- 1Department of Molecular, Cellular & Developmental Biology, University of Colorado Boulder, Boulder, CO, United States
- 2Department of Obs & Gynae, Northwestern University, Chicago, IL, United States
- 3Department of Biophysics, All India Institute of Medical Science, New Delhi, India
- 4Department of Microbiology, Immunology, and Infectious Diseases, College of Medicine and Medical Sciences, Arabian Gulf University, Manama, Bahrain
- 5Department of Medical Microbiology & Immunology, Ras Al Khaimah (RAK) College of Medical Sciences, Ras Al Khaimah (RAK) Medical and Health Sciences University, Ras Al Khaimah, United Arab Emirates
- 6Department of Biology, College of Science University of Hail, Hail, Saudi Arabia
- 7Department of Clinical Laboratory Sciences, College of Applied Medical Sciences, King Khalid University, Abha, Saudi Arabia
A complex structure known as a biofilm is formed when a variety of bacterial colonies or a single type of cell in a group sticks to a surface. The extracellular polymeric compounds that encase these cells, often consisting of proteins, eDNA, and polysaccharides, exhibit strong antibiotic resistance. Concerns about biofilm in the pharmaceutical industry, public health, and medical fields have sparked a lot of interest, as antibiotic resistance is a unique capacity exhibited by these biofilm-producing bacteria, which increases morbidity and death. Biofilm formation is a complicated process that is controlled by several variables. Insights into the processes to target for the therapy have been gained from multiple attempts to dissect the biofilm formation process. Targeting pathogens within a biofilm is profitable because the bacterial pathogens become considerably more resistant to drugs in the biofilm state. Although biofilm-mediated infections can be lessened using the currently available medications, there has been a lot of focus on the development of new approaches, such as bioinformatics tools, for both treating and preventing the production of biofilms. Technologies such as transcriptomics, metabolomics, nanotherapeutics and proteomics are also used to develop novel anti-biofilm agents. These techniques help to identify small compounds that can be used to inhibit important biofilm regulators. The field of appropriate control strategies to avoid biofilm formation is expanding quickly because of this spurred study. As a result, the current article addresses our current knowledge of how biofilms form, the mechanisms by which bacteria in biofilms resist antibiotics, and cutting-edge treatment approaches for infections caused by biofilms. Furthermore, we have showcased current ongoing research utilizing the CRISPR/Cas9 gene editing system to combat bacterial biofilm infections, particularly those brought on by lethal drug-resistant pathogens, concluded the article with a novel hypothesis and aspirations, and acknowledged certain limitations.
1 Introduction
It has long been known that microbiological infections exist, and since then, scientists have worked tirelessly to eradicate both established and new infections that cause infectious diseases and create antimicrobial drugs to cure and get rid of the contagious illness. Antimicrobials are a diverse group of substances that can fight a broad range of pathogenic microbes, including bacteria, protozoa, fungi, viruses and parasites (Dutt et al., 2022; Ahmad et al., 2023). From the early 20th century, these substances have been employed to treat infected individuals and they have greatly assisted in reducing the most infectious rates of morbidity and mortality (Dutt et al., 2022). In 1928, penicillin was discovered by Alexander Fleming, and it entered clinical usage in the 1940s at the perfect moment for the second world war (Gaynes, 2017). In just four years of usage, the first strains of penicillin-resistant bacteria, a new species of bacteria appeared, which led to the development of antibiotic resistance. As a result, AMR has quickened and expanded to include more harmful species because of the continuous exposure and indiscriminate use of antibiotics in clinical and farming environments. Numerous modern antibiotics have lost their efficacy because of the development of AMR and scientists are working continuously to comprehend how AMR works to create new antimicrobials (Dutt et al., 2022).
Looking towards bacteria, it feels that the capacity to form biofilm is shared by mostly all the bacteria and it is considered a universal attribute. In biofilms, the extracellular matrix formed by the cells themselves holds the groups of bacteria or multicellular communities together. Different bacteria use different ways to create biofilms, these mechanisms soften depending on the environment in which they are found as well as strain-specific characteristics (Percival et al., 2011). He was Antonie van Leeuwenhoek who first saw “animalcules “on his teeth in the 17th century. The bottle effect was first noted in marine microorganisms in 1940. This demonstrates that germs proliferate more frequently on surfaces (Percival et al., 2011; Dutt et al., 2022).
Then, in 1943, Zobell created biofilms and discovered that the number of bacteria on surfaces was higher than that of the surrounding seawater (Zobell, 1943). Today, in scientific language, we define biofilms as the microbial communities that are attached to a substrate and covered in extracellular polymeric substance (EPS), which is secreted by these bacteria (Costerton et al., 1987; Donlan, 2001; Zuberi et al., 2017b). Microbial biofilms can be found on many surfaces in aquatic environments, damp structures, plant roots, human tooth or dental implants, catheters, medical equipment, sutures, etc. They can even be found in human and animal tissues in pathogenic forms that can release toxins into the surrounding extracellular matrix (Donlan, 2001; Percival et al., 2011). They can evade the human response (Cangui-Panchi et al., 2022, Cangui-Panchi et al., 2023).
In addition, biofilms can be found in symbiotic form in aquatic bodies, wastewater filters and the alimentary canals of humans and animals (Costerton et al., 1981; Dutt et al., 2022). Because of their resistance to antibiotics, biofilms, which are the most common in natural settings, can infect both humans and animals (Mah, 2012; Sinclair, 2019). Thus, it is crucial to comprehend the mechanism of biofilm-led resistance to antibiotics. This review will cover antimicrobial resistance (AMR), specifically the mechanism underlying biofilm-led AMR, possible pharmacological or drug candidates and present modalities that are used to target bacteria within the biofilm. We will also focus on current ongoing research like the CRISPR/Cas9 gene editing system to fight bacterial biofilm infections.
2 Revisiting bacteria biofilm ultrastructural and its antibiotic survival strategies
Bacterial biofilms are groups of sessile microbes that are entrenched and connected to substratum, within the self-generated non-crystalline pool of the extracellular matrix (Zuberi et al., 2017a, Zuberi et al., 2017b). These bacterial communities are distinct from planktonic ones concerning several methods like transcription, gene expression and growth rate since they live in various stressed environments with increased cell density, osmolarity, nutrient shortage, etc (Sharma et al., 2019). Bacterial biofilm is a dynamic three-dimensional structure that is processed by a heterogenous group of bacterial communities and the bacteria living in these dynamic strictures are shielded from many environmental stressors, including desiccation, immune attack, protozoan ingestion, antimicrobial target, etc., hence making them more resistant and superior over the planktonic form of bacteria (Wilkins et al., 2014; Sharma et al., 2019). The process of developing this three-dimensional structure is a multi-step procedure. It begins when bacteria first attach themselves to the surface and create an unbreakable bond that is followed by other bacterial colonization (Sharma et al., 2019). The bacteria that colonize first are called primary colonizers in biofilm and the secondary colonizers are the ones that attach to the primary colonizers (MaChado and Cerca, 2015). This stage led to changes in the expression of genes or proteins by bacteria. The second stage of the exponential growth phase is characterized by the secretion of exopolysaccharides and the creation of water channels by these bacteria, enabling the nutrient supply to mature biofilms. Finally, the cell surface detachment begins the relaunched or recycled biofilm development on the fresh surfaces (Sharma et al., 2019). This detachment step is usually triggered by stress factors such as a limited nutritional environment and antibiotics. Usually, the cells in the inner or deep layers of biofilm become latent or dormant cells, while the ones on the top layers are metabolically active.
Biofilms can host multiple bacterial species and can lead to a complex system that can accommodate bacterial cell densities between 108–1011 cells g-1 wet weight (Morgan-Sagastume et al., 2008). Water makes up to 97% of its matrix major constituents and the other contents include proteins, soluble or gel-forming polysaccharides, extra-cellar DNA (eDNA) and non-soluble elements like cellulose, amyloids, pili, fimbria and flagella that deliver structural and functional properties to these biofilms (Flemming and Wingender, 2010; Flemming et al., 2016).
Because of their increased resistance to antibiotics and disinfectants, bacterial biofilms are a major contributing factor to chronic infections. They can also interfere with phagocytosis and other immune system functions. As a result, microorganisms within biofilms become less vulnerable to various antibiotic medicines, hence posing an imminent challenge in the field of therapeutics (Høiby et al., 2010; Amato et al., 2014; Flemming et al., 2016; Dutt et al., 2022).
3 The elucidation of mechanisms governing bacterial biofilm resistance and its dynamics
The term antimicrobials is used for substances that kill microorganisms, inhibit their growth, and prevent or treat diseases or infections in animals, humans, and plants. They included a wide variety of antivirals, antifungals, and antiparasitics. The capacity of microorganisms to withstand an antimicrobial at a higher dose for an extended duration is known as antimicrobial resistance and is usually measured in terms of its minimum inhibitory concentration (Brauner et al., 2016; Ahmad et al., 2017, Ahmad et al., 2018). In biofilms, antibiotic tolerance or resistance may occur simultaneously. Through the introduction of foreign genetic material that codes for resistance genes by horizontal gene transfer (HGT) between the bacterial cells of the biofilm or through genetic mutation, microorganisms within the biofilm develop antibiotic resistance. As a subset of antimicrobial resistance (AMR), antibiotic resistance (ABR) occurs when bacteria develop resistance to antibiotics despite the drug’s effectiveness against them (Dutt et al., 2022). Usually, antibiotic resistance can be acquired extrinsically, adaptively or intrinsically. The classic example of intrinsically acquired resistance is the susceptibility of gram-positive bacteria against antibiotics like daptomycin or vancomycin over gram-negative bacteria due to the difference in their cell wall composition. Conversely, acquired resistance results from either mutation or horizontal gene transfer (HGT). In addition, during adaptation, the bacteria may quickly modify their pattern of gene expression and translation in response to other environmental conditions or stimuli including stress (Blair et al., 2015; Dutt et al., 2022).
On the other hand, tolerance refers to a microorganism’s capability to endure antibiotics at concentrations greater than their inhibitory effect for a specific period (Arzanlou et al., 2017; Hall and Mah, 2017). For a brief duration, tolerance is a form of adaptation that represents shifts in cellular activity from active to latent state. Like antibiotic entrapment to the extracellular polymeric substance (EPS) in the absence of target attachment induces tolerance and causes bacteria cell dormancy. Persistence is an exceptional form of tolerance, where persisters refer to the tolerant form of cells within that bacterial population that can survive the antibiotics but can be killed at long exposure (Wilmaerts et al., 2019). Biofilm-mediated resistance is a multifaceted type of resistance that necessitates tolerance in addition to the antibiotic resistance mechanism. Furthermore, the state and developmental stage of the biofilm, its growth conditions, and the microbial species present within it also contribute to this process (Hall and Mah, 2017; Dutt et al., 2022). Some of the mechanisms of antimicrobial resistance (AMR) that are related to biofilm-mediated resistance are limiting the permeability or blocking access to antimicrobials; altering the targets of antibiotics through mutations; and breaking down the antimicrobials through enzymatic hydrolysis or chemical alteration. In this review, we tried to discuss each of them under the following headings and they have been vividly illustrated in Figure 1.
