- 1Grupo de Investigación INPAC, Unidad de Investigación, Fundación Universitaria Sanitas, Bogotá, Colombia
- 2Dirección Académica, Universidad Nacional de Colombia, Sede de La Paz, La Paz, Colombia
- 3Grupo de Investigación INPAC, Specialized Laboratory, Clinica Universitaria Colombia, Clínica Colsanitas S.A., Bogotá, Colombia
- 4Keralty, Sanitas International Organization, Grupo de Investigación INPAC, Fundación Universitaria Sanitas, Bogotá, Colombia
- 5Infectious Diseases Department, Clinica Universitaria Colombia, Clínica Colsanitas S.A., Bogotá, Colombia
Background: The diagnosis and treatment of lung, colon, and gastric cancer through the histologic characteristics and genomic biomarkers have not had a strong impact on the mortality rates of the top three global causes of death by cancer.
Methods: Twenty-five transcriptomic analyses (10 lung cancer, 10 gastric cancer, and 5 colon cancer datasets) followed our own bioinformatic pipeline based on the utilization of specialized libraries from the R language and DAVID´s gene enrichment analyses to identify a regulatory metafirm network of transcription factors and target genes common in every type of cancer, with experimental evidence that supports its relationship with the unlocking of cell phenotypic plasticity for the acquisition of the hallmarks of cancer during the tumoral process. The network’s regulatory functional and signaling pathways might depend on the constant crosstalk with the microbiome network established in the oral-gut-lung axis.
Results: The global transcriptomic network analysis highlighted the impact of transcription factors (SOX4, TCF3, TEAD4, ETV4, and FOXM1) that might be related to stem cell programming and cancer progression through the regulation of the expression of genes, such as cancer-cell membrane receptors, that interact with several microorganisms, including human T-cell leukemia virus 1 (HTLV-1), the human papilloma virus (HPV), the Epstein–Barr virus (EBV), and SARS−CoV−2. These interactions can trigger the MAPK, non-canonical WNT, and IFN signaling pathways, which regulate key transcription factor overexpression during the establishment and progression of lung, colon, and gastric cancer, respectively, along with the formation of the microbiome network.
Conclusion: The global transcriptomic network analysis highlights the important interaction between key transcription factors in lung, colon, and gastric cancer, which regulates the expression of cancer-cell membrane receptors for the interaction with the microbiome network during the tumorigenic process.
1 Introduction
Cancers are a leading cause of mortality, accounting for 10 million deaths worldwide every year. Most cancer deaths (75.1%) occur in low- and middle-income countries, where capital spending is lower at 49.5%. In high income countries like the US, where the cancer mortality rate is lower than the median, twice the amount of capital is spent on cancer care than in low-income countries, in an attempt to improve cancer outcomes (Chow et al., 2022). The estimated global economic cost of cancers between 2020 and 2050 is $25.2 trillion international dollars. The three cancers with the highest economic investment are tracheal, bronchus, and lung cancer (15.4%); colon and rectum cancer (10.9%); and breast cancer (7.7%) (Chen et al., 2023). Lung cancer has the second highest incidence (11.4% new cases) after breast cancer (11.7%) and is the leading cause of cancer-associated deaths worldwide (18%) (Siegel et al., 2023). Colon cancer has the third highest incidence (10%) and is the second leading cause of cancer-associated deaths worldwide (9.4%) (Siegel et al., 2023). Gastric cancer has the fifth highest incidence (5.6%), after breast, lung, colorectum and prostate cancer, and is the third leading cause of cancer-associated deaths worldwide (7.7%) (Siegel et al., 2023).
Approximately 80–85% of lung cancers are non-small cell lung cancer (NSCLC), which has three main subtypes: adenocarcinoma, squamous cell carcinoma, and large cell carcinoma. Approximately 10–15% of all lung cancers are small cell lung cancer (SCLC), and its main subtypes are small cell carcinoma and mixed small cell/large cell cancer or combined small cell lung cancer (Zhang et al., 2023). The regions with the highest risk of lung cancer are Polynesia, Micronesia, Eastern Asia, Europe, and Northern America (Siegel et al., 2023). The distinction between SCLC and NSCLC is a key parameter in the therapeutic management of lung cancer, selecting chemotherapy regimens in NSCLC patients lacking targetable EGFR and BRAF mutations, ALK and ROS1 rearrangements, and PD-L1 overexpression (Galli and Rossi, 2021). Targeted therapies based on druggable oncogenic molecular alterations and immunotherapy interfering with the PD1/PD-L1 checkpoint are used in lung cancer treatment (Salmani-Javan et al., 2024).
Colon cancer has three major histological types: adenocarcinoma, mucinous adenocarcinoma, and signet ring cell carcinoma (Wu et al., 2019). Adenocarcinoma originating from epithelial cells of the colorectal mucosa is the most frequent (>90%) type, and well differentiated tumors (>95%) are characterized by glandular formation (Rawla et al., 2019). Rare types include neuroendocrine, squamous cell, adenosquamous, spindle cell, and undifferentiated carcinomas (Fleming et al., 2012). The regions with the highest risk of colon cancer are Europe, Eastern Asia, and North and South America (Siegel et al., 2023). Adenocarcinoma patients have the best prognoses, whereas signet ring cell carcinoma patients have the poorest prognoses, and some patients with these different pathological types have no statistical difference at the early stages of the disease, suggesting that, right now, different treatment strategies specific for a particular histological subset should be applied only in the advanced stages of colon cancer (Ackermann et al., 2018). Currently, there are no established clinical guidelines for the treatment of the different pathological types, as previous studies on the prognostic effects have yielded conflicting results (Ackermann et al., 2018).
Gastric cancer classifications [intestinal type, diffuse type, and mixed type (Lauren, 1965) and the World Health Organization (mixed gastric mucinous adenocarcinoma) (Kushima, 2022)] have been used in clinicopathological diagnoses (Meng et al., 2022a). The well differentiated intestinal type is sporadic and highly associated with environmental factors, especially Helicobacter pylori infection (Takenaka et al., 2007). The diffuse type is undifferentiated and characterized by the loss of expression of the adhesion protein E-cadherin, which is related to tumor metastasis (Guilford et al., 1998). Gastric adenocarcinomas are the most frequent (>90%) and are classified as cardia and non-cardia based on their anatomic site, and the main subtypes are intestinal and diffuse (Yang et al., 2011). The regions with the highest risk of gastric cancer risk are East Asia, Eastern Europe, and Central and South America (Siegel et al., 2023). The incidence varies between regions, showing a high heterogeneity attributed to the commensal and infectious microbiota community, environmental factors, epigenetic programming, and genetic traits such as immune response receptors characteristic of every population ancestry (Otálora-Otálora et al., 2023b). Gastric cancer treatment includes chemotherapy, molecularly targeted therapies, and therapeutic agents such as immune checkpoint inhibitors (ICIs) selected for every patient as humanized monoclonal antibodies that target inhibitory receptors (CTLA-4, PD-1, LAG-3, TIM-3, and PD-L1) expressed on T lymphocytes, antigen presenting cells, and tumor cells, where they elicit an anti-tumor response by stimulating the immune system (Franzin et al., 2020); they are effective in the microsatellite instability and ICI subtypes but quite ineffective in the GS subtype (Kim et al., 2018).
The treatment of gut and lung cancer through histologic characteristics is limited by the high tumor morphological heterogeneity making every case extremely unique, and the identification of genomic biomarkers (Otálora-Otálora et al., 2023b) is still limited by the small number of patients with a specific variation, thus this therapeutic approach has not significantly impacted the mortality rates of the top three global causes of cancer deaths (Siegel et al., 2023). The identification of targetable oncogenic drivers in solid tumor and liquid biopsy using high-throughput deep sequencing methods can change significantly the paradigm of histology determination as one of the mandatory steps in future therapeutic strategies, and genomics as the only sequencing technology involved in the development of treatments against cancer (Galli and Rossi, 2021). We have developed a bioinformatics pipeline that is capable of identifying the most frequently differentially expressed genes (DEGs) and transcription factors (TFs) during the establishment and progression of every type of tumor pathology as key biomarkers due to their association with the regulation of biological processes and signaling pathways related to the acquisition of the hallmarks of cancer (Otálora-Otálora et al., 2023a). We address the great complexity of cancer in a holistic way from the transcriptome. The transcriptome reflects the genetics, epigenetics, and microenvironment of tumor cells that largely determine their phenotype. The identification of co-expressed common and unique DEGs between cancer types and related inflammatory diseases (Otálora-Otálora et al., 2019, Otálora-Otálora et al., 2022a, Otálora-Otálora et al., 2022b, Otálora-Otálora et al., 2023a) helps to identify the following: gene regulatory networks (GRNs), which contain promising targets for the pharmaceutical treatment of cancer (Zhou et al., 2019); co-regulatory networks (CRNs), which contain protein-protein interaction complexes of TFs regulating transcription at every stage of the tumorigenic process (Otálora-Otálora et al., 2023a); and transcriptional regulatory networks (TRNs), which represent the group of TFs that can regulate the all-important DEGs for the acquisition of the hallmarks of cancer (Otálora-Otálora et al., 2023b).
The polymorphic microbiome is a new dimension included in the hallmarks of cancer, along with epigenetic reprogramming, and both are considered constitute distinctive enabling characteristics that facilitate the acquisition of the hallmarks of cancer (Figure 1) (Hanahan, 2022). Our bioinformatic pipeline has evolved to identify a transcriptional regulatory metafirm of gut and lung cancer, the regulatory function of which might depend on the constant crosstalk with the microbiome network established in the gut-lung axis (Otálora-Otálora et al., 2023b). In this study, we aim to achieve the following: 1) identify the common DEGs in gut and lung cancers, highlighting the membrane receptors that are key for the communication between the host cells and the microbiota, and the downstream activation of signaling pathways involved in the regulation of gene expression, particularly the expression of key TFs that control the expression of the other DEGs in every type of cancer; 2) identify the regulatory interactions between the key TFs in a TRN as functional blocks of genes co-expressed for the control of gene expression and the acquisition of the hallmarks of cancer, and analyze its regulatory function over the other DEGs; and 3) perform a global transcriptomic network analysis combining the results of gene ontology and network bioinformatic analysis, along with a deep review of the scientific data, which ultimately might highlight the importance of the microbiota in the regulation of genomic (DNA mutations), transcriptomic (RNA expression), epigenomic, (DNA methylation, non-coding RNAs, histone, and protein modifications), proteomic, and metabolomic process stability through the establishment of a specific TRN in cancer-related cells, which in turn may control the interaction with the microbiome network to unlock phenotypic plasticity and acquire the hallmarks of cancer (Figure 1). Therefore, the highlighted TFs in a cancer TRN that could communicate with the microbiome network through specific membrane receptors may become interesting candidates that could be used as multiomic biomarkers for the development of specific diagnostic tools and treatments against cancer.
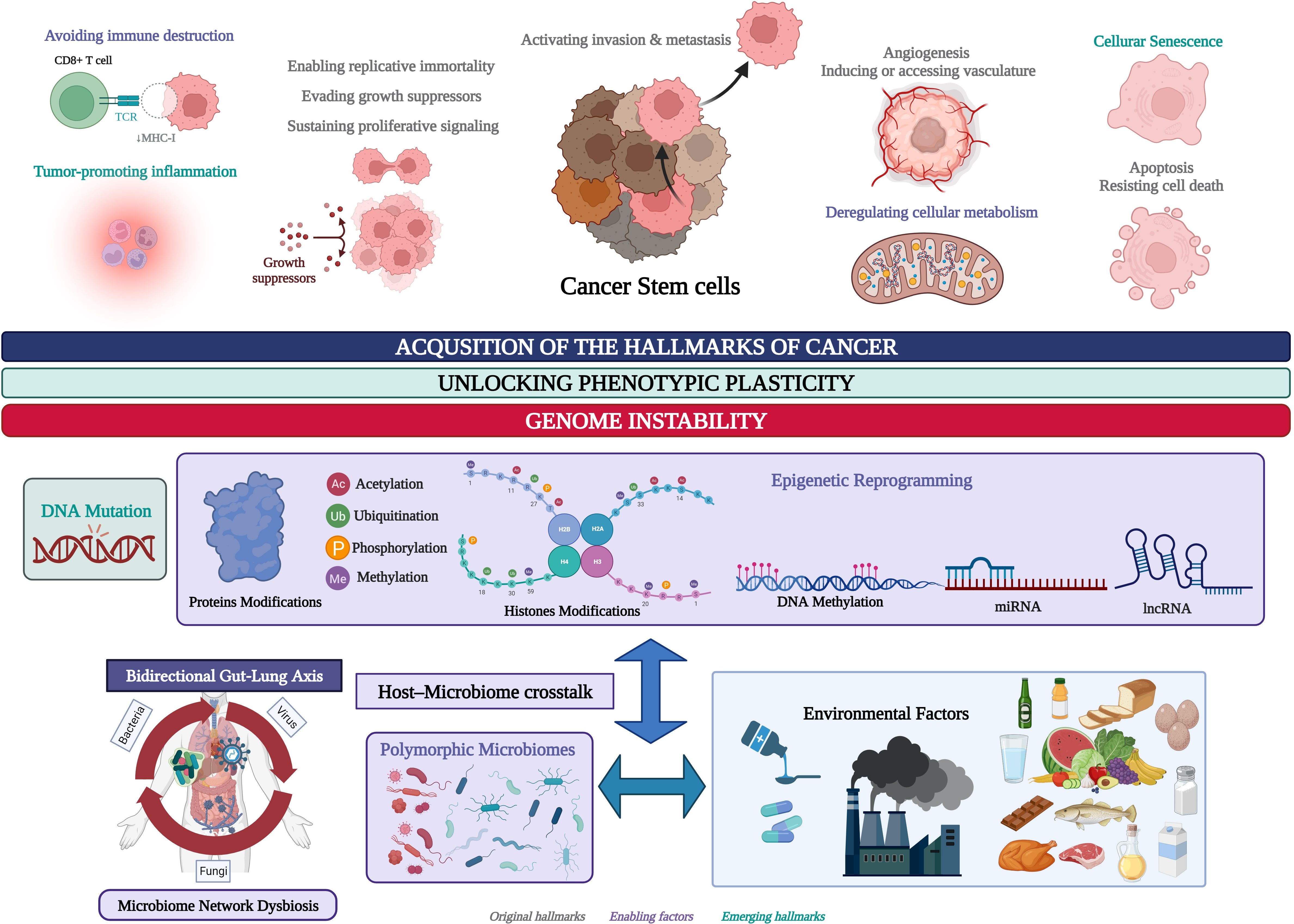
Figure 1. Environmental factors and the genomic and epigenomic regulatory processes involved in the unlocking of the phenotypic plasticity in cancer stem cells (CSC) and the acquisition of the hallmarks of cancer. Created with BioRender.com.
2 Materials and methods
2.1 Data selection, quality control, and construction of gene expression matrices
An advanced search was conducted on the National Center for Biotechnology Information (NCBI) GEO database (http://www.ncbi.nlm.nih.gov/geo/) to identify studies analyzing global gene expression in lung cancer, colon cancer, and gastric cancer. The search tool was used with the keywords “lung cancer,” “colon cancer,” and “gastric cancer”. Studies were limited to Homo sapiens as the organism, with expression profiling by array or RNA sequencing as the dataset type. The inclusion criteria encompassed studies that: (1) utilized any version of microarrays or any chemical of next generation sequencing; (2) analyzed at least three samples of each cancer type and at least three normal tissue samples for comparison; (3) provided raw data availability; and (4) passed quality control measures. All datasets underwent a quality control (QC) process to ensure their suitability for analysis. The initial step involved assessing signal comparability across different samples in each dataset to verify technical consistency. We then conducted a thorough examination of transcriptome correlations between samples to identify any significant deviations that might indicate poor quality samples. Principal component analysis (PCA) was employed as an additional tool for detecting low-quality samples. PCA helps visualize the variance within the dataset and allows for the identification of samples that significantly deviate from the main group. Samples exhibiting extreme variance or those that were outliers in the PCA plot were flagged as low quality. These low-quality samples, along with any datasets that did not meet our quality standards, were subsequently excluded from further analysis. Additionally, after processing and cleaning the datasets, we conducted an over-representation pathway analysis using the DAVID (Database for Annotation, Visualization, and Integrated Discovery) tool. This analysis was performed on the lists of DEGs obtained from each dataset individually. The aim was to confirm that the genes identified as differentially expressed were associated with known biological pathways relevant to cancer. This additional validation provided an extra layer of confidence in our results, ensuring that the selected genes were not only significantly different in expression but also implicated in critical biological processes related to the disease.
Of the total number of datasets analyzed, ten lung cancer datasets, five colon cancer datasets, and ten gastric cancer datasets (Table 1), passed the quality control phase and were used to obtain a gene expression matrix with Limma library in R (Ritchie et al., 2015), or DESeq2 (Love et al., 2014). For Affymetrix data, processing was conducted using the Limma R/Bioconductor software package (Ritchie et al., 2015). Data normalization and log2 transformation were carried out using the Robust Multi-array Average (RMA) algorithm. Owing to the presence of multiple probes for the same gene on Affymetrix chips, only the most informative probe, demonstrating the highest variability across experimental groups, was retained, whereas redundant probes were discarded. As noted in Table 1, some datasets have differences in the number of cases and controls. We have this in account by using Limma. Limma’s strength lies in its ability to fit linear models and adjust for varying group sizes. It employs an empirical Bayes approach that stabilizes the variance estimates by shrinking them toward a common value. This method enhances the accuracy of variance estimates, allowing Limma to efficiently handle datasets with unequal sample sizes. Consequently, Limma provides reliable and precise results even when there are significant differences in the sizes of the experimental groups. For RNA-Seq data analysis, preprocessing began directly with the gene count table available in GEO. Normalization of these count tables was performed using the method implemented in DESeq2 (Love et al., 2014) to address differences in library size and composition across samples. Subsequently, differential expression analysis was carried out using DESeq2 to identify genes exhibiting significant expression changes between experimental conditions. For both Affymetrix and RNA-Seq data, a gene was considered differentially expressed if the fold change in expression exceeded 1.5 or was less than −1.5, and the adjusted p-value was less than 0.05.
2.2 Gene functional annotation and gene networks analysis
The DEG lists of every dataset in every type of cancer were compared to identify the common overregulated and downregulated genes, highlighting membrane receptors, and TFs in at least seven datasets of lung and gastric cancer, and at least three datasets of colon cancer (Supplementary Tables 1-3). DAVID´s annotation tool was used to identify the related biological functions and signaling pathways associated with the common overregulated DEGs and deregulated TFs in every type of cancer (Sherman et al., 2022). The TRNs were constructed with the expression matrix of common TFs in every type of cancer in the Reconstruction of Transcriptional regulatory Networks and analysis of regulons (RTN) library (Groenevelt et al., 2024). The GRNs and CRNs were constructed with the expression matrix of common overexpressed DEGs, and deregulated TFs in every type of cancer with the CoRegNet library (Nicolle et al., 2015). The regulatory interactions between common deregulated TFs were analyzed based on TRNs and CRNs, and the regulators (TFs) of key receptors were identified through the analysis of the GRNs. All libraries were used under our own bioinformatic pipeline (Supplementary Methods 2).
2.3 Global transcriptomic network analysis of gut and lung cancer
Common DEGs of membrane receptors, TFs, and DEGs involved in signaling pathways associated with the interaction of cancer-related cells and microorganisms were highlighted by DAVID´s annotation analysis of all common DEGs in each type of cancer. The Results section focuses on the main TFs of every cancer type according to the TRN analysis and features their regulatory function according to the biological processes and signaling pathways that might control them during the tumorigenic process. The Discussion section considers the experimental evidence related to the TFs and signaling pathways involved in the host cell and microbiota interactions directly or through microbiota expressed proteins, with specific membrane receptors, identified as common DEGs in every cancer type. BioRender was used to show, in one image, all the knowledge generated by scientific research in the field of host-microbiome crosstalk, through cancer transcriptomic studies. Lung, colon, and gastric cancer TRNs represent intricate gene regulatory programs involved in tumorigenesis, whereas the co-regulatory analysis of TFs assesses the formation of key protein-protein interaction complexes that might be important for unlocking the phenotypic plasticity of cancer-related cells through the control of key regulatory programs in a specific spatial, temporal, and sequential manner, probably according to their communication with the characteristic microbiome network. Consequently, a global transcriptomic analysis was carried out based on a bioinformatic analysis to organize and present all the scientific evidence present in several publications in the field of microbiome and cancer, in an image. This image features the best known microorganisms and their proteins that interact with key membrane receptors deregulated in every cancer type, the actual evidence of the signaling pathways that they might activate, and the following control of gene expression programs through the activation of cancer TRNs that might be related to the unlocking of phenotypic plasticity and acquisition of the hallmarks of cancer, as well as the control of the gene expression of key TFs over the gene expression of the main membrane receptors that interact with the microbiome network, representing the impact of the TRN in the communication with the microbiome network during the tumorigenic process. In the Conclusion, we summarize for every cancer type, the key TFs and their regulatory interactions, the membrane receptors and the best-known microorganisms that interact with them, and the signaling pathways, with experimental evidence that might be used to establish the current state of microbiome and cancer research, which can be used as the guiding core and starting point for future multiomic studies in this field.
