- Department of Veterinary Biosciences, The Ohio State University, Columbus, OH, United States
Ehrlichiosis is a potentially life-threatening disease caused by infection with the obligatory intracellular bacteria Ehrlichia species. Ehrlichia japonica infection of mice provides an animal model of ehrlichiosis as it recapitulates full-spectrum and lethal ehrlichiosis in humans. The E. japonica transposon mutant of EHF0962, which encodes a previously uncharacterized hypothetical protein, is attenuated in both infection and virulence in mice. EHF0962 was hence named here as resistance-inducing protein of Ehrlichia (RipE). Using this ΔripE mutant, we studied how RipE protein contributes to Ehrlichia pathogenesis. Ehrlichia species have an intracellular developmental cycle and a brief extracellular stage to initiate a new cycle of infection. Majority of RipE proteins were expressed on the surface of the smaller infectious dense-core stage of bacteria. Extracellular ΔripE E. japonica contained significantly less adenosine triphosphate (ATP) and lost infectivity more rapidly in culture compared with wild-type (WT) E. japonica. Genetic complementation in the ΔripE mutant or overexpression of ripE in WT E. japonica significantly increased bacterial ATP levels, and RipE-overexpressing E. japonica was more virulent in mice than WT E. japonica. RipE is conserved among Ehrlichia species. Immunization of mice with recombinant RipE induced an in vitro infection-neutralizing antibody, significantly prolonged survival time after a lethal dose of E. japonica challenge, and cross-protected mice from infection by Ehrlichia chaffeensis, the agent of human monocytic ehrlichiosis. Our findings shed light on the extracellular stage of Ehrlichia, highlighting the importance of RipE and ATP levels in Ehrlichia for extracellular resistance and the next cycle of infection. Thus, RipE is a critical Ehrlichia protein for infection as such can be a potential vaccine target for ehrlichiosis.
Introduction
The family Anaplasmataceae consists of a group of Gram-negative, obligate intracellular alpha-proteobacteria that cause nonspecific febrile diseases in susceptible animals and humans (Rikihisa, 1991; Dumler et al., 2001; Rikihisa, 2010). Although the diseases are usually self-limiting in humans, severe illness can occur upon infection with Ehrlichia chaffeensis or Anaplasma phagocytophilum, with some cases proven fatal (Bakken and Dumler, 2000). Notably, the Centers for Disease Control and Prevention must be notified for every infection case, which shows that disease incidence has increased significantly in the past two decades (CDC, 2024). E. chaffeensis causes the systemic disease human monocytic ehrlichiosis (HME), for which doxycycline is the only treatment (CDC, 2024). To facilitate current efforts to develop an HME vaccine (McGill et al., 2016; Thomas, 2016; Budachetri et al., 2020, Budachetri et al., 2022), more research is needed to understand HME pathogenesis and to discover effective therapeutic and vaccine targets.
An ehrlichial species was recently characterized by whole-genome sequencing and classified as Ehrlichia japonica sp. nov (Lin et al., 2021; Oren and Garrity, 2022). This species was originally isolated from Ixodes ovatus ticks in Japan and was previously known as an I. ovatus ehrlichia (IOE) agent or Ehrlichia sp. HF strain. Phylogenetic analysis revealed that E. japonica is closely related to E. chaffeensis based on 16S rRNA and GroEL protein sequences (Shibata et al., 2000). Laboratory mice challenged with E. japonica develop an acute systemic infection followed by death that resembles severe HME (Shibata et al., 2000; Sotomayor et al., 2001; Zhang et al., 2024). Thus, over the past several years, E. japonica infection in immunocompetent mice has been increasingly used as a model for studying HME (Shibata et al., 2000; Okada et al., 2001, Okada et al., 2003; Winslow et al., 2005; Ismail et al., 2006; Habib et al., 2016; Haloul et al., 2019; Ahmed and Ismail, 2020; Ismail et al., 2022; Sharma et al., 2023). As E. japonica causes a dose-dependent, full-spectrum disease with an LD50 of approximately 100 bacteria (Bekebrede et al., 2020), this mouse model provides an excellent way to investigate ehrlichiosis pathogenesis in vivo and to bridge the knowledge gap between E. chaffeensis infection and severe HME.
To overcome the mammalian immune system, establish infection, and cause disease within the host, Ehrlichia must utilize additional strategies, such as in vivo virulence factors, beyond those required for infection of eukaryotic cells in culture (Rikihisa, 2021). However, the vast majority of Ehrlichia virulence factors and their functions in vivo remain undetermined. According to recently published whole-genome sequencing data, E. japonica contains 866 protein-coding genes (Lin et al., 2021). Besides those genes essential for protein or nucleotide biosynthesis and key metabolic pathways, the functions of 244 proteins remain unknown and hence such proteins are annotated as hypothetical proteins. Because Ehrlichia spp. and other members of the family Anaplasmataceae have evolved to have a relatively small genome (Dunning Hotopp et al., 2006; Rikihisa, 2015), it is very likely that at least some, if not all, of these hypothetical proteins could have played key roles in the evolutionary success of Ehrlichia spp. To investigate the functions of these unknown Ehrlichia genes, we used a Himar1 transposon random mutagenesis system (Felsheim et al., 2006) to create a library of E. japonica that comprises at least 158 distinct mutants, carrying genetic mutations at different loci (Bekebrede et al., 2020). Among the mutants that can replicate well in cell culture but are attenuated in mouse virulence is the H59, a clone of a Himar1 insertional mutant disrupting EHF_0962 gene (EHF_0962::Himar1), which caused significantly lower bacterial loads in the blood, liver, and spleen with reduced clinical signs at day 7 post-infection (pi) (Bekebrede et al., 2020). EHF_0962 encodes a previously uncharacterized hypothetical protein with 120 amino acid residues (molecular mass, ~13.5 kDa, pI 5.78, GenBank accession no. WP_052349286.1) (Lin et al., 2021), which was named in this study as resistance-inducing protein of Ehrlichia (RipE). Here, we characterized RipE functions in E. japonica pathogenesis in mice. Our results suggest that RipE helps bacteria survival during the extracellular stage of Ehrlichia, thereby facilitating in vivo infection.
Results
Characteristics of the ΔripE mutant and RipE
To study the functions of RipE in E. japonica pathogenesis, we first confirmed the stable clonality of the ΔripE mutant H59 (Bekebrede et al., 2020). Himar1 insertion site-specific flanking PCR for ripE (Supplementary Table S1) (Bekebrede et al., 2020) showed that the ΔripE mutant produced a single, larger PCR product containing the Himar1 insert (2,254 bp) compared with WT E. japonica (421 bp, Figures 1A, B). Himar1 insertions occur mostly once and rarely twice per genome (Cartman and Minton, 2010; Cain et al., 2020); therefore, to verify that there were no additional Himar1 insertions in the ΔripE mutant genome, quantitative PCR (qPCR) was performed, and the results showed a ratio of 1:1 for the copy number of the mCherry gene vs. the 16S rRNA gene, which is a single copy per Ehrlichia genome (Figure 1C). Wild-type (WT) E. japonica and the ΔripE mutant had similar growth curves when cultured in DH82 canine macrophages or ISE6 tick cells (Bekebrede et al., 2020). The intracellular micro-colonies (morulae) formed by WT and ΔripE E. japonica are essentially indistinguishable by HEMA3 staining (Bekebrede et al., 2020) (Figure 1D), whereas the ΔripE mutant could be easily distinguished from WT E. japonica owing to its mCherry fluorescence under a fluorescence microscope (Figure 1D). To investigate whether the ripE gene is involved in mammalian endothelial tropism and infection, we also compared the growth curves of WT and ΔripE E. japonica in the RF/6A rhesus monkey endothelial cell line, but no significant difference was observed (Supplementary Figure S1).
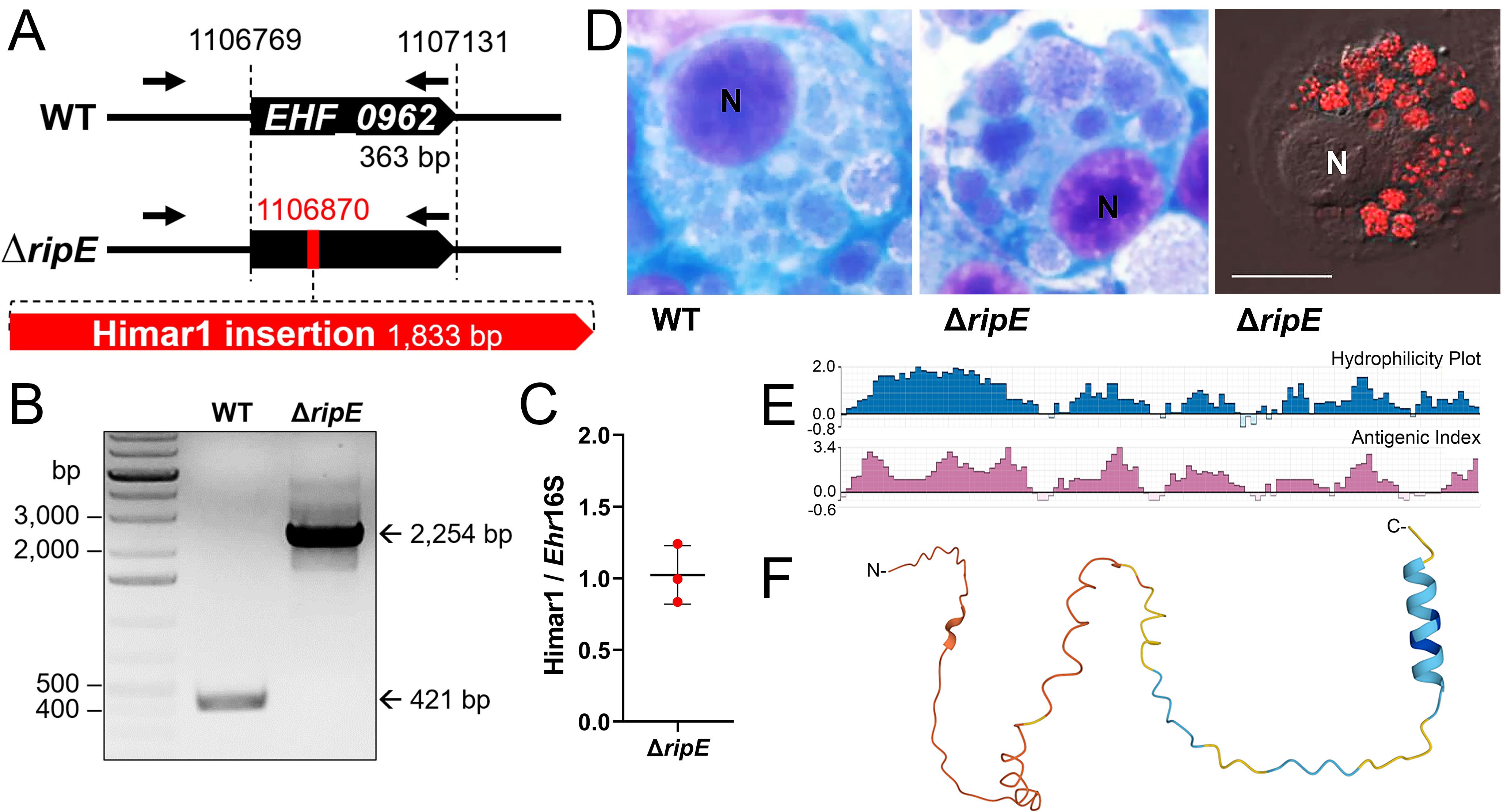
Figure 1. Clonality of ΔripE E. japonica and bioinformatic analysis of RipE protein. (A) Diagram of E. japonica genome showing ΔripE with Himar1 transposon insertion in locus EHF_0962 (ripE). GenBank Reference Sequence number: NZ_CP007474.1. ORFs (black pentagon arrows). Himar1 insertion containing spectinomycin/streptomycin resistance gene and mCherry (red pentagon arrow). ripE-specific flanking PCR primers (arrows). (B) ripE-specific flanking PCR for ΔripE E. japonica clonality verification. (C) Copy numbers of the mCherry gene vs. the single-copy Ehrlichia 16S rRNA gene in ΔripE E. japonica genome determined by qPCR from three different batches of cultures. (D) WT and ΔripE E. japonica cultured in DH82 cells. Note the characteristic Ehrlichia micro-colonies (morulae) by HEMA3 stain in the cytoplasm of infected DH82 cells, and ΔripE mutants were also detected by mCherry fluorescence using a Leica Thunder fluorescence microscope. N, nucleus. Scale bar, 10 µm. (E) Antigenic index and hydrophilicity plot of RipE by Protean prediction. (F) RipE protein 3D structure prediction by AlphaFold.
Protein Blast search revealed that RipE homologs were identified in all sequenced Ehrlichia spp., including E. muris, E. chaffeensis, E. canis, and E. ruminantium (Table 1). However, no RipE homologs could be found beyond the genus Ehrlichia, even in other closely related members in the family Anaplasmataceae such as Anaplasma and Neorickettsia spp., suggesting that RipE is a unique protein that evolved among Ehrlichia spp. As RipE lacks any known motifs cataloged in the NCBI database, bioinformatic analyses were performed to determine its secondary-structure characteristics. Analysis using Protean (DNASTAR Lasergene) revealed that RipE consists of six hydrophilic regions each with a high antigenic index intervened with short hydrophobic linkers (Figure 1E). Three-dimensional structure prediction based on AlphaFold (Jumper et al., 2021) indicated that RipE contains mostly intrinsically disordered regions, which are flexible and lack well-defined three-dimensional structures except for one α-helix at the C-terminus and a short β-sheet near the N-terminus (Figure 1F).
RipE expression by E. japonica in DH82 cells
Western blotting with a mouse antiserum developed against full-length recombinant RipE (rRipE) demonstrated that native RipE was expressed by WT E. japonica but was indeed absent in the ΔripE mutant (Figure 2A). However, in agreement with previous growth curve analysis by PCR based on Ehrlichia 16S rRNA (Bekebrede et al., 2020), the growth of WT and ΔripE E. japonica in DH82 cells was also similar as determined by Western blotting with an antibody (Ohashi et al., 1998) against the recombinant Ehrlichia major outer-membrane protein P28 (rP28) (Figure 2A). Inside host cells, Ehrlichia spp. undergo a biphasic developmental cycle (Zhang et al., 2007; Rikihisa, 2010), i.e., a nonreplicating infectious dense-core (DC) cell form (0.4–0.6 µm) and a replicating noninfectious reticulate cell (RC) form (>0.8 µm) (Popov et al., 1995). To determine the expression of ripE mRNA by E. japonica, synchronized cultures of WT E. japonica-infected DH82 cells were established as previously described (Liu et al., 2012). The E. japonica growth curve was first determined based on reverse transcription-qPCR (RT-qPCR) analysis comparing the levels of Ehrlichia 16S rRNA with dog GAPDH (Figure 2B). The results revealed a lengthy lag phase of Ehrlichia growth up to 36 h pi, followed by slow growth between 36 and 48 h and exponential growth between 60 and 84 h pi (Figure 2B). RT-qPCR using ripE-specific primers (Supplementary Table S1) demonstrated that the expression of ripE mRNA peaked at 48–60 h pi in synchronous cultures prior to the exponential growth (Figure 2B). Immunofluorescence microscopy showed that, at 3 days pi, the majority of E. japonica-containing morulae were positive for RipE (Figure 2C). To further characterize RipE expression among individual Ehrlichia of various sizes, host cell-free E. japonica was purified and immunofluorescence staining was performed using antibodies against RipE and P28 or CtrA, a transcription factor of the two-component system of Ehrlichia, which is primarily expressed at the DC stage (Cheng et al., 2011). Quantitation of RipE-expressing E. japonica (RipE+) among all P28-positive Ehrlichia showed that the majority (~80%) were in DC form, with a diameter of <0.5 μm (Figures 2D, E). Similarly, there was a significant correlation between RipE- and CtrA-positive Ehrlichia morulae (Figures 2F, G).
