- Stomatology Center, Gansu Provincial Hospital, Lanzhou, Gansu, China
The oral microbiome comprises over 700 distinct species, forming complex biofilms essential for maintaining oral and systemic health. When the microbial homeostasis in the periodontium is disrupted, pathogens within the biofilm can cause periodontitis and peri-implantitis, inducing host immune responses. Understanding the role of microbial communities and the immune mechanisms in oral health and disease is crucial for developing improved preventive, diagnostic and therapeutic strategies. However, many questions remain about how changes in bacterial populations contribute to the development and progression of these conditions. An electronic and manual literature search was conducted using PubMed, Excerpta Medica, Frontiers Reports and the Wiley Online Library databases for relevant articles. Data from these publications were extracted and the overall findings were summarized in a narrative manner. The variations in microbial communities and immune responses of periodontitis and peri-implantitis are explored. Dysbiosis of the subgingival microbiome—characterized by an increase in pathogenic bacteria such as Porphyromonas gingivalis, Tannerella forsythia, and Aggregatibacter actinomycetemcomitans—plays a pivotal role in the initiation and progression of periodontitis. As for peri-implantitis, alterations include a higher abundance of opportunistic pathogens and reduced microbial diversity around implants. Moreover, oral dysbiosis potentially influencing systemic health through immune-mediated pathways. Regional immunity of periodontium involving neutrophils, T helper cells-17, and immune-related cytokines is crucial for maintaining periodontal homeostasis and responding to microbial imbalances. Additionally, the impact of non-mechanical treatments—such as probiotics and laser therapy—on the oral microbiome is discussed, demonstrating their potential in managing microbial dysbiosis. These findings underscore that bacterial dysbiosis is a central factor in the development of periodontitis and peri-implantitis. Maintaining microbial balance is essential for preventing these diseases, and interventions targeting the microbiome could enhance treatment outcomes. Strategies focusing on controlling pathogenic bacteria, modulating immune responses, and promoting tissue regeneration are key to restoring periodontal stability. Further research is needed to clarify the mechanisms underlying the transition from peri-implant mucositis to peri-implantitis and to optimize prevention and treatment approaches, considering the complex interactions between the microbiome and host immunity.
1 Introduction
The composition of the oral microbiome can vary greatly between individuals and even within the same individual, depending on factors such as age, lifestyle and genetic background (Di Stefano et al., 2022). Typically, hundreds of microbial species coexist in a mutually dependent and harmonious relationship with the host, forming a large commensal. The environment of the oral cavity, including optimal temperature, humidity and the abundant nutrient sources creates ideal conditions for the survival of bacteria, fungi and viruses. Meanwhile, the stable microbial community in the oral cavity plays a crucial role in maintaining the body’s internal homeostasis. For instance, niches in the subgingival regions of the oral cavity provide an ideal habitat for certain anaerobic proteolytic bacteria (Socransky and Haffajee, 2005). The commensal serves as a natural defense barrier against external or opportunistic pathogens. The oral microbiome acquires nutrients from food residues, with their metabolic byproducts absorbed by the host, supporting cardiovascular health and promoting the mineralization of hard tissues (Kapil et al., 2010). Therefore, the significance of the oral microbiota to human health is comparable to that of the gut microbiota. Given the unique ecosystem of the oral cavity, only about half of the microbial species can be cultured in vitro (Aas et al., 2005), and a substantial proportion of the oral microbiome remains uncharacterized, which presents challenges to comprehensive understanding the human microbiome and describing its roles in health and disease.
Data from the Human Microbiome Project (HMP) reveal that the oral microbiome comprises over 700 distinct species. These microorganisms seldom exist in a planktonic state. Instead, they aggregate within various microenvironments, embedded in the extracellular polymeric matrix (EPM) (Bowen et al., 2018), forming biofilm communities, which are highly organized in structure and function (Arweiler and Netuschil, 2016). The EPM supplies essential substances such as water, polysaccharides, proteins, lipids and DNA, which support microbial colonization and stabilize the microbial community. Moreover, EPM facilitates the adherence of the biofilm to host tissues, enhancing both interspecies and intraspecies interactions while providing protection from host immune defenses and antimicrobial agents (Cugini et al., 2019). Gram-positive and Gram-negative bacteria within oral biofilms exchange information via quorum sensing, a mechanism through which bacteria secrete diffusible signaling molecules. This process coordinates a range of physiological and pathological activities for the microbiome, such as making biofilm formation and growth, adapting to environmental changes in oral cavity, competing for superiority against potential rivals, and expressing virulence factors that enable pathogens to cause disease (Shao and Demuth, 2010). These biofilms could proliferate on the mineralized surfaces of dental enamel, leading to periodontitis; or adhere to the surfaces of titanium implants, contributing to both peri-implant mucositis and peri-implantitis. Alterations in the oral microbiota are evident in various diseases, impacting both host metabolism and immune responses. As microbial ecological balance is disrupted, pathogenic bacteria outcompete commensal species and result in a range of oral diseases, including dental caries, periodontitis, oral candidiasis and potentially more severe infections, which are even associated with herpes simplex virus co-infection. The complex anatomical and structural organization of periodontal tissues makes them well-suited for microbial colonization.
Periodontitis and peri-implantitis are infectious diseases that are both initiated by pathogenic microorganisms in dental plaque and perpetuated by multiple factors, including the host immune response (Papapanou et al., 2018). Dysbiosis of the subgingival microbiome along with the emergence of periodontitis-associated pathogens plays a pivotal role in the initiation and progression of periodontitis (Mombelli, 2018). Currently, 16S Ribosomal RNA (16S rRNA) sequencing, metagenomics, and metabolomics are widely used to analyze the biological information of microbial communities (Belibasakis and Manoil, 2021), and over 400 bacterial species have been identified in subgingival plaque using these culture-independent techniques (Di Stefano et al., 2022). Bacterial balance and host immune homeostasis are essential for maintaining the health of periodontal and peri-implant tissues. Alterations in the composition and function of the oral microbiome can change symbiotic interactions between microbial communities and the host, ultimately impacting both oral and systemic health. Similarly, the peri-implant environment shapes the resident microbiota. Understanding the ecological triggers of microbial pathogenicity is crucial for developing improved preventive, diagnostic and therapeutic strategies. However, many questions remain to be explored. Therefore, this review focuses on the role of microbial communities in oral health, the relationship between the microbiome and the health of periodontal and peri-implant tissues, and provids a comprehensive overview of how bacterial population changes contribute to the development and progression of periodontitis and peri-implantitis.
2 Methods
Electronic and manual search was conducted for each of the addressed subjects. The PubMed database of the US National Library of Medicine, the Excerpta Medica database of Elsevier, and the Wiley online library were screened for relevant articles (i.e. consensus reports, reviews, experimental studies in animals and humans/observational studies, randomized/controlled clinical studies, meta‐analyses). Data from publications were extracted and overall findings were summarized in a narrative manner.
3 Observations and discussion
3.1 Colonization changes of subgingival microbiota
In healthy periodontal tissues, a wide variety of oral microorganisms coexist to collectively maintain microenvironmental homeostasis. Approximately ten predominant genera contribute to this balance, each fulfilling distinct roles (Roberts and Darveau, 2015). Streptococcus acts as a pioneer species in biofilm formation, initially adhering to teeth surfaces by producing extracellular polysaccharides via glycosyltransferases, which in turn enable the attachment of other microorganisms. Through the production of organic acids, such as lactic acid, Streptococcus helps regulate the local pH environment (Boisen et al., 2021). Additionally, it produces antimicrobial substances like hydrogen peroxide, which inhibit pathogenic bacteria, including cariogenic and periodontal pathogens (Sumioka et al., 2017; Baty et al., 2022). Beyond these functions, Streptococcus also interacts with the host immune system to strengthen mucosal barrier integrity and prevent the overactivation of inflammatory responses (Ingendoh-Tsakmakidis et al., 2020). Veillonella acts as a secondary colonizer, establishing a mutualistic relationship with Streptococcus. By metabolizing lactic acid produced by Streptococcus as the carbon source, Veillonella supports other microbial species within the biofilm, helps regulate local acidity, and indirectly protects tooth enamel from erosion (Violant et al., 2014; Zhang and Huang, 2023). Actinomyces works in synergy with Streptococcus to establish a mature biofilm structure. By degrading complex carbohydrates, it produces acidic metabolites that other microbial species utilize (Liy et al., 2008). However, Actinomyces displays a dual nature: it helps maintain ecological balance under healthy conditions but, when overabundant, can contribute to root caries or chronic periodontitis (Meuric et al., 2017; Del Pilar Angarita-Díaz et al., 2024). Corynebacterium serves as a structural backbone in mature biofilms, providing physical attachment points for other microbial species (Rogers et al., 2011). Under healthy conditions, it inhibits the overgrowth of pathogenic bacteria (Meuric et al., 2017). Rothia, recognized as a marker of oral health, is strongly linked to a balanced and healthy oral environment through its special nitrate reduction effect. It contributes to the breakdown of carbohydrates and proteins, supporting the metabolic exchange within microbial communities. Moreover, it may interact with the host immune system to suppress overactive inflammatory responses (Fatahi-Bafghi, 2021; Mazurel et al., 2023). Neisseria plays a role in the initial formation of periodontal biofilms. It also converts salivary nitrate into nitrite, contributing to the host’s redox homeostasis and vascular function modulation (Pignatelli et al., 2020; Rosier et al., 2022). In a healthy oral environment, it supports the ecological stability of the microbial community. Capnocytophaga attaches to the tooth surface by producing extracellular polysaccharides, enhancing the stability of biofilms. It interacts with host immune cells, potentially modulating local inflammation through the secretion of regulatory factors (Bolton et al., 1985; Idate et al., 2020). This bacterium exhibits a dual nature: at low abundance, it contributes to maintaining a healthy microbial balance, while at high abundance, it is often associated with periodontal disease (Ciantar et al., 2005). Haemophilus works synergistically with Streptococcus and Actinomyces to maintain the stability of the oral ecosystem (Del Pilar Angarita-Díaz et al., 2024). Fusobacterium acts as a “bridge” species within the biofilm, linking early colonizers and late-stage pathogenic bacteria by facilitating interspecies connections (Horiuchi et al., 2020). It plays a role in modulating host immune responses, thereby promoting the dynamic equilibrium of the biofilm. Moreover, Fusobacterium contributes to the degradation of complex organic compounds, supporting the coexistence of various microbial communities (Li et al., 2020). Prevotella breaks down complex carbohydrates and releases organic acids that serve as an energy source for other microbial species (Gellman et al., 2023). This genus also demonstrates a dual nature: at low abundance, it contributes to maintaining a healthy microbial ecosystem, but at higher abundance, it is frequently linked to the development of periodontal diseases (Sharma et al., 2022).
The stable ecological environment of the gingival sulcus can be disrupted by changes in the host or microbiome. The salivary flow redistributes the various cytokines and immunoglobulins produced by the immunocytes, and then will influence microbial colonization. The proliferation of certain bacteria, such as Porphyromonas gingivalis, could modify the nutrient environment at the colonization site, disrupting the symbiotic balance between the microbiota and host (Hajishengallis, 2014). Additionally, regulation of bacteriophage impacts the composition and abundance of microbial communities (Wang et al., 2013). Consequently, host-driven species-specific inhibition and microbial competition will contribute to potential pathogen colonization and enhance the pathogenicity of the microbiome.
As a foreign object, the implant creates an initial pocket after placement, which rapidly attracts the attachment of the salivary pellicle to its surface. Within 30 minutes, the adhesion and colonization of early colonizers can be observed in the implant sulcus, and over the next two weeks, the early microbes develop into an organized biofilm community within the peri-implant space (Belibasakis et al., 2015), offering surface receptors for the attachment and proliferation of late colonizers (Kolenbrander et al., 2010). The most common taxonomic groups found in the subgingival plaque samples from dental implants at 4 weeks were Streptococcus (20%), Fusobacterium (16%), Neisseria (12%) and Prevotella (9%), while the most common taxa in the subgingival plaque samples from dental implants changed at 12 weeks were Streptococcus (22%), Fusobacterium (16%), Neisseria (8%) and Rothia (7%). Meanwhile, within the subgingival plaque samples from control teeth at 4 weeks, the most common taxonomic groups were Streptococcus (22%), Fusobacterium (17%), Neisseria (7%) and Prevotella (6%), while Fusobacterium (20%), Streptococcus (18%), Prevotella (8%) and Veillonella (6%) were more common at 12 weeks. Initially, periodontal bacteria such as Corynebacterium, Actinomyces, Selenomonas, and Campylobacter are difficult to detect around the implant, but their abundance steadily rises after the 12th week of post-implantation (Dabdoub et al., 2013; Schaumann et al., 2014). In addition, in the fourth week, the expression of granulocyte-macrophage colony-stimulating factor (GM-CSF) was significantly increased in both gingival crevicular fluid (GCF) and peri-implant crevicular fluid (PICF), not only that, higher interleukin-10 (IL-10) levels were detected in samples from failed implants during this period (Payne et al., 2017). The microscopic evidence indicates that the 4th to 12th week post-implantation is a critical risk period for implant failure.
3.2 Alterations of the oral microbiota in periodontitis
Periodontal disease has emerged as a major public health concern that affects an estimated 20% to 50% of the global population, with its prevalence anticipated to rise in the coming years. Microorganisms play a pivotal role in regulating the acid-base balance within the oral cavity (Struzycka, 2014; Gupta et al., 2024). When compared to healthy controls, patients with chronic gingivitis display a more alkaline salivary pH, whereas those with chronic periodontitis tend to have a more acidic pH, indicating that pH variation in the oral cavity probably depends on the severity of periodontal inflammation (Baliga et al., 2013). This view leads to changes in the characterization of microbial diversity and alterations in the composition of the microbiota. In the healthy oral cavity, the predominant microbial species include Streptococcus, Prevotella, Haemophilus, Fusobacterium, and Veillonella. The composition and abundance of these microorganisms vary significantly across distinct anatomical regions, including saliva, buccal mucosa, hard palate, palatine tonsils, subgingival or supragingival areas, pharynx and dorsal surface of the tongue (Maruyama et al., 2014). In healthy periodontal tissues, the predominant microbial community consists of Gram-positive facultative anaerobes, such as Streptococcus and Actinomyces. Among them, Proteobacteria are especially abundant, forming symbiotic associations with several species within the Firmicutes phylum. These bacteria do not typically cause inflammation but rather exist in a symbiotic relationship with the host, and they are essential for maintaining oral health through producing antimicrobial substances and competing resources to inhibit pathogenic colonization (Jung and Lee, 2023). However, when the healthy microecological balance is disrupted, pathogenic germs proliferate freely, thereby triggering and worsening inflammation and tissue damage (Chen et al., 2022b; Gasmi Benahmed et al., 2022) (Figure 1) (Table 1). In diseased periodontal pockets, bacteria such as Treponema, Porphyromonas, Fusobacterium and Prevotella are highly abundant, and the number of bacteria such as Fusobacterium and Selenomonas increases with the periodontal pockets deepened (Gonçalves et al., 2012).
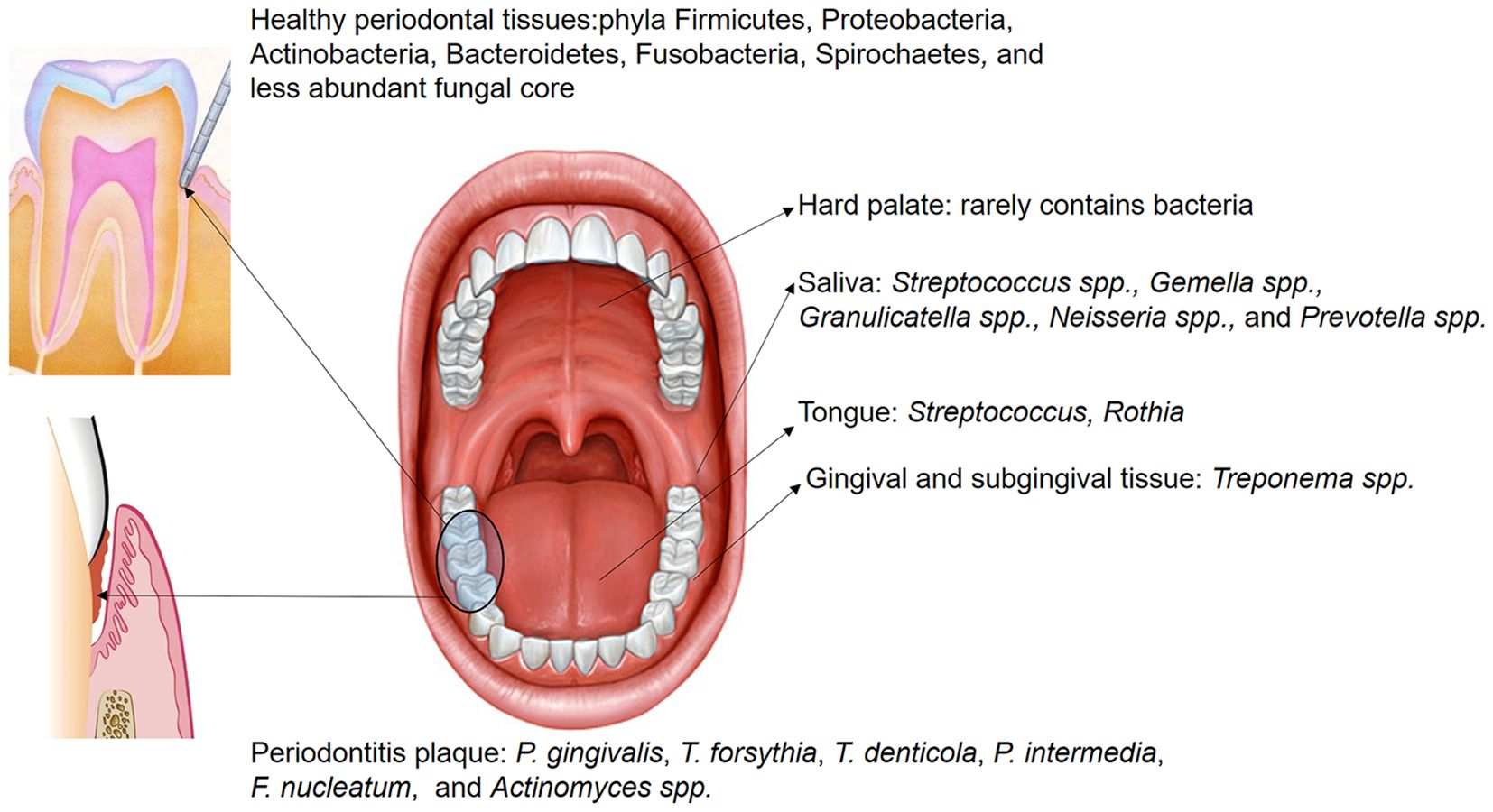
Figure 1. The distribution of microbiota within different oral regions exhibits overlap across sites; however, certain bacteria are predominantly localized in specific areas. Saliva hosts numerous non-pathogenic microorganisms, including Streptococcus spp., Gemella spp., Granulicatella spp., Neisseria spp., and Prevotella spp. In the healthy gingival sulcus, the dominant phyla are Firmicutes, Proteobacteria, Actinobacteria, Bacteroidetes, Fusobacteria, Spirochaetes, and a smaller core of fungal species. Conversely, in subgingival plaque of periodontitis, the abundance of pathogenic species, such as P. gingivalis, T. forsythia, T. denticola, P. intermedia, F. nucleatum, and Actinomyces spp., is significantly increased.