3 Results
3.1 Differentially expressed genes and transcription factors in tumorigenic processes of the gut-lung axis
Ten gene expression datasets of lung cancer were analyzed; 417 common overregulated genes and 438 downregulated genes were identified in at least seven of the datasets (Supplementary Table 1) (Otálora-Otálora et al., 2023a). Five gene expression datasets of colon cancer were analyzed; 474 common overregulated genes and 640 downregulated genes were identified in at least three of the datasets (Supplementary Table 2). Ten gene expression datasets of gastric cancer were analyzed; 195 common overregulated genes and 309 downregulated were identified in at least seven of the datasets (Supplementary Table 3). Eighteen overregulated and 16 downregulated TFs were identified in the lung cancer common genes list, 37 overregulated and seven downregulated TFs were identified in the colon cancer common DEGs list, and 10 overregulated and 12 downregulated TFs were identified in the gastric cancer common DEGs list. There were seven overregulated and four downregulated TFs in common between lung and colon cancer, three overregulated and three downregulated TFs in common between lung and gastric cancer, five overregulated and three downregulated TFs in common between colon and gastric cancer, and two overregulated and two downregulated TFs in common between the three types of cancer (Figure 2).
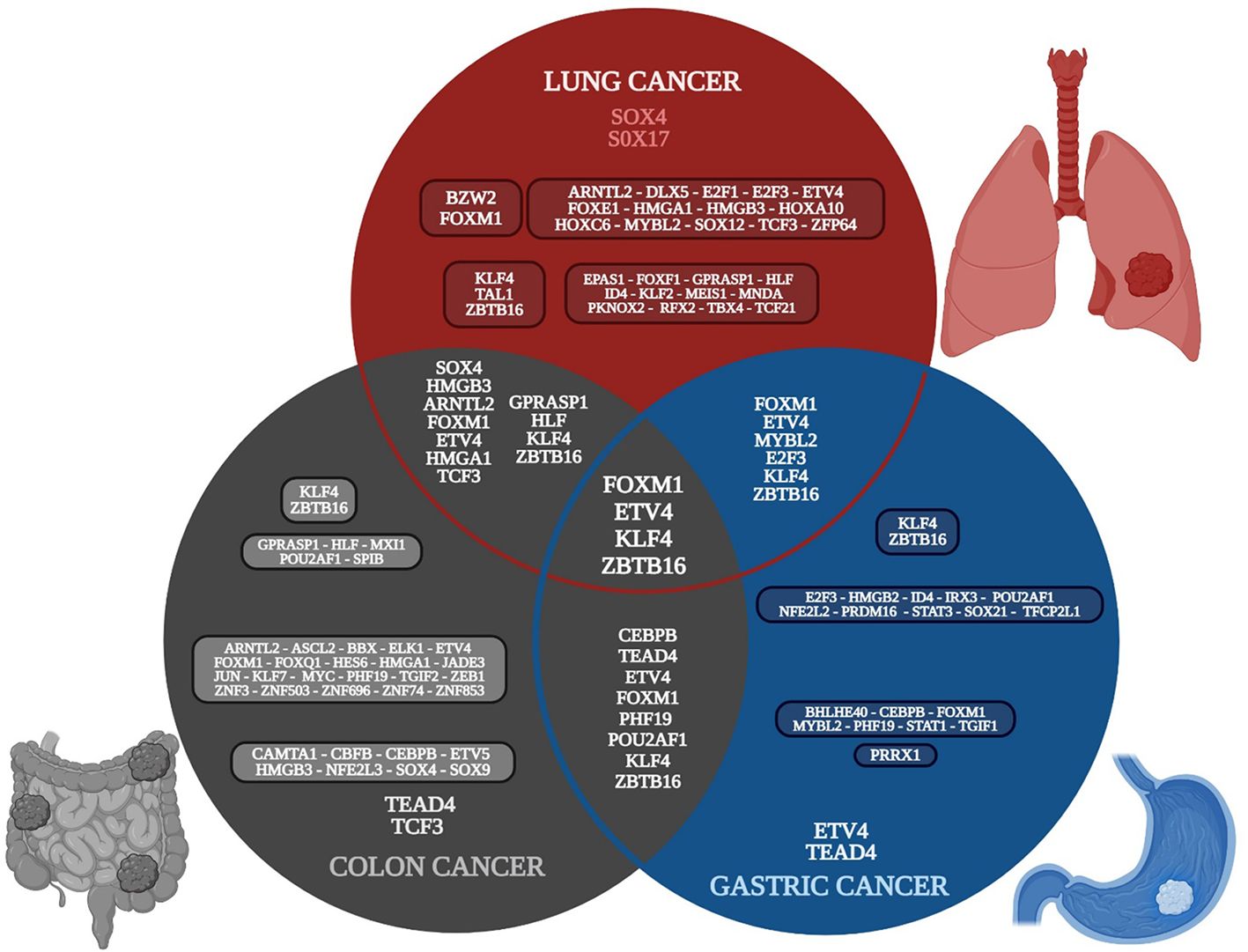
Figure 2. Venn diagram of the transcriptomic metafirm of gut-lung axis tumorigenic processes, with the common and unique deregulated transcription factors of each type of cancer. Created with BioRender.com.
Lung cancer common overregulated DEGs are related to epigenetic reprogramming (phosphorylation, acetylation, methylation, hydroxylation, and ubiquitin-like conjugations [Ubls]), the formation of extracellular exosomes, cell division, apoptosis, adhesion, proliferation, and junctions, the regulation of transcription, the epigenetic regulation of gene expression, DNA damage, repair, recombination, and replication, the formation of TF complexes, the regulation of stem cell population maintenance, and signaling pathways involved in DNA replication, cellular senescence, base excision repair, viral carcinogenesis, the biosynthesis of co-factors, mismatch repair, the cell cycle, purines, and metabolism, Wnt, hepatitis B (HBV), Epstein–Barr (EBV), human papillomavirus (HPV), and human T-cell leukemia virus 1 infection (HTLV-I) (Supplementary Table 1). Colon cancer common overregulated DEGs are related to epigenetics reprogramming (phosphorylation, acetylation, methylation, and ubl conjugation), the formation of extracellular exosomes, cell adhesion, proliferation, apoptosis, junctions, migration, and division, the regulation of transcription, DNA damage, repair, and replication, the formation of TF complexes, and stem cell proliferation, as well as signaling involved in the cell cycle, proteoglycans in cancer, cellular senescence, amoebiasis, malaria, and hepatitis C virus (HCV), EBV, HPV, and HTLV-I infection (Supplementary Table 2). Gastric cancer overregulated common DEGs are related to epigenetic reprogramming (hydroxylation, phosphorylation, and ubl conjugation), the formation of extracellular exosomes, cell adhesion, proliferation, migration, division, apoptosis, junctions, and growth, DNA replication, angiogenesis, the regulation of telomerase activity, the regulation of the epithelial to mesenchymal transition (EMT), inflammatory response, angiogenesis, gene expression regulation, cellular response to TNF, innate immunity, and host-virus interaction, as well as signaling involved in malaria, amoebiasis, pertussis, Kaposi sarcoma-associated herpesvirus, and HPV (Supplementary Table 3).
3.2 Transcriptional and gene regulatory network analysis
3.2.1 Lung cancer
SOX4 is overregulated in all ten lung cancer datasets and is related to: 1) apoptosis along with E2F1; 2) cell proliferation along with FOXM1, DLX5, and E2F3; 3) the formation of TF complexes along with E2F3, ARNTL2, HMGA1, HOXA10, and TCF3; 4) the positive regulation of transcription along with E2F1, FOXM1, ETV4, DLX5, FOXE1, HMGA1, and TCF3; and 5) the positive regulation of the Wnt signaling pathway along with DLX5 (Supplementary Table 1). FOXM1 is overregulated in nine lung cancer datasets and is related to: 1) the negative regulation of transcription along with E2F1, FOXE1, and HMGA1; 2) cellular senescence along with E2F1, E2F3, and MYBL2; and 3) DNA repair and damage. E2F1 is overregulated in seven lung cancer datasets and is related to: 1) HPV infection; 2) HBV and EBV virus infection along with E2F3; 3) the cell cycle and pathways in cancer along with E2F3; and 4) HTVL-I infection along with E2F3 and TCF3. According to the TRN of lung cancer (Figure 3), SOX4, FOXM1, ETV4, HOXC6, and E2F3 are the main positive regulators, whereas SOX17, KLF4, and ZBTB16 are the main negative regulators of transcription, controlling the expression of other TFs and target genes in lung cancer. Additionally, the TFs are capable of forming co-regulatory complexes in NSCLC (DLX5, BZW2, E2F3, FOXM1, HMGA1, HMGB3, HOXC6, MYBL2, and SOX4) and SCLC (DLX5, E2F1, E2F3, FOXM1, HOXC6, SOX4, and TCF3). According to DAVID analysis, SOX4 is regulated by acetylation, whereas DLX5 is regulated by phosphorylation; both positively regulate transcription and the canonical Wnt signaling pathway (Supplementary Table 1). SOX4 has 140 target genes in the GRN, which are in the nucleus, cytoplasm, cytoskeleton, and membrane, and are related to epigenetic reprogramming (phosphorylation and acetylation), host-virus interactions, five membrane receptors (HMMR, LRP8, PTPRF, SRPRB, and TNFRSF21), and signaling pathways related to HTLV-I and HBV infection. According to the TRN analysis, SOX4 can be regulated by HOXC6, whereas DLX5 can be regulated by SOX4, FOXM1, and ETV4 (Figure 3), and according to the co-regulatory network analysis, they both participate in the formation of TF complexes in both subtypes of lung cancer (NSCLC and SCLC) (Supplementary Table 1).
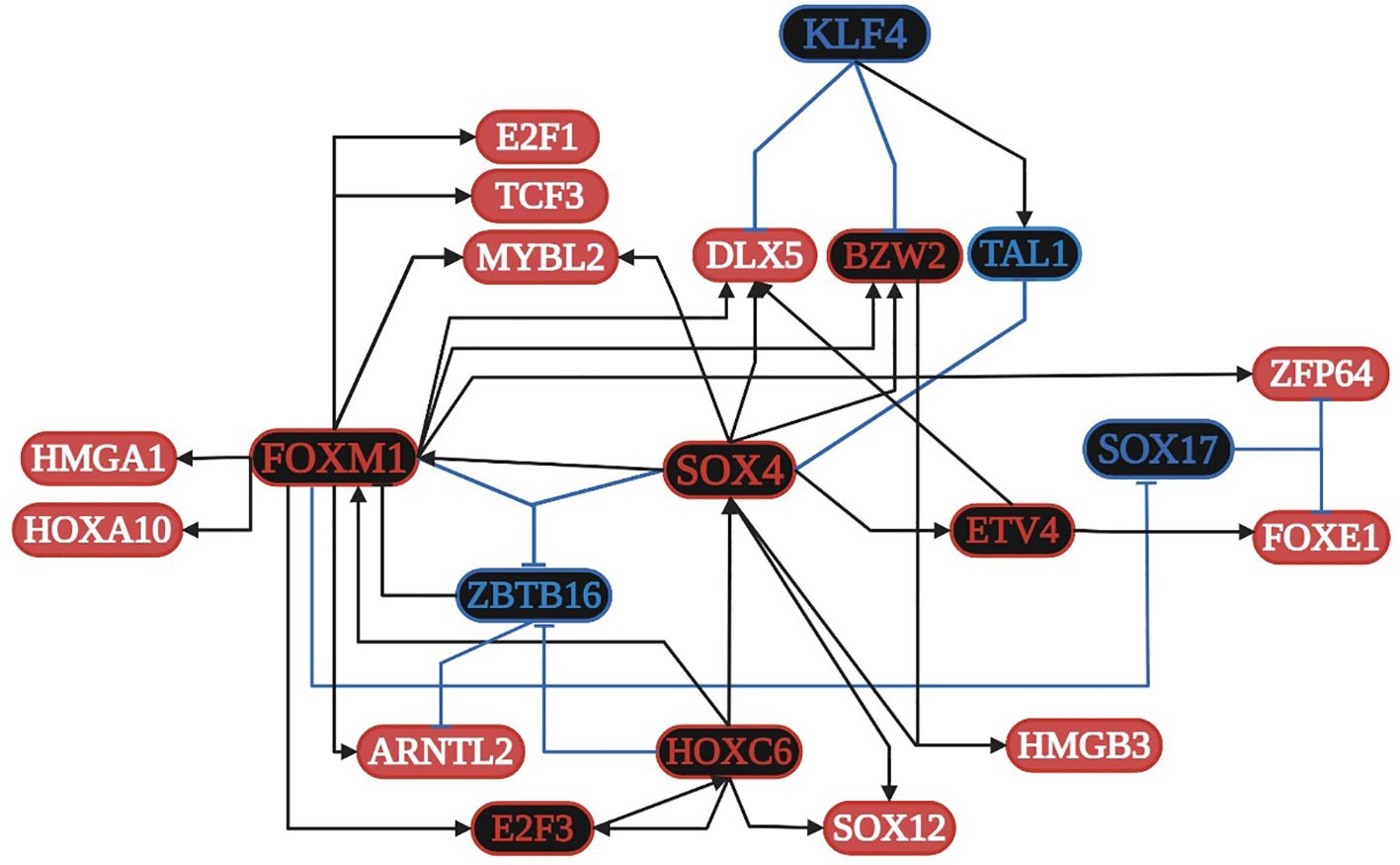
Figure 3. Transcriptional regulatory network (TRN) of common transcription factors (TFs) in lung cancer. Downregulated TFs are in blue, upregulated TFs are in red, and the key overregulated and downregulated TFs are in black. The black pointed arrows represent overregulation and the blue flat arrows represent downregulation. Created with BioRender.com.
3.2.2 Colon cancer
TCF3 is overregulated in four of the five datasets and is related to: 1) the positive regulation of transcription along with SOX4, SOX9, CEBPB, FOXM1, ETV4, JUN, ELK1, ARNTL2, and HMGA1; 2) the formation of a transcription regulatory complex along with TEAD4, JUN, SOX4, SOX9, ARNTL2, HES6, and HMGA1; 3) the positive regulation of transcription from the RNA polymerase II promoter along with TEAD4, ELK1, ETV4, ARNTL2, CAMTA1, HMGA1, CEBPB, ETV5, JUN, KLF7, MYC, SOX4, SOX9, ASCL2, CBFB, FOXM1, and ZEB1; 4) the negative regulation of transcription from the RNA polymerase II promoter along with CEBPB, ETV5, JUN, KLF7, MYC, NFE2L3, PHF19, SOX4, SOX9, ASCL2, CBFB, FOXM1, FOXQ1, HES6, TCFL5, ZEB1, and ZNF3; and 5) HTLV-I infection along with ELK1, JUN, and MYC. SOX4 is overregulated in four of the five datasets and is related to: 1) the positive regulation of proliferation along with SOX9, FOXM1, and MYC; 2) the regulation of apoptosis along with JUN, SOX9, and MYC; 3) the regulation of translation along with MYC; and 4) the regulation of the Wnt signaling pathway along with SOX9. JUN is overregulated in three of the five datasets and is related to: 1) colon cancer signaling pathways along with MYC; 2) the focal adhesion pathway along with ELK1; 3) pathways in cancer along with ELK1 and MYC; 4) EBV infection along with MYC; and 5) angiogenesis. MYC is overregulated in three of the five datasets and is related to: 1) cellular senescence along with FOXM1; 2) proteoglycans in cancer along with ELK1; and 3) HCV infection (Supplementary Table 2). According to the TRN analysis (Figure 4), TCF3 is related to the upregulation of TEAD4, SOX4, ETV5, CEBPB, CBFB, HMGB3, and NFE2L3; TEAD4 is related to the upregulation of TCF3, ETV5, FOXM1, CAMTA1, CEBPB, CBFB, and NFE2L3; SOX4 is related to the overregulation of TCF3, SOX9, CEBPB, NFE2L3, HMGB3, JUN, and HES6; and FOXM1 is related to the overregulation of TCF3, TEAD4, SOX4, ETV4, MYC, ELK1, ARNTL2, HMGA1, and PHF19. The co-regulatory analysis suggests the formation of protein integration complexes between overregulated TFs (CAMTA1, CBFB, CEBPB, ETV4, FOXM1, HES6, JUN, MYC, NFE2L3, PHF19, SOX4, SOX9, and TEAD4) controls transcriptional regulatory function during the acquisition of the hallmarks of cancer.
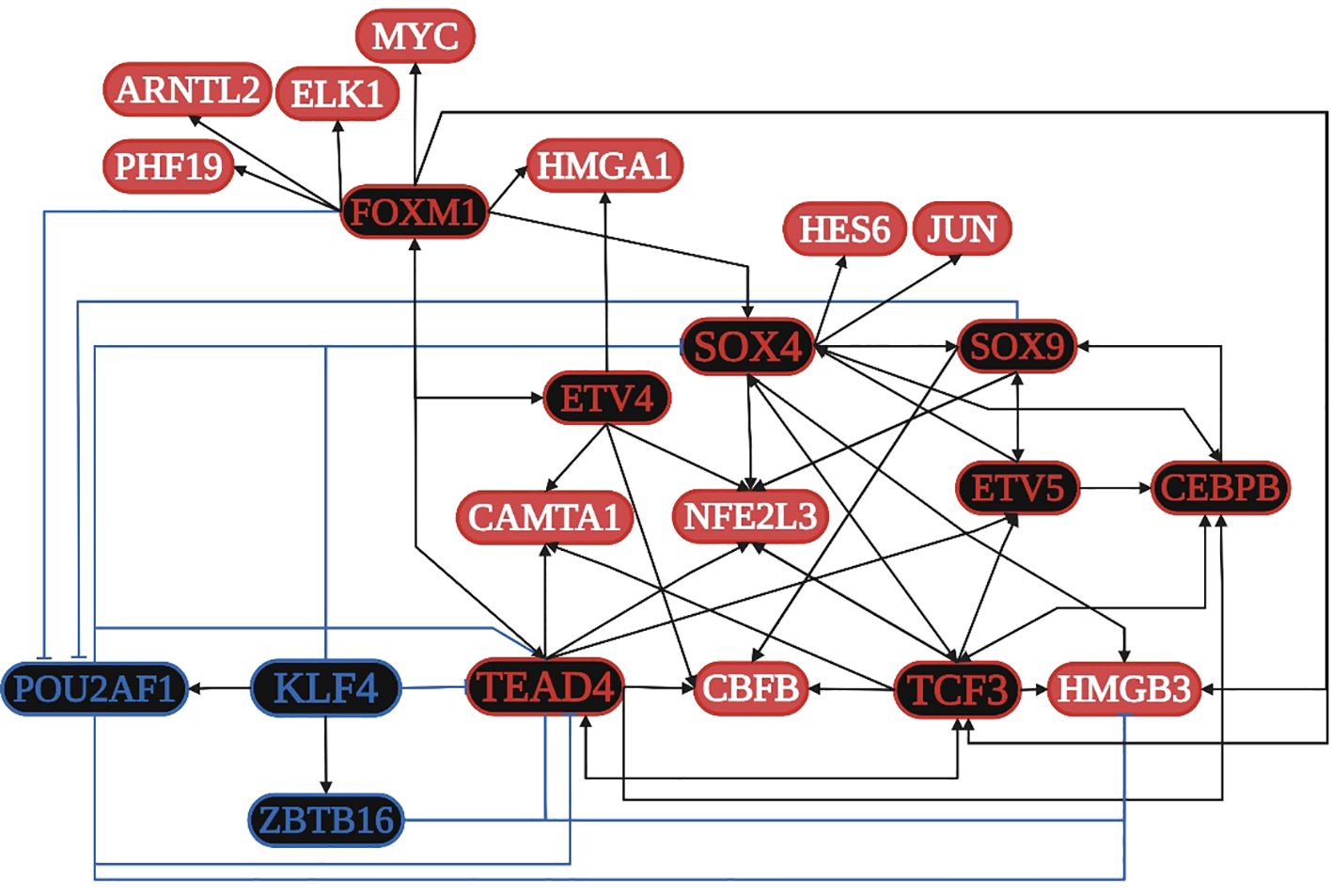
Figure 4. Transcriptional regulatory network (TRN) of common transcription factors (TFs) in colon cancer. Downregulated TFs are in blue, upregulated TFs are in red, and the key overregulated and downregulated TFs are in black. The black pointed arrows represent overregulation, and the blue flat arrows represent downregulation. Created with BioRender.com.
3.2.3 Gastric cancer
STAT1 is overregulated in seven datasets and is related to: 1) angiogenesis; 2) the formation of the macromolecular complex; 3) type I interferon, cytokine-mediated signaling, and pathways in cancer; and 4) Kaposi sarcoma-associated herpes (KSH) and HPV infection. CEBPB is overregulated in seven datasets and is related to 1) the regulation of the inflammatory response, 2) the cellular response to amino acid stimulus, and 3) the TNF and IL-17 signaling pathways. TGIF1 is overregulated in seven datasets and is related to 1) the cellular response to growth factor stimulus and 2) the TGF-beta signaling pathway. FOXM1 is overregulated in seven datasets and is related to 1) the regulation of cell proliferation and 2) the cellular senescence along with MYBL2. TEAD4, ETV4, and PRRX1 are overexpressed in nine and seven of the ten datasets analyzed, but they are not in DAVID´s annotation analysis (Supplementary Table 3). According to the TRN analysis (Figure 5), TEAD4 and ETV4 are related to the overregulation of STAT1, MYBL2, CEBPB, PHF19, BHLHE40, TGIF1, and FOXM1. PRRX1 is related to the overregulation of STAT1 and CEBPB. FOXM1 is related to the overregulation of TEAD4 and MYBL2. STAT1 is related to the overregulation of PRRX1 and CEBPB. MYBL2 is related to the overregulation of ETV4 and FOXM1. CEBPB is related to the overregulation of STAT1 and BHLHE40. TEAD4 is also related to the downregulation of KLF4, whereas FOXM1 and MYBL2 are related to the downregulation of ZBTB16. The co-regulatory analysis suggests that the formation of protein-protein interaction complexes between eight overregulated TFs (BHLHE40, ETV4, FOXM1, MYBL2, PHF19, PRRX1, STAT1, and TEAD4) controls transcriptional regulatory function.