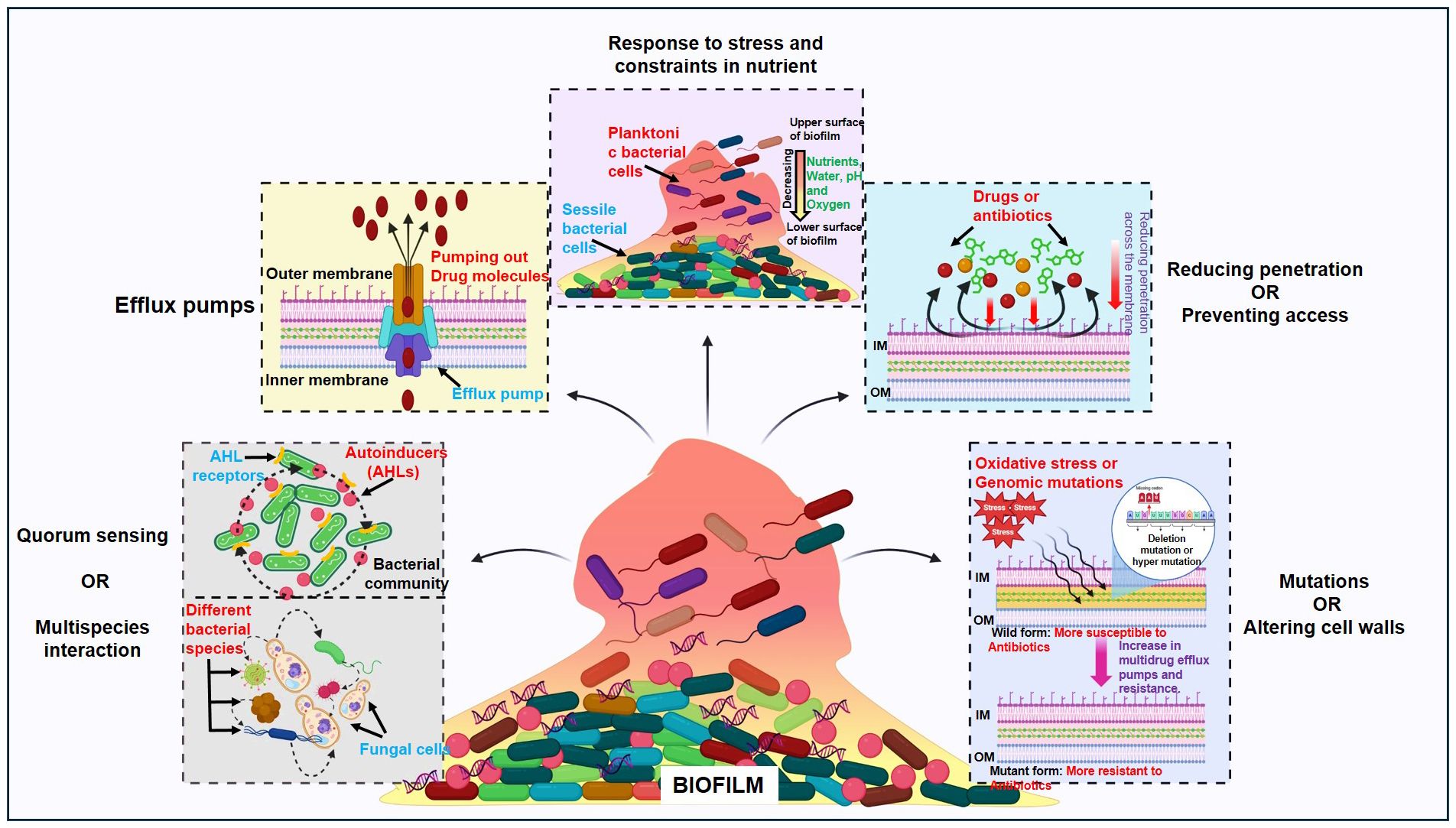
Figure 1 Regulatory pathways controlling biofilm bacterial resistance: Quorum sensing or multispecies interaction, efflux pumps, response to stress and constraints in nutrients, reducing penetration or preventing access and mutations or altering cell walls.
3.1 Quorum sensing and multispecies interaction
Bacterial; cells communicate with each other through a mechanism called quorum sensing that includes the synthesis, section, and reaction in response to the extracellular signalling molecules that are called autoinducers (AIs). As bacterial population density rises, these AIs build up the environment, and the bacterial cell uses this to carry out the actions that are advantageous when carried out by bacterial colonies operating simultaneously (Arevalo-Ferro et al., 2003; Di Cagno et al., 2011; Rutherford and Bassler, 2012; Zuberi et al., 2017b). Bioluminescence, competence, biofilm synthesis and virulence factor synthesis are some of the major processes regulated by QS (Rutherford and Bassler, 2012). To start the process of gene transcription for surface proteins, virulence proteins or proteins related to biofilm formation, these auto-inducers are secreted by bacteria and identified by other bacteria by their cell surface receptors. The main types of autoinducers include the acyl-homoserine lactones (AHL) that are found in Gram-negative bacteria, secondarily modified oligopeptides that are associated with Gram-positive bacteria, and a class of 4,5-dihydroxy-2,3-pentonedione-derived signal molecules termed autoinducer-2 (AI-2) that are found in both Gram-negative as well as the Gram-positive bacteria (Li and Nair, 2012). It has been demonstrated by different studies that the bacteria-bearing genes related to quorum sensing like luxS, lasR, and rhlR are more resistant to the antibacterial treatment (Dutt et al., 2022). The classic example comes from P. aeruginosa biofilms lacking genes rhlR and lasR, which were found more tobramycin-sensitive than their biofilms of the wild type (Bjarnsholt et al., 2005). Similarly, it was found that S. aureus lacking QS-specific agrD was less resistant than its matched wild-type (Yarwood et al., 2004). Moreover, E. faecalis fsrA and gelE mutants for quorum sensing and its controlled protease were less able to produce biofilms when antibiotics like gentamycin or daptomycin were present (Dale et al., 2015).
Multispecies interaction is another factor that drives antibiotic tolerance, for instance, it was found that the polymicrobial biofilms of Finogoldia magna, S. aureus and E. faecalis were twice as resistant as P. aeruginosa mono-species biofilm (Dalton et al., 2011). Similarly, M. catarrhalis released beta-lactamase in a dual-species model that shielded S. pneumonia against amoxicillin (Budhani and Struthers, 1998; Perez et al., 2014). Additionally, research has been done on the relationship that develops among fungi and bacteria in a multispecies biofilm. In C. albicans and S. aureus biofilms, the fungal matrix ingredient beta-1,3 glucan, which is thought to function as a barrier against vancomycin, increased the resistance of Staphylococcus to the antibiotic (Adam et al., 2002; Harriott & Noverr, 2009). Additionally, it was discovered that C. albicans can produce more alcohol, which in turn increases P. aeruginosa biofilm development (Chen et al., 2014).
3.2 Efflux pumps
Nearly all bacterial species contain efflux pumps. Bacterial efflux pumps are grouped into five families depending on attributes such as composition, substrates, energy sources and several transmembrane-spanning regions. They include the resistance-nodulation-division (RND) family, the ATP (adenosine triphosphate)-binding cassette (ABC) superfamily, the major facilitator superfamily (MFS), the multidrug and toxic compound extrusion (MATE) family and the small multidrug resistance (SMR) family (Sun et al., 2014). This process gives bacterial cells resistance because it transfers a drug in and out of the cell despite adhering to an intracellular target (Poole, 2007). According to certain theories, planktonic resistance in P. aeruginosa to low concentrations of ofloxacin is caused by several multidrug efflux pump systems including MaxAB-OprM (Brooun et al., 2000). It is thought that PA1875–1877, a significant multidrug efflux pump, plays a role in the biofilm resistance of P. aeruginosa (Zhang and Mah, 2008). The deletion of PA1875, PA1876 and PA1876 increased the sensitivity of biofilm to certain antibiotic drugs like gentamicin, ciprofloxacin and tobramycin by two to four times; however planktonic cells susceptibility was not significantly impacted (Zhang and Mah, 2008). Likewise, it was also claimed that the MexCD-OprJ or MexAB-OprM efflux pumps were also the cause of P. aeruginosa biofilms resistance to azithromycin (Gillis et al., 2005; Pamp et al., 2008).
3.3 Response to stress and constraints in nutrition
The Gradients of water, nutrients, pH, waste product dispersion and signalling molecules are usually dependent and determined by the three-dimensional dynamic structure and architecture of biofilms (de Beer et al., 1994a; Anderl et al., 2000; Borriello et al., 2004; Stewart and Franklin, 2008; Williamson et al., 2012; Stewart et al., 2016). It is seen that the cells close to the surface of a biofilm microcolonies use up most of the available nutrients, creating an impoverished region deeper down, that develops a variety of psychological states including anaerobic, microaerobic, aerobic and fermentative conditions (Stewart and Franklin, 2008; Flemming et al., 2016; Dutt et al., 2022). This process also affects the growth of those underlying cells and leads to dormant cells due to the scarcity of oxygen and nutrients in the lower layers of biofilm. In the other study, they discovered a unique characteristic when they cultured multidrug resistance E. faecalis strains straight from the biofilm stage after they had been stored at 70°C for 16 to 18 months. According to them, the re-cultured bacterial cells were found only to grow after 60–72 hours of incubation at 37°C and they turned out to be extra resistant to the antibiotics (Borriello et al., 2006). Tolerance to antibiotics that target the protein synthesis process or DNA gyrase like ciprofloxacin or tobramycin is thought to arise from the lower metabolic activity and possible dormancy in cells in the oxygen-deprived area of biofilms (Borriello et al., 2006; Dutt et al., 2022).
It is well known that colistin, which works on the cell membrane, can damage slowly regrowing cells (Haagensen et al., 2007). On the other hand, the observation of colistin-tolerant cells in oxygen-rich locations causes doubts about the relationship between antibiotic tolerance and the slow growth rate of cells (Pamp et al., 2008; Chiang et al., 2012). Yogesh and Anjali investigated this fact in 2021, discovering that colistin did not affect biofilm-forming E. faecalis (Dutt et al., 2022). In regions with high levels of oxygen, observable cellular activity and protein production have also been seen (Walters et al., 2003; Werner et al., 2004). P. aeruginosa can maintain anaerobic conditions through denitrification and fermentation. Supplementing nitrate or L-arginine might boost metabolic activity in nutrient-deprived regions, making them more susceptible to tobramycin as well as ciprofloxacin (Borriello et al., 2006).
3.4 Reducing penetration or preventing access
The structure and makeup of the extracellular matrix (ECM) can significantly impact antibiotic penetration, its entry into cells and ultimately, antibiotic effectiveness through gradients of dispersion (de Beer et al., 1994b). Antibiotic diffusion is also dependent on its interaction with the ECM constituents. Extracellular DNA, for instance, strengths Pseudomonas biofilm resistance to aminoglycosides but not to fluoroquinolones or beta-lactam antibiotics (Mulcahy et al., 2008; Doroshenko et al., 2014; Wilton et al., 2016). Similarly, eDNA strengthens the Staphylococcus epidermis biofilm’s ability to resist glycopeptides. It has been observed that negatively charged aminoglycosides (tobramycin) and glycopeptides (vancomycin) bind to negatively charged eDNA (Doroshenko et al., 2014). Additionally, it has been shown that the interaction between eDNA and vancomycin is 100 times stronger than that between peptides D-Ala-D-Ala and vancomycin in peptidoglycan precursors, that could lead to build-up eDNA in the ECM (Doroshenko et al., 2014). A multi-species biofilm can also contain antibiotic-modifying enzymes that can be released and found in the extracellular matrix (ECM), which other susceptible bacterial species can employ. As an example, Moraxella catarrhalis releases beta-lactamases that shield S. pneumonia and H. sinfluenza from ampicillin and amoxicillin respectively (Armbruster et al., 2010; Perez et al., 2014). Thus, to conclude, the biofilm structure and its architecture can change both the exposure of cells and the diffusion of drugs or antibiotics through them.
3.5 Mutations and altering cell walls via enzymatic process
Genomic mutations can lead to antibiotic resistance, even without strong selective stress or pressure. Mutations occur at a rate of 10-10-10-9 per nucleotide per generation in most bacteria (Woodford and Ellington, 2007; Schroeder et al., 2018). It has been reported that oxidative stress-causing agents can also accelerate the mutation rate, causing multidrug efflux pumps, mutagenesis, and resistance (Van Acker and Coenye, 2017). Defects in mutS, mutL, and uvrD genes can increase mutation frequency up to 100-fold according to the report (Leong et al., 1986; Schaaper and Dunn, 1987). Bacteria when possessing hypermutators, which can gain advantageous mutations under selection stress and may cause antimicrobial resistance, provided an excellent example of evolutionary mutations (Eliopoulos and Blazquez, 2003). This specific phenotype, which is resistant to ciprofloxacin and rifampicin, has also been reported in Pseudomonas biofilms (Driffield et al., 2008). In addition to the previously listed phenotypic traits, hypermutations have also been observed in S. aureus and H. influenza isolates from cystic fibrosis infections, but not in Enterobacteriaceae isolates from acute UTIs; this suggests that hypermutability is preferred in specific contexts (Prunier et al., 2003; Román et al., 2004; Kovacs et al., 2013). For bacteria like E. faecalis and S. aureus to produce biofilms, the dltA genes are essential (Gross et al., 2001; Fabretti et al., 2006). This has been shown by the reduction of vancomycin resistance in the strains of S. aureus following the deletion of the dltA gene. The dltABCD operon, crucial for D-alanylation of teichoic acid in gram-positive species was identified as a biofilm-specific gentamicin tolerance gene in streptococcus mutants, a dental pathogen causing infective endocarditis (Peschel et al., 2000; Neuhaus and Baddiley, 2003; Nilsson et al., 2016).
4 Exploring health conditions linked to the presence and impact of bacterial biofilm resistance
Roughly 80% of recurring and chronic microbial illnesses are caused by biofilms of bacteria. Biofilm-containing microbial cells have demonstrated 10–1000 times greater tolerance to drugs than planktonic cells. Infections linked to biofilms can be widely separated into two categories (Mah, 2012). Either biofilm might grow on the abiotic surfaces that include knee replacements, implants, dental units, catheters, contact lenses, screws, pins or prosthetic valves and joints or they are host tissue related that leads to chronic wounds, endocarditis, cystic fibrosis lings or chronic otitis media (Donlan, 2001; Burmølle et al., 2010). The infections related to the urinary tract and bloodstream are usually caused via a biofilm that initially developed on the medical implants associated with them and the only way to treat such infection is to get rid of those implants that not only raise the price of the therapy, but it also causes other health-related issues to the patients (Costerton et al., 2005; Sharma et al., 2019). Several of the primary infections associated with bacterial biofilms that are responsible for human illness are mentioned in Table 1.