Smoking is one of the most significant risk factors associated with the onset and progression of periodontal tissue inflammation. It impairs neutrophil chemotaxis and phagocytic function, disrupts humoral immunity, alters the expression levels of serum Immunoglobulin (Ig) G and IgE, increases the production of reactive oxygen species (ROS) and the release of proteases like collagenase and elastase, which directly contribute to periodontal tissue destruction (Huang and Shi, 2019). Research showed that smoking-induced subgingival dysbiosis occurs before clinical symptoms of periodontal disease (Tamashiro et al., 2023). Chemicals in tobacco such as nicotine and benzo[a]pyrene exhibit direct antimicrobial effects upon contact with microbial communities, leading to non-specific bactericidal effects that reduce normal microbiota and immune cell populations, creating opportunities for pathogenic bacteria to proliferate (McEachern et al., 2015). Furthermore, tobacco smoke and its chemicals significantly affect oxygen levels of local tissue and immunoglobulin concentrations of salivary (Tarbiah et al., 2019; Rinaldi et al., 2023), indirectly promoting changes in the microbial environment by altering habitats or disrupting host immunity and the biofilm formation process. Reduced immune function including diminished activity of oral neutrophils and macrophages, coupled with systemic chronic inflammation impairs the body’s microbial clearance, facilitating the colonization and growth of pathogenic bacteria. Smoking cessation remains the most effective method for prevention and treatment; however, the chronic inflammation caused by microbial imbalance is often difficult to reverse and may even be irreversible (Domagala-Kulawik, 2008).
Local irritants play a crucial role in shaping the microbiota within periodontal tissues. Despite significant progress in dental restorative materials and prosthetics over the past few decades, restoration failure rates continue to pose a challenge for both clinicians and researchers. Alterations in surface integrity, ion release and dissolution from dental fillings may promote bacterial adhesion, resulting in rougher restoration surfaces that influence biofilm formation and development (Yoshihara et al., 2017). Compared to smooth surfaces, rough surfaces provide more irregular areas, many of which are anaerobic regions and create an ideal environment for microbial colonization, facilitating the formation and maturation of oral plaque (Di Stefano et al., 2022). Additionally, deeper and larger depressions on rough surfaces increase the contact area, providing a more favorable interface for bacterial colonization and biofilm formation. These areas protect germs from shear forces, such as rinsing and brushing, during the initial reversible attachment phase, allowing for stronger and irreversible adhesion (Song et al., 2015). As a result, microbial colonies on rough restorative surfaces are more difficult to eliminate, leading to the formation of mature biofilms. On the other hand, dental restorative materials in the oral cavity are vulnerable to bacterial corrosion. Plaque can degrade the components of these materials, potentially compromising marginal integrity, promoting the development and progression of secondary caries, periodontitis and peri-implantitis (Li et al., 2014). The chemical properties of restorative materials can also affect microbial behavior. For instance, fluoride released from glass ionomer cement (GIC) reduces acid production, inhibits acid resistance and diminishes extracellular polymeric substance (EPS) formation in the plaque, particularly in cariogenic biofilms such as those formed by Streptococcus mutans. Fluoride also inhibits bacterial exopolysaccharide formation, preventing the increase of cariogenic bacteria, thereby suppressing the formation of cariogenic-dominant biofilms (Jung et al., 2016). Some polymeric components in composite resins have demonstrated inhibitory effects on biofilm formation (Ionescu et al., 2012); however, shortening the light-curing time could significantly increase the number of unpolymerized monomers on the material’s surface, which may contribute to increased colonization of S. mutans on composite resin surfaces (Brambilla et al., 2009).
Various stimuli ultimately result in the dominance of pathogenic bacteria. Porphyromonas gingivalis, one of the primary pathogens responsible for periodontitis, is known as a “keystone pathogen” or “ecopathogen”, signifying its critical role in initiating microbial dysbiosis during the disease’s progression (Gasmi Benahmed et al., 2022). Through the complex pathogenic mechanisms, P. gingivalis disrupts the balance of the entire microbial community and leads to the synergistic growth of other pathogens, primarily Tannerella forsythia and Spirochetes, which further intensify inflammation and tissue destruction (Mysak et al., 2014). Unlike other Gram-negative bacteria, the lipopolysaccharides (LPS) from P. gingivalis has a unique structure that activates Toll-like receptors (TLRs) in vivo (Jia et al., 2019), particularly TLR2 and TLR4. The activated TLR2 interacts with the capsular polysaccharide on the surface of P. gingivalis, resulting in bidirectional regulation of the immune response. This interaction combined with fimbriae and outer membrane vesicles enables P. gingivalis to evade host immune surveillance while inducing a fierce inflammatory response (Bostanci and Belibasakis, 2012; Lunar Silva and Cascales, 2021; Reyes, 2021; Zheng et al., 2021). Moreover, gingipains produced by P. gingivalis excessively activate the secretion of pro-inflammatory cytokines—primarily IL-1β, IL-6 and tumor necrosis factor-α (TNF-α). These chymotrypsin-like proteases, including Arginine-specific Gingipain and Lysine-specific Gingipain, also degrade defense proteins of parasitifer, such as immunoglobulins and complement, inhibiting the local immune response and facilitating periodontal tissue destruction (Bengtsson et al., 2015; Kadowaki, 2021). P. gingivalis is not confined to local effects in the oral cavity, it enters systemic circulation via bloodstream and contributes to the development and progression of systemic diseases by inducing systemic inflammation and abnormal immune activation (Klarström Engström et al., 2015).
T. forsythia forms the “Red Complex” in collaborates with Treponema denticola and P. gingivalis, colonizing and thriving in the anaerobic environment of periodontal pockets, and mutually contributing to the destruction of periodontal tissues (Suzuki et al., 2013). The Red Complex establishes a structured multispecies biofilm, which plays a key role in the progression of chronic periodontitis. This biofilm not only protects the bacteria from systemic immune responses but also enhances their resistance to antimicrobial treatments (Ong et al., 2017). Additionally, T. forsythia exacerbates inflammation through protease secretion and immune evasion. LPS on the cell wall of T. forsythia directly stimulates immune cells and lead to the secretion of pro-inflammatory cytokines such as IL-1, IL-6 and TNF-α (Pani et al., 2021). During active periods of periodontitis, the interaction between T. forsythia and P. gingivalis becomes more frequent, amplifying the inflammatory response. Studies indicated that all the rabbits co-infected with P. gingivalis and T. forsythia developed abscesses, while rabbits infected with either bacterium alone did not develop abscesses. Co-culture experiments results showed that T. forsythia exhibited a faster growth rate, suggesting a symbiotic nutritional relationship between the bacterial species (Verma et al., 2010).
Aggregatibacter actinomycetemcomitans is a Gram-negative facultative anaerobic bacillus, which is primarily found in the late stages of periodontitis or localized aggressive periodontitis (LAP) (Gholizadeh et al., 2017). This occurrence may be associated with early microbial colonization influenced by genetic factors (Åberg et al., 2015), and it is also considered a risk marker for the progression of periodontal attachment loss (Fine et al., 2007). The fimbriae and various adhesins produced by A. actinomycetemcomitans have been shown to be important for its proliferation in different ecological niches within the human oral cavity (Fine et al., 2010). After gaining the competitive advantage, A. actinomycetemcomitans primarily releases the leukotoxin LtxA to disrupt the anti-inflammatory function of leukocytes. The variability in virulence among different strains of this bacterium is related to gene expression, indicating that LtxA expression is regulated by both environmental and genetic factors (Johansson, 2011). Currently there are seven serotypes of A. actinomycetemcomitans (a–g) that have been identified based on the immunodominant antigen, which is an O-polysaccharide of the LPS. The JP2 genotype of A. actinomycetemcomitans harbors a 530-bp deletion in the promoter region of the leukotoxin operon, which is highly leukotoxic and has stronger pathogenic potential (Åberg et al., 2015). Macrophages are one of the primary producers of pro-inflammatory cytokines such as IL-1 and TNF. Studies have demonstrated that monocytes and macrophages produce pro-inflammatory cytokines, including TNF-α and IL-1β, in response to metabolic products of A. actinomycetemcomitans (Henderson et al., 2010). Macrophages exhibit heightened sensitivity to the pro-inflammatory response induced by LtxA, which specifically activates caspase-1, resulting in excessive secretion of IL-1β (Kelk et al., 2003, 2005); moreover, increased expression of inflammasome components could also be detected in co-cultures with A. actinomycetemcomitans (Belibasakis and Johansson, 2012). What’s more, LPS present in various pathogenic bacteria enhance pro-IL-1β expression in macrophages, which, along with inflammasomes and caspase-1, induces secondary stimulation of macrophages, leading to more IL-1β secretion and promoting periodontal tissue loss (Kelk et al., 2005, 2011). In contrast, significantly lower levels of IL-1 are often detected in the GCF of patients during the recovery period of periodontal treatment (Holmlund et al., 2004). The diversity of LtxA expression suggests that this toxin plays a significant role in the pathogenesis of aggressive periodontitis. Compared with patients with chronic periodontitis, adolescents (average age of 15 years) harboring highly leukotoxic strains of A. actinomycetemcomitans (both JP2 and non-JP2 genotypes) are at higher risk for localized periodontal attachment loss.
The ultimate consequence of uncontrolled periodontal tissue infection is alveolar bone loss. The primary objective of periodontal therapy is to prevent further disease progression, alleviate symptoms, reduce the risk of tooth loss, and explore the possibility of regenerating damaged periodontal tissue. In terms of etiological treatment, controlling and eliminating plaque-retentive factors is essential for reducing deep pocket inflammation and improving clinical attachment levels (CAL). In addition to full-oral mechanical debridement, the use of adjunctive chemical agents in combination with local and systemic antimicrobials is required to eliminate deep biofilms effectively in the subgingival area (Sanz et al., 2020). However, inappropriate medicines and treatment with chemical reagents can lead to oral microbiome dysbiosis. For example, chlorhexidine-containing medications may further reduce the pH of the acidic oral environment in periodontitis patients, which thus more suitable for treating gingivitis (Bescos et al., 2020). Beyond periodontal infections, dysbiosis can also cause oral mucosal ulcers, erosions and sensory abnormalities, while excessive use of antimicrobials may result in salivary glands inflammation, nerve numbness and allergic reactions (Herrera et al., 2002). Therefore, while eliminating the pathogenic bacteria, clinicians must consider systemic side effects, microbiological adverse reactions, and the significant risk of developing or exacerbating antibiotic resistance—all of which pose challenges for future periodontal therapy.
3.3 Alterations of the oral microbiota in peri-implant inflammation
Dental implants achieve long-term stability primarily through osseointegration. It represents a revolutionary innovation in the field of dentistry, aimed at replacing missing teeth and restoring masticatory, occlusal and aesthetic functions. Compared with traditional restorative methods, implant-based rehabilitation is considered a safer and more effective treatment option. The success rate of implant restoration is directly associated with advancements in implant surface process, design, materials and techniques (Alves et al., 2022). However, the incidence of implant-related complications has been increasing yearly, and the loss of hard and soft tissues around implants is influenced by multiple factors. For example, patients with a history of chronic or aggressive periodontitis are at a higher risk of implant failure (Sgolastra et al., 2015), while genetic polymorphisms may also increase the likelihood of developing peri-implantitis (Cardoso et al., 2024); additionally, poor oral hygiene is considered one more potential contributing factor to peri-implantitis (Ball and Darby, 2022), and smoking can also cause peri-implantitis as well as periodontitis.
The microbial composition of healthy peri-implant and periodontal sites overlap, but the proportions differ. The compositions of the biofilm around dental implants are similar to that surrounding adjacent natural teeth, making the teeth’s microbiome a “reservoir” for peri-implant biofilm (Belibasakis, 2014). However, there are also notable differences. Due to the direct integration of the implant with the alveolar bone, biofilms consisting of fewer species on healthy implants tend to have lower density and a simpler structure. These biofilms predominantly contain Gram-positive facultative anaerobic cocci and rods, also including Actinomyces, Veillonella, as well as low levels of Clostridia and Bacteroides (Hultin et al., 2002; Canullo et al., 2015; Jung and Lee, 2023). Studies have shown that healthy peri-implant tissues are primarily colonized by bacteria from the phyla Firmicutes and Proteobacteria. The Streptococcus and Neisseria species are particularly abundant, accounting for over 40% of the microbiota. Additionally, Staphylococcus, Candida and Veillonella are present in lower proportions in conditions simulating implant health (Sousa et al., 2022). This situation stands in stark contrast to the biofilms in peri-implantitis, which are denser, more diverse, and harbor more pathogenic species.
The risk factors and indicators for bone breakdown around implants and natural teeth share some similarities. Peri-implant infections can be categorized into peri-implant mucositis and peri-implantitis. Peri-implant mucositis is diagnosed if bleeding on probing (BOP) or suppuration is detected without radiographic evidence of alveolar bone loss exceeding initial remodeling. Similar to gingivitis around natural teeth, peri-implant mucositis shows a well-defined inflammatory infiltrate, primarily composed of vascular structures, plasma cells and lymphocytes located lateral to the junctional epithelium, without extending into the connective tissue of the alveolar crest (Araujo and Lindhe, 2018; Berglundh et al., 2018). If detected early and treated properly, peri-implant mucositis is regarded as a reversible condition. However, if not managed effectively, peri-implant mucositis can progress, causing bone resorption exceeding 2 mm from the initial remodeling and an increase in probing depth (PD) beyond 6 mm (Berglundh et al., 2018). At this stage, it is classified as peri-implantitis and the damage to surrounding tissues induces an inflammatory response mediated by the activation of innate immune cells such as macrophages, dendritic cells, mast cells and neutrophils. The release of pro-inflammatory cytokines like IL-1 and TNF-α is mainly promoted by neutrophils, extending the inflammation into the supporting bone, leading to both osteolytic and inflammatory tissue damage (Baseri et al., 2020), indicating that the inflammation has advanced to involve the surrounding alveolar bone.
Peri-implant infections are also regarded as polymicrobial diseases, with multispecies biofilms and their byproducts or metabolites as the primary drivers of the inflammatory response, commonly referred to as “peri-implantitis” (Lindhe and Meyle, 2008). Fundamentally, peri-implantitis is an endogenous mixed infection that occasionally involves atypical oral bacteria. This implies that external factors may also contribute to the infection, although defining the extent of this contribution remains difficult (Kumar, 2019). In long term follow-up studies, peri-implantitis was observed in 28-56% of implant recipients and different degrees of infections were detected in 12-43% of implant sites (Roos-Jansåker et al., 2006; Zitzmann and Berglundh, 2008). Among these cases, approximately 12.4% of peri-implant diseases were directly associated with dental plaque, 17.1% were related to surgical factors, 32.4% were linked to prosthetic factors and 13.3% were due to biomechanical factors, with about 25% involving a combination of these factors (Canullo et al., 2017). It is suggested that the causes of peri-implant diseases are complex, involving not only patient-specific conditions but also the risk of bacterial infection during surgery or prosthetic restoration. Research has shown that patients with a history of periodontitis are more susceptible to peri-implant diseases, with bacteria potentially migrating from the periodontal tissues of natural teeth to the surrounding implant area (Cho-Yan Lee et al., 2012; Pjetursson et al., 2012). The unique structure of implants causes inflammation to progress more rapidly. Oral microbiome dysbiosis accelerating the production of cytokines, chemokines, prostaglandins and proteases, further exacerbating tissue destruction and broadening the extent of the lesions (Komatsu et al., 2020; Giro et al., 2021). A research found that implants in the posterior region are six times more likely to develop inflammation than those in the anterior region, with the majority of periodontal pathogens, especially the red complex, being present around implants (Albertini et al., 2015). As inflammation progresses, it can lead to implant loss, maxillary sinusitis (Park et al., 2019), jaw fractures, and other complications including infections in adjacent implants or natural teeth (O’Sullivan et al., 2006). Furthermore, prolonged exposure of the implant’s metal surface to biofilm or physiological friction at the implant-abutment interface can cause corrosion and wear of the implant, releasing titanium ions, microparticles, and even nanoparticles that may affect surrounding tissues and enhance macrophage-mediated inflammation (Apaza-Bedoya et al., 2017; Pettersson et al., 2017). This microbial-induced biocorrosion of the implant surface could exacerbate titanium particle release and bio-implant neopathy (Mombelli et al., 2018), ultimately resulting in pathological bone resorption. Apatzidou et al. (Apatzidou et al., 2017) used 16S rRNA sequencing to analyze the microbial composition of peri-implantitis sites and non-adjacent healthy periodontal sites in patients undergoing periodontal treatment. Their results indicated that healthy periodontal sites had greater microbial diversity, associated with higher levels of Actinomyces and Streptococcus. In contrast, Prevotella and Porphyromonas were closely linked to the severity of peri-implantitis.