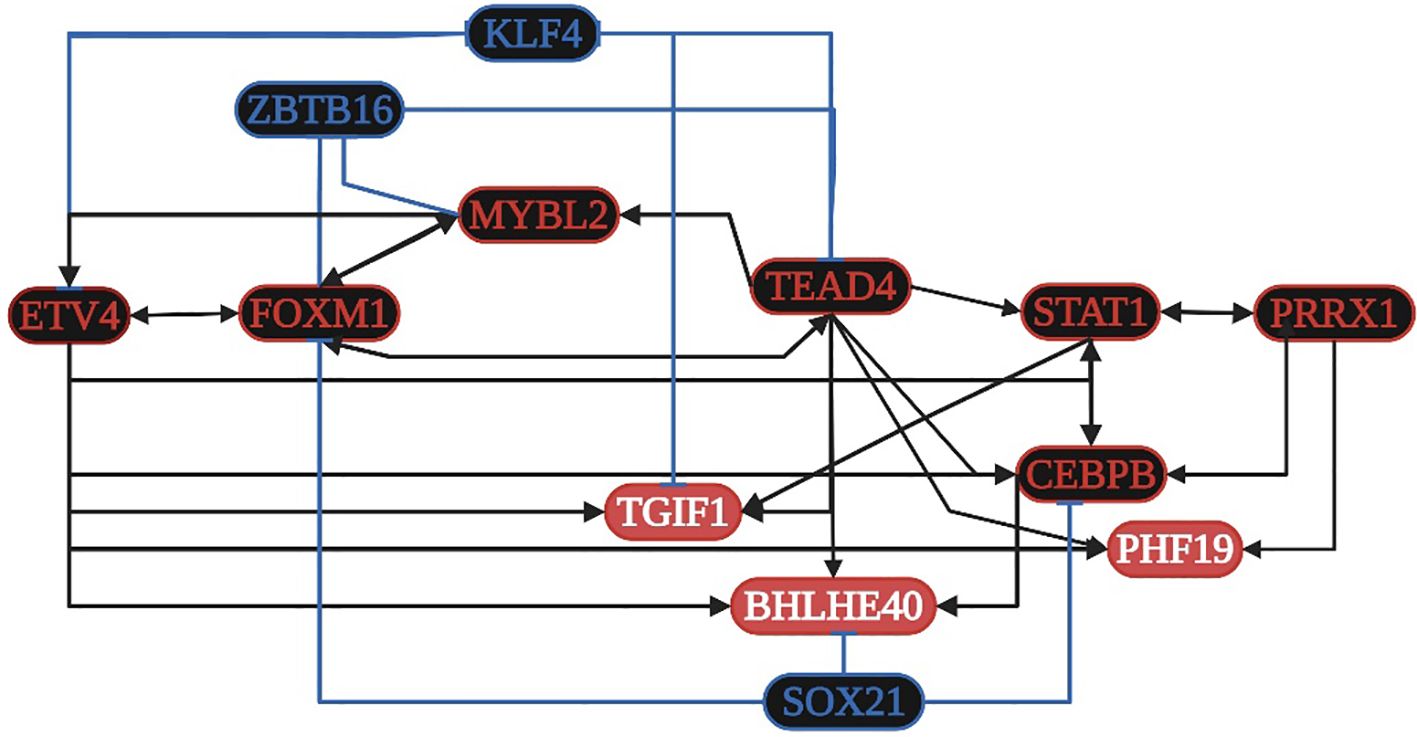
Figure 5. Transcriptional regulatory network (TRN) of common transcription factors (TFs) in gastric cancer. Downregulated TFs are in blue, upregulated TFs are in red, and the key overregulated and downregulated TFs are in black. The black pointed arrows represent overregulation, and the blue flat arrows represent downregulation. Created with BioRender.com.
4 Discussion
Bioinformatic analysis of high-throughput sequencing methodologies, such as microarrays and RNA sequencing, allows the identification of all the transcriptionally deregulated genes (DEGs) involved in the modulation of biological processes and signaling pathways related to tumor cells grade, differentiation status, metastatic potential, and patients’ survival (Otálora-Otálora et al., 2019, Otálora-Otálora et al., 2023a). The human genome has information for approximately 1,400 regulatory genes known as TFs, representing approximately 6% of all human protein coding genes. DNA-binding TFs recognize cis-regulatory elements of target genes, which make them the most direct regulators of gene transcription during cellular differentiation, development, and the response to external factors through the activation and/or inhibition of specific signaling pathways (Weidemüller et al., 2021). In normal cells and tumor cells, TF coding genes can be regulated positively or negatively by genetic and epigenetic mechanisms (Figure 1) that control protein localization on the binding site, resulting in a loss or gain of function (Whiteside and Goodbourn, 1993; Filtz et al., 2014).
The global transcriptomic network analysis highlighted the impact of five TFs, SOX4, TCF3, TEAD4, ETV4, and FOXM1, in gut and lung cancer (Figure 2). SOX4 is an important developmental TF known to regulate stemness, differentiation, progenitor development, and signaling pathways, including the TGF-β, p53, PI3K-Akt, and Wnt pathways, which strengthen its expression (Moreno, 2020). SOX4 shows increased expression in NSCLC tissues, which is specifically correlated with differentiated degree status, the clinical stage, T classification, N classification, M classification, and poor overall patient survival (Wang et al., 2015). SOX4 is a known target gene of the TGF-β signaling pathway, via the direct binding of SMAD2/3 in complex with SMAD4 to the SOX4 gene promoter region, and has been shown to be related to the regulation of neural-related nature target genes in SCLC lung tumors with neuroendocrine characteristics (Castillo et al., 2012). In lung cancer, BMP5 is a common gene but it is downregulated, as are TGF-β receptors and SMADs; therefore, none of them may be involved in the regulation of SOX4 and DLX5 expression. However, according to the TRN analysis, SOX4 can be regulated by HOXC6, whereas DLX5 can be regulated by SOX4, FOXM1, and ETV4 (Figure 3); therefore, its regulation might be controlled by the pathways related to these TFs. In colon cancer, BMP7 is common overregulated gene, as are TGFB2, TGFBI, and TGIF2, which might be involved in the regulation of SOX4 (Supplementary Table 2). Junction plakoglobin (JUP), also known as γ-catenin, a major component of the submembrane of adherens junctions and desmosomes in mammalian cells, can interact with cadherin 3 in adherens junctions in the cytoplasmic component; both are common overregulated genes in lung cancer (Supplementary Table 1) and bind to SOX4 via two trypsinized fragments (Figure 6), while Wnt signaling induces the nuclear colocalization of SOX4 and JUP (Lai et al., 2011). In lung cancer, SOX4 expression is probably related to the overregulation of WNT5A; then, SOX4, FOXM1, TCF3, and JUP might form a stable protein complex with other factors and co-factors for the regulation of transcriptional targets (Figure 6). SOX4 overexpression promotes sphere formation and the self-renewal of colorectal cancer cells (Figure 2), which directly bind to the HDAC1 promoter, encouraging HDAC1 transcription and thereby stem cell maintenance, Wnt, Notch, the cell cycle, and transcriptional misregulation pathways in cancer (Liu et al., 2021b). Forkhead box protein M1 (FOXM1) is overexpressed in NSCLC and SCLC, is related to the regulation tumorigenesis, cell cycle progression,cancer therapy resistance, and metastasis, as it can translocate to the nucleus and bind to the regulatory regions of several target genes crucial for the survival of cancer cells (Zhang et al., 2015; Liang et al., 2021). FOXM1 is a key cell cycle regulator that plays a key role in embryogenesis and cell proliferation and has been strongly linked to solid tumors like colon cancer, where it is linked to reduced disease-free survival, which suggests it is an important prognostic marker (Rather et al., 2023). Furthermore, in gastric cancer, FOXM1 is related to proliferation and invasion, and when it is co-expressed with hTERT it might be involved in cell-cycle-related pathways and positively related to advanced stages and poor outcomes (Tang et al., 2023). In the transcriptomic analysis, there were three other common DEGs (NEK2, GREM1, and HSP90AB1) related to polymerase activity (Supplementary Table 3) that might be related to the process.
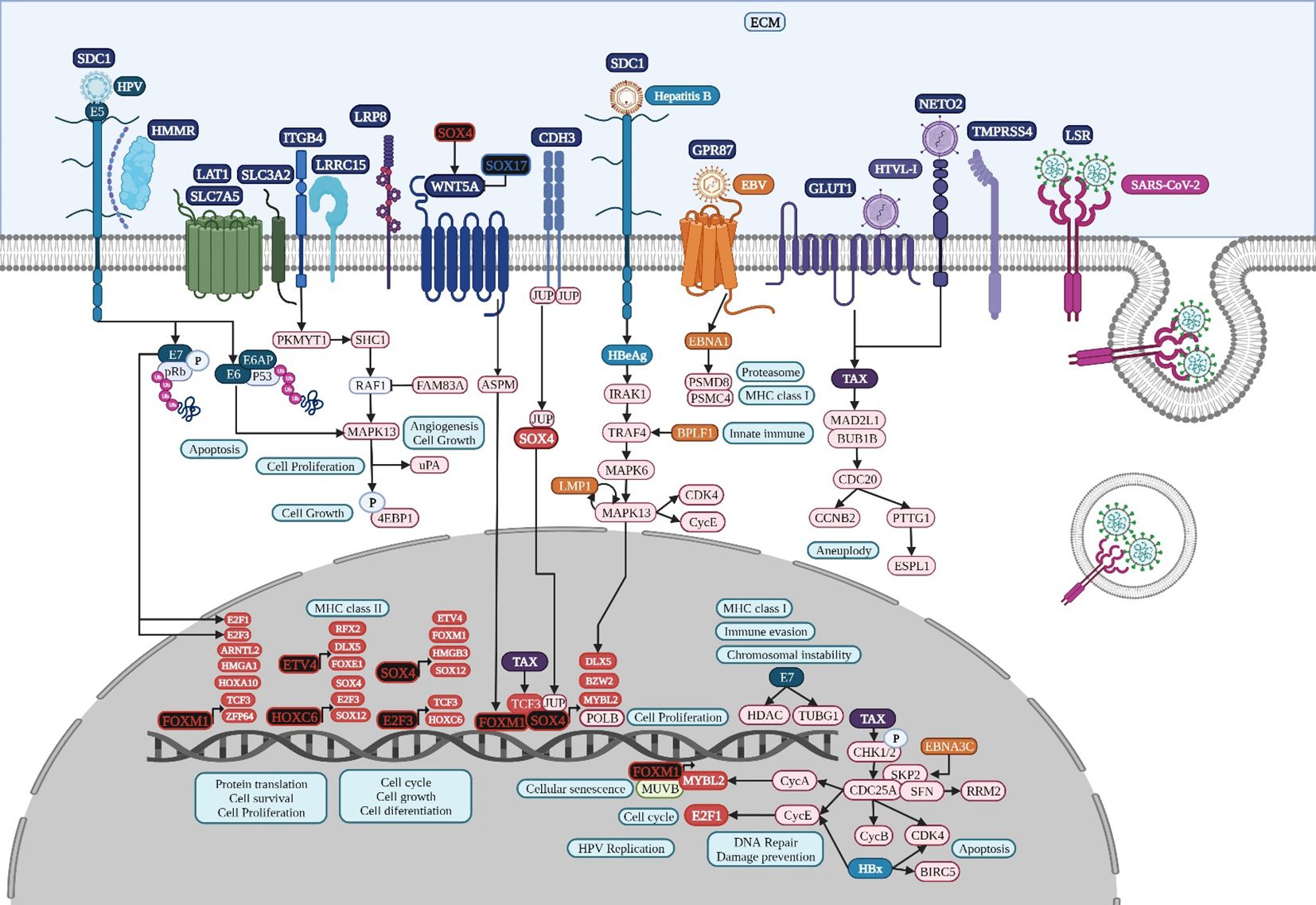
Figure 6. Microbiome interaction with the membrane receptors of lung cancer-related cells, activating signaling pathways involved in transcriptional regulation during lung tumorigenesis. Upregulated genes and TFs are in red and the key upregulated TFs are in black. Created with BioRender.com.
The transcription factor 3 gene (TCF3) is a key TF overexpressed in lung cancer (Figure 2) and is mainly involved in the cell cycle, cell division, and proliferation (Zhang et al., 2018). TCF3 is primarily a transcriptional repressor (Gregorieff and Clevers, 2005) and is not expressed in adult intestine (Wong et al., 2002). Non-canonical WNT signaling could be related to TCF3 upregulation in colon cancer (Figure 4), along with other key overregulated TFs, and the formation of transcriptional regulatory complexes according to DAVID´s analysis (Supplementary Table 2); therefore, it might be involved in the control of gene expression for the acquisition of the hallmarks of cancer (Figure 1). TCF3 (E2A immunoglobulin enhancer-binding factors E12/E47) E12 forms heterodimers with other basic helix-loop-helix proteins during cell differentiation, whereas E47 can homo and heterodimerize, promoting tumor angiogenesis and proliferation (Peinado et al., 2004); both act as transcriptional repressors of E-cadherin during EMT, which is linked to tumor aggressiveness (Perez-Moreno et al., 2001). TCF3 upregulation is caused by promoter hypomethylation during development, colon cancer progression (Li et al., 2014), and the transcriptional upregulation of multiple cyclin-dependent kinase inhibitors like CDKN1A, p15INK4B, and p16INK4B (Pagliuca et al., 2000), and probably CDKN3 in colon cancer (Supplementary Table 2). According to the RTN analysis, the overregulation of TCF3 might be controlled by TEAD4, SOX4, CEBPB, and FOXM1 (Figure 4).
TEAD4 belongs to a transcriptional enhancer activator domain family of TFs, has a profound impact on physiological and pathological processes through gene expression regulation during cell survival, cell proliferation, tissue regeneration, and stem cell maintenance (Gu et al., 2020), controls chemoresistance, promotes EMT, ECM remodeling, the secretion of paracrine factors, and heterotypic cellular communication, and is related to several signaling pathways (Liu et al., 2024). According to the RTN analysis, the overregulation of TEAD4 might be controlled by TCF3 and FOXM1 (Figure 4). In colon cancer, TEAD4 may also form a complex with TCF3 under the regulation of Wnt (Jiao et al., 2017). The non-canonical Wnt signaling pathway might be involved in colon cancer progression (Figure 7), specifically in planar cell polarity (PCP), in which WNT2 binds to FZD3, a frizzled transmembrane receptor, to activate ankyrin repeat domain 16 (ANKRD16), ras homolog gene family members B and Q (RHOB and RHOQ), and a mitogen-activated protein kinase, MAP3K20, modifying JUN phosphorylation and its transcriptional regulatory function, which affects cell polarity and cytoskeleton organization (Gómez-Orte et al., 2013). In colon cancer, WNT2 might bind to FZD3 to upregulate AXIN2, as well as RNF43 for its negative feedback regulation (Jho et al., 2002), in which the modification and degradation of β-catenin are key pathway events in tumor progression (Zhao et al., 2022).
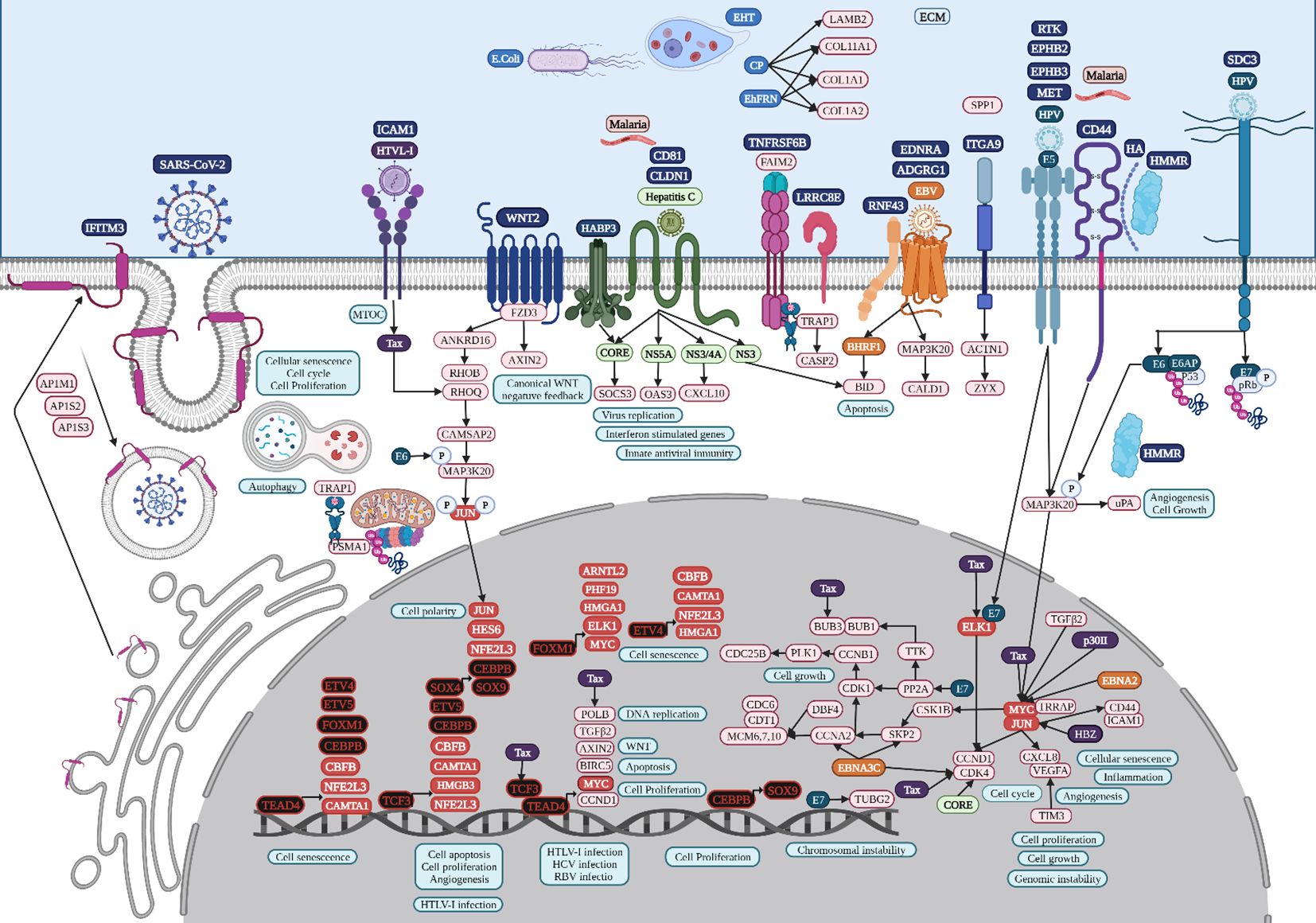
Figure 7. Microbiome interaction with the membrane receptors of colon cancer-related cells, activating signaling pathways involved in transcriptional regulation during colon tumorigenesis. Upregulated genes and TFs are in red and the key upregulated TFs are in black. Created with BioRender.com.
TEAD4 stimulates the glycolysis and proliferation of gastric cancer cells (Zhan et al., 2023). The TNF-α-ERK-VGLL1-TEAD4 pathway upregulates integrin αV expression, increasing the adhesion and invasive ability of gastric cancer cells (Hwang et al., 2022). ETV4 is a TF of the E26 transformation‐specific (ETS) family and plays an important role in tissue development, promoting the growth and metastasis of gastric cancer (Lu et al., 2019). ETV4 is also significantly related to growth at the advanced stage, lymph node metastasis, and the poor prognosis of NSCLCs, with a direct regulatory effect on matrix metalloproteinase 1 (MMP1), which is a common overregulated gene (Supplementary Table 1) related to cell proliferation and migration, and its co-overexpression is associated with a poor prognosis in human NSCLCs, suggesting that it could be a useful biomarker of tumor progression and worse outcomes in NSCLCs (Wang et al., 2020). Moreover, ETV4 is one of the most expressed genes in colon adenocarcinoma, and it is related to cell proliferation, colony formation, and cell migration (Fonseca et al., 2021). ETV4 is a transcription activator of TNF‐α, promoting hepatic inflammation in hepatocellular carcinoma (Qi et al., 2023). Several hallmark pathways are significantly enriched in patients with high PRRX1, including IFN-γ response, IL6-Jak-Stat3 signaling, TNF-α signaling via NF-kB, and IFN-α response in uveal melanoma cancer patients (Meng et al., 2022b). There is evidence that suggests an important role of TEAD4, ETV4, and PRRX1 regulatory activity, the main TFs identified in the TRN analysis (Figure 2), during the TNF and IFN singling pathway in gastric tumorigenesis (Figure 8), along with STAT1 and CEBPB, that might be validated experimentally, to establish their importance during the acquisition of the hallmarks of cancer. Signal transducer and activator of transcription 1 (STAT1) has been linked to anti-tumor immune responses, and H. pylori might be involved in the regulation of its expression (Li et al., 2022). In gastric cancer, elevated alpha-inducible protein 6 (IFI6) and FKBP prolyl isomerase 10 (FKBP10) (Supplementary Table 3) could be responsible for the activation of STAT1 expression, diminishing antiviral responses (James et al., 2020).
