- 1Universidad UTE, Centro de Investigación Biomédica (CENBIO), Facultad de Ciencias de la Salud Eugenio Espejo, Quito, Ecuador
- 2Escuela de Medicina, Colegio de Ciencias de la Salud Quito, Universidad San Francisco de Quito USFQ, Quito, Ecuador
- 3Instituto de Microbiología, Universidad San Francisco de Quito USFQ, Quito, Ecuador
Biofilms are complex microbial communities in which planktonic and dormant bacteria are enveloped in extracellular polymeric substances (EPS) such as exopolysaccharides, proteins, lipids, and DNA. These multicellular structures present resistance to conventional antimicrobial treatments, including antibiotics. The formation of biofilms raises considerable concern in healthcare settings, biofilms can exacerbate infections in patients and compromise the integrity of medical devices employed during treatment. Similarly, certain bacterial species contribute to bulking, foaming, and biofilm development in water environments such as wastewater treatment plants, water reservoirs, and aquaculture facilities. Additionally, food production facilities provide ideal conditions for establishing bacterial biofilms, which can serve as reservoirs for foodborne pathogens. Efforts to combat antibiotic resistance involve exploring various strategies, including bacteriophage therapy. Research has been conducted on the effects of phages and their individual proteins to assess their potential for biofilm removal. However, challenges persist, prompting the examination of refined approaches such as drug-phage combination therapies, phage cocktails, and genetically modified phages for clinical applications. This review aims to highlight the progress regarding bacteriophage-based approaches for biofilm eradication in different settings.
1 Introduction
Biofilms are sophisticated microbial communities aggregated in a self-generated extracellular matrix, which anchors the cells together and facilitates communication and resource distribution among them (Penesyan et al., 2021). The architecture of biofilms endows them with distinct characteristics compared to planktonic (free-living) cells, including altered metabolism and enhanced resistance to external stressors (Lee et al., 2014; Yin et al., 2019).
The clinical significance of biofilms predominantly stems from their heightened resistance to antimicrobial agents, posing an enormous challenge in treating biofilm-associated infections (Schulze et al., 2021; Ali et al., 2023). Cells within biofilms exhibit antimicrobial resistance that can be up to a thousand times greater than that of planktonic cells (Mah and O’Toole, 2001; Sharma et al., 2019). Biofilms are implicated in a wide array of chronic infections and are responsible for the failure of numerous antimicrobial treatments, particularly in the context of medical device- and tissue-associated infections. The current treatment regimens, often relying on conventional antimicrobials, are increasingly proving inadequate in eradicating these resilient microbial communities (Usui et al., 2023).
Given the limitations of existing therapies and the escalating threat of multidrug-resistant microbes, there is an urgent need to develop novel anti-biofilm strategies. Innovative approaches, such as the use of antimicrobial peptides, nanotechnology, surface modifications of medical devices, and bacteriophage applications, are being explored to combat biofilm-associated infections (Yasir et al., 2018; Li et al., 2020; Mohanta et al., 2023; Kushwaha et al., 2024). Understanding the complex biology of biofilms and exploring bacteriophages as potential biocontrol agents are critical in addressing the biofilm-related challenges in medical and industrial environments. This review aims to provide a comprehensive overview of the basic biofilm biology, the resistance mechanisms within biofilms, and the emerging role of bacteriophages in biofilm control.
2 Biofilm formation
Biofilms, crucial in diverse environments, are complex microbial communities encapsulated in an extracellular polymeric substances (EPS) matrix (Li et al., 2022; Krishnan et al., 2023). Understanding each step of biofilm formation—from initial attachment through irreversible adhesion, microcolony formation, maturation, to dispersion—is crucial for developing effective eradication strategies. Each phase presents unique targets and mechanisms that can be disrupted to prevent biofilm formation or to dismantle existing biofilms. For instance, interventions at the initial attachment stage can prevent biofilm establishment, while strategies targeting biofilm maturation can disrupt the protective EPS matrix, enhancing the efficacy of antimicrobial agents. Therefore, detailed knowledge of these processes is essential for devising comprehensive and effective biofilm control measures, ultimately improving infection management and patient outcomes (Armbruster and Parsek, 2018; Nie et al., 2021).
1. Initial attachment: The initial phase involves microorganisms adhering to surfaces via physical forces (van der Waals forces, electrostatic attractions, hydrophobic effects). Bacterial appendages play a crucial role in surface movement, enabling the bacteria to explore and find optimal attachment sites. Additionally, pili, such as Type I and Type IV, facilitate adhesion by establishing strong interactions with the surface. These initial interactions are reversible, allowing the bacteria to detach and relocate if the conditions are unfavorable (Richter et al., 2017; Ramzan et al., 2022).
2. Irreversible attachment: The transition to irreversible attachment is marked by the production of EPS, which anchors the bacteria firmly to the surface. This EPS matrix not only strengthens adhesion but also forms a protective barrier that shields the microbial community from environmental stressors, including antimicrobial agents and components of the immune response. During this phase, these microorganisms can undergo significant genetic shifts, prioritizing the synthesis of EPS and reducing the expression of motility-related genes (Chávez-Jacobo et al., 2023; Guttenplan et al., 2010; Kim et al., 2023a).
3. Microcolony formation: As bacteria continue to produce EPS and proliferate, they form structured microcolonies, which are clusters of bacterial cells within the EPS matrix. This stage is characterized by spatial organization and differentiation within the biofilm, influenced by environmental gradients such as nutrient availability, oxygen concentration, and waste accumulation. Quorum sensing, a key cell-density signaling mechanism, is crucial in regulating gene expression associated with EPS production and biofilm maturation. This process ensures coordinated behavior among the bacterial population, facilitating efficient biofilm development (Zhang et al., 2018; Oyewole et al., 2022; Gulec and Eckford, 2023).
4. Biofilm maturation: In this phase, microcolonies merge, creating a layered, three-dimensional structure. EPS is crucial for structure and protection, creating diverse microenvironments within the biofilm. These niches allow for metabolic specialization among microbial populations. Additionally, the development of water channels, which occurs in this phase, is essential for nutrient distribution and waste removal, while quorum sensing ensures coordinated gene expression throughout the biofilm, regulating behaviors like virulence and resistance (Azulay et al., 2022; Xiu et al., 2022).
5. Dispersion: During this phase, cells detach from the mature biofilm to colonize new surfaces through passive mechanisms, such as fluid shear, and active, regulated processes initiated by environmental changes. Active dispersion involves the degradation of the EPS matrix, often facilitated by enzymes such as dispersin B, as well as phenotypic shifts that enable bacteria to revert to a planktonic, mobile phase (Rumbaugh and Sauer, 2020). Environmental triggers such as changes in nutrient levels or oxygen availability can initiate active dispersion, leading to the release of bacteria to colonize new environments. This process is crucial in clinical settings as it facilitates the spread of infections, particularly on medical devices, and contributes to the difficulty in treating biofilm-associated infections due to the rapid re-establishment of biofilms and increased resistance to antimicrobial agents (Werneburg et al., 2019).
Understanding these stages helps devise strategies to manage biofilm-related issues, emphasizing the dynamic nature of microbial communities and their interaction with environments. The study of biofilms bridges microbiology, molecular biology, and environmental sciences, offering insights into microbial behavior and potential control methods in medical and industrial contexts.
3 Bacteriophages-biofilm interaction in nature
The interactions between bacteriophages and biofilms are complex and rely on several factors determining biofilm destruction, coexistence, or limited phage efficacy in controlling biofilms.
The susceptibility of bacterial cells to phage infection can lead to the destruction of biofilms. When the biofilm is susceptible, bacteriophages attach to receptors on bacterial cells, inject their genetic material, and take over the bacterial machinery to replicate (Sutherland et al., 2004), leading to the lysis of infected cells and destroying the biofilm (Fernández et al., 2018; Papaianni et al., 2020). The availability of specific receptor sites on the biofilm bacteria’s surface is crucial for phage attachment and infection (Uchiyama et al., 2014). The polysaccharide-degrading enzymes produced by bacteriophages weaken the biofilm structure, allowing phages to penetrate and disperse the biofilm, releasing individual bacteria, making them more susceptible to other antimicrobial agents (González et al., 2018; Chegini et al., 2020). Phages can also produce endolysins, which hydrolyze the peptidoglycan in bacterial cell walls from within, contributing to the release of newly formed viral particles (Abdelrahman et al., 2021).
The biofilm composition and structure affect both the coexistence of phages with bacteria and limit the effectiveness of phages in controlling biofilm formation. The biofilm’s extracellular matrix, composed of polysaccharides, proteins, and DNA, can hinder phage diffusion and penetration, preventing them from reaching and killing biofilm bacteria (Limoli et al., 2015). In some cases, phages can promote biofilm formation and trigger specific responses in bacteria, increasing the adhesion, stability, and matrix production, allowing phages and bacteria to coexist within the biofilm (Secor et al., 2020). The presence, physiological state, and physical environment of different microorganisms (bacteria and fungi) influence phage accessibility to the biofilm (Bull et al., 2018; Ferriol-González and Domingo-Calap, 2020).
It is important to note that the interaction between phages and biofilms is still an active area of research. Further studies are needed to fully understand the complex dynamics between phages and biofilms in different environments and bacterial species.
3.1 Advantages and limitations of bacteriophages as antibacterial agents
Bacteriophages possess features such as self-replication within host bacteria, leading to increased concentrations in biofilms, and rapid replication, achieving high population densities swiftly (Talapko and Škrlec, 2020). They exhibit lytic activity against bacteria and produce enzymes that degrade the biofilm matrix, thereby enhancing biofilm penetration, infection, and elimination (Abedon, 2015). The continuous interactions between phages and hosts in biofilms drive evolutionary adaptations, potentially enhancing phage efficacy in disrupting biofilms (Talapko and Škrlec, 2020). All those characteristics, which are part of phages’ lifestyle are advantageous to use them as antibacterial agents.
On the other hand, limitations include the high specificity of bacteriophages for their hosts and the development of phage resistance by biofilm bacteria (Talapko and Škrlec, 2020). Bacterial defense mechanisms within the biofilm, such as CRISPR-Cas systems, plasmids, genomic islands, modification and mutation of surface receptors, or production of substances that inhibit phage attachment, prevent bacteriophages from infecting and eliminating biofilm communities (Cady and O’Toole, 2011; Römling and Balsalobre, 2012; Hassan et al., 2021; Ngiam et al., 2022). In addition, the biofilm matrix acts as a physical barrier, hindering phage movement and penetration (Talapko and Škrlec, 2020; Hassan et al., 2021; Ngiam et al., 2022).
4 Phage penetration and innovative phage delivery systems
4.1 Penetration strategies
Bacteriophages have several strategies for penetrating biofilms, one of which involves the production of an enzyme called polysaccharide depolymerase. This enzyme can digest polysaccharide components of bacterial cell walls by identifying and binding to specific ligands on the bacterial surface, facilitating phage genome transfer (Knecht et al., 2019). Depolymerases are subdivided into several classifications according to their target in biofilms: capsular polysaccharides, lipopolysaccharides (LPS), O-polysaccharides or exopolysaccharides (Pires et al., 2016; Knecht et al., 2019). Based on their biochemical properties, depolymerases are classified into hydrolases and lyases. Phage hydrolases catalyze the breaking of chemical bonds with water, displaying activities such as sialidases, xylosidases, levanases, rhamnosidases, dextranases, and peptidases. Conversely, lyases catalyze the breaking of chemical bonds through mechanisms other than hydrolysis, encompassing hyaluronate, alginate, and pectin/pectate lyases (Pires et al., 2016; Knecht et al., 2019; Topka-Bielecka et al., 2021). These depolymerases exhibit high substrate specificity, playing a crucial role in the bacteriophage’s ability to attach, invade, and ultimately destroy host bacteria (Yan et al., 2014).
Interestingly, bacteriophages have been genetically modified to express depolymerase during infection, enabling the simultaneous degradation of the bacterial cell wall and the biofilm matrix, thereby enhancing the chances of biofilm destruction (Lu and Collins, 2007). Furthermore, phages can produce endolysins, bactericidal proteins that hydrolyze bacterial cell walls, facilitating the release of phage particles (Briers et al., 2009; Abdelrahman et al., 2021). These endolysins, in conjunction with proteins such as holin, breach the bacterial cytoplasmic membrane to access the peptidoglycan layer (Wang et al., 2000). Additionally, the protein spanin contributes to the degradation of the outer membrane in Gram-negative hosts, further aiding in the bacteriophage’s infection process (Cahill and Young, 2019).
Phages can also switch between two life stages, lytic and lysogenic, contributing to their ability to penetrate and destroy the biofilm. During the lysogenic phase, the phage’s DNA merges with the bacterium’s genetic material, enabling the phage to penetrate and spread within the biofilm without destroying the host cells immediately (Weinbauer et al., 2003). This allows the phages to remain dormant until favorable conditions are present in the biofilm (Weinbauer et al., 2003). The control of the lytic and lysogenic phases involves various elements. Regulatory factors, including bacteriophage Lambda repressors CI and Cro, determine the lysogenic state; these elements were identified in previous studies (Carrasco et al., 2016; Lee et al., 2022). Other factors that can impact the lysogeny-lysis switch of phages are quorum sensing and the metabolic state of the host (Laganenka et al., 2019).
In clinical settings, an alternative to effectively reach and destroy biofilms is combining multiple bacteriophage mixtures with different host ranges into one solution. Bacteriophage cocktails can easily penetrate and destroy biofilms such as the ones from Pseudomonas aeruginosa, Enterococcus faecalis, and Methicillin-resistant Staphylococcus aureus (MRSA) (Hanlon, 2007; Kaur et al., 2016; Kwiatek et al., 2017; Khalifa et al., 2018). Another alternative is the phage-antibiotic synergy (PAS). In this approach, the phages can initiate biofilm breakdown, allowing antibiotics to penetrate more effectively and kill the bacteria more efficiently. Conversely, antibiotics can weaken the bacteria, making them more susceptible to phage infection (Morrisette et al., 2020). Other approaches involve using nanoparticles to improve phage penetration. For instance, a study by Quan et al. (2019) used magnetic nanoparticles to create artificial channels in infectious biofilms, improving the penetration and effectiveness of antimicrobial treatment. The experiment successfully demonstrated the creation of artificial channels in S. aureus biofilms, which significantly enhanced the penetration of the antibiotic gentamicin and the bacterial killing efficacy. This method offers a simple and effective way to eradicate infectious biofilms when combined with existing antibiotic therapies (Quan et al., 2019). The following sections provide more examples of these approaches.