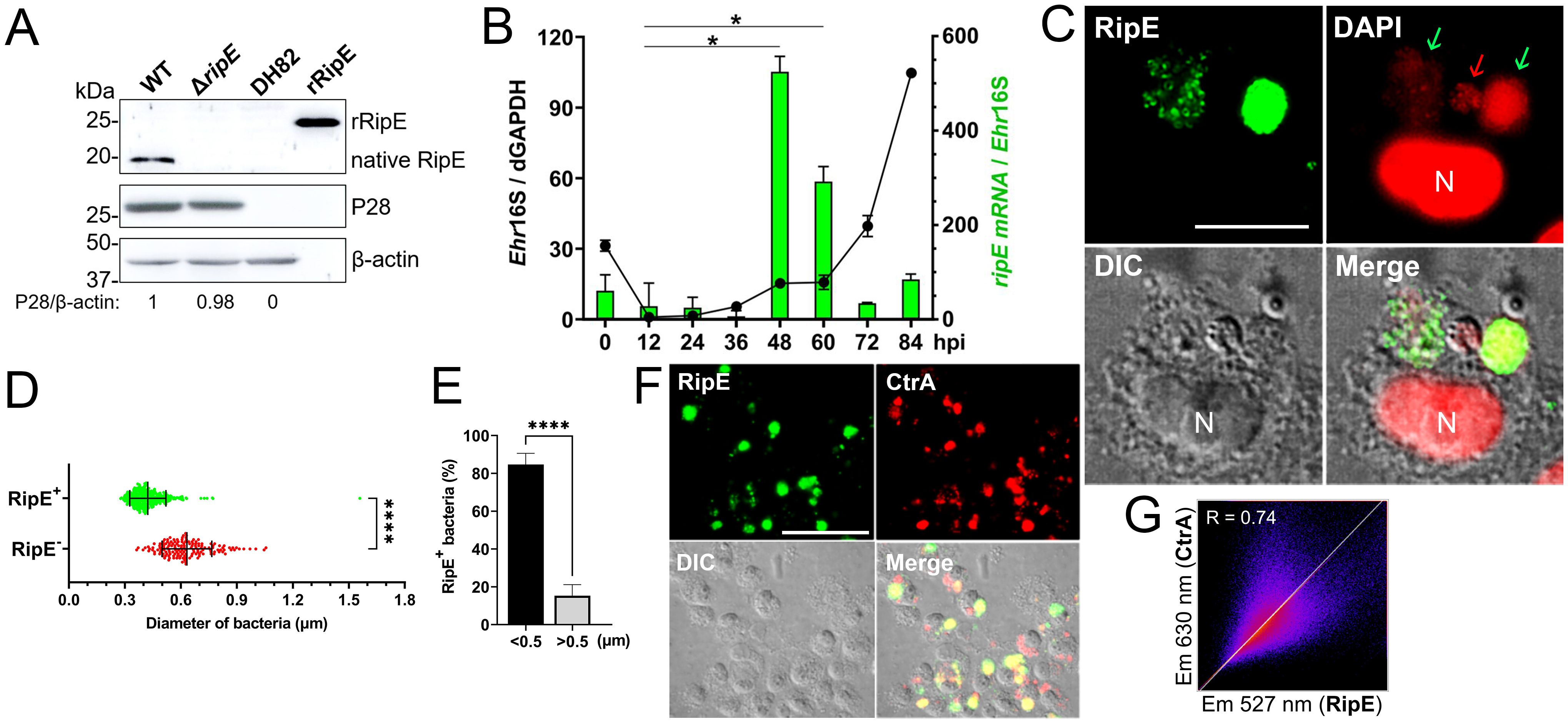
Figure 2. RipE is not expressed by the ΔripE mutant and differentially expressed by WT E. japonica. (A) Lysates of WT and ΔripE E. japonica-infected and uninfected DH82 cells and rRipE were analyzed by Western blotting using antibodies against rRipE, Ehrlichia P28, and β-actin. (B) Growth curve of Ehrlichia (black line) and expression of RipE mRNA (green bar) by WT E. japonica in DH82 cells were analyzed by RT-qPCR. E. japonica 16S rRNA (Ehr16S) expression was normalized by dog GAPDH mRNA (dGAPDH). hpi, hours post infection. RipE mRNA/Ehr16S with the ratio at 12 hpi set as 1. Data are displayed by the 2−ΔΔCT method and shown as means ± standard deviations (n = 3). The result is a representative of two independent experiments. *p < 0.05 by ANOVA. (C) WT E. japonica-infected DH82 cells at 3 dpi were fixed and labeled with mouse anti-rRipE and goat anti-mouse IgG (AF488, green). DNA was stained with DAPI (pseudo-colored in red). N, nucleus. Scale bar, 10 µm. (D, E) Host cell-free WT E. japonica was fixed and double labeled with mouse anti-rRipE (AF488, green) and rabbit anti-P28 (AF555, red). The diameter of individual WT bacteria was measured using ImageJ software. (D) Size distributions of P28-labeled E. japonica with or without RipE labeling. RipE+, RipE-positive, and P28-positive (green) bacteria. RipE−, RipE-negative and P28-positive bacteria. Vertical bars indicate the mean and standard deviation. ****, Significantly different (p < 0.0001) by two-tailed Student’s t-test (total bacteria, n = 565). (E) The percentage of RipE+ bacteria in two size groups: >0.5 µm and <0.5 µm. Data indicate the mean ± standard deviation of immunofluorescence images (n = 6) from Panel (D) ****, Significantly different (p < 0.0001) by two-tailed Student’s t-test. (F) Double immunofluorescence labeling of WT E. japonica-infected DH82 cells with mouse anti-rRipE (AF488, green) and rabbit anti-Ehrlichia CtrA (AF555, red). Merge, fluorescence images merged with differential interference contrast (DIC) image. Scale bar, 50 µm. (G) Pearson’s correlation coefficient (R = 0.74) of RipE and CtrA in WT E. japonica morulae in DH82 cells from panel (F) as determined by using ImageJ software with the Coloc 2 plugin.
RipE is expressed by E. japonica in mice, and ΔripE proliferates less in the blood and tissues than WT
Upon intraperitoneal (i.p.) inoculation, E. japonica expressed ripE mRNA in various tissues of mice and levels were higher in the blood and spleen than in the liver and peritoneal lavage at day 7 pi (Figure 3A). To investigate the time course of proliferation of ΔripE bacteria in vivo, mice were inoculated i.p. with ΔripE or WT E. japonica, and bacterial load in blood samples was determined by qPCR during the early infection period. Up to day 5 pi, bacterial load did not differ significantly between the ΔripE- and WT-infected mouse groups (Figure 3B). From days 5 to 7 pi, however, the bacterial load of WT E. japonica was significantly greater than that of ΔripE E. japonica (Figure 3B). E. japonica infection in mouse blood via i.p. injection involves multiple steps, including the infection of resident macrophages in the peritoneal cavity and the subsequent spread to circulating blood. To examine E. japonica proliferation directly in the blood, mice were inoculated intravenously (i.v.) through the retro-orbital venous plexus with host cell-free ΔripE or WT E. japonica. RT-qPCR data showed that, as early as 6 h pi, the abundance of WT E. japonica in the blood was significantly greater than that of ΔripE, which remained higher throughout the 2-day infection time course (Figure 3C). In addition, mice inoculated i.v. with WT E. japonica showed signs of severe illness at day 3 pi and were moribund or had died by day 4, whereas mice inoculated with ΔripE did not exhibit any signs of illness at day 4 pi.
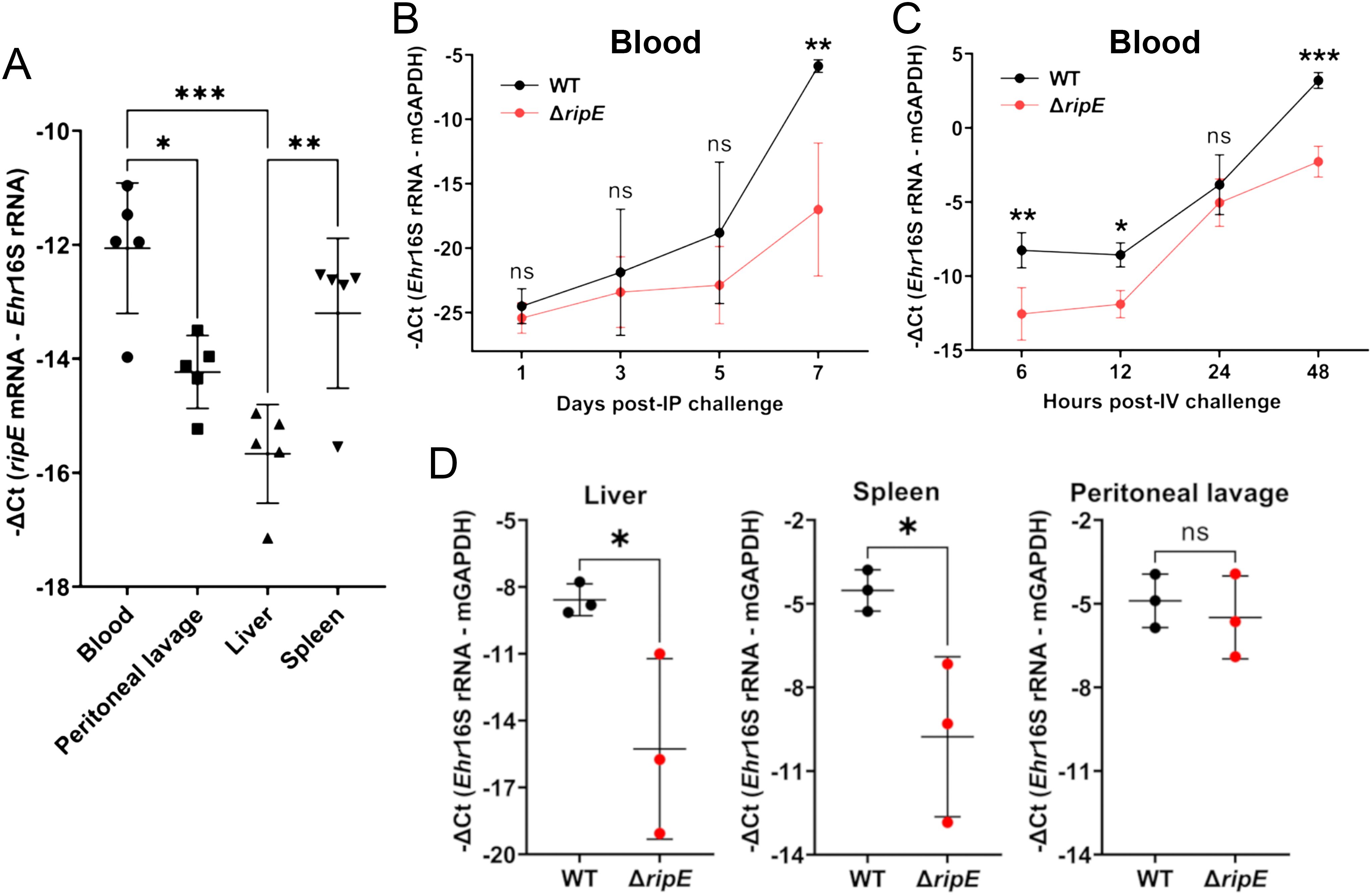
Figure 3. RipE is expressed by E. japonica in mice and the ΔripE mutant has reduced infection in the blood and tissues compared to WT. (A) ripE mRNA expression of WT E. japonica in mouse tissues at 7 days pi. ICR mice were inoculated i.p. with infected DH82 cells containing ~24,000 WT E. japonica. Relative amount of ripE mRNA was analyzed by RT-qPCR and normalized against Ehrlichia (Ehr) 16S rRNA. Data indicate the mean ± standard deviation (n = 5). Results were analyzed with one-way ANOVA followed by Tukey’s multiple comparisons. ***p < 0.001, **p < 0.01, *p < 0.05. (B) Temporal bacteria loads (Ehr 16S rRNA) in the blood of C57BL/6 mice i.p. inoculated with infected DH82 cells containing ~1,000 WT or ΔripE E. japonica by qPCR. (C) Temporal bacteria loads (Ehr 16S rRNA) in the blood of C57BL/6 mice i.v. inoculated with 1 × 107 host cell-free WT or ΔripE E. japonica by RT-qPCR. (B, C) Input DNA or RNA was normalized by mouse GAPDH. Data indicate the mean ± standard deviation (n = 3). Results were analyzed with repeated-measures ANOVA followed by Šídák multiple comparisons. ***p < 0.001, **p < 0.01, *p < 0.05, ns, not significantly different. (D) Bacteria loads in the tissues of C57BL/6 mice i.p. inoculated with infected DH82 cells containing ~100,000 WT or ΔripE E. japonica. Ehr 16S rRNA was analyzed by RT-qPCR and normalized by mouse GAPDH mRNA. Data indicate the mean ± standard deviation (n = 3). *p < 0.05, ns, not significantly different by the Student’s t-test.
To better understand the role of RipE during the early phase of infection, we inoculated mice (i.p.) with ~1,000-fold greater numbers of ΔripE or WT E. japonica than used for the experiment shown in Figure 2B, followed by euthanasia and tissue sampling for subsequent RT-qPCR analysis. As early as 3 days pi, the bacterial load of the ΔripE mutant was significantly lower in the liver and spleen compared with WT E. japonica (Figure 3D); for peritoneal lavage, however, the difference was not statistically significant (Figure 3D). Taken together, these data suggested that RipE may be involved in systemic infection and bacterial spread to various organs via blood.
Extracellular ΔripE E. japonica loses infectivity more rapidly and has lower ATP content than WT E. japonica
As an obligatory intracellular bacterium, E. japonica must bind and enter host cells (Rikihisa, 2010). We therefore examined whether RipE plays a role in this process. Using two-step fluorescent labeling, we quantified host cell surface-bound (non-internalized) vs. internalized Ehrlichia upon incubation with DH82 and RF/6A cells as previously described (Mohan Kumar et al., 2013), revealing no significant difference in bacterial binding or entry between ΔripE and WT E. japonica (Figure 4).
