- 1Department of Bacteriology, Division for Infection Control and Environmental Health, Norwegian Institute of Public Health, Oslo, Norway
- 2Department of Biosciences, Section for Genetics and Evolutionary Biology, University of Oslo, Oslo, Norway
The genus Neisseria, which colonizes mucosal surfaces, includes both commensal and pathogenic species that are exclusive to humans. The two pathogenic Neisseria species are closely related but cause quite different diseases, meningococcal sepsis and meningitis (Neisseria meningitidis) and sexually transmitted gonorrhea (Neisseria gonorrhoeae). Although obvious differences in bacterial niches and mechanisms for transmission exists, pathogenic Neisseria have high levels of conservation at the levels of nucleotide sequences, gene content and synteny. Species of Neisseria express broad-spectrum O-linked protein glycosylation where the glycoproteins are largely transmembrane proteins or lipoproteins localized on the cell surface or in the periplasm. There are diverse functions among the identified glycoproteins, for example type IV biogenesis proteins, proteins involved in antimicrobial resistance, as well as surface proteins that have been suggested as vaccine candidates. The most abundant glycoprotein, PilE, is the major subunit of pili which are an important colonization factor. The glycans attached can vary extensively due to phase variation of protein glycosylation (pgl) genes and polymorphic pgl gene content. The exact roles of glycosylation in Neisseria remains to be determined, but increasing evidence suggests that glycan variability can be a strategy to evade the human immune system. In addition, pathogenic and commensal Neisseria appear to have significant glycosylation differences. Here, the current knowledge and implications of protein glycosylation genes, glycan diversity, glycoproteins and immunogenicity in pathogenic Neisseria are summarized and discussed.
1 Introduction
Glycosylation is one of the most abundant and complex post translational modifications (PTMs) of living organisms. Bacteria have the ability to synthesize a variety of sugar structures such as capsular polysaccharides, lipooligosaccharides or lipopolysaccharides, peptidoglycans as well as N- and O-linked glycans of proteins. Understanding the biological significance of bacterial protein glycosylation has been hindered in part by the considerable diversity in structures and functions of glycans.
Early gas chromatographic analysis of Neisseria gonorrhoeae pili indicated the presence of 1–2 hexose groups per pilin subunit (Robertson et al., 1977). The pilin subunit PilE was later found to undergo O-linked glycosylation in both Neisseria meningitidis (Stimson et al., 1995) and N. gonorrhoeae (Parge et al., 1995). Further studies have shown that glycosylation has significant effects on the antigenicity and immunogenicity of the PilE protein (Børud et al., 2010). In 2009, with identification of eleven additional glycoproteins in N. gonorrhoeae (Vik et al., 2009) and AniA (aka NirK) in N. meningitidis (Ku et al., 2009), the first general O-linked protein glycosylation system in bacteria was described and is now one of the better characterized systems. In Gram negative bacteria, such O-linked protein glycosylation is characterized by the glycan being synthesized on an undecaprenyl pyrophosphate (Und-PP) lipid carrier in the cytoplasm, its subsequent flipping across the inner membrane to the periplasm and oligosaccharyltransferase (O-OTase) - mediated glycosylation of either serine or threonine residues of target proteins (Nothaft and Szymanski, 2010). In addition to Neisseria, O-linked protein glycosylation of this type has also been identified in Burkholderia (Lithgow et al., 2014), Francisella (Egge-Jacobsen et al., 2011), Acinetobacter (Iwashkiw et al., 2012), and the plant pathogen Ralstonia solanacearum (Elhenawy et al., 2016).
The genus Neisseria consist of Gram negative, oxidase-positive diplococci that are associated with mucosal surfaces. The two pathogenic Neisseria species are human restricted, substantial threats to global health and closely related, but cause very different diseases, meningococcal disease (N. meningitidis) and sexually transmitted gonorrhea (N. gonorrhoeae). N. meningitidis is most often a commensal bacterium with varying carriage prevalence (3–30%) in the human oropharynx (Trotter and Greenwood, 2007) that occasionally can cause invasive disease resulting in severe meningitis and/or septicemia. N. gonorrhoeae causes gonorrhea by primary colonization of the epithelium of the male urethra and female ectocervix and endocervix but can occasionally also cause pelvic inflammatory disease. The gonococcus can infect other mucous membranes in the genitourinary tract, rectum, oral cavity, pharynx, and eyes and might cause disseminated gonococcal infections. Despite their apparent differences, these pathogenic species are remarkably conserved and comprise a distinct clade in phylogenetic relationship to other Neisseria species (Bennett et al., 2012).
