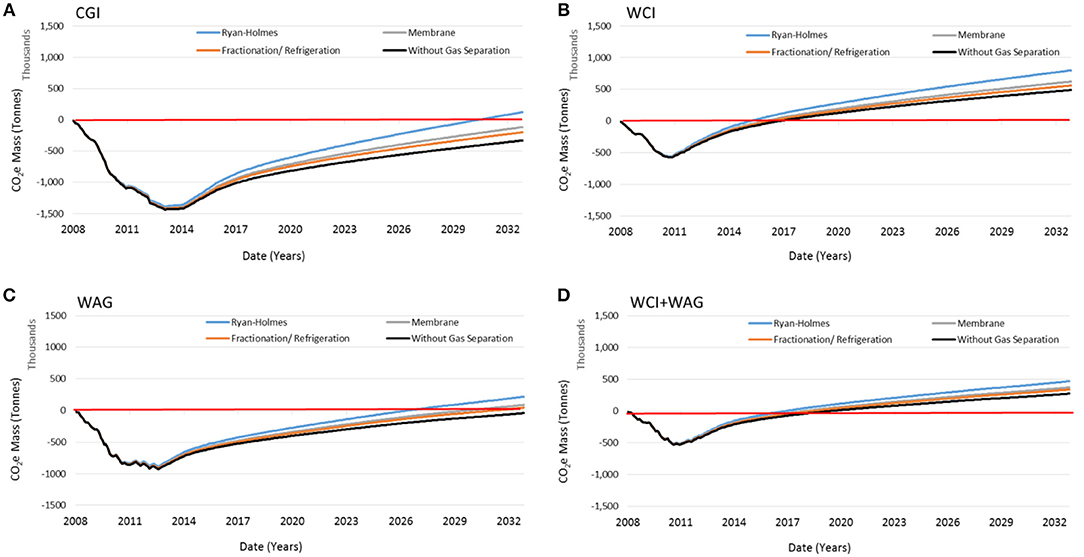
Figure 8. Carbon balance (CO2e emissions minus CO2 storage) of the gate-to-grave CCUS system with stacked saline carbon storage for: (A) continuous gas injection, (B) water curtain injection, (C) water alternating gas, and (D) Hybrid WAG + WCI. Núñez-López et al. (2019).
Results like this, which demonstrate that all CO2-EOR operations produce negative emissions oil during the first several years of production are critical in the context of the urgency of climate change mitigation. Further details of the study can be found in Núñez-López et al. (2019).
CCUS Economics
The proposition that CO2-EOR can be a bridge to deploying CCUS on a larger scale stems from the fact that revenues associated with selling CO2 to an EOR operator can result in substantial income to offset the cost of employing CCS for emissions-heavy industry. This fact has been cited as an opportunity to speed commercial adoption on a large enough scale to provide a means of climate mitigation (Massachusetts Institute of Technology, 2010). The revenue generated from sales of CO2 at capture plants can be enough to offset the cost of the capture technology and transportation to an EOR site. According to the Global CCS Institute (2019), for example, this was the case at the Terrell, Enid Fertilizer, and Great Plains CCS facilities.
Determination of a reservoir to be geologically amenable to CO2-EOR (as discussed in previous sections) does not necessarily imply that CO2-EOR will be economical. In general, the costs of a CO2-EOR operation vary depending on three basic extrinsic parameters: oil price, CO2 cost, and storage tax credit (Ettehadtavakkol et al., 2014). The single largest EOR project cost is the purchase of CO2. CO2-related costs, including the capital costs of CO2 supply, injection, and recycling, can amount to 25–50% of the cost per barrel of EOR oil produced (Kuuskraa et al., 2009).
For this reason, operators design the EOR flood such that the use of CO2 is optimized and purchases are minimized. Oil reservoirs with higher capital cost requirements and less favorable CO2 utilization rates (volume of CO2 needed to produce one barrel of oil) will not achieve economic targets without advanced, highly efficient CO2-EOR technology, and without tax or other fiscal incentives for storing CO2 (National Energy Technology Laboratory, 2010). The efficiency of the CO2 displacement process, which controls critical economic parameters such as oil production rate, CO2 utilization rate, and CO2 recycle ratio, is affected by reservoir rock characteristics, oil quality, production history, and other site-specific parameters (Núñez-López et al., 2019).
To transition to a large-scale CO2-EOR with storage, operators must account for the additional costs of undertaking storage: additional monitoring, measuring, and verification (MMV), and closure activities, which can all influence project costs. The economic costs of reducing emissions from large point sources must be smaller than the additional costs associated with storage (International Energy Agency, 2015). Though companies employing CO2-EOR generally do not make the price of CO2 publically available, the price of CO2 for EOR is known to be generally linked to the price of oil. A general rule to the price an EOR operator is willing to pay is about 2% of the price of a barrel of West Texas Intermediate (WTI) oil per million cubic feet or 28,316.85 million cubic meters of CO2 (Kuuskraa et al., 2011; Middleton, 2013). A price of $40/tCO2 is prevalent in the literature (Middleton, 2013; Martin et al., 2017).