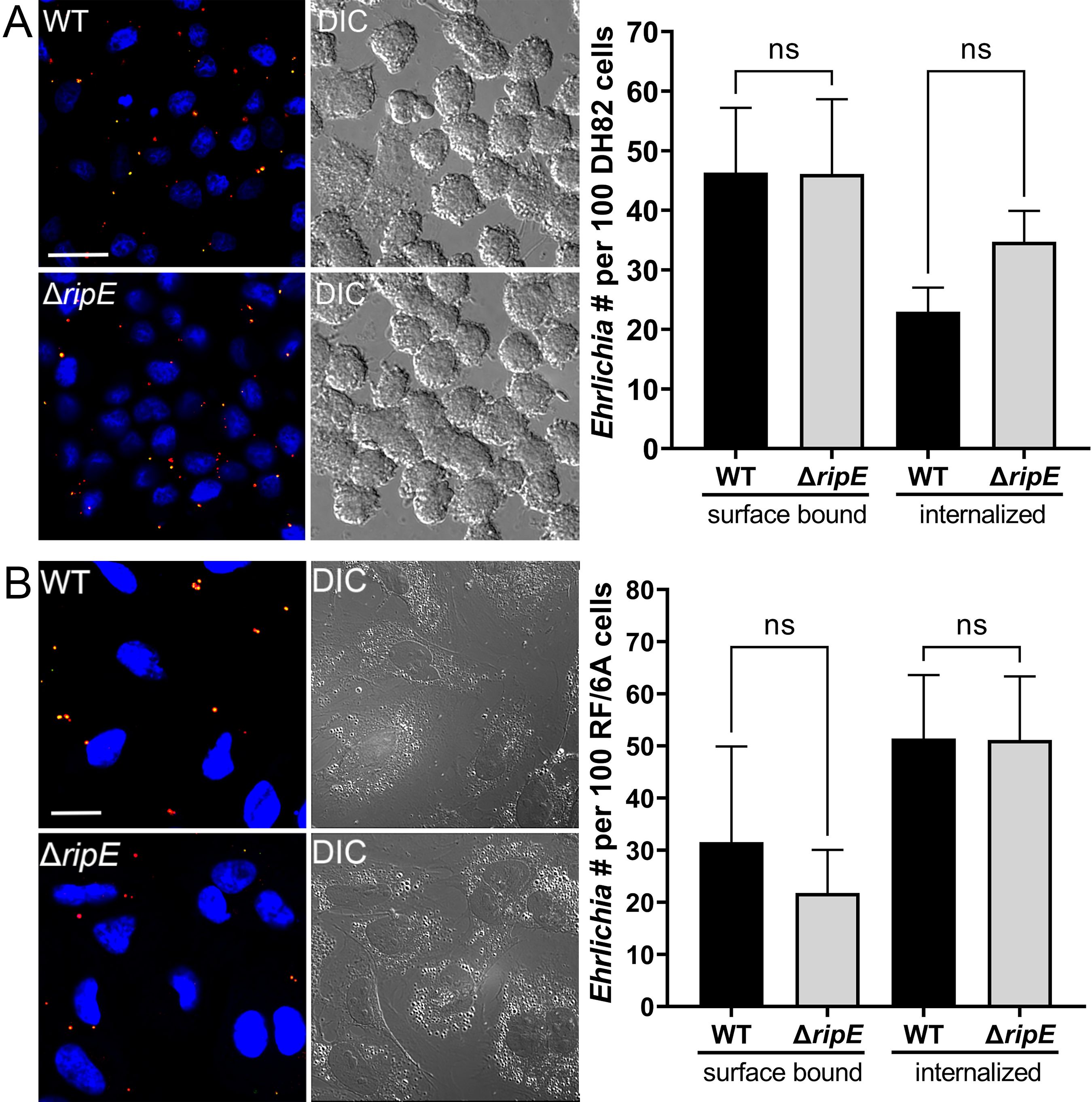
Figure 4. Host cell-free WT and ΔripE E. japonica showed no difference in binding and entering mammalian cells in culture. (Left) Immunofluorescence image showing host cell-free WT and ΔripE E. japonica incubated with DH82 for 30 min (A) or RF/6A cells for 60 min (B). Cells were fixed and stained with anti-P28 (AF488, green), and then stained with anti-P28 (AF555, red) with saponin permeabilization. Scale bar, 20 µm. (Right) The numbers of bound bacteria (yellow) and internalized bacteria (red) in 100 cells were scored in ImageJ software. ns, not significantly different by the Student’s t-test (n = 3).
Ehrlichia must be released from infected host monocytes/macrophages and survive the extracellular stage to infect new host cells and spread to other tissues/organs. Therefore, we next compared the infectivity of host cell-free Ehrlichia that had been briefly preincubated in culture medium up to 60 min. Without preincubation, freshly isolated ΔripE and WT E. japonica had the same infectivity on DH82 cells (Figures 5A, B). Both ΔripE and WT E. japonica progressively lost their infectivity in the host cell-free medium, but ΔripE lost its infectivity significantly faster than WT did (Figure 5A).
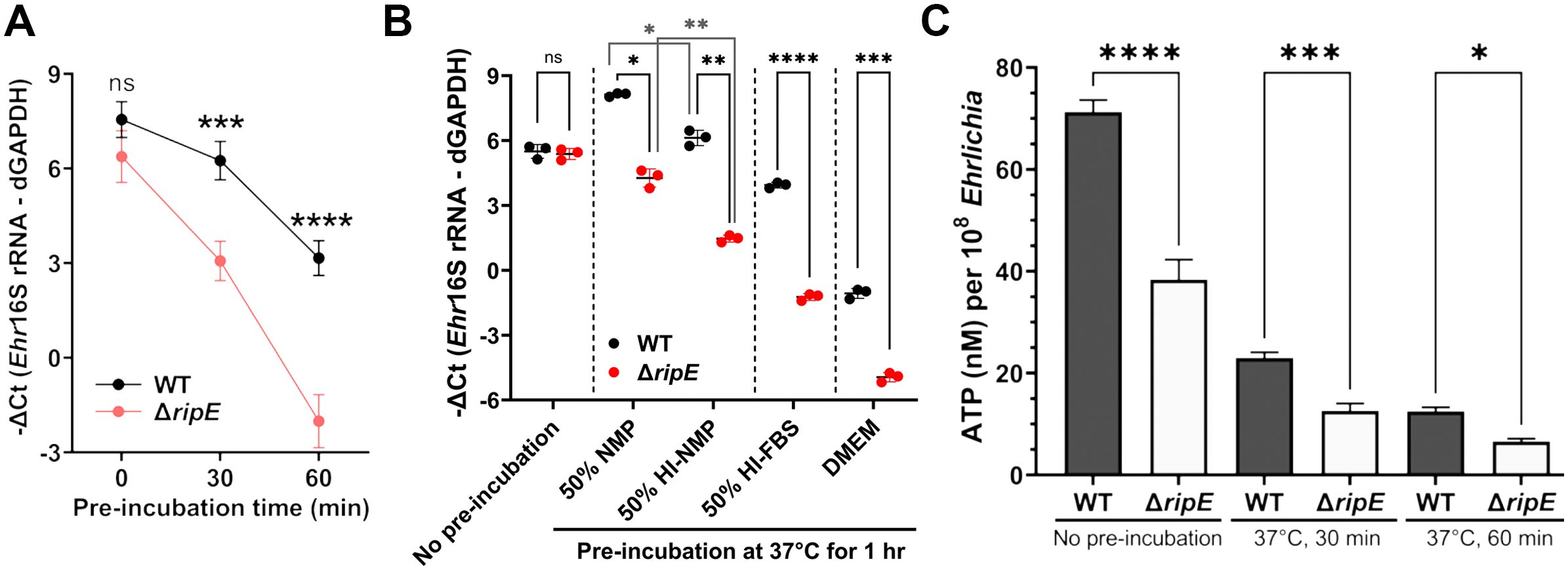
Figure 5. Host cell-free ΔripE E. japonica more rapidly loses infectivity and ATP reserve in culture than WT E. japonica. (A) Temporal loss of infectivity of host cell-free WT or ΔripE E. japonica preincubated at 37°C in culture medium (DMEM with 4% heat-inactivated FBS) in DH82 cells (MOI 2,500–3,000). (B) Infectivity of host cell-free WT and ΔripE E. japonica preincubated at 37°C for 1 h in different media in DH82 cells (MOI 500–1,000). NMP, normal mouse serum; HI, heat inactivated at 56°C for 30 min. (A, B) The infected DH82 cells were harvested at 2 dpi for Ehrlichia 16S rRNA-specific RT-qPCR. Input RNA was normalized by dog GAPDH (dGAPDH). Data indicate the mean ± standard deviation (n = 3). Results were analyzed with repeated-measures ANOVA followed by Šídák multiple comparisons (A) or one-way ANOVA followed by Tukey’s multiple comparisons (B). ****p < 0.0001, ***p < 0.001, **p < 0.01, *p < 0.05, ns, not significant different. (C) Levels of ATP in host cell-free WT or ΔripE E. japonica incubated in 4% heat-inactivated FBS for 0, 30, and 60 min. ATP levels were determined using the Luminescent ATP Detection Assay Kit normalized with the number of Ehrlichia determined by Ehr16S rRNA gene-specific qPCR. Data indicate the mean ± standard deviation of triplicate samples. ****p < 0.0001, ***p < 0.001, *p < 0.05, significantly different based on one-way ANOVA followed by Tukey’s multiple comparisons.
Extracellular Ehrlichia is exposed to the serum, and serum complement resistance has been reported for certain Rickettsia spp (Riley et al., 2018). Thus, to determine the effects of serum on extracellular Ehrlichia, and whether E. japonica is complement-resistant, we preincubated host cell-free Ehrlichia (WT or ΔripE) in 50% normal mouse plasma (NMP), which mimics mouse blood, and 50% heat-inactivated NMP; negative controls include 50% heat-inactivated fetal bovine serum (FBS, used in cell culture of E. japonica) and serum-free Dulbecco’s modified Eagle medium (DMEM). The presence of serum in the culture media profoundly protected the infectivity of extracellular Ehrlichia regardless of the type of serum in both WT and ΔripE mutant (Figure 5B). The infectivity of E. japonica was significantly lower by heat inactivation of serum components (Figure 5B), suggesting that E. japonica is complement-resistant, and heat-labile plasma components are rather beneficial for extracellular Ehrlichia. The ΔripE mutant lost its infectivity significantly more than WT E. japonica in each medium tested, including in the absence of serum (Figure 5B), implying that the presence of RipE helps maintain the infectivity of extracellular Ehrlichia via a mechanism that is independent of factors in the serum.
Freshly isolated Ehrlichia can transiently produce the essential chemical-energy component adenosine triphosphate (ATP) (Weiss et al., 1989). We therefore quantified the time course of the total ATP in host cell-free Ehrlichia using the Luminescent ATP Detection Assay Kit (see Materials and Methods). The total ATP levels in host cell-free Ehrlichia were significantly higher in WT than in the ΔripE mutant regardless of the incubation time in the culture medium containing 4% heat-inactivated FBS (Figure 5C).
Genomic complementation of the ΔripE mutant partially restores the Ehrlichia ATP level, and overexpression of ripE increases the ATP level and in vivo virulence
To test if RipE produced within Ehrlichia counteracts reduced ATP levels in the extracellular ΔripE mutant and increases Ehrlichia virulence in mice, we performed genomic complementation of the ΔripE mutant with ripE or overexpression of ripE in WT E. japonica bacteria by Himar1 mutagenesis with the newly constructed pCis-FLAG-RipE-Gent-Himar plasmid, which encodes a gentamicin resistance gene and FLAG-RipE (Figure 6A). A total of three transformed WT (WT+; genomic insertion of Himar 1, including Eja+0814, Eja+1020, and Eja+1231) and one transformed ΔripE (ΔripE+; genomic insertion of Himar1, H59+1014) E. japonica were obtained and stably cultured in DH82 cells (Bekebrede et al., 2020) (Table 2). In subsequent experiments, WT+ (Eja+0814) was used as a representative of E. japonica overexpressing RipE, whereas ΔripE+ (H59+1014) was used to represent rescued ΔripE E. japonica. ripE-gene-specific flanking PCR confirmed the original Himar1 insertion in ΔripE and ΔripE+, whereas the ripE gene was intact in WT and WT+ (Figure 6B).
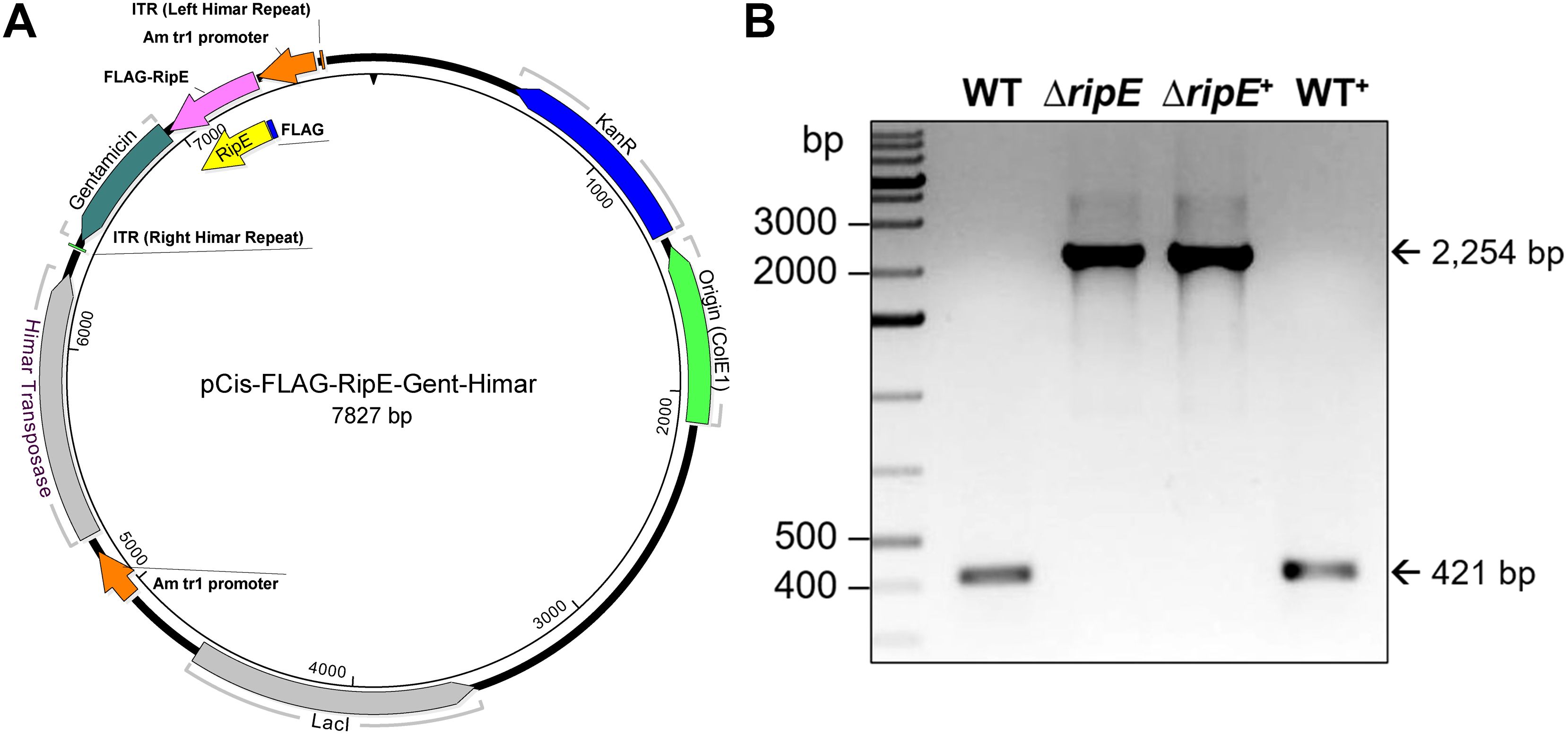
Figure 6. Plasmid construction for genomic complementation of ripE in ΔripE E. japonica and overexpression of ripE in WT E. japonica. (A) pCis-FLAG-RipE-Gent-Himar plasmid map. (B) ripE gene-specific flank PCR for ΔripE and ΔripE+ clonality verification. Corresponding amplicon sizes for WT (421 bp) and the original Himar1 insertion disrupted ripE gene (2,254 bp) are indicated by arrows.
Immunofluorescence staining revealed that WT+ E. japonica overexpressed RipE within more than 80% of the Ehrlichia morulae, much higher than what was observed for WT E. japonica, which typically contained 20-30% RipE-positive morulae (Figure 7A). Genomic complementation of the ΔripE mutant partially restored ripE expression in ΔripE+ E. japonica, although RipE abundance was relatively low, i.e., less than 5% RipE-positive morulae (Figure 7A). Western blotting confirmed that WT+ E. japonica overexpressed approximately 9-fold or 60-fold more RipE protein than WT or ΔripE+ E. japonica in culture, respectively (Figure 7B).