5 Understanding the current impact of antibiotic resistance and its governing mechanisms
S. aureus, S. pneumoniae, K. pneumoniae, P. aeruginosa, E. coli and E. faecium are the most persistent and common multidrug-resistant bacteria that are linked to significantly high rates of death and morbidity worldwide (Dutt et al., 2022). Additionally, according to some reports, high levels of antibiotic resistance have also been linked to cancer-related neutropenia. Furthermore, because of biofilm biofilm-forming tendency of antibiotic-resistant bacteria, managing and treating newborn sepsis becomes a challenge in many clinical settings. In healthcare and hospital settings almost all procedures, surgeries, transplantation and intensive care are not typically carried out without antibiotics. However, with the increasing failure of first and second-generation antibiotics, the demands of expensive and time-consuming research for the next generation of antibiotics are increasing. Treating such resistant bacteria-mediated infections is complicated and requires the use of more costly and hazardous alternative treatments or higher doses (Magiorakos et al., 2012; Nesher and Rolston, 2014). Below we discuss some mechanisms that bacteria employ to confer antibiotic resistance:
5.1 Altering or safeguarding targets
Antibiotics are made with the specific intention of binding to their targets with a high degree of affinity and interfering with their regular activities. When targets modify structurally, antibiotics attach to them less effectively. Moreover, mutation also plays an important role in developing resistance by modifying the antibiotic target (e.g. the single nucleotide polymorphism mutation in the gene that codes the target). For instance, Rifampin resistance arises from an amino acid change in the rpoB gene. This mutation results in a decrease in rifampin binding affinity for its target, whereas transcription persists (Munita and Arias, 2016). In other bacteria like streptococcus pneumonia, the secretion of some binding proteins like penicillin-binding proteins (PBPs) lessens their target binding affinity with many beta-lactam antibiotics (Nagai et al., 2002). Moreover, antibiotic resistance can also be attained through post-translational alteration of genes that don’t include any mutation like the 16sRNA methylation in erythromycin ribosome methylase (erm) gene family leads to the modification of the drug binding sites that consequently prevents the binding of macrolides, lacosamide’s or streptogramin with 16sRNA (Kumar et al., 2014). Also, the A2503 residue methylation through chloramphenicol florfenicol resistance genes prevents and inhibits the binding efficiency of antibiotics like oxazolidinones, lacosamide, phenicol’s or stereograms with the target (23S rRNA) (Long et al., 2006).
5.2 Cell membrane or cell wall adaptation/alteration
In the case of Enterobacteriaceae, the bacterial membrane decreased permeability to carbapenems and that results in making them resistant to carbapenems antibiotics. Mosty in this family the resistance mechanism is mediated by the downregulation of porins (OmpC and OmpF) expression or due to its replacement by more selective porins or membrane channels (Baroud et al., 2013). Likewise, the lowered permeability of antibiotics like erythromycin, azithromycin, clarithromycin or azithromycin makes V. cholerae, S. Enteric and P. aeruginosa-like gram-negative bacteria resistant. Furthermore, by using efflux pumps bacteria also force antibiotics out into the extracellular matrix, which stops them from reaching their intended target (Wiese et al., 1999).
5.3 Ribosome protection
Certain bacteria develop a resistance mechanism called ribosome protection. As an inhibitor of bacterial protein synthesis, tetracycline causes the production of ribosome protection proteins by the bacteria, which attach to the ribosome target and stop tetracycline from binding to the ribosome (Roberts, 2005; Dutt et al., 2022). In these situations, the synthesis of ribosome protective proteins allows bacteria to proliferate despite the presence of tetracycline.
5.4 Enzymatic breakdown of antimicrobial substances
Antibiotics can also be rendered inactive by bacteria, which can likewise change their structural makeup and stop them from entering cells. This process is mainly through hydrolysis. Enzymes like chloramphenicol acetyltransferase or carbapenems degrade antibiotics including macrolides, aminoglycosides, phenicol as well as β-lactams (Dutt et al., 2022). Both the extended and early spectrum β-lactamases are active enzymes against β-lactams and oxyimino-cephalosporins (Lynch et al., 2013). TEM-1 β-lactamase and SHV-1 (sulfhydryl variable active site) enzymes, which are expressed by the plasmids in E. coli are good examples of such degrading enzymes that hydrolyse the multiple kinds of extended-spectrum cephalosporins. Furthermore, it is also reported that the modification or changes in the functional group of antimicrobials caused by degrading enzymes also contribute to antibiotic resistance.
6 Present treatment modalities targeting bacteria with biofilm-forming abilities
The fact that biofilm functions as a self-motivation mechanism during the pathogenic process helps to explain it. There have been several approaches and methodologies that have been employed to understand its antibiotic resistance nature and to target its residing bacteria. Some of its common approaches are the use of natural products, plant extracts, surface coatings, antibiotics, hydrogels, peptides, lasers during photodynamic therapy (PDT) and nanomedicines or nanoparticles (Hernández-Sierra et al., 2008; Lonn-Stensrud et al., 2008; Kolodkin-Gal et al., 2010; Hochbaum et al., 2011; Iannitelli et al., 2011; Kulshrestha et al., 2014; Muñoz-Egea et al., 2016; Misba and Khan, 2018; Misba et al., 2019). The dramatic portrayal of these treatment strategies i.e. Photodynamic therapy (PDT), small molecules as polypeptides or quorum sensing inhibitors, antibiotics, hydrogels and nanoparticles are shown in Figure 2.
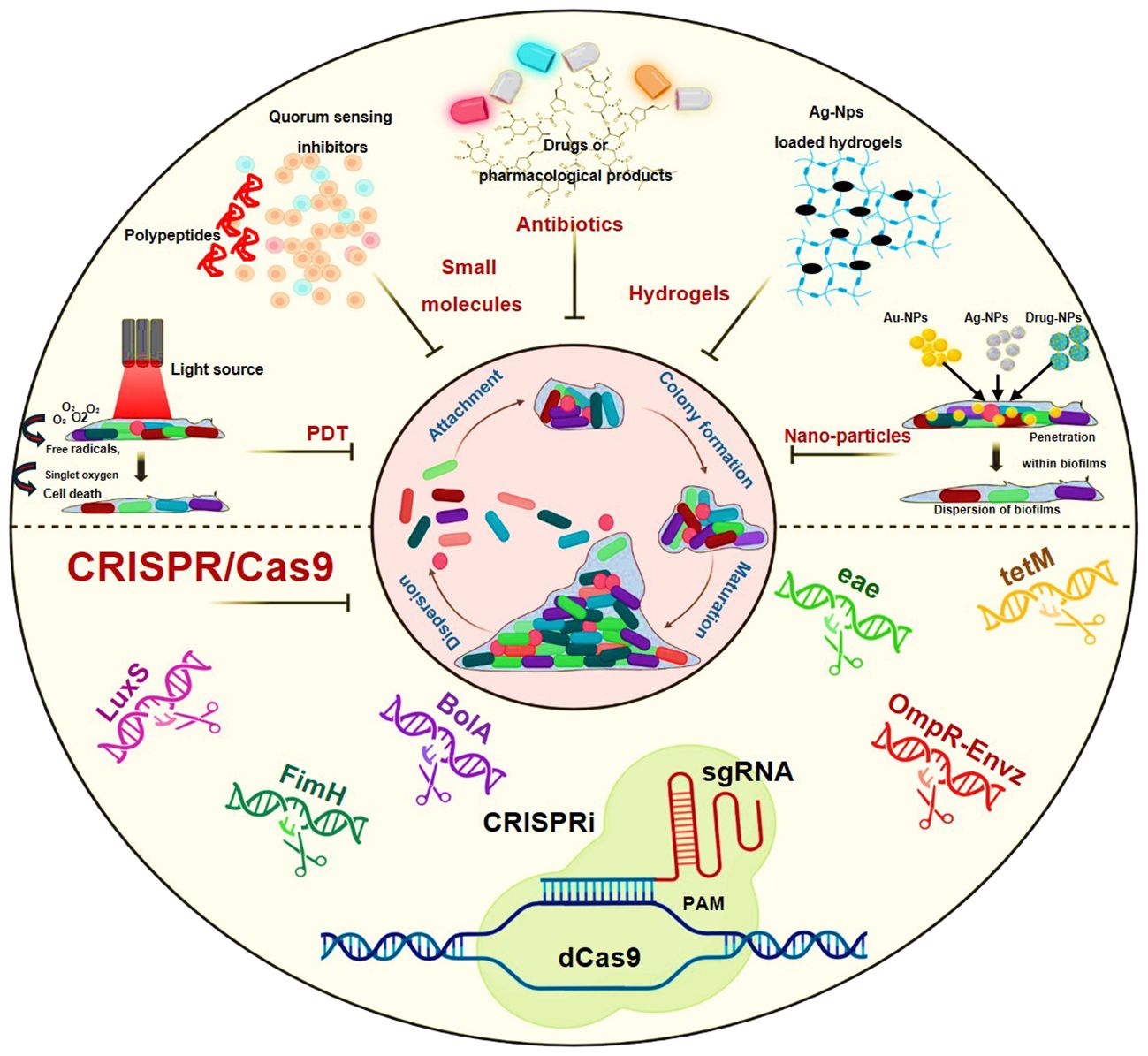
Figure 2 Schematic representation showing treatment modalities against biofilm-mediated infections: Photodynamic therapy (PDT), Small molecules as polypeptides or quorum sensing inhibitors, Antibiotics, Hydrogels, nanoparticles and the CRISPR/Cas9.
The present treatment modalities utilize mainly the traditional methods to combat these biofilms that mainly focus on the dispersal of biofilm or its eradication or inhibition through antibiotics, small molecules inhibitors, enzymes, quorum sensing inhibitors, hydrogels etc (Dutt et al., 2022). Table 2 shows the list of potential drug candidates and small molecules that are found effective in the mitigation of biofilm-related infections.
Biofilm growth on implanted medical devices, prosthetic surfaces or biomaterials may be controlled by modifying the attachment surface, like coating the external surfaces. Many coating materials and biomaterials have been established, that make the target surface unfavourable for bacterial attachment. Moreover, the use of therapeutic agents and inhibitors against biofilm formation on dental implants or dental filling material has also demonstrated positive effects in combating biofilm-associated infections (Sharma et al., 2019).
As mentioned earlier too, the bacteria in biofilm form are more resistant and tolerant to antibiotics as compared to their planktonic form. Quorum sensing is a principal pathway that leads to biofilm-mediated bacterial maintenance and survival. Hence certain strategies that lead to the dispersal of biofilm or targeting quorum sensing mechanism have exhibited promising results in addressing biofilm-mediated infections (Lonn-Stensrud et al., 2008; McDougald et al., 2012; Guilhen et al., 2017; Roy et al., 2018; Sharma et al., 2019). Moreover, co-treatment or combination therapy that consists of antibiotics or drugs along with a biofilm dispersal agent has also demonstrated efficacy in these cases but has some limitations due to inappropriate concentration of both components and still research is going on (Barraud et al., 2006; Marvasi et al., 2014; Reffuveille et al., 2015; Roizman et al., 2017).
With the new research development and advancements in technologies, the future proposed methods to combat biofilm-mediated antibiotic infections include the use of nanoparticles, antimicrobial peptides, photodynamic therapy and implementation of gene editing technologies like CRISPR/Cas9 (Figure 2). Antimicrobial peptides are also considered as an alternative to antibiotics in eliminating biofilm-mediated infections, they are well-studied biofilm-eradicating agents (Flemming et al., 2008; Baltzer and Brown, 2011). They are ubiquitous in nature and cationic in nature. They consist of 5–90 amino acids (van Boxtel et al., 2017). Despite their exceptional potential to disrupt cell membranes, their mechanism of action is yet to be studied in the case of biofilms.
As reported, nanoparticles are also promising drug delivery systems that tend to penetrate deep due to their small size. Among all drug carriers, they are one of the most effective and explored drug carriers. In the case of biofilm-mediated infections, these particles enter the cells break the biofilm barrier and increase the availability of drugs or antibiotics to the bacterial cells. They have high efficacy, low toxicity, efficient penetration power and high site-specificity for drug release when they are given along with the drug (Hernández-Sierra et al., 2008; Harris et al., 2009; Kulshrestha et al., 2014).