There are several project design and operator choice decisions that influence the return of the CO2-EOR with storage project. The forthcoming National Petroleum Council study report on CCUS will likely have a thorough outline of the project-associated costs of a CO2-EOR project (Tip Meckel, personal communication). Transport and storage costs are outlined in a report from the U.S. Department of Energy (DOE) (National Energy Technology Laboratory, 2013) and the costs associated with verifying CO2 storage during and after EOR are outlined by Godec et al. (2017). King et al. (2013) studied the cost of vertically integrated systems and found that using anthropogenic CO2 in EOR projects generally results in a negative profit (aka financial loss), but the State CO2-EOR Deployment Work Group have reported profit from sourcing anthropogenic CO2 (State CO2-EOR Deployment Work Group, 2016). Varied business models and cost data make project cost studies difficult (Jablonowski and Singh, 2010).
U.S. Legal and Regulatory Framework
As early as the 1970s, targeted efforts by the Federal government were made to increase national security by subsidizing tertiary oil recovery to remove financial risk, rather than bring new fields into production, as a way to boost domestic production (Massachusetts Institute of Technology, 2010). Legislation like the 1976 Emergency Petroleum Allocation Act, the President's 1977 National Energy Plan, and Windfall Profits Taxes in the 1980s all supported the advancement of CO2-EOR (Dooley et al., 2009). Incentives were codified with the U.S. Federal EOR Tax Incentive in 1986 that applied to costs associated with CO2 flood installation, purchase, and injection, triggering a boom in CO2-EOR national growth (National Energy Technology Laboratory, 2010).
While geologists and engineers have increasingly gained confidence in the ability of EOR to effectively store carbon dioxide, several risks, logistical, and financial, still exist for the technology in the context of CCS. The role of a legal and regulatory framework will be crucial in the scale-up of CCS for widespread, commercial deployment to reconcile complex relationships.
To date, fluctuating support and the rate of funding and project emergence has slowed deployment. To counter this, political commitments will be necessary to buoy the recent technological advancements. The first regulatory acknowledgment of the associated risks and long-term nature of CCS was in the early amendments to both the London Protocol and the OSPAR Convention (Havercroft et al., 2018). Five main models of the legislation and regulatory frameworks have emerged since then, demonstrating roadmaps for future frameworks (Havercroft et al., 2018), summarized here:
• Stand-alone legislation dealing with CCS as a stand-alone technology
• Stand-alone legislation confined to specific projects
• Adaptation or amendment of existing and familiar petroleum and gas regimes
• Mixed regimes involving a stand-alone regime coupled with significant adaptation to existing legislation
• Adaptation of existing environmental laws to develop a comprehensive CCS regime.
Carbon dioxide enhanced oil recovery (CO2-EOR) in the U.S. has benefitted from a long history of oil and gas development, particularly in Texas, where the first large-scale CO2-EOR project was demonstrated in the Permian Basin in the early 1970s at SACROC (Hill et al., 2013). The U.S. legal and regulatory framework for CO2-EOR (e.g., mineral rights, subsurface data) has largely been addressed since then (Kuuskraa et al., 2011). Because the original intent of CO2 injection was for tertiary oil production for economic benefit and not permanent storage (Kovscek and Cakici, 2005; Leach et al., 2011; Ettehadtavakkol et al., 2014), the existing U.S. framework for CO2-EOR is largely based on existing oil and gas regulations. Emerging CCS frameworks have begun to distinguish between CO2 injection for permanent storage and CO2 injection for EOR as mutually-exclusive endeavors.
Many active projects benefit from policy-blend initiatives. One example is the Texas Clean Energy Project, a combination of private finance benefitting from a federal grant (e.g., D.O.E. funding), federal tax credits, and state bills (Kapetaki and Scowcroft, 2017). Because CO2 capture and storage currently has no direct market, additional government incentives have been pursued in recent years. In February 2018, the 45Q tax credit was amended and expanded under the FUTURE Act. The incentive increased to a 10-year ramp up to $35 and $50 per ton of CO2, respectively. The $35 per ton of CO2 was also expanded to include CO2 utilization other than CO2-EOR (e.g., converting captured CO2 to fuels) and the cap of 75 million tons was removed (Zapantis et al., 2018). The 45Q expansion amendments follow many recommendations laid out by State CO2-EOR Deployment Work Group 2016).
In 2015, the EPA issued regulatory guidance that enabled CO2 injected during EOR to also be classed as stored CO2 (Global CCS Institute, 2015). CO2 injection activities associated with EOR are regulated under Class II of EPA's Underground Injection Control (UIC) Program (as opposed to Class VI wells for geologic sequestration of CO2) and most states have primary enforcement authority—or primacy (Primary Enforcement Authority for the Underground Injection Control Program, n.d.). The EPA has released guidance on how a project can transition into a storage project under Class VI (Environmental Protection Agency, 2013). Sites aiming to transition will need to plan for increased regulatory requirements like monitoring and verification of storage (Adelman, 2018).