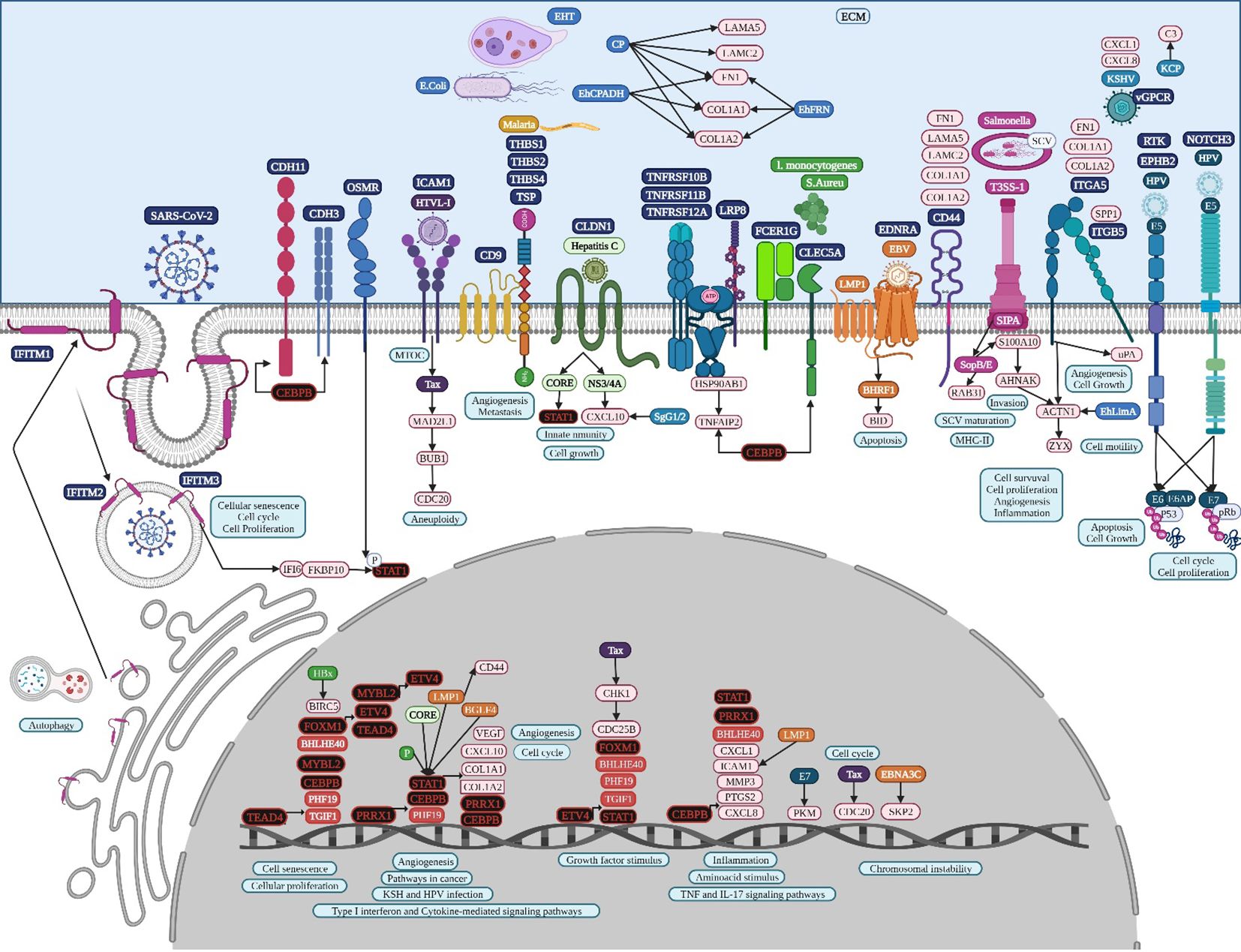
Figure 8. Microbiome interaction with the membrane receptors of gastric cancer-related cells, activating signaling pathways involved in transcriptional regulation during gastric tumorigenesis. Upregulated genes and TFs are in red and the key upregulated TFs are in black. Created with BioRender.com.
Transcriptomic studies of gut and lung cancer have been performed and analyzed by several scientific groups around the world. In colon cancer, there are transcriptomic analyses comparing tumor cases and healthy controls, identifying the differences in global transcriptional regulatory programs, showing a large reduction of transcriptional interactions in tumor networks, TFs, and target genes, while the average gene expression is conserved and some TFs increased their connectivity (Cordero et al., 2014). One study analyzed the signatures of co-deregulated genes and their transcriptional regulators in five datasets, highlighting 17 hub co-upregulated genes and 18 hub co-downregulated genes, including three well-known TFs and three kinases, as critical genes in colon cancer (Mastrogamvraki and Zaravinos, 2020). In gastric cancer, there are transcriptomic analyses comparing tumor cases and healthy controls, in which microarray datasets were analyzed to make a protein-protein interaction network with the retrieval of an interacting genes database and TCGA-STAD datasets, identifying the top TFs according to the calculation of the regulatory impact factor (Wang, 2017) (Zhao et al., 2021). An algorithm named mRBioM was even developed for the identification of potential mRNA biomarkers from the complete transcriptomic RNA profiles of gastric adenocarcinoma (Dong et al., 2021), of which some were TFs and cell receptors; however, it did not identify the main regulators of transcription related to the acquisition of the hallmarks of cancer or suggest any other biological processes in which all might be involved during the tumorigenic process. In our lung cancer bioinformatic analysis of TF RUNX2 (Otálora-Otálora et al., 2022a), we analyzed and compared our pipeline and results (Otálora-Otálora et al., 2019, Otálora-Otálora et al., 2022b) with previous studies, finding eleven publications assessing the lung cancer TRN with different microarray and RNA-Seq studies, and performing direct and very different bioinformatics analyses on datasets created or selected from the databases. None attempted to perform a global analysis of lung cancer, and most conducted the analysis with a reduced number of datasets for each subtype of lung cancer independently. None of them tried to select deregulated genes unique to lung cancer that are not deregulated in other lung diseases or other types of cancer, and none performed a joint co-regulatory analysis to study the cooperative and coordinated regulatory functions of TFs. However, TFs identified using our bioinformatic pipelines are also in some of the other studies but their regulatory functions during tumor processes were not deeply analyzed. Therefore, regardless of the cell types, the detection methodology of gene expression, and the bioinformatics methodology used, there is a group of regulatory genes or TFs (Otálora-Otálora et al., 2023a).
DAVID´s gene enrichment analyses in lung, colon, and gastric cancer highlights the activation of tumorigenic signaling pathways through the interaction of specific cancer-cell membrane receptors with several microorganisms, including HTLV-1, HPV, EBV, and SARS−CoV−2, which might be related to the establishment of the TRN of TFs in lung cancer (Figure 3), colon cancer (Figure 4), and gastric cancer (Figure 5), and these may be related to the formation of the microbiome network, controlling the gene expression regulation of the specific cancer-cell membrane receptors (Table 2). HTLV-1 is an enveloped single stranded RNA human deltaretrovirus that causes lifelong infection of CD4+ and CD8+ T-cells, monocytes, and other lymphoid and non-lymphoid cells via the ubiquitous glucose transporter-1 (GLUT1) and neuropilin (Einsiedel et al., 2021). GLUT1, also known as solute carrier family 2 (SLC2A1), is a common overregulated gene in lung cancer that might also function as a receptor for HTLV-1 (Manel et al., 2003). GLUT1 is a transmembrane protein involved in passive glucose transport and has a high glucose affinity compared with other transporters; therefore, it might be key in tissues where glucose is the main energy source (Kokeza et al., 2023). GLUT1 is overexpressed in various solid and hematological malignancies, such as colorectal carcinomas, gastrointestinal stromal tumors, and lung cancers (Zhang et al., 2019), but the correlation with the grade and stage of the tumor and the clinical outcome has not been defined yet (Schuurbiers et al., 2014). The HTLV-1 transactivator protein Tax is a potent activator of a variety of transcription pathways and interacts with cell cycle components dysregulating normal cell cycle controls, leading to several cellular abnormalities, including aneuploidy, the regulation of TCF3 (Figure 6), and the immortalization of T-cells, all of which play key roles in oncogenesis (Currer et al., 2012). Increased CHK1/2 activity by Tax during S phase restricts the CDK-dependent phosphorylation of FOXM1, preventing the premature expression of G2/M genes, including those encoding cyclin-dependent kinase 4, cyclin B1, and cyclin A2 (Branigan et al., 2021), all common DEGs in lung cancer (Supplementary Table 1). In lung cancer, cyclin-dependent kinase 3 may inactivate retinoblastoma and DREAM through phosphorylation for the temporal control of expression of two gene sets: the expression of the first gene set peaks in G1/S for DNA synthesis, and it might be activated by E2F1; the second set reaches maximum expression during G2/M for mitosis, coordinated by MuvB, MYBL2, and FOXM1 (Fischer et al., 2022), a complex that regulates cell cycle progression and entry into senescence (Figure 6). NETO2 (Neuropilin and tolloid-like 2) is a common overexpressed gene in lung cancer that encodes a transmembrane protein containing two extracellular CUB domains followed by a low-density lipoprotein class A domain (Figure 6), which may also be related to HTLV-1 infection, as it has been associated with clinical stage and lymph node metastasis, cell proliferation, apoptosis, tumor growth, migration, and EMT, increasing the phosphorylation of ERK (Xu et al., 2021a).
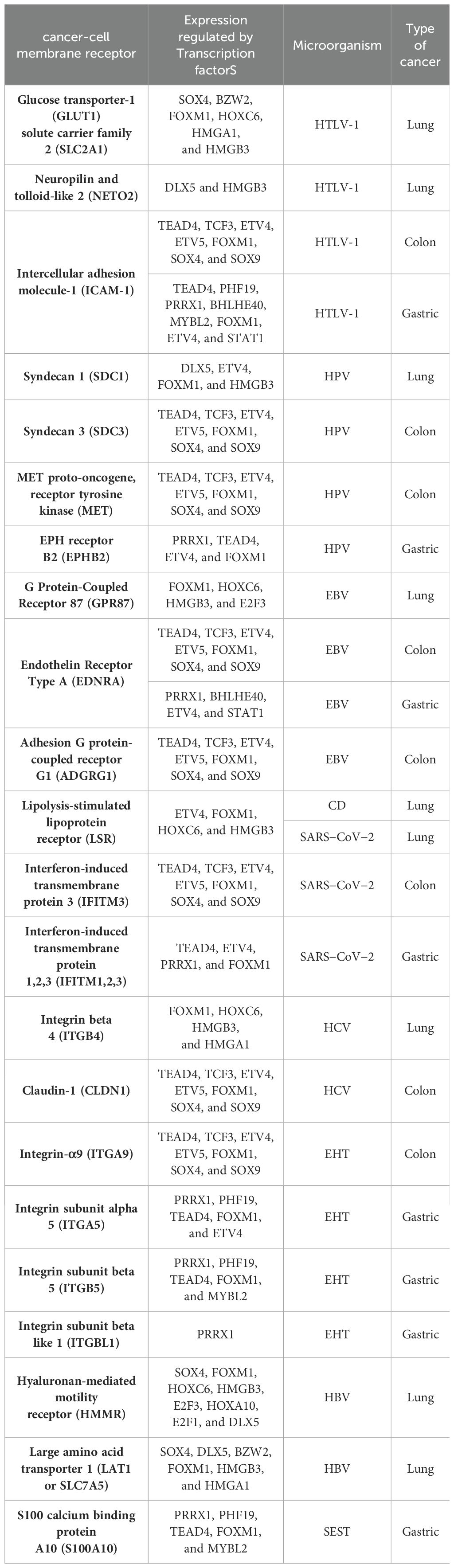
Table 2. Cancer-cell membrane receptors related to the interaction of key microorganisms according to KEGG signaling pathways and DAVID´s annotation analysis, and the regulation of their gene expression by key transcription factors.
HTLV-1 spreads directly between lymphocytes and other cells via a specialized cell-cell contact, named the virological synapse, which is accompanied by the orientation of the microtubule-organizing center in the infected T cell toward the cell contact region with the non-infected target cell, followed by intracellular Tax protein expression and the stimulation of intercellular adhesion molecule-1 (ICAM-1) on the cell surface to trigger microtubule organization center polarization in the HTLV-1–infected colon cell (Figure 7), key in the migration and activation of signaling pathways (Nejmeddine et al., 2009). HTLV-1 Tax acts on genes indirectly by binding several TFs, such as TCF3, ELK1, and MYC in colon cancer, as well as POLB, BUB3, and CDK4, according to the HTLV-1 infection signaling pathway (Figure 7). HTLV-1 HBZ might bind to JunD, possibly to mediate the activation of ICAM-1 expression, which increases the efficiency of HTLV-1 infection (Fazio et al., 2019). HTLV-1 pX ORF II encodes two proteins, p13II and p30II, multifunctional regulators that modulate Tax-responsive element-mediated transcription and repress cell and viral gene expression to favor cell survival (Green, 2004), which deregulates host signaling pathways involved in aberrant cell growth and proliferation through the induction of lysine-acetylation of c-MYC oncoprotein (Figure 7) and the inhibition of apoptosis, contributing to HTLV-1-induced carcinogenesis (Romeo et al., 2015).
HTLV-1 may spread between gastric cancer cells, followed by the combination of intracellular Tax protein expression and the stimulation of ICAM-1 on the cell surface to trigger microtubule organization center polarization (Nejmeddine et al., 2009) (Figure 8). HTLV-1 Tax directly targets G2 and mitotic regulator hsMAD1, activates mitotic spindle checkpoint function following chromosomal mis-segregation, and controls the G1/S check point, resulting in aberrant anaphase progression, chromosomal instability, DNA aneuploidy, and continuous cellular proliferation (de la Fuente et al., 2006). Tax promotes activation of the anaphase promoting complex (APC)-APCCdc20p, leading to a reduction in Pds1p/securin and Clb2p/cyclin B levels (Liu et al., 2003). Tax represses cellular DNA repair by binding to Chk2 and Chk1, impairing kinase activities in vitro and in vivo, silencing cellular checkpoints, which guard against DNA structural damage and chromosomal mis-segregation, thus increasing the appearance of a mutant phenotype and perturbing dynamic complexes that coordinate the processes of cell cycle regulation and DNA repair (Park et al., 2004).
HPV is a family of more than 200 small non-enveloped double-stranded circular DNA viruses that can be subdivided in two main groups: high risk and low risk, based on their ability to induce several types of cancers, including lung carcinomas in smoker and non-smoker subjects (Osorio et al., 2022b). HPV infects the basal cells of the mucosal epithelium through capsid proteins L1 and L2, which induce internalization of the virus, probably through the attachment to syndecan 1 (SDC1) (Ozbun and Campos, 2021). Early genomic regions E1 and E2 are the first transcribed for HPV genome amplification; E2 protein is a TF, containing viral DNA binding and transactivation domains (McBride, 2013), E5 is a multipass protein that activates receptors and induces proliferation, E6 inhibits apoptosis by interacting with p53, inducing its degradation and increasing telomerase activity, and E7 binds to protein phosphatase 2A subunits, releasing and activating TFs like E2F involved in cell cycle progression (Figure 6). Then, E6 and E7 induce genome amplification and uncontrolled proliferation in growth arrested differentiated cells, increasing the infected area to finally package HPV genome into L1 and L2 capsid protein and exit cells, which have lost nuclear and cytoplasmic integrity, aided by the E4 protein, which disrupts cytokeratin filaments (Medda et al., 2021). E7 oncoprotein rapidly induces centrosome abnormalities thereby causing the formation of supernumerary mitotic spindle poles and increasing the risk of chromosome mis-segregation (Figure 6) through a process that involves an increase in PLK4 mRNA steady-state levels (Korzeniewski et al., 2011) and tubulin gamma 1 (Starita et al., 2004), which are both overregulated common genes in lung cancer (Supplementary Table 1). HPV16 E7 recruits histone deacetylase HDAC1 and histone demethylase JARID1B or KDM5B to the regulatory region upstream of the TLR9 transcriptional start site and reduces H4 acetylation and H3K4me3, leading to the downregulation of TLR9 expression and the evasion of innate immune responses (Hasan et al., 2013). The treatment of HPV-positive tumor cells with an HDAC inhibitor increases the surface expression of the major histocompatibility complex class I (MHC-I) molecules, increasing the susceptibility of tumor cells to E7-specific CD8+ T cells (Lee et al., 2013). HPV oncoprotein E7 interacts with the DNA methyltransferase DNMT1, an overregulated common gene in lung cancer (Supplementary Table 1), stimulating its methyltransferase activity (Burgers et al., 2007) to induce epigenetic reprogramming in tumor cells.
HPV capsid proteins L1 and L2 may induce virus internalization, probably through the attachment to syndecan 3 (SDC3) (Figure 7), a known HPV-binding receptor during colon tumorigenic processes (Mukherjee et al., 2023) and a common overregulated gene in colon cancer. SDC3 could also be a WNT2 receptor in colon cancer and a potential regulator for the development of chronic inflammation (Chen et al., 2022). Early HPV genomic regions E1, E2, E5, E6, and E7 might be fulfilling some of the same functions as lung cancer (Medda et al., 2021). Furthermore, E6 can selectively upregulate WNT4, JIP1, and JIP2 translation, resulting in the activation of the non-canonical WNT-PCP-JNK pathway through the phosphorylation of mitogen-activated protein kinase (Figure 7) to promote cell proliferation and tumor growth (Zhao et al., 2019). HPV may also bypass IFITM restriction and use certain IFN-inducible proteins to facilitate virus infection (Westrich et al., 2017). HPV16 E5 may increase MET levels, a growth factor receptor critical for tumor cell invasion, motility, and cancer metastasis (Scott et al., 2018). Furthermore, MET induction by E5 requires EGFR, which is also increased by E5 at the mRNA level. Crosstalk between c-MET and various membrane protein partners, including the EGFR, α6β4 integrin, and CD44, results in additional signaling response modulation (Raj et al., 2022). CD44 upregulation enables cell self-renewal, inflammation, and migration at multiple stages and is related to a poor prognosis (Wang et al., 2019). Cell surface interaction between HMMR, CD44, HA, and tyrosine kinases activates MAP kinase cascade, which, in absence of intracellular HMMR, can regulate a mitogenic response involved in cell proliferation and random motility, and in the presence of intracellular HMMR, MAPKs bind to protein partners, which allows HMMR to enter the nucleus to regulate the expression of MYC, controlling its stabilization via AURKA (Otto et al., 2009), microtubule dynamics via the centrosome, and cell cycle progression also via AURKA, a targeting protein for TPX2, hence controlling the expression of genes involved in cell motility, such as matrix metallopeptidases (Raj et al., 2022). HPV capsid proteins L1 and L2 may induce virus internalization, probably through the attachment to neurogenic locus notch homolog protein 3 (NOTCH3) or EPH receptor B2 (EPHB2) in gastric cancer (Figure 8). HPV E7 may regulate the formation of TF complexes to control cell cycle progression and promote aberrant cellular proliferation (Das et al., 2021), as suggested by the intersect of HPV and the NOTCH signaling pathway in gastric cancer (Xie and Yan, 2023). NOTCH3 is associated with more aggressive disease and poor prognosis, acting as a molecular switch in angiogenesis and the release from tumor dormancy (Inder et al., 2017). In cancer cells, EPHB2 may promote EMT (Gao et al., 2014), and in small extracellular vesicles, EPHB2 may promote angiogenesis, inducing ephrin-B reverse signaling, and STAT phosphorylation (Sato et al., 2019).
EBV is a ubiquitous gamma herpesvirus that causes persistent infections and some lymphoid and epithelial tumors (Osorio et al., 2022a) and might use G protein-coupled receptor (GPCR) signaling (Zhang et al., 2016), like GPR87, a common overregulated DEG in lung cancer (Figure 6). Viral GPCRs are composed of seven membrane-spanning helices and intracellular and extracellular domains and have a ligand-independent signaling capacity or constitutive activation, but behave like human chemokine receptors, but behave like human chemokine receptors to guide immune cells to the site of inflammation and participate in tumor cells survival, growth, and metastasis (Zhang et al., 2016). The EBV open reading frame (BILF1) evades the host immune system by downregulating MHC class I and is capable of inducing signaling-mediated tumorigenesis (Fares et al., 2019). The N-terminal part of the large BPLF1 protein contains the catalytic site for ubiquitin ligase and deubiquitinase activity and suppresses TLR-mediated activation as a mechanism to counteract the innate antiviral immunity of infected hosts (van Gent et al., 2014). EBV nuclear antigen 1 (EBNA1) is required for the replication and maintenance of the EBV’s extrachromosomal genome in the host cell, with a GAr signal (glycine and alanine rich segment) that interferes with a protein’s degradation and allows the virus to escape host immunity via the MHC class I pathway (Finley, 2009). The EBV-encoded LMP1 oncogene is involved in the transformation, proliferation, and metastasis of several EBV-associated tumors, which are related to its ability to upregulate anti-apoptotic proteins and growth signals and the expression of p38 (MAPK13) in response to stimuli such as stress or primary infection that lead to an increase in LMP1 promoter activity, and may allow the cells to escape apoptosis, suggesting the presence of a positive autoregulatory loop in LMP1 upregulation (Johansson et al., 2010). EBV nuclear antigen (EBNA)3C functions as a transcriptional regulator by interacting with several well-known cellular and viral TFs (Kumar et al., 2009). EBNA3C recruits SKP2 E3 ligase activity to facilitate the degradation of p27KIP1 and pRb, and interacts with cyclin A, cyclin D1, p53, E2F1, and CHK2 (Bhattacharjee et al., 2016). EBV might use G protein-coupled receptor (GPCR) signaling (Zhang et al., 2016), like EDNRA and ADGRG1, which are common DEGs in colon cancer. EBV Bam HI fragment H rightward open reading frame (BHRF1) is a viral homolog of cellular BCL-2 pro-survival proteins (vBCL-2s) and confers strong resistance to diverse apoptotic stimuli and interacts with the cellular pro-apoptotic BCL-2 protein BID in colon cancer (Figure 7) to inhibit DNA-damage-induced apoptosis (Fitzsimmons et al., 2020). EBV EBNA2 induces transcription of the MYC oncogene and decreases lytic EBV replication (Münz, 2019). EBNA3C stabilizes c-Myc and recruits c-Myc and its co-factor Skp2 to c-Myc-dependent promoters, which result in increased c-Myc-dependent transcription (Kumar et al., 2009). Cyclin A, an activator of S phase progression, binds tightly to EBNA3C to stimulate cyclin A-dependent kinase activity and cell cycle progression (Knight and Robertson, 2004). EBNA3C increases the activity of the cyclin D1-CDK4 complex toward histone H1 and a truncated mutant of pRb, increasing pRb poly-ubiquitination and thereby increasing its degradation and abolishing its growth suppressive function (Saha et al., 2011).