In studies involving photodynamic therapy, some researchers have demonstrated its notable efficiency in fighting against biofilm-mediated antibiotic resistance, attributed to their tendency to generate reactive oxygen species (ROS) (Misba and Khan, 2018; Misba et al., 2019). On the other hand, considering gene editing technologies, numerous reports highlight the promising data regarding CRISPR/Cas9 and its derivatives like CRISPRi, in thwarting biofilms and their related infections (Zuberi et al., 2017a, Zuberi et al., 2017b; Azam et al., 2020; Zuberi et al., 2022). In the subsequent section, we will aim to delineate the potential avenues for the advancement of CRISPR/Cas9 and its barriers to overcome.
7 Anticipated opportunities, hypotheses and hindrances in utilizing CRISPR/Cas9 gene editing system to target bacterial biofilms
According to reports, CRISPR/Cas9 (Clustered regularly interspaced short palindromic repeat) exists approximately in 50% of bacterial genomes and 87% of archaeal genomes and has been acknowledged as an adaptive immune system in bacteria (Ran et al., 2015; Hille et al., 2018; Watson et al., 2021; Mayorga-Ramos et al., 2023). The CRISPR/Cas9 system has exhibited promising potential in recent years in the advancement and development of next-generation antimicrobial medicines or drugs to fight infections that are bought out by antibiotic resistance bacteria (Getahun et al., 2022; Mayorga-Ramos et al., 2023).
Targeting the genes that confer virulence and antibiotic resistance in bacteria has been a common application of this mechanism. CRISPR/Cas9 can be employed in two different ways: a pathogen-focused strategy and a gene-focused approach, depending on where the target gene is located (Li et al., 2016; Shabbir et al., 2018; Tang et al., 2019). Targeting chromosome regions to cause bacterial cell death is one pathogen-focused method. On the other hand, the gene-focused strategy includes focusing on the plasmids that may carry antibiotic-resistance genes (Palacios Araya et al., 2021; Nie et al., 2022). In such cases, the plasmid is eliminated, and the bacteria becomes antibiotic susceptible. The role of CRISPR/Cas9 has come across many times to target the genes that are implicated in antibiotic resistance (Nie et al., 2022; Tao et al., 2022). A study published by Bikard et al, used CRISPR/Cas9 to target the mecA gene (responsible for methicillin resistance) in USA300, the clinical isolates of S. aureus. His results revealed a marked decrease in the pollution of S. aureus in the mixed bacterial population as compared to the control group (Bikard et al., 2014).
In another research, a mouse skin colonization model was used to demonstrate that CRISPR/Cas9 was successful in specifically decreasing the colonization of Staphylococcus bacteria, in contrast to alternative treatment scenarios (Bikard et al., 2014; Wang et al., 2019). Moreover, in a separate study published by Ates et al., it was illustrated that resistance genes (aacA, grlA, grlB and mecA) in MRSA strains when targeted through CRISPR/Cas9 by designed CRISPR plasmids harbouring specific sgRNA, increase their susceptibility against antibiotics, hence changes their resistance profile (Juszczuk-Kubiak, 2024). Moreover, the use of pCasCure plasmids also came across in reversing the susceptibility of Enterobacteriaceae against carbapenems. pCasCure was reported successful in especially cleaving genes like balKPC, blaOXA-48 and blaNDM and targeting their corresponding plasmids (Hao et al., 2020).
The other team led by Yosef used the CRISPR/Cas9 system to eliminate plasmids containing blaCTXM-15 and blaNDM-1 (beta-lactamase genes) to eradicate E. coli that produce extended-spectrum beta-lactamases (ESBLs) (Yosef et al., 2015). The CRISPR/Cas system that targets are, the virulence factor in E. coli O157:H7 (EHEC), subsequently resulted in a 20-fold drop in viable cell counts, as shown by Citorik et al (Citorik et al., 2014). Rodrigues et al. tried to specifically eliminate the tetracycline (tetM)and erythromycin (ermB) in E. faecalis in both vitro and vivo conditions. His in vivo data demonstrated a considerable reduction in the percentage of antibiotic-resistant E. faecalis within the gut of mice (Rodrigues et al., 2019).
Askoura et al, revealed that S. enterica biofilm development, cell adhesion and cell invasion were impacted by CRISPR/Cas9 system that targeted sdiA (Askoura et al., 2021). Additionally, it’s noteworthy to mention the two of our studies that were published in 2017 manifesting the role of CRISPRi (CRISPR interference: derivative of CRISPR/Cas9) in restraining biofilm-mediated infections that are caused by clinical strains of E. coli. In one study we targeted luxS, the main quorum sensing gene in E. coli and in another article we tried to knockdown fimH gene and targeted bacterial adherence property through CRISPRi (Zuberi et al., 2017a, Zuberi et al., 2017b). Quorum sensing is one of the most important mechanisms that govern bacterial biofilm resistance against antibiotics, while the fimH gene plays a crucial role in bacterial virulence by contributing the fimbriae production. In both of our studies, CRISPRi demonstrated its highest level of effectiveness and exhibited optimal performance in targeting its specific genes and its related mechanisms, hence manifesting its lead role in biofilm-induced infections like urinary tract infections (UTI).
Additionally, in another study published by our group, we illustrated the role of CRISPRi in targeting the bolA gene (Azam et al., 2020). It has been discovered already that curli and fimbria formation have a role in the production of biofilms and are directly related to bacterial pathogenicity. BolA is a conserved protein and a transcriptional factor that is involved in bacterial motility and biofilm formation so by targeting this gene through CRISPR gene silencing we tried to combat these biofilm-mediated infections. We also targeted the OmpR/EnvZ, a two-component regulatory mechanism that is involved in transcriptional regulation when osmolarity changes. The main aim of that study was to elucidate the function of OmpR/EnvZ in controlling biofilm through curli and fimbriae production (Zuberi et al., 2022).
All our studies demonstrated exceptional outcomes and obtained outstanding success regarding the use of CRISPRi against biofilm-mediated infections. Not only this but these findings generated fresh insights and sparked innovative scientific discussion platforms regarding the use of this gene editing technology in combating biofilm-mediated infections, which represents a significant barrier within the realm of antibiotic resistance.
Analogous to the protective mechanism of thorns on a rose this gene editing technology also has certain intrinsic limitations that necessitate scientific scrutiny and consideration like the delivery challenge, which is the primary concern (Mayorga-Ramos et al., 2023). However, recent scientific researchers have suggested and hypothesized several different potential solutions to use this technology, such as employing the use of nanoparticles in conjugation with the CRISPR system (Li et al., 2018; Duan et al., 2021; Wan et al., 2021; Yan et al., 2021). Not only this but some studies have also proposed the concept of direct delivery of CRISPRi-edited bacterial cells to the infection site. This approach may facilitate the transfer of this gene editing mechanism to other virulent or antibiotic-resistant bacterial cells at the site of infection via natural conjugation of horizontal gene transfer mechanism (Ji et al., 2014; Watson et al., 2018; Barrangou et al., 2022).
8 Conclusion and future prospective
It is a known fact that Biofilm complicates the infections that are associated with communicable as well as non-communicable diseases. Other than that, the role of biofilm in post-operative infections, digestive disorders, cystic fibrosis, atherosclerotic arteries and infective endocarditis also has been documented in various studies and reports. Surface adherence is considered the prevailing mode of bacterial growth culminating in biofilm formation. Consequently, the biofilm environment fosters the emergence of antibiotic resistance or antimicrobial resistance. The understanding of how this lifestyle or environment influences the antimicrobial resistance evolution is still limited. Different hypotheses and explanations have emerged in this context like the proximity of bacterial cells in biofilms may facilitate the horizontal transfer and persistence of resistance genes within these bacterial populations.
The role of Biofilms in antibiotic resistance opens avenues and new vistas for repurposing existing drugs targeting biofilm. Forecasting antibiotic resistance by analysing the population of biofilm formers would be helpful in this scenario. Moreover, the use of nanoparticles in biomaterials and different drug delivery modes could also be considered as alternative options. Furthermore, to develop new and effective anti-biofilm agents computational and new sequencing technologies involving bioinformatic tools are also needed.
Currently, most of the studies focus on in silico screening, omics studies, and machine learning to identify the specific targets for anti-biofilm agents. To predicate antibiotic resistance and formulate therapeutic strategies it is essential to address potential protein targets, biofilm formation mechanisms and pathways. Integrated labs and computational science can lead to the development of successful anti-biofilm agents. RNA-Seq, a high-throughput technology, can assay gene regulation and expression, identifying transcriptomic signatures distinct to biofilms and bacterial dispersion.
As mentioned earlier, in addition to the advancements further research in the field of gene editing technology like CRISPR, is essential. It is imperative to address barriers in a CRISPR-like delivery system, as the advantages of utilizing this gene editing technology far outweigh its limitations, provided the delivery issue can be effectively resolved (Mayorga-Ramos et al., 2023). Moreover, through this mechanism, it could also become possible to target various genes associated with other pathways leading to bacterial biofilm formation and antibiotic resistance. For instance, genes responsible for efflux pumps could be the ones that can be specifically targeted, thereby enhancing our ability to combat antibiotic resistance.
Author contributions
AZ: Software, Supervision, Writing – original draft, Writing – review & editing. NA: Conceptualization, Formal analysis, Investigation, Methodology, Supervision, Visualization, Writing – original draft, Writing – review & editing. HA: Validation, Writing – review & editing. MS: Methodology, Writing – review & editing. IA: Validation, Writing – review & editing.
Funding
The author(s) declare that no financial support was received for the research, authorship, and/or publication of this article.
Acknowledgments
NA would like to thank Department of Health & Research, (Ministry of Health and Family Welfare, Govt. of India for awarded Young Scientist.
Conflict of interest
The authors declare that the research was conducted in the absence of any commercial or financial relationships that could be construed as a potential conflict of interest.
Publisher’s note
All claims expressed in this article are solely those of the authors and do not necessarily represent those of their affiliated organizations, or those of the publisher, the editors and the reviewers. Any product that may be evaluated in this article, or claim that may be made by its manufacturer, is not guaranteed or endorsed by the publisher.
References
Adam, B., Baillie, G. S., Douglas, L. J. (2002). Mixed species biofilms of Candida albicans and Staphylococcus epidermidis. J. Med. Microbiol. 51, 344–349. doi: 10.1099/0022-1317-51-4-344
Ahmad, N., Ali, S. M., Khan, A. U. (2017). First reported New Delhi metallo-β-lactamase-1-producing Cedecea lapagei. Int. J. Antimicrobial Agents 49, 118–119. doi: 10.1016/j.ijantimicag.2016.10.001
Ahmad, N., Khalid, S., Ali, S. M., Khan, A. U. (2018). Occurrence of blaNDM variants among enterobacteriaceae from a neonatal intensive care unit in a Northern India hospital. Front. Microbiol. 9. doi: 10.3389/fmicb.2018.00407
Ahmad, N., Joji, R. M., Shahid, M. (2023). Evolution and implementation of One Health to control the dissemination of antibiotic-resistant bacteria and resistance genes: A review. Front. Cell. Infection Microbiol. 12. doi: 10.3389/fcimb.2022.1065796
Ali, F., Sangwan, P. L., Koul, S., Pandey, A., Bani, S., Abdullah, S. T., et al. (2012). 4-Epi-pimaric acid: a phytomolecule as a potent antibacterial and anti-biofilm agent for oral cavity pathogens. Eur. J. Clin. Microbiol. Infect. Dis. 31, 149–159.
Amato, S. M., Fazen, C. H., Henry, T. C., Mok, W. W., Orman, M. A., Sandvik, E. L., et al. (2014). The role of metabolism in bacterial persistence. Front. Microbiol. 5. doi: 10.3389/fmicb.2014.00070
Anderl, J. N., Franklin, M. J., Stewart, P. S. (2000). Role of antibiotic penetration limitation in Klebsiella pneumoniae biofilm resistance to ampicillin and ciprofloxacin. Antimicrobial Agents Chemotherapy 44, 1818–1824. doi: 10.1128/AAC.44.7.1818-1824.2000
Antoniani, D., Rossi, E., Rinaldo, S., Bocci, P., Lolicato, M., Paiardini, A., et al. (2013). The immunosuppressive drug azathioprine inhibits biosynthesis of the bacterial signal molecule cyclic-di-gmp by interfering with intracellular nucleotide pool availability. Appl. Microbiol. Biotechnol. 97, 7325–7336.