Studies of protein glycosylation have revealed that Neisseria species encompass numerous glycoproteins and display high intra- and interstrain glycoform variability (Ku et al., 2009; Vik et al., 2009; Børud et al., 2010, 2011; Anonsen et al., 2012b; Børud et al., 2014; Anonsen et al., 2017; Hadjineophytou et al., 2024). To date, it has been shown that one strain has the capacity to express between 7 and 15 different glycoforms by differing combinations of glycosyltransferases and the glycan O-acetylation (Anonsen et al., 2017; Wang et al., 2021). The complexity can be attributed to different molecular mechanisms and evolutionary events such as the pgl gene content, phase variable pgl genes, and allelic pgl variants where hypermorph, hypomorph, amorphic and even neomorph glycosyltransferases have been identified (Chamot-Rooke et al., 2007; Børud et al., 2011; Johannessen et al., 2012; Børud et al., 2014). In addition, Neisseria species display glycan microheterogeneity (different glycan structures at the same site of a protein) and macroheterogeneity (presence/absence of glycans at a particular residue).
2 Pilin is the major glycoprotein
Neisseria employ diverse mechanisms to alter the structure of their immune-exposed surface antigens. These involve hypervariable loci in their genomes; acting as diversity hotspots. This diversity provides a vast repertoire of epitopes for surface antigens and facilitate evasion of adaptive immune responses targeting specific variants. The type IV pilus colonization factor represents a major surface exposed antigen, and it is assembled in the periplasm and transported through the outer membrane by a complex involving over 20 different proteins. Type IV pili in Neisseria are homopolymers of the PilE protein that can extend several micrometers from the cell surface and are involved in initial attachment to epithelial and endothelial cells (Nassif et al., 1997), natural competence (Fussenegger et al., 1997) and twitching motility (Wolfgang et al., 1998).
A gonorrhea pilus vaccine was shown to be safe and antigenic, and it elicited a broad antibody response in serum and genital secretions that blocked attachment of gonococci to epithelial cells (Mcchesney et al., 1982). Disappointingly, a large-scale trial of the vaccine showed no evidence for protection; most probably due to pilus variation between the vaccine strain and the circulating strains (Boslego et al., 1991). The PilE pilin protein subunit is subject to high frequency, antigenic variation through gene conversion that results in dramatic changes in primary structure. At the time of the vaccine trial, it was not recognized that PilE was glycosylated. The neisserial pilins have been shown to have many different PTMs, including the glycan variants that will be further detailed here, but also phosphoform modifications such as phosphoethanolamine, phosphocholine, and phosphoglycerol (Stimson et al., 1996; Weiser et al., 1998; Hegge et al., 2004; Aas et al., 2006; Chamot-Rooke et al., 2011). The phosphoform modifications that are found near sites of glycan attachment might modulate pilin antigenicity and have the potential for dynamic interplay between the PTMs (Anonsen et al., 2012a). For instance, it has been suggested that pili modified with both glycan and phosphocholine is required for efficient meningococcal adherence to platelet activating factor receptor on human airway cells (Jen et al., 2013).
Two distinct classes of PilE have been identified where Class I pili are expressed by all N. gonorrhoeae isolates and certain N. meningitidis isolates, while Class II pili are found in the other N. meningitidis isolates. Class I pili exhibit high variability involving frequent gene conversion events via homologous recombination between the pilE locus and a series of inactive pilS silent, truncated gene copies (Hagblom et al., 1985; Zhang et al., 1992). In contrast to the situation for Class I pilin that carries a single glycosylated residue, N. meningitidis isolates that express antigenic invariable Class II pilin display multiple pilin glycosylation sites (Gault et al., 2015). Gault and colleagues hypothesized that N. meningitidis isolates that express antigenic invariable Class II pilins carry multiple pilin glycosylation sites to evade the immune system by fully masking protein epitopes (Gault et al., 2015).
In one earlier study, elimination of meningococcal pilin glycosylation was associated with modest increases in piliation levels and adherence to human epithelial cells (Virji et al., 1993; Marceau et al., 1998). It has also been suggested that gonococcal infection of the cervical epithelium requires the combined action of pilin, porin and iC3b (an opsonin and ligand for complement 3 receptor (CR3)) (Edwards et al., 2002), and that the pilin glycan is required for binding to iC3b and thus involved in activation of the CR3 (Jennings et al., 2011). PilE glycosylation has also been shown to impact on type IV pilin functions such as autoagglutination, the efficiency of pilin subunit polymerization, and the dynamics of pilus extension - retraction (Vik et al., 2012; Gault et al., 2015). In addition to PilE, several additional type IV biogenesis or related proteins (PilQ, PilN, PilH, PilI, PilJ, PilV, ComP) are glycosylated (Hadjineophytou et al., 2022, 2024) (Table 1). In contrast, the more distant commensal neisserial species have O-OTases that supported glycosylation of other proteins, but not pilin (Hadjineophytou et al., 2022). Interestingly, Acinetobacter have two functional O-OTases where one glycosylates type IV pilin, while the other glycosylates multiple proteins (Harding et al., 2015). It appears that closely related bacterial O-OTases have evolved to target distinct substrates.