Additionally, targeting ligands or antibodies can facilitate phages’ penetration through the biofilm matrix, effectively reaching and infecting bacterial cells (Amankwah et al., 2021). Phages can also be genetically engineered to produce cell wall-degrading enzymes and display biofilm-specific peptides, thus allowing further binding and penetration of biofilms (Chegini et al., 2020; Zhao et al., 2023). Notably, the accessibility of phages to the biofilm can be affected by the types of microorganisms forming the structure, their physiological state, and the physical environment.
4.2 Nanoparticle-based delivery
Nanoparticle-based delivery systems have emerged as a promising strategy to enhance the efficacy of bacteriophage therapy in biofilm control by using the unique properties of nanoparticles to improve phage stability, targeting, and penetration into biofilms (Vera-González and Shukla, 2020). By combining bacteriophages with nanoparticles, natural compounds, and disinfectants, the destruction of biofilms can be more effective (Chegini et al., 2020). Various types of nanoparticles have been used to improve phage stability, targeting, and penetration into biofilms, including metallic (e.g., silver and gold nanoparticles) and polymeric (e.g., Poly Lactic-co-Glycolic Acid (PLGA) and chitosan) nanoparticles (Ahiwale et al., 2017; Abdelsattar et al., 2021).
Chitosan nanoparticles have several outstanding properties that make them useful in various biomedical applications, such as biocompatibility and biodegradability (Ke et al., 2021). Additionally, they can encapsulate and release drugs in a controlled manner, improving the efficacy of pharmacological treatments and reducing side effects; for biofilm control and eradication, chitosan has been combined with bacteriophages (Sharifi-Rad et al., 2021). Encapsulating bacteriophages in chitosan nanoparticles can protect them from harsh gastrointestinal conditions and improve delivery to the target site for effective results. Adamu and coworkers synthesized chitosan nanoparticles to encapsulate phages targeting Escherichia coli, offering considerable protection of the ΦKAZ14 bacteriophage against enzymatic degradation and acidic environments. This innovation makes the phage suitable for oral applications, improving its delivery (Adamu Ahmad et al., 2016). In a similar approach using chitosan, Li and coworkers introduced an innovative method of using polyvalent phages attached to magnetic colloidal nanoparticle clusters (CNCs) under a small magnetic field. The phage PEL1 immobilized onto chitosan-coated Fe3O4-based CNCs showed enhanced phage loading and improved biofilm penetration. The author concluded this conjugation method could broaden the use of phages for microbial control by improving their delivery to relatively hard-to-reach areas within biofilms (Li et al., 2017).
Gold nanoparticles (AuNPs) have been used to target phages in P. aeruginosa biofilms, demonstrating a significant reduction in biofilm production, which suggests that phage-inspired AuNPs could serve as potent therapeutic agents against human pathogens (Ahiwale et al., 2017). Similar research used AuNPs but combined with the C3 phage to treat P. aeruginosa in planktonic and biofilm states. This combination exhibited high stability under various temperatures, pH levels, and salt concentrations, indicating a more efficient delivery (Abdelsattar et al., 2021).
In a recent approach, a phage-Chlorin e6 (Ce6)-manganese dioxide nanocomposite (PCM) was created, combining the benefits of phage therapy with other therapeutic modalities. The phage component of the nanocomposite plays a crucial role in targeting host bacteria and aiding in the efficient delivery of Ce6 to penetrate biofilms. By incorporating phages into the nanocomposite, the study improves the targeting of host bacteria, facilitating the effective delivery of Ce6 within the biofilm structure (Wang et al., 2023).
Similarly, Manoharadas and coworkers showed an enhanced phage delivery method by combining green-synthesized silver nanoparticles and bacteriophages to effectively disperse pre-formed S. aureus biofilms from inert surfaces. This approach not only removes the biofilms but also prevents the establishment of new infections and subsequent colonization by causing the loss of viability of the biofilm-entrapped bacterial cells (Manoharadas et al., 2021).
Recent advancements in nanoparticle-based delivery systems have emphasized the importance of surface modifications and functionalization to enhance their further interaction with biofilms and bacteriophages. The attachment of polyethylene glycol (PEG) chains to nanoparticles, known as PEGylation, has improved nanoparticles’ stability and circulation time, thereby enhancing the delivery of bacteriophages to biofilm sites (Mura et al., 2013). Additionally, dual-functional nanoparticles that combine targeting ligands with antimicrobial agents offer a promising strategy for enhancing the specificity and efficacy of bacteriophage therapy. For example, mannose-functionalized nanoparticles have been developed to target lectin receptors on the surface of biofilm-forming bacteria, improving phage adherence and biofilm disruption (Pawde et al., 2020). Furthermore, stimuli-responsive nanoparticles that release their payload in response to environmental triggers such as pH or enzymatic activity have shown potential in improving the targeted delivery of bacteriophages within biofilms (Karimi et al., 2016).
4.3 Liposome and hydrogel encapsulation-based delivery
Liposomes and vesicles constitute a versatile delivery system for hydrophilic and hydrophobic substances and represent the most promising vehicles for a variety of therapeutic agents, including bacteriophages. Liposome and vesicle encapsulation have recently emerged as an efficient approach for enhancing the efficient delivery and improved the efficacy of phages used to treat biofilms (Kaszuba et al., 1997; Sihorkar and Vyas, 2001). These exploit lipid-based carriers’ biocompatibility and tailor-made nature to improve biofilm phage interactions such as stability, targeting, or penetration into the biofilm structures (Singla et al., 2016).
The mechanisms to enhance bacteriophage therapy by using liposomes are: (I) Protection from environmental stress, where the encapsulation of bacteriophages in liposomes protects them from different environmental stressors, such as pH changes and enzymatic degradation, and thereby maintain their infectivity (Loh et al., 2021), (II) improved penetration into the biofilm thanks to the presence of the lipid bilayer structure that facilitates the delivery of phages into biofilms due to easy fusion with the bacterial membrane (Malik et al., 2017), and (III) targeted delivery through the functionalization with the targeting moieties to ensure that the phages are specifically targeted on the bacterial biofilms, which decreases off-target effects and enhances the therapeutic impact.
Recent advancements in liposomes and hydrogels for bacteriophage delivery have demonstrated significant potential in enhancing biofilm removal. In the context of orthopedic device infections, engineered injectable hydrogels encapsulating P.aeruginosa bacteriophages have shown promising results. These hydrogels, capable of controlled phage release, retain bacteriolytic activity and effectively target planktonic and biofilm bacteria without affecting human mesenchymal stromal cells. The authors concluded that the hydrogels efficiently deliver bacteriophages to treat localized bone infections (Wroe et al., 2020).
Furthermore, incorporating bacteriophages into liposomes and other amphiphilic nanoparticles offers the advantage of targeted delivery through ligand interaction with target cells. Although this approach presents challenges, such as ensuring amphiphilic properties or conjugation with anchoring molecules, it provides a sophisticated means of enhancing the directed delivery of bacteriophages (Loh et al., 2021).
Finally, an innovative delivery system has been developed for local tissue regeneration and infection control using bacteriophage-loaded alginate-nanohydroxyapatite hydrogel. This system efficiently encapsulates bacteriophages, with release influenced by environmental pH, without compromising their viability or functionality, thus improving their delivery. The hydrogels showed good tissue response and exhibited excellent antimicrobial effectiveness, inhibiting the attachment and colonization of multidrug-resistant E. faecalis (Barros et al., 2020).
Together, these studies illustrate the diverse strategies and significant progress made in using nanoparticle-encapsulated bacteriophages to address biofilm-related infections, paving the way for innovative treatments that enhance targeted delivery and efficacy.
4.4 Genetically engineered bacteriophages
Phage engineering has shown promise in enhancing the effectiveness of tailored phage therapies in combating biofilm-related challenges. Most genetic engineering approaches for biofilm control focus on improving the specificity, affinity, and efficacy of bacteriophages rather than enhancing their delivery to eradicate biofilms (Lu and Collins, 2007; Gutiérrez et al., 2016; Chegini et al., 2020; Yue et al., 2022). Another application of genetic engineering of phages is the potential to extend their natural host range and modify phage display (Khambhati et al., 2023). However, improved delivery is often a beneficial consequence rather than the primary goal of these modifications. Recently, to overcome the challenge of eradicating biofilms in water distribution systems, the filamentous coliphage M13 was engineered to enhance biofilm affinity and deliver lytic polyvalent phages. Modified M13 showed a significantly higher affinity for P.aeruginosa biofilms and improved delivery capabilities (Sun et al., 2022). This tunable approach could enable enhanced phage delivery and higher biofilm eradication efficacy to expand the scope of phage applications. A summary of the innovations regarding phage delivery is shown in Figure 1.
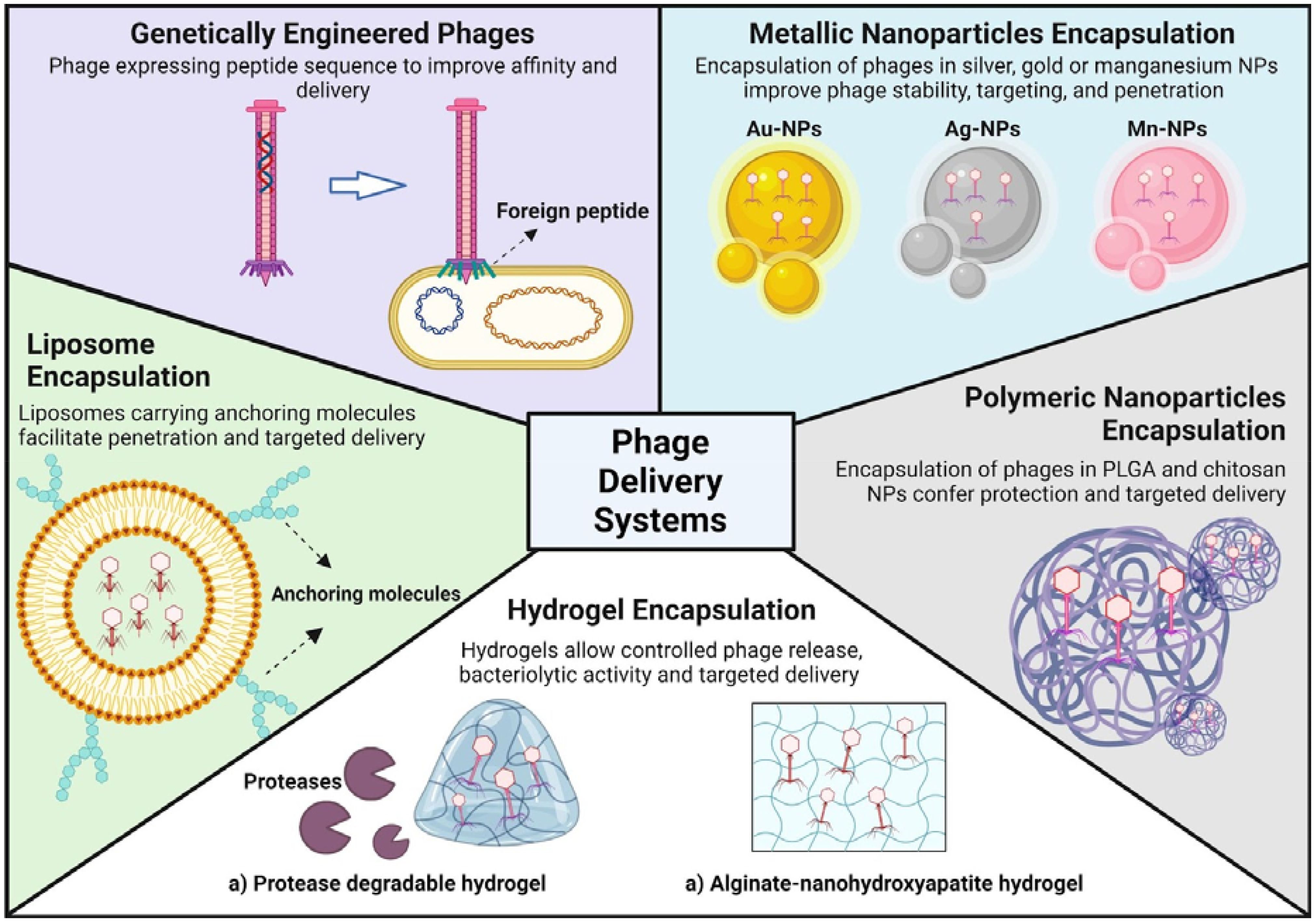
Figure 1. Advanced encapsulation strategies for phage delivery systems. The figure illustrates various methodologies for enhancing the stability and targeting of phages for therapeutic applications. Genetically engineered phages are modified to express specific peptides that improve their delivery efficiency. Liposomes, metallic nanoparticles (silver, gold, magnesium, etc.) and polymeric nanoparticles (PLGA and chitosan) are utilized to encapsulate phages, thereby bolstering their stability, penetration capabilities and ensuring targeted delivery. Moreover, the use of hydrogels (protease-degradable and alginate-nanohydroxyapatite) allows for controlled release and targeted delivery while maintain the phages innate bacteriolytic activity.
Exploring innovative approaches to intentionally enhance phage delivery, such as engineering phages to improve phage entrance or withstand harsh conditions within biofilms, could offer significant advancements in biofilm control strategies. This gap suggests an opportunity for innovative approaches to enhance phage delivery to biofilms. For example, engineering phages to produce surface modifications that increase their ability to penetrate biofilms or survive harsh biofilm environments could significantly improve their therapeutic efficacy. Such targeted delivery strategies would be a novel direction for research and development in bacteriophage therapy.
5 Using bacteriophages to dismantle biofilms
The rise of antibiotic-resistant microorganisms has become a significant global public health concern, especially in chronic infections associated with bacterial biofilms growing over medical instruments. These biofilms demonstrate heightened antibiotic tolerance and host immunity (Singh et al., 2022a; Cangui-Panchi et al., 2023). Phage therapy, recognized for its efficacy against antibiotic-resistant bacteria, is being explored by several research groups worldwide (Pires et al., 2020). Technological advancements have facilitated phage-based therapy research, revealing promising results in addressing antibiotic-resistant bacterial infections. Notably, phages have reduced contamination in medical catheters (Joo et al., 2023; Erol et al., 2024). However, phage application shows potential for the sanitation of medical instruments and holds great promise for a prospective application in clinical practice and patient treatment (Suh et al., 2022).