Under the Clean Air Act subparts RR and UU, CO2-EOR facilities are required by law to report greenhouse gas data to the EPA annually. Subpart RR applies to facilities that inject CO2 for sequestration and subpart UU applies to facilities that inject CO2 underground for any reason, including enhanced oil and gas recovery. The first edition of the non-legally-binding ISO standard 27916:2019, titled “Carbon dioxide capture, transportation and geological storage—Carbon dioxide storage using enhanced oil recovery (CO2-EOR),” was published in January of 2019 to help operators resolve the previous complexities of accounting for long-term storage in CO2-EOR projects, including how to account for recycling and anthropogenic CO2 use when mixed with non-anthropogenic sources. The standard accounting does not include lifecycle emissions (International Organization for Standardization, 2019).
Role of CO2-EOR in the Larger Decarbonization Picture
The latest IPCC Special Report (IPCC, 2018) calls for the large-scale transformation of a global energy system that needs to be urgently decarbonized in order to mitigate the impending effects of climate change. Renewable energy and energy efficiency are identified as the most cost-effective pathways to achieve 90% of the emission reduction goals, with CCUS acknowledged in most models as a critical technology in the mitigation portfolio. The IEA Sustainable Development Scenario (SDS) allocates to CCUS 7% of cumulative emission reductions needed by 2040.
The need to reduce GHG emissions is unquestionable, but the subject of decarbonization of energy systems is complex, as developed and developing societies still rely on fossil fuels. Some developing countries justifiably resist carrying out the costs of decarbonization, not having contributed to the global problem as developed nations have. At the same time, developed nations resist bearing those costs alone in a competitive global economy.
According to IEA's Key World Energy Statistics (International Energy Agency, 2018) wind and solar still supply <5% of the world's energy, with fossil fuels continuing to supply a steady 80%. Unquestionably, fossil fuels currently provide the energy foundation upon which global societies function, and any sudden shift in the composition of that foundation can potentially destabilize the global economy and key elements of modern society.
The energy transition will require a balanced action plan in which CCUS plays a critical role, as the only technology with large enough scale to significantly reduce emissions from coal and gas power generation, as well as the only technology through which industries like steel, cement and petrochemicals can be decarbonized (Bui et al., 2018). Without other carbon utilization technologies quickly outpacing CO2-EOR, negative emission technologies like bioenergy with CCS (BECCS) and direct air capture (DAC) will need geologic carbon storage.
In CCUS, the means for decarbonization is the mass of captured CO2 that can be stored in oil reservoirs through EOR as well as in saline aquifers underneath the same EOR footprint. Simultaneously, CO2-EOR can supply reduced carbon oil to support the energy foundation, as well as provide what is most critically needed, time to find long lasting climate solutions.
The Future of Negative Emissions Oil: Scaling Up for Climate Mitigation
An industrial-scale EOR-storage implementation faces several challenges. The revenue of EOR must be matched with accelerating measures such as those seen in other countries that lower the price of commercial-scale implementation, including: tax credits (carrot), carbon pricing (stick), emissions regulation, capital grants, and state ownership of CCS facilities (Global CCS Institute, 2019).
Only nine natural CO2 reservoirs remain commercially viable in the U.S. Natural reservoirs accounted for 85% of all U.S. CO2 supply in 2010. Anthropogenic, captured CO2 from natural gas processing and hydrocarbon conversions are the source for the majority of the remaining CO2 for EOR (DiPietro et al., 2012). The lack of available CO2 limits the growth of EOR (Benson et al., 2012). A study by Advanced Resources International (ARI) states that an additional 4–47 billion barrels of domestic resources could be economically recovered using CO2-EOR and at least 8 billion tons of CO2 could be sequestered in the U.S. by using EOR (Advanced Resources International Inc, 2011).
Carbon dioxide enhanced oil recovery (CO2-EOR) offers large CO2 storage capacity potential and could accommodate a major portion of the CO2 captured from industrial facilities for the next 30 years (Godec et al., 2013). Assuming that renewable energy generation continues to increase and displace fossil fuel electricity generation, industry-generated CO2 (steel mills, cement, chemicals) will continue to contribute a larger portion of national emissions. These are often considered “locked-in” emissions because a decarbonized alternative does not exist. The only way to decarbonize those industries is through CCS.
In order to be considered for its climate mitigation value, CO2-EOR projects must transition to using either anthropogenic CO2 captured at industrial sources or CO2 from DAC. Combining DAC with CO2-EOR/storage on a commercial scale is the goal of a recent project announced by Carbon Engineering and Oxy Low Carbon Ventures, where a DAC facility is being designed to capture 500,000 tons of CO2 a year directly from the atmosphere for EOR and subsequent geologic storage in a Permian Basin oil field (Global CCS Institute, 2019). This project is being regarded as “the world's largest direct air capture and sequestration facility.” However, for scale-up to be sufficient to significantly impact national emissions, capture will have to happen across sectors, starting with the most economical prospects.