EBV also uses GPCR signaling (Zhang et al., 2016), such as EDNRA, a common DEG in gastric cancer. BHRF1 also interacts with the cellular pro-apoptotic BCL-2 protein BID (Figure 8) to inhibit DNA-damage-induced apoptosis (Fitzsimmons et al., 2020). EBNA3C may also function in gastric cancer as a transcriptional regulator by interacting with several well-known cellular and viral TFs (Kumar et al., 2009), and recruits SKP2 to facilitate the degradation of p27KIP1 and pRb (Bhattacharjee et al., 2016). EBV LMP1 expression in gastric carcinomas may lead to tumor growth avoiding its apoptotic effects and immunologically mediated elimination (Sheu et al., 1998). LMP1 induces STAT1 expression, which probably induces tyrosine phosphorylation depending on the secretion of IFNs (Najjar et al., 2005), and transcriptional activity mediated by the specialized C-terminal activating region 1 or 2 cytoplasmic domains of LMP-1 (Richardson et al., 2003). LMP1 induces the expression of CD44 on the cell surface, a molecule implicated in increased tumor growth and dissemination (Zhu et al., 2021). EBV BGLF4 protein kinase may have a similar function as cellular cyclin-dependent kinase, regulating multiple cellular and viral substrates, which represses the poly(I:C)-stimulated expression of endogenous IFN-beta mRNA and the phosphorylation of STAT1 at Tyr701, which promotes the expression of downstream genes, suppressing host innate immune responses and facilitating virus replication (Wang et al., 2009). The C-terminal activator region 1 of LMP1 delivers a cooperating signal to induce ICAM1 mRNA in response to various inflammatory mediators, including bacterial lipopolysaccharide, phorbol esters, oxidant stress, and pro-inflammatory cytokines, such as TNFα, IL-1β, and γ-IFN (Mehl et al., 2001).
The lipolysis-stimulated lipoprotein receptor (LSR) is the target molecule for cell binding and the internalization of Clostridium difficile and might cooperate with the LDL receptors (Yen et al., 2008) to regulate cell proliferation, invasion, and migration (Zhang and Ma, 2021), probably via MAPK signaling (Nagase et al., 2022). LSR may also activate the SARS−CoV−2 S proteins and increase the viral infection of lung cancer cells (Gong et al., 2023), along with host co-receptors that might be involved in cell infection, such as NETO2, GRP87, and transmembrane protease serine 4 (TMPRSS4) (Avdonin et al., 2023) (Figure 6). Interferon-induced transmembrane protein 3 (IFITM3) is a common overregulated gene in all five datasets of colon cancer and is an immune-related protein involved in tumor transformation, with protein turnover controlled by autophagy (Friedlová et al., 2022). Y20 phosphorylation of IFITM3 hinders adaptor complex AP-2 recognizing its YEML motif, which is responsible for IFITM3 endocytosis (Chesarino et al., 2014). In colon cancer, adaptor related protein complex 1 subunit mu 1 (AP1M1), subunit sigma 2 (AP1S2), and subunit sigma 3 (AP1S3) are common overregulated genes and might be related to the accumulation of IFITM3 protein on the cell surface (Figure 7). IFITM3 mutation within its endocytosis-promoting YXXФ motif converts IFITM3 into an enhancer of SARS-CoV-2 infection by promoting virus-cell fusion (Shi et al., 2021). Krüppel-like factor 4 (KLF4) is a common downregulated TF in cancer (Figure 2) that would inhibit IFITM3 (Li et al., 2011) and SOX4 expression (Figure 4). IFITM3 might be related to CCND1 and CDK4 upregulation (Figure 7) during cell growth (Gan et al., 2019). The IFITM family may also be mutated in gastric cancer, regulating the entry of viruses into host cells (Prelli Bozzo et al., 2021), activating IFI6 and FKBP10 (Figure 8) and leading to TF phosphorylation like STAT1 (DeDiego et al., 2019). IFITM1 overexpression is related to the migration and invasiveness of gastric cancer cells (Lee et al., 2012). IFITM2 promotes gastric cancer progression by promoting cell migration and invasion, and inducing EMT (Xu et al., 2017), and interacts with the SARS-CoV-2 S at the cell surface and virus-cell fusion in early endosomes (Prelli Bozzo et al., 2021). IFTIM3 promotes gastric cancer progression, metastasis, stemness, and chemoresistance through the crosstalk between signaling pathways (Chu et al., 2022) and the activation of integrin signaling pathways (Friedlová et al., 2022). IFITM2 and IFITM3 promote human coronavirus OC43 infection, as three distinct mutations in tyrosine phosphorylation convert IFITM1 and IFITM3 from inhibitors to enhancers of SARS-CoV and MERS-CoV spike protein-mediated entry, challenging the “rigid-membrane” hypothesis (Table 1) (Zhao et al., 2018).
HCV might be involved in the activation of important tumorigenic signaling pathways of lung (Figure 6) and colon cancer (Figure 7), interacting with several membrane receptors (Table 2). HCV upregulates mRNA and protein expression levels of SLC3A2 through NS3/4A-mediated oxidative stress, as well as SLC3A2/LAT1 complex levels, contributing to HCV-mediated pathogenesis (Nguyen et al., 2018). Solute carrier family 3 member 2 (SLC3A2) can associate with integrin-β chains like Integrin beta 4 (ITGB4) in lung cancer, thereby influencing integrin signaling, cell survival, and cell migration (Fort et al., 2007). In lung cancer, protein kinase membrane-associated tyrosine/threonine 1 (PKMYT1) might be related to the activation of the MAPK signaling pathway and 4EBP1 phosphorylation (Figure 6) (Fuchs and Bode, 2005), promoting cell proliferation and apoptosis resistance (Zhang et al., 2020). ITGB4 is a heterodimer that is a non-covalently associated transmembrane glycoprotein receptor that forms complexes that vary in their ligand-binding specificities. It is an important component of the ECM that affects cell adhesion, migration, invasion, proliferation, and apoptosis during viral infection, and its phosphorylation at Y1510 is involved in the regulation of the MAPK-MEK1-ERK1/2 signaling pathway (Meng et al., 2020). In lung cancer, ITGB4 might be related to leucine-rich repeat-containing protein 15 (LRRC15), which is involved in cell-cell and cell-matrix interactions and overexpressed in mesenchymal-derived tumors (Ray et al., 2022), exerting a metastatic invasion role in lung cancer (Ruan et al., 2022). ITGB4 and urokinase-type plasminogen activator (uPA) encoded by PLAU, two common overregulated genes in lung cancer, promote angiogenesis via ERK1/2 phosphorylation, leading to cell growth (LaRusch et al., 2010; Breuss and Uhrin, 2012). The overexpression of eukaryotic translation initiation factor 4E (eIF4E)-binding protein 1 (4EBP1) in NSCLC patients has been related to a lower survival (Tang et al., 2022). 4EBP1 is a usual phosphorylation target of the mTOR and MAPK-ERK signaling pathways (Figure 6), with HPV-E6 as intermediary, causing its release from eIF4E to allow cap-dependent translation, protein synthesis, and cell growth to meet the increased metabolic demand (She et al., 2010). Low-density lipoprotein receptor-related (LDLR) protein 8 (LRP8) is a common overexpressed gene in lung cancer tissues and cell lines and is correlated with poor clinicopathological characteristics and prognosis by modulating the Wnt signaling pathway (Fang et al., 2022). LDLRs are highly conserved receptors for multiple alphaviruses, which infect vertebrate species and insect vectors separated by hundreds of millions of years of evolutionary history (Clark et al., 2022). HCV core protein directly binds to STAT1 to induce its hetero- or homodimerization, resulting in HCV resistance to IFN therapy (Anjum et al., 2013). HCV-1b core protein also induces miR-93-5p upregulation and inhibits the IFN signaling pathway by directly targeting IFNAR1, whereas the miR-93-5p-IFNAR1 axis regulates STAT1 phosphorylation, which plays a crucial role in cancer development (He et al., 2018). The multi-step process of HCV entry might be facilitated by various host factors, including the tight junction protein claudin-1 (CLDN1), which is required for efficient HCV virion accumulation at the tight junction from the basolateral membrane (So et al., 2023). BID contains a specific cleavage site recognized by HCV NS3/NS4A proteases, downstream apoptotic molecules of the mitochondrial pathway (Hsu et al., 2003). Chemokine (C-X-C motif) ligand (CXCL)10 belongs to the ELR-CXC family and is a pro-inflammatory cytokine secreted upon IFN-γ stimulation by different cell types. It is involved in a wide variety of processes such as chemotaxis, differentiation, innate defense following viral infection with the activation of peripheral immune cells, and the regulation of cell growth (Liu et al., 2011), and is induced by HCV NS3/4A (Schaefer et al., 2011). HCV core is the first synthesized protein upon viral infection, regulates viral and cell expression, induces tumorigenesis, modulates apoptosis, and suppresses host immunity (Ray and Ray, 2001). HCV core protein has a pro-proliferative role through the increase of c-myc stability (Park et al., 2011). IFN-γ production and T-cell responses are negatively regulated by suppressors of cytokine signaling (SOCS) family members SOCS1 and SOCS3 through the inhibition of the Jak-STAT pathway (Bode et al., 2003). HCV core protein inhibits T-cell responses by interacting with gC1qR (complement component 1 Q subcomponent-binding protein, mitochondrial [C1QBP] or hyaluronan-binding protein 1 [HABP1]), and SOCS1/3, suppressing STAT1/3 (Kittlesen et al., 2000). In colon cancer, HABP3 is a common overregulated gene that might be involved in the interaction with SOCS3 and the expression of IFN-stimulated genes (Figure 7). HCV NS5A confers innate immune evasion by interacting with 2′,5′-oligoadenylate synthetase (2′,5′-OAS) and inhibiting IFN antiviral activity (Taguchi et al., 2004). Human monoclonal transbodies that interfere with HCV NS5A activities have led to HCV replication inhibition and host immunity restoration (Glab-ampai et al., 2017).
Entamoeba histolytica trophozoites (EHT) might be involved in the activation of important tumorigenic signaling pathways in colon (Figure 7) and gastric cancer (Figure 8), interacting with several membrane receptors (Table 2). EHT causes substantial damage to the colonic epithelial cells that detach from the substrate to eventually be phagocytosed by the parasite (Cornick and Chadee, 2017), causing amoebiasis in 50 million people and killing 100.000 individuals around the world (Shirley et al., 2020). EHT Fibronectin (FN)-binding molecule (EhFNR) is like human β1 integrin (Talamás-Rohana et al., 1998) and is involved in adhesion, migration, and the invasion process, as well as the mobilization of the receptor molecule from internal vesicles to the plasma membrane, playing an important role during tumor development (Hernández-Ramírez et al., 2007). FN is a major host ECM component that induces actin remodeling in the parasite in a RAB21-dependent manner, forming invadosomes that promote the chemotactic migration of the metastatic cancer cells and non-transformed cells by remodeling the ECM (Emmanuel et al., 2015). RAB31 may be involved in the formation of invadosomes in colon cancer, promoting actin dot formation under an FN-induced signal in EHT, invasion, and pathogen virulence (Figure 7). The interaction with enteropathogenic Escherichia coli could modify the virulence of EH to cause amebiasis, resulting in a marked upregulation of EH cysteine proteinase (CP) virulence factors, which are critical in tumor pathogenesis and progression (Fernández-López et al., 2019). The overexpression of the EHT cysteine protease EhCP112 provokes major epithelial injury, increasing intestinal epithelial permeability, likely due to apical erosion and claudin-1 and claudin-2 degradation (Cuellar et al., 2017). The key EHT virulence factor that elicits the fast release of mucin by goblet cells as cysteine protease 5 (EhCP5) couples with goblet cell αvβ3 receptors and degrades the colonic mucus layer at the site of invasion (Cornick et al., 2016), suggesting that integrin-α9 (ITGA9) might be related to amoebiasis infection. ITGA9 is expressed in colonic glandular epithelial cells at the fetal stage and in colon adenocarcinoma, but not in normal adults (Xu et al., 2021b). ACTN1 may positively interact with ITGA9 to promote proliferation, invasion, and EMT in colon cancer (Wang et al., 2023).
The initial epithelial damage produced by EHT is characterized by the opening of tight junctions, followed by a dramatic drop in transepithelial electrical resistance with the participation of EhCPADH complex that affects claudin-1 and occludin (Betanzos et al., 2013), and damages adherens junctions and desmosomes (Hernández-Nava et al., 2017). EhFNR may also be involved in adhesion, migration, and the invasion process, as well as the mobilization of the receptor molecule from internal vesicles to the plasma membrane, playing an important role during gastric tumor development (Hernández-Ramírez et al., 2007). RAB31 may also be involved in the formation of invadosomes in gastric cancer by promoting actin dot formation under the fibronectin-induced signal in EHT and thus playing an important role during invasion and modulating pathogen virulence (Figure 8). The interaction with enteropathogenic E. coli could modify the virulence of EH to cause amebiasis, resulting in a marked upregulation of EH CP during tumor pathogenesis and progression (Fernández-López et al., 2019). Integrin subunit alpha 5 (ITGA5) and integrin subunit beta 5 (ITGB5) may form a heterodimer in gastric cancer that positively interacts with ACTN1 (Figure 8) to promote proliferation, invasion, and EMT (Wang et al., 2023). Both integrins participate in the integrin-mediated signaling pathway, viral entry into the host, HPV infection, the phagosome, focal adhesion, ECM-receptor interaction, and the cell junction (Supplementary Table 3). ITGA5 participates in the positive regulation of cell migration, along with CLDN1 and Ephrin type-B receptor 2 (EPHB2), and angiogenesis, along with EPHB2, TNFAIP2, and TNFRSF12A. ITGB5 expression contributes to a poor prognosis and is significantly associated with ECM organization, cell-substrate adhesion, focal adhesion, ECM-receptor interaction, and the phagosome (Liu et al., 2021a). The key virulence factor in live EHT may also elicit the fast release of mucin by goblet cells as EhCP5 couples with goblet cell αvβ3 receptors and degrades the colonic mucus layer at the site of invasion (Cornick et al., 2016), suggesting that ITGA5, ITGB5, and integrin subunit beta like 1 (ITGBL1) might be related to amoebiasis infection in gastric cancer.
HBV might be involved in the activation of important tumorigenic signaling pathways of lung cancer (Figure 6), interacting with several membrane receptors (Table 2). HBV is a small single-strand circular DNA virus that may be able to attach to heparan sulfate (HS) molecules and cell-free HS or SDC1, a transmembrane (type I) HS proteoglycan (HSPG), which occurs within clusters of integrins of the extracellular matrix (ECM) (Shi et al., 2013), which are receptors encoded by a common DEG in lung cancer (Supplementary Table 1). HBV may also attach to members of the solute carrier family (Somiya et al., 2016), such as SLC7A5, another common overregulated gene in lung cancer (Supplementary Table 1). The interaction between SDC1 and HMMR, the hyaluronan-mediated motility receptor overregulated in lung cancer, is related to tumor cell motility and differentiation (Yeh et al., 2018), as well as the pathological stage, T classification, lymph node metastasis, and distant metastasis (Li et al., 2021). The interaction of HMMR with SLC7A11 activates ferroptosis, enhances the cytotoxic effect of CD8 +T cells, and regulates the tumor immune microenvironment (Shan et al., 2023), suggesting an interaction with SLC7A5 in lung cancer. SLC7A5 or large amino acid transporter 1 (LAT1) is a heterodimeric transmembrane protein complex that catalyzes amino acid transport. It belongs to the SLC7-APC (amino acid-polyamine-organocation) superfamily (Scalise et al., 2018), participates in the immunosuppressive lung tumor microenvironment, and is associated with a low response to immunotherapy (Liu et al., 2022), high cancer stem cell (CSC) activity, and shorter overall survival (Liu et al., 2021c). Solute carrier family 3 member 2 (SLC3A2), or CD98hc, encodes another subunit of heterodimeric amino acid transporter that is overregulated in six lung cancer datasets and establishes a heterodimeric transmembrane protein complex with SLC7A5 to catalyze amino acid transport (Chiduza et al., 2019). HBV has evolved strategies to counter Toll-like receptor responses by suppressing their expression, regulating downstream signaling pathways related to adaptive immunity, and facilitating viral persistence (Du et al., 2022), like the non-canonical p38 mitogen-activated protein kinase (MAPK13) pathways, and probably the following phosphorylation and activation of DLX5 (Figure 6). HBV X protein (HBx) affects transcription and HBV replication, and it seems to be implicated in the regulation of BIRC5, cyclin E, and CDK4, and induces p16 hypermethylation, retinoblastoma phosphorylation, and E2F and DNMT1 activity (Tavakolian et al., 2020).
Salmonella enterica serovar Typhimurium (SEST) might be involved in the activation of important tumorigenic signaling pathways in gastric cancer (Figure 8), interacting with membrane receptors (Table 2). SEST resides in a membrane-bound compartment called the Salmonella-containing vacuole (SCV), which interacts with early endosomes to acquire a subset of late endosomal/lysosomal proteins through four characterized regulators of endocytic recycling, present on the SCV after invasion (Smith et al., 2005), from where they deliver effector proteins to the host cell via the Salmonella pathogenesis island 2 (SPI-2) type III secretion system (T3SS), inhibiting the process of antigen presentation by mature MHCII molecules (Azimi et al., 2020). SPI-5 encodes Salmonella outer protein B, which is involved in neutrophil recurrence (Wallis and Galyov, 2000), the interaction with the chloride channel, ion balance in the host cell, the management of SVC to inhibit lysosome vacuole fusion (Jantsch et al., 2011), and the returning of Rab5 to the SCV, causing the aggregation of phosphatidylinositide-3-phosphate (Mallo et al., 2008). ARF6 and Rab4 associate immediately but their presence diminishes 60 min post-infection, whereas syntaxin13 association peaks at 60 min to regulate the recycling of MHC class I. RAB11 association also peaks at 60 min to regulate the recycling of CD44 (Smith et al., 2005). RAB31 is a common overregulated gene in gastric cancer and it might be able to distinguish the intracellular vacuole of human-adapted and broad-host SEST, which may have implications for the understanding of the marked differences between SEST’s biology and the fine-tuning of T3SSs activity to adapt their function to the unique requirements of each SEST because differences in a single type III secretion effector protein result in fundamental changes to Salmonella’s intracellular niche (Spanò et al., 2011). Annexin A2 (phosphatidylinositol (4,5)-bisphosphate binding protein), p11 (S100 calcium binding protein A10 (S100A10)), and AHNAK nucleoprotein are required for the T3SS-mediated Salmonella invasion of cultured epithelial cells, and T3SS effector SopB is required for the recruitment of AnxA2 and AHNAK to Salmonella invasion sites; Salmonella can then intersect the host cell actin pathway via AnxA2 (Jolly et al., 2014). In gastric cancer, S100A10 and AHNAK2 are common DEGs that might be involved in Salmonella infection; however, annexin A2 is not (Supplementary Table 3), suggesting that the pathway might be actin independent or another protein is recruited to actin assembly sites at cellular membranes (Figure 8).
The study of the specific mechanisms by which the TRN and microbiome networks related to viral, bacterial, fungal, and parasite infections influence cancer establishment and progression in the oral-gut-lung axis will continue with: (1) single-cell transcriptomics and epigenomics in circulating extracellular vesicles (host-cells and microbially derived extracellular vesicles) and tissue tumoral cells in gastric, colon, and lung cancer patients to validate the TRN and analyze its relationship with the microbiome network; (2) proteomic and metabolomic analyses in circulating extracellular vesicles (host-cells and microbially derived extracellular vesicles) and tissue tumoral cells in gastric, colon, and lung cancer, in the same cohort, to study the formation of protein-protein interaction networks by TFs and transcription co-factors, the binding of gene expression regulatory complexes to their specific target genes, and the posttranslational regulation mediated by these regulatory complexes; (3) metaproteomic and metabolomic analyses of the microbiome network, which can activate or repress certain signal transduction pathways involved in inflammatory and tumorigenic processes; (4) multiomic analyses of inflammatory diseases, such as periodontitis, pulmonary arterial hypertension, and inflammatory bowel disease, in the development of tumorigenic processes in our population; (5) the development of three-dimensional cell organoid models of healthy individuals and cancer patients to study the response to microbiome and epidrugs controlling regulatory and epigenomics mechanisms related to the transcriptional and posttranslational regulation of host-microbiome TF networks in the treatment of tumorigenic processes within the framework of personalized medicine; and (6) exploring exosomes as new generation vehicles for cancer treatment, drug delivery, the control of signaling pathways, and genomic expression through the regulation of transcriptomic and epigenomic mechanisms. All steps are crucial for developing targeted advanced therapies against lung and gut cancer.