5.1 Environmental sanitation
5.1.1 Phage application: prevention of biofilm in water environment
Contamination of water sources by enteric pathogens poses a significant threat to public health. These microorganisms can originate from various human activities, such as runoff from livestock facilities, intensive farming practices, and wastewater treatment plants (WWTPs) effluents. Infrastructure failures in sewage systems can exacerbate this issue, resulting in fecal pollution of surface waters (Shivaram et al., 2023). Traditional methods of microbial removal include chemical disinfection and antibiotic treatments, contributing to the emergence of antibiotic resistance among waterborne pathogens (Mancuso et al., 2021).
Bacteriophage therapy has emerged as a promising approach to combat antibiotic-resistant bacteria (Karthikeyan and Meyer, 2006; Shivaram et al., 2023). In WWTPs, filamentous bacteria commonly cause bulking and foaming, reducing treatment efficiency (Ballesté et al., 2022). Laboratory studies have shown that isolated phages targeting these foam-causing bacteria can prevent foam stabilization, offering potential solutions to this issue (Petrovski et al., 2011; Liu et al., 2015). Moreover, bacterial biofilms on membrane-based WWTPs can impair treatment processes (Wu et al., 2017). Lytic phages have demonstrated effectiveness in inhibiting bacterial fouling of membranes (Goldman et al., 2009; Bhattacharjee et al., 2015; Ayyaru et al., 2018); for instance, phage DTP1 showed promise as a biocontrol agent against Delftia tsuruhatensis ARB1, an antibiotic-resistant bacterium commonly found in WWTPs (Bhattacharjee et al., 2015).
P. aeruginosa biofilms frequently clog filters in water treatment plants and increase cleaning costs (Tian et al., 2021). Studies have explored the use of phages in combination with other disinfectants to achieve significant reductions (up to 96% removal rate) in P. aeruginosa biofilms, highlighting the potential of phage-based interventions in water treatment (Zhang and Hu, 2013). Antibiotic-resistant Vibrio species have been isolated from aquatic environments like dams, boreholes, and tap water (Maje et al., 2020); in aquaculture, preventive antibiotic treatment is commonly applied to reduce pathogenic Vibrio spp. and its biofilm (Tian et al., 2021; Lomelí-Ortega et al., 2023; Srisangthong et al., 2023). Also, lysins derived from a Vibrio parahaemolyticus phage have been proposed as a promising alternative to reducing antibiotic overuse in aquaculture (Matamp and Bhat, 2019). Overall, there is great potential in using phage-based interventions to eliminate waterborne pathogen contamination and reduce the reliance on antibiotics in water treatment and aquaculture practices.
5.1.2 Phage application: prevention of biofilm in the food industry
In agriculture and livestock food production, infectious diseases pose significant challenges to food sustainability, causing crop losses, animal welfare issues, and environmental pollution with antimicrobial agents (Abebe et al., 2020). These diseases can also lead to emerging infectious diseases in humans and animals, facilitated by zoonotic transmission from microbial contamination (Rohr et al., 2019). Biofilms formed by foodborne pathogens have substantial public health implications, particularly in mixed-species biofilms common in the food industry (Galié et al., 2018). Bacteriophages have also been proposed as a green biocontrol tool for eliminating these microorganisms in food production (Moye et al., 2018).
Research has employed bacteriophages (Phage K and T4-like phages) for the treatment of S. aureus and E. coli that induced mastitis, a prevalent infectious disease in livestock (da Silva Duarte et al., 2018; Loponte et al., 2021). In 2006, the U.S. Food and Drug Administration (FDA) approved a phage cocktail sanitation product (ListShieldTM) to eliminate Listeria monocytogenes and marked a milestone for subsequent phage-based food preservation products (Zhang, 2018; Vikram et al., 2021). Biofilm formation on food production surfaces, often resistant to biocides, is a significant challenge (Meade et al., 2020). A recent study assessed the effectiveness of phage cocktails in the elimination of Shiga-toxigenic E. coli (STEC) growth over several types of surfaces (polystyrene well plates, stainless, steel, and high-density polyethylene) and found that STEC populations were reduced to undetectable levels after 16 hours of treatment (Jaroni et al., 2023). Similarly, another study used phage phT4A to reduce E. coli biofilm growth over plastic and reported its maximum inhibition percentage after only 6 hours of phage application (Brás et al., 2024).
Some studies have assessed the potential use of phage cocktails to combat dual-species biofilms, which are also relatively common biofilm arrangements over food production surfaces (Kim et al., 2023b). The effectiveness of these approaches depends on the combination of bacteria inside the mixed-species biofilm. For instance, one study showed that phages are more effective in eliminating single species than mixed biofilms in an E. coli and Salmonella enteritidis biofilm (Milho et al., 2019). In contrast, it has been suggested that phages tackling Staphylococcus lentus and Pseudomonas fluorescens mixed biofilms are able to eliminate their respective host within the biofilm (Sillankorva et al., 2010; Milho et al., 2019). Although studies have demonstrated the efficacy of phages in controlling single-species biofilms, further research is needed to explore their application in mixed-species biofilms in food environments.
5.2 Clinical treatment
The global impact of antibiotic-resistant bacteria, contributing to over 1.27 million annual deaths, underscores the urgent need for novel treatments (Antimicrobial Resistance Collaborators, 2022; Fongang et al., 2023). Bacterial biofilms are particularly problematic due to their ability to cause chronic and resilient infections. These biofilms offer protection and tolerance to antibiotics, antiseptics, antimicrobials, and host immune responses, leading to persistent infections (Yang et al., 2012). Eradicating biofilms within a host proves difficult due to physiological and physical barriers. Moreover, the minimum inhibitory concentration of antibiotics required against biofilms can be substantially higher than planktonic bacteria (Høiby et al., 2011). Biofilms on medical surfaces, such as catheters and implants, exacerbate the problem (Srivastava et al., 2019; Joo et al., 2023; Erol et al., 2024). Phage therapy has demonstrated significant potential in combating these infections through various therapeutic approaches (Figure 2). A summary of bacteriophage-based clinical studies is shown in Table 1.
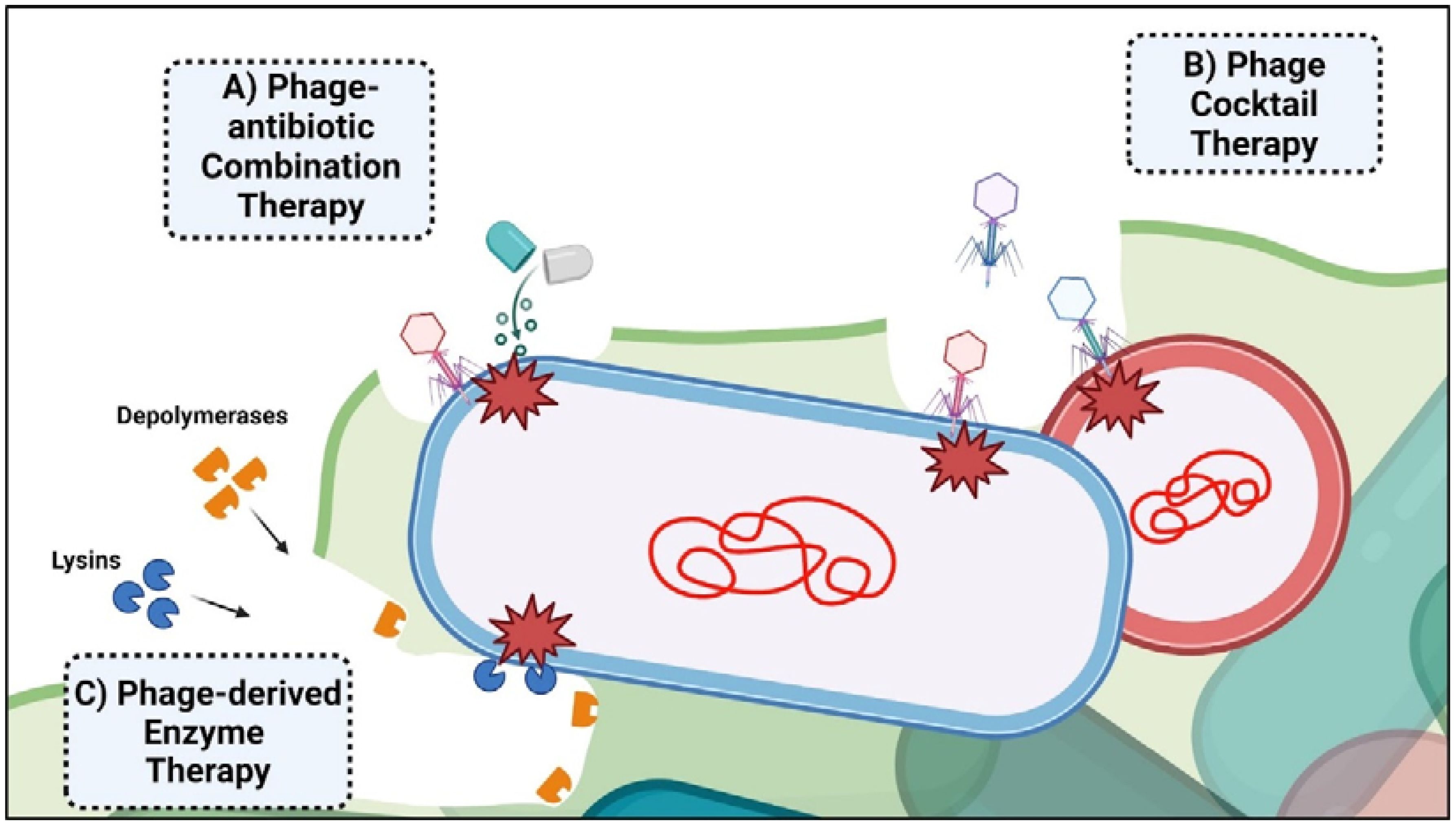
Figure 2. Bacteriophage-based therapeutic approaches employed for biofilm-derived infection. The figure illustrates the three main phage-associated mechanisms employed for clinical treatment. (A) Phage-antibiotic combination therapy can disrupt biofilm formation, making bacteria more vulnerable to phage attack and the effects of antibiotics. (B) Phage cocktail therapy involves using a mixture of bacteriophages to target and eliminate multiple strains or species of bacteria in poly-bacterial infections. (C) Phage-derived enzyme therapy utilizes enzymes, such as lysins and depolymerases, to degrade bacterial cell walls and biofilm matrices, degrading biofilms and eliminating bacteria.
5.2.1 Bacteriophage and antibiotic combination therapy
The use of bacteriophages and antibiotics during a combination therapy has shown a synergizing effect that increases the effectiveness of biofilm eradication. This approach reduces antibiotic concentrations while maintaining efficacy (Morrisette et al., 2020). In 2018, a study assessed the synergistic effect of pre-treatment application of Sb-1 S. aureus phage followed by antibiotic administration and demonstrated the effective elimination of MRSA biofilms (Tkhilaishvili et al., 2018). Previously, combination therapy of T4 phage and antibiotics showed potential to reduce biofilms formed by antibiotic-resistant and phage-resistant E. coli biofilms (Coulter et al., 2014). Notably, studies in P. aeruginosa biofilms using phage-antibiotic combination therapy also demonstrated high efficacy. In 2017, one study assessed the effects of the concurrent administration of a 12-phage cocktail PP1131 with various antibiotics. It concluded that this combination exhibited efficacy in treating bacterial infections in laboratory rats, compared to administering only one of the components individually (Oechslin et al., 2017).
More recently, some clinical studies have already applied the combination of phage and antibiotic treatments in patients with recurrent or unresolved infections (Table 1). For instance, during a case study, a 62-year-old woman was treated with a combination of phage Pa53 and meropenem to treat a chronic right hip prosthesis infection caused by P. aeruginosa since 2016. The patient could self-administer the phage suspension with no observable side effects during the treatment. A 2-year follow-up evaluation concluded the success of the treatment as no signs of infection relapse were found (Cesta et al., 2023). The question regarding the effectiveness of phage application with other types of drugs has also started to be investigated. One study observed that certain anticancer drugs can inhibit the activity of potential therapeutic phages in an S. aureus infection model (Li et al., 2023). Immunocompromised cancer patients are highly vulnerable to opportunistic infection; for example, lung cancer patients can present high rates of S. aureus-derived pneumonia during chemotherapy (Rolston, 2017). Chemotherapeutic drugs can be seen as a limitation for using antibiotic approaches such as antibiotics or phage therapy due to interference or incompatibility. Li and coworkers studied the synergetic potential of phages with common anti-cancer drugs. Combining phage K with Doxorubicin effectively eliminated S. aureus intracellular infections and migration (Li et al., 2023).
5.2.2 Bacteriophage cocktail therapy
Recent findings suggest the efficacy of phage cocktails against bacterial biofilms, particularly in multispecies environments, offering advantages like a broader host range and reduced risk of phage resistance (Morrisette et al., 2020). Using multiple phages enables the recognition of different bacterial receptors, which increases their effectiveness (Singh et al., 2022b; Naknaen et al., 2023). For example, a study utilized six lytic phages against a wide range of P. aeruginosa clinical isolates and assessed biofilm-inhibiting in static and dynamic biofilm models. The phage cocktail effectively eliminated most biofilm biomass within 4 hours for the static model and within 48 hours in the dynamic biofilm model (Alves et al., 2016). Another promising phage cocktail (EFDG1 and EFLK1) was able to target and eliminate planktonic and biofilm cultures of vancomycin-resistant E. faecalis V583 strains (Khalifa et al., 2018).
A major pathogen in orthopedic and joint implant infections is Staphylococcus, specifically coagulase-negative Staphylococcus or MRSA (Bozhkova et al., 2013; Lu et al., 2022). Using phage cocktails combined with linezolid reduced adherence to MRSA after being surgically implanted in the intramedullary canal of the mouse femur bone (Kaur et al., 2016). Finally, in a phase I clinical trial, nine patients with S. aureus-derived chronic rhinosinusitis were treated with intranasal irrigants of phage cocktail AB-SA01 and presented no serious adverse effects (Ooi et al., 2019).