Arevalo-Ferro, C., Hentzer, M., Reil, G., Görg, A., Kjelleberg, S., Givskov, M., et al. (2003). Identification of quorum-sensing regulated proteins in the opportunistic pathogen Pseudomonas aeruginosa by proteomics. Environ. Microbiol. 5, 1350–1369. doi: 10.1046/j.1462–2920.2003.00532.x
Armbruster, C. E., Hong, W., Pang, B., Weimer, K. E., Juneau, R. A., Turner, J., et al. (2010). Indirect Pathogenicity of Haemophilus influenzae and Moraxella catarrhalis in Polymicrobial Otitis Media Occurs via Interspecies Quorum Signaling. mBio 1, 10–128. doi: 10.1128/mBio.00102-10
Arzanlou, M., Chai, W. C., Venter, H. (2017). Intrinsic, adaptive and acquired antimicrobial resistance in Gram-negative bacteria. Essays Biochem. 61, 49–59. doi: 10.1042/EBC20160063
Askoura, M., Almalki, A. J., Lila, A. S. A., Almansour, K., Alshammari, F., Khafagy, E. S., et al. (2021). Alteration of Salmonella enterica Virulence and Host Pathogenesis through Targeting sdiA by Using the CRISPR-Cas9 System. Microorganisms 9, 2564. doi: 10.3390/microorganisms9122564
Azam, M. W., Zuberi, A., Khan, A. U. (2020). bolA gene involved in curli amyloids and fimbriae production in E. coli: exploring pathways to inhibit biofilm and amyloid formation. J. Biol. Research-Thessaloniki 27, 10. doi: 10.1186/s40709–020-00120–7
Baltzer, S. A., Brown, M. H. (2011). Antimicrobial peptides – promising alternatives to conventional antibiotics. Microbial Physiol. 20, 228–235. doi: 10.1159/000331009
Baroud, Á., Dandache, I., Araj, G. F., Wakim, R., Kanj, S., Kanafani, Z., et al. (2013). Underlying mechanisms of carbapenem resistance in extended-spectrum β-lactamase-producing Klebsiella pneumoniae and Escherichia coli isolates at a tertiary care centre in Lebanon: role of OXA-48 and NDM-1 carbapenemases. Int. J. Antimicrobial Agents 41, 75–79. doi: 10.1016/j.ijantimicag.2012.08.010
Barrangou, R., Hassett, D. J., Hwang, S. H., Rice, S. A., Kjelleberg, S., Webb, J. S. (2022). CRISPR-cas biology CRISPR-cas, horizontal gene transfer, and the flexible (Pan) genome. CRISPR: Biol. Appl. doi: 10.1002/9781683673798
Barraud, N., Hassett, D. J., Hwang, S. H., Rice, S. A., Kjelleberg, S., Webb, J. S. (2006). Involvement of nitric oxide in biofilm dispersal of Pseudomonas aeruginosa. J. Bacteriol. 188, 7344–7353. doi: 10.1128/JB.00779-06
Baumgartner, M., Lang, M., Holley, H., Crepaz, D., Hausmann, B., Pjevac, P., et al. (2021). Mucosal biofilms are an endoscopic feature of irritable bowel syndrome and ulcerative colitis. Gastroenterology 161, 1245–1256.e20. doi: 10.1053/j.gastro.2021.06.024
Bezerra, T. F. P., Padua, F. G., de, M., Gebrim, E. M. M. S., Saldiva, P. H. N., Voegels, R. L. (2011). Biofilms in chronic rhinosinusitis with nasal polyps. Otolaryngol Head Neck Surg. 144, 612–616. doi: 10.1177/0194599811399536
Bikard, D., Euler, C. W., Jiang, W., Nussenzweig, P. M., Goldberg, G. W., Duportet, X. (2014). Exploiting CRISPR-Cas nucleases to produce sequence-specific antimicrobials. Nat. Biotechnol. 32, 1146–1150. doi: 10.1038/nbt.3043
Bjarnsholt, T., Jensen, P. Ø., Burmølle, M., Hentzer, M., Haagensen, J. A., Hougen, H. P., et al. (2005). Pseudomonas aeruginosa tolerance to tobramycin, hydrogen peroxide and polymorphonuclear leukocytes is quorum-sensing dependent. Microbiology 151, 373–383. doi: 10.1099/mic.0.27463-0
Bjarnsholt, T., Jensen, P. Ø., Fiandaca, M. J., Pedersen, J., Hansen, C. R., Andersen, C. B., et al. (2009). Pseudomonas aeruginosa biofilms in the respiratory tract of cystic fibrosis patients. Pediatr. Pulmonol. 44, 547–558. doi: 10.1002/ppul.21011
Blair, J. M. A., Webber, M. A., Baylay, A. J., Ogbolu, D. O., Piddock, L. J. (2015). Molecular mechanisms of antibiotic resistance. Nat. Rev. Microbiol. 13, 42–51. doi: 10.1038/nrmicro3380
Borriello, G., Werner, E., Roe, F., Kim, A. M., Ehrlich, G. D., Stewart, P. S. (2004). Oxygen limitation contributes to antibiotic tolerance of Pseudomonas aeruginosa in biofilms. Antimicrobial Agents Chemotherapy 48, 2659–2664. doi: 10.1128/AAC.48.7.2659-2664.2004
Borriello, G., Richards, L., Ehrlich, G. D., Stewart, P. S. (2006). Arginine or nitrate enhances antibiotic susceptibility of Pseudomonas aeruginosa in biofilms. Antimicrobial Agents Chemotherapy 50, 382–384. doi: 10.1128/AAC.50.1.382-384.2006
Brauner, A., Fridman, O., Gefen, O., Balaban, N. Q. (2016). Distinguishing between resistance, tolerance and persistence to antibiotic treatment. Nat. Rev. Microbiol. 14, 320–330. doi: 10.1038/nrmicro.2016.34
Brooun, A., Liu, S., Lewis, K. (2000). A dose-response study of antibiotic resistance in Pseudomonas aeruginosa biofilms. Antimicrobial Agents Chemotherapy 44, 640–646. doi: 10.1128/AAC.44.3.640-646.2000
Budhani, R. K., Struthers, J. K. (1998). Interaction of Streptococcus pneumoniae and Moraxella catarrhalis: Investigation of the Indirect Pathogenic Role of β-Lactamase-Producing Moraxellae by Use of a Continuous-Culture Biofilm System. Antimicrobial Agents Chemotherapy 42, 2521–2526. doi: 10.1128/AAC.42.10.2521
Burmølle, M., Thomsen, T. R., Fazli, M., Dige, I., Christensen, L., Homøe, P., et al. (2010). Biofilms in chronic infections – a matter of opportunity – monospecies biofilms in multispecies infections. FEMS Immunol. Med. Microbiol. 59, 324–336. doi: 10.1111/j.1574–695X.2010.00714.x
Cangui-Panchi, S. P., Ñacato-Toapanta, A. L., Enríquez-Martínez, L. J., Reyes, J., Garzon-Chavez, D., Machado, A. (2022). Biofilm-forming microorganisms causing hospital-acquired infections from intravenous catheter: A systematic review. Curr. Res. Microbial Sci. 3, 100175. doi: 10.1016/j.crmicr.2022.100175
Cangui-Panchi, S. P., Ñacato-Toapanta, A. L., Enríquez-Martínez, L. J., Salinas-Delgado, G. A. (2023). Battle royale: Immune response on biofilms – host-pathogen interactions. Curr. Res. Immunol. 4, 100057. doi: 10.1016/j.crimmu.2023.100057
Carron, M. A., Tran, V. R., Sugawa, C., Coticchia, J. M. (2006). Identification of Helicobacter pylori biofilms in human gastric mucosa. J. Gastrointest Surg. 10, 712–717. doi: 10.1016/j.gassur.2005.10.019
Chen, A. I., Dolben, E. F., Okegbe, C., Harty, C. E., Golub, Y. (2014). Candida albicans Ethanol Stimulates Pseudomonas aeruginosa WspR-Controlled Biofilm Formation as Part of a Cyclic Relationship Involving Phenazines. PloS Pathog. 10, e1004480. doi: 10.1371/journal.ppat.1004480
Chiang, W.-C., Pamp, S. J., Nilsson, M., Givskov, M., Tolker-Nielsen, T. (2012). The metabolically active subpopulation in Pseudomonas aeruginosa biofilms survives exposure to membrane-targeting antimicrobials via distinct molecular mechanisms. FEMS Immunol. Med. Microbiol. 65, 245–256. doi: 10.1111/j.1574-695X.2012.00929.x
Chorell, E., Pinkner, J. S., Phan, G., Edvinsson, S., Buelens, F., Remaut, H., et al. (2010). Design and synthesis of c-2 substituted thiazolo and dihydrothiazolo ring-fused 2-pyridones; pilicides with increased antivirulence activity. J. Med. Chem. 53, 5690–5695.
Citorik, R. J., Mimee, M., Lu, T. K. (2014). Sequence-specific antimicrobials using efficiently delivered RNA-guided nucleases. Nat. Biotechnol. 32, 1141–1145. doi: 10.1038/nbt.3011
Costerton, J. W., Cheng, K. J., Geesey, G. G., Ladd, T. I., Nickel, J. C., Dasgupta, M., et al. (1987). Bacterial biofilms in nature and disease. Annu. Rev. Microbiol. 41, 435–464. doi: 10.1146/annurev.mi.41.100187.002251
Costerton, J. W., Irvin, R. T., Cheng, K. J. (1981). The bacterial glycocalyx in nature and disease. Annu. Rev. Microbiol. 35, 299–324. doi: 10.1146/annurev.mi.35.100181.001503
Costerton, J. W., Montanaro, L., Arciola, C. R. (2005). Biofilm in implant infections: its production and regulation. Int. J. Artif. Organs 28, 1062–1068. doi: 10.1177/039139880502801103
Coticchia, J. M., Sugawa, C., Tran, V. R., Gurrola, J., Kowalski, E., Carron, M. A. (2006). Presence and density of Helicobacter pylori biofilms in human gastric mucosa in patients with peptic ulcer disease. J. Gastrointest Surg. 10, 883–889. doi: 10.1016/j.gassur.2005.12.009
Dale, J. L., Cagnazzo, J., Phan, C. Q., Barnes, A. M., Dunny, G. M. (2015). Multiple roles for enterococcus faecalis glycosyltransferases in biofilm-associated antibiotic resistance, cell envelope integrity, and conjugative transfer. Antimicrobial Agents Chemotherapy 59, 4094–4105. doi: 10.1128/AAC.00344-15
Dalton, T., Dowd, S. E., Wolcott, R. D., Sun, Y., Watters, C., Griswold, J. A., et al. (2011). An in vivo polymicrobial biofilm wound infection model to study interspecies interactions. PloS One 6, e27317. doi: 10.1371/journal.pone.0027317
de Beer, D., Stoodley, P., Roe, F., Lewandowski, Z. (1994a). Effects of biofilm structures on oxygen distribution and mass transport. Biotechnol. Bioengineering 43, 1131–1138. doi: 10.1002/bit.260431118
de Beer, D., Stoodley, P., Lewandowski, Z. (1994b). Liquid flow in heterogeneous biofilms. Biotechnol. Bioengineering 44, 636–641. doi: 10.1002/bit.260440510
DePas, W. H., Starwalt-Lee, R., Sambeek, L. V., Kumar, S. R., Gradinaru, V., Newman, D. K. (2016). Exposing the three-dimensional biogeography and metabolic states of pathogens in cystic fibrosis sputum via hydrogel embedding, clearing, and rRNA labeling. mBio 7, e00796–e00716. doi: 10.1128/mbio.00796-16
Di Cagno, R., De Angelis, M., Calasso, M., Gobbetti, M. (2011). Proteomics of the bacterial cross-talk by quorum sensing. J. Proteomics 74, 19–34. doi: 10.1016/j.jprot.2010.09.003
Donlan, R. (2001). Biofilms and device-associated infections. Emerging Infect. Dis. 7, 277–281. doi: 10.3201/eid0702.010226
Doroshenko, N., Tseng, B. S., Howlin, R. P., Deacon, J., Wharton, J. A., Thurner, P. J., et al. (2014). Extracellular DNA impedes the transport of vancomycin in staphylococcus epidermidis biofilms preexposed to subinhibitory concentrations of vancomycin. Antimicrobial Agents Chemotherapy 58, 7273–7282. doi: 10.1128/AAC.03132-14
Driffield, K., Miller, K., Bostock, J. M., O'neill, A. J., Chopra, I. (2008). Increased mutability of Pseudomonas aeruginosa in biofilms. J. Antimicrobial Chemotherapy 61, 1053–1056. doi: 10.1093/jac/dkn044
Duan, L., Ouyang, K., Xu, X., Xu, L., Wen, C., Zhou, X., et al. (2021). Nanoparticle delivery of CRISPR/cas9 for genome editing. Front. Genet. 12. doi: 10.3389/fgene.2021.673286
Dutt, Y., Dhiman, R., Singh, T., Vibhuti, A., Gupta, A., Pandey, R. P., et al. (2022). The association between biofilm formation and antimicrobial resistance with possible ingenious bio-remedial approaches. Antibiotics. MDPI 11, 930. doi: 10.3390/antibiotics11070930
Eliopoulos, G. M., Blazquez, J. (2003). Hypermutation as a factor contributing to the acquisition of antimicrobial resistance. Clin. Infect. Dis. 37, 1201–1209. doi: 10.1086/378810
Fabretti, F., Theilacker, C., Baldassarri, L., Kaczynski, Z., Kropec, A., Holst, O., et al. (2006). Alanine esters of enterococcal lipoteichoic acid play a role in biofilm formation and resistance to antimicrobial peptides. Infection Immun. 74, 4164–4171. doi: 10.1128/IAI.00111-06
Fazli, M., Bjarnsholt, T., Kirketerp-Møller, K., Jørgensen, A., Andersen, C. B., Givskov, M., et al. (2011). Quantitative analysis of the cellular inflammatory response against biofilm bacteria in chronic wounds. Wound Repair Regener. 19, 387–391. doi: 10.1111/j.1524-475x.2011.0
Flemming, K., Klingenberg, C., Cavanagh, J. P., Sletteng, M., Stensen, W., Svendsen, J. S., et al. (2008). High in vitro antimicrobial activity of synthetic antimicrobial peptidomimetics against staphylococcal biofilms. J. Antimicrobial Chemotherapy 63, 136–145. doi: 10.1093/jac/dkn464
Flemming, H.-C., Wingender, J., Szewzyk, U., Steinberg, P, Rice, S. A, Kjelleberg, S. (2016). Biofilms: an emergent form of bacterial life. Nat. Rev. Microbiol. 14, 563–575. doi: 10.1038/nrmicro.2016.94
Flemming, H.-C., Wingender, J. (2010). The biofilm matrix. Nat. Rev. Microbiol. 8, 623–633. doi: 10.1038/nrmicro2415
Gaynes, R. (2017). The discovery of penicillin—new insights after more than 75 years of clinical use. Emerging Infect. Dis. 23, 849–853. doi: 10.3201/eid2305.161556
Getahun, Y. A., Ali, D. A., Taye, B. W., Alemayehu, Y. A. (2022). Multidrug-resistant microbial therapy using antimicrobial peptides and the CRISPR/cas9 system. Veterinary Medicine: Res. Rep. 13, 173–190. doi: 10.2147/VMRR.S366533
Ghosh, A., Jayaraman, N., Chatterji, D. (2020). Small-molecule inhibition of bacterial biofilm. ACS Omega 5, 3108–3115.