5 Conclusion
The global transcriptomic network analysis of gut and lung cancer highlights the impact of five TFs (SOX4, TCF3, TEAD4, ETV4, and FOXM1) that might be related to stem cell programing and cancer progression through the regulation of the expression of an important number of common deregulated genes, such as cancer-cell membrane receptors that interact with several microorganisms, including HTLV-1, HPV, EBV, and SARS−CoV−2. The regulatory function of SOX4, FOXM1, and ETV4, over other key TFs (Figure 3) and common DEGs, was highlighted in lung cancer, establishing key co-regulatory complexes in NSCLC and SCLC. The regulatory function of TEAD4, TCF3, ETV4, SOX4, and FOXM1, over other key TFs (Figure 4) and common DEGs, was highlighted in colon cancer, establishing key co-regulatory complexes. The regulatory function of TEAD4, ETV4, PRRX1, and FOXM1, over other key TFs (Figure 5) and common DEGs, was highlighted in gastric cancer, establishing key co-regulatory complexes. ETV4 and FOXM1 are the two common overregulated TFs in the three types of cancer and are important in the regulation of other key TFs and DEGs, as well as the formation of co-regulatory complexes, during the tumorigenic process in the gut-lung axis. KLF4 and ZBTB16 are the two common downregulated TFs in the three types of cancer that might control key overregulated TFs and DEGs, and therefore, their expression must be controlled by key overregulated TFs during the tumorigenic process. There are specific overregulated cancer-cell membrane receptors crucial for the interaction with HTVL-1, HPV, EBV, and SARS−CoV−2 in every type of cancer, which are regulated by the key TFs (Table 2) and might be involved in the regulation of the MAPK signaling pathway (Figure 6), the non-canonical Wnt signaling pathway (Figure 7), and the regulation of the IFN signaling pathway (Figure 8) during the lung and gut tumorigenic process.
Our global transcriptomic analysis suggests a complex crosstalk between the microbiome and cancer TRN, as shown by the co-expression of common DEGs that codify for membrane receptors and TFs, and consequently, the possible participation in the same biological process, along with all experimental studies that have demonstrated that the interaction of the microbiome and specific receptors identified as common DEGs in cancer might be able to activate signaling pathways that regulate gene expression. The actual evidence in the field highlighted receptors that might be involved in the crosstalk between the microbiome network and the host cell TRN in a spatial, temporal, and sequential manner during the tumorigenic process in the gut-lung axis. Additionally, the evidence identified signaling pathways activated downstream that might be implicated in the regulation of the host cancer cell TRN, the regulation of gene expression during the establishment and progression of cancer, and in turn in the regulation of the communication with the microbiome network.
The regulatory function of key TFs over all common DEGs must be validated experimentally to fully understand how they are involved in the interaction of host cancer cells and microbiome gene expression networks that might be able to unlock cell phenotypic plasticity for the acquisition of the hallmarks of cancer in the gut-lung axis. All our findings must be experimentally validated with proper methodologies to specifically prove how, when, and where: 1) the microbiome network is interacting with the membrane receptors of cancer-related cells; 2) the host-microbiome interaction activates the signaling pathways related to gene expression; and 3) the cancer TRN is regulating the crosstalk with the microbiome network. In vitro studies of single and multiple microorganism infection are necessary to gain insight into general entry mechanisms and the activation and/or silencing of specific signaling pathways, as are studies involving patient cohorts that aim to identify the clinical relevance of molecular host-virus interactions, which are essential in the development of novel diagnostic and treatment approaches that control the formation of microorganism-host protein complexes. Then, it will be necessary to identify which signaling pathway is regulating the expression of which TFs and which DEGs are being controlled by which TFs, and highlighting between those, the genes that codify for membrane receptors that interact with the microbiome network; only then will the regulation circle of the tumorigenic processes between the microbiome and cancer TRNs be fully complete.
Data availability statement
The original contributions presented in the study are included in the article/Supplementary Material. Further inquiries can be directed to the corresponding author.
Ethics statement
Ethical approval was not required for the study involving humans in accordance with the local legislation and institutional requirements. Written informed consent to participate in this study was not required from the participants or the participants’ legal guardians/next of kin in accordance with the national legislation and the institutional requirements.
Author contributions
BO-O: Conceptualization, Formal analysis, Investigation, Methodology, Writing – original draft, Writing – review & editing. CP-G: Data curation, Formal analysis, Methodology, Software, Writing – review & editing. JL-R: Investigation, Writing – review & editing. NP-A: Data curation, Formal analysis, Methodology, Software, Writing – review & editing. CA-G: Writing – review & editing. MI-R: Writing – review & editing. CÁ-M: Conceptualization, Investigation, Writing – review & editing.
Funding
The author(s) declare that no financial support was received for the research, authorship, and/or publication of this article.
Conflict of interest
Authors JL-R and CÁ-M were employed by the company Clínica Colsanitas S.A.
The remaining authors declare that the research was conducted in the absence of any commercial or financial relationships that could be construed as a potential conflict of interest.
Publisher’s note
All claims expressed in this article are solely those of the authors and do not necessarily represent those of their affiliated organizations, or those of the publisher, the editors and the reviewers. Any product that may be evaluated in this article, or claim that may be made by its manufacturer, is not guaranteed or endorsed by the publisher.
Supplementary material
The Supplementary Material for this article can be found online at: https://www.frontiersin.org/articles/10.3389/fcimb.2024.1425388/full#supplementary-material
Supplementary Methods 1 | Bioinformatic pipeline.
Supplementary Table 1 | Lung cancer common DEGs selection, annotation, and gene regulatory networks.
Supplementary Table 2 | Colon cancer common DEGs selection, annotation, and gene regulatory networks.
Supplementary Table 3 | Gastric cancer common DEGs selection, annotation, and gene regulatory networks.
References
Ackermann, C. J., Guller, U., Jochum, W., Schmied, B. M., Warschkow, R. (2018). The prognostic value of signet ring cell histology in stage I/II colon cancer—a population-based, propensity score-matched analysis. Int. J. Colorectal Dis. 33, 1183–1193. doi: 10.1007/s00384-018-3096-5
Anjum, S., Afzal Sohail, M., Ahmad, T., Aslam, B., Waheed, Y., Shafi, T., et al. (2013). Mutations in the STAT1-interacting domain of the hepatitis C virus core protein modulate the response to antiviral therapy. Mol. Med. Rep. 8, 487–492. doi: 10.3892/mmr.2013.1541
Avdonin, P. P., Rybakova, E. Y., Trufanov, S. K., Avdonin, P. V. (2023). SARS-CoV-2 receptors and their involvement in cell infection. Biochem. (Mosc) Suppl. Ser. A Membr Cell Biol. 17, 1–11. doi: 10.1134/S1990747822060034
Azimi, T., Zamirnasta, M., Sani, M. A., Soltan Dallal, M. M., Nasser, A. (2020). Molecular mechanisms of salmonella effector proteins: A comprehensive review. Infect. Drug Resist. 13, 11–26. doi: 10.2147/IDR.S230604
Betanzos, A., Javier-Reyna, R., García-Rivera, G., Bañuelos, C., González-Mariscal, L., Schnoor, M., et al. (2013). The EhCPADH112 complex of Entamoeba histolytica interacts with tight junction proteins occludin and claudin-1 to produce epithelial damage. PloS One 8, e65100. doi: 10.1371/journal.pone.0065100
Bhattacharjee, S., Ghosh Roy, S., Bose, P., Saha, A. (2016). Role of EBNA-3 family proteins in EBV associated B-cell lymphomagenesis. Front. Microbiol. 7. doi: 10.3389/fmicb.2016.00457
Bode, J. G., Ludwig, S., Ehrhardt, C., Albrecht, U., Erhardt, A., Schaper, F., et al. (2003). IFN-alpha antagonistic activity of HCV core protein involves induction of suppressor of cytokine signaling-3. FASEB J. 17, 488–490. doi: 10.1096/fj.02-0664fje
Branigan, T. B., Kozono, D., SChade, A. E., Deraska, P., Rivas, H. G., Sambel, L., et al. (2021). MMB-FOXM1-driven premature mitosis is required for CHK1 inhibitor sensitivity. Cell Rep. 34, 108808. doi: 10.1016/j.celrep.2021.108808
Breuss, J. M., Uhrin, P. (2012). VEGF-initiated angiogenesis and the uPA/uPAR system. Cell Adh Migr 6, 535–615. doi: 10.4161/cam.22243
Burgers, W. A., Blanchon, L., Pradhan, S., Launoit, Y.d., Kouzarides, T., Fuks, F. (2007). Viral oncoproteins target the DNA methyltransferases. Oncogene 26, 1650–1655. doi: 10.1038/sj.onc.1209950
Castillo, S. D., Matheu, A., Mariani, N., Carretero, J., Lopez-Rios, F., Lovell-Badge, R., et al. (2012). Novel transcriptional targets of the SRY-HMG box transcription factor SOX4 link its expression to the development of small cell lung cancer. Cancer Res. 72, 176–186. doi: 10.1158/0008-5472.CAN-11-3506
Chen, C., Luo, L., Xu, C., Yang, X., Liu, T., Luo, J., et al. (2022). Tumor specificity of WNT ligands and receptors reveals universal squamous cell carcinoma oncogenes. BMC Cancer 22, 790. doi: 10.1186/s12885-022-09898-2
Chen, S., Cao, Z., Prettner, K., Kuhn, M., Yang, J., Jiao, L., et al. (2023). Estimates and projections of the global economic cost of 29 cancers in 204 countries and territories from 2020 to 2050. JAMA Oncol. 9, 465–472. doi: 10.1001/jamaoncol.2022.7826
Chesarino, N. M., McMichael, T. M., Hach, J. C., Yount, J. S. (2014). Phosphorylation of the antiviral protein interferon-inducible transmembrane protein 3 (IFITM3) dually regulates its endocytosis and ubiquitination. J. Biol. Chem. 289, 11986–11992. doi: 10.1074/jbc.M114.557694
Chiduza, G. N., Johnson, R. M., Wright, G. S. A., Antonyuk, S. V., Muench, S. P., Hasnain, S. S. (2019). LAT1 (SLC7A5) and CD98hc (SLC3A2) complex dynamics revealed by single-particle cryo-EM. Acta Crystallogr. D Struct. Biol. 75, 660–669. doi: 10.1107/S2059798319009094
Chow, R. D., Bradley, E. H., Gross, C. P. (2022). Comparison of cancer-related spending and mortality rates in the US vs 21 high-income countries. JAMA Health Forum 3, e221229–e221229. doi: 10.1001/jamahealthforum.2022.1229
Chu, P.-Y., Huang, W.-C., Tung, S.-L., Tsai, C.-Y., Chen, C. J., Liu, Y.-C., et al. (2022). IFITM3 promotes Malignant progression, cancer stemness and chemoresistance of gastric cancer by targeting MET/AKT/FOXO3/c-MYC axis. Cell Biosci. 12, 124. doi: 10.1186/s13578-022-00858-8
Clark, L. E., Clark, S. A., Lin, C., Liu, J., Coscia, A., Nabel, K. G., et al. (2022). VLDLR and ApoER2 are receptors for multiple alphaviruses. Nature 602, 475–480. doi: 10.1038/s41586-021-04326-0
Cordero, D., Solé, X., Crous-Bou, M., Sanz-Pamplona, R., Paré-Brunet, L., Guinó, E., et al. (2014). Large differences in global transcriptional regulatory programs of normal and tumor colon cells. BMC Cancer 14. doi: 10.1186/1471-2407-14-708
Cornick, S., Chadee, K. (2017). Entamoeba histolytica: Host parasite interactions at the colonic epithelium. Tissue Barriers 5, e1283386. doi: 10.1080/21688370.2017.1283386
Cornick, S., Moreau, F., Chadee, K. (2016). Entamoeba histolytica Cysteine Proteinase 5 Evokes Mucin Exocytosis from Colonic Goblet Cells via αvβ3 Integrin. PloS Pathog. 12, e1005579. doi: 10.1371/journal.ppat.1005579
Cuellar, P., Hernández-Nava, E., García-Rivera, G., Chávez-Munguía, B., Schnoor, M., Betanzos, A., et al. (2017). Entamoeba histolytica EhCP112 Dislocates and Degrades Claudin-1 and Claudin-2 at Tight Junctions of the Intestinal Epithelium. Front. Cell Infect. Microbiol. 7. doi: 10.3389/fcimb.2017.00372
Currer, R., Van Duyne, R., Jaworski, E., Guendel, I., Sampey, G., Das, R., et al. (2012). HTLV tax: a fascinating multifunctional co-regulator of viral and cellular pathways. Front. Microbiol. 3. doi: 10.3389/fmicb.2012.00406
Das, T., Zhong, R., Spiotto, M. T. (2021). Notch signaling and human papillomavirus-associated oral tumorigenesis. Adv. Exp. Med. Biol. 1287, 105–122. doi: 10.1007/978-3-030-55031-8_8
DeDiego, M. L., Nogales, A., Martinez-Sobrido, L., Topham, D. J. (2019). Interferon-induced protein 44 interacts with cellular FK506-binding protein 5, negatively regulates host antiviral responses, and supports virus replication. mBio 10. doi: 10.1128/mBio.01839-19
de la Fuente, C., Gupta, M. V., Klase, Z., Strouss, K., Cahan, P., McCaffery, T., et al. (2006). Involvement of HTLV-I Tax and CREB in aneuploidy: a bioinformatics approach. Retrovirology 3, 43. doi: 10.1186/1742-4690-3-43
Dong, C., Rao, N., Du, W., Gao, F., Lv, X., Wang, G., et al. (2021). mRBioM: an algorithm for the identification of potential mRNA biomarkers from complete transcriptomic profiles of gastric adenocarcinoma. Front. Genet. 12. doi: 10.3389/fgene.2021.679612
Du, Y., Wu, J., Liu, J., Zheng, X., Yang, D., Lu, M. (2022). Toll-like receptor-mediated innate immunity orchestrates adaptive immune responses in HBV infection. Front. Immunol. 13. doi: 10.3389/fimmu.2022.965018
Einsiedel, L., Chiong, F., Jersmann, H., Taylor, G. P. (2021). Human T-cell leukaemia virus type 1 associated pulmonary disease: clinical and pathological features of an under-recognised complication of HTLV-1 infection. Retrovirology 18, 1. doi: 10.1186/s12977-020-00543-z
Emmanuel, M., Nakano, Y. S., Nozaki, T., Datta, S. (2015). Small GTPase Rab21 mediates fibronectin induced actin reorganization in Entamoeba histolytica: implications in pathogen invasion. PloS Pathog. 11, e1004666. doi: 10.1371/journal.ppat.1004666
Fang, Z., Zhong, M., Zhou, L., Le, Y., Wang, H., Fang, Z. (2022). Low-density lipoprotein receptor-related protein 8 facilitates the proliferation and invasion of non-small cell lung cancer cells by regulating the Wnt/β-catenin signaling pathway. Bioengineered 13, 6807–6818. doi: 10.1080/21655979.2022.2036917
Fares, S., Spiess, K., Olesen, E. T. B., Zuo, J., Jackson, S., Kledal, T. N., et al. (2019). Distinct roles of extracellular domains in the epstein-barr virus-encoded BILF1 receptor for signaling and major histocompatibility complex class I downregulation. mBio 10. doi: 10.1128/mBio.01707-18
Fazio, A. L., Kendle, W., Hoang, K., Korleski, E., Lemasson, I., Polakowski, N. (2019). Human T-cell leukemia virus type 1 (HTLV-1) bZIP factor upregulates the expression of ICAM-1 to facilitate HTLV-1 infection. J. Virol. 93. doi: 10.1128/JVI.00608-19
Fernández-López, L. A., Gil-Becerril, K., Galindo-Gómez, S., Estrada-García, T., Ximénez, C., Leon-Coria, A., et al. (2019). Entamoeba histolytica Interaction with Enteropathogenic Escherichia coli Increases Parasite Virulence and Inflammation in Amebiasis. Infect. Immun. 87. doi: 10.1128/IAI.00279-19
Filtz, T. M., Vogel, W. K., Leid, M. (2014). Regulation of transcription factor activity by interconnected post-translational modifications. Trends Pharmacol. Sci. 35, 76–85. doi: 10.1016/j.tips.2013.11.005
Finley, D. (2009). Recognition and processing of ubiquitin-protein conjugates by the proteasome. Annu. Rev. Biochem. 78, 477–513. doi: 10.1146/annurev.biochem.78.081507.101607
Fischer, M., SChade, A. E., Branigan, T. B., Müller, G. A., DeCaprio, J. A. (2022). Coordinating gene expression during the cell cycle. Trends Biochem. Sci. 47, 1009–1022. doi: 10.1016/j.tibs.2022.06.007
Fitzsimmons, L., Cartlidge, R., Chang, C., Sejic, N., Galbraith, L. C. A., Suraweera, C. D., et al. (2020). EBV BCL-2 homologue BHRF1 drives chemoresistance and lymphomagenesis by inhibiting multiple cellular pro-apoptotic proteins. Cell Death Differ 27, 1554–1568. doi: 10.1038/s41418-019-0435-1
Fleming, M., Ravula, S., Tatishchev, S. F., Wang, H. L. (2012). Colorectal carcinoma: Pathologic aspects. J. Gastrointest Oncol. 3, 153–173. doi: 10.3978/j.issn.2078-6891.2012.030
Fonseca, A. S., Ramão, A., Bürger, M. C., de Souza, J. E. S., Zanette, D. L., de Molfetta, G. A., et al. (2021). ETV4 plays a role on the primary events during the adenoma-adenocarcinoma progression in colorectal cancer. BMC Cancer 21. doi: 10.1186/s12885-021-07857-x
Fort, J., de la Ballina, L. R., Burghardt, H. E., Ferrer-Costa, C., Turnay, J., Ferrer-Orta, C., et al. (2007). The structure of human 4F2hc ectodomain provides a model for homodimerization and electrostatic interaction with plasma membrane. J. Biol. Chem. 282, 31444–31452. doi: 10.1074/jbc.M704524200
Franzin, R., Netti, G. S., Spadaccino, F., Porta, C., Gesualdo, L., Stallone, G., et al. (2020). The use of immune checkpoint inhibitors in oncology and the occurrence of AKI: where do we stand? Front. Immunol. 11. doi: 10.3389/fimmu.2020.574271
Friedlová, N., Zavadil Kokáš, F., Hupp, T. R., Vojtěšek, B., Nekulová, M. (2022). IFITM protein regulation and functions: Far beyond the fight against viruses. Front. Immunol. 13. doi: 10.3389/fimmu.2022.1042368
Fuchs, B. C., Bode, B. P. (2005). Amino acid transporters ASCT2 and LAT1 in cancer: Partners in crime? Semin. Cancer Biol. 15, 254–266. doi: 10.1016/j.semcancer.2005.04.005
Galli, G., Rossi, G. (2021). Lung cancer histology-driven strategic therapeutic approaches. Shanghai Chest 4, 1–16. doi: 10.21037/shc.2020.01.03
Gan, C. P., Sam, K. K., Yee, P. S., Zainal, N. S., Lee, B. K. B., Abdul Rahman, Z. A., et al. (2019). IFITM3 knockdown reduces the expression of CCND1 and CDK4 and suppresses the growth of oral squamous cell carcinoma cells. Cell Oncol. (Dordr) 42, 477–490. doi: 10.1007/s13402-019-00437-z
Gao, Q., Liu, W., Cai, J., Li, M., Gao, Y., Lin, W., et al. (2014). EphB2 promotes cervical cancer progression by inducing epithelial-mesenchymal transition. Hum. Pathol. 45, 372–381. doi: 10.1016/j.humpath.2013.10.001
Glab-ampai, K., Chulanetra, M., Malik, A. A., Juntadech, T., Thanongsaksrikul, J., Srimanote, P., et al. (2017). Human single chain-transbodies that bound to domain-I of non-structural protein 5A (NS5A) of hepatitis C virus. Sci. Rep. 7, 15042. doi: 10.1038/s41598-017-14886-9
Gómez-Orte, E., Sáenz-Narciso, B., Moreno, S., Cabello, J. (2013). Multiple functions of the noncanonical Wnt pathway. Trends Genet. 29, 545–553. doi: 10.1016/j.tig.2013.06.003
Gong, Y., An, Y., Wang, C., Wang, Z., Kong, F., Jiang, M., et al. (2023). LSR is a cell-intrinsic defence factor against SARS-CoV-2 infection in small intestine. doi: 10.21203/rs.3.rs-3579436/v1
Green, P. L. (2004). HTLV-1 p30II: selective repressor of gene expression. Retrovirology 1, 40. doi: 10.1186/1742-4690-1-40
Gregorieff, A., Clevers, H. (2005). Wnt signaling in the intestinal epithelium: from endoderm to cancer. Genes Dev. 19, 877–890. doi: 10.1101/gad.1295405
Groenevelt, C., Gordon, R., Wang, X., Fletcher, M., Markowetz, F., Meyer, K., et al. (2024). Package ‘ RTN.’. 1–70. Available at: https://bioconductor.org/packages/release/bioc/html/RTN.html.