More recently, the application of phage cocktails and antibiotic courses has already been investigated in several clinical trials. For example, during a case study, a 54-year-old male patient was treated with a combination of two phages (PNM and PT07) and antibiotics (ceftazidime/avibactam and amikacin) to treat a left ventricular assist device driveline infection caused by multidrug-resistant P. aeruginosa. Follow-up evaluations were recorded at six weeks, 34 weeks, and 48 months after intervention, where the patient showed no signs of relapse (Racenis et al., 2023). The same year, a necessary clinical trial assessed the effect of an antibiotic phage cocktail in combination with several etiotropic antibiotics (Fedorov et al., 2023). During this trial, twenty-three adult patients with deep periprosthetic joint infection received phage-antibiotic therapeutic intervention during and after surgery, with two patients presenting mild adverse effects (fever) after phage administration, which were eventually resolved. In the end, the periprosthetic joint infection relapse rate was around 12 times lower than that in the control group (Fedorov et al., 2023).
5.2.3 Bacteriophage-derived enzymes application
Several limitations can interfere with phage penetration, propagation, and diffusion through the EPS matrix, hindering the use of lytic phages to eliminate biofilms. Phage-derived enzymes, namely endolysins and depolymerizes, have emerged as potential mechanisms of EPS matrix degradation, as explained in Section 4.1. The efficacy of endolysins as tools for biofilm treatment has been extensively covered in the literature (de Miguel et al., 2020; Ghose and Euler, 2020; Abdelrahman et al., 2021).
In the field of clinical trials, some advancements have been made in the elimination of biofilm-forming bacteria of medical relevance. For instance, research has demonstrated that the phage depolymerase KPO1K1 can eliminate Klebsiella pneumoniae B5055 even on an old biofilm matrix (Fernandes and São-José, 2018). Recently, the multidrug-resistant clinical strain Kl 315 of K. pneumoniae and its biofilm were also shown to be effectively eliminated by phage displaying depolymerase activity (Zurabov et al., 2023).
In this regard, both endolysins and depolymerizes exhibit a narrow antibacterial spectrum, offering advantages over broad-spectrum antibiotics. They are suitable for selective elimination of drug-resistant pathogenic bacteria with lesser adverse effects on the host microbiome (Singh et al., 2022b).
5.2.4 Novel bacteriophage-based clinical approaches
In recent years, novel bacteriophage approaches have gained significant attention as promising strategies for treating biofilm-associated infections, effectively addressing the limitations of traditional antibiotics and earlier phage therapies. These approaches have been assessed in vivo models and tackle critical challenges such as efficient phage delivery, resilience against harsh environmental conditions within the host, neutralization by host antibodies, and the internalization of phages to combat intracellular infections. As mentioned in Section 4, bacteriophage encapsulation represents an innovative method for biofilm infection treatment, leveraging bacteriophages’ inherent potential while overcoming issues related to their stability and targeted delivery (Yan et al., 2021).
Clinical applications of hydrogel-based bacteriophage encapsulation have shown significant potential in vivo for treating multidrug-resistant (MDR) infections. In 2015, a study evaluated the temperate phage ФPan70 for its efficacy against MDR P. aeruginosa in planktonic, biofilm, and mouse burn models. The phage significantly reduced bacterial populations and improved the survival rate of burned mice from 80% to 100%. Researchers suggested that phages could prevent bacterial spread into the bloodstream and enhance immune responses at the injection site (Holguín et al., 2015).
Further advancements in wound therapy have led to the development of wound dressings composed of chitosan, sodium alginate, and carboxymethyl cellulose, targeting MDR bacteria and biofilm-mediated infections in mice wound models (Mabrouk et al., 2022; Dehari et al., 2023; Mukhopadhyay et al., 2023). For instance, a study highlighted the synergistic effect of a phage-ciprofloxacin hydrogel in a mouse wound healing model, showing enhanced wound healing and improving mice recovery (Shafigh Kheljan et al., 2023). Figure 3 showcases the concept of wound dressing with hydrogel-coated bacteriophages. Some studies suggest that hydrogel-coated bacteriophages can be delivered via injectable application into specific tissues. For instance, a recent study utilized a fracture-related infection (FRI) mouse model to assess the effectiveness of a hydrogel containing both a phage cocktail and antibiotic meropenem to treat a P. aeruginosa FRI. Compared with the application of free bacteriophages, the phage-meropenem hydrogel exhibited a lower incidence of phage resistance and reduced serum neutralization (Chen et al., 2023).
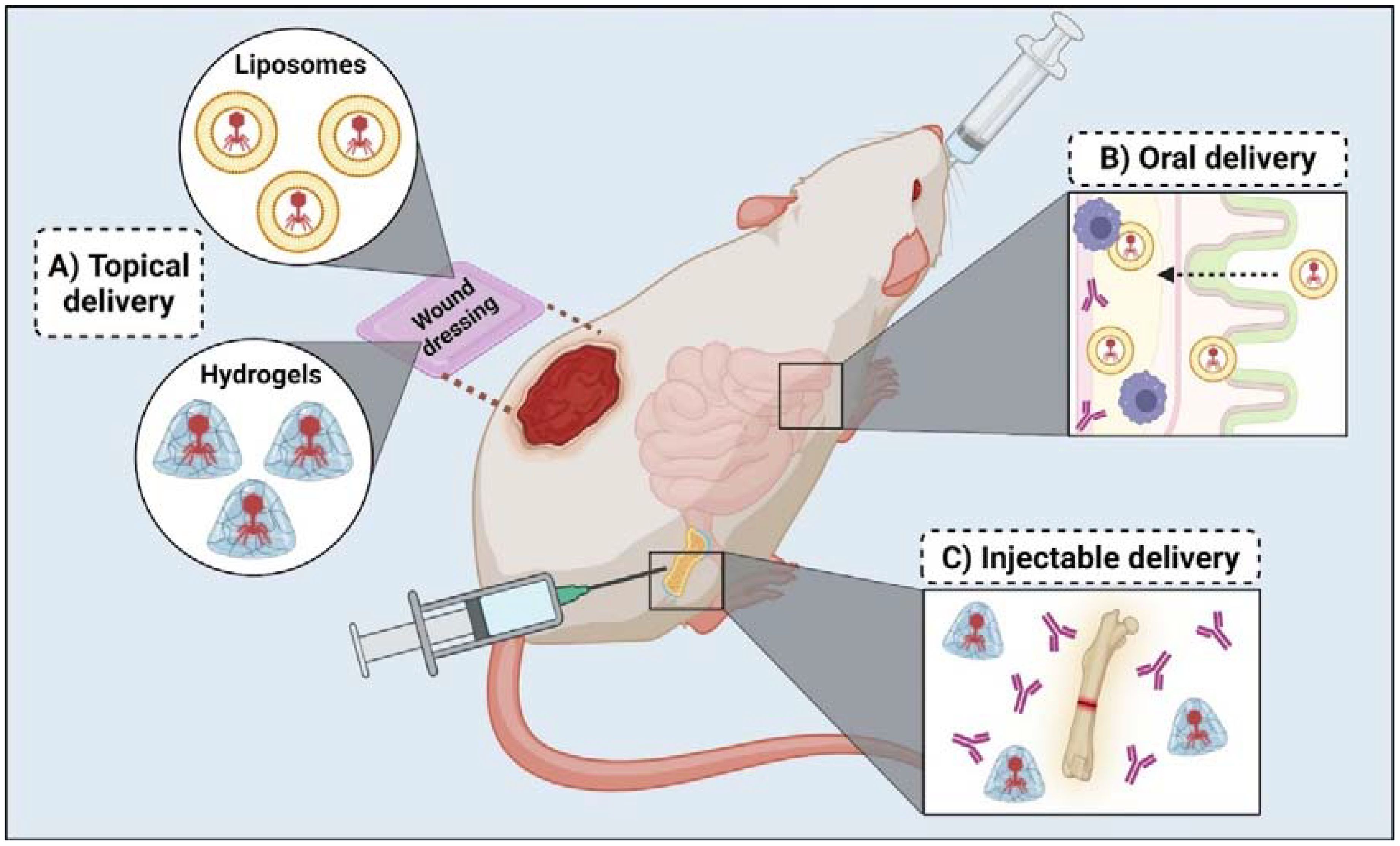
Figure 3. In vivo studies regarding bacteriophage delivery for clinical application. (A) Topical delivery has been used in mice wound models and involves wound dressing coated with liposomes or hydrogels containing bacteriophages. (B) Oral delivery mainly uses liposome encapsulation to protect bacteriophages on their transit to the intestinal tract, facilitate transportation through the intestinal layer and avoid antibody interference. (C) Injectable delivery allows the intradermal and intramuscular application of bacteriophage therapy in case of bone and prosthetic joint infection; it usually involves using hydrogels to protect bacteriophages from antibodies and other immune responses.
On the other hand, encapsulating bacteriophages in liposomes also shows promise in enhancing their stability and protection from the host immune system, ensuring controlled release at the infection site (Figure 3). Studies have employed various methods for effective phage encapsulation, such as a microfluidic-based technique to create liposomes with adjustable sizes (Cinquerrui et al., 2018). In a 2016 study, liposomes were evaluated for their ability to deliver phages intracellularly and their susceptibility to anti-phage antibodies. Liposome-encapsulated phages were fully protected from neutralizing antibodies, whereas free phages were neutralized within three hours. Additionally, liposome-encapsulated phages were able to enter mouse peritoneal macrophages and achieve a 94.6% killing rate of intracellular K. pneumoniae (Singla et al., 2016).
Another in vivo study in mice examined the biodistribution of orally administered, liposome-encapsulated fluorescent bacteriophages and their transport through intestinal cell layers. This study showed that liposome-encapsulated phages persisted longer in the stomach and adhered to the intestinal membrane, suggesting greater long-term efficacy of phage therapy (Otero et al., 2019). Murine models are not the only in vivo models used for these studies. For instance, in 2022, a study demonstrated that asymmetric phosphatidylserine/phosphatidic acid (PS/PA) liposomes, used in both prophylactic and treatments, reduced bacterial burden in zebrafish embryos infected with P. aeruginosa by enhancing macrophage phagocytic activity. In this regard, combining liposomes and phage cocktails improves antimicrobial effects and is effective against phage-resistant bacteria (Cafora et al., 2022).
Phage engineering has also shown great potential to develop novel approaches for targeting infections in clinical settings. Genetic modifications can enhance the expression of lytic enzymes, such as phage hydrolases, and facilitate the degradation of the biofilm matrix. For instance, genetically modified (GM) T7 E. coli phages have enhanced biofilm matrix degradation efficacy (Waturangi et al., 2021).
Studies have shown that phages can be engineered to augment their antimicrobial properties. In 2023, modification of the capsid of K1F phages increased their affinity for human tissue, potentially enhancing their ability to target intracellular infections caused by their host bacteria, E. coli K1 (Williams et al., 2023). Furthermore, eliminating lysogeny-related genes in Ef11 E. faecalis phages has shown promising results in eradicating E. faecalis biofilm populations, including vancomycin-resistant strains (Tinoco et al., 2016). Overall, genetic modification of phages also represents a valuable tool to enhance the effectiveness of phage-based biofilm treatment in clinical settings.
6 Future perspectives and challenges
Bacteriophage therapy, an innovative approach for targeting biofilm-associated infections and antibiotic-resistant bacteria, holds significant potential. However, its application is accompanied by significant challenges and limitations that necessitate critical examination. These challenges encompass a range of technical, biological, and regulatory issues that must be addressed to harness the full potential of phages in clinical and environmental applications.
6.1 Enhancing phage efficacy and delivery
A primary limitation of bacteriophage therapy is its narrow host range, which restricts its application to specific bacterial strains (Magaziner and Salmond, 2022; Ratnakar, 2022). This specificity, though beneficial in minimizing off-target effects, significantly limits the broader clinical applicability of phage therapy, especially in polymicrobial infections. Phage cocktails, comprising multiple phages with varying host ranges, have been suggested as a solution to this limitation (Schooley et al., 2017; Zaczek-Moczydłowska et al., 2020). However, the formulation of such cocktails must carefully consider the potential antagonistic interactions between phages, which could compromise their efficacy. Additionally, identifying and formulating effective phage cocktails is resource-intensive and requires extensive screening and validation.
6.2 Addressing phage resistance
Similar to antibiotics, bacteriophages face the challenge of bacterial resistance. Bacteria can evolve mechanisms to evade phage predation (Teklemariam et al., 2023), such as altering surface receptors required for phage attachment and infection (Rendueles et al., 2023). This phenomenon requires ongoing monitoring and the development of new phages, a process that demands significant resources and time. To mitigate resistance, strategies such as combining phages with antibiotics have shown promise in enhancing biofilm eradication and preventing resistance (Lu et al., 2023; Meneses et al., 2023).
6.3 Structural complexity of biofilms
The dense extracellular matrix of biofilms presents a significant barrier to effective phage therapy. This matrix can impede phage penetration and reduce bactericidal efficiency. Advanced delivery systems, including nanoparticles, liposomes, and hydrogels, are being explored to enhance phage stability and facilitate deeper penetration into biofilm structures (Kluzek et al., 2022; Costa et al., 2023). Despite promising results, these delivery mechanisms’ development and clinical application remain in their early stages and require further validation.
6.4 Stability in various environments
The stability of phages in different environments, including the human body, is a concern. Factors such as pH, temperature, and the presence of immune components can influence phage viability and activity. Strategies like encapsulating phages in protective carriers or engineering phages to evade immune detection are under investigation (Arias et al., 2022; Hufziger et al., 2022; Gao and Feng, 2023). However, these approaches add complexity to treatment protocols.
6.5 Environmental and industrial applications
Beyond clinical settings, phage therapy holds potential for environmental sanitation and industrial applications. Phages can be utilized to control biofilms in water treatment plants (Shivaram et al., 2023), food processing environments (Vikram et al., 2021; Brás et al., 2024), and agricultural settings (Ascaño et al., 2022), providing sustainable and effective alternatives to chemical treatments. Future research should focus on optimizing phage application methods to ensure environmental compatibility and effectiveness across diverse conditions.