Bains et al. (2017) conclude that, based on the purity of the stream (lower cost), the relative contribution to overall industrial process emissions, and the proximity to potential storage reservoirs, the best implementation path forward is to prioritize initial capture efforts on ethanol production facilities, then cement and ammonia industries, and finally natural gas processing and ethylene oxide production plants. The lowest cost opportunities for deploying CCS have been found to be in the Midwest and along the Gulf Coast, where extensive geologic storage reservoirs are co-located with EOR operations (Bains et al., 2017).
The hub nature of scale-up will require intensive upfront time and financial investments as one capture, transport, or storage value chain is completed. Because of this, anthropogenic-supplied CO2 is expected to be delivered in “bulky increments” (Marston, 2018), and early adopters are vulnerable to infrastructure devaluation, stranded assets, and missing markets (Zapantis et al., 2019). Banks and insurance companies offering high risk premiums and insurance can help offset risk (Zapantis et al., 2019). However, Dooley et al. (2010) suggest that this will lead to oligopoly (where a small number of suppliers control supply) when CO2 quantities are scarce at the beginning of scale up, which enables CO2 to have a positive price. As more anthropogenic CO2 sources become available, a set price on carbon will be needed to sustain values.
The scale-up problem that CCS faces is largely the result of an insufficient value on carbon—presenting risks to private sector investments and, by proxy banking institutions, which have the potential to be partially alleviated through policy and regulation (Zapantis et al., 2019). The CO2 pipeline infrastructure scale-up needed between customers and suppliers of anthropogenic CO2 to meet a U.S. climate policy case scenario laid out in Wallace et al. (2015) is unprecedented but comparable to pipeline projections in other sectors. Turning the market focus from the value of oil to climate mitigation would likely require additional funding to help offset the “technology valley of death” that many emerging technologies face (i.e., technologies that are technically proven but unable to bridge the gap to commercial-scale). Because companies are already implementing the technology outside of a stable political and economic framework, the private sector is assuming the economic, technical, construction, and operational risks, but these risks could be partially abated by a policy infrastructure.
Carbon dioxide enhanced oil recovery (CO2-EOR) is the main conduit through which companies planning to or already employing CCS find value in the face of political uncertainty. For simplicity, Marston and Moore (2008) advises to keep CCS frameworks in the hands of the oil and gas regulatory authority since many years will transpire before commercial scale volumes of CO2 from power plants exceed the capacity of the EOR industry.
The Global CCS Institute (2015) concluded that the U.S. framework has not dealt with CCS in a fully-integrated, comprehensive manner at the state or the federal level. Proper division of state and federal responsibilities will be required for commercialization (Ekins et al., 2017). By incorporating what Ekins et al. (2017) call “flexibility instruments” that incorporate legal adaptation in legislation, federal, and local governments can better navigate any unintended consequences of adopted legislation.
The lack of a comprehensive CCS regulatory regime has been cited by numerous experts as a primary obstacle to deployment (Davies et al., 2013). The current mix of federal and state policies is what the State CO2-EOR Deployment Work Group 2016) describes as “too cumbersome for project developers to utilize effectively.” The Work Group recommends that Congress establish federal price stabilization contracts, or contracts for differences (CfD) to reduce price volatility between capture facilities and EOR operators (in non-vertically-integrated projects), to make carbon capture eligible for tax-exempt private activity bonds (PABs), and master limited partnerships (MLPs) to provide debt and equity on more favorable terms. There are generalized models of CCS regulatory frameworks that have the potential to support commercial-scale development country-wide (Ekins et al., 2017, p. 83; Jacobs and Craig, 2017).
Conclusions
Carbon dioxide enhanced oil recovery (CO2-EOR) has potential for decarbonization during the first several years of operation. The timing of EOR net emission reductions (the first years, not the last) is of critical importance given the urgent need to abate climate change. The near-term profitability of this climate mitigation opportunity can accelerate deployment of CCS in general and economically incentivize research in support of this goal. As we get closer to maxing out the global carbon budget, CCS will become an increasingly important carbon removal technology.
Carbon dioxide enhanced oil recovery (CO2-EOR) is the only commercially established carbon utilization option that provides large-scale permanent storage for captured CO2, and CCS is the only technology through which industries like steel, cement, and petrochemicals can be decarbonized. As part of an already established market, carbon storage paired with EOR can be a profitable activity that also reduces greenhouse gas emissions. Until other utilizations for carbon under CCUS become more widely adopted than CO2-EOR, carbon removal technologies like BECCS and DAC projects will require a geological carbon storage counterpart, and the reservoir knowledge gleaned from CO2-EOR projects will prove worthwhile even if oil production and use slows.