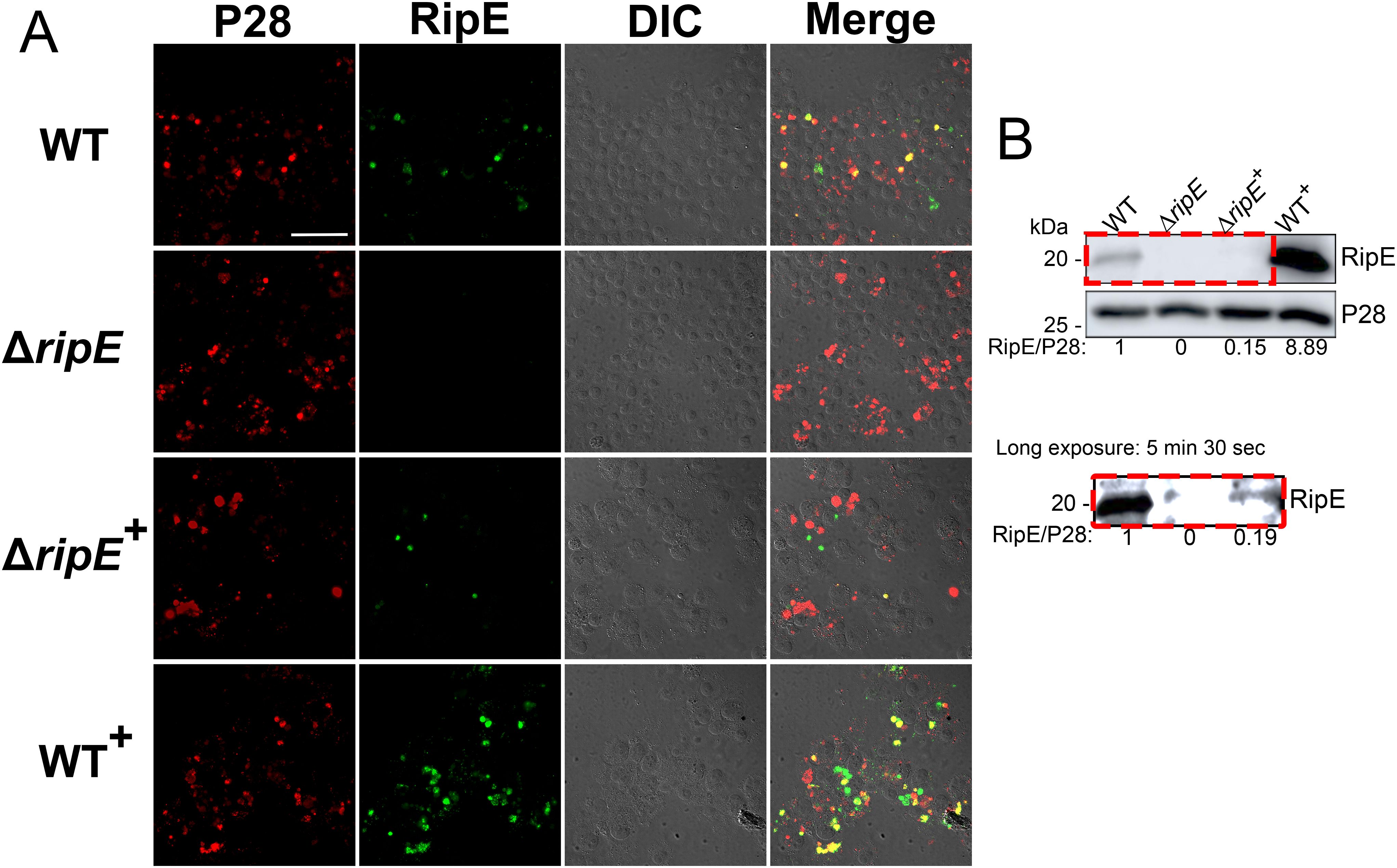
Figure 7. Genomic complementation or overexpression of ripE. (A) Double immunofluorescence labeling of WT, ΔripE, RipE-complemented ΔripE (ΔripE+), and RipE-overexpressing WT (WT+) E. japonica-infected DH82 cells with rabbit anti-Ehrlichia P28 (AF555, red) and mouse anti-rRipE (AF488, green). Merge, fluorescence image merged with differential interference contrast (DIC) image. Scale bar, 50 µm. (B) Lysates of WT, ΔripE, ΔripE+, and WT+ E. japonica-infected DH82 cells were analyzed by Western blotting using anti-rRipE and anti-P28. Numbers below each band are relative ratios of RipE band intensities normalized by P28, with the ratio of WT set as 1. Red dashed box on the lower panel showed the image under long exposure time (5 min and 30 s).
To test if RipE amounts within Ehrlichia correlate with ATP levels in extracellular Ehrlichia, the time course of ATP loss in extracellular WT, ΔripE, and the transformed E. japonica in the culture medium that contains 4% heat-inactivated FBS was examined. Without incubation at 37°C, WT+ E. japonica had the highest average ATP level, followed by WT, ΔripE+, and ΔripE E. japonica (Figure 8A). After incubation at 37°C for 30 or 60 min, however, the ATP levels dropped greatly in all groups, although WT+ E. japonica maintained a significantly higher ATP level compared to WT, ΔripE+, and ΔripE E. japonica (Figure 8A).
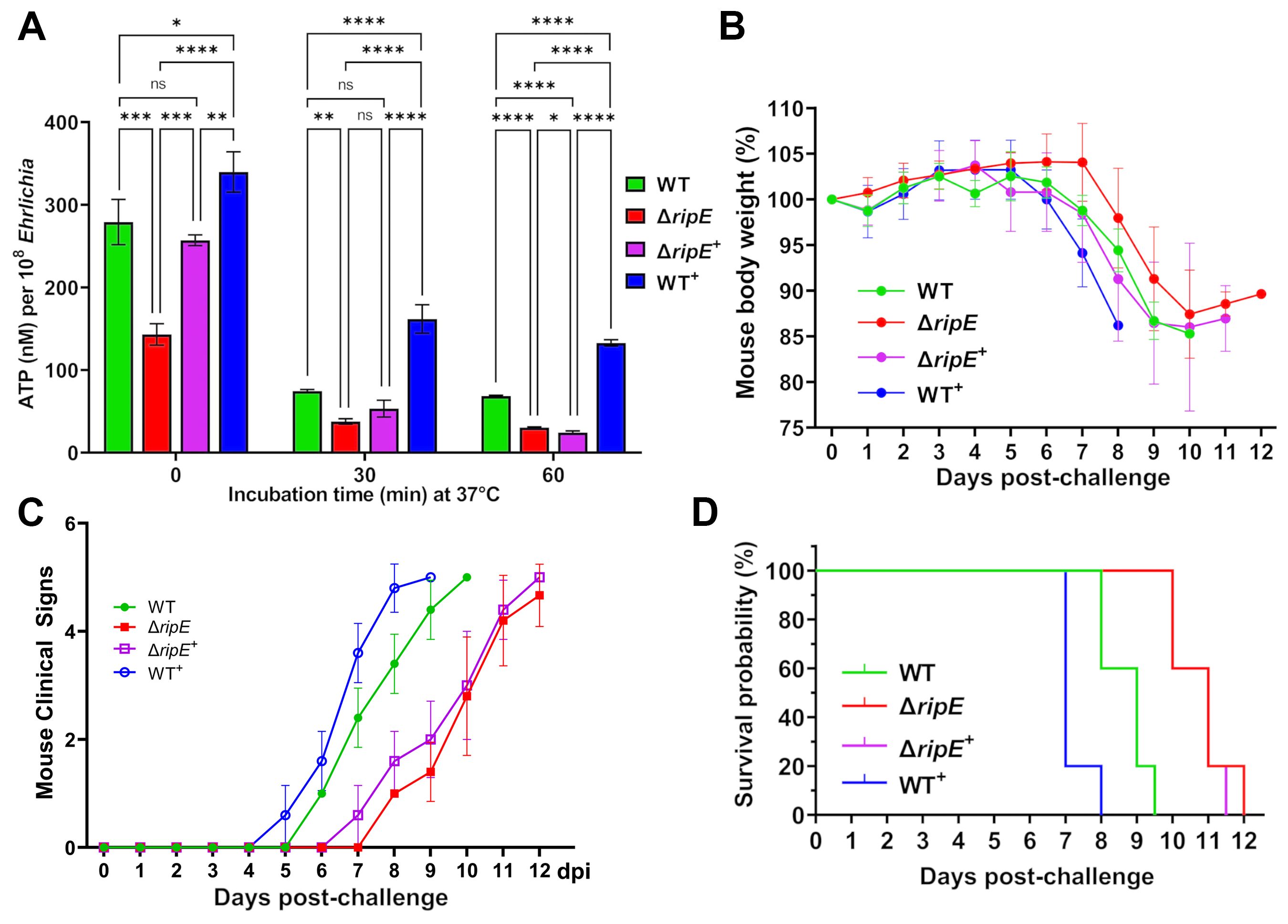
Figure 8. RipE expression increases Ehrlichia ATP reserve and in vivo virulence. (A) Levels of ATP in host cell-free WT, ΔripE, ΔripE+, and WT+ E. japonica incubated in 4% heat-inactivated FBS for 0, 30, and 60 min. ATP levels were determined using the Luminescent ATP Detection Assay Kit and normalized with bacterial numbers determined by Ehrlichia 16S rRNA gene-specific qPCR. Data indicate mean ± standard deviation of triplicate samples. ****p < 0.0001; ***p < 0.001; **p < 0.01; *p < 0.05; significantly different, ns, not significantly different based on one-way ANOVA followed by Tukey’s multiple comparisons. (B–D) ICR mice (4-week-old male, five mice per group) were i.p. inoculated with WT, ΔripE, ΔripE+, and WT+ E. japonica-infected DH82 cells. The number of Ehrlichia inoculated was determined by Ehrlichia 16S rRNA-specific qPCR: WT, 5,195 bacteria/mouse; ΔripE, 5,902; ΔripE+, 4,654; and WT+, 9,490. (B) Body weight changes. (C) Signs of illness (ruffled fur coat, hunched back, and squinty eyes, and reluctance to move) were assigned the rating of 0 to 4 for severity and 5 for death. Statistical analysis was performed using two-way repeated-measures ANOVA and showed that clinical signs of the infected mice were significantly more severe in those challenged with E. japonica expressing RipE in the order of WT+ > WT >> ΔRipE+ than the ΔRipE mutant that does not express RipE (p < 0.01). Data indicate mean ± standard deviation of five mice. (D) Kaplan–Meier survival curves. Compared with mice inoculated with WT, mice inoculated with ΔripE and ΔripE+ survived significantly longer (p < 0.01), whereas mice inoculated with WT+ died significantly earlier (p < 0.01) analyzed by log-rank (Mantel–Cox) test (n = 5). There was no significant difference between ΔripE and ΔripE+ (p = 0.663).
To test whether intrinsic RipE levels correlate with Ehrlichia virulence in vivo, ICR mice were inoculated i.p. with WT, ΔripE, ΔripE+, or WT+ E. japonica (five mice per group). Mice were weighed daily and monitored for clinical signs. Although the body weight changes were not dramatically different among the groups (Figure 8B), the onset and severity of clinical signs of the infected mice were much earlier and significantly more severe in those challenged with E. japonica expressing RipE in the order of WT+ > WT >> ΔripE+ than the ΔripE mutant that does not express RipE (Figure 8C). Kaplan–Meier survival curves showed that WT+ E. japonica was significantly more virulent than WT and ΔripE E. japonica (Figure 8D); however, loss of virulence in ΔripE E. japonica was not restored in ΔripE+ E. japonica (Figure 8D), in agreement with the observed low level of RipE expression (Figures 7A, B).
RipE is a bacterial outer-membrane protein
To study mechanisms by which RipE is involved in maintaining the infectivity and ATP levels in extracellular E. japonica, we analyzed the subcellular localization of RipE in E. japonica using DeepLocPro, an AI-based prokaryotic deep-learning algorithm (Izadi-Pruneyre et al., 1999; Moreno et al., 2024). By this analysis, RipE protein was predicted to localize to the bacterial membranes or be secreted. Immunofluorescence labeling using RipE- and outer-membrane protein P28-specific antibodies demonstrated that RipE colocalized with P28, showing ring-like labeling patterns in both E. japonica-infected DH82 cells that had been permeabilized with saponin (Figure 9A) and in host cell-free WT E. japonica without permeabilization (Figure 9B). These results suggested that RipE was expressed on the Ehrlichia surface.
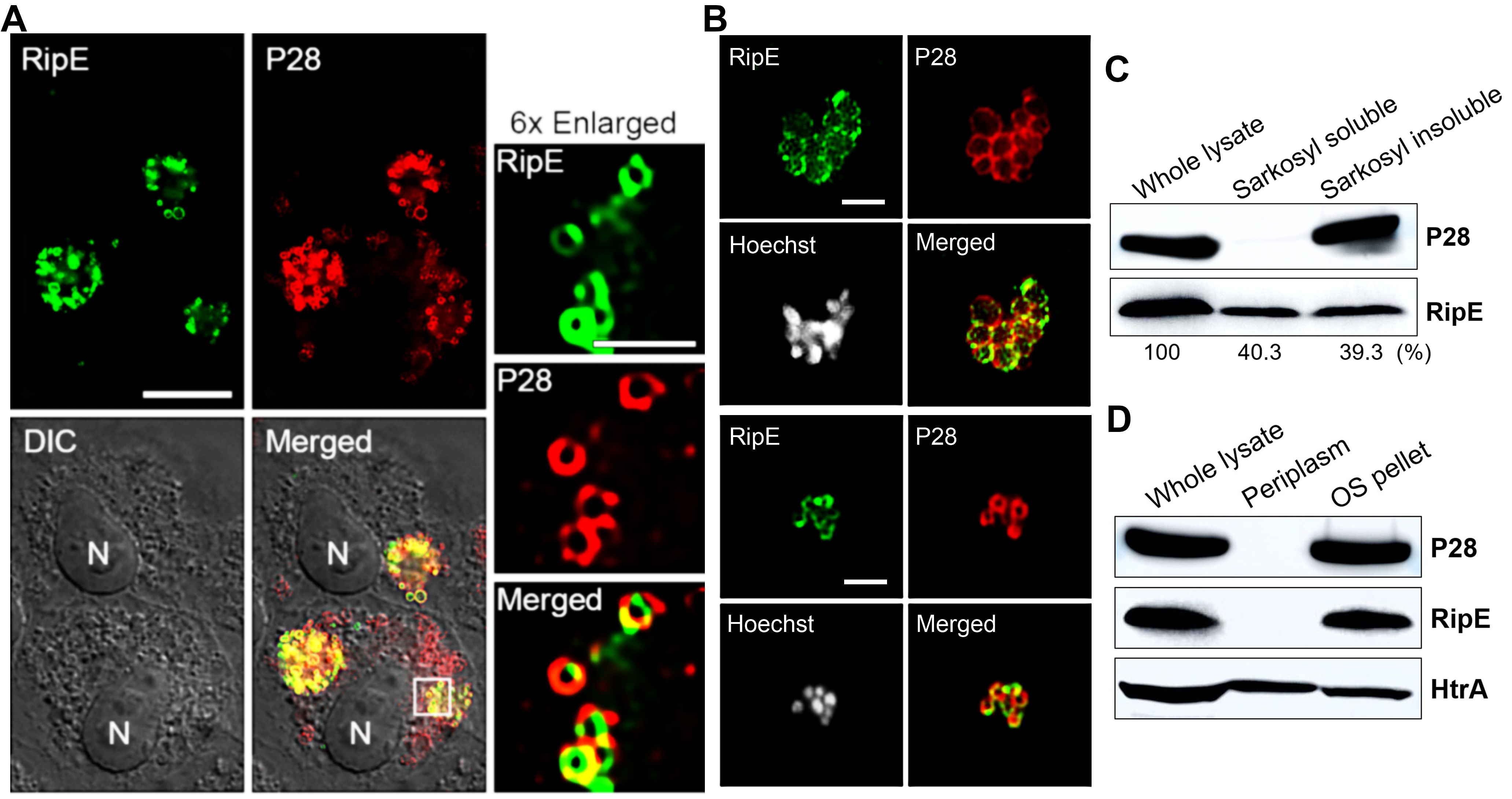
Figure 9. RipE is present on the outer membrane of Ehrlichia. (A) Immunofluorescence labeling of E. japonica overexpressing RipE (WT+) in DH82 cells with mouse anti-rRipE (AF488, green) and rabbit anti-P28 IgG (AF555, red) with membrane permeabilization by saponin. Scale bar, 10 µm. Merged, fluorescence images merged with DIC image. N, nucleus. Boxed area was enlarged 6× on the right. Scale bar, 2 µm. (B) Immunofluorescence labeling of spontaneously released WT E. japonica with mouse anti-rRipE (AF488, green) and rabbit anti-P28 IgG (AF555, red) without membrane permeabilization. DNA was stained by Hoechst 33342 and pseudocolored gray. Scale bar, 2 µm. (C) Western blot analysis of the whole lysate, and Sarkosyl-soluble and -insoluble (outer membrane) fractions of purified WT Ehrlichia with mouse anti-rRipE and rabbit anti-P28. Numbers below each band are percentages of RipE protein relative to the whole lysate (set as 100%). (D) Western blot analysis of the whole lysate, soluble (periplasm), and insoluble fractions by osmotic shock (OS) of purified WT E. japonica with mouse anti-rRipE and rabbit anti-Ehrlichia P28 and anti-HtrA sera.