6.6 Phage-host interactions and evolutionary dynamics
Understanding the complex interactions between phages and their bacterial hosts is crucial for successfully applying phage therapy. Phage-host dynamics are influenced by various factors, including the genetic diversity of phages and bacteria, environmental conditions (Fister et al., 2016; Goehlich et al., 2024), and the presence of other microorganisms (Alkhalil, 2023). Studying these interactions can provide insights into the mechanisms of bacterial resistance and inform the development of more effective phage therapies.
6.7 Integration with other therapies
Combining phage therapy with other treatment modalities, such as antibiotics, antimicrobial peptides, and immune modulators, has shown promise in enhancing therapeutic outcomes (Coulter et al., 2014; Morrisette et al., 2020; Joo et al., 2023; Lu et al., 2023). This approach requires careful consideration of potential interactions, dosing regimens, and treatment protocols. Clinical trials and studies are needed to evaluate the safety and efficacy of combination therapies and establish guidelines for their use in clinical practice.
6.8 Release of endotoxins by lysis of Gram-negative bacteria
A critical impediment to the deployment of phage therapy for infections caused by Gram-negative bacteria is the simultaneous release of endotoxins, particularly LPS, during the lysis of bacterial cells. Gram-negative bacteria are characterized by an outer membrane enriched with LPS, a potent elicitor of human immune responses. Bacteriophage-induced lysis releases these cell wall components, including endotoxins, into the extracellular milieu, precipitating a severe inflammatory reaction. This response, known as endotoxin shock or septic shock, is marked by symptoms such as fever and hypotension, which can lead to fatal outcomes if not adequately controlled. The efficacy of phage therapy in reducing bacterial populations is paradoxically undermined by the exacerbation of clinical symptoms due to the rapid liberation of endotoxins (Hibstu et al., 2022). Strategies to mitigate this issue include the development of genetically engineered phages designed to either preclude the release of endotoxins or facilitate their degradation post-release (Matsuda et al., 2005; Łobocka et al., 2021).
In conclusion, while bacteriophage therapy offers a promising alternative to traditional antibiotics, continued research, innovation, and a multidisciplinary approach that addresses its myriad challenges and complexities is needed. The path forward involves enhancing phage delivery systems, developing strategies to mitigate resistance, expanding host range specificity, navigating the regulatory landscape, and fostering public engagement to ensure phage therapy’s sustainable and effective application across multiple domains.
Author contributions
LG: Conceptualization, Data curation, Formal Analysis, Methodology, Supervision, Writing – review & editing. AM-R: Investigation, Methodology, Validation, Visualization, Writing – original draft. SC-P: Formal Analysis, Investigation, Resources, Writing – review & editing. CB-O: Data curation, Formal Analysis, Validation, Writing – original draft.
Funding
The author(s) declare that no financial support was received for the research, authorship, and/or publication of this article.
Acknowledgments
Figures were created with BioRender.com.
Conflict of interest
The authors declare that the research was conducted in the absence of any commercial or financial relationships that could be construed as a potential conflict of interest.
Publisher’s note
All claims expressed in this article are solely those of the authors and do not necessarily represent those of their affiliated organizations, or those of the publisher, the editors and the reviewers. Any product that may be evaluated in this article, or claim that may be made by its manufacturer, is not guaranteed or endorsed by the publisher.
References
Abdelrahman, F., Easwaran, M., Daramola, O. I., Ragab, S., Lynch, S., Oduselu, T. J., et al. (2021). Phage-encoded endolysins. Antibiot. (Basel) 10, 124. doi: 10.3390/antibiotics10020124
Abdelsattar, A. S., Nofal, R., Makky, S., Safwat, A., Taha, A., El-Shibiny, A. (2021). The synergistic effect of biosynthesized silver nanoparticles and phage ZCSE2 as a novel approach to combat multidrug-resistant Salmonella enterica. Antibiot. (Basel) 10, 678. doi: 10.3390/antibiotics10060678
Abebe, E., Gugsa, G., Ahmed, M. (2020). Review on major food-borne zoonotic bacterial pathogens. J. Trop. Med. 2020, 4674235. doi: 10.1155/2020/4674235
Abedon, S. T. (2015). Ecology of anti-biofilm agents II: Bacteriophage exploitation and biocontrol of biofilm bacteria. Pharm. (Basel) 8, 559–589. doi: 10.3390/ph8030559
Adamu Ahmad, K., Sabo Mohammed, A., Abas, F. (2016). Chitosan nanoparticles as carriers for the delivery of ΦKAZ14 bacteriophage for oral biological control of colibacillosis in chickens. Molecules 21, 256. doi: 10.3390/molecules21030256
Ahiwale, S. S., Bankar, A. V., Tagunde, S., Kapadnis, B. P. (2017). A bacteriophage mediated gold nanoparticles synthesis and their anti-biofilm activity. Indian J. Microbiol. 57, 188–194. doi: 10.1007/s12088-017-0640-x
Ali, A., Zahra, A., Kamthan, M., Husain, F. M., Albalawi, T., Zubair, M., et al. (2023). Microbial biofilms: Applications, clinical consequences, and alternative therapies. Microorganisms 11, 1934. doi: 10.3390/microorganisms11081934
Alkhalil, S. S. (2023). The role of bacteriophages in shaping bacterial composition and diversity in the human gut. Front. Microbiol. 14. doi: 10.3389/fmicb.2023.1232413
Alves, D. R., Perez-Esteban, P., Kot, W., Bean, J. E., Arnot, T., Hansen, L. H., et al. (2016). A novel bacteriophage cocktail reduces and disperses Pseudomonas aeruginosa biofilms under static and flow conditions. Microbial. Biotechnol. 9, 61–74. doi: 10.1111/1751-7915.12316
Amankwah, S., Abdella, K., Kassa, T. (2021). Bacterial biofilm destruction: A focused review on the recent use of phage-based strategies with other antibiofilm agents. Nanotechnol. Sci. Appl. 14, 161–177. doi: 10.2147/NSA.S325594
Antimicrobial Resistance Collaborators (2022). Global burden of bacterial antimicrobial resistance in 2019: A systematic analysis. Lancet 399, 629–655. doi: 10.1016/S0140-6736(21)02724-0
Arias, C. F., Acosta, F. J., Bertocchini, F., Herrero, M. A., Fernández-Arias, C. (2022). The coordination of anti-phage immunity mechanisms in bacterial cells. Nat. Commun. 13, 7412. doi: 10.1038/s41467-022-35203-7
Armbruster, C. R., Parsek, M. R. (2018). New insight into the early stages of biofilm formation. Proc. Natl. Acad. Sci. U.S.A. 115, 4317–4319. doi: 10.1073/pnas.1804084115
Ascaño, M. A., Caiñza, M. A., Dy, R. L. (2022). “Sustainable Phage-Based Strategies to Control Bacterial Diseases in Agriculture,” in Agricultural biocatalysis: Biological and chemical applications (Jenny Stanford Publishing, New York), 41–79. doi: 10.1201/9781003313144-3
Ayyaru, S., Choi, J., Ahn, Y.-H. (2018). Biofouling reduction in a MBR by the application of a lytic phage on a modified nanocomposite membrane. Environ. Sci.: Water Res. Technol. 4, 1624–1638. doi: 10.1039/C8EW00316E
Azulay, D. N., Spaeker, O., Ghrayeb, M., Wilsch-Bräuninger, M., Scoppola, E., Burghammer, M., et al. (2022). Multiscale X-ray study of Bacillus subtilis biofilms reveals interlinked structural hierarchy and elemental heterogeneity. Proc. Natl. Acad. Sci. U.S.A. 119, e2118107119. doi: 10.1073/pnas.2118107119
Ballesté, E., Blanch, A. R., Muniesa, M., García-Aljaro, C., Rodríguez-Rubio, L., Martín-Díaz, J., et al. (2022). Bacteriophages in sewage: Abundance, roles, and applications. FEMS Microbes 3, xtac009. doi: 10.1093/femsmc/xtac009
Barros, J. A. R., de Melo, L. D. R., da Silva, R. A. R., Ferraz, M. P., de Rodriguez Azeredo, J. C. V., de Carvalho Pinheiro, V. M., et al. (2020). Encapsulated bacteriophages in alginate-nanohydroxyapatite hydrogel as a novel delivery system to prevent orthopedic implant-associated infections. Nanomedicine 24, 102145. doi: 10.1016/j.nano.2019.102145
Bhattacharjee, A. S., Choi, J., Motlagh, A. M., Mukherji, S. T., Goel, R. (2015). Bacteriophage therapy for membrane biofouling in membrane bioreactors and antibiotic-resistant bacterial biofilms. Biotechnol. Bioeng. 112, 1644–1654. doi: 10.1002/bit.25574
Blasco, L., López-Hernández, I., Rodríguez-Fernández, M., Pérez-Florido, J., Casimiro-Soriguer, C. S., Djebara, S., et al. (2023). Case report: Analysis of phage therapy failure in a patient with a P. aeruginosa prosthetic vascular graft infection. Front. Med. (Lausanne) 10. doi: 10.3389/fmed.2023.1199657
Bozhkova, S. A., Tikhilov, R. M., Krasnova, M. V., Rukina, A. N. (2013). Orthopedic implant-associated infection: The main etiological agents, local resistance and antimicrobial therapy recommendations. Travmatol. ortop. Ross. 19, 5–15. doi: 10.21823/2311-2905-2013–4-5-15
Brás, A., Braz, M., Martinho, I., Duarte, J., Pereira, C., Almeida, A. (2024). Effect of bacteriophages against biofilms of Escherichia coli on food processing surfaces. Microorganisms 12, 366. doi: 10.3390/microorganisms12020366
Briers, Y., Schmelcher, M., Loessner, M. J., Hendrix, J., Engelborghs, Y., Volckaert, G., et al. (2009). The high-affinity peptidoglycan binding domain of Pseudomonas phage endolysin KZ144. Biochem. Biophys. Res. Commun. 383, 187–191. doi: 10.1016/j.bbrc.2009.03.161
Bull, J. J., Christensen, K. A., Scott, C., Jack, B. R., Crandall, C. J., Krone, S. M. (2018). Phage-bacterial dynamics with spatial structure: Self-on organization around phage sinks can promote increased cell densities. Antibiot. (Basel) 7, 1–25. doi: 10.3390/antibiotics7010008
Cady, K. C., O’Toole, G. A. (2011). Non-identity-mediated CRISPR-bacteriophage interaction mediated via the Csy and Cas3 proteins. J. Bacteriol. 193, 3433–3445. doi: 10.1128/JB.01411-10
Cafora, M., Poerio, N., Forti, F., Loberto, N., Pin, D., Bassi, R., et al. (2022). Evaluation of phages and liposomes as combination therapy to counteract P. aeruginosa infection in wild-type and CFTR-null models. Front. Microbiol. 13. doi: 10.3389/fmicb.2022.979610
Cahill, J., Young, R. (2019). Phage lysis: Multiple genes for multiple barriers. Adv. Virus Res. 103, 33–70. doi: 10.1016/bs.aivir.2018.09.003
Cangui-Panchi, S. P., Ñacato-Toapanta, A. L., Enríquez-Martínez, L. J., Salinas-Delgado, G. A., Reyes, J., Garzon-Chavez, D., et al. (2023). Battle royale: Immune response on biofilms - host-pathogen interactions. Curr. Res.Immunol. 4, 100057. doi: 10.1016/j.crimmu.2023.100057
Carrasco, B., Escobedo, S., Alonso, J. C., Suárez, J. E. (2016). Modulation of Lactobacillus casei bacteriophage A2 lytic/lysogenic cycles by binding of Gp25 to the early lytic mRNA. Mol. Microbiol. 99, 328–337. doi: 10.1111/mmi.13234
Cesta, N., Pini, M., Mulas, T., Materazzi, A., Ippolito, E., Wagemans, J., et al. (2023). Application of phage therapy in a case of a chronic hip-prosthetic joint infection due to P. aeruginosa: An Italian real-life experience and in vitro analysis. Open Forum Infect. Dis. 10, ofad051. doi: 10.1093/ofid/ofad051
Chávez-Jacobo, V. M., Becerra-Rivera, V. A., Guerrero, G., Dunn, M. F. (2023). The Sinorhizobium meliloti NspS-MbaA system affects biofilm formation, exopolysaccharide production and motility in response to specific polyamines. Microbiol. (Reading Engl) 169, 1–15. doi: 10.1099/mic.0.001293
Chegini, Z., Khoshbayan, A., Taati Moghadam, M., Farahani, I., Jazireian, P., Shariati, A. (2020). Bacteriophage therapy against P. aeruginosa biofilms: A review. Ann. Clin. Microbiol. Antimicrob. 19, 45. doi: 10.1186/s12941-020-00389-5
Chen, B., Benavente, L. P., Chittò, M., Wychowaniec, J. K., Post, V., D’Este, M., et al. (2023). Alginate microbeads and hydrogels delivering meropenem and bacteriophages to treat P. aeruginosa fracture-related infections. J. Control. Release 364, 159–173. doi: 10.1016/j.jconrel.2023.10.029