Gu, C., Huang, Z., Chen, X., Liu, C., Rocco, G., Zhao, S., et al. (2020). TEAD4 promotes tumor development in patients with lung adenocarcinoma via ERK signaling pathway. Biochim. Biophys. Acta (BBA) - Mol. Basis Dis. 1866, 165921. doi: 10.1016/j.bbadis.2020.165921
Guilford, P., Hopkins, J., Harraway, J., McLeod, M., McLeod, N., Harawira, P., et al. (1998). E-cadherin germline mutations in familial gastric cancer. Nature 392, 402–405. doi: 10.1038/32918
Hanahan, D. (2022). Hallmarks of cancer: new dimensions. Cancer Discovery 12, 31–46. doi: 10.1158/2159-8290.CD-21-1059
Hasan, U. A., Zannetti, C., Parroche, P., Goutagny, N., Malfroy, M., Roblot, G., et al. (2013). The human papillomavirus type 16 E7 oncoprotein induces a transcriptional repressor complex on the Toll-like receptor 9 promoter. J. Exp. Med. 210, 1369–1387. doi: 10.1084/jem.20122394
He, C.-L., Liu, M., Tan, Z.-X., Hu, Y.-J., Zhang, Q.-Y., Kuang, X.-M., et al. (2018). Hepatitis C virus core protein-induced miR-93-5p up-regulation inhibits interferon signaling pathway by targeting IFNAR1. World J. Gastroenterol. 24, 226–236. doi: 10.3748/wjg.v24.i2.226
Hernández-Nava, E., Cuellar, P., Nava, P., Chávez-Munguía, B., Schnoor, M., Orozco, E., et al. (2017). Adherens junctions and desmosomes are damaged by Entamoeba histolytica: Participation of EhCPADH complex and EhCP112 protease. Cell Microbiol. 19. doi: 10.1111/cmi.12761
Hernández-Ramírez, V. I., Rios, A., Angel, A., Magos, M. A., Pérez-Castillo, L., Rosales-Encina, J. L., et al. (2007). Subcellular distribution of the Entamoeba histolytica 140 kDa FN-binding molecule during host-parasite interaction. Parasitology 134, 169–177. doi: 10.1017/S0031182006001260
Hsu, E. C., Hsi, B., Hirota-Tsuchihara, M., Ruland, J., Iorio, C., Sarangi, F., et al. (2003). Modified apoptotic molecule (BID) reduces hepatitis C virus infection in mice with chimeric human livers. Nat. Biotechnol. 21, 519–525. doi: 10.1038/nbt817
Hwang, M.-A., Won, M., Im, J.-Y., Kang, M.-J., Kweon, D.-H., Kim, B.-K. (2022). TNF-α Secreted from macrophages increases the expression of prometastatic integrin αV in gastric cancer. Int. J. Mol. Sci. 24. doi: 10.3390/ijms24010376
Inder, S., O’Rourke, S., McDermott, N., Manecksha, R., Finn, S., Lynch, T., et al. (2017). The Notch-3 receptor: A molecular switch to tumorigenesis? Cancer Treat Rev. 60, 69–76. doi: 10.1016/j.ctrv.2017.08.011
James, C. D., Fontan, C. T., Otoa, R., Das, D., Prabhakar, A. T., Wang, X., et al. (2020). Human papillomavirus 16 E6 and E7 synergistically repress innate immune gene transcription. mSphere 5. doi: 10.1128/mSphere.00828-19
Jantsch, J., Chikkaballi, D., Hensel, M. (2011). Cellular aspects of immunity to intracellular Salmonella enterica. Immunol. Rev. 240, 185–195. doi: 10.1111/j.1600-065X.2010.00981.x
Jho, E., Zhang, T., Domon, C., Joo, C.-K., Freund, J.-N., Costantini, F. (2002). Wnt/beta-catenin/Tcf signaling induces the transcription of Axin2, a negative regulator of the signaling pathway. Mol. Cell Biol. 22, 1172–1183. doi: 10.1128/MCB.22.4.1172-1183.2002
Jiao, S., Li, C., Hao, Q., Miao, H., Zhang, L., Li, L., et al. (2017). VGLL4 targets a TCF4-TEAD4 complex to coregulate Wnt and Hippo signalling in colorectal cancer. Nat. Commun. 8, 14058. doi: 10.1038/ncomms14058
Johansson, P., Jansson, A., Rüetschi, U., Rymo, L. (2010). The p38 signaling pathway upregulates expression of the Epstein-Barr virus LMP1 oncogene. J. Virol. 84, 2787–2797. doi: 10.1128/JVI.01052-09
Jolly, C., Winfree, S., Hansen, B., Steele-Mortimer, O. (2014). The Annexin A2/p11 complex is required for efficient invasion of Salmonella Typhimurium in epithelial cells. Cell Microbiol. 16, 64–77. doi: 10.1111/cmi.2014.16.issue-1
Kim, S. T., Cristescu, R., Bass, A. J., Kim, K.-M., Odegaard, J. I., Kim, K., et al. (2018). Comprehensive molecular characterization of clinical responses to PD-1 inhibition in metastatic gastric cancer. Nat. Med. 24, 1449–1458. doi: 10.1038/s41591-018-0101-z
Kittlesen, D. J., Chianese-Bullock, K. A., Yao, Z. Q., Braciale, T. J., Hahn, Y. S. (2000). Interaction between complement receptor gC1qR and hepatitis C virus core protein inhibits T-lymphocyte proliferation. J. Clin. Invest. 106, 1239–1249. doi: 10.1172/JCI10323
Knight, J. S., Robertson, E. S. (2004). Epstein-Barr virus nuclear antigen 3C regulates cyclin A/p27 complexes and enhances cyclin A-dependent kinase activity. J. Virol. 78, 1981–1991. doi: 10.1128/JVI.78.4.1981-1991.2004
Kokeza, J., Strikic, A., Ogorevc, M., Kelam, N., Vukoja, M., Dilber, I., et al. (2023). The effect of GLUT1 and HIF-1α Expressions on glucose uptake and patient survival in non-small-cell lung carcinoma. Int. J. Mol. Sci. 24. doi: 10.3390/ijms241310575
Korzeniewski, N., Treat, B., Duensing, S. (2011). The HPV-16 E7 oncoprotein induces centriole multiplication through deregulation of Polo-like kinase 4 expression. Mol. Cancer 10, 61. doi: 10.1186/1476-4598-10-61
Kumar, P., Murakami, M., Kaul, R., Saha, A., Cai, Q., Robertson, E. S. (2009). Deregulation of the cell cycle machinery by Epstein-Barr virus nuclear antigen 3C. Future Virol. 4, 79–91. doi: 10.2217/17460794.4.1.79
Kushima, R. (2022). The updated WHO classification of digestive system tumours-gastric adenocarcinoma and dysplasia. Pathologe 43, 8–15. doi: 10.1007/s00292-021-01023-7
Lai, Y.-H., Cheng, J., Cheng, D., Feasel, M. E., Beste, K. D., Peng, J., et al. (2011). SOX4 interacts with plakoglobin in a Wnt3a-dependent manner in prostate cancer cells. BMC Cell Biol. 12, 50. doi: 10.1186/1471-2121-12-50
LaRusch, G. A., Mahdi, F., Shariat-Madar, Z., Adams, G., Sitrin, R. G., Zhang, W. M., et al. (2010). Factor XII stimulates ERK1/2 and Akt through uPAR, integrins, and the EGFR to initiate angiogenesis. Blood 115, 5111–5120. doi: 10.1182/blood-2009-08-236430
Lauren, P. (1965). The two histological main types of gastric carcinoma: diffuse and so-called intestinal-type carcinoma. An attempt at a histo-clinical classification. Acta Pathol. Microbiol. Scand. 64, 31–49. doi: 10.1111/apm.1965.64.1.31
Lee, J., Goh, S.-H., Song, N., Hwang, J.-A., Nam, S., Choi, I. J., et al. (2012). Overexpression of IFITM1 has clinicopathologic effects on gastric cancer and is regulated by an epigenetic mechanism. Am. J. Pathol. 181, 43–52. doi: 10.1016/j.ajpath.2012.03.027
Lee, S. Y., Huang, Z., Kang, T. H., Soong, R.-S., Knoff, J., Axenfeld, E., et al. (2013). Histone deacetylase inhibitor AR-42 enhances E7-specific CD8+ T cell-mediated antitumor immunity induced by therapeutic HPV DNA vaccination. J. Mol. Med. 91, 1221–1231. doi: 10.1007/s00109-013-1054-9
Li, C., Cai, S., Wang, X., Jiang, Z. (2014). Hypomethylation-associated up-regulation of TCF3 expression and recurrence in stage II and III colorectal cancer. PloS One 9, 1–10. doi: 10.1371/journal.pone.0112005
Li, X., Pan, K., Vieth, M., Gerhard, M., Li, W., Mejías-Luque, R. (2022). JAK-STAT1 signaling pathway is an early response to helicobacter pylori infection and contributes to immune escape and gastric carcinogenesis. Int. J. Mol. Sci. 23. doi: 10.3390/ijms23084147
Li, D., Peng, Z., Tang, H., Wei, P., Kong, X., Yan, D., et al. (2011). KLF4-mediated negative regulation of IFITM3 expression plays a critical role in colon cancer pathogenesis. Clin. Cancer Res. 17, 3558–3568. doi: 10.1158/1078-0432.CCR-10-2729
Li, X., Zuo, H., Zhang, L., Sun, Q., Xin, Y., Zhang, L. (2021). Validating HMMR expression and its prognostic significance in lung adenocarcinoma based on data mining and bioinformatics methods. Front. Oncol. 11. doi: 10.3389/fonc.2021.720302
Liang, S.-K., Hsu, C.-C., Song, H.-L., Huang, Y.-C., Kuo, C.-W., Yao, X., et al. (2021). FOXM1 is required for small cell lung cancer tumorigenesis and associated with poor clinical prognosis. Oncogene 40, 4847–4858. doi: 10.1038/s41388-021-01895-2
Liu, M., Guo, S., Stiles, J. K. (2011). The emerging role of CXCL10 in cancer (Review). Oncol. Lett. 2, 583–589. doi: 10.3892/ol.2011.300
Liu, M., Hu, W., Meng, X., Wang, B. (2024). TEAD4: A key regulator of tumor metastasis and chemoresistance - Mechanisms and therapeutic implications. Biochim. Biophys. Acta (BBA) - Rev. Cancer 1879, 189050. doi: 10.1016/j.bbcan.2023.189050
Liu, Y.-H., Li, Y.-L., Shen, H.-T., Chien, P.-J., Sheu, G.-T., Wang, B.-Y., et al. (2021c). L-type amino acid transporter 1 regulates cancer stemness and the expression of programmed cell death 1 ligand 1 in lung cancer cells. Int. J. Mol. Sci. 22. doi: 10.3390/ijms222010955
Liu, B., Liang, M.-H., Kuo, Y., Liao, W., Boros, I., Kleinberger, T., et al. (2003). Human T-lymphotropic virus type 1 oncoprotein tax promotes unscheduled degradation of Pds1p/securin and Clb2p/cyclin B1 and causes chromosomal instability. Mol. Cell Biol. 23, 5269–5281. doi: 10.1128/MCB.23.15.5269-5281.2003
Liu, D., Liu, S., Fang, Y., Liu, L., Hu, K. (2021a). Comprehensive analysis of the expression and prognosis for ITGBs: identification of ITGB5 as a biomarker of poor prognosis and correlated with immune infiltrates in gastric cancer. Front. Cell Dev. Biol. 9. doi: 10.3389/fcell.2021.816230
Liu, Y., Ma, G., Liu, J., Zheng, H., Huang, G., Song, Q., et al. (2022). SLC7A5 is a lung adenocarcinoma-specific prognostic biomarker and participates in forming immunosuppressive tumor microenvironment. Heliyon 8, e10866. doi: 10.1016/j.heliyon.2022.e10866
Liu, J., Qiu, J., Zhang, Z., Zhou, L., Li, Y., Ding, D., et al. (2021b). SOX4 maintains the stemness of cancer cells via transcriptionally enhancing HDAC1 revealed by comparative proteomics study. Cell Biosci. 11, 23. doi: 10.1186/s13578-021-00539-y
Love, M. I., Huber, W., Anders, S. (2014). Moderated estimation of fold change and dispersion for RNA-seq data with DESeq2. Genome Biol. 15, 550. doi: 10.1186/s13059-014-0550-8
Lu, Y.-X., Ju, H.-Q., Liu, Z.-X., Chen, D.-L., Wang, Y., Zhao, Q., et al. (2019). Correction: ME1 regulates NADPH homeostasis to promote gastric cancer growth and metastasis. Cancer Res. 79, 3789. doi: 10.1158/0008-5472.CAN-19-1611
Mallo, G. V., Espina, M., Smith, A. C., Terebiznik, M. R., Alemán, A., Finlay, B. B., et al. (2008). SopB promotes phosphatidylinositol 3-phosphate formation on Salmonella vacuoles by recruiting Rab5 and Vps34. J. Cell Biol. 182, 741–752. doi: 10.1083/jcb.200804131
Manel, N., Kim, F. J., Kinet, S., Taylor, N., Sitbon, M., Battini, J.-L. (2003). The ubiquitous glucose transporter GLUT-1 is a receptor for HTLV. Cell 115, 449–459. doi: 10.1016/S0092-8674(03)00881-X
Mastrogamvraki, N., Zaravinos, A. (2020). Signatures of co-deregulated genes and their transcriptional regulators in colorectal cancer. NPJ Syst. Biol. Appl. 6, 1–16. doi: 10.1038/s41540-020-00144-8
McBride, A. A. (2013). The papillomavirus E2 proteins. Virology 445, 57–79. doi: 10.1016/j.virol.2013.06.006
Medda, A., Duca, D., Chiocca, S. (2021). Human papillomavirus and cellular pathways: hits and targets. Pathogens 10. doi: 10.3390/pathogens10030262
Mehl, A. M., Floettmann, J. E., Jones, M., Brennan, P., Rowe, M. (2001). Characterization of intercellular adhesion molecule-1 regulation by epstein-barr virus-encoded latent membrane protein-1 identifies pathways that cooperate with nuclear factor κB to activate transcription*. J. Biol. Chem. 276, 984–992. doi: 10.1074/jbc.M003758200
Meng, Z., Chen, Y., Wu, W., Yan, B., Zhang, L., Chen, H., et al. (2022b). PRRX1 is a novel prognostic biomarker and facilitates tumor progression through epithelial-mesenchymal transition in uveal melanoma. Front. Immunol. 13. doi: 10.3389/fimmu.2022.754645
Meng, X., Liu, P., Wu, Y., Liu, X., Huang, Y., Yu, B., et al. (2020). Integrin beta 4 (ITGB4) and its tyrosine-1510 phosphorylation promote pancreatic tumorigenesis and regulate the MEK1-ERK1/2 signaling pathway. Bosn J. Basic Med. Sci. 20, 106–116. doi: 10.17305/bjbms.2019.4255
Meng, N.-L., Wang, Y., Wang, H.-L., Zhou, J.-L., Wang, S. (2022a). Research on the histological features and pathological types of gastric adenocarcinoma with mucinous differentiation. Front. Med. 9. doi: 10.3389/fmed.2022.829702
Moreno, C. S. (2020). SOX4: The unappreciated oncogene. Semin. Cancer Biol. 67, 57–64. doi: 10.1016/j.semcancer.2019.08.027
Mukherjee, A., Ye, Y., Wiener, H. W., Kuniholm, M. H., Minkoff, H., Michel, K., et al. (2023). Variations in genes encoding human papillomavirus binding receptors and susceptibility to cervical precancer. Cancer Epidemiol. Biomarkers Prev. 32, 1190–1197. doi: 10.1158/1055-9965.EPI-23-0300
Münz, C. (2019). Latency and lytic replication in Epstein-Barr virus-associated oncogenesis. Nat. Rev. Microbiol. 17, 691–700. doi: 10.1038/s41579-019-0249-7
Nagase, Y., Hiramatsu, K., Funauchi, M., Shiomi, M., Masuda, T., Kakuda, M., et al. (2022). Anti-lipolysis-stimulated lipoprotein receptor monoclonal antibody as a novel therapeutic agent for endometrial cancer. BMC Cancer 22, 679. doi: 10.1186/s12885-022-09789-6
Najjar, I., Baran-Marszak, F., Le Clorennec, C., Laguillier, C., Schischmanoff, O., Youlyouz-Marfak, I., et al. (2005). Latent membrane protein 1 regulates STAT1 through NF-kappaB-dependent interferon secretion in Epstein-Barr virus-immortalized B cells. J. Virol. 79, 4936–4943. doi: 10.1128/JVI.79.8.4936-4943.2005
Nejmeddine, M., Negi, V. S., Mukherjee, S., Tanaka, Y., Orth, K., Taylor, G. P., et al. (2009). HTLV-1–Tax and ICAM-1 act on T-cell signal pathways to polarize the microtubule-organizing center at the virological synapse. Blood 114, 1016–1025. doi: 10.1182/blood-2008-03-136770
Nguyen, N. N. T., Lim, Y.-S., Nguyen, L. P., Tran, S. C., Luong, T. T. D., Nguyen, T. T. T., et al. (2018). Hepatitis C Virus Modulates Solute carrier family 3 member 2 for Viral Propagation. Sci. Rep. 8, 15486. doi: 10.1038/s41598-018-33861-6
Nicolle, R., Radvanyi, F., Elati, M. (2015). CoRegNet: Reconstruction and integrated analysis of co-regulatory networks. Bioinformatics 31, 3066–3068. doi: 10.1093/bioinformatics/btv305
Osorio, J. C., Blanco, R., Corvalán, A. H., Muñoz, J. P., Calaf, G. M., Aguayo, F. (2022a). Epstein-barr virus infection in lung cancer: insights and perspectives. Pathogens 11. doi: 10.3390/pathogens11020132
Osorio, J. C., Candia-Escobar, F., Corvalán, A. H., Calaf, G. M., Aguayo, F. (2022b). High-risk human papillomavirus infection in lung cancer: mechanisms and perspectives. Biol. (Basel) 11. doi: 10.3390/biology11121691
Otálora-Otálora, B. A., Florez, M., López-Kleine, L., Canas Arboleda, A., Grajales Urrego, D. M., Rojas, A. (2019). Joint transcriptomic analysis of lung cancer and other lung diseases. Front. Genet. 10. doi: 10.3389/fgene.2019.01260
Otálora-Otálora, B. A., González Prieto, C., Guerrero, L., Bernal-Forigua, C., Montecino, M., Cañas, A., et al. (2022a). Identification of the transcriptional regulatory role of RUNX2 by network analysis in lung cancer cells. Biomedicines 10, 1–18. doi: 10.3390/biomedicines10123122
Otálora-Otálora, B. A., López-Kleine, L., Rojas, A. (2023a). Lung cancer gene regulatory network of transcription factors related to the hallmarks of cancer. Curr. Issues Mol. Biol. 45, 434–464. doi: 10.3390/cimb45010029
Otálora-Otálora, B. A., López-Rivera, J. J., Aristizábal-Guzmán, C., Isaza-Ruget, M. A., Álvarez-Moreno, C. A. (2023b). Host transcriptional regulatory genes and microbiome networks crosstalk through immune receptors establishing normal and tumor multiomics metafirm of the oral-gut-lung axis. Int. J. Mol. Sci. 24. doi: 10.3390/ijms242316638
Otálora-Otálora, B. A., Osuna-Garzón, D. A., Carvajal-Parra, M. S., Cañas, A., Montecino, M., López-Kleine, L., et al. (2022b). Identifying general tumor and specific lung cancer biomarkers by transcriptomic analysis. Biol. (Basel) 11. doi: 10.3390/biology11071082
Otto, T., Horn, S., Brockmann, M., Eilers, U., Schüttrumpf, L., Popov, N., et al. (2009). Stabilization of N-Myc is a critical function of Aurora A in human neuroblastoma. Cancer Cell 15, 67–78. doi: 10.1016/j.ccr.2008.12.005
Ozbun, M. A., Campos, S. K. (2021). The long and winding road: human papillomavirus entry and subcellular trafficking. Curr. Opin. Virol. 50, 76–86. doi: 10.1016/j.coviro.2021.07.010
Pagliuca, A., Gallo, P., De Luca, P., Lania, L. (2000). Class A helix-loop-helix proteins are positive regulators of several cyclin-dependent kinase inhibitors’ promoter activity and negatively affect cell growth. Cancer Res. 60, 1376–1382.