To confirm the surface localization of RipE, E. japonica was fractionated by the sodium dodecyl sarcosine (Sarkosyl) solubilization method, which is an amphiphilic, ionic detergent that has been used to isolate the E. chaffeensis outer-membrane fraction (Ohashi et al., 1998). Sarkosyl fractionation of purified WT E. japonica resulted in soluble (cytosol, inner membrane, and periplasm) and insoluble (outer membrane) fractions. Using Ehrlichia P28 as a control, the results showed that native RipE of WT E. japonica was present in both Sarkosyl-soluble and Sarkosyl-insoluble fractions at a ratio of approximately 1:1 (Figure 9C), confirming that ~50% of RipE localizes to the outer membrane. To test if the remaining 50% of RipE is present in the periplasm of E. japonica, the osmotic shock method (Cheon et al., 2021) was adapted for isolating Ehrlichia periplasmic proteins (Kumagai et al., 2010). The results showed that, unlike the control E. japonica HtrA protein, which is a serine protease normally present in the E. chaffeensis periplasm and outer surface (Kumagai et al., 2010), RipE was absent in the periplasmic fraction (Figure 9D), suggesting that ~50% RipE is localized in the inner membrane or cytoplasm of E. japonica.
rRipE induces a robust humoral response with the production of an E. japonica-neutralizing antibody and is a vaccine candidate for ehrlichiosis
Anti-RipE antibody produced using rRipE recognized denatured protein by Western blotting (Figures 2A, 7B, 9C, D) and native protein by immunofluorescence staining (Figures 2C, F, 7A, 9A, B), indicating that rRipE is antigenic and capable of inducing a robust humoral response in mice. Indeed, in vitro neutralization experiments revealed that mouse anti-rRipE antisera could significantly reduce E. japonica infection in vitro in a dose-dependent manner compared to NMP (Figure 10). The neutralizing effect of the mouse anti-RipE antiserum for reducing E. japonica infection in vitro was similar to that of anti-rP28 (Figure 10), which can neutralize E. chaffeensis infection in immunocompetent mice (Ohashi et al., 1998) and protect severe combined immunodeficiency mice from fatal E. chaffeensis infection (Li et al., 2001).
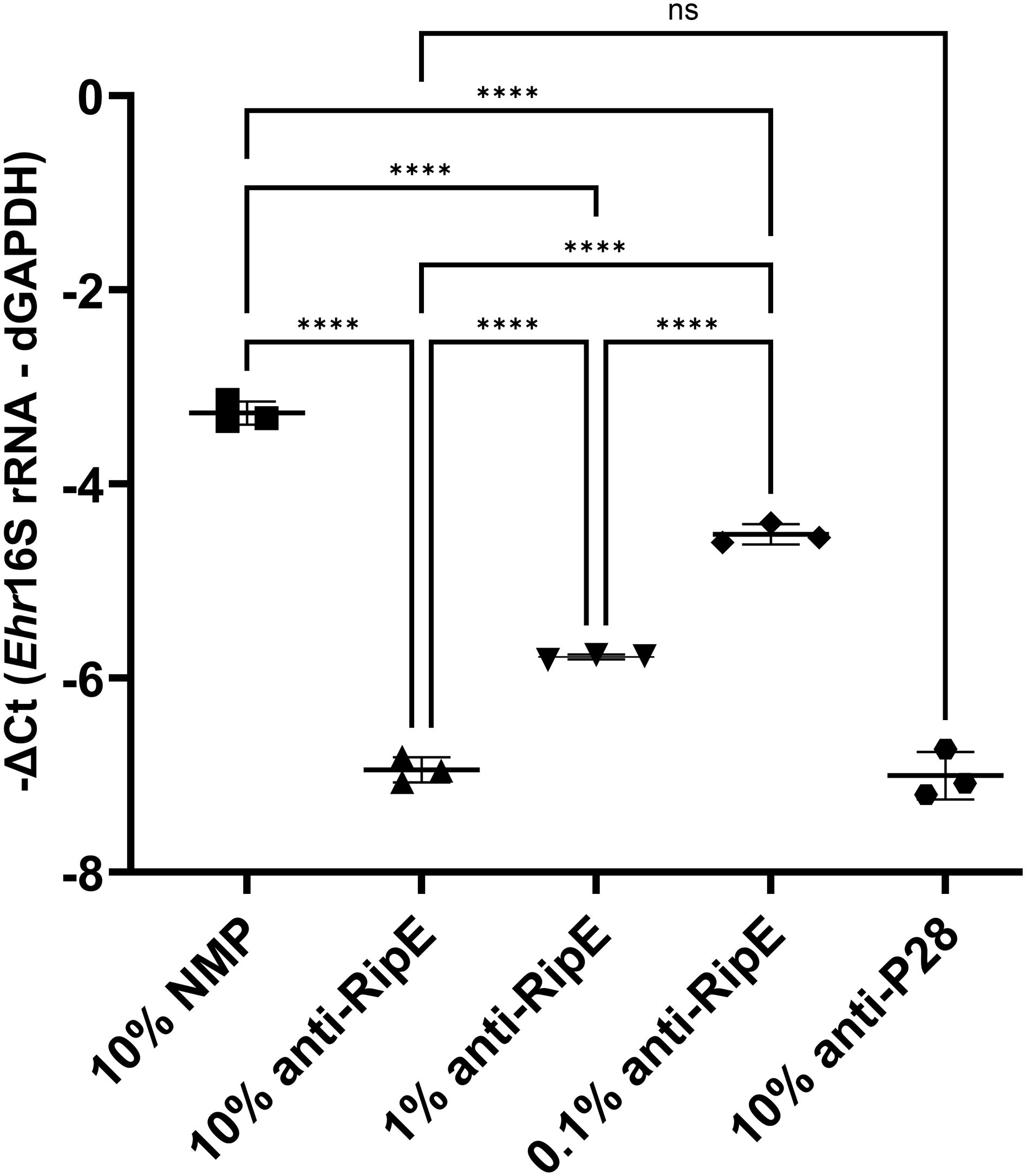
Figure 10. In vitro neutralization of E. japonica by anti-rRipE. Freshly isolated host cell-free WT E. japonica (~1,000 MOI) was preincubated with 10% normal mouse plasma (NMP), 10%, 1%, or 0.1% mouse anti-rRipE serum, or 10% rabbit anti-P28 serum at 37°C for 30 min. Sera and plasma were all diluted in the culture medium. The mixtures of Ehrlichia and antisera were added to DH82 cells and incubated at 37°C, and infection was determined at 48 hpi by Ehrlichia 16S rRNA gene-specific qPCR. Input DNA was normalized by dog GAPDH (dGAPDH). ****, significantly different (p < 0.0001); ns, not significantly different based on one-way ANOVA followed by Tukey’s multiple comparisons (n = 3). The result is representative of two independent experiments.
To test whether the rRipE-induced Ehrlichia-neutralizing antibody could prevent fatal ehrlichiosis in vivo, we immunized five mice three times with rRipE at 2-week intervals followed by a lethal challenge with WT E. japonica through i.p. injection. An enzyme-linked immunosorbent assay (ELISA) demonstrated that all rRipE-immunized mice generated a significant rRipE-specific IgG after the third immunization (Figure 11A), whereas the sham-immunized controls did not. At day 7 post-WT E. japonica challenge, all sham control mice had lost more than 10% of body weight (Figure 11B) and appeared severely ill or moribund, characterized by ruffled haircoat, hunched back, squinty eyes, and reluctance to move, whereas rRipE-immunized mice experienced lesser body weight loss, with mice being relatively normal, alert, and responsive. Bacterial load in the blood of rRipE-immunized mice was significantly lower than in sham controls at 5 days pi (Figure 11C). At 7–8 days pi, however, bacterial load did not differ between the two groups (Figure 11C). Kaplan–Meier curves showed that rRipE-immunized mice survived significantly longer than the sham controls, although all mice eventually succumbed to fatal ehrlichiosis up to 11 days pi (Figure 11D).
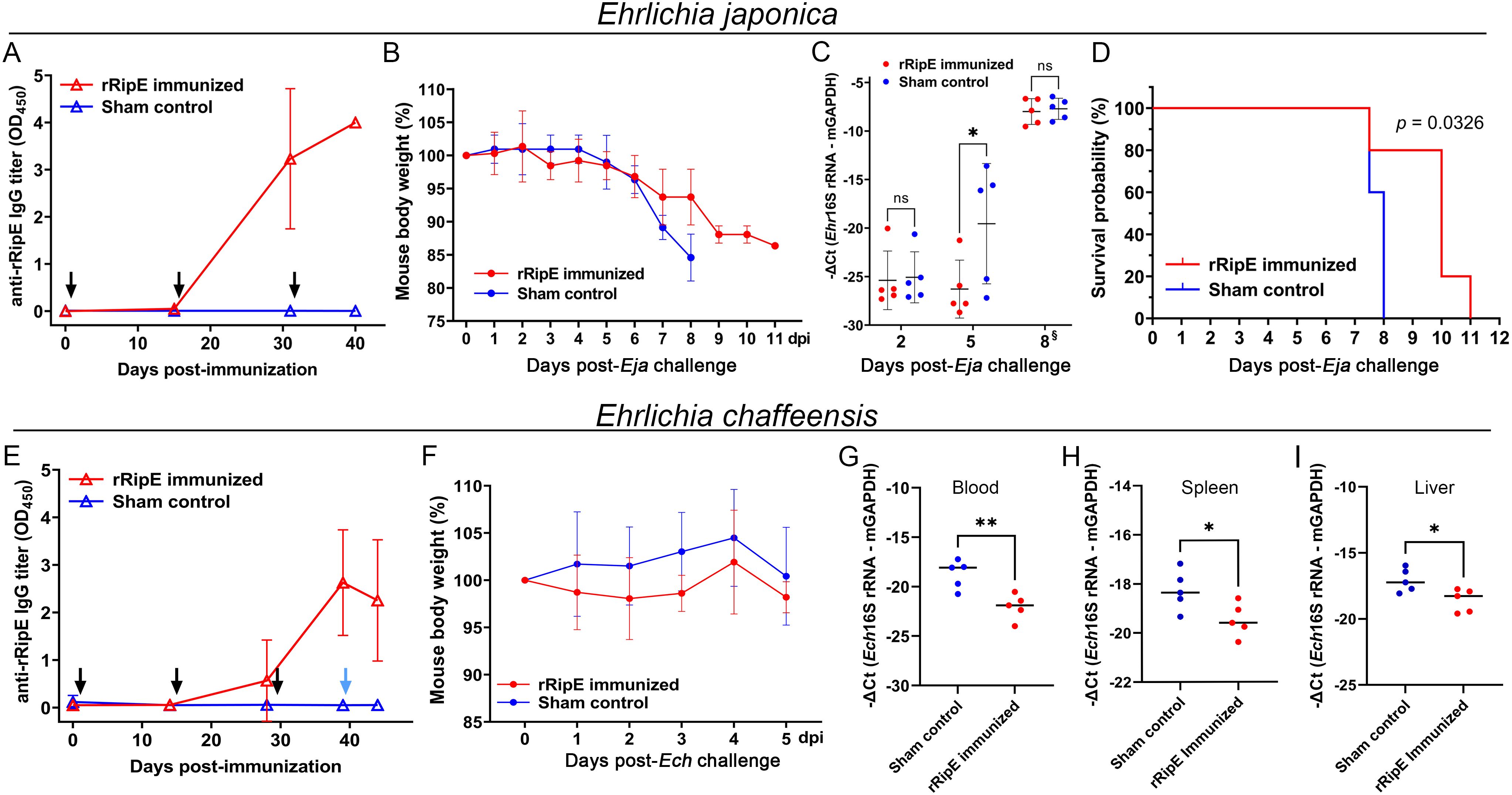
Figure 11. rRipE immunization increased the survival time of mice challenged with a lethal dose of E. japonica. ICR (A–D) or C57BL/6 (E–I) mice were subcutaneously injected with rRipE plus QuilA adjuvant (rRipE immunized) or QuilA alone (Sham control), and challenged by i.p. inoculation of infected DH82 cells containing a lethal dose of WT E. japonica (11,750) at 9 days post third immunization [Eja, (A–D)], or infected THP-1 cells containing E. chaffeensis (~120,000) at 11 days post third immunization [Ech, (E–I)], respectively. (A, E) Titers of rRipE-specific antibody in mice sera were measured by ELISA using rRipE as the antigen. Black arrows indicate days on which mice were vaccinated, and blue arrow denotes the day on which mice were challenged with E. chaffeensis. Data indicate the mean ± standard deviation (n = 5). (B, F) Mouse body weight changes in rRipE-immunized and sham-immunized mice upon challenge with WT E. japonica (B) or E. chaffeensis (F) (n = 5). (C) Bacterial loads of E. japonica in the blood of rRipE-immunized and control mice by Ehrlichia 16S rRNA gene-specific qPCR normalized by mouse GAPDH (mGAPDH). Data indicate the mean ± standard deviation (n = 5). Results were analyzed with repeated-measures ANOVA followed by Šídák multiple comparisons. *p < 0.05; **p < 0.01; ns, not significantly different. §Mouse blood samples were collected at 8 days pi or at the time of death (two control mice and one rRipE immunized mice were found dead a few hours before 8 days pi). (D) Kaplan–Meier survival curves for rRipE- and sham-immunized mice following lethal challenge with WT E. japonica. Compared with control mice, rRipE-immunized mice survived significantly longer (p < 0.05) by log-rank (Mantel–Cox) test (n = 5). (G–I) Bacterial loads of E. chaffeensis in the rRipE-immunized and sham-immunized mice on day 5 post-challenge were determined using Ehrlichia 16S rRNA gene normalized by mouse GAPDH (mGAPDH). (G) Blood samples, qPCR. (H) Spleen and (I) liver samples, RT-qPCR. The scatter plot shows the normalized Ehrlichia levels in individual mice, with the horizontal bar representing the mean value. **p < 0.01; *p < 0.05 by Student’s t-test.
As E. japonica and E. chaffeensis RipE share 46% amino acid identities (Table 1), we tested whether mice vaccinated with E. japonica rRipE could protect infection by the human ehrlichiosis agent E. chaffeensis. Mice immunization with E. japonica rRipE or sham control as described above was challenged i.p. with E. chaffeensis-infected DH82 cells (~1.2 × 105 bacteria per mouse). Similarly, all rRipE-immunized mice generated an abundance of rRipE-specific IgG after the third immunization (Figure 11E). Mouse infection with E. chaffeensis did not exhibit any apparent clinical signs (weight loss, lethargy, anorexia, squinting eyes, or ruffled fur) throughout the experimental course (Figure 11F). However, E. chaffeensis loads in the blood, spleen, and liver samples of rRipE-immunized mice were significantly lower than those of sham controls at 5 days post-challenge (Figures 11G–I), suggesting that RipE can be a potential vaccine candidate for human ehrlichiosis.