Cinquerrui, S., Mancuso, F., Vladisavljević, G. T., Bakker, S. E., Malik, D. J. (2018). Nanoencapsulation of bacteriophages in liposomes prepared using microfluidic hydrodynamic flow focusing. Front. Microbiol. 9. doi: 10.3389/fmicb.2018.02172
Costa, M. J., Pastrana, L. M., Teixeira, J. A., Sillankorva, S. M., Cerqueira, M. A. (2023). Bacteriophage delivery systems for food applications: Opportunities and perspectives. Viruses 15, 1271. doi: 10.3390/v15061271
Coulter, L. B., McLean, R. J. C., Rohde, R. E., Aron, G. M. (2014). Effect of bacteriophage infection in combination with tobramycin on the emergence of resistance in E. coli and P. aeruginosa biofilms. Viruses 6, 3778–3786. doi: 10.3390/v6103778
da Silva Duarte, V., Dias, R. S., Kropinski, A. M., Campanaro, S., Treu, L., Siqueira, C., et al. (2018). Genomic analysis and immune response in a murine mastitis model of vB_EcoM-UFV13, a potential biocontrol agent for use in dairy cows. Sci. Rep. 8, 6845. doi: 10.1038/s41598-018-24896-w
Dehari, D., Kumar, D. N., Chaudhuri, A., Kumar, A., Kumar, R., Kumar, D., et al. (2023). Bacteriophage entrapped chitosan microgel for the treatment of biofilm-mediated polybacterial infection in burn wounds. Int. J. Biol. Macromol. 253, 127247. doi: 10.1016/j.ijbiomac.2023.127247
de Miguel, T., Rama, J. L. R., Sieiro, C., Sánchez, S., Villa, T. G. (2020). Bacteriophages and lysins as possible alternatives to treat antibiotic-resistant urinary tract infections. Antibiot. (Basel) 9, 466. doi: 10.3390/antibiotics9080466
Doub, J. B., Chan, B., Johnson, A. J. (2023). Salphage: Salvage bacteriophage therapy for a chronic Enterococcus faecalis prosthetic joint infection. IDCases 33, e01854. doi: 10.1016/j.idcr.2023.e01854
Doub, J. B., Ng, V. Y., Lee, M., Chi, A., Lee, A., Würstle, S., et al. (2022a). Salphage: Salvage bacteriophage therapy for recalcitrant MRSA prosthetic joint infection. Antibiot. (Basel) 11, 616. doi: 10.3390/antibiotics11050616
Doub, J. B., Ng, V. Y., Wilson, E., Corsini, L., Chan, B. K. (2021). Successful treatment of a recalcitrant Staphylococcus epidermidis prosthetic knee infection with intraoperative bacteriophage therapy. Pharm. (Basel) 14, 231. doi: 10.3390/ph14030231
Doub, J. B., Shishido, A., Srikumaran, U., Haskoor, J., Tran-Nguyen, P., Lee, M., et al. (2022b). Salphage: Salvage bacteriophage therapy for a recalcitrant Klebsiella pneumoniae prosthetic shoulder infection - a case report. Acta Orthop. 93, 756–759. doi: 10.2340/17453674.2022.4579
Erol, H. B., Kaskatepe, B., Yildiz, S., Altanlar, N., Bayrakdar, F. (2024). Characterization of two bacteriophages specific to Acinetobacter baumannii and their effects on catheters biofilm. Cell Biochem. Funct. 42, e3966. doi: 10.1002/cbf.3966
Fedorov, E., Samokhin, A., Kozlova, Y., Kretien, S., Sheraliev, T., Morozova, V., et al. (2023). Short-term outcomes of phage-antibiotic combination treatment in adult patients with periprosthetic hip joint infection. Viruses 15, 396. doi: 10.3390/v15020499
Fernandes, S., São-José, C. (2018). Enzymes and mechanisms employed by tailed bacteriophages to breach the bacterial cell barriers. Viruses 10. doi: 10.3390/v10080396
Fernández, L., Rodríguez, A., García, P. (2018). Phage or foe: An insight into the impact of viral predation on microbial communities. ISME J. 12, 1171–1179. doi: 10.1038/s41396-018-0049-5
Ferriol-González, C., Domingo-Calap, P. (2020). Phages for biofilm removal. Antibiot. (Basel) 9, 268. doi: 10.3390/antibiotics9050268
Fister, S., Robben, C., Witte, A. K., Schoder, D., Wagner, M., Rossmanith, P. (2016). Influence of environmental factors on phage-bacteria interaction and on the efficacy and infectivity of phage P100. Front. Microbiol. 7. doi: 10.3389/fmicb.2016.01152
Fongang, H., Mbaveng, A. T., Kuete, V. (2023). “Global burden of bacterial infections and drug resistance,” in African Flora to Fight Bacterial Resistance, Part I: Standards for the Activity of Plant-Derived Products (London: Elsevier), 1–20. doi: 10.1016/bs.abr.2022.08.001
Galié, S., García-Gutiérrez, C., Miguélez, E. M., Villar, C. J., Lombó, F. (2018). Biofilms in the food industry: Health aspects and control methods. Front. Microbiol. 9. doi: 10.3389/fmicb.2018.00898
Gao, Z., Feng, Y. (2023). Bacteriophage strategies for overcoming host antiviral immunity. Front. Microbiol. 14. doi: 10.3389/fmicb.2023.1211793
Ghose, C., Euler, C. W. (2020). Gram-negative bacterial lysins. Antibiot. (Basel) 9, 74. doi: 10.3390/antibiotics9020074
Goehlich, H., Roth, O., Sieber, M., Chibani, C. M., Poehlein, A., Rajkov, J., et al. (2024). Suboptimal environmental conditions prolong phage epidemics in bacterial populations. Mol. Ecol. 33, e17050. doi: 10.1111/mec.17050
Goldman, G., Starosvetsky, J., Armon, R. (2009). Inhibition of biofilm formation on UF membrane by use of specific bacteriophages. J. Memb. Sci. 342, 145–152. doi: 10.1016/j.memsci.2009.06.036
González, S., Fernández, L., Gutiérrez, D., Campelo, A. B., Rodríguez, A., García, P. (2018). Analysis of different parameters affecting diffusion, propagation and survival of staphylophages in bacterial biofilms. Front. Microbiol. 9. doi: 10.3389/fmicb.2018.02348
Gulec, F., Eckford, A. W. (2023). A stochastic biofilm disruption model based on quorum sensing mimickers. IEEE Trans. Mol. Biol. Multi-Scale Commun. 9, 346–350. doi: 10.1109/TMBMC.2023.3292321
Gutiérrez, D., Rodríguez-Rubio, L., Martínez, B., Rodríguez, A., García, P. (2016). Bacteriophages as weapons against bacterial biofilms in the food industry. Front. Microbiol. 7. doi: 10.3389/fmicb.2016.00825
Guttenplan, S. B., Blair, K. M., Kearns, D. B. (2010). The EpsE flagellar clutch is bifunctional and synergizes with EPS biosynthesis to promote B. subtilis biofilm formation. PloS Genet. 6, e1001243. doi: 10.1371/journal.pgen.1001243
Hanlon, G. W. (2007). Bacteriophages: An appraisal of their role in the treatment of bacterial infections. Int. J. Antimicrob. Agents 30, 118–128. doi: 10.1016/j.ijantimicag.2007.04.006
Hassan, A. Y., Lin, J. T., Ricker, N., Anany, H. (2021). The age of phage: Friend or foe in the new dawn of therapeutic and biocontrol applications? Pharm. (Basel) 14, 199. doi: 10.3390/ph14030199
Hibstu, Z., Belew, H., Akelew, Y., Mengist, H. M. (2022). Phage therapy: A different approach to fight bacterial infections. Biologics 16, 173–186. doi: 10.2147/BTT.S381237
Høiby, N., Ciofu, O., Johansen, H. K., Song, Z., Moser, C., Jensen, P.Ø., et al. (2011). The clinical impact of bacterial biofilms. Int. J. Oral. Sci. 3, 55–65. doi: 10.4248/IJOS11026
Holguín, A. V., Rangel, G., Clavijo, V., Prada, C., Mantilla, M., Gomez, M. C., et al. (2015). Phage ΦPan70, a putative temperate phage, controls P. aeruginosa in planktonic, biofilm and burn mouse model assays. Viruses 7, 4602–4623. doi: 10.3390/v7082835
Hufziger, K. A., Farquharson, E. L., Werner, B. G., Chen, Q., Goddard, J. M., Nugen, S. R. (2022). In vivo capsid engineering of bacteriophages for oriented surface conjugation. ACS Appl. Bio Mater. 5, 5104–5112. doi: 10.1021/acsabm.2c00428
Jaroni, D., Litt, P. K., Bule, P., Rumbaugh, K. (2023). Effectiveness of bacteriophages against biofilm-forming shiga-toxigenic E. coli in vitro and on food-contact surfaces. Foods 12, 2787. doi: 10.3390/foods12142787
Joo, H., Wu, S. M., Soni, I., Wang-Crocker, C., Matern, T., Beck, J. P., et al. (2023). Phage and antibiotic combinations reduce Staphylococcus aureus in static and dynamic biofilms grown on an implant material. Viruses 15, 460. doi: 10.3390/v15020460
Karimi, M., Eslami, M., Sahandi-Zangabad, P., Mirab, F., Farajisafiloo, N., Shafaei, Z., et al. (2016). pH-Sensitive stimulus-responsive nanocarriers for targeted delivery of therapeutic agents. Wiley Interdiscip. Rev. Nanomed. Nanobiotechnol. 8, 696–716. doi: 10.1002/wnan.1389
Karn, S. L., Bhartiya, S. K., Pratap, A., Saroj, S. K., Kumar, R., Sahu, M., et al. (2024). A randomized, placebo-controlled, double-blind clinical trial of bacteriophage cocktails in chronic wound infections. Int. J. Low. Extrem. Wounds, 15347346231226342. doi: 10.1177/15347346231226342
Karthikeyan, K. G., Meyer, M. T. (2006). Occurrence of antibiotics in wastewater treatment facilities in Wisconsin, USA. Sci. Total Environ. 361, 196–207. doi: 10.1016/j.scitotenv.2005.06.030
Kaszuba, M., Robinson, A. M., Song, Y. H., Creeth, J. E., Jones, M. N. (1997). The visualisation of the targeting of phospholipid liposomes to bacteria. Colloids Surfaces B: Biointerfaces 8, 321–332. doi: 10.1016/S0927-7765(97)00007-6
Kaur, S., Harjai, K., Chhibber, S. (2016). In vivo assessment of phage and linezolid based implant coatings for treatment of methicillin resistant S. aureus (MRSA) mediated orthopaedic device related infections. PloS One 11, e0157626. doi: 10.1371/journal.pone.0157626
Ke, C.-L., Deng, F.-S., Chuang, C.-Y., Lin, C.-H. (2021). Antimicrobial actions and applications of chitosan. Polym. (Basel) 13, 904. doi: 10.3390/polym13060904
Khalifa, L., Gelman, D., Shlezinger, M., Dessal, A. L., Coppenhagen-Glazer, S., Beyth, N., et al. (2018). Defeating antibiotic- and phage-resistant E. faecalis using a phage cocktail in vitro and in a clot model. Front. Microbiol. 9. doi: 10.3389/fmicb.2018.00326
Khambhati, K., Bhattacharjee, G., Gohil, N., Dhanoa, G. K., Sagona, A. P., Mani, I., et al. (2023). Phage engineering and phage-assisted CRISPR-Cas delivery to combat multidrug-resistant pathogens. Bioeng. Transl. Med. 8, e10381. doi: 10.1002/btm2.10381
Kim, U., Lee, S.-Y., Oh, S.-W. (2023b). A review of mechanism analysis methods in multi-species biofilm of foodborne pathogens. Food Sci. Biotechnol. 32, 1665–1677. doi: 10.1007/s10068-023-01317-x
Kim, B., Madukoma, C. S., Shrout, J. D., Nerenberg, R. (2023a). Effect of EPS production on the performance of membrane-based biofilm reactors. Water Res. 240, 120101. doi: 10.1016/j.watres.2023.120101
Kluzek, M., Oppenheimer-Shaanan, Y., Dadosh, T., Morandi, M. I., Avinoam, O., Raanan, C., et al. (2022). Designer liposomic nanocarriers are effective biofilm eradicators. ACS Nano 16, 15792–15804. doi: 10.1021/acsnano.2c04232
Knecht, L. E., Veljkovic, M., Fieseler, L. (2019). Diversity and function of phage encoded depolymerases. Front. Microbiol. 10. doi: 10.3389/fmicb.2019.02949
Krishnan, S., Patil, S. A., Nancharaiah, Y. V. (2023). “Environmental microbial biofilms,” in Material-Microbes Interactions (Cambridge, MA, USA: Elsevier), 3–45. doi: 10.1016/B978-0-323-95124-1.00013-9
Kushwaha, S. O., Sahu, S. K., Yadav, V. K., Rathod, M. C., Patel, D., Sahoo, D. K., et al. (2024). Bacteriophages as a potential substitute for antibiotics: A comprehensive review. Cell Biochem. Funct. 42, e4022. doi: 10.1002/cbf.4022
Kwiatek, M., Parasion, S., Rutyna, P., Mizak, L., Gryko, R., Niemcewicz, M., et al. (2017). Isolation of bacteriophages and their application to control P. aeruginosa in planktonic and biofilm models. Res. Microbiol. 168, 194–207. doi: 10.1016/j.resmic.2016.10.009
Laganenka, L., Sander, T., Lagonenko, A., Chen, Y., Link, H., Sourjik, V. (2019). Quorum sensing and metabolic state of the host control lysogeny-lysis switch of bacteriophage T1. MBio 10, e01884-19. doi: 10.1128/mBio.01884-19
Lee, H. J., Kim, H. J., Lee, S. J. (2022). Control of λ lysogenic E. coli cells by synthetic λ phage carrying cIantisense. ACS Synth. Biol. 11, 3829–3835. doi: 10.1021/acssynbio.2c00409
Lee, K. W. K., Periasamy, S., Mukherjee, M., Xie, C., Kjelleberg, S., Rice, S. A. (2014). Biofilm development and enhanced stress resistance of a model, mixed-species community biofilm. ISME J. 8, 894–907. doi: 10.1038/ismej.2013.194
Li, J., Zheng, H., Leung, S. S. Y. (2023). Potential of bacteriophage therapy in managing S. aureus infections during chemotherapy for lung cancer patients. Sci. Rep. 13, 9534. doi: 10.1038/s41598-023-36749-2
Li, L.-L., Yu, P., Wang, X., Yu, S.-S., Mathieu, J., Yu, H.-Q., et al. (2017). Enhanced biofilm penetration for microbial control by polyvalent phages conjugated with magnetic colloidal nanoparticle clusters (CNCs). Environ. Sci.: Nano 4, 1817–1826. doi: 10.1039/C7EN00414A