Carbon dioxide enhanced oil recovery (CO2-EOR) is at the nexus of energy production and environmental protection, reflecting various tensions in the competing spheres. CO2-EOR has benefitted from decades of technical experience and policy development under an oil and gas regulatory framework while CCS has remained largely under a separate environmental law framework. As CO2-EOR becomes a more appealing and viable entryway to scale up CCS infrastructure, the two legal and regulatory frameworks will have to be reconciled so that the storage achieved during CO2-EOR is supported by robust documentation and procedure.
Though large technology scale-ups take time, they are not unprecedented. The most direct corollary to the type of massive, game-changing buildout that CO2-EOR requires is the development of unconventional natural gas resources. By exploiting the existing conventional oil and natural gas resources, the unconventional natural gas infrastructure developed a critical market mass use in the U.S. within roughly a decade, or what has been called, “seemingly serendipitous development” (Massachusetts Institute of Technology, 2010). With the economy refocusing on a low-carbon future, what was previously ignored as a climate mitigation option may become the basis of a large, multi-pronged market under a carbon capture, storage, and utilization framework. EOR is one way that existing infrastructure can best leverage carbon prices to develop a climate mitigation technology for a shifting energy landscape.
Author Contributions
VN-L conceptualized the article and contributed to sections Fundamentals of CO2-EOR, Commercial Motivation for CO2-EOR, Associated Storage of CO2 through EOR, Carbon Lifecycle Analysis of CCUS Systems, and Role of CO2-EOR in the Larger Decarbonization Picture. EM helped in the conceptualization of the article and contributed to sections CCUS Economics, U.S. Legal and Regulatory Framework, and The Future of Negative Emissions Oil: Scaling Up for Climate Mitigation.
Funding
This study was funded by the Gulf Coast Carbon Center, an Industrial Associates program within the Bureau of Economic Geology (BEG) of the University of Texas at Austin.
Conflict of Interest
The authors declare that the research was conducted in the absence of any commercial or financial relationships that could be construed as a potential conflict of interest.
Acknowledgments
We would like to acknowledge the Gulf Coast Carbon Center (GCCC) at the Bureau of Economic Geology (BEG) of the University of Texas at Austin for funding this study.
References
Adelman, D. E. (2018). “Chapter 7: Confronting the bleak economics of CCS in the United States,” in Carbon Capture and Storage: Emerging Legal and Regulatory Issues, 2nd Edn, eds I. Havercroft, R. Macrory, and R. Stewart (Hart Publishing).
Advanced Resources International Inc (ARI) (2011). Global Technology Roadmap for CCS in Industry–Sectoral Assessment–CO2 Enhanced Oil Recovery. Report prepared for United Nations Industrial Development Organization.
Aycaguer, A. C., Lev-On, M., and Winer, A. M. (2001). Reducing carbon dioxide emissions with enhanced oil recovery projects: a life cycle assessment approach. Energy Fuels 15, 303–308. doi: 10.1021/ef000258a
Bachu, S. (2016). Identification of oil reservoirs suitable for CO2-EOR and CO2 storage (CCUS) using reserves databases, with application to Alberta, Canada. Int. J. Greenh. Gas Control 44, 152–165. doi: 10.1016/j.ijggc.2015.11.013
Bains, P., Psarras, P., and Wilcox, J. (2017). CO2 capture from the industry sector. Prog. Energy Combust. Sci. 63, 146–172. doi: 10.1016/j.pecs.2017.07.001
Benson, S. M., Bennaceur, K., Cook, P., Davison, J., de Coninck, H., Farhat, K., et al. (2012). “Carbon capture and storage,” in Global Energy Assessment–Toward a Sustainable Future, eds L. Gomez-Echeverri, T. B. Johansoon, N. Nakicenovic, and A. Patwardhan (Cambridge; New York, NY: Cambridge University Press; Laxenburg: International Institute for Applied Systems Analysis), 993–1068.
Bui, M., Adjiman, C., Bardow, A., Anthony, E., Boston, A., Brown, S., et al. (2018). Carbon capture and storage (CCS): the way forward. Energy Environ. Sci. 11, 1062–1176. doi: 10.1039/c7ee02342a
Caudle, B. H., and Dyes, A. B. (1958). “Improving miscible displacement by gas-water injection,” in Paper presented at 32nd Annual Fall Meeting of Society of Petroleum Engineers, Vol. 213 (Dallas, TX: Petroleum Transactions, AIME), 281–283.
Cooney, G., Littlefield, J., Marriott, J., and Skone, T. (2015). Evaluating the climate benefits of CO2-enhanced oil recovery using life cycle analysis. Environ. Sci. Technol. 12, 7491–7500. doi: 10.1021/acs.est.5b00700
Davies, L. L., Uchitel, K., and Ruple, J. (2013). Understanding barriers to commercial-scale carbon capture and sequestration in the United States: an empirical assessment. Energy Policy 59, 745–761. doi: 10.1016/j.enpol.2013.04.033
DiPietro, P., Balash, P., and Wallace, M. (2012). A Note on Sources of CO2 Supply for Enhanced Oil Recovery Operations. SPE Economics & Management, 69–74.