Discussion
As obligate intracellular bacteria, Ehrlichia spp. need to be extracellular in order to spread within the host and transmit between the susceptible cells. However, this process is poorly understood. The Ehrlichia in vivo virulence factor RipE (Bekebrede et al., 2020) is the first example of a bacterial molecule that helps maintain infectivity in the extracellular stage of Ehrlichia and its ATP levels (Bekebrede et al., 2020). RipE protein was expressed mostly at the DC stage of E. japonica and partially on the bacterial surface. There are clear correlations between RipE expression levels, bacterial ATP levels, and mouse virulence among WT E. japonica and ΔripE mutants. For obligate intracellular bacteria, the dogma has been that the extracellular, resistant forms, such as the elementary bodies (EBs) of Chlamydia trachomatis, are metabolically dormant so that they can reserve relatively higher energy than reticular bodies (RBs) until they reach the next susceptible host cell (Tipples and McClarty, 1993). However, Omsland et al. (2012) showed that both EBs and RBs of C. trachomatis can perform de novo protein synthesis and generate ATP in axenic culture, and proteomics analysis suggests that EBs of C. trachomatis are metabolically active (Skipp et al., 2016), which provides them the advantage and the ability to rapidly deploy bacterial effectors immediately upon contact with host cell plasma membrane and establish infection (Cossé et al., 2018). Protein and DNA synthesis was also demonstrated in host cell-free E. chaffeensis organisms incubated in an axenic medium containing amino acids, nucleotides, and different energy sources, albeit the biosynthetic activity was mostly observed in RCs rather than in DCs (Eedunuri et al., 2018). The detailed molecular mechanisms by which RipE modulates ATP levels and supports extracellular survival of E. japonica remain to be studied.
Despite the report of targeted mutagenesis (Hove et al., 2022) and genetic complementation (Wang et al., 2017) for E. chaffeensis, and functional trans-complementation for E. japonica (Zhang et al., 2024), restoration of in vitro and in vivo biological functions of mutated genes has been challenging. By transforming ΔripE and WT E. japonica with another newly constructed Himar1 plasmid encoding gentamicin resistance and ripE genes (Figure 6A), we successfully created genetically complemented/rescued ΔripE (ΔripE+) and RipE-overexpressing (WT+) E. japonica. Genomic complementation of the ΔripE mutant partially restored Ehrlichia ATP level, and overexpression of ripE increased Ehrlichia ATP level. WT+-challenged mice succumbed to fatal ehrlichiosis much earlier than the WT, suggesting that RipE overexpression promotes Ehrlichia in vivo infection. The expression level of RipE protein in ΔripE+ E. japonica was, however, relatively low. It is known that genetic insertion by Himar1 transposon usually occurs once per genome or rarely twice (Lampe et al., 1999; Felsheim et al., 2006; Le Breton et al., 2006; Cain et al., 2020). Himar1 insertion not only alters gene functions by intragenic insertion of the transposon but also may disrupt important regulatory elements of the genome (Lampe et al., 1999; Munderloh et al., 2012), resulting in either a lethal phenotype lost during bacterial isolation or a transformed bacterium that cannot survive alone. ΔripE+ E. japonica contains at least two Himar1 insertions, including the original insertion disrupting ripE and the second one disrupting mutS gene. It is possible that the double Himar1 insertions resulted in the instability of some essential genes for ripE expression. While the current work demonstrates significant progress, the molecular complementation methods of obligatory intracellular bacteria remain to be improved at multiple fronts.
Considering RipE functions, it is peculiar why RipE is required only in in vivo infection of E. japonica, but not in cell culture. Ehrlichia species are fastidious and infect only specific types of host cells, such as monocytes/macrophages (Rikihisa, 1991). For cell culture, as we use overabundant host cells such as DH82 canine macrophages that are permissive to Ehrlichia infection, Ehrlichia has a short extracellular stage to initiate a new cycle of infection. However, in vivo, especially in the circulating blood of a mammalian host, these permissive blood monocytes are much less; thus, Ehrlichia needs to be extracellular for a relatively longer period before infecting next host cells. This may be one of the reasons why RipE is required in vivo for effective Ehrlichia infection.
The host cell-free Ehrlichia in this study were isolated by sonication and filtration, kept on ice, and subsequently used for in vitro and in vivo (i.v. inoculation) experiments immediately (within 30 min) to minimize the loss of viability and infectivity of these extracellular Ehrlichia. Our results showed that host cell-free Ehrlichia are capable of replicating in the mouse peripheral blood within 24 h following i.v. inoculation (Figure 3C). Li and Winslow (2003) reported that extracellular Ehrlichia not only can survive in the mouse plasma but also have limited ability to proliferate outside host cells. Host cell-free E. japonica preincubated in medium containing 50% NMP showed significantly higher infectivity compared to 50% heat-inactivated NMP, 50% heat-inactivated FBS, or DMEM. Ehrlichia has a highly fragile membrane that lacks LPS and peptidoglycan (Lin and Rikihisa, 2003). The presence of serum in the medium could physically protect the fragile extracellular Ehrlichia. Ehrlichia is known to incorporate cholesterol and other lipids (Lin and Rikihisa, 2003; Lin et al., 2020) and metabolize l-glutamine (Rikihisa et al., 1994; Lin et al., 2016). It is possible that lipids, lipoproteins, amino acids, vitamins, or other nutrients in the mammalian plasma/serum can support extracellular Ehrlichia survival. Heat inactivation (56°C, 30 min) denatures or inactivates not only complements, but also some of these molecules in the plasma (Pellerin et al., 2021; Zhang et al., 2024), resulting in reduced infectivity of extracellular Ehrlichia.
E. chaffeensis is spontaneously cleared by the immune system of immunocompetent mice and does not cause any significant clinical signs or mortalities. The current study is the first to demonstrate that immunization can delay the fatal ehrlichiosis caused by E. japonica, a highly virulent bacterium in mice. Of note, rRipE immunization significantly reduced bacteremia on day 5 post-challenge, but not at the later stage (day 8 post-challenge) when challenged with a high lethal dosage of ~10,000 E. japonica (Figure 11C). With respect to relationships between bacteremia and clinical disease, however, our previous study showed that clinical disease is related to tissue macrophage infection, rather than peripheral blood monocyte infection (Zhang et al., 2024). For the rRipE immunization and E. japonica challenge study, we aim to establish survival curves; thus, mice were kept until they succumbed to fatal ehrlichiosis, and therefore, comparable tissues/organs from the same time point (e.g., day 8 post-challenge) were not available for analysis in this case. On the other hand, for the E. chaffeensis challenge, Ehrlichia loads were significantly lower in blood and tissue samples collected on day 5 post-challenge.
E. japonica is highly virulent to laboratory mice, and the infection with ~5,000–11,000 bacteria (~50- to 100-fold of LD50 of 100 bacteria per mouse) results in death within 7–9 days after the i.p. challenge (Figures 8D, 11C) (Bekebrede et al., 2020). It is unlikely that a detectable antibody titer against RipE or other E. japonica antigens is developed during this short period. Luo et al. reported that HME patient serum and sera from dogs with chronic E. canis infection also do not develop specific antibodies against RipE homologous proteins, ECH_0079 of E. chaffeensis and Ecaj_0047 of E. canis, respectively (Luo et al., 2020). This suggests that RipE-specific humoral immune response is not elicited during natural infections. On the other hand, our data showed that rRipE is immunogenic and capable of inducing a robust humoral response and Ehrlichia-specific neutralizing antibody in mice. Previous studies have shown that mice and dogs immunized with recombinant P28 or OMP-1B protein (major outer-membrane proteins), VirB2 (type IV secretion pilus protein), or EtpE (entry-triggering protein of Ehrlichia) of E. chaffeensis are protected from E. chaffeensis infection (Ohashi et al., 1998; Budachetri et al., 2020, Budachetri et al., 2022). rRipE immunization cross-protected mice from E. chaffeensis infection; thus, RipE can be an additional vaccine candidate against E. chaffeensis infection. The current study also poses challenges in developing Ehrlichia vaccines. Single-target vaccines may not be highly effective against Ehrlichia species as these obligate intracellular pathogens possess multiple virulence factors that compensate for the reduced ability of extracellular survival and help them evade host immunity (Yan et al., 2018; Budachetri et al., 2020; Rikihisa, 2021; Zhang et al., 2021; Budachetri et al., 2022). Therefore, a multivalent vaccine targeting multiple key virulence factors is expected to have a higher chance of success.
Materials and methods
Ethics statement for laboratory animal use
Animal experiments in this study were performed under approved protocols in accordance with guidelines of the Institutional Animal Care and Use Committee of the Ohio State University (OSU). The OSU has continued accreditation by the Association for Assessment and Accreditation of Laboratory Animal Care International, Animal Welfare Assurance from Public Health Services, United States Department of Agriculture, and is in full compliance with the PHS Policy and the Federal Animal Welfare Regulations.
Protein sequence analysis and structural prediction
The E. japonica RipE protein sequence was analyzed by the Protean program (DNASTAR Lasergene) (Plasterer, 2000), and the three-dimensional structure diagram was rendered by the AlphaFold algorithm (https://alphafold.ebi.ac.uk/entry/X5H2X1) (Jumper et al., 2021). DeepLocPro (https://ku.biolib.com/deeplocpro) was used to predict the protein localization.
Cloning, antibodies, and immunofluorescence assay
The gene encoding full-length ripE (EHF_0962) was PCR amplified using primers shown in Supplementary Table S1 and cloned into pET33b(+) (Novagen, Gibbstown, NJ) for expressing rRipE with 6×His-tagged on both N- and C-terminus (rRipE). rRipE protein was affinity purified from soluble fractions in transformed Escherichia coli BL21(DE3) (New England Biolabs, Ipswich, MA) using HisPur Cobalt Resin (Thermo Fisher) as previously described (Kumagai et al., 2006). Mouse antiserum against rRipE was developed by immunizing C57BL/6 mice (Envigo, Indianapolis, IN) three times with subcutaneous injection of rRipE (100 µg per mouse) admixed with 10 µg of Quil-A adjuvant (InvivoGen, San Diego, CA) at 2-week intervals. Other antibodies used in this study include rabbit anti-Ehrlichia P28 (Ohashi et al., 1998), rabbit anti-β-actin (Sigma-Aldrich, St. Louis, MO), and Alexa Fluor (AF) 488- or AF555-conjugated goat anti-mouse or anti-rabbit IgG (Invitrogen, Carlsbad, CA).
For immunofluorescence assay, Ehrlichia-infected DH82 or RF/6A cells or host cell-free Ehrlichia were fixed with 4% paraformaldehyde (PFA), washed, and incubated with primary antibodies and then fluorescence-conjugated secondary antibodies diluted in PGS permeabilization buffer (PBS with 0.1% gelatin, 0.05% BSA, and 0.3% saponin) or nonpermeable blocking buffer (PBS with 0.1% gelatin and 0.05% BSA). DAPI (4′,6′-diamidino-2-phenylindole, Invitrogen) or the cell-permeant Hoechst 33342 (Invitrogen) was used to stain nucleic acid. Fluorescence with overlay differential interference contrast (DIC) images were acquired with a DeltaVision PersonalDV deconvolution microscope system (GE Healthcare Life Sciences, Marlborough, MA) or a THUNDER imaging system with computational clearing to remove out-of-focus blur background (Leica Microsystems, Deerfield, IL). Image analysis was performed with ImageJ software (National Institutes of Health, Bethesda, MD). Antibodies used and dilutions are summarized in Supplementary Table S2.
Culture and purification of E. japonica, and separation of membrane fractions
The canine histiocytic leukemia cell line DH82 (Wellman et al., 1988) was cultured in DMEM (Dulbecco’s Modified Eagle’s Medium; Corning, Corning, NY) supplemented with 4% FBS (Atlanta Biologicals, Lawrenceville, GA) and 4 mM l-glutamine (Gln, Gibco, Waltham, MA) in a humidified incubator at 37°C with 5% CO2. The monkey endothelial cell line RF/6A was cultured in advanced MEM (Corning) with 8% FBS and 4 mM l-Gln. E. chaffeensis Arkansas strain (Dawson et al., 1991) was cultured in THP-1 cells (ATCC, Manassas, VA) (Barnewall and Rikihisa, 1994) in RPMI 1640 medium (Mediatech, Manassas, VA) supplemented with 8% FBS and 2 mM l-Gln.
E. japonica, ΔripE, and other Himar1 mutants were cultured in DH82 cells with medium supplemented with 4% FBS, 4 mM Gln, and 0.1 µg/mL cycloheximide (MilliporeSigma, Burlington, MA) (Bekebrede et al., 2020). Ehrlichia infectivities were examined by HEMA3 staining (Thermo Fisher, Waltham, MA) of cytocentrifuged slides using Shandon CytoSpin 4 centrifuge (Thermo Fisher). Host cell-free E. japonica was purified from highly infected DH82 cells by sonication on ice for 8 s twice at output setting 2 using a fine tip on a W380 Sonicator (Heat Systems, Newtown, CT). Following centrifugation at 700 × g to remove unbroken host cells and nuclei, host cell-free bacteria were pelleted at 10,000 × g. To obtain Ehrlichia outer-membrane fractions, host cell-free E. japonica was solubilized with 0.1% Sarkosyl, and outer-membrane fractions were collected by ultracentrifugation as previously described (Ohashi et al., 1998). The osmotic shock method was used for extraction of bacterial periplasm and membrane fractions as previously described (Cheon et al., 2021).
Synchronous culture of Ehrlichia was performed as previously described (Cheng et al., 2008; Liu et al., 2012). Briefly, sonicated infected cells were passed through a 2.7-µm syringe filter and subjected to a second round of strong sonication on ice (30 s, twice, at output setting 4.5) to destroy RC forms. The remaining viable DC forms were pelleted and incubated with DH82 cells at 100 multiplicity of infection (MOI) at 37°C for 1 h with periodic shaking, followed by washing to remove uninternalized bacteria.
The growth curves of Ehrlichia in RF/6A cells were conducted by incubating host cell-free Ehrlichia with RF/6A cells at 300–400 MOI in a 24-well plate for 2 h at 37°C, followed by PBS washing to remove unbound Ehrlichia and culturing in RF/6A medium. Infected RF/6A cells were harvested at 0, 1, 3, and 5 dpi for DNA extraction and gene-specific qPCR as described below.
PCR, qPCR, and RT-qPCR
DNA and RNA samples were purified using the DNeasy Blood & Tissue Kit (Qiagen) and the RNeasy Mini Kit (Qiagen), respectively, according to the manufacturer’s instructions. Flanking PCR for ΔripE clonality verification was performed with gene-specific primers (Supplementary Table S1) as previously described (Bekebrede et al., 2020). cDNA was synthesized from 0.5 to 1 µg of RNA using the Maxima H Minus First Strand cDNA Synthesis Kit with random hexamer primers (Thermo Fisher). qPCR and RT-qPCR analysis were performed using Maxima SYBR Green/ROX Master Mix (Thermo Fisher) according to the manufacturer’s protocols in Mx3005P (Stratagene, Waltham, MA) or the AriaMx Real-Time PCR System (Agilent, Santa Clara, CA).
In vitro infectivity, binding, and neutralization assay
To determine the binding, internalization, and infectivity of WT or ΔripE E. japonica, host cell-free bacteria were preincubated in various media or antisera at 37°C for 30–60 min, including 50% NMP diluted in PBS with or without heat inactivation at 56°C for 30 min, 50% heat-inactivated FBS, serum-free DMEM, or neutralizing antisera against RipE or Ehrlichia P28. Treated Ehrlichia was incubated with RF/6A or DH82 cells, and the bacterial infectivity was determined at 2 dpi by Ehrlichia 16S rRNA-specific qPCR. To examine bound or internalized E. japonica, two rounds of immunostaining with anti-P28 were performed at 2 h pi as described previously (Mohan Kumar et al., 2013). Briefly, to detect surface-bound Ehrlichia, PFA-fixed cells were first labeled with anti-P28 and AF488-conjugated secondary antibody diluted in nonpermeable buffer. To detect internalized Ehrlichia, the second round of staining was performed with anti-P28 and AF555-conjugated secondary antibody diluted in PGS buffer.