Li, Y., Xiao, P., Wang, Y., Hao, Y. (2020). Mechanisms and control measures of mature biofilm resistance to antimicrobial agents in the clinical context. ACS Omega 5, 22684–22690. doi: 10.1021/acsomega.0c02294
Li, Z., Wang, X., Wang, J., Yuan, X., Jiang, X., Wang, Y., et al. (2022). Bacterial biofilms as platforms engineered for diverse applications. Biotechnol. Adv. 57, 107932. doi: 10.1016/j.biotechadv.2022.107932
Limoli, D. H., Jones, C. J., Wozniak, D. J. (2015). Bacterial extracellular polysaccharides in biofilm formation and function. Microbiol. Spectr. 3. doi: 10.1128/microbiolspec.MB-0011-2014
Liu, M., Gill, J. J., Young, R., Summer, E. J. (2015). Bacteriophages of wastewater foaming-associated filamentous Gordonia reduce host levels in raw activated sludge. Sci. Rep. 5, 13754. doi: 10.1038/srep13754
Łobocka, M., Dąbrowska, K., Górski, A. (2021). Engineered bacteriophage therapeutics: Rationale, challenges and future. BioDrugs 35, 255–280. doi: 10.1007/s40259-021-00480-z
Loh, B., Gondil, V. S., Manohar, P., Khan, F. M., Yang, H., Leptihn, S. (2021). Encapsulation and delivery of therapeutic phages. Appl. Environ. Microbiol. 87, e01979-20. doi: 10.1128/AEM.01979-20
Lomelí-Ortega, C. O., Barajas-Sandoval, D. R., Martínez-Villalobos, J. M., Jaramillo, C. R., Chávez, E. M., Gómez-Gil, B., et al. (2023). A broad-host-range phage cocktail selectively and effectively eliminates Vibrio species from shrimp aquaculture environment. Microb. Ecol. 86, 1443–1446. doi: 10.1007/s00248-022-02118-1
Loponte, R., Pagnini, U., Iovane, G., Pisanelli, G. (2021). Phage therapy in veterinary medicine. Antibiot. (Basel) 10, 421. doi: 10.3390/antibiotics10040421
Lu, H., Li, Z., Elbaz, A., Ni, S.-Q. (2023). Synergistic action of phages and lytic proteins with antibiotics: A combination strategy to target bacteria and biofilms. BMC Microbiol. 23, 149. doi: 10.1186/s12866-023-02881-2
Lu, T. K., Collins, J. J. (2007). Dispersing biofilms with engineered enzymatic bacteriophage. Proc. Natl. Acad. Sci. U.S.A. 104, 11197–11202. doi: 10.1073/pnas.0704624104
Lu, Y., Cai, W.-J., Ren, Z., Han, P. (2022). The role of staphylococcal biofilm on the surface of implants in orthopedic infection. Microorganisms 10, 1909. doi: 10.3390/microorganisms10101909
Mabrouk, S., Abdellatif, G. R., Abu Zaid, A. S., Aziz, R. K., Aboshanab, K. M. (2022). In Vitro and pre-clinical evaluation of locally isolated phages, vB_Pae_SMP1 and vB_Pae_SMP5, formulated as hydrogels against carbapenem-resistant P. aeruginosa. Viruses 14, 2760. doi: 10.3390/v14122760
Magaziner, S. J., Salmond, G. P. C. (2022). A novel T4- and λ-based receptor binding protein family for bacteriophage therapy host range engineering. Front. Microbiol. 13. doi: 10.3389/fmicb.2022.1010330
Mah, T. F., O’Toole, G. A. (2001). Mechanisms of biofilm resistance to antimicrobial agents. Trends Microbiol. 9, 34–39. doi: 10.1016/S0966-842X(00)01913-2
Maje, M. D., Kaptchouang Tchatchouang, C. D., Manganyi, M. C., Fri, J., Ateba, C. N. (2020). Characterisation of Vibrio species from surface and drinking water sources and assessment of biocontrol potentials of their bacteriophages. Int. J. Microbiol. 2020, 1–15. doi: 10.1155/2020/8863370
Malik, D. J., Sokolov, I. J., Vinner, G. K., Mancuso, F., Cinquerrui, S., Vladisavljevic, G. T., et al. (2017). Formulation, stabilisation and encapsulation of bacteriophage for phage therapy. Adv. Colloid Interface Sci. 249, 100–133. doi: 10.1016/j.cis.2017.05.014
Mancuso, G., Midiri, A., Gerace, E., Biondo, C. (2021). Bacterial antibiotic resistance: The most critical pathogens. Pathogens 10, 1310. doi: 10.3390/pathogens10101310
Manoharadas, S., Altaf, M., Alrefaei, A. F., Devasia, R. M., Badjah Hadj, A. Y. M., Abuhasil, M. S. A. (2021). Concerted dispersion of S. aureus biofilm by bacteriophage and “green synthesized” silver nanoparticles. RSC Adv. 11, 1420–1429. doi: 10.1039/D0RA09725J
Matamp, N., Bhat, S. G. (2019). Phage endolysins as potential antimicrobials against multidrug resistant Vibrio alginolyticus and Vibrio parahaemolyticus: Current status of research and challenges ahead. Microorganisms 7, 84. doi: 10.3390/microorganisms7030084
Matsuda, T., Freeman, T. A., Hilbert, D. W., Duff, M., Fuortes, M., Stapleton, P. P., et al. (2005). Lysis-deficient bacteriophage therapy decreases endotoxin and inflammatory mediator release and improves survival in a murine peritonitis model. Surgery 137, 639–646. doi: 10.1016/j.surg.2005.02.012
Meade, E., Savage, M., Garvey, M. (2020). Investigation of alternative biocidal options against foodborne multidrug resistant pathogens. Eur. J. Exp. Biol. 10, 2248–9215. doi: 10.36648/2248-9215.10.3.105
Meneses, L., Brandão, A. C., Coenye, T., Braga, A. C., Pires, D. P., Azeredo, J. (2023). A systematic review of the use of bacteriophages for in vitro biofilm control. Eur. J. Clin. Microbiol. Infect. Dis. 42, 919–928. doi: 10.1007/s10096-023-04638-1
Milho, C., Silva, M. D., Alves, D., Oliveira, H., Sousa, C., Pastrana, L. M., et al. (2019). E. coli and Salmonella enteritidis dual-species biofilms: Interspecies interactions and antibiofilm efficacy of phages. Sci. Rep. 9, 18183. doi: 10.1038/s41598-019-54847-y
Mohanta, Y. K., Chakrabartty, I., Mishra, A. K., Chopra, H., Mahanta, S., Avula, S. K., et al. (2023). Nanotechnology in combating biofilm: A smart and promising therapeutic strategy. Front. Microbiol. 13. doi: 10.3389/fmicb.2022.1028086
Morrisette, T., Kebriaei, R., Lev, K. L., Morales, S., Rybak, M. J. (2020). Bacteriophage therapeutics: A primer for clinicians on phage-antibiotic combinations. Pharmacotherapy 40, 153–168. doi: 10.1002/phar.2358
Moye, Z. D., Woolston, J., Sulakvelidze, A. (2018). Bacteriophage applications for food production and processing. Viruses 10, 205. doi: 10.3390/v10040205
Mukhopadhyay, S., To, K. K. W., Liu, Y., Bai, C., Leung, S. S. Y. (2023). A thermosensitive hydrogel formulation of phage and colistin combination for the management of multidrug-resistant A. baumannii wound infections. Biomater. Sci. 12, 151–163. doi: 10.1039/D3BM01383A
Mura, S., Nicolas, J., Couvreur, P. (2013). Stimuli-responsive nanocarriers for drug delivery. Nat. Mater. 12, 991–1003. doi: 10.1038/nmat3776
Naknaen, A., Samernate, T., Wannasrichan, W., Surachat, K., Nonejuie, P., Chaikeeratisak, V. (2023). Combination of genetically diverse Pseudomonas phages enhances the cocktail efficiency against bacteria. Sci. Rep. 13, 8921. doi: 10.1038/s41598-023-36034-2
Ngiam, L., Weynberg, K. D., Guo, J. (2022). The presence of plasmids in bacterial hosts alters phage isolation and infectivity. ISME Commun. 2, 75. doi: 10.1038/s43705-022-00158-9
Nie, P., Alarcon, F., López-Montero, I., Orgaz, B., Valeriani, C., Pica Ciamarra, M. (2021). In-silico modeling of early-stage biofilm formation. Soft Mater. 19, 346–358. doi: 10.1080/1539445X.2021.1887220
Oechslin, F., Piccardi, P., Mancini, S., Gabard, J., Moreillon, P., Entenza, J. M., et al. (2017). Synergistic interaction between phage therapy and antibiotics clears P. aeruginosa infection in endocarditis and reduces virulence. J. Infect. Dis. 215, 703–712. doi: 10.1093/infdis/jiw632
Ooi, M. L., Drilling, A. J., Morales, S., Fong, S., Moraitis, S., Macias-Valle, L., et al. (2019). Safety and tolerability of bacteriophage therapy for chronic rhinosinusitis due to S. aureus. JAMA Otolaryngol. Head Neck Surg. 145, 723–729. doi: 10.1001/jamaoto.2019.1191
Otero, J., García-Rodríguez, A., Cano-Sarabia, M., Maspoch, D., Marcos, R., Cortés, P., et al. (2019). Biodistribution of liposome-encapsulated bacteriophages and their transcytosis during oral phage therapy. Front. Microbiol. 10. doi: 10.3389/fmicb.2019.00689
Oyewole, O. A., Raji, R. O., Yakubu, J. G. (2022). “The role of quorum sensing in microbial biofilm formation,” in Microbial biofilms: Applications and control (CRC Press, Boca Raton), 47–63. doi: 10.1201/9781003184942-4
Papaianni, M., Cuomo, P., Fulgione, A., Albanese, D., Gallo, M., Paris, D., et al. (2020). Bacteriophages promote metabolic changes in bacteria biofilm. Microorganisms 8, 480. doi: 10.3390/microorganisms8040480
Pawde, D. M., Viswanadh, M. K., Mehata, A. K., Sonkar, R., Narendra, Poddar, S., et al. (2020). Mannose receptor targeted bioadhesive chitosan nanoparticles of clofazimine for effective therapy of tuberculosis. Saudi Pharm. J. 28, 1616–1625. doi: 10.1016/j.jsps.2020.10.008
Penesyan, A., Paulsen, I. T., Kjelleberg, S., Gillings, M. R. (2021). Three faces of biofilms: a microbial lifestyle, a nascent multicellular organism, and an incubator for diversity. NPJ Biofilms Microbiomes 7, 80. doi: 10.1038/s41522-021-00251-2
Petrovski, S., Seviour, R. J., Tillett, D. (2011). Prevention of Gordonia and Nocardia stabilized foam formation by using bacteriophage GTE7. Appl. Environ. Microbiol. 77, 7864–7867. doi: 10.1128/AEM.05692-11
Pires, D. P., Costa, A. R., Pinto, G., Meneses, L., Azeredo, J. (2020). Current challenges and future opportunities of phage therapy. FEMS Microbiol. Rev. 44, 684–700. doi: 10.1093/femsre/fuaa017
Pires, D. P., Oliveira, H., Melo, L. D. R., Sillankorva, S., Azeredo, J. (2016). Bacteriophage-encoded depolymerases: their diversity and biotechnological applications. Appl. Microbiol. Biotechnol. 100, 2141–2151. doi: 10.1007/s00253-015-7247-0
Quan, K., Zhang, Z., Chen, H., Ren, X., Ren, Y., Peterson, B. W., et al. (2019). Artificial channels in an infectious biofilm created by magnetic nanoparticles enhanced bacterial killing by antibiotics. Small 15, e1902313. doi: 10.1002/smll.201902313
Racenis, K., Lacis, J., Rezevska, D., Mukane, L., Vilde, A., Putnins, I., et al. (2023). Successful bacteriophage-antibiotic combination therapy against multidrug-resistant P. aeruginosa left ventricular assist device driveline infection. Viruses 15, 1210. doi: 10.3390/v15051210
Ramzan, A., Rehman, T., But, M. S., Imran, M., Qaisar, U. (2022). Biofilm formation and its regulation by extracellular appendages in P. aeruginosa. World J. Biol. Biotechnol. 7, 1. doi: 10.33865/wjb.007.03.0516
Ratnakar, I. S. (2022). Phage therapy: Challenges and opportunities. Fine Focus 8, 12–35. doi: 10.33043/FF.8.1.12-35
Rendueles, O., de Sousa, J. A. M., Rocha, E. P. C. (2023). Competition between lysogenic and sensitive bacteria is determined by the fitness costs of the different emerging phage-resistance strategies. eLife 12, e83479. doi: 10.7554/eLife.83479
Richter, L. V., Franks, A. E., Weis, R. M., Sandler, S. J. (2017). Significance of a posttranslational modification of the PilA protein of geobacter sulfurreducens for surface attachment, biofilm formation, and growth on insoluble extracellular electron acceptors. J. Bacteriol. 199, e00716-16. doi: 10.1128/JB.00716-16
Rohr, J. R., Barrett, C. B., Civitello, D. J., Craft, M. E., Delius, B., DeLeo, G. A., et al. (2019). Emerging human infectious diseases and the links to global food production. Nat. Sustain. 2, 445–456. doi: 10.1038/s41893-019-0293-3
Rolston, K. V. I. (2017). Infections in cancer patients with solid tumors: A Review. Infect. Dis. Ther. 6, 69–83. doi: 10.1007/s40121-017-0146-1
Römling, U., Balsalobre, C. (2012). Biofilm infections, their resilience to therapy and innovative treatment strategies. J. Intern. Med. 272, 541–561. doi: 10.1111/joim.12004
Rumbaugh, K. P., Sauer, K. (2020). Biofilm dispersion. Nat. Rev. Microbiol. 18, 571–586. doi: 10.1038/s41579-020-0385-0
Schoeffel, J., Wang, E. W., Gill, D., Frackler, J., Horne, B., Manson, T., et al. (2022). Successful use of salvage bacteriophage therapy for a recalcitrant MRSA knee and hip prosthetic joint infection. Pharm. (Basel) 15, 177. doi: 10.3390/ph15020177
Schooley, R. T., Biswas, B., Gill, J. J., Hernandez-Morales, A., Lancaster, J., Lessor, L., et al. (2017). Development and use of personalized bacteriophage-based therapeutic cocktails to treat a patient with a disseminated resistant A. baumannii infection. Antimicrob. Agents Chemother. 61. doi: 10.1128/AAC.00954-17
Schulze, A., Mitterer, F., Pombo, J. P., Schild, S. (2021). Biofilms by bacterial human pathogens: Clinical relevance - development, composition and regulation - therapeutical strategies. Microb. Cell 8, 28–56. doi: 10.15698/mic2021.02.741