Dooley, J. J., Dahowski, R. T., and Davidson, C. L. (2009). Comparing existing pipeline networks with the potential scale of future U.S. CO2 pipeline networks. Energy Proc. 1, 1595–1602. doi: 10.1016/j.egypro.2009.01.209
Dooley, J. J., Dahowski, R. T., and Davidson, C. L. (2010). CO2-Driven Enhanced Oil Recovery as a Stepping Stone to What? Pacific Northwest National Laboratory, Prepared for the U.S. Department of Energy under Contract DE-AC05-76RL01830.
Ekins, P., Hughes, N., Pye, S., Winning, M., Macrory, R., Milligan, B., et al. (2017). The Role of CCS in Meeting Climate Policy Targets. Global Carbon Capture and Storage Institute (GCCSI).
Environmental Protection Agency (EPA) (2013). Draft Underground Injection Control (UIC) Program Guidance on Transitioning Class II Wells to Class VI Wells. Washington, DC: US Environmental Protection Agency.
Ettehadtavakkol, A., Lake, L. W., and Bryant, S. L. (2014). CO2-EOR and storage design optimization. Int. J. Greenh. Gas Control 25, 79–92. doi: 10.1016/j.ijggc.2014.04.006
Fox, C. E. (2009). CO2 EOR Carbon Balance at SACROC. LP (Power Point Presentation). Houston, TX: Kinder Morgan CO2 Company.
Global CCS Institute (GCCSI) (2019). Oxy and Carbon Engineering Partner to Combine Direct Air Capture and Enhanced Oil Recovery Storage. News and Media report, 5th June 2019.
Global CCS Institute (GCCSI) (2015). The Global Status of CCS 2015 Summary Report. Global CCS Institute.
Godec, M. L., Kuuskraa, V. A., and Dipietro, P. (2013). Opportunities for using anthropogenic CO2 for enhanced oil recovery and CO2 storage. Energy Fuels 8, 4183–4189. doi: 10.1021/ef302040u
Godec, M. L., Riestenberg, D., and Cyphers, S. (2017). Potential issues and costs associated with verifying CO2 storage during and after CO2-EOR. Energy Proc. 114, 7399–7414. doi: 10.1016/j.egypro.2017.03.1870
Havercroft, I., Macrory, R., and Stewart, R. B. (eds.). (2018). Carbon Capture and Storage: Emerging Legal and Regulatory Issues. Hart Publishing.
Hill, B., Hovorka, S. D., and Melzer, S. (2013). Geologic carbon storage through enhanced oil recovery. Energy Proc. 37, 6808–6830. doi: 10.1016/j.egypro.2013.06.614
Hosseininoosheri, P., Hosseini, S. A., Nuñez-López, V., and Lake, L. W. (2018). Impact of field development strategies on CO2 trapping mechanisms in a CO2-EOR field: A case study in the permian basin (SACROC unit). Int. J. Greenh. Gas. Con. 72, 92–104. doi: 10.1016/j.ijggc.2018.03.002
International Energy Agency (IEA) (2008b). CO2 Capture and Storage: A Key Abatement Option. Paris: IEA/OECD.
International Energy Agency (IEA) (2015). Storing CO2 Through Enhanced Oil Recovery: Combining EOR With CO2 Storage (EOR+) for profit. Insights Series 2015. Paris: International Energy Agency
International Energy Agency (IEA) (2018). Key World Energy Statistics. Available online at: https://www.iea.org/newsroom/events/statistics-release-of-key-world-energy-statistics-2018.html (accessed April, 2019).
International Energy Agency (IEA) (2013). Technology Roadmap–Carbon Capture and Storage. Available online at: https://webstore.iea.org/technology-roadmap-carbon-capture-and-storage-2013 (accessed April, 2019).
International Energy Agency (IEA) (2011). Technology Roadmap–Carbon Capture and Storage in Industrial Applications. Available online at: https://webstore.iea.org/technology-roadmap-carbon-capture-and-storage-in-industrial-applications (accessed April, 2019).
International Organization for Standardization (ISO) (2019). ISO 27916:2019 Carbon Dioxide Capture, Transportation and Geological Storage—Carbon Dioxide Storage Using Enhanced Oil Recovery (CO2-EOR). Available online at: https://www.iso.org/standard/65937.html
IPCC (2005). “IPCC special report on carbon dioxide capture and storage,” in Prepared by Working Group III of the Intergovernmental Panel on Climate Change, eds B. Metz, O. Davidson, H. C. de Coninck, M. Loos, and L. A. Meyer (Cambridgel; New York, NY: Cambridge University Press), 442.