Studies have shown that in early-stage peri-implant mucositis, Escherichia coli still accounts for nearly 50% of the bacterial population in contrast to the microbiota around healthy implants, where the proportion of Neisseria meningitidis (HMT669) significantly decreases, and pathogenic bacteria such as Porphyromonas and Treponema remain at low levels (Sousa et al., 2022; Feng et al., 2024). While Peptostreptococcus micros is typically present in low abundance around healthy implants, it becomes enriched in peri-implantitis sites (Jung and Lee, 2023). Additionally, several less pathogenic bacterial species have been detected at higher levels in peri-implantitis, including Alloprevotella sp. (HMT473), Enterobacteriaceae spp., Parvimonas micra (HMT111), Peptostreptococcus stomatis (HMT112), Gemella morbillorum (HMT046), Prevotella saccharolytica (HMT781), Pasteurellaceae spp., Parvimonas spp., and Klebsiella pneumoniae (HMT731). The anaerobic bacterial around implants usually does not occur in the initial stages of inflammation but begin to increase after two weeks and reach higher levels by the third week. At the same time, the abundance of Proteobacteria decreases significantly (Sousa et al., 2022), suggesting that the progression has already evolve from mucositis to peri-implantitis, along with a significant change in bacterial composition. As the inflammation continues to progress, levels of Leptotrichia, Propionibacterium and Prevotella decrease, while Actinomyces, Peptococcus, Campylobacter, non-mutans Streptococcus, Butyrivibrio and S. mutans are found at elevated levels compared to healthy implants (Kumar et al., 2012). Another study found that T. forsythia was commonly detected among peri-implantitis patients, with 24% of patients testing positive for Prevotella intermedia, and 39% of patients testing positive for T. denticola of cases. Moreover, mixed bacterial infections were detected at inflamed peri-implant sites, such as P. gingivalis + T. forsythia (33%) and T. forsythia + T. denticola (24%). Notably, A. actinomycetemcomitans was not found in any peri-implantitis patients in this study (Albertini et al., 2015), suggesting that titanium implants may influence the colonization of periodontal pathogens and even change the microbial diversity of surrounding tissues. In addition to anaerobic periodontal bacteria, some rare oral microorganisms such as Pseudomonas aeruginosa and Staphylococcus aureus, exhibit higher adhesion to titanium surfaces and could even cause early postoperative infections likely due to the multidrug resistance of those bacteria (Truong et al., 2010; Cobo et al., 2011; D’Ovidio et al., 2011; Sendra et al., 2024). Interestingly, the use of certain low-concentration oral disinfectants in early postoperative open wounds can promote the growth of these bacteria, particularly P. aeruginosa (Morales-Fernández et al., 2014). Some fungi can also colonize the peri-implant pocket. This situation emphasizes the need for comprehensive antimicrobial strategies to protect the delicate microbial environment around implants. For instance, Candida albicans can adhere to and colonize mucosal tissues or inert materials, causing not only inflammatory damage to surrounding tissues but also forming complex biofilms with other pathogens. These biofilms worsen the persistence and severity of infections, making treatment more challenging (Salerno et al., 2011). Additionally, viruses such as human cytomegalovirus, human herpesvirus types 4 and 5, and Epstein–Barr virus type 1 as part of the biofilm complex have been identified in peri-implant inflammatory tissues. However, there is currently no definitive evidence proving their direct association with the etiology of inflammation (Pérez-Chaparro et al., 2016).
3.4 The correlation between oral microbiota and intestinal microbiota
Given the direct connection between the oral cavity and the gastrointestinal tract, the oral microbiota unavoidably interacts with and influences the gastrointestinal microbiota. But research on the influence of gut microbiota on the oral microbiota remains limited. The gut microbiota as the largest microbial community in the human body, is primarily composed of Firmicutes, Bacteroidetes, Actinobacteria and Proteobacteria. Firmicutes are Gram-positive bacteria with over 200 genera constituting 60%–80% of the community, the most important of which include Ruminococcus, Clostridium, Eubacterium, Lactobacillus, Faecalibacterium, Roseburia and Mycoplasma; Bacteroidetes are primarily Gram-negative including the genera Bacteroides, Prevotella and Xylanibacter, comprising 20%–30% of the microbiota; Actinobacteria are Gram-positive represent less than 10% and notably include Bifidobacterium; Proteobacteria are Gram-negative make up less than 1% of the microbiota and consist of genera such as Escherichia, Desulfovibrio and Enterobacter; the phylum Verrucomicrobia occupies an even smaller proportion of the gut microbiota, represented by genera like Akkermansia, Fusobacterium and Cyanobacteria (Bao et al., 2022).
According to a relevant report, the oral microbiota of mice in the early stage of diabetes resembles that of healthy mice. However, with rising blood glucose levels, the pathogenic potential of the oral microbiota increases, evidenced by significant alteration in β-diversity and a marked increase in periodontitis-related bacteria (Xiao et al., 2017). In addition, patients with inflammatory bowel disease (IBD) are frequently observed to concurrently suffer from chronic periodontitis, as both diseases involve inflammation closely related to host immune responses and microbial dysbiosis (Muhvić-Urek et al., 2016; de Mello-Neto et al., 2021). Studies indicate a higher detection rate of Fusobacterium nucleatum in the colons of IBD patients and the strains isolated from inflamed tissue shows greater invasiveness compared with those from healthy control tissue (Strauss et al., 2011). In addition, compared with control subjects, IBD patients have lower IL-4 expression levels in GCF and higher IL-18 levels in serum. Severe IBD is often associated with worse alveolar bone resorption, likely due to reduced IL-4 in GCF limiting the inhibition of osteoclast activity (Figueredo et al., 2017). Therefore, it can be inferred that cytokines resulting from gut dysbiosis are related to systemic inflammation and probably impact the development and progression of chronic periodontitis (Figure 2). On the other hand, the imbalance of oral microbiota also affects the microbial homeostasis throughout the body system. Under physiological conditions, stomach acid and bile generally inhibit the migration of oral microbiota to the gastrointestinal tract, but in patients with periodontitis or peri-implantitis, certain acid-resistant bacteria in the oral cavity could withstand H+ (the low pH) conditions and protease activity, potentially increasing the systemic spread of these pathogens (Liu et al., 2021). In animal models with periodontitis, genomic DNA of F. nucleatum has been detected in various systemic organs including the blood, heart, lungs and liver. Accordingly, some researchers have proposed the “oral-gut-liver axis” hypothesis, suggesting that periodontopathic microorganisms might infiltrate the systemic circulation via damaged gingival pocket epithelium or collagen fibers and matrix within adjacent connective tissue. This infiltration could lead to bacteremia or endotoxemia, enabling pathogenic bacteria to spread and colonization in various organs throughout the body (Acharya et al., 2017).
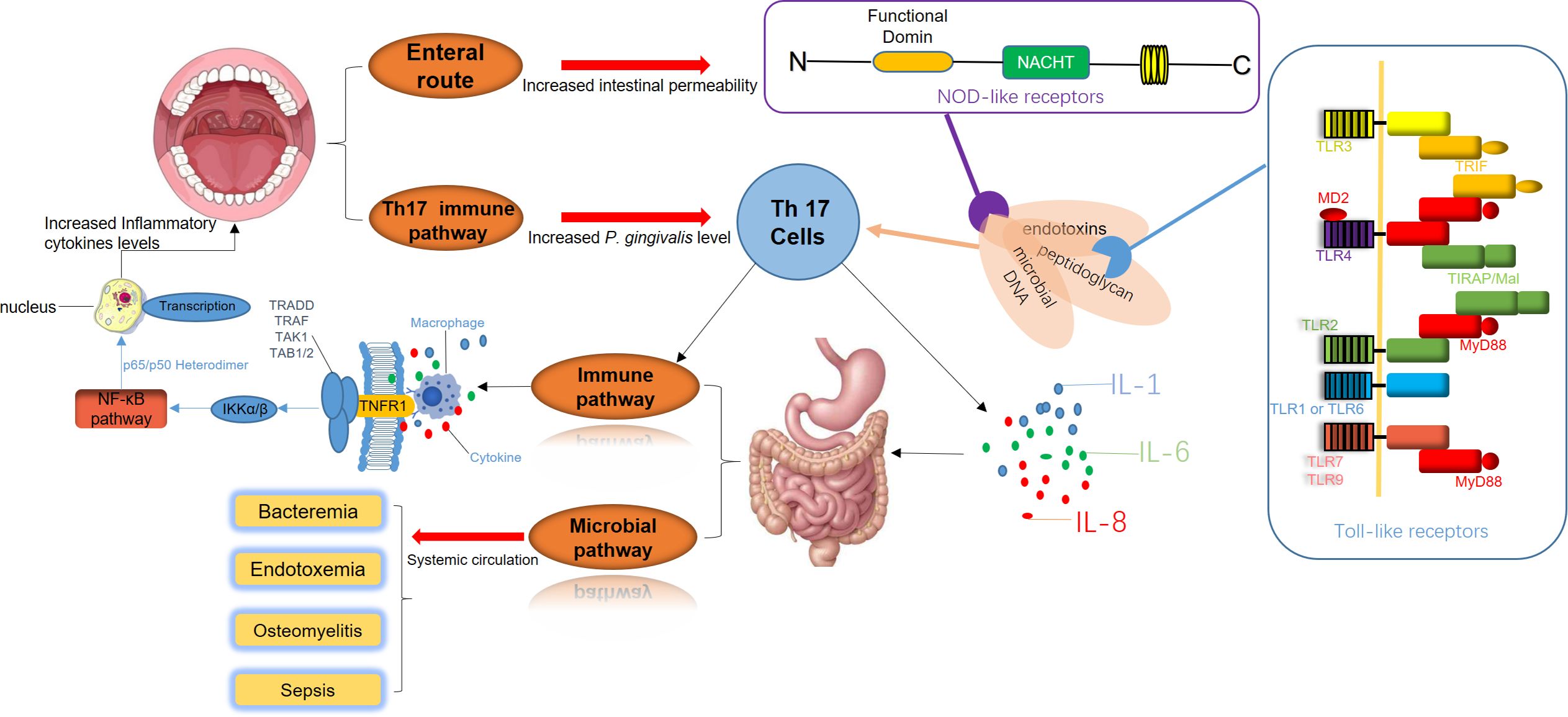
Figure 2. The disruption of periodontal and gastrointestinal microbiota is a significant trigger of systemic immune responses. Periodontal pathogens release virulence factors such as endotoxins, peptidoglycans, and microbial DNA, which directly enter the digestive system. These components interact with Toll-like receptors (TLRs) on the intestinal epithelial surface and Nod-like receptors (NLRs) within cells, initiating inflammatory signaling cascades. Simultaneously, Th17 cell-mediated immune pathways are activated, promoting the secretion of pro-inflammatory cytokines, including IL-1, IL-6, and IL-8. This inflammatory milieu intensifies intestinal inflammation, creating a feedback loop that further amplifies the activation of Th17 cells and macrophages. As a result, systemic immune pathways are enhanced, elevating inflammatory cytokine levels and accelerating the progression of periodontal inflammation. Additionally, bacteria and their inflammatory mediators from periodontal sites can enter the systemic circulation. This process leads to bacteremia, endotoxemia, and in severe cases, sepsis. These systemic infections can result in severe complications, including osteomyelitis and widespread bacterial dissemination.
Apart from the effects of salivary flow and blood circulation pathways, dysbiosis of the oral microbiota could also directly influence immune pathways by disrupting the balance between innate and adaptive immunity, potentially leading to immune-mediated inflammatory diseases (IMIDs). T helper cells-17 (Th17 cells) and the IL-17/IL-23 signaling axis play critical roles in adaptive immune responses in this process. Moreover, microbial imbalance is considered to be a primary driver of protective immune responses in barrier tissues. The periodontal pathogens accumulate in the gut due to oral inflammation. Their toxic byproducts and pro-inflammatory cytokines, such as TNF-α and IL-1β produced by local tissues result in immune responses in the gut barrier, with IL-17 exerting a particularly notable effect (Kitamoto et al., 2020). Besides, elevated IL-17 expression in GCF have been observed in patients with periodontitis, suggesting that gut microbiota dysbiosis induces subsequent endotoxemiam, and the Th17 cell-mediated gut immune response may underlie the relation between chronic periodontitis and systemic diseases (Beura et al., 2016).
3.5 Regional immunity caused by periodontal homeostasis and imbalance
The periodontal microbiota, epithelial barrier, soft tissue extracellular matrix (ECM) and bone-tissue coupling system form an intricate regulatory network that collectively sustains periodontal homeostasis. Since the concept of “homeostasis medicine” was proposed (Wang and Qin, 2022), research on the etiology, treatment, and preventive strategies for periodontal and peri-implant inflammation has altered from localized focus to the systemic approach. The body regulates periodontal environment stability via receptors on various immune cell surfaces, signaling pathways and secreted cytokines. In healthy physiological states, subgingival plaque exhibits low pathogenicity, while the physical barrier of periodontal mucosa remains intact. The moderate immune response primarily involves neutrophils, macrophages and T-cell subsets (γδT-cells) to execute effective surveillance, control local microbiota and tolerate symbiotic microbes and harmless antigens to a certain extent (Belkaid and Harrison, 2017). Periodontal supporting tissues also undergo physiological remodeling in response to moderate external stimuli without inducing significant tissue destruction. The dysregulation of periodontal homeostasis begins with the localized colonization of core periodontal pathogens, which destabilizes the epithelial homeostasis. Pathogens disrupt regional immune surveillance and microbial control mechanisms, heightening the pathogenicity of the subgingival microbiota and disturbing the microbiome-epithelium balance. Stimulated by microbial activity, tissue and resident immune cells release substantial levels of pro-inflammatory mediators such as cytokines and chemokines, which subsequently activate regional immune responses through positive feedback (Hajishengallis, 2015). In this phase, cytokines produced by the residential cell population have the main function to stimulate cells migration to sites of infection and enhance the expression of adhesion molecules for neutrophils on the internal vessel surfaces and increase the synthesis of other proinflammatory cytokines (Di Stefano et al., 2022). Besides, the expression of ECM-metabolizing enzymes is upregulated in this state, while ECM synthesis is impaired, ultimately compromising gingival soft tissue homeostasis; the osteoblast-osteoclast coupling balance is disrupted, resulting in osteoclast activation and subsequent alveolar bone resorption (Figure 3).
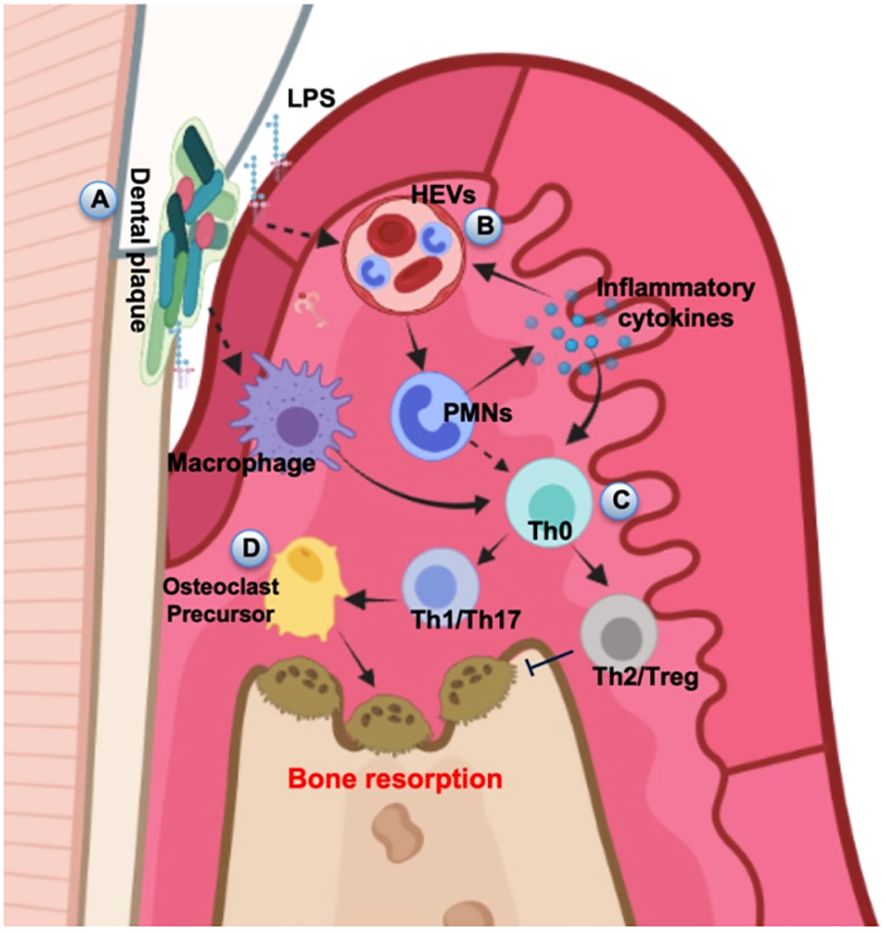
Figure 3. Schematic diagram of alveolar bone destruction in inflammatory response. (A) Initially, pathogenic microorganisms and toxic products in dental plaque stimulate periodontal tissue, directly inducing macrophages to cause local inflammation and immune response. (B) LPS and inflammatory cytokines activate the HEVs leading to vascular hyperpermeability and PMN transmigration, and this process can also provide feedback to regulate the release of inflammatory cytokines. (C) Upstream stimulation drives T helper cells to differentiate into several subsets for cellular immunity. (D) The amplification of local immune response leads to the development of inflammation and results in the progression of periodontal destruction and bone resorption. The diagram was devised from (Di Stefano et al., 2022).