Gillis, R. J., White, K. G., Choi, K. H., Wagner, V. E., Schweizer, H. P., Iglewski, B. H. (2005). Molecular basis of azithromycin-resistant Pseudomonas aeruginosa biofilms. Antimicrobial Agents Chemotherapy 49, 3858–3867. doi: 10.1128/AAC.49.9.3858-3867.2005
Gristina, A. G., Oga, M., Webb, L. X., Hobgood, C. D. (1985). Adherent bacterial colonization in the pathogenesis of osteomyelitis. Science 228, 990–993. doi: 10.1126/science.4001933
Gross, M., Cramton, S. E., Götz, F., Peschel, A. (2001). Key role of teichoic acid net charge in Staphylococcus aureus colonization of artificial surfaces. Infection Immun. 69, 3423–3426. doi: 10.1128/IAI.69.5.3423-3426.2001
Guilhen, C., Forestier, C., Balestrino, D. (2017). Biofilm dispersal: multiple elaborate strategies for dissemination of bacteria with unique properties. Mol. Microbiol. 105, 188–210. doi: 10.1111/mmi.13698
Haagensen, J. A. J., Klausen, M., Ernst, R. K., Miller, S. I., Folkesson, A., Tolker-Nielsen, T. (2007). Differentiation and distribution of colistin- and sodium dodecyl sulfate-tolerant cells in Pseudomonas aeruginosa biofilms. J. Bacteriology 189, 28–37. doi: 10.1128/JB.00720-06
Hall, C. W., Mah, T.-F. (2017). Molecular mechanisms of biofilm-based antibiotic resistance and tolerance in pathogenic bacteria. FEMS Microbiol. Rev. 41, 276–301. doi: 10.1093/femsre/fux010
Hall-Stoodley, L., Hu, F. Z., Gieseke, A., Nistico, L., Nguyen, D., Hayes, J., et al. (2006). Direct detection of bacterial biofilms on the middle-ear mucosa of children with chronic otitis media. Jama 296, 202–211. doi: 10.1001/jama.296.2.202
Han, Z., Pinkner, J. S., Ford, B., Obermann, R., Nolan, W., Wildman, S. A., et al. (2010). Structure-Based Drug Design and Optimization of Mannoside Bacterial FimH Antagonists. J. Med. Chem. 53, 4779–4792.
Hardy, L., Jespers, V., Dahchour, N., Mwambarangwe, L., Musengamana, V., Vaneechoutte, M., et al. (2015). Unravelling the bacterial vaginosis-associated biofilm: a multiplex Gardnerella vaginalis and Atopobium vaginae fluorescence in situ hybridization assay using peptide nucleic acid probes. PloS One 10, e0136658. doi: 10.1371/journal.pone.0136658
Hao, M., He, Y., Zhang, H., Liao, X. P., Liu, Y. H., Sun, J., et al. (2020). CRISPR-cas9-mediated carbapenemase gene and plasmid curing in carbapenem-resistant Enterobacteriaceae. Antimicrobial Agents Chemotherapy 64, 10–128. doi: 10.1128/AAC.00843-20
Harriott, M. M., Noverr, M. C. (2009). Candida albicans and Staphylococcus aureus Form Polymicrobial Biofilms: Effects on Antimicrobial Resistance. Antimicrobial Agents Chemotherapy 53, 3914–3922. doi: 10.1128/AAC.00657-09
Harris, F., Dennison, S., Phoenix, D. (2009). Anionic antimicrobial peptides from eukaryotic organisms. Curr. Protein Pept. Sci. 10, 585–606. doi: 10.2174/138920309789630589
Hernández-Sierra, J. F., Ruiz, F., Pena, D. C. C., Martínez-Gutiérrez, F., Martínez, A. E., Guillén, A. D. J., et al. (2008). The antimicrobial sensitivity of Streptococcus mutans to nanoparticles of silver, zinc oxide, and gold. Nanomedicine: Nanotechnology Biol. Med. 4, 237–240. doi: 10.1016/j.nano.2008.04.005
Hille, F., Richter, H., Wong, S. P., Bratovič, M., Ressel, S., Charpentier, E. (2018). The biology of CRISPR-cas: backward and forward. Cell 172, 1239–1259. doi: 10.1016/j.cell.2017.11.032
Hochbaum, A. I., Kolodkin-Gal, I., Foulston, L., Kolter, R., Aizenberg, J., Losick, R. (2011). Inhibitory Effects of d -Amino Acids on Staphylococcus aureus Biofilm Development. J. Bacteriology 193, 5616–5622. doi: 10.1128/JB.05534-11
Homøe, P., Bjarnsholt, T., Wessman, M., Sørensen, H. C. F., Johansen, H. K. (2009). Morphological evidence of biofilm formation in greenlanders with chronic suppurative otitis media. Eur. Arch. Otorhinolaryngol. 266, 1533–1538. doi: 10.1007/s00405-009-0940-9
Høiby, N., Bjarnsholt, T., Givskov, M., Molin, S., Ciofu, O. (2010). Antibiotic resistance of bacterial biofilms. Int. J. Antimicrobial Agents 35, 322–332. doi: 10.1016/j.ijantimicag.2009.12.011
Huigens, R. W., Richards, J. J., Parise, G., Ballard, T. E., Zeng, W., Deora, R., et al. (2007). Inhibition of Pseudomonas aeruginosa Biofilm Formation with Bromoageliferin Analogues. J. Am. Chem. Soc 129, 6966–6967.
Iannitelli, A., Grande, R., Di Stefano, A., Di Giulio, M., Sozio, P., Bessa, L. J., et al. (2011). Potential antibacterial activity of carvacrol-loaded poly(DL-lactide-co-glycolide) (PLGA) nanoparticles against microbial biofilm. Int. J. Mol. Sci. 12, 5039–5051. doi: 10.3390/ijms12085039
James, G. A., Swogger, E., Wolcott, R., deLancey Pulcini, E., Secor, P., Sestrich, J., et al. (2008). Biofilms in chronic wounds. Wound Repair Regener. 16, 37–44. doi: 10.1111/j.1524-475x.2007.00321.x
Johani, K., Fritz, B. G., Bjarnsholt, T., Lipsky, B. A., Jensen, S. O., Yang, M., et al. (2019). Understanding the microbiome of diabetic foot osteomyelitis: insights from molecular and microscopic approaches. Clin. Microbiol. Infect. 25, 332–339. doi: 10.1016/j.cmi.2018.04.036
Ji, W., Lee, D., Wong, E., Dadlani, P., Dinh, D., Huang, V., et al. (2014). Specific Gene repression by CRISPRi system transferred through bacterial conjugation. ACS Synthetic Biol. 3, 929–931. doi: 10.1021/sb500036q
Juszczuk-Kubiak, E. (2024). Molecular aspects of the functioning of pathogenic bacteria biofilm based on quorum sensing (QS) signal-response system and innovative non-antibiotic strategies for their elimination. Int. J. Mol. Sci. 25, 2655. doi: 10.3390/ijms25052655
Kelly, S. R., Jensen, P. R., Henkel, T. P., Fenical, W., Pawlik, J. R. (2003). Effects of caribbean sponge extracts on bacterial attachment. Aquat. Microb. Ecol. 31, 175–182.
Kennedy, P., Brammah, S., Wills, E. (2010). Burns, biofilm and a new appraisal of burn wound sepsis. Burns 36, 49–56. doi: 10.1016/j.burns.2009.02.017
Kolodkin-Gal, I., Romero, D., Cao, S., Clardy, J., Kolter, R., Losick, R. (2010). d -amino acids trigger biofilm disassembly. Science 328, 627–629. doi: 10.1126/science.1188628
Kovacs, B., Le Gall-David, S., Vincent, P., Le Bars, H., Buffet-Bataillon, S., Bonnaure-Mallet, M., et al. (2013). Is biofilm formation related to the hypermutator phenotype in clinical Enterobacteriaceae isolates? FEMS Microbiol. Lett. 347, 116–122. doi: 10.1111/1574-6968.12229
Kulshrestha, S., Khan, S., Meena, R., Singh, B. R., Khan, A. U. (2014). A graphene/zinc oxide nanocomposite film protects dental implant surfaces against cariogenic Streptococcus mutans. Biofouling 30, 1281–1294. doi: 10.1080/08927014.2014.983093
Kumar, N., Radhakrishnan, A., Wright, C. C., Chou, T. H., Lei, H. T., Bolla, J. R., et al. (2014). Crystal structure of the transcriptional regulator Rv1219c of Mycobacterium tuberculosis. Protein Sci. 23, 423–432. doi: 10.1002/pro.2424
Lanter, B. B., Sauer, K., Davies, D. G. (2014). Bacteria present in carotid arterial plaques are found as biofilm deposits which may contribute to enhanced risk of plaque rupture. mBio 5, e01206–e01214. doi: 10.1128/mbio.01206-14
Lee, J.-H., Regmi, S. C., Kim, J.-A., Cho, M. H., Yun, H., Lee, C.-S., et al. (2011). Apple flavonoid phloretin inhibits escherichia coli o157:h7 biofilm formation and ameliorates colon inflammation in rats. Infect. Immun. 79, 4819–4827.