IPCC (2018). “Global warming of 1.5°C. An IPCC Special Report on the impacts of global warming of 1.5°C above pre-industrial levels and related global greenhouse gas emission pathways,” in The Context of Strengthening the Global Response to the Threat of Climate Change, Sustainable Development, and Efforts to Eradicate Poverty, eds V. Masson-Delmotte, P. Zhai, H. O. Pörtner, D. Roberts, J. Skea, P.R. Shukla, A. Pirani, W. Moufouma-Okia, C. Péan, R. Pidcock, S. Connors, J. B. R. Matthews, Y. Chen, X. Zhou, M. I. Gomis, E. Lonnoy, T. Maycock, M. Tignor, and T. Waterfield (IPCC).
Jablonowski, C. J., and Singh, A. (2010). “A survey of CO2-EOR and CO2 storage project costs,” in SPE International Conference on CO2 Capture, Storage, and Utilization (New Orleans, LA). doi: 10.2118/139669-MS
Jacobs, W. B., and Craig, M. (2017). Legal pathways to widespread carbon capture and sequestration. Environ. Law Rep. 47, 1022–1047.
Jaramillo, P., Griffin, W. M., and McCoy, S. T. (2009). Life cycle inventory of CO2 in an enhanced oil recovery system. Environ. Sci. Tech. 43, 8027–8032. doi: 10.1021/es902006h
Jensen, G. (2019). Assessing the Potential for CO2-EOR and CO2 Storage in Depleted Oil Pools in Southeastern Saskatchewan, Canada. 2019 AAPG Annual Convention and Exhibition, San Antonio, TX, May 19–22.
Juanes, R., and Blunt, M. J. (2007). Impact of viscous fingering on the prediction of optimum WAG ratio. Soc. Pet. Eng. J. 12, 486–495. doi: 10.2118/99721-PA
Kapetaki, Z., and Scowcroft, J. (2017). Overview of carbon capture and storage (CCS) demonstration project business models: risks and enablers on the two sides of the Atlantic. Energy Proc. 114, 6623–6630. doi: 10.1016/j.egypro.2017.03.1816
King, C. W., Gülen, G., Cohen, S. M., and Nuñez-Lopez, V. (2013). The system-wide economics of a carbon dioxide capture, utilization, and storage network: Texas Gulf Coast with pure CO2-EOR flood. Environ. Res. Lett. 8:034030. doi: 10.1088/1748-9326/8/3/034030
Kovscek, A. R. (2002). Screening criteria for CO2 storage in oil reservoirs. Petrol. Sci. Technol. 20, 841–866. doi: 10.1081/lft-120003717
Kovscek, A. R., and Cakici, M. D. (2005). Geologic storage of carbon dioxide and enhanced oil recovery. II. Cooptimization of storage and recovery. Energy Conv. Manag. 46, 1941–1956. doi: 10.1016/j.enconman.2004.09.009
Kuuskraa, V., Ferguson, R., and Van Leeuwen, T. (2009). Storing CO2 and Producing Domestic Crude Oil with Next Generation CO2-EOR Technology, Report DOE/NETL2009/1350 prepared by Advanced Resources International, Inc. for Department of Energy, Pittsburgh, PA: National Energy Technology Laboratory.
Kuuskraa, V. A., Van Leeuwen, T., and Wallace, M. (2011). Improving Domestic Energy Security and Lowering CO2 Emissions with “Next Generation” CO2-Enhanced Oil Recovery (CO2-EOR). Pittsburgh, PA: National Energy Technology Laboratory.
Lake, L. W., Johns, R. T., Rossen, W. R., and Pope, G. A. (2014). Fundamentals of Enhanced Oil Recovery, Richardson, TX: Society of Petroleum Engineers.
Leach, A., Mason, C. F., and van't Veld, K. (2011). Co-optimization of enhanced oil recovery and carbon sequestration. Res. Energy Econ. 33, 893–912. doi: 10.1016/j.reseneeco.2010.11.002
Marston, P. M. (2017). Incidentally speaking: a systematic assessment and comparison of incidental storage of CO2 during EOR with other near-term storage options. Energy Proc. 114, 7422–7430. doi: 10.1016/j.egypro.2017.03.1872
Marston, P. M. (2018). “Regulation of carbon dioxide pipelines: the US experience and a view to the future” in Carbon Capture and Storage: Emerging Legal and Regulatory Issues, 2nd Edn, eds I. Havercroft, R. Macrory, and R. Stewart (Hart Publishing).
Marston, P. M., and Moore, P. A. (2008). From EOR to CCS: the evolving legal and regulatory framework for carbon capture and storage. Energy Law J. 29, 421–490.
Martin, D., Johnson, K., Stolberg, A., Zhang, X., and De Young, C. (2017). Carbon dioxide removal options: A literature review identifying carbon removal potentials and costs (Dissertation/master's thesis). University of Michigan, Ann Arbor, MI, United States.