Both dental implants and natural teeth breach the periodontal mucosal barrier and expose within the oral microbial environment. However, this does not mean that this barrier cannot play a practical role. The gingival sulcular epithelium responds to subgingival microbial stimuli by releasing defensins, antimicrobial peptides and cytokines, which activate regional immunity to manage microbial communities and support periodontal homeostasis (Ross and Herzberg, 2016). The junctional epithelium at the sulcus base has a weaker barrier function. Neutrophils and tissue fluid containing antimicrobial agents and cytokines permeate into the gingival sulcus, forming GCF, which further contributes to periodontal stability (Moutsopoulos and Konkel, 2018); however, around implants, the lack of robust junctional epithelium enhances susceptibility to severe inflammatory assaults. Furthermore, solitary chemosensory cells dispersed within the epithelial layer could detect microbial stimuli via bitter taste receptors, thereby performing immune surveillance functions (Zheng et al., 2019). Neutrophils are the predominant innate immune cells in healthy gingiva, where both a deficiency and overactivity of neutrophils can result in periodontal damage (Xiao et al., 2017; Hajishengallis, 2020). When gingival sulcus and junctional epithelial cells are stimulated by pathogenic bacteria, they induce specific immune cells to express pro-inflammatory mediators, activating downstream signaling pathways that drive extensive neutrophil infiltration. This neutrophil-driven pathway of tissue damage mediated by chemotactic factors is termed the “matrix-neutrophil axis” (Hajishengallis and Chavakis, 2013; Williams et al., 2021). Moreover, neutrophils in the gingiva of inflammatory patients remain hyperactivated and highly reactive that is difficult to reverse in a short term following periodontal therapy (Ling et al., 2015). The above observations indicated that epithelial cells, fibroblasts and other tissue cells not only experience inflammatory damage but may play the role of initiators or signal transmitters during the progression of homeostatic imbalance, and the maintenance of periodontal homeostasis may necessitate a balanced level of neutrophil infiltration. During adaptive immunity, Th17 cells serve as the primary source of IL-17 at mucosal barriers and locally destabilized periodontal tissues are often accompanied by a marked increase in IL-17 expression and Th17 cell infiltration (Beklen et al., 2007; Kitamoto et al., 2020). IL-17 enhances neutrophil adhesion to vascular endothelium, promotes fibroblast secretion of ECM degrading enzymes, and upregulates RANKL expression to activate osteoclasts, thereby disrupting soft tissue metabolism and bone coupling homeostasis (Kotake et al., 2012; Miossec and Kolls, 2012); elevated local immune activation induces substantial expression of pro-inflammatory cytokines, including IL-1 and IL-17, which in turn activate osteoclasts and inhibit osteoblast function through the RANKL/NF-κB/osteoprotegerin signaling axis, causing bone resorption in periodontitis (Lin et al., 2014; Pan et al., 2019). Besides, studies reported that IL-17 and IL-21 secreted by Th17 cells could induce differentiation, B-cell proliferation and IgG production (Ettinger et al., 2007; Hickman-Brecks et al., 2011). Neutralization of IL-17 antibodies has been shown to alleviate alveolar bone resorption caused by periodontitis (Hajishengallis, 2020). Thus, Th17 cells are widely recognized as critical contributors to immune homeostasis imbalance in the periodontal region, mediating periodontal instability through multiple mechanisms. Another inflammatory mediator, IL-18, activates the NF-κB pathway, leading fibroblasts, neutrophils and monocytes to release matrix metalloproteinase (MMPs), which result in periodontal tissue degradation (Beklen et al., 2007; Wang et al., 2019). In addition, osteoblasts and osteoclasts work in tandem to establish bone-coupling homeostasis, ensuring continuous bone formation and remodeling. Notably, approximately 50% of T cells and 90% of B cells in the gingival tissues of periodontitis patients express RANKL, implying that lymphocyte-derived immune cells may be a significant source of RANKL, contributing to bone-coupling imbalance (Kawai et al., 2006). Through disruption of bone-coupling homeostasis, B cells may actively drive periodontal instability.
The periodontal barrier encounters diverse stimuli such as mechanical forces from mastication and food antigens. The composition of local subgingival microbiota is highly complex, but the barrier function is relatively weak. The body needs to maintain local homeostasis through active and complex regulatory mechanisms. Based on the previously discussed factors leading to the maintenance and disruption of periodontal homeostasis, a range of novel strategies aimed at restoring periodontal stability is currently under investigation. These strategies include controlling pathogenic microbiota, modulating immune responses in the periodontal region and guiding the regeneration of soft and hard tissues. Among these, suppressing the progression of immune-mediated inflammation driven by pathogenic microorganisms remains the primary focus.
3.6 The differences and similarities between periodontitis and peri implantitis
The peri-implant tissues harbor approximately 400 microbial species, which is significantly fewer than those found in healthy periodontal tissues. However, research showed that the microbial characteristics of healthy peri-implant and periodontal sites share greater similarities compared to inflamed regions (Zheng et al., 2015). The distinctions from health to disease in the periodontal and peri-implant tissues are not determined solely by the presence or absence of specific pathogens but rather reflected in taxonomic changes across the entire microbial community. Furthermore, the changes in microbial diversity are marked by a reduction in the number of bacterial species under healthy conditions and a gradual increase in complexity as disease progresses, underscoring the importance of maintaining a balanced microbiome to prevent disease onset (Belibasakis and Manoil, 2021; Jung and Lee, 2023). The necessity of regular monitoring and early intervention has been proposed to protect the health of periodontal and peri-implant tissues. In the first few months following implant placement, the biofilm’s taxonomic composition around the implant shows only slight differences; however, microbial diversity is lower than that around adjacent teeth (Payne et al., 2017). Alpha diversity analysis reveals no significant differences in the diversity of the subgingival plaque community between patients with peri-implantitis and those with peri-implant health, suggesting that pathogenic bacteria associated with peri-implant inflammation may be present from the outset (Yu et al., 2019) (Figure 4). However, compared to healthy teeth, elevated levels of Prevotella, Treponema, Leptotrichia, S. mutans, Butyrivibrio, Catonella, Propionibacter and Lactococcus are observed in the peri-implant sulcus during the early phase of implantation (Kumar et al., 2012), suggesting an increased risk of inflammation. While non-specific opportunistic pathogens are less abundant around healthy tissues, S. aureus, P. aeruginosa, and C. albicans can still be detected. Additionally, bacterial diversity increases in inflamed peri-implant tissues with more opportunistic pathogens including S. intermedius, S. aureus, S. mitis and Haemophilus influenzae being identified (Padial-Molina et al., 2016). Furthermore, almost all cases of peri-implant inflammation were accompanied by bleeding on probing, and the probing results closely matched the average values recorded from periodontal tissues. A study found that when comparing pathogens between implant and tooth samples from the same individual, 54% of patients showed identical results in both sample types, while 45% showed differing results. In 39% of patients, the implant sample was positive for periodontal pathogens, while the tooth sample was negative. Only 6% of patients showed a positive tooth sample and a negative implant sample (Albertini et al., 2015).
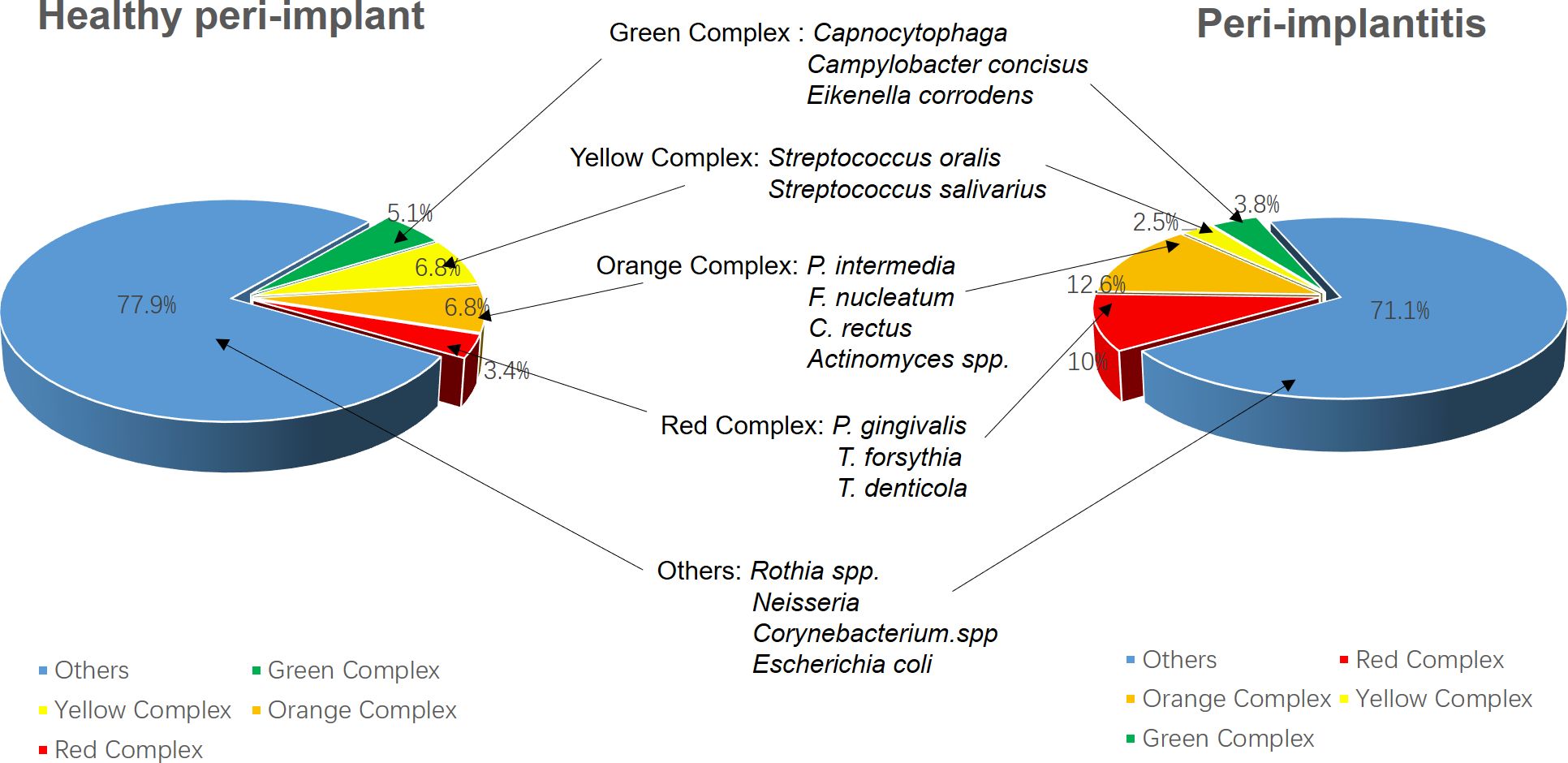
Figure 4. The diversity of microbial communities shows no significant differences between peri-implantitis patients and those with healthy peri-implant tissues. However, peri-implantitis is characterized by a notable increase in pathogenic bacterial abundance; meanwhile, the proportion of opportunistic pathogens from the yellow and green complexes decreases, suggesting that peri-implantitis is primarily dominated by the red complex. The data were derived from (Al-Ahmad et al., 2018).
The immunological events driving peri-implant infections are qualitatively similar with those in periodontal infections, primarily involving Gram-negative bacteria. However, peri-implant infections have a broader inflammatory scope and lead to more rapid tissue destruction (Belibasakis et al., 2015). Natural teeth are anchored to the alveolar bone by the periodontal ligament (PDL), while dental implants are directly anchored to the alveolar bone through osseointegration. The lack of the PDL around implant limits the blood supply, reducing the availability of nutrients and immune cells necessary to combat early bacterial infections (Araujo and Lindhe, 2018; Berglundh et al., 2018). Additionally, the fibers in the alveolar ridge connective tissue are arranged circumferentially rather than vertically, impairing the capacity of the physical barrier against bacterial invasion into the submucosa and leaving the peri-implant tissues in a more fragile “open wound” state. Subtle variations across multiple factors result in slight differences between the microbial communities of peri-implantitis and periodontitis. In general, several critical periodontal pathogens, including P. gingivalis, T. denticola, T. forsythia, A. actinomycetemcomitans, P. intermedia, F. nucleatum, and Campylobacter spp., are strongly associated with peri-implantitis (Zheng et al., 2015). Despite significant overlap, the microbiota of peri-implantitis exhibits distinct differences compared to that of periodontitis. Research has identified approximately 22 high-abundance pathogenic species in peri-implantitis, 21 of which are also commonly found in periodontitis. However, certain species that are prevalent in periodontitis, such as F. nucleatum subsp. vincentii, A. cardiffensis, Olsenella spp., Selenomonas sputigena, and Corynebacterium matruchotii, are rarely detected in peri-implantitis (Komatsu et al., 2020) (Figure 5). Smoking profoundly affects the oral microbiome, altering its composition in tissues. In inflammation of both peri-implant and natural periodontal, P. gingivalis and T. denticola are more prevalent in smokers than in non-smokers, both the two types of pathogens with prevalence rates of 90% and 60% in smokers compared with those 69% and 34% in non-smokers, respectively; however, T. forsythia and P. intermedia were more frequently observed in non-smokers, with prevalence rates of 69% and 47%, respectively, compared with 60% and 30% in smokers; on the other hand, for some opportunistic microorganisms, P. aeruginosa is more commonly found in smokers than in non-smokers, with the prevalence rates of 20% and 9%, respectively (Albertini et al., 2015). Although both periodontitis and peri-implantitis are plaque-induced inflammatory conditions, some other local factors may also be associated with this complication due to their role in promoting plaque retention. These modifiable factors including surgical factors (e.g., improper implant placement, failure of bone reconstruction), prosthetic factors (e.g., inadequate design of implant-supported restorations, improper distribution of stress in the restoration), or biomechanical factors (e.g., overload, excessive occlusal pressure) may contribute to the development of unfavorable conditions, promoting the transition from physiological bone loss to peri-implant disease. Therefore, disease classification has to integrate factors that related to surgery, prosthetics and biomechanics to better address these issues (Canullo et al., 2017).
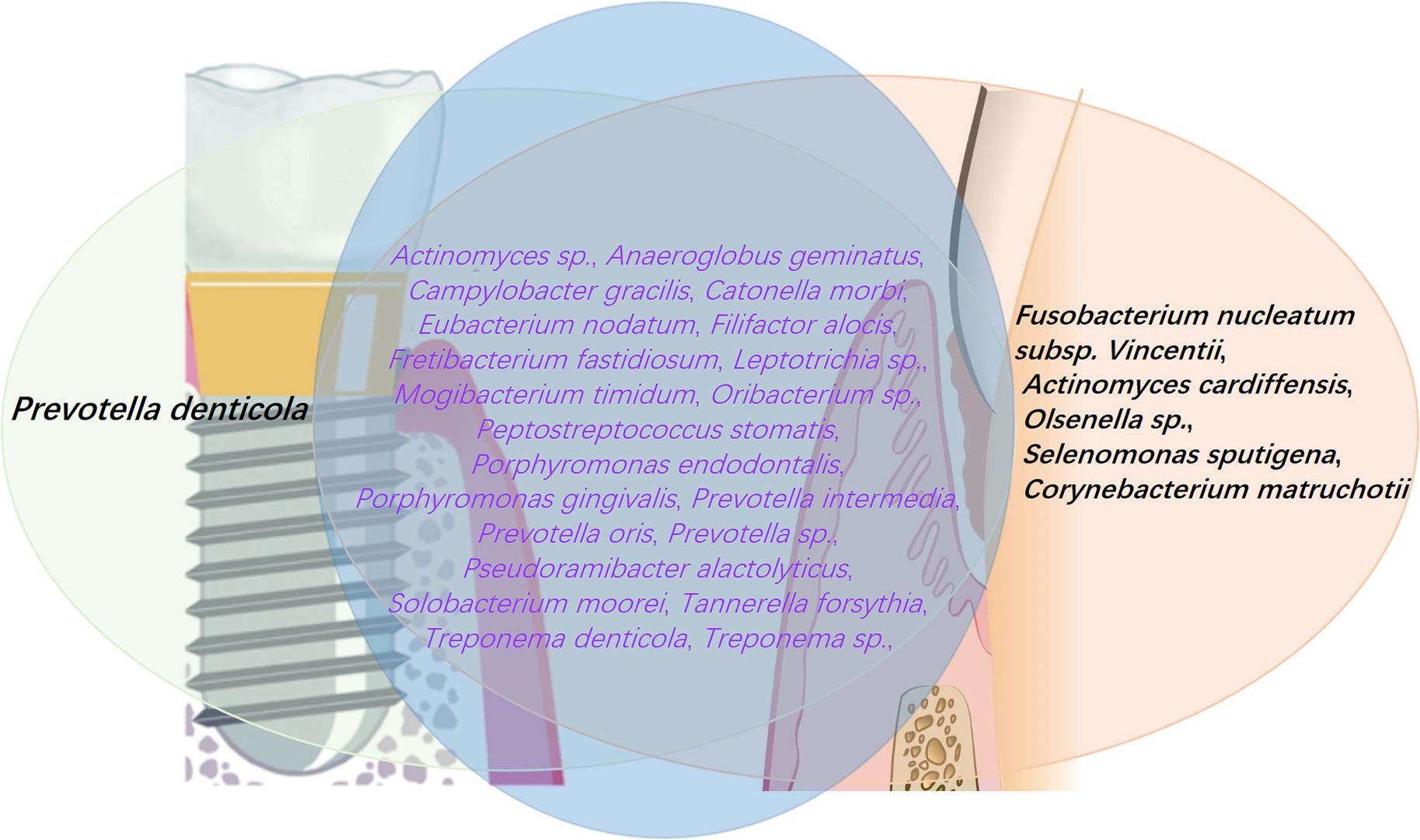
Figure 5. Periodontitis is characterized by the presence of 26 high-prevalence pathogens, 21 of which are also found in peri-implantitis. However, certain pathogens are unique to periodontitis, including F. nucleatum subsp. Vincentii, A. cardiffensis, Olsenella sp., S. sputigena, and C. matruchotii. Conversely, P. denticola is predominantly associated with peri-implantitis, playing a key role in the inflammatory processes and biofilm development characteristic of this condition. The data were derived from (Komatsu et al., 2020).