As obligate intracellular bacteria, Ehrlichia spp. gradually lose infectivity when released from the infected host cells and thus need to be used immediately for both in vitro and in vivo experiments following purification. Aliquots of the inoculum (Ehrlichia-infected cell culture or host cell-free Ehrlichia) were saved for DNA extraction and qPCR, and the absolute bacteria inoculum was quantified by qPCR using Ehrlichia 16S rDNA cloned into pUC19 plasmids as standards (Teymournejad et al., 2017). Therefore, the exact MOI used in the experiments was determined after the inoculation of cells or mouse infection (Bekebrede et al., 2020), causing inoculum variations among different experiments.
Mouse studies
Several mouse strains have been initially used for E. japonica, aka IOE infection (Fujita, 1994). The most frequently used strains include ddY, C57BL/6, and ICR, which show similar clinical signs with fatal E. japonica infection (Shibata et al., 2000; Sotomayor et al., 2001; Okada et al., 2003; Bekebrede et al., 2020). We previously established and demonstrated a dose-dependent infection of E. japonica in ICR mice with an LD50 of 100 bacteria per mouse, which well recapitulates the full spectrum of human ehrlichiosis (Bekebrede et al., 2020). In this study, C57BL/6 mice were used in the time-course experiments to compare the infection of WT vs. ΔripE E. japonica as C57BL/6 are inbred mice, which will likely minimize the individual mouse differences and maximize the potential difference/significance directly related to the ripE gene in the infected mouse. ICR mice are outbred strain, which represents a population of heterogenous genome background and is used mainly for the immunization (with rRipE) experiments in this study to demonstrate the immune response and protectivity of rRipE immunization in a relatively diverse population, mimicking the human populations.
C57BL/6 mice were originally from Envigo (currently Inotiv; West Lafayette, IN) and bred at OSU animal facility. ICR mice were purchased from Inotiv and used at 4–8 weeks old. Mice were i.p. or retro-orbitally (intravenous, i.v.) (Yardeni et al., 2011) injected with WT, ΔripE, ΔripE+, or WT+ E. japonica-infected DH82 cells. To test whether rRipE immunization can protect the mouse from fatal ehrlichiosis caused by E. japonica, ICR mice were first immunized with rRipE as described earlier or sham-immunized with Quil-A only and challenged with i.p. inoculation of ~11,750 WT E. japonica at 9 days post third immunization. Mice were weighted and monitored for clinical signs daily. For the E. japonica challenge, a small volume (20–50 µL) of blood samples was collected through facial vein phlebotomy at the time points shown in each figure for Ehrlichia 16S qPCR to determine the bacteria loads.
To test whether rRipE immunization can protect mice infection from human ehrlichiosis agent E. chaffeensis, C57BL/6 mice were immunized with rRipE as described above and challenged at 11 days post third immunization with i.p. inoculation of infected THP-1 cells containing ~115,000 E. chaffeensis for 5 days. Mice were euthanized by CO2 inhalation followed by cervical dislocation, and the resident peritoneal cells were collected by rinsing the peritoneal cavity with 5–10 mL of sterile PBS containing 2 mM sodium EDTA for at least three times. Blood, liver, and spleen samples were also harvested and stored at –20°C for DNA extraction, or preserved in RNAlater buffer (Qiagen, Germantown, MD) and stored at –80°C until RNA extraction.
ELISA and Western blot analysis
The wells of a 96-well flat-bottom microtiter plate (Nunc MaxiSorp, MilliporeSigma) were coated with 1 μg of rRipE or BSA, and ELISA analyses of sera from sham-vaccinated or vaccinated mice were performed as previously described (Budachetri et al., 2020). For Western blot analysis, Ehrlichia-infected DH82 cells were lysed in RIPA buffer (150 mM NaCl, 25 mM Tris-HCl, 1% Triton X-100, and 0.5% w/v sodium deoxycholate) with freshly added 1× Protease Inhibitor Cocktail (MilliporeSigma). The cell lysates and rRipE were subject to Western blotting using primary antibodies and horseradish peroxidase-conjugated secondary antibodies (SeraCare, Milford, MA). Mouse antisera against rRipE were pre-adsorbed with uninfected DH82 cell lysate transferred onto a nitrocellulose membrane (Bio-Rad, Hercules, CA) to reduce nonspecific binding. Reacting bands were visualized with Pierce ECL Western Blotting Substrate (Thermo Fisher), and images were captured and quantitated by the Amersham AI680QC gel documentation system (GE Healthcare, Marlborough, MA).
Luminescent ATP detection assay
ATP levels were determined by using the Luminescent ATP Detection Assay Kit (Abcam, Cambridge, UK) according to the manufacturer’s instructions. Briefly, host cell-free Ehrlichia and dilutions of ATP standards in DMEM were transferred into a white, flat-bottom 96-well plate (BrandTech, Wertheim, Germany), and the detergent component was added to assist cell lysis and ATP stabilization. For luminescence development, substrate solution was added and incubated for 10 min, and the ATP levels were measured by a Synergy HTX multimode reader (BioTek, Winooski, VT).
Genomic complementation and overexpression of ripE
pCis-FLAG-RipE-Gent-Himar plasmid was constructed by replacing Himar1 insertion sequences flanked by 5′- and 3′-inverted terminal repeats (ITRs) in the pCis-FLAG-Etf-2-SS-Himar A7 plasmid (Cheng et al., 2013; Yan et al., 2018) with E. japonica ripE and gentamicin resistance (Gent) genes. Gent gene was amplified from pGGA-Tuf-Cherry-Gent plasmids (Supplementary Table S1) (Hove et al., 2022). The resulting plasmid encodes FLAG-ripE and Gent genes with polycistronic expression driven by the Anaplasma marginale transcriptional regulator 1 (Tr1) promoter (Figure 6A). Plasmids were transformed into dam–/dcm– competent E. coli (New England Biolabs) and purified using the EndoFree Maxiprep Plasmid Kit (Qiagen).
E. japonica transformation was performed as described previously with minor modifications (Yan et al., 2018). Briefly, host cell-free E. japonica was resuspended in 300 mM sucrose solution, mixed with 10–15 µg of plasmid in a 0.2-cm gap cuvette (Bio-Rad), and electroporated at 2,500 V, 25 µF, and 400 Ω using a Gene Pulser Xcell Electroporation System (Bio-Rad). The transformed E. japonica was used to infect DH82 cells for 2 days and selected in DMEM medium containing 1% FBS, 4 mM l-Gln, 0.1 µg/mL cycloheximide, and 20 µg/mL gentamicin. The culture medium was replaced two to three times a week until the infection rate reached ≥80% in 2–4 weeks. Successfully transformed E. japonica became visible on cytocentrifuged slides within 17 to 31 days pi. The new insertion site in the transformed E. japonica was determined using semi-random, two-step PCR (ST-PCR) and sequencing as previously described (Cheng et al., 2013; Bekebrede et al., 2020) and then verified by insertion site-specific flanking PCR primers (Supplementary Table S1).
Statistical analysis
All statistical analyses were performed using GraphPad Prism 9 (GraphPad Software, Boston, MA). For data with only one time point, unpaired Student’s t-test or one-way analysis of variance (ANOVA) with Tukey’s multiple comparisons was used. Data with multiple time points were analyzed with repeated-measures ANOVA and Šídák’s multiple comparisons test. Kaplan–Meier survival curves were analyzed by log-rank (Mantel–Cox) test. In all tests, p < 0.05 was considered statistically significant.
Data availability statement
The original contributions presented in the study are included in the article/Supplementary Material. Further inquiries can be directed to the corresponding author.
Ethics statement
Ethical approval was not required for the studies on humans in accordance with the local legislation and institutional requirements because only commercially available established cell lines were used. The animal study was approved by Institutional Animal Care and Use Committee of the Ohio State University. The study was conducted in accordance with the local legislation and institutional requirements.
Author contributions
RC: Conceptualization, Data curation, Formal analysis, Investigation, Methodology, Software, Validation, Visualization, Writing – original draft, Writing – review & editing. ML: Formal analysis, Investigation, Methodology, Resources, Supervision, Validation, Visualization, Writing – review & editing. ND: Methodology, Visualization, Writing – review & editing. SD: Methodology, Writing – review & editing. JK: Methodology, Writing – review & editing. YR: Conceptualization, Funding acquisition, Investigation, Methodology, Project administration, Resources, Supervision, Visualization, Writing – original draft, Writing – review & editing.
Funding
The author(s) declare financial support was received for the research, authorship, and/or publication of this article. This work was partially supported by the National Institutes of Health grant R01 AI152223 to YR. The funders had no role in study design, data collection and interpretation, or the decision to submit the work for publication.
Acknowledgments
We thank Dr. Roman Ganta at the University of Missouri for providing pGGA-Tuf-Cherry-Gent plasmid and Ulrike Munderloh at the University of Minnesota (St. Paul, MN) for providing pCis-mCherry-SS-Himar-A7 plasmid.
Conflict of interest
The authors declare that the research was conducted in the absence of any commercial or financial relationships that could be construed as a potential conflict of interest.
The author(s) declared that they were an editorial board member of Frontiers, at the time of submission. This had no impact on the peer review process and the final decision.
Publisher’s note
All claims expressed in this article are solely those of the authors and do not necessarily represent those of their affiliated organizations, or those of the publisher, the editors and the reviewers. Any product that may be evaluated in this article, or claim that may be made by its manufacturer, is not guaranteed or endorsed by the publisher.
Supplementary material
The Supplementary Material for this article can be found online at: https://www.frontiersin.org/articles/10.3389/fcimb.2024.1416577/full#supplementary-material
References
Ahmed, I., Ismail, N. (2020). M1 and M2 Macrophages Polarization via mTORC1 Influences Innate Immunity and Outcome of Ehrlichia Infection. J. Cell Immunol. 2, 108–115. doi: 10.33696/immunology.2.029
Bakken, J. S., Dumler, J. S. (2000). Human granulocytic ehrlichiosis. Clin. Infect. Dis. 31, 554–560. doi: 10.1086/313948
Barnewall, R. E., Rikihisa, Y. (1994). Abrogation of gamma interferon-induced inhibition of Ehrlichia chaffeensis infection in human monocytes with iron-transferrin. Infect. Immun. 62, 4804–4810. doi: 10.1128/iai.62.11.4804-4810.1994
Bekebrede, H., Lin, M., Teymournejad, O., Rikihisa, Y. (2020). Discovery of in vivo virulence genes of obligatory intracellular bacteria by random mutagenesis. Front. Cell Infect. Microbiol. 10. doi: 10.3389/fcimb.2020.00002
Budachetri, K., Lin, M., Chien, R. C., Zhang, W., Brock, G. N., Rikihisa, Y. (2022). Efficacy and immune correlates of OMP-1B and virB2-4 vaccines for protection of dogs from tick transmission of ehrlichia chaffeensis. mBio 13, e0214022. doi: 10.1128/mbio.02140-22
Budachetri, K., Teymournejad, O., Lin, M., Yan, Q., Mestres-Villanueva, M., Brock, G. N., et al. (2020). An entry-triggering protein of ehrlichia is a new vaccine candidate against tick-borne human monocytic ehrlichiosis. mBio 11, e00895-20. doi: 10.1128/mBio.00895-20
Cain, A. K., Barquist, L., Goodman, A. L., Paulsen, I. T., Parkhill, J., van Opijnen, T. (2020). A decade of advances in transposon-insertion sequencing. Nat. Rev. Genet. 21, 526–540. doi: 10.1038/s41576-020-0244-x
Cartman, S. T., Minton, N. P. (2010). A mariner-based transposon system for in vivo random mutagenesis of Clostridium difficile. Appl. Environ. Microbiol. 76, 1103–1109. doi: 10.1128/AEM.02525-09
CDC (2024). National Notifiable Diseases Surveillance System, Weekly Tables of Infectious Disease Data (Atlanta, GA: Centers for Disease Control and Prevention, Division of Health Informatics and Surveillance).
Cheng, Z., Miura, K., Popov, V. L., Kumagai, Y., Rikihisa, Y. (2011). Insights into the CtrA regulon in development of stress resistance in obligatory intracellular pathogen Ehrlichia chaffeensis. Mol. Microbiol. 82, 1217–1234. doi: 10.1111/j.1365-2958.2011.07885.x
Cheng, C., Nair, A. D., Indukuri, V. V., Gong, S., Felsheim, R. F., Jaworski, D., et al. (2013). Targeted and random mutagenesis of Ehrlichia chaffeensis for the identification of genes required for in vivo infection. PLoS Pathog. 9, e1003171. doi: 10.1371/journal.ppat.1003171
Cheng, Z., Wang, X., Rikihisa, Y. (2008). Regulation of type IV secretion apparatus genes during Ehrlichia chaffeensis intracellular development by a previously unidentified protein. J. Bacteriol 190, 2096–2105. doi: 10.1128/JB.01813-07
Cheon, D. H., Lee, S., Yang, W. S., Hwang, S., Jang, H., Kim, M. J., et al. (2021). Optimization of a lysis method to isolate periplasmic proteins from Gram-negative bacteria for clinical mass spectrometry. Proteomics Clin. Appl. 15, e2100044. doi: 10.1002/prca.202100044
Cossé, M. M., Hayward, R. D., Subtil, A. (2018). One face of chlamydia trachomatis: The infectious elementary body. Curr. Top. Microbiol. Immunol. 412, 35–58. doi: 10.1007/82_2016_12
Dawson, J. E., Anderson, B. E., Fishbein, D. B., Sanchez, J. L., Goldsmith, C. S., Wilson, K. H., et al. (1991). Isolation and characterization of an Ehrlichia sp. from a patient diagnosed with human ehrlichiosis. J. Clin. Microbiol. 29, 2741–2745. doi: 10.1128/jcm.29.12.2741-2745.1991
Dumler, J. S., Barbet, A. F., Bekker, C. P., Dasch, G. A., Palmer, G. H., Ray, S. C., et al. (2001). Reorganization of genera in the families Rickettsiaceae and Anaplasmataceae in the order Rickettsiales: unification of some species of Ehrlichia with Anaplasma, Cowdria with Ehrlichia and Ehrlichia with Neorickettsia, descriptions of six new species combinations and designation of Ehrlichia equi and 'HGE agent' as subjective synonyms of Ehrlichia phagocytophila. Int. J. Syst. Evol. Microbiol. 51, 2145–2165. doi: 10.1099/00207713-51-6-2145
Dunning Hotopp, J. C., Lin, M., Madupu, R., Crabtree, J., Angiuoli, S. V., Eisen, J., et al. (2006). Comparative genomics of emerging human ehrlichiosis agents. PLoS Genet. 2, e21. doi: 10.1371/journal.pgen.0020021
Eedunuri, V. K., Zhang, Y., Cheng, C., Chen, L., Liu, H., Omsland, A., et al. (2018). Protein and DNA synthesis demonstrated in cell-free Ehrlichia chaffeensis organisms in axenic medium. Sci. Rep. 8, 9293. doi: 10.1038/s41598-018-27574-z
Felsheim, R. F., Herron, M. J., Nelson, C. M., Burkhardt, N. Y., Barbet, A. F., Kurtti, T. J., et al. (2006). Transformation of anaplasma phagocytophilum. BMC Biotechnol. 6, 42. doi: 10.1186/1472-6750-6-42
Fujita, H. (1994). Ehrlichial organisms isolated from Ixodes ovatus ticks and field rodents in Japan. Ann. Rep. Ohara Hosp. 37, 13–17.