Lee, M. R., Pawlowski, K. S., Luong, A., Furze, A. D., Roland, P. S. (2009). Biofilm presence in humans with chronic suppurative otitis media. Otolaryngol. - Head Neck Surg. 141, 567–571. doi: 10.1016/j.otohns.2009.08.010
Leong, P. M., Hsia, H. C., Miller, J. H. (1986). Analysis of spontaneous base substitutions generated in mismatch-repair-deficient strains of Escherichia coli. J. Bacteriology 168, 412–416. doi: 10.1128/jb.168.1.412-416.1986
Li, R., Fang, L., Tan, S., Yu, M., Li, X., He, S., et al. (2016). Type I CRISPR-Cas targets endogenous genes and regulates virulence to evade mammalian host immunity. Cell Res. 26, 1273–1287. doi: 10.1038/cr.2016.135
Li, M., Fan, Y. N., Chen, Z. Y., Luo, Y. L., Wang, Y. C., Lian, Z. X., et al. (2018). Optimized nanoparticle-mediated delivery of CRISPR-Cas9 system for B cell intervention. Nano Res. 11, 6270–6282. doi: 10.1007/s12274-018-2150-5
Li, Z., Nair, S. K. (2012). Quorum sensing: How bacteria can coordinate activity and synchronize their response to external signals? Protein Sci. 21, 1403–1417. doi: 10.1002/pro.2132
Long, K. S., Poehlsgaard, J., Kehrenberg, C., Schwarz, S., Vester, B. (2006). The cfr rRNA methyltransferase confers resistance to phenicols, lincosamides, oxazolidinones, pleuromutilins, and streptogramin A antibiotics. Antimicrobial Agents Chemotherapy 50, 2500–2505. doi: 10.1128/AAC.00131-06
Lonn-Stensrud, J., Landin, M. A., Benneche, T., Petersen, F. C., Scheie, A. A. (2008). Furanones, potential agents for preventing Staphylococcus epidermidis biofilm infections? J. Antimicrobial Chemotherapy 63, 309–316. doi: 10.1093/jac/dkn501
Lynch, J. P., Clark, N. M., Zhanel, G. G. (2013). ‘Evolution of antimicrobial resistance among Enterobacteriaceae (focus on extended spectrum β-lactamases and carbapenemases)’. Expert Opin. Pharmacotherapy 14, 199–210. doi: 10.1517/14656566.2013.763030
MaChado, A., Cerca, N. (2015). Influence of biofilm formation by Gardnerella vaginalis and other anaerobes on bacterial vaginosis. J. Infect. Dis. 212, 1856–1861. doi: 10.1093/infdis/jiv338
Magiorakos, A.-P., Srinivasan, A., Carey, R. B., Carmeli, Y., Falagas, M. E., Giske, C. G., et al. (2012). Multidrug-resistant, extensively drug-resistant and pandrug-resistant bacteria: an international expert proposal for interim standard definitions for acquired resistance. Clin. Microbiol. Infection 18, 268–281. doi: 10.1111/j.1469-0691.2011.03570.x
Mah, T.-F. (2012). Biofilm-specific antibiotic resistance. Future Microbiol. 7, 1061–1072. doi: 10.2217/fmb.12.76
Mallmann, C., Siemoneit, S., Schmiedel, D., Petrich, A., Gescher, D. M., Halle, E., et al. (2010). Fluorescence in situ hybridization to improve the diagnosis of endocarditis: a pilot study. Clin. Microbiol. Infect. 16, 767–773. doi: 10.1111/j.1469-0691.2009.02936.x
Marrie, T. J., Cooper, J. H., Costerton, J. W. (1987). Ultrastructure of cardiac bacterial vegetations on native valves with emphasis on alterations in bacterial morphology following antibiotic treatment. Can. J. Cardiol. 3, 275–280.
Marvasi, M., Chen, C., Carrazana, M., Durie, I. A., Teplitski, M. (2014). Systematic analysis of the ability of Nitric Oxide donors to dislodge biofilms formed by Salmonella enterica and Escherichia coli O157:H7. AMB Express 4, 42. doi: 10.1186/s13568-014-0042-y
Mayorga-Ramos, A., Zúñiga-Miranda, J., Carrera-Pacheco, S. E., Barba-Ostria, C., Guamán, L. P. (2023). CRISPR-cas-based antimicrobials: design, challenges, and bacterial mechanisms of resistance. ACS Infect. Dis. 9, 1283–1302. doi: 10.1021/acsinfecdis.2c00649
McDougald, D., Rice, S. A., Barraud, N., Steinberg, P. D., Kjelleberg, S. (2012). Should we stay or should we go: mechanisms and ecological consequences for biofilm dispersal. Nat. Rev. Microbiol. 10, 39–50. doi: 10.1038/nrmicro2695
Misba, L., Abdulrahman, H., Khan, A. U. (2019). Photodynamic efficacy of toluidine blue O against mono species and dual species bacterial biofilm. Photodiagnosis Photodyn. Ther. 26, 383–388. doi: 10.1016/j.pdpdt.2019.05.001
Misba, L., Khan, A. U. (2018). Enhanced photodynamic therapy using light fractionation against Streptococcus mutans biofilm: type I and type II mechanism. Future Microbiol. 13, 437–454. doi: 10.2217/fmb-2017-0207
Morgan-Sagastume, F., Larsen, P., Nielsen, J. L., Nielsen, P. H. (2008). Characterization of the loosely attached fraction of activated sludge bacteria. Water Res. 42, 843–854. doi: 10.1016/j.watres.2007.08.026
Mulcahy, H., Charron-Mazenod, L., Lewenza, S. (2008). Extracellular DNA chelates cations and induces antibiotic resistance in pseudomonas aeruginosa biofilms. PloS Pathog. 4, e1000213. doi: 10.1371/journal.ppat.1000213
Munita, J. M., Arias, C. A. (2016). Mechanisms of antibiotic resistance. Microbiol. Spectr. 4, 481–511. doi: 10.1128/microbiolspec.VMBF-0016-2015
Muñoz-Egea, M.-C., García-Pedrazuela, M., Mahillo-Fernandez, I., Esteban, J. (2016). Effect of antibiotics and antibiofilm agents in the ultrastructure and development of biofilms developed by nonpigmented rapidly growing mycobacteria. Microbial Drug Resistance 22, 1–6. doi: 10.1089/mdr.2015.0124
Nagai, K., Davies, T. A., Jacobs, M. R., Appelbaum, P. C. (2002). Effects of amino acid alterations in penicillin-binding proteins (PBPs) 1a, 2b, and 2x on PBP affinities of penicillin, ampicillin, amoxicillin, cefditoren, cefuroxime, cefprozil, and cefaclor in 18 clinical isolates of penicillin-susceptible, -intermediate, and -resistant pneumococci. Antimicrobial Agents Chemotherapy 46, 1273–1280. doi: 10.1128/AAC.46.5.1273-1280.2002
Nesher, L., Rolston, K. V. I. (2014). The current spectrum of infection in cancer patients with chemotherapy related neutropenia. Infection 42, 5–13. doi: 10.1007/s15010-013-0525-9
Neuhaus, F. C., Baddiley, J. (2003). A continuum of anionic charge: structures and functions of d -alanyl-teichoic acids in gram-positive bacteria. Microbiol. Mol. Biol. Rev. 67, 686–723. doi: 10.1128/MMBR.67.4.686-723.2003
Nickel, J. C., Costerton, J. W. (1993). Bacterial localization in antibiotic-refractory chronic bacterial prostatitis. Prostate 23, 107–114. doi: 10.1002/pros.2990230204
Nickel, J. C., Emtage, J., Costerton, J. W. (1985). Ultrastructural microbial ecology of infection-induced urinary stones. J. Urol. 133, 622–627. doi: 10.1016/s0022-5347(17)49116-6
Nie, M., Dong, Y., Cao, Q., Zhao, D., Ji, S., Huang, H., et al. (2022). CRISPR contributes to adhesion, invasion, and biofilm formation in streptococcus agalactiae by repressing capsular polysaccharide production. Microbiol. Spectr. 10, e02113-21. doi: 10.1128/spectrum.02113-21
Nilsson, M., Rybtke, M., Givskov, M., Høiby, N., Twetman, S., Tolker-Nielsen, T. (2016). The dlt genes play a role in antimicrobial tolerance of Streptococcus mutans biofilms. Int. J. Antimicrobial Agents 48, 298–304. doi: 10.1016/j.ijantimicag.2016.06.019
Palacios Araya, D., Palmer, K. L., Duerkop, B. A. (2021). CRISPR-based antimicrobials to obstruct antibiotic-resistant and pathogenic bacteria. PloS Pathog. 17, e1009672. doi: 10.1371/journal.ppat.1010153
Pamp, S. J., Gjermansen, M., Johansen, H. K., Tolker‐Nielsen, T. (2008). Tolerance to the antimicrobial peptide colistin in Pseudomonas aeruginosa biofilms is linked to metabolically active cells, and depends on the pmr and mexAB-oprM genes. Mol. Microbiol. 68, 223–240. doi: 10.1111/j.1365-2958.2008.06152.x
Percival, S. L., Malic, S., Cruz, H., Williams, D. W. (2011). Introduction to Biofilms, 41–68. doi: 10.1007/978–3-642–21289-5_2
Perez, A. C., Pang, B., King, L. B., Tan, L., Murrah, K. A., Reimche, J. L., et al. (2014). Residence of Streptococcus pneumoniae and Moraxella catarrhalis within polymicrobial biofilm promotes antibiotic resistance and bacterial persistence in vivo. Pathog. Dis. 70, 280–288. doi: 10.1111/fim.2014.70.issue-3
Peschel, A., Vuong, C., Otto, M., Gö̈tz, F. (2000). The d -Alanine Residues of Staphylococcus aureus Teichoic Acids Alter the Susceptibility to Vancomycin and the Activity of Autolytic Enzymes. Antimicrobial Agents Chemotherapy 44, 2845–2847. doi: 10.1128/AAC.44.10.2845-2847.2000
Poole, K. (2007). Efflux pumps as antimicrobial resistance mechanisms. Ann. Med. 39, 162–176. doi: 10.1080/07853890701195262
Prunier, A., Malbruny, B., Laurans, M., Brouard, J., Duhamel, J. F., Leclercq, R. (2003). High rate of macrolide resistance in Staphylococcus aureus strains from patients with cystic fibrosis reveals high proportions of hypermutable strains. J. Infect. Dis. 187, 1709–1716. doi: 10.1086/374937
Qvist, T., Eickhardt, S., Kragh, K. N., Andersen, C. B., Iversen, M., Høiby, N., et al. (2015). Chronic pulmonary disease with Mycobacterium abscessus complex is a biofilm infection. Eur. Respir. J. 46, 1823–1826. doi: 10.1183/13993003.01102-2015
Ran, F. A., Cong, L., Yan, W. X., Scott, D. A., Gootenberg, J. S., Kriz, A. J., et al. (2015). In vivo genome editing using Staphylococcus aureus Cas9. Nature 520, 186–191. doi: 10.1038/nature14299
Rasmussen, L., White, E. L., Pathak, A., Ayala, J. C., Wang, H., Wu, J., et al. (2011). A high-throughput screening assay for inhibitors of bacterial motility identifies a novel inhibitor of the na+-driven flagellar motor and virulence gene expression in vibrio cholerae. Antimicrob. Agents Chemother. 55, 4134–4143.
Reffuveille, F., Fuente-Núñez, C. D. L., Fairfull-Smith, K. E., Hancock, R. E. (2015). Potentiation of ciprofloxacin action against Gram-negative bacterial biofilms by a nitroxide. Pathog. Dis. 73, ftv016. doi: 10.1093/femspd/ftv016
Roberts, M. C. (2005). Update on acquired tetracycline resistance genes. FEMS Microbiol. Lett. 245, 195–203. doi: 10.1016/j.femsle.2005.02.034
Rodrigues, M., McBride, S. W., Hullahalli, K., Palmer, K. L., Duerkop, B. A. (2019). Conjugative delivery of CRISPR-cas9 for the selective depletion of antibiotic-resistant enterococci. Antimicrobial Agents Chemotherapy 63, 10–128. doi: 10.1128/AAC.01454-19
Roizman, D., Vidaillac, C., Givskov, M., Yang, L. (2017). In vitro evaluation of biofilm dispersal as a therapeutic strategy to restore antimicrobial efficacy. Antimicrobial Agents Chemotherapy 61, 10–128. doi: 10.1128/AAC.01088-17
Román, F., Cantón, R., Pérez-Vázquez, M., Baquero, F., Campos, J. (2004). Dynamics of long-term colonization of respiratory tract by Haemophilus influenzae in cystic fibrosis patients shows a marked increase in hypermutable strains. J. Clin. Microbiol. 42, 1450–1459. doi: 10.1128/JCM.42.4.1450-1459.2004
Romanova, Y., Mulabaev, N. S., Tolordava, E. R., Seregin, A. V., Seregin, I. V., Alexeeva, N. V., et al. (2015). Microbial communities on kidney stones. Mol. Genet. Microbiol. Virol. 30, 78–84. doi: 10.3103/s089141681502007x
Rosen, D. A., Hooton, T. M., Stamm, W. E., Humphrey, P. A., Hultgren, S. J. (2007). Detection of intracellular bacterial communities in human urinary tractinfection. PloS Med. 4, e329.
Roy, R., Tiwari, M., Donelli, G., Tiwari, V. (2018). Strategies for combating bacterial biofilms: A focus on anti-biofilm agents and their mechanisms of action. Virulence 9, 522–554. doi: 10.1080/21505594.2017.1313372
Rutherford, S. T., Bassler, B. L. (2012). Bacterial quorum sensing: its role in virulence and possibilities for its control. Cold Spring Harbor Perspect. Med. 2, a012427–a012427. doi: 10.1101/cshperspect.a012427
Saiman, L., Marshall, B. C., Mayer-Hamblett, N., Burns, J. L., Quittner, A. L., Cibene, D. A., et al. (2003). Azithromycin in patients with cystic fibrosis chronically infected with pseudomonas aeruginosa: a randomized controlled trial. JAMA 290, 1749–1756.
Sambanthamoorthy, K., Gokhale, A. A., Lao, W., Parashar, V., Neiditch, M. B., Semmelhack, M. F., et al. (2011). Identification of a novel benzimidazole that inhibits bacterial biofilm formation in a broad-spectrum manner. Antimicrob. Agents Chemother. 55, 4369–4378.