3.7 The impact of non-mechanical treatment of inflammation on microorganisms
Mechanical debridement (MD) alone often insufficient to meet both efficiency and minimally invasive standards required for treating peri-implant diseases. Combining non-surgical treatments with MD for better outcomes has become an increasingly common approach (Dentino et al., 2013; Majzoub et al., 2021). However, different non-surgical options exhibit varying levels of efficacy, making it necessary to explore the optimal treatment combinations and understand the differences in therapeutic outcomes to guide clinical practice. Research have shown that all isolated strains of P. aeruginosa are sensitive to ciprofloxacin, ceftazidime, and aminoglycosides, while the isolated strains of S. aureus are sensitive to methicillin. Nevertheless, antibiotic resistance remains an unavoidable problem, and the non-selective bactericidal effects of antibiotics are directly linked to microbial dysbiosis (Alves et al., 2022). Probiotic supplements administered as an adjunct to supragingival scaling and root planing have been shown to partially suppress the proliferation of pathogenic bacteria, thereby mitigating microbial dysbiosis (Vivekananda et al., 2010; Alves et al., 2022). As such, Probiotics are regarded as a safe and effective biological intervention, with studies in animal models certified that specific probiotic strains administered orally effectively inhibit alveolar bone loss. These probiotics influence the overall development and stability of the microbiota in the oral cavity by modulating the colonization potential of pathogens, thereby supporting and stabilizing the host immune system and reducing the production of pro-inflammatory cytokines (Lauritano et al., 2019; Yang et al., 2020a). Although these bacteria may not directly eradicate pathogens, this approach may provide some clinical efficacy in reducing periodontal pocket depth in periodontitis (Peña et al., 2019). Research indicated that Rothia species harbor nitrate reductase enzymes, which catalyze the reduction of nitrate (NO3−) to nitrite (NO2−). In this process, Rothia directly utilize nitrate-rich foods as an additional energy source, a capability particularly prominent in the hypoxic or anoxic conditions of the gingival sulcus. The nitrite is subsequently metabolized into nitric oxide (NO), a bioactive molecule with potent antimicrobial properties, which reduces the pathogenic bacterial burden and mitigates periodontal inflammation (Mazurel et al., 2023). Furthermore, nitrate-metabolizing bacteria contribute to pH regulation and redox balance in the gingival sulcus, improving the microbial habitat conditions and supporting the co-growth of other beneficial bacterial species (Yamamoto et al., 2024). The lactic acid bacterium Lactobacillus reuteri (primarily Gram-positive) has been demonstrated to outcompete pathogens for adhesion to epithelial tissues and gain a colonization advantage. It reduces soft tissue damage by inhibiting the production of MMP-8 in periodontal tissues (İnce et al., 2015), induces controlled oxidative stress in surrounding cells through 3-hydroxypropionaldehyde formation to protect native tissues (Tekce et al., 2015), and decreases the expression levels of pro-inflammatory cytokines such as TNF-α, IL-1β, IL-6 and IL-8 in patients with peri-implant mucositis, effectively inhibiting the growth of A. actinomycetemcomitans, P. gingivalis, and P. intermedia (Kõll-Klais et al., 2005; Szkaradkiewicz et al., 2014), Moreover, P. gingivalis, P. intermedia, Streptococcus salivarius, and S. aureus, among other common pathogens associated with periodontitis or peri-implantitis are highly sensitive to these lactic acid bacteria (Mulla et al., 2021). The most common oral bacterium, S. salivarius, has been shown to have beneficial effects on implant-associated biofilms. Probiotics derived from S. salivarius produce bacteriocins that inhibit quorum sensing signals and reduce S. intermedius biofilm formation on titanium implant surfaces (Vacca et al., 2020). Although Streptococcus sanguinis and Streptococcus uberis are relatively low in abundance, they could produce hydrogen peroxide to change the local environment, effectively inhibiting the growth of A. actinomycetemcomitans (Laleman et al., 2015). To sum up, these probiotics may be considered adjuncts in non-surgical treatments to prevent biofilm-related oral diseases. Currently, no specific studies revealed whether interactions exist between probiotics and fungi (Martorano-Fernandes et al., 2020).
Due to the rough and helical structure of implant surfaces, ultrasonic scaling is often insufficient for complete bacterial removal. Moreover, excessive use of antimicrobials may increase bacterial resistance, potentially leading to secondary infections. Currently, laser technology is becoming widely adopted in oral disease treatment, this approach provides several advantages including high patient acceptance, ease of use, minimally invasive application, tissue hemostasis and accelerated healing. In addition, lasers are especially effective at removing granulation tissue from implant surfaces without causing melting, cracking or deformation (Shibli, 2018). Unlike natural roots or surrounding biological tissues, the effectiveness of different laser types with varying wavelengths may differ when used on titanium surfaces. Studies have indicated that CO2 and diode lasers are not effective in removing plaque biofilms from root surfaces or titanium implants. These types of lasers have only been used in addition to mechanical treatment procedures (Chen et al., 2022a). In contrast, the Er laser has shown significant bactericidal potential in periodontal treatment, effectively eliminating bacterial contaminants from the textured surfaces of implants (Cosgarea et al., 2023; Aoki et al., 2024). The Er: YAG laser directly impairs bacterial viability by disrupting LPS and reducing anaerobic bacterial counts (Chen et al., 2022a), and it also mitigates inflammatory damage by regulating the miR-155/SIRT1 axis and the activation of IL-6 and NF-κB signaling pathways, thereby affecting osteogenic differentiation, inflammation, proliferation, apoptosis and autophagy (Ogita et al., 2015; Lin et al., 2021; Ng et al., 2024) — ultimately preserving tissue and minimizing damage. Antimicrobial photodynamic therapy (aPDT) employs laser and photosensitizer at specific wavelengths to generate ROS, inducing superoxide dismutation reaction that disrupts bacterial lipid layers. ROS penetrate bacterial lipid membranes, leading to protein leakage and selectively eliminating anaerobes and their metabolites. The above therapies have been shown to significantly reduce P. intermedia/nigrescens, Fusobacterium spp., and beta-hemolytic Streptococcus, even decreasing plaque accumulation by up to 70% (Shibli et al., 2003; Mao et al., 2019).
Dietary component nitrate has garnered significant attention for its critical role in maintaining periodontal tissue health. This importance is highlighted by its interactions with the oral microbiota, its capacity to regulate inflammatory responses, and its promising potential as an adjunctive therapeutic option for managing periodontal disease (Rosier et al., 2022). Recent studies demonstrate that nitrate serves as a substrate for nitrate-reducing bacteria, with Rothia serving as the predominant genus in this process, including Veillonella and Actinomyces, which enzymatically convert NO3− into nitrite NO2−. Nitrite subsequently acts as a precursor for NO, a critical signaling molecule with diverse biological effects (Wicaksono et al., 2020; Schlagenhauf, 2022). NO exerts substantial antimicrobial activity by inhibiting the key periodontal pathogens such as P. gingivalis and F. nucleatum. It also modulates the abundance of approximately 20 microbial species, including notable genera such as Campylobacter, Fusobacterium, Neisseria, Prevotella, Rothia, Selenomonas, Staphylococcus, Streptococcus and others. This microbial regulation helps maintain oral microbial homeostasis and reduces dysbiosis within periodontal pockets (L’Heureux et al., 2023). In addition, NO exhibits robust immunomodulatory properties, suppressing the production of pro-inflammatory cytokines to prevent excessive immune activation. This modulation reduces inflammatory burden, thereby protecting against periodontal tissue destruction. Furthermore, NO regulates critical pathways such as NF-κB signaling and ROS production, facilitating a balance between protective inflammation and tissue damage. Additionally, it enhances endothelial cell function and angiogenesis, improving vascular perfusion and promoting tissue repair — essential processes for periodontal regeneration (Rammos et al., 2015; Yang et al., 2020b). Nitrate-derived NO also synergizes with antimicrobial therapies, restoring microbial balance and supporting long-term periodontal stability. Integrating nitrate-based strategies into periodontal treatment paradigms represents an innovative approach, with nitrate supplementation showing potential as an adjunctive therapy for periodontal disease. Future explorations should focus on understanding nitrate bioavailability and microbial dynamics. Personalized strategies for optimizing nitrate metabolism are also essential to fully harness its therapeutic potential in periodontal disease management and oral health maintenance (Rosier et al., 2020). While excessive local application of nitrate (at concentrations exceeding 100 nM) may exhibit toxic effects on oral microbiota, its application as a prebiotic, either alone or in conjunction with nitrate-reducing probiotics, represents a promising adjunctive approach for the treatment of periodontitis (Wicaksono et al., 2020; Mazurel et al., 2023).
4 Conclusion
Bacterial dysbiosis is considered one of the central causes driving the development of periodontitis and peri-implantitis. The proliferation of pathogenic bacteria, biofilm formation and dysregulation of systemic immune responses collectively contribute to the onset and progression of these inflammatory diseases. To maintain the delicate balance between bone resorption and regeneration, the osteoclast-osteoblast equilibrium must be properly regulated to ensure effective alveolar bone remodeling and homeostasis. During bacterial infections, the inflammasome activation could induce bacterial dissemination and uncontrolled bone destruction. These conditions are commonly seen in periodontitis, periapical inflammation, peri-implantitis and other related diseases, all of which share similar mechanisms. Uncontrolled inflammasome activity also enhances the activation of macrophages, monocytes, neutrophils and other adaptive immune cells such as Th17 cells, resulting in increased osteoclast numbers and suppressed osteoblast activity, ultimately eventuating alveolar bone loss. Additionally, osteocytes play a key role in this process by secreting many cytokines that regulate alveolar bone resorption and formation in response to inflammatory signal changes (Huang et al., 2020).
The peri-implant microbiota exhibits notable differences from the periodontal niche, including lower diversity. Changes in microbial community composition are inextricably linked to transitions between health and disease, with host-microbiota interactions may contributing to the development of peri-implantitis. Insights into the specificity of the oral microbiome underscore the complexity and challenges of managing oral infections. This review discussed the differences in common microbial species between healthy periodontal and inflamed regions, as well as their pathogenic mechanisms. Understanding the mechanisms of changes in bacterial flora is of great significance for the prevention, diagnosis and treatment of oral diseases. This review suggests that dysbiosis in the oral microbiome may act as the initial disturbance in bacterial species balance, with pathogenic bacteria gaining dominance by forming robust biofilms, which directly or indirectly intensify tissue destruction. Inflammation often progresses in a non-linear increasing pattern and is frequently accompanied by significant crestal bone loss. Plaque control through routine maintenance and early intervention helps retain a healthy oral environment and significantly reduces the risk of periodontal inflammation. Besides, further research is needed to clarify the mechanisms underlying the transition from peri-implant mucositis to peri-implantitis and to optimize current prevention and treatment strategies.
Author contributions
ZC: Writing – original draft, Writing – review & editing. PW: Data curation, Formal analysis, Supervision, Writing – review & editing. WG: Funding acquisition, Supervision, Writing – review & editing.
Funding
The author(s) declare that financial support was received for the research, authorship, and/or publication of this article. This study was supported by the grants from the Science and Technology Project of Lanzhou (grant No. 2022-5-64).
Conflict of interest
The authors declare that the research was conducted in the absence of any commercial or financial relationships that could be construed as a potential conflict of interest.
Generative AI statement
The author(s) declare that no Generative AI was used in the creation of this manuscript.
Publisher’s note
All claims expressed in this article are solely those of the authors and do not necessarily represent those of their affiliated organizations, or those of the publisher, the editors and the reviewers. Any product that may be evaluated in this article, or claim that may be made by its manufacturer, is not guaranteed or endorsed by the publisher.