Massachusetts Institute of Technology (MIT) (2010). “Role of enhanced oil recovery in accelerating the deployment of carbon capture and sequestration,” in MIT Energy Initiative and Bureau of Economic Geology at UT Austin Symposium.
Melzer, S. (2012). Carbon Dioxide Enhanced Oil Recovery (CO2 EOR): Factors Involved in Adding Carbon Capture, Utilization and Storage (CCUS) to Enhanced Oil Recovery. Report for the National Enhanced Oil Recovery Initiative, Center for Climate and Energy Solutions.
Merchand, D. (2017). “Enhanced oil recovery–the history of CO2 conventional WAG injection techniques developed from lab in the 1950s to 2017,” in Carbon Management Technology Conference (Houston, TX).
Middleton, R. S. (2013). A new optimization approach to energy network modeling: anthropogenic CO2 capture coupled with enhanced oil recovery. Int. J. of Energy Res. 37, 1794–1810. doi: 10.1002/er.2993
National Energy Technology Laboratory (NETL) (2010). Carbon Dioxide Enhanced Oil Recovery: Untapped Domestic Energy Supply and Long Term Carbon Storage Solution. Strategic Center for Natural Gas and Oil (SCNGO); National Energy Technology Laboratory. Available online at: https://netl.doe.gov/sites/default/files/netl-file/CO2_EOR_Primer.pdf (accessed March, 2019).
National Energy Technology Laboratory (NETL) (2013). Carbon Dioxide Transport and Storage Costs in NETL Studies: Quality Guidelines for Energy Systems Studies. NETL report.
Núñez-López, V., Gil-Egui, R., and Hosseini, S. A. (2019). Environmental and operational performance of CO2-EOR as a CCUS technology: a Cranfield example with dynamic LCA considerations: Energies 12:15. doi: 10.3390/en12030448
Núñez-López, V., Wood, D. H., Ambrose, W. A., and Hovorka, S. D. (2008). Quick-look assessments to identify optimal CO2-EOR storage sites: Environ. Geol. 54, 1695–1706. doi: 10.1007/s00254-007-0944-y
Primary Enforcement Authority for the Underground Injection Control Program. (n.d.) Retrieved from: https://www.epa.gov/uic/primary-enforcement-authority-underground-injection-control-program (accessed April, 2019).
Stalkup, F.I. Jr. (1984). “Miscible displacement,” in SPE of AIME, Monograph, Vol. 8 (Richardson, TX).
State CO2-EOR Deployment Work Group (2016). Putting the Puzzle Together: State and Federal Policy Drivers for Growing America's Carbon Capture and CO2-EOR Industry. Steyer-Taylor Center for Energy Policy and Finance.
Stewart, J., and Haszeldine, S. (2014). Carbon Accounting for Carbon Dioxide Enhanced Oil Recovery. Scottish Carbon Capture and Storage. Available online at: http://www.sccs.org.uk (accessed April, 2019).
United Nations (2015). Paris Agreement. Available online at: https://unfccc.int/sites/default/files/english_paris_agreement.pdf (accessed April, 2019).
Wallace, M., Goudarzi, L., Callahan, K., and Wallace, R. (2015). A Review of the CO2 Pipeline Infrastructure. U.S. Department of Energy's. Available online at: https://www.energy.gov/sites/prod/files/2015/04/f22/QER%20Analysis%20-%20A%20Review%20of%20the%20CO2%20Pipeline%20Infrastructure%20in%20the%20U.S_0.pdf (accessed March, 2019).
Walsh, M., and Lake, L. W. (1989). Applying fractional flow theory to solvent flooding and chase fluids. J. Petrol. Sci. Eng. 2, 281–303.
Zapantis, A., Consoli, C., and Havercroft, I. (2018). CCS Policy Indicator (CCS-PI). Thought Leadership Report. Global CCS Institute (GCCSI).
Keywords: CO2-EOR, negative emissions, decarbonization, CCS, CCUS, carbon capture, storage
Citation: Núñez-López V and Moskal E (2019) Potential of CO2-EOR for Near-Term Decarbonization. Front. Clim. 1:5. doi: 10.3389/fclim.2019.00005
Received: 03 May 2019; Accepted: 03 September 2019;
Published: 27 September 2019.
Edited by:
Jennifer Wilcox, Worcester Polytechnic Institute, United StatesReviewed by:
Volker Sick, University of Michigan, United StatesLyla Taylor, University of Sheffield, United Kingdom
Copyright © 2019 Núñez-López and Moskal. This is an open-access article distributed under the terms of the Creative Commons Attribution License (CC BY). The use, distribution or reproduction in other forums is permitted, provided the original author(s) and the copyright owner(s) are credited and that the original publication in this journal is cited, in accordance with accepted academic practice. No use, distribution or reproduction is permitted which does not comply with these terms.
*Correspondence: Vanessa Núñez-López, dmFuZXNzYS5udW5lekBiZWcudXRleGFzLmVkdQ==