Habib, S., El Andaloussi, A., Hisham, A., Ismail, N. (2016). NK cell-mediated regulation of protective memory responses against intracellular ehrlichial pathogens. PLoS One 11, e0153223. doi: 10.1371/journal.pone.0153223
Haloul, M., Oliveira, E. R. A., Kader, M., Wells, J. Z., Tominello, T. R., El Andaloussi, A., et al. (2019). mTORC1-mediated polarization of M1 macrophages and their accumulation in the liver correlate with immunopathology in fatal ehrlichiosis. Sci. Rep. 9, 14050. doi: 10.1038/s41598-019-50320-y
Hove, P., Madesh, S., Nair, A., Jaworski, D., Liu, H., Ferm, J., et al. (2022). Targeted mutagenesis in Anaplasma marginale to define virulence and vaccine development against bovine anaplasmosis. PLoS Pathog. 18, e1010540. doi: 10.1371/journal.ppat.1010540
Ismail, N., Sharma, A., Soong, L., Walker, D. H. (2022). Review: protective immunity and immunopathology of ehrlichiosis. Zoonoses (Burlingt) 2. doi: 10.15212/zoonoses-2022-0009
Ismail, N., Stevenson, H. L., Walker, D. H. (2006). Role of tumor necrosis factor alpha (TNF-alpha) and interleukin-10 in the pathogenesis of severe murine monocytotropic ehrlichiosis: increased resistance of TNF receptor p55- and p75-deficient mice to fatal ehrlichial infection. Infect. Immun. 74, 1846–1856. doi: 10.1128/IAI.74.3.1846-1856.2006
Izadi-Pruneyre, N., Wolff, N., Redeker, V., Wandersman, C., Delepierre, M., Lecroisey, A. (1999). NMR studies of the C-terminal secretion signal of the haem-binding protein, HasA. Eur. J. Biochem. 261, 562–568. doi: 10.1046/j.1432-1327.1999.00305.x
Jumper, J., Evans, R., Pritzel, A., Green, T., Figurnov, M., Ronneberger, O., et al. (2021). Highly accurate protein structure prediction with AlphaFold. Nature 596, 583–589. doi: 10.1038/s41586-021-03819-2
Kumagai, Y., Cheng, Z., Lin, M., Rikihisa, Y. (2006). Biochemical activities of three pairs of Ehrlichia chaffeensis two-component regulatory system proteins involved in inhibition of lysosomal fusion. Infect. Immun. 74, 5014–5022. doi: 10.1128/IAI.00735-06
Kumagai, Y., Matsuo, J., Hayakawa, Y., Rikihisa, Y. (2010). Cyclic di-GMP signaling regulates invasion by Ehrlichia chaffeensis of human monocytes. J. Bacteriol 192, 4122–4133. doi: 10.1128/JB.00132-10
Lampe, D. J., Akerley, B. J., Rubin, E. J., Mekalanos, J. J., Robertson, H. M. (1999). Hyperactive transposase mutants of the Himar1 mariner transposon. Proc. Natl. Acad. Sci. U.S.A. 96, 11428–11433. doi: 10.1073/pnas.96.20.11428
Le Breton, Y., Mohapatra, N. P., Haldenwang, W. G. (2006). In vivo random mutagenesis of Bacillus subtilis by use of TnYLB-1, a mariner-based transposon. Appl. Environ. Microbiol. 72, 327–333. doi: 10.1128/Aem.72.1.327-333.2006
Li, J. S., Winslow, G. M.. (2003). Survival, replication, and antibody susceptibility of Ehrlichia chaffeensis outside of host cells. Infect Immun. 71, 4229–37. doi: 10.1128/IAI.71.8.4229-4237.2003
Li, J. S., Yager, E., Reilly, M., Freeman, C., Reddy, G. R., Reilly, A. A., et al. (2001). Outer membrane protein-specific monoclonal antibodies protect SCID mice from fatal infection by the obligate intracellular bacterial pathogen Ehrlichia chaffeensis. J. Immunol. 166, 1855–1862. doi: 10.4049/jimmunol.166.3.1855
Lin, M., Grandinetti, G., Hartnell, L. M., Bliss, D., Subramaniam, S., Rikihisa, Y. (2020). Host membrane lipids are trafficked to membranes of intravacuolar bacterium Ehrlichia chaffeensis. Proc. Natl. Acad. Sci. U.S.A. 117, 8032–8043. doi: 10.1073/pnas.1921619117
Lin, M., Liu, H., Xiong, Q., Niu, H., Cheng, Z., Yamamoto, A., et al. (2016). Ehrlichia secretes Etf-1 to induce autophagy and capture nutrients for its growth through RAB5 and class III phosphatidylinositol 3-kinase. Autophagy 12, 2145–2166. doi: 10.1080/15548627.2016.1217369
Lin, M., Rikihisa, Y. (2003). Ehrlichia chaffeensis and Anaplasma phagocytophilum lack genes for lipid A biosynthesis and incorporate cholesterol for their survival. Infect. Immun. 71, 5324–5331. doi: 10.1128/IAI.71.9.5324-5331.2003
Lin, M., Xiong, Q., Chung, M., Daugherty, S. C., Nagaraj, S., Sengamalay, N., et al. (2021). Comparative analysis of genome of ehrlichia sp. HF, a model bacterium to study fatal human ehrlichiosis. BMC Genomics 22, 11. doi: 10.1186/s12864-020-07309-z
Liu, H., Bao, W., Lin, M., Niu, H., Rikihisa, Y. (2012). Ehrlichia type IV secretion effector ECH0825 is translocated to mitochondria and curbs ROS and apoptosis by upregulating host MnSOD. Cell Microbiol. 14, 1037–1050. doi: 10.1111/j.1462-5822.2012.01775.x
Luo, T., Patel, J. G., Zhang, X., Walker, D. H., McBride, J. W. (2020). Ehrlichia chaffeensis and E. canis hypothetical protein immunoanalysis reveals small secreted immunodominant proteins and conformation-dependent antibody epitopes. NPJ Vaccines 5, 85. doi: 10.1038/s41541-020-00231-1
McGill, J. L., Nair, A. D., Cheng, C., Rusk, R. A., Jaworski, D. C., Ganta, R. R. (2016). Vaccination with an attenuated mutant of ehrlichia chaffeensis induces pathogen-specific CD4+ T cell immunity and protection from tick-transmitted wild-type challenge in the canine host. PLoS One 11, e0148229. doi: 10.1371/journal.pone.0148229
Mohan Kumar, D., Yamaguchi, M., Miura, K., Lin, M., Los, M., Coy, J. F., et al. (2013). Ehrlichia chaffeensis uses its surface protein EtpE to bind GPI-anchored protein DNase X and trigger entry into mammalian cells. PLoS Pathog. 9, e1003666. doi: 10.1371/journal.ppat.1003666
Moreno, J., Nielsen, H., Winther, O., Teufel, F. (2024). Predicting the subcellular location of prokaryotic proteins with DeepLocPro. bioRxiv 2024, 2001.2004.574157. doi: 10.1101/2024.01.04.574157
Munderloh, U., Burkhardt, N., Herron, M., Oliva Chavez, A., Nelson, C., Kurtti, T. (2012). “Intracellular pathogens II: rickettsiales,” in The Way Forward: Improving Genetic Systems. (ASM Press New York, NY), 271–272.
Ohashi, N., Zhi, N., Zhang, Y., Rikihisa, Y. (1998). Immunodominant major outer membrane proteins of Ehrlichia chaffeensis are encoded by a polymorphic multigene family. Infect. Immun. 66, 132–139. doi: 10.1128/IAI.66.1.132-139.1998
Okada, H., Tajima, T., Kawahara, M., Rikihisa, Y. (2001). Ehrlichial proliferation and acute hepatocellular necrosis in immunocompetent mice experimentally infected with the HF strain of Ehrlichia, closely related to Ehrlichia chaffeensis. J. Comp. Pathol. 124, 165–171. doi: 10.1053/jcpa.2000.0447
Okada, H., Usuda, H., Tajima, T., Kawahara, M., Yoshino, T., Rikihisa, Y. (2003). Distribution of ehrlichiae in tissues as determined by in-situ hybridization. J. Comp. Pathol. 128, 182–187. doi: 10.1053/jcpa.2002.0624
Omsland, A., Sager, J., Nair, V., Sturdevant, D. E., Hackstadt, T. (2012). Developmental stage-specific metabolic and transcriptional activity of Chlamydia trachomatis in an axenic medium. Proc. Natl. Acad. Sci. U.S.A. 109, 19781–19785. doi: 10.1073/pnas.1212831109
Oren, A., Garrity, G. M. (2022). Validation List no. 206. Valid publication of new names and new combinations effectively published outside the IJSEM. Int. J. Syst. Evol. Microbiol. 72, 005422. doi: 10.1099/ijsem.0.005422
Pellerin, F. A., Caneparo, C., Pellerin, E., Chabaud, S., Pelletier, M., Bolduc, S. (2021). Heat-Inactivation of fetal and newborn sera did not impair the expansion and scaffold engineering potentials of fibroblasts. Bioengineering (Basel) 8, 184. doi: 10.3390/bioengineering8110184
Plasterer, T. N. (2000). PROTEAN. Protein sequence analysis and prediction. Mol. Biotechnol. 16, 117–125. doi: 10.1385/MB:16:2:117
Popov, V. L., Chen, S. M., Feng, H. M., Walker, D. H. (1995). Ultrastructural variation of cultured Ehrlichia chaffeensis. J. Med. Microbiol. 43, 411–421. doi: 10.1099/00222615-43-6-411
Rikihisa, Y. (1991). The tribe Ehrlichieae and ehrlichial diseases. Clin. Microbiol. Rev. 4, 286–308. doi: 10.1128/CMR.4.3.286
Rikihisa, Y. (2010). Anaplasma phagocytophilum and Ehrlichia chaffeensis: subversive manipulators of host cells. Nat. Rev. Microbiol. 8, 328–339. doi: 10.1038/nrmicro2318
Rikihisa, Y. (2015). Molecular pathogenesis of ehrlichia chaffeensis infection. Annu. Rev. Microbiol. 69, 283–304. doi: 10.1146/annurev-micro-091014-104411
Rikihisa, Y. (2021). The "Biological weapons" of ehrlichia chaffeensis: novel molecules and mechanisms to subjugate host cells. Front. Cell Infect. Microbiol. 11. doi: 10.3389/fcimb.2021.830180
Rikihisa, Y., Zhang, Y., Park, J. (1994). Inhibition of infection of macrophages with Ehrlichia risticii by cytochalasins, monodansylcadaverine, and taxol. Infect. Immun. 62, 5126–5132. doi: 10.1128/iai.62.11.5126-5132.1994
Riley, S. P., Fish, A. I., Del Piero, F., Martinez, J. J. (2018). Immunity against the Obligate Intracellular Bacterial Pathogen Rickettsia australis Requires a Functional Complement System. Infect. Immun. 86, e00139-18. doi: 10.1128/IAI.00139-18
Sharma, A. K., El Andaloussi, A., Ismail, N. (2023). Evasion of host antioxidative response via disruption of NRF2 signaling in fatal Ehrlichia-induced liver injury. PLoS Pathog. 19, e1011791. doi: 10.1371/journal.ppat.1011791
Shibata, S., Kawahara, M., Rikihisa, Y., Fujita, H., Watanabe, Y., Suto, C., et al. (2000). New Ehrlichia species closely related to Ehrlichia chaffeensis isolated from Ixodes ovatus ticks in Japan. J. Clin. Microbiol. 38, 1331–1338. doi: 10.1128/JCM.38.4.1331-1338.2000
Skipp, P. J., Hughes, C., McKenna, T., Edwards, R., Langridge, J., Thomson, N. R., et al. (2016). Quantitative proteomics of the infectious and replicative forms of chlamydia trachomatis. PLoS One 11, e0149011. doi: 10.1371/journal.pone.0149011
Sotomayor, E. A., Popov, V. L., Feng, H. M., Walker, D. H., Olano, J. P. (2001). Animal model of fatal human monocytotropic ehrlichiosis. Am. J. Pathol. 158, 757–769. doi: 10.1016/S0002-9440(10)64018-7
Teymournejad, O., Lin, M., Rikihisa, Y. (2017). Ehrlichia chaffeensis and its invasin etpE block reactive oxygen species generation by macrophages in a DNase X-dependent manner. MBio 8, e01551-17. doi: 10.1128/mBio.01551-17
Thomas, S. (2016). Development of structure-based vaccines for ehrlichiosis. Methods Mol. Biol. 1403, 519–534. doi: 10.1007/978-1-4939-3387-7_29
Tipples, G., McClarty, G. (1993). The obligate intracellular bacterium Chlamydia trachomatis is auxotrophic for three of the four ribonucleoside triphosphates. Mol. Microbiol. 8, 1105–1114. doi: 10.1111/j.1365-2958.1993.tb01655.x
Wang, Y., Wei, L., Liu, H., Cheng, C., Ganta, R. R. (2017). A genetic system for targeted mutations to disrupt and restore genes in the obligate bacterium, Ehrlichia chaffeensis. Sci. Rep. 7, 15801. doi: 10.1038/s41598-017-16023-y
Weiss, E., Williams, J. C., Dasch, G. A., Kang, Y. H. (1989). Energy metabolism of monocytic Ehrlichia. Proc. Natl. Acad. Sci. U.S.A. 86, 1674–1678. doi: 10.1073/pnas.86.5.1674
Wellman, M. L., Krakowka, S., Jacobs, R. M., Kociba, G. J. (1988). A macrophage-monocyte cell line from a dog with Malignant histiocytosis. In Vitro Cell Dev. Biol. 24, 223–229. doi: 10.1007/BF02623551
Winslow, G. M., Bitsaktsis, C., Yager, E. (2005). Susceptibility and resistance to monocytic ehrlichiosis in the mouse. Ann. N Y Acad. Sci. 1063, 395–402. doi: 10.1196/annals.1355.071
Yan, Q., Lin, M., Huang, W., Teymournejad, O., Johnson, J. M., Hays, F. A., et al. (2018). Ehrlichia type IV secretion system effector Etf-2 binds to active RAB5 and delays endosome maturation. Proc. Natl. Acad. Sci. U.S.A. 115, E8977–E8986. doi: 10.1073/pnas.1806904115
Yardeni, T., Eckhaus, M., Morris, H. D., Huizing, M., Hoogstraten-Miller, S. (2011). Retro-orbital injections in mice. Lab. Anim. (NY) 40, 155–160. doi: 10.1038/laban0511-155
Zhang, T., Chien, R. C., Budachetri, K., Lin, M., Boyaka, P., Huang, W., et al. (2024). Ehrlichia effector TRP120 manipulates bacteremia to facilitate tick acquisition. mBio 15, e0047624. doi: 10.1128/mbio.00476-24
Zhang, W., Lin, M., Yan, Q., Budachetri, K., Hou, L., Sahni, A., et al. (2021). An intracellular nanobody targeting T4SS effector inhibits Ehrlichia infection. Proc. Natl. Acad. Sci. U.S.A. 118, e2024102118. doi: 10.1073/pnas.2024102118
Keywords: Ehrlichia, RipE, bacterial ATP, genetic complementation, transposon mutant, mouse, CtrA
Citation: Chien RC, Lin M, Duan N, Denton S, Kawahara J and Rikihisa Y (2024) RipE expression correlates with high ATP levels in Ehrlichia, which confers resistance during the extracellular stage to facilitate a new cycle of infection. Front. Cell. Infect. Microbiol. 14:1416577. doi: 10.3389/fcimb.2024.1416577
Received: 12 April 2024; Accepted: 28 August 2024;
Published: 01 October 2024.
Edited by:
Joseph James Gillespie, University of Maryland, United StatesReviewed by:
Jere W. McBride, University of Texas Medical Branch at Galveston, United StatesHwan Kim, Stony Brook University, United States
Copyright © 2024 Chien, Lin, Duan, Denton, Kawahara and Rikihisa. This is an open-access article distributed under the terms of the Creative Commons Attribution License (CC BY). The use, distribution or reproduction in other forums is permitted, provided the original author(s) and the copyright owner(s) are credited and that the original publication in this journal is cited, in accordance with accepted academic practice. No use, distribution or reproduction is permitted which does not comply with these terms.
*Correspondence: Yasuko Rikihisa, cmlraWhpc2EuMUBvc3UuZWR1