References
Aas, J. A., Paster, B. J., Stokes, L. N., Olsen, I., Dewhirst, F. E. (2005). Defining the normal bacterial flora of the oral cavity. J. Clin. Microbiol. 43, 5721–5732. doi: 10.1128/jcm.43.11.5721-5732.2005
Åberg, C. H., Kelk, P., Johansson, A. (2015). Aggregatibacter actinomycetemcomitans: virulence of its leukotoxin and association with aggressive periodontitis. Virulence 6, 188–195. doi: 10.4161/21505594.2014.982428
Acharya, C., Sahingur, S. E., Bajaj, J. S. (2017). Microbiota, cirrhosis, and the emerging oral-gut-liver axis. JCI Insight 2. doi: 10.1172/jci.insight.94416
Al-Ahmad, A., Muzafferiy, F., Anderson, A. C., Wölber, J. P., Ratka-Krüger, P., Fretwurst, T., et al. (2018). Shift of microbial composition of peri-implantitis-associated oral biofilm as revealed by 16S rRNA gene cloning. J. Med. Microbiol. 67, 332–340. doi: 10.1099/jmm.0.000682
Albaghdadi, S. Z., Altaher, J. B., Drobiova, H., Bhardwaj, R. G., Karched, M. (2021). In vitro characterization of biofilm formation in prevotella species. Front. Oral. Health 2. doi: 10.3389/froh.2021.724194
Albertini, M., López-Cerero, L., O’Sullivan, M. G., Chereguini, C. F., Ballesta, S., Ríos, V., et al. (2015). Assessment of periodontal and opportunistic flora in patients with peri-implantitis. Clin. Oral. Implants Res. 26, 937–941. doi: 10.1111/clr.12387
Alves, C. H., Russi, K. L., Rocha, N. C., Bastos, F., Darrieux, M., Parisotto, T. M., et al. (2022). Host-microbiome interactions regarding peri-implantitis and dental implant loss. J. Transl. Med. 20, 425. doi: 10.1186/s12967-022-03636-9
Aoki, A., Mizutani, K., Taniguchi, Y., Lin, T., Ohsugi, Y., Mikami, R., et al. (2024). Current status of Er: YAG laser in periodontal surgery. Jpn Dent. Sci. Rev. 60, 1–14. doi: 10.1016/j.jdsr.2023.11.002
Apatzidou, D., Lappin, D. F., Hamilton, G., Papadopoulos, C. A., Konstantinidis, A., Riggio, M. P. (2017). Microbiome of peri-implantitis affected and healthy dental sites in patients with a history of chronic periodontitis. Arch. Oral. Biol. 83, 145–152. doi: 10.1016/j.archoralbio.2017.07.007
Apaza-Bedoya, K., Tarce, M., Benfatti, C. A. M., Henriques, B., Mathew, M. T., Teughels, W., et al. (2017). Synergistic interactions between corrosion and wear at titanium-based dental implant connections: A scoping review. J. Periodontal Res. 52, 946–954. doi: 10.1111/jre.12469
Araujo, M. G., Lindhe, J. (2018). Peri-implant health. J. Periodontol 89 Suppl 1, S249–s256. doi: 10.1002/jper.16-0424
Arweiler, N. B., Netuschil, L. (2016). The oral microbiota. Adv. Exp. Med. Biol. 902, 45–60. doi: 10.1007/978-3-319-31248-4_4
Baliga, S., Muglikar, S., Kale, R. (2013). Salivary pH: A diagnostic biomarker. J. Indian Soc. Periodontol 17, 461–465. doi: 10.4103/0972-124x.118317
Ball, J., Darby, I. (2022). Mental health and periodontal and peri-implant diseases. Periodontol 2000 90, 106–124. doi: 10.1111/prd.12452
Bao, J., Li, L., Zhang, Y., Wang, M., Chen, F., Ge, S., et al. (2022). Periodontitis may induce gut microbiota dysbiosis via salivary microbiota. Int. J. Oral. Sci. 14, 32. doi: 10.1038/s41368-022-00183-3
Baseri, M., Radmand, F., Hamedi, R., Yousefi, M., Kafil, H. S. (2020). Immunological aspects of dental implant rejection. BioMed. Res. Int. 2020, 7279509. doi: 10.1155/2020/7279509
Baty, J. J., Stoner, S. N., Scoffield, J. A. (2022). Oral commensal streptococci: gatekeepers of the oral cavity. J. Bacteriol 204, e0025722. doi: 10.1128/jb.00257-22
Beklen, A., Ainola, M., Hukkanen, M., Gürgan, C., Sorsa, T., Konttinen, Y. T. (2007). MMPs, IL-1, and TNF are regulated by IL-17 in periodontitis. J. Dent. Res. 86, 347–351. doi: 10.1177/154405910708600409
Belibasakis, G. N. (2014). Microbiological and immuno-pathological aspects of peri-implant diseases. Arch. Oral. Biol. 59, 66–72. doi: 10.1016/j.archoralbio.2013.09.013
Belibasakis, G. N., Charalampakis, G., Bostanci, N., Stadlinger, B. (2015). Peri-implant infections of oral biofilm etiology. Adv. Exp. Med. Biol. 830, 69–84. doi: 10.1007/978-3-319-11038-7_4
Belibasakis, G. N., Johansson, A. (2012). Aggregatibacter actinomycetemcomitans targets NLRP3 and NLRP6 inflammasome expression in human mononuclear leukocytes. Cytokine 59, 124–130. doi: 10.1016/j.cyto.2012.03.016
Belibasakis, G. N., Manoil, D. (2021). Microbial community-driven etiopathogenesis of peri-implantitis. J. Dent. Res. 100, 21–28. doi: 10.1177/0022034520949851
Belkaid, Y., Harrison, O. J. (2017). Homeostatic immunity and the microbiota. Immunity 46, 562–576. doi: 10.1016/j.immuni.2017.04.008
Bengtsson, T., Khalaf, A., Khalaf, H. (2015). Secreted gingipains from Porphyromonas gingivalis colonies exert potent immunomodulatory effects on human gingival fibroblasts. Microbiol. Res. 178, 18–26. doi: 10.1016/j.micres.2015.05.008
Berglundh, T., Armitage, G., Araujo, M. G., Avila-Ortiz, G., Blanco, J., Camargo, P. M., et al. (2018). Peri-implant diseases and conditions: Consensus report of workgroup 4 of the 2017 World Workshop on the Classification of Periodontal and Peri-Implant Diseases and Conditions. J. Clin. Periodontol 45 Suppl 20, S286–s291. doi: 10.1111/jcpe.12957
Bescos, R., Ashworth, A., Cutler, C., Brookes, Z. L., Belfield, L., Rodiles, A., et al. (2020). Effects of Chlorhexidine mouthwash on the oral microbiome. Sci. Rep. 10, 5254. doi: 10.1038/s41598-020-61912-4
Beura, L. K., Hamilton, S. E., Bi, K., Schenkel, J. M., Odumade, O. A., Casey, K. A., et al. (2016). Normalizing the environment recapitulates adult human immune traits in laboratory mice. Nature 532, 512–516. doi: 10.1038/nature17655
Boisen, G., Davies, J. R., Neilands, J. (2021). Acid tolerance in early colonizers of oral biofilms. BMC Microbiol. 21, 45. doi: 10.1186/s12866-021-02089-2
Bolton, R. W., Kluever, E. A., Dyer, J. K. (1985). In vitro immunosuppression mediated by an extracellular polysaccharide from Capnocytophaga ochracea. Influence of macrophages. J. Periodontal Res. 20, 251–259. doi: 10.1111/j.1600-0765.1985.tb00432.x
Bostanci, N., Belibasakis, G. N. (2012). Porphyromonas gingivalis: an invasive and evasive opportunistic oral pathogen. FEMS Microbiol. Lett. 333, 1–9. doi: 10.1111/j.1574-6968.2012.02579.x
Bowen, W. H., Burne, R. A., Wu, H., Koo, H. (2018). Oral biofilms: pathogens, matrix, and polymicrobial interactions in microenvironments. Trends Microbiol. 26, 229–242. doi: 10.1016/j.tim.2017.09.008
Brambilla, E., Gagliani, M., Ionescu, A., Fadini, L., García-Godoy, F. (2009). The influence of light-curing time on the bacterial colonization of resin composite surfaces. Dent. Mater 25, 1067–1072. doi: 10.1016/j.dental.2009.02.012
Canullo, L., Peñarrocha, M., Monje, A., Catena, A., Wang, H. L., Peñarrocha, D. (2017). Association between clinical and microbiologic cluster profiles and peri-implantitis. Int. J. Oral. Maxillofac. Implants 32, 1054–1064. doi: 10.11607/jomi.6043
Canullo, L., Peñarrocha-Oltra, D., Covani, U., Rossetti, P. H. (2015). Microbiologic and clinical findings of implants in healthy condition and with peri-implantitis. Int. J. Oral. Maxillofac. Implants 30, 834–842. doi: 10.11607/jomi.3947
Cardoso, J. M., Ribeiro, A. C., Botelho, J., Proença, L., Noronha, S., Alves, R. C. (2024). The influence of genetic polymorphisms on the expression of interleukin-1beta, prostaglandin E2 and tumor necrosis factor alpha in peri-implant crevicular fluid: A cross-sectional study. Int. J. Mol. Sci. 25. doi: 10.3390/ijms25010651
Chen, J. H., Lin, Y. C., Kung, J. C., Yan, D. Y., Chen, I. H., Jheng, Y. S., et al. (2022a). Efficacy of Er: YAG laser for the peri-implantitis treatment and microbiological changes: a randomized controlled trial. Lasers Med. Sci. 37, 3517–3525. doi: 10.1007/s10103-022-03627-8
Chen, T., Marsh, P. D., Al-Hebshi, N. N. (2022b). SMDI: an index for measuring subgingival microbial dysbiosis. J. Dent. Res. 101, 331–338. doi: 10.1177/00220345211035775
Cheng, T., Wen, P., Yu, R., Zhang, F., Li, H., Xu, X., et al. (2024). Integrative microbiome and metabolome profiles reveal the impacts of periodontitis via oral-gut axis in first-trimester pregnant women. J. Transl. Med. 22, 819. doi: 10.1186/s12967-024-05579-9
Cho-Yan Lee, J., Mattheos, N., Nixon, K. C., Ivanovski, S. (2012). Residual periodontal pockets are a risk indicator for peri-implantitis in patients treated for periodontitis. Clin. Oral. Implants Res. 23, 325–333. doi: 10.1111/j.1600-0501.2011.02264.x
Ciantar, M., Gilthorpe, M. S., Hurel, S. J., Newman, H. N., Wilson, M., Spratt, D. A. (2005). Capnocytophaga spp. in periodontitis patients manifesting diabetes mellitus. J. Periodontol 76, 194–203. doi: 10.1902/jop.2005.76.2.194
Cobo, J., Miguel, L. G., Euba, G., Rodríguez, D., García-Lechuz, J. M., Riera, M., et al. (2011). Early prosthetic joint infection: outcomes with debridement and implant retention followed by antibiotic therapy. Clin. Microbiol. Infect. 17, 1632–1637. doi: 10.1111/j.1469-0691.2010.03333.x
Cosgarea, R., Roccuzzo, A., Jepsen, K., Sculean, A., Jepsen, S., Salvi, G. E. (2023). Efficacy of mechanical/physical approaches for implant surface decontamination in non-surgical submarginal instrumentation of peri-implantitis. A systematic review. J. Clin. Periodontol 50 Suppl 26, 188–211. doi: 10.1111/jcpe.13762
Cugini, C., Shanmugam, M., Landge, N., Ramasubbu, N. (2019). The role of exopolysaccharides in oral biofilms. J. Dent. Res. 98, 739–745. doi: 10.1177/0022034519845001
D’Ovidio, C., Carnevale, A., Pantaleone, G., Piattelli, A., Di Bonaventura, G. (2011). First report of an acute purulent maxillary sinusitis caused by Pseudomonas aeruginosa secondary to dental implant placement in an immunocompetent patient. Br. Dent. J. 211, 205–207. doi: 10.1038/sj.bdj.2011.723
Dabdoub, S. M., Tsigarida, A. A., Kumar, P. S. (2013). Patient-specific analysis of periodontal and peri-implant microbiomes. J. Dent. Res. 92, 168s–175s. doi: 10.1177/0022034513504950
Del Pilar Angarita-Díaz, M., Fong, C., Medina, D. (2024). Bacteria of healthy periodontal tissues as candidates of probiotics: a systematic review. Eur. J. Med. Res. 29, 328. doi: 10.1186/s40001-024-01908-2
de Mello-Neto, J. M., Nunes, J. G. R., Tadakamadla, S. K., da Silva Figueredo, C. M. (2021). Immunological traits of patients with coexistent inflammatory bowel disease and periodontal disease: A systematic review. Int. J. Environ. Res. Public Health 18. doi: 10.3390/ijerph18178958
Dentino, A., Lee, S., Mailhot, J., Hefti, A. F. (2013). Principles of periodontology. Periodontol 2000 61, 16–53. doi: 10.1111/j.1600-0757.2011.00397.x
Di Stefano, M., Polizzi, A., Santonocito, S., Romano, A., Lombardi, T., Isola, G. (2022). Impact of oral microbiome in periodontal health and periodontitis: A critical review on prevention and treatment. Int. J. Mol. Sci. 23. doi: 10.3390/ijms23095142
Domagala-Kulawik, J. (2008). Effects of cigarette smoke on the lung and systemic immunity. J. Physiol. Pharmacol. 59 Suppl 6, 19–34.
Ettinger, R., Sims, G. P., Robbins, R., Withers, D., Fischer, R. T., Grammer, A. C., et al. (2007). IL-21 and BAFF/BLyS synergize in stimulating plasma cell differentiation from a unique population of human splenic memory B cells. J. Immunol. 178, 2872–2882. doi: 10.4049/jimmunol.178.5.2872
Fatahi-Bafghi, M. (2021). Characterization of the Rothia spp. and their role in human clinical infections. Infect. Genet. Evol. 93, 104877. doi: 10.1016/j.meegid.2021.104877
Feng, Z., Zhu, J., Zhang, L., Li, C., Su, D., Wang, H., et al. (2024). Microbiological and functional traits of peri-implant mucositis and correlation with disease severity. mSphere 9, e0005924. doi: 10.1128/msphere.00059-24
Figueredo, C. M., Martins, A. P., Lira-Junior, R., Menegat, J. B., Carvalho, A. T., Fischer, R. G., et al. (2017). Activity of inflammatory bowel disease influences the expression of cytokines in gingival tissue. Cytokine 95, 1–6. doi: 10.1016/j.cyto.2017.01.016
Fine, D. H., Markowitz, K., Furgang, D., Fairlie, K., Ferrandiz, J., Nasri, C., et al. (2007). Aggregatibacter actinomycetemcomitans and its relationship to initiation of localized aggressive periodontitis: longitudinal cohort study of initially healthy adolescents. J. Clin. Microbiol. 45, 3859–3869. doi: 10.1128/jcm.00653-07
Fine, D. H., Markowitz, K., Furgang, D., Velliyagounder, K. (2010). Aggregatibacter actinomycetemcomitans as an early colonizer of oral tissues: epithelium as a reservoir? J. Clin. Microbiol. 48, 4464–4473. doi: 10.1128/jcm.00964-10
Gasmi Benahmed, A., Kumar Mujawdiya, P., Noor, S., Gasmi, A. (2022). Porphyromonas gingivalis in the development of periodontitis: impact on dysbiosis and inflammation. Arch. Razi Inst 77, 1539–1551. doi: 10.22092/ari.2021.356596.1875
Gellman, R. H., Olm, M. R., Terrapon, N., Enam, F., Higginbottom, S. K., Sonnenburg, J. L., et al. (2023). Hadza Prevotella require diet-derived microbiota-accessible carbohydrates to persist in mice. Cell Rep. 42. doi: 10.1016/j.celrep.2023.113233
Gholizadeh, P., Pormohammad, A., Eslami, H., Shokouhi, B., Fakhrzadeh, V., Kafil, H. S. (2017). Oral pathogenesis of Aggregatibacter actinomycetemcomitans. Microb. Pathog. 113, 303–311. doi: 10.1016/j.micpath.2017.11.001
Giro, G., Tebar, A., Franco, L., Racy, D., Bastos, M. F., Shibli, J. A. (2021). Treg and TH17 link to immune response in individuals with peri-implantitis: a preliminary report. Clin. Oral. Investig. 25, 1291–1297. doi: 10.1007/s00784-020-03435-w
Gonçalves, L. F., Fermiano, D., Feres, M., Figueiredo, L. C., Teles, F. R., Mayer, M. P., et al. (2012). Levels of Selenomonas species in generalized aggressive periodontitis. J. Periodontal Res. 47, 711–718. doi: 10.1111/j.1600-0765.2012.01485.x
Gupta, A., Shivachandran, A., Saleena, L. M. (2024). Oral microbiome insights: Tracing acidic culprits in dental caries with functional metagenomics. Arch. Oral. Biol. 168, 106064. doi: 10.1016/j.archoralbio.2024.106064
Hajishengallis, G. (2014). Immunomicrobial pathogenesis of periodontitis: keystones, pathobionts, and host response. Trends Immunol. 35, 3–11. doi: 10.1016/j.it.2013.09.001
Hajishengallis, G. (2015). Periodontitis: from microbial immune subversion to systemic inflammation. Nat. Rev. Immunol. 15, 30–44. doi: 10.1038/nri3785
Hajishengallis, G. (2020). New developments in neutrophil biology and periodontitis. Periodontol 2000 82, 78–92. doi: 10.1111/prd.12313
Hajishengallis, G., Chavakis, T. (2013). Endogenous modulators of inflammatory cell recruitment. Trends Immunol. 34, 1–6. doi: 10.1016/j.it.2012.08.003
Henderson, B., Ward, J. M., Ready, D. (2010). Aggregatibacter (Actinobacillus) actinomycetemcomitans: a triple A* periodontopathogen? Periodontol 2000 54, 78–105. doi: 10.1111/j.1600-0757.2009.00331.x
Herrera, D., Sanz, M., Jepsen, S., Needleman, I., Roldán, S. (2002). A systematic review on the effect of systemic antimicrobials as an adjunct to scaling and root planing in periodontitis patients. J. Clin. Periodontol 29 Suppl 3, 136–159. doi: 10.1034/j.1600-051x.29.s3.8.x
Hickman-Brecks, C. L., Racz, J. L., Meyer, D. M., LaBranche, T. P., Allen, P. M. (2011). Th17 cells can provide B cell help in autoantibody induced arthritis. J. Autoimmun 36, 65–75. doi: 10.1016/j.jaut.2010.10.007
Holmlund, A., Hänström, L., Lerner, U. H. (2004). Bone resorbing activity and cytokine levels in gingival crevicular fluid before and after treatment of periodontal disease. J. Clin. Periodontol 31, 475–482. doi: 10.1111/j.1600-051X.2004.00504.x
Horiuchi, A., Kokubu, E., Warita, T., Ishihara, K. (2020). Synergistic biofilm formation by Parvimonas micra and Fusobacterium nucleatum. Anaerobe 62, 102100. doi: 10.1016/j.anaerobe.2019.102100