Park, H. U., Jeong, J.-H., Chung, J. H., Brady, J. N. (2004). Human T-cell leukemia virus type 1 Tax interacts with Chk1 and attenuates DNA-damage induced G2 arrest mediated by Chk1. Oncogene 23, 4966–4974. doi: 10.1038/sj.onc.1207644
Park, S.-H., Lim, J. S., Lim, S.-Y., Tiwari, I., Jang, K. L. (2011). Hepatitis C virus Core protein stimulates cell growth by down-regulating p16 expression via DNA methylation. Cancer Lett. 310, 61–68. doi: 10.1016/j.canlet.2011.06.012
Peinado, H., Marin, F., Cubillo, E., Stark, H.-J., Fusenig, N., Nieto, M. A., et al. (2004). Snail and E47 repressors of E-cadherin induce distinct invasive and angiogenic properties in vivo. J. Cell Sci. 117, 2827–2839. doi: 10.1242/jcs.01145
Perez-Moreno, M. A., Locascio, A., Rodrigo, I., Dhondt, G., Portillo, F., Nieto, M. A., et al. (2001). A new role for E12/E47 in the repression of E-cadherin expression and epithelial-mesenchymal transitions. J. Biol. Chem. 276, 27424–27431. doi: 10.1074/jbc.M100827200
Prelli Bozzo, C., Nchioua, R., Volcic, M., Koepke, L., Krüger, J., Schütz, D., et al. (2021). IFITM proteins promote SARS-CoV-2 infection and are targets for virus inhibition in vitro. Nat. Commun. 12, 4584. doi: 10.1038/s41467-021-24817-y
Qi, D., Lu, M., Xu, P., Yao, X., Chen, Y., Gan, L., et al. (2023). Transcription factor ETV4 promotes the development of hepatocellular carcinoma by driving hepatic TNF-α signaling. Cancer Commun. (Lond) 43, 1354–1372. doi: 10.1002/cac2.12482
Raj, S., Kesari, K. K., Kumar, A., Rathi, B., Sharma, A., Gupta, P. K., et al. (2022). Molecular mechanism(s) of regulation(s) of c-MET/HGF signaling in head and neck cancer. Mol. Cancer 21, 31. doi: 10.1186/s12943-022-01503-1
Rather, T. B., Parveiz, I., Bhat, G. A., Rashid, G., Wani, R. A., Khan, I. Y., et al. (2023). Evaluation of Forkhead BOX M1 (FOXM1) gene expression in colorectal cancer. Clin. Exp. Med. 23, 2385–2405. doi: 10.1007/s10238-022-00929-7
Rawla, P., Sunkara, T., Barsouk, A. (2019). Epidemiology of colorectal cancer: incidence, mortality, survival, and risk factors. Prz Gastroenterol. 14, 89–103. doi: 10.5114/pg.2018.81072
Ray, U., Pathoulas, C. L., Thirusangu, P., Purcell, J. W., Kannan, N., Shridhar, V. (2022). Exploiting LRRC15 as a novel therapeutic target in cancer. Cancer Res. 82, 1675–1681. doi: 10.1158/0008-5472.CAN-21-3734
Ray, R. B., Ray, R. (2001). Hepatitis C virus core protein: intriguing properties and functional relevance. FEMS Microbiol. Lett. 202, 149–156. doi: 10.1111/fml.2001.202.issue-2
Richardson, C., Fielding, C., Rowe, M., Brennan, P. (2003). Epstein-Barr virus regulates STAT1 through latent membrane protein 1. J. Virol. 77, 4439–4443. doi: 10.1128/JVI.77.7.4439-4443.2003
Ritchie, M. E., Phipson, B., Wu, D., Hu, Y., Law, C. W., Shi, W., et al. (2015). limma powers differential expression analyses for RNA-sequencing and microarray studies. Nucleic Acids Res. 43, e47–e47. doi: 10.1093/nar/gkv007
Romeo, M. M., Ko, B., Kim, J., Brady, R., Heatley, H. C., He, J., et al. (2015). Acetylation of the c-MYC oncoprotein is required for cooperation with the HTLV-1 p30(II) accessory protein and the induction of oncogenic cellular transformation by p30(II)/c-MYC. Virology 476, 271–288. doi: 10.1016/j.virol.2014.12.008
Ruan, Y., Chen, L., Xie, D., Luo, T., Xu, Y., Ye, T., et al. (2022). Mechanisms of cell adhesion molecules in endocrine-related cancers: A concise outlook. Front. Endocrinol. 13. doi: 10.3389/fendo.2022.865436
Saha, A., Halder, S., Upadhyay, S. K., Lu, J., Kumar, P., Murakami, M., et al. (2011). Epstein-barr virus nuclear antigen 3C facilitates G1-S transition by stabilizing and enhancing the function of cyclin D1. PloS Pathog. 7, e1001275. doi: 10.1371/journal.ppat.1001275
Salmani-Javan, E., Jadid, M. F. S., Zarghami, N. (2024). Recent advances in molecular targeted therapy of lung cancer: Possible application in translation medicine. Iran J. Basic Med. Sci. 27, 122–133. doi: 10.22038/IJBMS.2023.72407.15749
Sato, S., Vasaikar, S., Eskaros, A., Kim, Y., Lewis, J. S., Zhang, B., et al. (2019). EPHB2 carried on small extracellular vesicles induces tumor angiogenesis via activation of ephrin reverse signaling. JCI Insight 4. doi: 10.1172/jci.insight.132447
Scalise, M., Galluccio, M., Console, L., Pochini, L., Indiveri, C. (2018). The human SLC7A5 (LAT1): the intriguing histidine/large neutral amino acid transporter and its relevance to human health. Front. Chem. 6. doi: 10.3389/fchem.2018.00243
Schaefer, C. J., Kossen, K., Lim, S. R., Lin, J.-H., Pan, L., Bradford, W., et al. (2011). Danoprevir monotherapy decreases inflammatory markers in patients with chronic hepatitis C virus infection. Antimicrob. Agents Chemother. 55, 3125–3132. doi: 10.1128/AAC.00131-11
Schuurbiers, O. C. J., Meijer, T. W. H., Kaanders, J. H. A. M., Looijen-Salamon, M. G., de Geus-Oei, L.-F., van der Drift, M. A., et al. (2014). Glucose metabolism in NSCLC is histology-specific and diverges the prognostic potential of 18FDG-PET for adenocarcinoma and squamous cell carcinoma. J. Thorac. Oncol. 9, 1485–1493. doi: 10.1097/JTO.0000000000000286
Scott, M. L., Coleman, D. T., Kelly, K. C., Carroll, J. L., Woodby, B., Songock, W. K., et al. (2018). Human papillomavirus type 16 E5-mediated upregulation of Met in human keratinocytes. Virology 519, 1–11. doi: 10.1016/j.virol.2018.03.021
Shan, G., Minchao, K., Jizhao, W., Rui, Z., Guangjian, Z., Jin, Z., et al. (2023). Resveratrol improves the cytotoxic effect of CD8 +T cells in the tumor microenvironment by regulating HMMR/Ferroptosis in lung squamous cell carcinoma. J. Pharm. BioMed. Anal. 229, 115346. doi: 10.1016/j.jpba.2023.115346
She, Q.-B., Halilovic, E., Ye, Q., Zhen, W., Shirasawa, S., Sasazuki, T., et al. (2010). 4E-BP1 is a key effector of the oncogenic activation of the AKT and ERK signaling pathways that integrates their function in tumors. Cancer Cell 18, 39–51. doi: 10.1016/j.ccr.2010.05.023
Sherman, B. T., Hao, M., Qiu, J., Jiao, X., Baseler, M. W., Lane, H. C., et al. (2022). DAVID: a web server for functional enrichment analysis and functional annotation of gene list, (2021 update). Nucleic Acids Res. 50, W216–W221. doi: 10.1093/nar/gkac194
Sheu, L. F., Chen, A., Wei, Y. H., Ho, K. C., Cheng, J. Y., Meng, C. L., et al. (1998). Epstein-Barr virus LMP1 modulates the Malignant potential of gastric carcinoma cells involving apoptosis. Am. J. Pathol. 152, 63–74.
Shi, Q., Jiang, J., Luo, G. (2013). Syndecan-1 serves as the major receptor for attachment of hepatitis C virus to the surfaces of hepatocytes. J. Virol. 87, 6866–6875. doi: 10.1128/JVI.03475-12
Shi, G., Kenney, A. D., Kudryashova, E., Zani, A., Zhang, L., Lai, K. K., et al. (2021). Opposing activities of IFITM proteins in SARS-CoV-2 infection. EMBO J. 40, e106501. doi: 10.15252/embj.2020106501
Shirley, D.-A., Hung, C.-C., Moonah, S. (2020). “94 - entamoeba histolytica (Amebiasis),”. Eds. Ryan, E. T., Hill, D. R., Solomon, T., Aronson, N. E., T., P. B. T.-H. T. M., E., I. D. Hunter's tropical medicine and emerging infectious diseases (Elsevier, London), 699–706. doi: 10.1016/B978-0-323-55512-8.00094-6
Siegel, R. L., Miller, K. D., Wagle, N. S., Jemal, A. (2023). Cancer statistic. CA Cancer J. Clin. 73, 17–48. doi: 10.3322/caac.21763
Smith, A. C., Cirulis, J. T., Casanova, J. E., Scidmore, M. A., Brumell, J. H. (2005). Interaction of the Salmonella-containing vacuole with the endocytic recycling system. J. Biol. Chem. 280, 24634–24641. doi: 10.1074/jbc.M500358200
So, C.-W., Sourisseau, M., Sarwar, S., Evans, M. J., Randall, G. (2023). Roles of epidermal growth factor receptor, claudin-1 and occludin in multi-step entry of hepatitis C virus into polarized hepatoma spheroids. PloS Pathog. 19, e1011887. doi: 10.1371/journal.ppat.1011887
Somiya, M., Liu, Q., Yoshimoto, N., Iijima, M., Tatematsu, K., Nakai, T., et al. (2016). Cellular uptake of hepatitis B virus envelope L particles is independent of sodium taurocholate cotransporting polypeptide, but dependent on heparan sulfate proteoglycan. Virology 497, 23–32. doi: 10.1016/j.virol.2016.06.024
Spanò, S., Liu, X., Galán, J. E. (2011). Proteolytic targeting of Rab29 by an effector protein distinguishes the intracellular compartments of human-adapted and broad-host Salmonella. Proc. Natl. Acad. Sci. U.S.A. 108, 18418–18423. doi: 10.1073/pnas.1111959108
Starita, L. M., Machida, Y., Sankaran, S., Elias, J. E., Griffin, K., Schlegel, B. P., et al. (2004). BRCA1-dependent ubiquitination of γ-tubulin regulates centrosome number. Mol. Cell Biol. 24, 8457–8466. doi: 10.1128/MCB.24.19.8457-8466.2004
Taguchi, T., Nagano-Fujii, M., Akutsu, M., Kadoya, H., Ohgimoto, S., Ishido, S., et al. (2004). Hepatitis C virus NS5A protein interacts with 2’,5’-oligoadenylate synthetase and inhibits antiviral activity of IFN in an IFN sensitivity-determining region-independent manner. J. Gen. Virol. 85, 959–969. doi: 10.1099/vir.0.19513-0
Takenaka, R., Okada, H., Kato, J., Makidono, C., Hori, S., Kawahara, Y., et al. (2007). Helicobacter pylori eradication reduced the incidence of gastric cancer, especially of the intestinal type. Aliment Pharmacol. Ther. 25, 805–812. doi: 10.1111/j.1365-2036.2007.03268.x
Talamás-Rohana, P., Hernández-Ramirez, V. I., Perez-García, J. N., Ventura-Juárez, J. (1998). Entamoeba histolytica contains a beta 1 integrin-like molecule similar to fibronectin receptors from eukaryotic cells. J. Eukaryot Microbiol. 45, 356–360. doi: 10.1111/j.1550-7408.1998.tb04549.x
Tang, Q., Liu, C., Zhang, S., He, L., Liu, Y., Wang, J., et al. (2023). FOXM1 increases hTERT protein stability and indicates poor prognosis in gastric cancer. Neoplasia (United States) 36. doi: 10.1016/j.neo.2022.100863
Tang, Y., Luo, J., Yang, Y., Liu, S., Zheng, H., Zhan, Y., et al. (2022). Overexpression of p-4EBP1 associates with p-eIF4E and predicts poor prognosis for non-small cell lung cancer patients with resection. PloS One 17, e0265465. doi: 10.1371/journal.pone.0265465
Tavakolian, S., Goudarzi, H., Faghihloo, E. (2020). Cyclin-dependent kinases and CDK inhibitors in virus-associated cancers. Infect. Agent Cancer 15, 27. doi: 10.1186/s13027-020-00295-7
van Gent, M., Braem, S. G. E., de Jong, A., Delagic, N., Peeters, J. G. C., Boer, I. G. J., et al. (2014). Epstein-Barr virus large tegument protein BPLF1 contributes to innate immune evasion through interference with toll-like receptor signaling. PloS Pathog. 10, e1003960. doi: 10.1371/journal.ppat.1003960
Wallis, T. S., Galyov, E. E. (2000). Molecular basis of Salmonella-induced enteritis. Mol. Microbiol. 36, 997–1005. doi: 10.1046/j.1365-2958.2000.01892.x
Wang, Y. (2017). Transcriptional regulatory network analysis for gastric cancer based on mRNA microarray. Pathol. Oncol. Res. 23, 785–791. doi: 10.1007/s12253-016-0159-1
Wang, Y., Ding, X., Liu, B., Li, M., Chang, Y., Shen, H., et al. (2020). ETV4 overexpression promotes progression of non–small cell lung cancer by upregulating PXN and MMP1 transcriptionally. Mol. Carcinog 59, 73–86. doi: 10.1002/mc.23130
Wang, J.-T., Doong, S.-L., Teng, S.-C., Lee, C.-P., Tsai, C.-H., Chen, M.-R. (2009). Epstein-Barr virus BGLF4 kinase suppresses the interferon regulatory factor 3 signaling pathway. J. Virol. 83, 1856–1869. doi: 10.1128/JVI.01099-08
Wang, R., Gao, Y., Zhang, H. (2023). ACTN1 interacts with ITGA5 to promote cell proliferation, invasion and epithelial-mesenchymal transformation in head and neck squamous cell carcinoma. Iran J. Basic Med. Sci. 26, 200–207. doi: 10.22038/IJBMS.2022.67056.14703
Wang, D., Hao, T., Pan, Y., Qian, X., Zhou, D. (2015). Increased expression of SOX4 is a biomarker for Malignant status and poor prognosis in patients with non-small cell lung cancer. Mol. Cell Biochem. 402, 75–82. doi: 10.1007/s11010-014-2315-9
Wang, Z., Tang, Y., Xie, L., Huang, A., Xue, C., Gu, Z., et al. (2019). The prognostic and clinical value of CD44 in colorectal cancer: A meta-analysis. Front. Oncol. 9. doi: 10.3389/fonc.2019.00309
Weidemüller, P., Kholmatov, M., Petsalaki, E., Zaugg, J. B. (2021). Transcription factors: Bridge between cell signaling and gene regulation. Proteomics 21, 1–14. doi: 10.1002/pmic.202000034
Westrich, J. A., Warren, C. J., Pyeon, D. (2017). Evasion of host immune defenses by human papillomavirus. Virus Res. 231, 21–33. doi: 10.1016/j.virusres.2016.11.023
Whiteside, S. T., Goodbourn, S. (1993). Signal transduction and nuclear targeting: regulation of transcription factor activity by subcellular localisation. J. Cell Sci. 104, 949–955. doi: 10.1242/jcs.104.4.949
Wong, M. H., Huelsken, J., Birchmeier, W., Gordon, J. I. (2002). Selection of multipotent stem cells during morphogenesis of small intestinal crypts of Lieberkuhn is perturbed by stimulation of Lef-1/beta-catenin signaling. J. Biol. Chem. 277, 15843–15850. doi: 10.1074/jbc.M200184200
Wu, X., Lin, H., Li, S. (2019). Prognoses of different pathological subtypes of colorectal cancer at different stages: A population-based retrospective cohort study. BMC Gastroenterol. 19, 1–8. doi: 10.1186/s12876-019-1083-0
Xie, L., Yan, J. (2023). γ-tocotrienol regulates gastric cancer by targeting notch signaling pathway. Hereditas 160, 15. doi: 10.1186/s41065-023-00277-w
Xu, J., Chen, T., Liao, L., Chen, T., Li, Q., Xu, J., et al. (2021a). NETO2 promotes esophageal cancer progression by inducing proliferation and metastasis via PI3K/AKT and ERK pathway. Int. J. Biol. Sci. 17, 259–270. doi: 10.7150/ijbs.53795
Xu, S., Zhang, T., Cao, Z., Zhong, W., Zhang, C., Li, H., et al. (2021b). Integrin-α9β1 as a novel therapeutic target for refractory diseases: recent progress and insights. Front. Immunol. 12. doi: 10.3389/fimmu.2021.638400
Xu, L., Zhou, R., Yuan, L., Wang, S., Li, X., Ma, H., et al. (2017). IGF1/IGF1R/STAT3 signaling-inducible IFITM2 promotes gastric cancer growth and metastasis. Cancer Lett. 393, 76–85. doi: 10.1016/j.canlet.2017.02.014
Yang, D., Hendifar, A., Lenz, C., Togawa, K., Lenz, F., Lurje, G., et al. (2011). Survival of metastatic gastric cancer: Significance of age, sex and race/ethnicity. J. Gastrointest Oncol. 2, 77–84. doi: 10.3978/j.issn.2078-6891.2010.025
Yeh, M.-H., Tzeng, Y.-J., Fu, T.-Y., You, J.-J., Chang, H.-T., Ger, L.-P., et al. (2018). Extracellular matrix–receptor interaction signaling genes associated with inferior breast cancer survival. Anticancer Res. 38, 4593 LP–4605. doi: 10.21873/anticanres.12764
Yen, F. T., Roitel, O., Bonnard, L., Notet, V., Pratte, D., Stenger, C., et al. (2008). Lipolysis stimulated lipoprotein receptor: A NOVEL MOLECULAR LINK BETWEEN HYPERLIPIDEMIA, WEIGHT GAIN, AND ATHEROSCLEROSIS IN MICE *. J. Biol. Chem. 283, 25650–25659. doi: 10.1074/jbc.M801027200
Zhan, L., Wu, W., Yang, Q., Shen, H., Liu, L., Kang, R. (2023). Transcription factor TEAD4 facilitates glycolysis and proliferation of gastric cancer cells by activating PKMYT1. Mol. Cell Probes 72, 101932. doi: 10.1016/j.mcp.2023.101932
Zhang, Q.-Y., Chen, X.-Q., Liu, X.-C., Wu, D.-M. (2020). PKMYT1 promotes gastric cancer cell proliferation and apoptosis resistance. Onco Targets Ther. 13, 7747–7757. doi: 10.2147/OTT.S255746
Zhang, J., Feng, H., Xu, S., Feng, P. (2016). Hijacking GPCRs by viral pathogens and tumor. Biochem. Pharmacol. 114, 69–81. doi: 10.1016/j.bcp.2016.03.021
Zhang, S., Li, M., Ji, H., Fang, Z. (2018). Landscape of transcriptional deregulation in lung cancer. BMC Genomics 19. doi: 10.1186/s12864-018-4828-1
Zhang, M., Ma, C. (2021). LSR promotes cell proliferation and invasion in lung cancer. Comput. Math Methods Med. 2021, 6651907. doi: 10.1155/2021/6651907
Zhang, Y., Vaccarella, S., Morgan, E., Li, M., Etxeberria, J., Chokunonga, E., et al. (2023). Global variations in lung cancer incidence by histological subtype in 2020: a population-based study. Lancet Oncol. 24, 1206–1218. doi: 10.1016/S1470-2045(23)00444-8
Zhang, B., Xie, Z., Li, B. (2019). The clinicopathologic impacts and prognostic significance of GLUT1 expression in patients with lung cancer: A meta-analysis. Gene 689, 76–83. doi: 10.1016/j.gene.2018.12.006
Zhang, J., Zhang, J., Cui, X., Yang, Y., Li, M., Qu, J., et al. (2015). FoxM1: a novel tumor biomarker of lung cancer. Available online at: www.ijcem.com/.
Zhao, H., Ming, T., Tang, S., Ren, S., Yang, H., Liu, M., et al. (2022). Wnt signaling in colorectal cancer: pathogenic role and therapeutic target. Mol. Cancer 21, 144. doi: 10.1186/s12943-022-01616-7
Zhao, X., Sehgal, M., Hou, Z., Cheng, J., Shu, S., Wu, S., et al. (2018). Identification of residues controlling restriction versus enhancing activities of IFITM proteins on entry of human coronaviruses. J. Virol. 92. doi: 10.1128/JVI.01535-17
Zhao, L., Wang, L., Zhang, C., Liu, Z., Piao, Y., Yan, J., et al. (2019). E6-induced selective translation of WNT4 and JIP2 promotes the progression of cervical cancer via a noncanonical WNT signaling pathway. Signal Transduct Target Ther. 4, 32. doi: 10.1038/s41392-019-0060-y
Zhao, X., Wu, S., Jing, J. (2021). Identifying diagnostic and prognostic biomarkers and candidate therapeutic drugs of gastric cancer based on transcriptomics and single-cell sequencing. Pathol. Oncol. Res. 27. doi: 10.3389/pore.2021.1609955
Zhou, X. H., Chu, X. Y., Xue, G., Xiong, J. H., Zhang, H. Y. (2019). Identifying cancer prognostic modules by module network analysis. BMC Bioinf. 20. doi: 10.1186/s12859-019-2674-z
Keywords: transcription factors, transcriptional regulatory network, cancer-related cells membrane receptors, microbiota, hallmarks of cancer, oral-gut-lung axis, gut-lung cancer
Citation: Otálora-Otálora BA, Payán-Gómez C, López-Rivera JJ, Pedroza-Aconcha NB, Aristizábal-Guzmán C, Isaza-Ruget MA and Álvarez-Moreno CA (2024) Global transcriptomic network analysis of the crosstalk between microbiota and cancer-related cells in the oral-gut-lung axis. Front. Cell. Infect. Microbiol. 14:1425388. doi: 10.3389/fcimb.2024.1425388
Received: 29 April 2024; Accepted: 15 July 2024;
Published: 20 August 2024.
Edited by:
Maurizio Sanguinetti, Catholic University of the Sacred Heart, ItalyReviewed by:
Neha Nanda, Harvard Medical School, United StatesLauren Ford, Imperial College London, United Kingdom
Copyright © 2024 Otálora-Otálora, Payán-Gómez, López-Rivera, Pedroza-Aconcha, Aristizábal-Guzmán, Isaza-Ruget and Álvarez-Moreno. This is an open-access article distributed under the terms of the Creative Commons Attribution License (CC BY). The use, distribution or reproduction in other forums is permitted, provided the original author(s) and the copyright owner(s) are credited and that the original publication in this journal is cited, in accordance with accepted academic practice. No use, distribution or reproduction is permitted which does not comply with these terms.
*Correspondence: Beatriz Andrea Otálora-Otálora, YmFvdGFsb3Jhb3RAdW5pc2FuaXRhcy5lZHUuY28=