Secor, P. R., Burgener, E. B., Kinnersley, M., Jennings, L. K., Roman-Cruz, V., Popescu, M., et al. (2020). Pf bacteriophage and their impact on Pseudomonas virulence, mammalian immunity, and chronic infections. Front. Immunol. 11. doi: 10.3389/fimmu.2020.00244
Shafigh Kheljan, F., Sheikhzadeh Hesari, F., Aminifazl, M. S., Skurnik, M., Gholadze, S., Zarrini, G. (2023). Design of phage-cocktail-containing hydrogel for the treatment of P. aeruginosa-infected wounds. Viruses 15, 803. doi: 10.3390/v15030803
Sharifi-Rad, J., Quispe, C., Butnariu, M., Rotariu, L. S., Sytar, O., Sestito, S., et al. (2021). Chitosan nanoparticles as a promising tool in nanomedicine with particular emphasis on oncological treatment. Cancer Cell Int. 21, 318. doi: 10.1186/s12935-021-02025-4
Sharma, D., Misba, L., Khan, A. U. (2019). Antibiotics versus biofilm: An emerging battleground in microbial communities. Antimicrob. Resist. Infect. Control 8, 76. doi: 10.1186/s13756-019-0533-3
Shivaram, K. B., Bhatt, P., Applegate, B., Simsek, H. (2023). Bacteriophage-based biocontrol technology to enhance the efficiency of wastewater treatment and reduce targeted bacterial biofilms. Sci. Total Environ. 862, 160723. doi: 10.1016/j.scitotenv.2022.160723
Sihorkar, V., Vyas, S. P. (2001). Biofilm consortia on biomedical and biological surfaces: delivery and targeting strategies. Pharm. Res. 18, 1247–1254. doi: 10.1023/A:1013073508318
Sillankorva, S., Neubauer, P., Azeredo, J. (2010). Phage control of dual species biofilms of Pseudomonas fluorescens and Staphylococcus lentus. Biofouling 26, 567–575. doi: 10.1080/08927014.2010.494251
Singh, A., Amod, A., Pandey, P., Bose, P., Pingali, M. S., Shivalkar, S., et al. (2022a). Bacterial biofilm infections, their resistance to antibiotics therapy and current treatment strategies. Biomed. Mater. 17, 022003. doi: 10.1088/1748-605X/ac50f6
Singh, A., Padmesh, S., Dwivedi, M., Kostova, I. (2022b). How good are bacteriophages as an alternative therapy to mitigate biofilms of nosocomial infections. Infect. Drug Resist. 15, 503–532. doi: 10.2147/IDR.S348700
Singla, S., Harjai, K., Katare, O. P., Chhibber, S. (2016). Encapsulation of bacteriophage in liposome accentuates its entry in to macrophage and shields it from neutralizing antibodies. PloS One 11, e0153777. doi: 10.1371/journal.pone.0153777
Srisangthong, I., Sangseedum, C., Chaichanit, N., Surachat, K., Suanyuk, N., Mittraparp-Arthorn, P. (2023). Characterization and genome analysis of Vibrio campbellii lytic bacteriophage OPA17. Microbiol. Spectr. 11, e0162322. doi: 10.1128/spectrum.01623-22
Srivastava, A., Chandra, N., Kumar, S. (2019). “The role of biofilms in medical devices and implants,” in Biofilms in human diseases: Treatment and control. Eds. Kumar, S., Chandra, N., Singh, L., Hashmi, M. Z., Varma, A. (Springer International Publishing, Cham), 151–165. doi: 10.1007/978-3-030-30757-8_11
Suh, G. A., Lodise, T. P., Tamma, P. D., Knisely, J. M., Alexander, J., Aslam, S., et al. (2022). Considerations for the use of phage therapy in clinical practice. Antimicrob. Agents Chemother. 66, e0207121. doi: 10.1128/aac.02071-21
Sun, R., Yu, P., Zuo, P., Villagrán, D., Mathieu, J., Alvarez, P. J. J. (2022). Biofilm control in flow-through systems using polyvalent phages delivered by peptide-modified M13 coliphages with enhanced polysaccharide affinity. Environ. Sci. Technol. 56, 17177–17187. doi: 10.1021/acs.est.2c06561
Sutherland, I. W., Hughes, K. A., Skillman, L. C., Tait, K. (2004). The interaction of phage and biofilms. FEMS Microbiol. Lett. 232, 1–6. doi: 10.1016/S0378-1097(04)00041-2
Talapko, J., Škrlec, I. (2020). The principles, mechanisms, and benefits of unconventional agents in the treatment of biofilm infection. Pharm. (Basel) 13, 299. doi: 10.3390/ph13100299
Teklemariam, A. D., Al-Hindi, R. R., Qadri, I., Alharbi, M. G., Ramadan, W. S., Ayubu, J., et al. (2023). The battle between bacteria and bacteriophages: A conundrum to their immune system. Antibiot. (Basel) 12, 381. doi: 10.3390/antibiotics12020381
Tian, F., Li, J., Nazir, A., Tong, Y. (2021). Bacteriophage - a promising alternative measure for bacterial biofilm control. Infect. Drug Resist. 14, 205–217. doi: 10.2147/IDR.S290093
Tinoco, J. M., Buttaro, B., Zhang, H., Liss, N., Sassone, L., Stevens, R. (2016). Effect of a genetically engineered bacteriophage on E. faecalis biofilms. Arch. Oral. Biol. 71, 80–86. doi: 10.1016/j.archoralbio.2016.07.001
Tkhilaishvili, T., Lombardi, L., Klatt, A.-B., Trampuz, A., Di Luca, M. (2018). Bacteriophage Sb-1 enhances antibiotic activity against biofilm, degrades exopolysaccharide matrix and targets persisters of S. aureus. Int. J. Antimicrob. Agents 52, 842–853. doi: 10.1016/j.ijantimicag.2018.09.006
Tkhilaishvili, T., Merabishvili, M., Pirnay, J.-P., Starck, C., Potapov, E., Falk, V., et al. (2021). Successful case of adjunctive intravenous bacteriophage therapy to treat left ventricular assist device infection. J. Infect. 83, e1–e3. doi: 10.1016/j.jinf.2021.05.027
Topka-Bielecka, G., Dydecka, A., Necel, A., Bloch, S., Nejman-Faleńczyk, B., Węgrzyn, G., et al. (2021). Bacteriophage-derived depolymerases against bacterial biofilm. Antibiot. (Basel) 10, 175. doi: 10.3390/antibiotics10020175
Uchiyama, J., Takemura-Uchiyama, I., Kato, S.-I., Sato, M., Ujihara, T., Matsui, H., et al. (2014). In silico analysis of AHJD-like viruses, S. aureus phages S24-1 and S13’, and study of phage S24-1 adsorption. Microbiologyopen 3, 257–270. doi: 10.1002/mbo3.166
Usui, M., Yoshii, Y., Thiriet-Rupert, S., Ghigo, J.-M., Beloin, C. (2023). Intermittent antibiotic treatment of bacterial biofilms favors the rapid evolution of resistance. Commun. Biol. 6, 275. doi: 10.1038/s42003-023-04601-y
Van Nieuwenhuyse, B., Galant, C., Brichard, B., Docquier, P.-L., Djebara, S., Pirnay, J.-P., et al. (2021). A case of in situ phage therapy against S. aureus in a bone allograft polymicrobial biofilm infection: Outcomes and phage-antibiotic interactions. Viruses 13, 1898. doi: 10.3390/v13101898
Vera-González, N., Shukla, A. (2020). Advances in biomaterials for the prevention and disruption of candida biofilms. Front. Microbiol. 11. doi: 10.3389/fmicb.2020.538602
Vikram, A., Woolston, J., Sulakvelidze, A. (2021). Phage biocontrol applications in food production and processing. Curr. Issues Mol. Biol. 40, 267–302. doi: 10.21775/cimb.040.267
Wang, I. N., Smith, D. L., Young, R. (2000). Holins: The protein clocks of bacteriophage infections. Annu. Rev. Microbiol. 54, 799–825. doi: 10.1146/annurev.micro.54.1.799
Wang, J., Zhao, S., Chen, J., Liu, X., Chen, H., Lu, T., et al. (2023). Phage-Ce6-manganese dioxide nanocomposite-mediated photodynamic, photothermal, and chemodynamic therapies to eliminate biofilms and improve wound healing. ACS Appl. Mater. Interfaces 15, 21904–21916. doi: 10.1021/acsami.3c01762
Waturangi, D. E., Kasriady, C. P., Guntama, G., Sahulata, A. M., Lestari, D., Magdalena, S. (2021). Application of bacteriophage as food preservative to control enteropathogenic E. coli (EPEC). BMC Res. Notes 14, 336. doi: 10.1186/s13104-021-05756-9
Weinbauer, M. G., Brettar, I., Höfle, M. G. (2003). Lysogeny and virus-induced mortality of bacterioplankton in surface, deep, and anoxic marine waters. Limnol. Oceanogr. 48, 1457–1465. doi: 10.4319/lo.2003.48.4.1457
Werneburg, G. T., Nguyen, A., Henderson, N., Sueur, A. L., Corcoran, A., Katz, A., et al. (2019). Progression and formation of bacterial biofilms on indwelling urinary catheters over time. J. Urol. 201, e1136. doi: 10.1097/01.JU.0000557322.77175.6f
Williams, J., Kerven, J., Chen, Y., Sagona, A. P. (2023). Genetic engineering of bacteriophage K1F with human epidermal growth factor to enhance killing of intracellular E. coli K1. ACS Synth. Biol. 12, 2094–2106. doi: 10.1021/acssynbio.3c00135
Wroe, J. A., Johnson, C. T., García, A. J. (2020). Bacteriophage delivering hydrogels reduce biofilm formation in vitro and infection in vivo. J. Biomed. Mater. Res. A 108, 39–49. doi: 10.1002/jbm.a.36790
Wu, B., Wang, R., Fane, A. G. (2017). The roles of bacteriophages in membrane-based water and wastewater treatment processes: A review. Water Res. 110, 120–132. doi: 10.1016/j.watres.2016.12.004
Xiu, W., Wan, L., Yang, K., Li, X., Yuwen, L., Dong, H., et al. (2022). Potentiating hypoxic microenvironment for antibiotic activation by photodynamic therapy to combat bacterial biofilm infections. Nat. Commun. 13, 3875. doi: 10.1038/s41467-022-31479-x
Yan, J., Mao, J., Xie, J. (2014). Bacteriophage polysaccharide depolymerases and biomedical applications. BioDrugs 28, 265–274. doi: 10.1007/s40259-013-0081-y
Yan, W., Banerjee, P., Xu, M., Mukhopadhyay, S., Ip, M., Carrigy, N. B., et al. (2021). Formulation strategies for bacteriophages to target intracellular bacterial pathogens. Adv. Drug Deliv. Rev. 176, 113864. doi: 10.1016/j.addr.2021.113864
Yang, L., Liu, Y., Wu, H., Song, Z., Høiby, N., Molin, S., et al. (2012). Combating biofilms. FEMS Immunol. Med. Microbiol. 65, 146–157. doi: 10.1111/j.1574-695X.2011.00858.x
Yasir, M., Willcox, M. D. P., Dutta, D. (2018). Action of antimicrobial peptides against bacterial biofilms. Mater. (Basel) 11, 2468. doi: 10.3390/ma11122468
Yin, W., Wang, Y., Liu, L., He, J. (2019). Biofilms: The microbial “protective clothing” in extreme environments. Int. J. Mol. Sci. 20, 3423. doi: 10.3390/ijms20143423
Yue, H., Li, Y., Yang, M., Mao, C. (2022). T7 phage as an emerging nanobiomaterial with genetically tunable target specificity. Adv. Sci. (Weinh) 9, e2103645. doi: 10.1002/advs.202103645
Zaczek-Moczydłowska, M. A., Young, G. K., Trudgett, J., Plahe, C., Fleming, C. C., Campbell, K., et al. (2020). Phage cocktail containing podoviridae and myoviridae bacteriophages inhibits the growth of Pectobacterium spp. under in vitro and in vivo conditions. PloS One 15, e0230842. doi: 10.1371/journal.pone.0230842
Zhang, L. (2018). “The use of biological agents in processing: Antimicrobials, fermentation, and antagonistic control,” in Packaging for nonthermal processing of food. Eds. Pascall, M. A., Han, J. H. (John Wiley & Sons, Ltd, Chichester, UK), 83–94. doi: 10.1002/9781119126881.ch5
Zhang, Y., Gao, J., Wang, L., Liu, S., Bai, Z., Zhuang, X., et al. (2018). Environmental adaptability and quorum sensing: Iron uptake regulation during biofilm formation by Paracoccus denitrificans. Appl. Environ. Microbiol. 84, e00865-18. doi: 10.1128/AEM.00865-18
Zhang, Y., Hu, Z. (2013). Combined treatment of P. aeruginosa biofilms with bacteriophages and chlorine. Biotechnol. Bioeng. 110, 286–295. doi: 10.1002/bit.24630
Zhao, M., Tan, X., Liu, Z.-Q., Dou, L., Liu, D., Pan, Y.-J., et al. (2023). Engineered phage with cell-penetrating peptides for intracellular bacterial infections. mSystems 8, e0064623. doi: 10.1128/msystems.00646-23
Keywords: bacteriophages, biofilms, antimicrobial design, antibiotic resistance, exopolysaccharide
Citation: Mayorga-Ramos A, Carrera-Pacheco SE, Barba-Ostria C and Guamán LP (2024) Bacteriophage-mediated approaches for biofilm control. Front. Cell. Infect. Microbiol. 14:1428637. doi: 10.3389/fcimb.2024.1428637
Received: 06 May 2024; Accepted: 29 July 2024;
Published: 07 October 2024.
Edited by:
Maria Gabriela Paraje, National University of Cordoba, ArgentinaReviewed by:
Marina Alejandra Golowczyc, Independent Researcher, La Plata, ArgentinaAshish Patel, Hemchandracharya North Gujarat University, India
İhsan Yaşa, Ege University, Türkiye
Copyright © 2024 Mayorga-Ramos, Carrera-Pacheco, Barba-Ostria and Guamán. This is an open-access article distributed under the terms of the Creative Commons Attribution License (CC BY). The use, distribution or reproduction in other forums is permitted, provided the original author(s) and the copyright owner(s) are credited and that the original publication in this journal is cited, in accordance with accepted academic practice. No use, distribution or reproduction is permitted which does not comply with these terms.
*Correspondence: Linda P. Guamán, bGluZGEuZ3VhbWFuQHV0ZS5lZHUuZWM=