Huang, C., Shi, G. (2019). Smoking and microbiome in oral, airway, gut and some systemic diseases. J. Transl. Med. 17, 225. doi: 10.1186/s12967-019-1971-7
Huang, X., Xie, M., Xie, Y., Mei, F., Lu, X., Li, X., et al. (2020). The roles of osteocytes in alveolar bone destruction in periodontitis. J. Transl. Med. 18, 479. doi: 10.1186/s12967-020-02664-7
Hultin, M., Gustafsson, A., Hallström, H., Johansson, L. A., Ekfeldt, A., Klinge, B. (2002). Microbiological findings and host response in patients with peri-implantitis. Clin. Oral. Implants Res. 13, 349–358. doi: 10.1034/j.1600-0501.2002.130402.x
Idate, U., Bhat, K., Kotrashetti, V., Kugaji, M., Kumbar, V. (2020). Molecular identification of Capnocytophaga species from the oral cavity of patients with chronic periodontitis and healthy individuals. J. Oral. Maxillofac. Pathol. 24, 397. doi: 10.4103/jomfp.JOMFP_33_20
İnce, G., Gürsoy, H., İpçi Ş, D., Cakar, G., Emekli-Alturfan, E., Yılmaz, S. (2015). Clinical and biochemical evaluation of lozenges containing lactobacillus reuteri as an adjunct to non-surgical periodontal therapy in chronic periodontitis. J. Periodontol 86, 746–754. doi: 10.1902/jop.2015.140612
Ingendoh-Tsakmakidis, A., Eberhard, J., Falk, C. S., Stiesch, M., Winkel, A. (2020). In vitro effects of streptococcus oralis biofilm on peri-implant soft tissue cells. Cells 9. doi: 10.3390/cells9051226
Ionescu, A., Wutscher, E., Brambilla, E., Schneider-Feyrer, S., Giessibl, F. J., Hahnel, S. (2012). Influence of surface properties of resin-based composites on in vitro Streptococcus mutans biofilm development. Eur. J. Oral. Sci. 120, 458–465. doi: 10.1111/j.1600-0722.2012.00983.x
Jia, L., Han, N., Du, J., Guo, L., Luo, Z., Liu, Y. (2019). Pathogenesis of Important Virulence Factors of Porphyromonas gingivalis via Toll-Like Receptors. Front. Cell Infect. Microbiol. 9. doi: 10.3389/fcimb.2019.00262
Johansson, A. (2011). Aggregatibacter actinomycetemcomitans leukotoxin: a powerful tool with capacity to cause imbalance in the host inflammatory response. Toxins (Basel) 3, 242–259. doi: 10.3390/toxins3030242
Jung, J. E., Cai, J. N., Cho, S. D., Song, K. Y., Jeon, J. G. (2016). Influence of fluoride on the bacterial composition of a dual-species biofilm composed of Streptococcus mutans and Streptococcus oralis. Biofouling 32, 1079–1087. doi: 10.1080/08927014.2016.1230607
Jung, H. J., Lee, W. (2023). Difference in microbiome compositions of healthy peri-implant sulcus and peri-implantitis sulcus from the same patient. Heliyon 9, e20303. doi: 10.1016/j.heliyon.2023.e20303
Kadowaki, T. (2021). Enzymatic characteristics and activities of gingipains from porphyromonas gingivalis. Methods Mol. Biol. 2210, 97–112. doi: 10.1007/978-1-0716-0939-2_10
Kapil, V., Milsom, A. B., Okorie, M., Maleki-Toyserkani, S., Akram, F., Rehman, F., et al. (2010). Inorganic nitrate supplementation lowers blood pressure in humans: role for nitrite-derived NO. Hypertension 56, 274–281. doi: 10.1161/hypertensionaha.110.153536
Kataoka, H., Taniguchi, M., Fukamachi, H., Arimoto, T., Morisaki, H., Kuwata, H. (2014). Rothia dentocariosa induces TNF-alpha production in a TLR2-dependent manner. Pathog. Dis. 71, 65–68. doi: 10.1111/2049-632x.12115
Kawai, T., Matsuyama, T., Hosokawa, Y., Makihira, S., Seki, M., Karimbux, N. Y., et al. (2006). B and T lymphocytes are the primary sources of RANKL in the bone resorptive lesion of periodontal disease. Am. J. Pathol. 169, 987–998. doi: 10.2353/ajpath.2006.060180
Kelk, P., Abd, H., Claesson, R., Sandström, G., Sjöstedt, A., Johansson, A. (2011). Cellular and molecular response of human macrophages exposed to Aggregatibacter actinomycetemcomitans leukotoxin. Cell Death Dis. 2, e126. doi: 10.1038/cddis.2011.6
Kelk, P., Claesson, R., Hänström, L., Lerner, U. H., Kalfas, S., Johansson, A. (2005). Abundant secretion of bioactive interleukin-1beta by human macrophages induced by Actinobacillus actinomycetemcomitans leukotoxin. Infect. Immun. 73, 453–458. doi: 10.1128/iai.73.1.453-458.2005
Kelk, P., Johansson, A., Claesson, R., Hänström, L., Kalfas, S. (2003). Caspase 1 involvement in human monocyte lysis induced by Actinobacillus actinomycetemcomitans leukotoxin. Infect. Immun. 71, 4448–4455. doi: 10.1128/iai.71.8.4448-4455.2003
Khemwong, T., Kobayashi, H., Ikeda, Y., Matsuura, T., Sudo, T., Kano, C., et al. (2019). Fretibacterium sp. human oral taxon 360 is a novel biomarker for periodontitis screening in the Japanese population. PloS One 14, e0218266. doi: 10.1371/journal.pone.0218266
Khocht, A., Orlich, M., Paster, B., Bellinger, D., Lenoir, L., Irani, C., et al. (2021). Cross-sectional comparisons of subgingival microbiome and gingival fluid inflammatory cytokines in periodontally healthy vegetarians versus non-vegetarians. J. Periodontal Res. 56, 1079–1090. doi: 10.1111/jre.12922
Kitamoto, S., Nagao-Kitamoto, H., Jiao, Y., Gillilland, M. G., 3rd, Hayashi, A., Imai, J., et al. (2020). The intermucosal connection between the mouth and gut in commensal pathobiont-driven colitis. Cell 182, 447–462.e414. doi: 10.1016/j.cell.2020.05.048
Klarström Engström, K., Khalaf, H., Kälvegren, H., Bengtsson, T. (2015). The role of Porphyromonas gingivalis gingipains in platelet activation and innate immune modulation. Mol. Oral. Microbiol. 30, 62–73. doi: 10.1111/omi.12067
Kolenbrander, P. E., Palmer, R. J., Jr., Periasamy, S., Jakubovics, N. S. (2010). Oral multispecies biofilm development and the key role of cell-cell distance. Nat. Rev. Microbiol. 8, 471–480. doi: 10.1038/nrmicro2381
Kõll-Klais, P., Mändar, R., Leibur, E., Marcotte, H., Hammarström, L., Mikelsaar, M. (2005). Oral lactobacilli in chronic periodontitis and periodontal health: species composition and antimicrobial activity. Oral. Microbiol. Immunol. 20, 354–361. doi: 10.1111/j.1399-302X.2005.00239.x
Komatsu, K., Shiba, T., Takeuchi, Y., Watanabe, T., Koyanagi, T., Nemoto, T., et al. (2020). Discriminating microbial community structure between peri-implantitis and periodontitis with integrated metagenomic, metatranscriptomic, and network analysis. Front. Cell Infect. Microbiol. 10. doi: 10.3389/fcimb.2020.596490
Kotake, S., Yago, T., Kawamoto, M., Nanke, Y. (2012). Role of osteoclasts and interleukin-17 in the pathogenesis of rheumatoid arthritis: crucial ‘human osteoclastology’. J. Bone Miner Metab. 30, 125–135. doi: 10.1007/s00774-011-0321-5
Kumar, P. S. (2019). Systemic risk factors for the development of periimplant diseases. Implant Dent. 28, 115–119. doi: 10.1097/id.0000000000000873
Kumar, P. S., Mason, M. R., Brooker, M. R., O’Brien, K. (2012). Pyrosequencing reveals unique microbial signatures associated with healthy and failing dental implants. J. Clin. Periodontol 39, 425–433. doi: 10.1111/j.1600-051X.2012.01856.x
L’Heureux, J. E., van der Giezen, M., Winyard, P. G., Jones, A. M., Vanhatalo, A. (2023). Localisation of nitrate-reducing and highly abundant microbial communities in the oral cavity. PloS One 18, e0295058. doi: 10.1371/journal.pone.0295058
Laleman, I., Yilmaz, E., Ozcelik, O., Haytac, C., Pauwels, M., Herrero, E. R., et al. (2015). The effect of a streptococci containing probiotic in periodontal therapy: a randomized controlled trial. J. Clin. Periodontol 42, 1032–1041. doi: 10.1111/jcpe.12464
Lauritano, D., Carinci, F., Palmieri, A., Cura, F., Caruso, S., Candotto, V. (2019). Reuterinos(®) as adjuvant for peri-implant treatment: A pilot study. Int. J. Immunopathol. Pharmacol. 33, 2058738419827745. doi: 10.1177/2058738419827745
Li, Y., Carrera, C., Chen, R., Li, J., Lenton, P., Rudney, J. D., et al. (2014). Degradation in the dentin-composite interface subjected to multi-species biofilm challenges. Acta Biomater 10, 375–383. doi: 10.1016/j.actbio.2013.08.034
Li, Q., Tan, L., Wang, H., Kou, Y., Shi, X., Zhang, S., et al. (2020). Fusobacterium nucleatum Interaction with Pseudomonas aeruginosa Induces Biofilm-Associated Antibiotic Tolerance via Fusobacterium Adhesin A. ACS Infect. Dis. 6, 1686–1696. doi: 10.1021/acsinfecdis.9b00402
Lin, D., Li, L., Sun, Y., Wang, W., Wang, X., Ye, Y., et al. (2014). IL-17 regulates the expressions of RANKL and OPG in human periodontal ligament cells via TRAF6/TBK1-JNK/NF-κB pathways. Immunology 144, 472–485. doi: 10.1111/imm.12395
Lin, T., Yu, C. C., Liu, C. M., Hsieh, P. L., Liao, Y. W., Yu, C. H., et al. (2021). Er: YAG laser promotes proliferation and wound healing capacity of human periodontal ligament fibroblasts through Galectin-7 induction. J. Formos Med. Assoc. 120, 388–394. doi: 10.1016/j.jfma.2020.06.005
Lindhe, J., Meyle, J. (2008). Peri-implant diseases: consensus report of the sixth european workshop on periodontology. J. Clin. Periodontol 35, 282–285. doi: 10.1111/j.1600-051X.2008.01283.x
Ling, M. R., Chapple, I. L., Matthews, J. B. (2015). Peripheral blood neutrophil cytokine hyper-reactivity in chronic periodontitis. Innate Immun. 21, 714–725. doi: 10.1177/1753425915589387
Liu, Y., Huang, W., Wang, J., Ma, J., Zhang, M., Lu, X., et al. (2021). Multifaceted impacts of periodontal pathogens in disorders of the intestinal barrier. Front. Immunol. 12. doi: 10.3389/fimmu.2021.693479
Liy, Y., Dan, J., Tao, H., Xuedong, Z. (2008). Regulation of urease expression of Actinomyces naeslundii in biofilms in response to pH and carbohydrate. Oral. Microbiol. Immunol. 23, 315–319. doi: 10.1111/j.1399-302X.2008.00430.x
Lunar Silva, I., Cascales, E. (2021). Molecular strategies underlying porphyromonas gingivalis virulence. J. Mol. Biol. 433, 166836. doi: 10.1016/j.jmb.2021.166836
Majzoub, J., Salami, A., Barootchi, S., Tavelli, L., Chan, H. L., Wang, H. L. (2021). The effect of non-surgical and surgical mechanical root debridement on infrabony defects: a retrospective study. Sci. Rep. 11, 19856. doi: 10.1038/s41598-021-99205-z
Mao, C., Xiang, Y., Liu, X., Zheng, Y., Yeung, K. W. K., Cui, Z., et al. (2019). Local photothermal/photodynamic synergistic therapy by disrupting bacterial membrane to accelerate reactive oxygen species permeation and protein leakage. ACS Appl. Mater Interfaces 11, 17902–17914. doi: 10.1021/acsami.9b05787
Martorano-Fernandes, L., Rodrigues, N. C., de Souza Borges, M. H., Cavalcanti, Y. W., de Almeida, L. F. D. (2020). Interkingdom interaction between C. albicans and S. salivarius on titanium surfaces. BMC Oral. Health 20, 349. doi: 10.1186/s12903-020-01334-w
Maruyama, N., Maruyama, F., Takeuchi, Y., Aikawa, C., Izumi, Y., Nakagawa, I. (2014). Intraindividual variation in core microbiota in peri-implantitis and periodontitis. Sci. Rep. 4, 6602. doi: 10.1038/srep06602
Mazurel, D., Carda-Diéguez, M., Langenburg, T., Žiemytė, M., Johnston, W., Martínez, C. P., et al. (2023). Nitrate and a nitrate-reducing Rothia aeria strain as potential prebiotic or synbiotic treatments for periodontitis. NPJ Biofilms Microbiomes 9, 40. doi: 10.1038/s41522-023-00406-3
McEachern, E. K., Hwang, J. H., Sladewski, K. M., Nicatia, S., Dewitz, C., Mathew, D. P., et al. (2015). Analysis of the effects of cigarette smoke on staphylococcal virulence phenotypes. Infect. Immun. 83, 2443–2452. doi: 10.1128/iai.00303-15
Meuric, V., Le-Gall-David, S., Boyer, E., Acuña-Amador, L., Martin, B., Fong, S. B., et al. (2017). Signature of microbial dysbiosis in periodontitis. Appl. Environ. Microbiol. 83. doi: 10.1128/aem.00462-17
Miossec, P., Kolls, J. K. (2012). Targeting IL-17 and TH17 cells in chronic inflammation. Nat. Rev. Drug Discovery 11, 763–776. doi: 10.1038/nrd3794
Miyoshi, T., Oge, S., Nakata, S., Ueno, Y., Ukita, H., Kousaka, R., et al. (2021). Gemella haemolysans inhibits the growth of the periodontal pathogen Porphyromonas gingivalis. Sci. Rep. 11, 11742. doi: 10.1038/s41598-021-91267-3
Mombelli, A. (2018). Microbial colonization of the periodontal pocket and its significance for periodontal therapy. Periodontol 2000 76, 85–96. doi: 10.1111/prd.12147
Mombelli, A., Hashim, D., Cionca, N. (2018). What is the impact of titanium particles and biocorrosion on implant survival and complications? A critical review. Clin. Oral. Implants Res. 29 Suppl 18, 37–53. doi: 10.1111/clr.13305
Morales-Fernández, L., Fernández-Crehuet, M., Espigares, M., Moreno, E., Espigares, E. (2014). Study of the hormetic effect of disinfectants chlorhexidine, povidone iodine and benzalkonium chloride. Eur. J. Clin. Microbiol. Infect. Dis. 33, 103–109. doi: 10.1007/s10096-013-1934-5
Moutsopoulos, N. M., Konkel, J. E. (2018). Tissue-specific immunity at the oral mucosal barrier. Trends Immunol. 39, 276–287. doi: 10.1016/j.it.2017.08.005
Muhvić-Urek, M., Tomac-Stojmenović, M., Mijandrušić-Sinčić, B. (2016). Oral pathology in inflammatory bowel disease. World J. Gastroenterol. 22, 5655–5667. doi: 10.3748/wjg.v22.i25.5655
Mulla, M., Mulla, M., Hegde, S., Koshy, A. V. (2021). In vitro assessment of the effect of probiotic lactobacillus reuteri on peri-implantitis microflora. BMC Oral. Health 21, 408. doi: 10.1186/s12903-021-01762-2
Mysak, J., Podzimek, S., Sommerova, P., Lyuya-Mi, Y., Bartova, J., Janatova, T., et al. (2014). Porphyromonas gingivalis: major periodontopathic pathogen overview. J. Immunol. Res. 2014, 476068. doi: 10.1155/2014/476068
Ng, M. Y., Yu, C. C., Chen, S. H., Liao, Y. W., Lin, T. (2024). Er: YAG laser alleviates inflammaging in diabetes-associated periodontitis via activation CTBP1-AS2/miR-155/SIRT1 axis. Int. J. Mol. Sci. 25. doi: 10.3390/ijms25042116
O’Sullivan, D., King, P., Jagger, D. (2006). Osteomyelitis and pathological mandibular fracture related to a late implant failure: a clinical report. J. Prosthet Dent. 95, 106–110. doi: 10.1016/j.prosdent.2005.10.014
Ogita, M., Tsuchida, S., Aoki, A., Satoh, M., Kado, S., Sawabe, M., et al. (2015). Increased cell proliferation and differential protein expression induced by low-level Er: YAG laser irradiation in human gingival fibroblasts: proteomic analysis. Lasers Med. Sci. 30, 1855–1866. doi: 10.1007/s10103-014-1691-4
Ong, H. S., Oettinger-Barak, O., Dashper, S. G., Darby, I. B., Tan, K. H., Reynolds, E. C. (2017). Effect of azithromycin on a red complex polymicrobial biofilm. J. Oral. Microbiol. 9, 1339579. doi: 10.1080/20002297.2017.1339579
Padial-Molina, M., López-Martínez, J., O’Valle, F., Galindo-Moreno, P. (2016). Microbial profiles and detection techniques in peri-implant diseases: a systematic review. J. Oral. Maxillofac. Res. 7, e10. doi: 10.5037/jomr.2016.7310
Pan, W., Wang, Q., Chen, Q. (2019). The cytokine network involved in the host immune response to periodontitis. Int. J. Oral. Sci. 11, 30. doi: 10.1038/s41368-019-0064-z
Pani, P., Tsilioni, I., McGlennen, R., Brown, C. A., Hawley, C. E., Theoharides, T. C., et al. (2021). IL-1B(3954) polymorphism and red complex bacteria increase IL-1β (GCF) levels in periodontitis. J. Periodontal Res. 56, 501–511. doi: 10.1111/jre.12850
Papapanou, P. N., Sanz, M., Buduneli, N., Dietrich, T., Feres, M., Fine, D. H., et al. (2018). Periodontitis: Consensus report of workgroup 2 of the 2017 World Workshop on the Classification of Periodontal and Peri-Implant Diseases and Conditions. J. Periodontol 89 Suppl 1, S173–s182. doi: 10.1002/jper.17-0721
Park, W. B., Han, J. Y., Oh, S. L. (2019). Maxillary sinusitis associated with peri-implantitis at sinus floor augmented sites: case series. Implant Dent. 28, 484–489. doi: 10.1097/id.0000000000000922
Park, K. K., Heuner, K., Göbel, U. B., Yoo, Y. J., Kim, C. K., Choi, B. K. (2002). Cloning and characterization of a major surface protein (MspTL) of Treponema lecithinolyticum associated with rapidly progressive periodontitis. FEMS Microbiol. Lett. 207, 185–192. doi: 10.1111/j.1574-6968.2002.tb11049.x