Sanderson, A. R., Leid, J. G., Hunsaker, D. (2006). Bacterial biofilms on the sinus mucosa of human subjects with chronic rhinosinusitis. Laryngoscope 116, 1121–1126. doi: 10.1097/01.mlg.0000221954.05467.54
Schaaper, R. M., Dunn, R. L. (1987). Spectra of spontaneous mutations in Escherichia coli strains defective in mismatch correction: the nature of in vivo DNA replication errors. Proc. Natl. Acad. Sci. 84, 6220–6224. doi: 10.1073/pnas.84.17.6220
Schroeder, J. W., Yeesin, P., Simmons, L. A., Wang, J. D. (2018). Sources of spontaneous mutagenesis in bacteria. Crit. Rev. Biochem. Mol. Biol. 53, 29–48. doi: 10.1080/10409238.2017.1394262
Shabbir, M. A., Wu, Q., Shabbir, M. Z., Sajid, A., Ahmed, S., Sattar, A., et al. (2018). The CRISPR-cas system promotes antimicrobial resistance in Campylobacter jejuni. Future Microbiol. 13, 1757–1774. doi: 10.2217/fmb-2018-0234
Sharma, D., Misba, L., Khan, A. U. (2019). Antibiotics versus biofilm: An emerging battleground in microbial communities. Antimicrobial Resistance Infection Control 8, 1–10. doi: 10.1186/s13756-019-0533-3
Sinclair, J. R. (2019). Importance of a One Health approach in advancing global health security and the Sustainable Development Goals. Rev. Scientifique Technique l’OIE 38, 145–154. doi: 10.20506/rst.issue.38.1.2937
Snow, D. E., Everett, J., Mayer, G., Cox, S. B., Miller, B., Rumbaugh, K., et al. (2016). The presence of biofilm structures in atherosclerotic plaques of arteries from legs amputated as a complication of diabetic foot ulcers. J. Wound Care 25, S16–S22. doi: 10.12968/jowc.2016.25.sup2.s16
Stewart, P. S., Zhang, T., Xu, R., Pitts, B., Walters, M. C., Roe, F., et al. (2016). ‘Reaction–diffusion theory explains hypoxia and heterogeneous growth within microbial biofilms associated with chronic infections’. NPJ Biofilms Microbiomes 2, 16012. doi: 10.1038/npjbiofilms.2016.12
Stewart, P. S., Franklin, M. J. (2008). Physiological heterogeneity in biofilms. Nat. Rev. Microbiol. 6, 199–210. doi: 10.1038/nrmicro1838
Sun, J., Deng, Z., Yan, A. (2014). Bacterial multidrug efflux pumps: Mechanisms, physiology and pharmacological exploitations. Biochem. Biophys. Res. Commun. 453, 254–267. doi: 10.1016/j.bbrc.2014.05.090
Swidsinski, A., Mendling, W., Loening-Baucke, V., Ladhoff, A., Swidsinski, S., Hale, L. P., et al. (2005a). Adherent biofilms in bacterial vaginosis. Obstetrics Gynecol. 106, 1013–1023. doi: 10.1097/01.aog.0000183594.45524.d2
Swidsinski, A., Weber, J., Loening-Baucke, V., Hale, L. P., Lochs, H. (2005b). Spatial organization and composition of the mucosal flora in patients with inflammatory bowel disease. J. Clin. Microbiol. 43, 3380–3389. doi: 10.1128/jcm.43.7.3380-3389.2005
Tang, B., Gong, T., Zhou, X., Lu, M., Zeng, J., Peng, X., et al. (2019). Deletion of cas3 gene in Streptococcus mutans affects biofilm formation and increases fluoride sensitivity. Arch. Oral. Biol. 99, 190–197. doi: 10.1016/j.archoralbio.2019.01.016
Tao, S., Chen, H., Li, N., Liang, W. (2022). The application of the CRISPR-cas system in antibiotic resistance. Infect. Drug Resist. 15, 4155–4168. doi: 10.2147/IDR.S370869
Trebino, M. A., Shingare, R. D., MacMillan, J. B., Yildiz, F. H. (2021). Strategies and approaches for discovery of small molecule disruptors of biofilm physiology. Molecules 29, 4582. doi: 10.3390/molecules26154582
Van Acker, H., Coenye, T. (2017). The role of reactive oxygen species in antibiotic-mediated killing of bacteria. Trends Microbiol. 25, 456–466. doi: 10.1016/j.tim.2016.12.008
van Boxtel, R., Wattel, A. A., Arenas, J., Goessens, W. H., Tommassen, J. (2017). Acquisition of carbapenem resistance by plasmid-encoded-ampC-expressing escherichia coli. Antimicrobial Agents Chemotherapy 61, 10–128. doi: 10.1128/AAC.01413-16
Walters, M. C., III, Roe, F., Bugnicourt, A., Franklin, M. J., Stewart, P. S. (2003). Contributions of antibiotic penetration, oxygen limitation, and low metabolic activity to tolerance of Pseudomonas aeruginosa biofilms to ciprofloxacin and tobramycin. Antimicrobial Agents Chemotherapy 47, 317–323. doi: 10.1128/AAC.47.1.317-323.2003
Wan, F., Draz, M. S., Gu, M., Yu, W., Ruan, Z., Luo, Q. (2021). Novel strategy to combat antibiotic resistance: A sight into the combination of CRISPR/cas9 and nanoparticles. Pharmaceutics 13, 352. doi: 10.3390/pharmaceutics13030352
Wang, F., Wang, L., Zou, X., Duan, S., Li, Z., Deng, Z., et al. (2019). Advances in CRISPR-Cas systems for RNA targeting, tracking and editing. Biotechnol. Adv. 37, 708–729. doi: 10.1016/j.biotechadv.2019.03.016
Watson, B. N. J., Steens, J. A., Staals, R. H., Westra, E. R., van Houte, S. (2021). Coevolution between bacterial CRISPR-Cas systems and their bacteriophages. Cell Host Microbe 29, 715–725. doi: 10.1016/j.chom.2021.03.018
Watson, B. N. J., Staals, R. H. J., Fineran, P. C. (2018). CRISPR-cas-mediated phage resistance enhances horizontal gene transfer by transduction. mBio 9, 10–128. doi: 10.1128/mBio.02406-17
Welch, J. L. M., Rossetti, B. J., Rieken, C. W., Dewhirst, F. E., Borisy, G. G. (2016). Biogeography of a human oral microbiome at the micron scale. Proc. Natl. Acad. Sci. 113, E791–E800. doi: 10.1073/pnas.1522149113
Wenderska, I. B., Chong, M., McNulty, J., Wright, G. D., Burrows, L. L. (2011). Palmitoyl-Dl-Carnitine is a multitarget inhibitor of Pseudomonas aeruginosa biofilm development. Chem. Biochem. 12, 2759–2766.
Werner, E., Roe, F., Bugnicourt, A., Franklin, M. J., Heydorn, A., Molin, S., et al. (2004). Stratified growth in Pseudomonas aeruginosa biofilms. Appl. Environ. Microbiol. 70, 6188–6196. doi: 10.1128/AEM.70.10.6188-6196.2004
Wiese, A., Brandenburg, K., Ulmer, A. J., Seydel, U., Müller-Loennies, S. (1999). The dual role of lipopolysaccharide as effector and target molecule. Biol. Chem. 38, 767–784. doi: 10.1515/BC.1999.097
Wilkins, M., Hall-Stoodley, L., Allan, R. N., Faust, S. N. (2014). ‘New approaches to the treatment of biofilm-related infections’. J. Infection 69, S47–S52. doi: 10.1016/j.jinf.2014.07.014
Williamson, K. S., Richards, L. A., Perez-Osorio, A. C., Pitts, B., McInnerney, K., Stewart, P. S., et al. (2012). Heterogeneity in pseudomonas aeruginosa biofilms includes expression of ribosome hibernation factors in the antibiotic-tolerant subpopulation and hypoxia-induced stress response in the metabolically active population. J. Bacteriology 194, 2062–2073. doi: 10.1128/JB.00022-12
Wilmaerts, D., Windels, E. M., Verstraeten, N., Michiels, J. (2019). General mechanisms leading to persister formation and awakening. Trends Genet. 35, 401–411. doi: 10.1016/j.tig.2019.03.007
Wilton, M., Charron-Mazenod, L., Moore, R., Lewenza, S. (2016). Extracellular DNA acidifies biofilms and induces aminoglycoside resistance in Pseudomonas aeruginosa. Antimicrobial Agents Chemotherapy 60, 544–553. doi: 10.1128/AAC.01650-15
Woodford, N., Ellington, M. J. (2007). The emergence of antibiotic resistance by mutation. Clin. Microbiol. Infection 13, 5–18. doi: 10.1111/j.1469-0691.2006.01492.x
Yan, J., Kang, D. D., Dong, Y. (2021). Harnessing lipid nanoparticles for efficient CRISPR delivery. Biomaterials Sci. 9, 6001–6011. doi: 10.1039/D1BM00537E
Yarwood, J. M., Bartels, D. J., Volper, E. M., Greenberg, E. P. (2004). Quorum sensing in Staphylococcus aureus biofilms. J. Bacteriology 186, 1838–1850. doi: 10.1128/JB.186.6.1838-1850.2004
Yosef, I., Manor, M., Kiro, R., Qimron, U. (2015). Temperate and lytic bacteriophages programmed to sensitize and kill antibiotic-resistant bacteria. Proc. Natl. Acad. Sci. 112, 7267–7272. doi: 10.1073/pnas.1500107112
Zhang, L., Mah, T.-F. (2008). Involvement of a novel efflux system in biofilm-specific resistance to antibiotics. J. Bacteriology 190, 4447–4452. doi: 10.1128/JB.01655-07
Zhou, E., Seminara, A. B., Kim, S.-K., Hall, C. L., Wang, Y., Lee, V. T. (2017). Thiol-benzo-triazolo-quinazolinone inhibits alg44 binding to c-di-gmp and reduces alginate production by pseudomonas aeruginosa. ACS Chem. Biol. 12, 3076–3085.
Zijnge, V., Leeuwen, M. B. M., Degener, J. E., Abbas, F., Thurnheer, T., Gmür, R., et al. (2010). Oral biofilm architecture on natural teeth. PloS One 5, e9321. doi: 10.1371/journal.pone.0009321
Zlosnik, J. E., Costa, P. S., Brant, R., Mori, P. Y., Hird, T. J., Fraenkel, M. C., et al. (2011). Mucoid and nonmucoid Burkholderia cepacia complex bacteria in cystic fibrosis infections. Am. J. Respir. Crit. Care Med. 183, 67 –672.
Zobell, C. E. (1943). The effect of solid surfaces upon bacterial activity. J. Bacteriology 46, 39–56. doi: 10.1128/jb.46.1.39-56.1943
Zuberi, A., Ahmad, N., Khan, A. U. (2017a). CRISPRi induced suppression of fimbriae gene (fimH) of a uropathogenic escherichia coli: an approach to inhibit microbial biofilms. Front. Immunol. 8. doi: 10.3389/fimmu.2017.01552
Zuberi, A., Azam, M. W., Khan, A. U. (2022). ‘CRISPR interference (CRISPRi) mediated suppression of ompR gene in E. coli: an alternative approach to inhibit biofilm’. Curr. Microbiol. 79, 78. doi: 10.1007/s00284–021-02760-x
Keywords: antibiotic resistance, biofilm, CRSISR/Cas9, bacteria, gene editing, infections
Citation: Zuberi A, Ahmad N, Ahmad H, Saeed M and Ahmad I (2024) Beyond antibiotics: CRISPR/Cas9 triumph over biofilm-associated antibiotic resistance infections. Front. Cell. Infect. Microbiol. 14:1408569. doi: 10.3389/fcimb.2024.1408569
Received: 28 March 2024; Accepted: 27 May 2024;
Published: 05 July 2024.
Edited by:
Vineet Kumar, Central University of Rajasthan, IndiaReviewed by:
Sanket Kaushik, Amity University Jaipur, IndiaAntónio Machado, Universidad San Francisco de Quito, Ecuador
Azaj Ahmed, The University of Iowa, United States
Copyright © 2024 Zuberi, Ahmad, Ahmad, Saeed and Ahmad. This is an open-access article distributed under the terms of the Creative Commons Attribution License (CC BY). The use, distribution or reproduction in other forums is permitted, provided the original author(s) and the copyright owner(s) are credited and that the original publication in this journal is cited, in accordance with accepted academic practice. No use, distribution or reproduction is permitted which does not comply with these terms.
*Correspondence: Nayeem Ahmad, ZHIubmF5ZWVtYWhtYWRhaWltc0BnbWFpbC5jb20=