Payne, J. B., Johnson, P. G., Kok, C. R., Gomes-Neto, J. C., Ramer-Tait, A. E., Schmid, M. J., et al. (2017). Subgingival microbiome colonization and cytokine production during early dental implant healing. mSphere 2. doi: 10.1128/mSphereDirect.00527-17
Peña, M., Barallat, L., Vilarrasa, J., Vicario, M., Violant, D., Nart, J. (2019). Evaluation of the effect of probiotics in the treatment of peri-implant mucositis: a triple-blind randomized clinical trial. Clin. Oral. Investig. 23, 1673–1683. doi: 10.1007/s00784-018-2578-8
Pérez-Chaparro, P. J., Duarte, P. M., Shibli, J. A., Montenegro, S., Lacerda Heluy, S., Figueiredo, L. C., et al. (2016). The current weight of evidence of the microbiologic profile associated with peri-implantitis: A systematic review. J. Periodontol 87, 1295–1304. doi: 10.1902/jop.2016.160184
Pérez-Chaparro, P. J., McCulloch, J. A., Mamizuka, E. M., Moraes, A., Faveri, M., Figueiredo, L. C., et al. (2018). Do different probing depths exhibit striking differences in microbial profiles? J. Clin. Periodontol 45, 26–37. doi: 10.1111/jcpe.12811
Pettersson, M., Kelk, P., Belibasakis, G. N., Bylund, D., Molin Thorén, M., Johansson, A. (2017). Titanium ions form particles that activate and execute interleukin-1β release from lipopolysaccharide-primed macrophages. J. Periodontal Res. 52, 21–32. doi: 10.1111/jre.12364
Pignatelli, P., Fabietti, G., Ricci, A., Piattelli, A., Curia, M. C. (2020). How periodontal disease and presence of nitric oxide reducing oral bacteria can affect blood pressure. Int. J. Mol. Sci. 21. doi: 10.3390/ijms21207538
Pjetursson, B. E., Helbling, C., Weber, H. P., Matuliene, G., Salvi, G. E., Brägger, U., et al. (2012). Peri-implantitis susceptibility as it relates to periodontal therapy and supportive care. Clin. Oral. Implants Res. 23, 888–894. doi: 10.1111/j.1600-0501.2012.02474.x
Rammos, C., Luedike, P., Hendgen-Cotta, U., Rassaf, T. (2015). Potential of dietary nitrate in angiogenesis. World J. Cardiol. 7, 652–657. doi: 10.4330/wjc.v7.i10.652
Reyes, L. (2021). Porphyromonas gingivalis. Trends Microbiol. 29, 376–377. doi: 10.1016/j.tim.2021.01.010
Richards, A. M., Abu Kwaik, Y., Lamont, R. J. (2015). Code blue: Acinetobacter baumannii, a nosocomial pathogen with a role in the oral cavity. Mol. Oral. Microbiol. 30, 2–15. doi: 10.1111/omi.12072
Rinaldi, S., Pieper, E., Schulz, T., Zimmermann, R., Luch, A., Laux, P., et al. (2023). Oral nicotine pouches with an aftertaste? Part 2: in vitro toxicity in human gingival fibroblasts. Arch. Toxicol. 97, 2343–2356. doi: 10.1007/s00204-023-03554-9
Roberts, F. A., Darveau, R. P. (2015). Microbial protection and virulence in periodontal tissue as a function of polymicrobial communities: symbiosis and dysbiosis. Periodontol 2000 69, 18–27. doi: 10.1111/prd.12087
Rogers, E. A., Das, A., Ton-That, H. (2011). Adhesion by pathogenic corynebacteria. Adv. Exp. Med. Biol. 715, 91–103. doi: 10.1007/978-94-007-0940-9_6
Roos-Jansåker, A. M., Lindahl, C., Renvert, H., Renvert, S. (2006). Nine- to fourteen-year follow-up of implant treatment. Part I: implant loss and associations to various factors. J. Clin. Periodontol 33, 283–289. doi: 10.1111/j.1600-051X.2006.00907.x
Rosier, B. T., Buetas, E., Moya-Gonzalvez, E. M., Artacho, A., Mira, A. (2020). Nitrate as a potential prebiotic for the oral microbiome. Sci. Rep. 10, 12895. doi: 10.1038/s41598-020-69931-x
Rosier, B. T., Takahashi, N., Zaura, E., Krom, B. P., MartÍnez-Espinosa, R. M., van Breda, S. G. J., et al. (2022). The importance of nitrate reduction for oral health. J. Dent. Res. 101, 887–897. doi: 10.1177/00220345221080982
Ross, K. F., Herzberg, M. C. (2016). Autonomous immunity in mucosal epithelial cells: fortifying the barrier against infection. Microbes Infect. 18, 387–398. doi: 10.1016/j.micinf.2016.03.008
Ruscitto, A., Sharma, A. (2018). Peptidoglycan synthesis in Tannerella forsythia: Scavenging is the modus operandi. Mol. Oral. Microbiol. 33, 125–132. doi: 10.1111/omi.12210
Salerno, C., Pascale, M., Contaldo, M., Esposito, V., Busciolano, M., Milillo, L., et al. (2011). Candida-associated denture stomatitis. Med. Oral. Patol Oral. Cir Bucal 16, e139–e143. doi: 10.4317/medoral.16.e139
Sanz, M., Herrera, D., Kebschull, M., Chapple, I., Jepsen, S., Beglundh, T., et al. (2020). Treatment of stage I-III periodontitis-The EFP S3 level clinical practice guideline. J. Clin. Periodontol 47 Suppl 22, 4–60. doi: 10.1111/jcpe.13290
Schaumann, S., Staufenbiel, I., Scherer, R., Schilhabel, M., Winkel, A., Stumpp, S. N., et al. (2014). Pyrosequencing of supra- and subgingival biofilms from inflamed peri-implant and periodontal sites. BMC Oral. Health 14, 157. doi: 10.1186/1472-6831-14-157
Schlagenhauf, U. (2022). On the role of dietary nitrate in the maintenance of systemic and oral health. Dent. J. (Basel) 10. doi: 10.3390/dj10050084
Sendra, E., Fernández-Muñoz, A., Zamorano, L., Oliver, A., Horcajada, J. P., Juan, C., et al. (2024). Impact of multidrug resistance on the virulence and fitness of Pseudomonas aeruginosa: a microbiological and clinical perspective. Infection 52, 1235–1268. doi: 10.1007/s15010-024-02313-x
Sgolastra, F., Petrucci, A., Severino, M., Gatto, R., Monaco, A. (2015). Periodontitis, implant loss and peri-implantitis. A meta-analysis. Clin. Oral. Implants Res. 26, e8–e16. doi: 10.1111/clr.12319
Shao, H., Demuth, D. R. (2010). Quorum sensing regulation of biofilm growth and gene expression by oral bacteria and periodontal pathogens. Periodontol 2000 52, 53–67. doi: 10.1111/j.1600-0757.2009.00318.x
Sharma, G., Garg, N., Hasan, S., Shirodkar, S. (2022). Prevotella: An insight into its characteristics and associated virulence factors. Microb. Pathog. 169, 105673. doi: 10.1016/j.micpath.2022.105673
Shen, X., Zhang, B., Hu, X., Li, J., Wu, M., Yan, C., et al. (2022). Neisseria sicca and Corynebacterium matruchotii inhibited oral squamous cell carcinomas by regulating genome stability. Bioengineered 13, 14094–14106. doi: 10.1080/21655979.2022.2078556
Shibli, J. A. (2018). Is laser the best choice for the treatment of peri-implantitis? Photomed Laser Surg. 36, 569–570. doi: 10.1089/pho.2018.4521
Shibli, J. A., Martins, M. C., Theodoro, L. H., Lotufo, R. F., Garcia, V. G., Marcantonio, E. J. (2003). Lethal photosensitization in microbiological treatment of ligature-induced peri-implantitis: a preliminary study in dogs. J. Oral. Sci. 45, 17–23. doi: 10.2334/josnusd.45.17
Socransky, S. S., Haffajee, A. D. (2005). Periodontal microbial ecology. Periodontol 2000 38, 135–187. doi: 10.1111/j.1600-0757.2005.00107.x
Socransky, S. S., Haffajee, A. D., Cugini, M. A., Smith, C., Kent, R. L., Jr (1998). Microbial complexes in subgingival plaque. J. Clin. Periodontol 25, 134–144. doi: 10.1111/j.1600-051x.1998.tb02419.x
Song, F., Koo, H., Ren, D. (2015). Effects of material properties on bacterial adhesion and biofilm formation. J. Dent. Res. 94, 1027–1034. doi: 10.1177/0022034515587690
Sousa, V., Spratt, D., Davrandi, M., Mardas, N., Beltrán, V., Donos, N. (2022). Oral microcosm biofilms grown under conditions progressing from peri-implant health, peri-implant mucositis, and peri-implantitis. Int. J. Environ. Res. Public Health 19. doi: 10.3390/ijerph192114088
Strauss, J., Kaplan, G. G., Beck, P. L., Rioux, K., Panaccione, R., Devinney, R., et al. (2011). Invasive potential of gut mucosa-derived Fusobacterium nucleatum positively correlates with IBD status of the host. Inflammation Bowel Dis. 17, 1971–1978. doi: 10.1002/ibd.21606
Struzycka, I. (2014). The oral microbiome in dental caries. Pol. J. Microbiol. 63, 127–135. doi: 10.33073/pjm-
Sumioka, R., Nakata, M., Okahashi, N., Li, Y., Wada, S., Yamaguchi, M., et al. (2017). Streptococcus sanguinis induces neutrophil cell death by production of hydrogen peroxide. PloS One 12, e0172223. doi: 10.1371/journal.pone.0172223
Suzuki, N., Yoneda, M., Hirofuji, T. (2013). Mixed red-complex bacterial infection in periodontitis. Int. J. Dent. 2013, 587279. doi: 10.1155/2013/587279
Szkaradkiewicz, A. K., Stopa, J., Karpiński, T. M. (2014). Effect of oral administration involving a probiotic strain of Lactobacillus reuteri on pro-inflammatory cytokine response in patients with chronic periodontitis. Arch. Immunol. Ther. Exp. (Warsz) 62, 495–500. doi: 10.1007/s00005-014-0277-y
Tamashiro, R., Strange, L., Schnackenberg, K., Santos, J., Gadalla, H., Zhao, L., et al. (2023). Smoking-induced subgingival dysbiosis precedes clinical signs of periodontal disease. Sci. Rep. 13, 3755. doi: 10.1038/s41598-023-30203-z
Tarbiah, N., Todd, I., Tighe, P. J., Fairclough, L. C. (2019). Cigarette smoking differentially affects immunoglobulin class levels in serum and saliva: An investigation and review. Basic Clin. Pharmacol. Toxicol. 125, 474–483. doi: 10.1111/bcpt.13278
Tekce, M., Ince, G., Gursoy, H., Dirikan Ipci, S., Cakar, G., Kadir, T., et al. (2015). Clinical and microbiological effects of probiotic lozenges in the treatment of chronic periodontitis: a 1-year follow-up study. J. Clin. Periodontol 42, 363–372. doi: 10.1111/jcpe.12387
Truong, V. K., Lapovok, R., Estrin, Y. S., Rundell, S., Wang, J. Y., Fluke, C. J., et al. (2010). The influence of nano-scale surface roughness on bacterial adhesion to ultrafine-grained titanium. Biomaterials 31, 3674–3683. doi: 10.1016/j.biomaterials.2010.01.071
Vacca, C., Contu, M. P., Rossi, C., Ferrando, M. L., Blus, C., Szmukler-Moncler, S., et al. (2020). In vitro Interactions between Streptococcus intermedius and Streptococcus salivarius K12 on a Titanium Cylindrical Surface. Pathogens 9. doi: 10.3390/pathogens9121069
Verma, R. K., Bhattacharyya, I., Sevilla, A., Lieberman, I., Pola, S., Nair, M., et al. (2010). Virulence of major periodontal pathogens and lack of humoral immune protection in a rat model of periodontal disease. Oral. Dis. 16, 686–695. doi: 10.1111/j.1601-0825.2010.01678.x
Violant, D., Galofré, M., Nart, J., Teles, R. P. (2014). In vitro evaluation of a multispecies oral biofilm on different implant surfaces. BioMed. Mater 9, 35007. doi: 10.1088/1748-6041/9/3/035007
Vivekananda, M. R., Vandana, K. L., Bhat, K. G. (2010). Effect of the probiotic Lactobacilli reuteri (Prodentis) in the management of periodontal disease: a preliminary randomized clinical trial. J. Oral. Microbiol. 2. doi: 10.3402/jom.v2i0.5344
Wang, F., Guan, M., Wei, L., Yan, H. (2019). IL−18 promotes the secretion of matrix metalloproteinases in human periodontal ligament fibroblasts by activating NF−κB signaling. Mol. Med. Rep. 19, 703–710. doi: 10.3892/mmr.2018.9697
Wang, J., Qi, J., Zhao, H., He, S., Zhang, Y., Wei, S., et al. (2013). Metagenomic sequencing reveals microbiota and its functional potential associated with periodontal disease. Sci. Rep. 3, 1843. doi: 10.1038/srep01843
Wang, S., Qin, L. (2022). Homeostatic medicine: a strategy for exploring health and disease. Curr. Med. (Cham) 1, 16. doi: 10.1007/s44194-022-00016-9
Wei, Y., Shi, M., Zhen, M., Wang, C., Hu, W., Nie, Y., et al. (2019). Comparison of subgingival and buccal mucosa microbiome in chronic and aggressive periodontitis: A pilot study. Front. Cell Infect. Microbiol. 9. doi: 10.3389/fcimb.2019.00053
Wicaksono, D. P., Washio, J., Abiko, Y., Domon, H., Takahashi, N. (2020). Nitrite production from nitrate and its link with lactate metabolism in oral veillonella spp. Appl. Environ. Microbiol. 86. doi: 10.1128/aem.01255-20
Williams, D. W., Greenwell-Wild, T., Brenchley, L., Dutzan, N., Overmiller, A., Sawaya, A. P., et al. (2021). Human oral mucosa cell atlas reveals a stromal-neutrophil axis regulating tissue immunity. Cell 184, 4090–4104.e4015. doi: 10.1016/j.cell.2021.05.013
Xiao, E., Mattos, M., Vieira, G. H. A., Chen, S., Corrêa, J. D., Wu, Y., et al. (2017). Diabetes enhances IL-17 expression and alters the oral microbiome to increase its pathogenicity. Cell Host Microbe 22, 120–128.e124. doi: 10.1016/j.chom.2017.06.014
Yamamoto, Y., Washio, J., Shimizu, K., Takahashi, N. (2024). Effects of Nitrate and Nitrite on Plaque pH Decrease and Nitrite-Producing and -Degrading Activities of Plaque in vitro. Caries Res. 58, 552–561. doi: 10.1159/000540017
Yang, Y., Li, S., Qu, Y., Wang, X., An, W., Li, Z., et al. (2020b). Nitrate partially inhibits lipopolysaccharide-induced inflammation by maintaining mitochondrial function. J. Int. Med. Res. 48, 300060520902605. doi: 10.1177/0300060520902605
Yang, L. C., Lin, S. W., Li, I. C., Chen, Y. P., Tzu, S. Y., Chou, W., et al. (2020a). Lactobacillus plantarum GKM3 and Lactobacillus paracasei GKS6 Supplementation Ameliorates Bone Loss in Ovariectomized Mice by Promoting Osteoblast Differentiation and Inhibiting Osteoclast Formation. Nutrients 12. doi: 10.3390/nu12071914
Yoshihara, K., Nagaoka, N., Maruo, Y., Sano, H., Yoshida, Y., Van Meerbeek, B. (2017). Bacterial adhesion not inhibited by ion-releasing bioactive glass filler. Dent. Mater 33, 723–734. doi: 10.1016/j.dental.2017.04.002
Yu, X. L., Chan, Y., Zhuang, L., Lai, H. C., Lang, N. P., Keung Leung, W., et al. (2019). Intra-oral single-site comparisons of periodontal and peri-implant microbiota in health and disease. Clin. Oral. Implants Res. 30, 760–776. doi: 10.1111/clr.13459
Zhang, S. M., Huang, S. L. (2023). The Commensal Anaerobe Veillonella dispar Reprograms Its Lactate Metabolism and Short-Chain Fatty Acid Production during the Stationary Phase. Microbiol. Spectr. 11, e0355822. doi: 10.1128/spectrum.03558-22
Zheng, X., Tizzano, M., Redding, K., He, J., Peng, X., Jiang, P., et al. (2019). Gingival solitary chemosensory cells are immune sentinels for periodontitis. Nat. Commun. 10, 4496. doi: 10.1038/s41467-019-12505-x
Zheng, H., Xu, L., Wang, Z., Li, L., Zhang, J., Zhang, Q., et al. (2015). Subgingival microbiome in patients with healthy and ailing dental implants. Sci. Rep. 5, 10948. doi: 10.1038/srep10948
Zheng, S., Yu, S., Fan, X., Zhang, Y., Sun, Y., Lin, L., et al. (2021). Porphyromonas gingivalis survival skills: Immune evasion. J. Periodontal Res. 56, 1007–1018. doi: 10.1111/jre.12915
Zhu, B., Macleod, L. C., Kitten, T., Xu, P. (2018). Streptococcus sanguinis biofilm formation & interaction with oral pathogens. Future Microbiol. 13, 915–932. doi: 10.2217/fmb-2018-0043
Keywords: periodontitis, peri-implantitis, oral microorganisms, immune homeostasis, microbial dysbiosis
Citation: Cui Z, Wang P and Gao W (2025) Microbial dysbiosis in periodontitis and peri-implantitis: pathogenesis, immune responses, and therapeutic. Front. Cell. Infect. Microbiol. 15:1517154. doi: 10.3389/fcimb.2025.1517154
Received: 25 October 2024; Accepted: 22 January 2025;
Published: 11 February 2025.
Edited by:
Pandu R. Gangula, Meharry Medical College, United StatesReviewed by:
Miguel Carda Diéguez, Fundación para el Fomento de la Investigación Sanitaria y Biomédica de la Comunitat Valenciana (FISABIO), SpainKabilan Velliyagounder, Rutgers, The State University of New Jersey, United States
Copyright © 2025 Cui, Wang and Gao. This is an open-access article distributed under the terms of the Creative Commons Attribution License (CC BY). The use, distribution or reproduction in other forums is permitted, provided the original author(s) and the copyright owner(s) are credited and that the original publication in this journal is cited, in accordance with accepted academic practice. No use, distribution or reproduction is permitted which does not comply with these terms.
*Correspondence: Weiyue Gao, Z2Fvd3kxMjIwQDE2My5jb20=