3 Diverse functions of neisserial glycoproteins
Altogether, over 50 glycoproteins have been identified in N. gonorrhoeae (Vik et al., 2009; Anonsen et al., 2012b; Hadjineophytou et al., 2024). The glycoproteins identified are mainly lipoproteins or transmembrane – domain containing proteins localized extracytoplasmically (as summarized in Table 1). A few of these glycoproteins have also been characterized in N. meningitidis (Stimson et al., 1995; Ku et al., 2009; Børud et al., 2010). However, immunoblotting with monoclonal glycan - specific antibodies shows a comparable repertoire of glycoproteins in both pathogenic species (Børud et al., 2010). Many diverse functions are associated with the glycoproteins, along with the type IV pilus biogenesis proteins, several glycoproteins are for instance involved in antimicrobial resistance or suggested as vaccine candidates for N gonorrhoeae.
Several gonococcal proteins that elicit bactericidal or functional blocking antibodies have been suggested as vaccine candidates are known glycoproteins (Table 1), i.e PilE (Boslego et al., 1991), Mip (Leuzzi et al., 2005; Humbert and Christodoulides, 2018), AniA (Shewell et al., 2017), PilQ (Haghi et al., 2012; Leduc et al., 2020), MetQ (Semchenko et al., 2017; Sikora et al., 2020), PorB (Zhu et al., 2005), ZnuD (Stork et al., 2010; Baarda et al., 2021) and PotF3 (Liu et al., 2017). Some vaccines against serogroup B meningococcal (MenB) disease have been based on outer membrane vesicles (OMVs) that include a broad range of OMV proteins, and the 4CMenB also contains recombinant protein antigens to increase the protection across diverse MenB strains (Holst et al., 2009; Micoli and Maclennan, 2020; Viviani et al., 2022). Although not the major antigens in these vaccines, there are numerous antigens that contribute to the protective effect against MenB strains that are known glycoproteins; PorB (Viviani et al., 2023), PilQ (Haghi et al., 2012), Ag473 (Chu et al., 2012), RmpM (Rosenqvist et al., 1999; Williams et al., 2014), and Mip (Humbert and Christodoulides, 2018) (Table 1). Given the fact that glycans impact on the immunogenicity, as shown for the PilE protein (Børud et al., 2010), glycan function and diversity may need to be considered when including glycoproteins in future vaccines. As such, the complete glycoproteomes, potential intra- and interspecies differences should be investigated to understand the full, biological significance of glycosylation.
Emergence of multidrug resistant N. gonorrhoeae is making treatment more difficult, and the risk of untreatable disease represents a major global public health concern. It is therefore important to understand the molecular and phenotypic mechanisms involved. The recent identification of additional glycoproteins revealed that several of those are involved in antimicrobial resistance mechanisms (Table 1) (Hadjineophytou et al., 2024). The detected glycoproteins are involved in efflux (MtrC, MtrD, MacA, MacB, FarA) and influx (PorB) of antimicrobials. The MtrCDE efflux pump exports diverse hydrophobic antimicrobials (macrolides, penicillin, ciprofloxacin and tetracyclin), while the MacAB efflux pump export macrolides and FarAB efflux pump export cationic antimicrobial peptides and long-chain fatty acids (Unemo and Shafer, 2014). The glycoproteins PorB/PenB, PilQ and PonA are involved in resistant to penicillin through different mechanisms (Unemo and Shafer, 2014). The potential influence of glycosylation of these proteins on antimicrobial resistance through molecular fine tuning or altering activity remains to be considered.
By examining known glycopeptides in N. gonorrhoeae MS11 replicates using Data-Independent Acquisition (DIA) analysis, different glycosylation occupancy frequencies were found without affecting the protein abundance (Hadjineophytou et al., 2024). This study also discovered that the glycan occupancy on glycoproteins was often low. There are few if any studies addressing potential regulation of protein glycosylation in Neisseria. However, information about regulation mechanisms could impact on our understanding of protein glycosylation; both on the level of individual glycoproteins and their functions, as well as on our understanding of glycan diversity and immune escape mechanisms.
4 Neisseria protein glycans are immunogenic
The surfaces of all cells in nature exhibit taxon-, species-, and cell-type-specific characteristics in their intricate layer of glycans (Varki, 2011). Serving as major components of outermost surface molecules, glycans play crucial roles in many processes. In general, this includes host-pathogen interactions, immunological recognition and activation, and differentiation between self and nonself through a sophisticated array of pathways and mechanisms. Microbes often exploit host glycans as targets for cellular binding and tissue invasion and some have developed mechanisms of glycan mimicry or extensive glycan variability to elude the host response. Additionally, microbial glycans can serve as a protective glycan shield by hindering access to underlying protein epitopes as reviewed elsewhere (Zhou and Cobb, 2021).
The adaptive immune system in vertebrate organisms primarily operates through the recognition of foreign peptide sequences. These sequences are directly acknowledged by the B cell surface Ig receptor and are also loaded into the grooves of major histocompatibility receptors for presentation to specific T-cell receptors (Hoogeboom and Tolar, 2016). When the peptide carries a small glycan, this component can introduce novel specificity to recognition of the peptide (Varki, 2017). In fact, PilE-associated glycans are immunogenic, as well as antigenically variable when expressed in different protein glycosylation (pgl) gene backgrounds (Børud et al., 2010).
Recently, we suggested that meningococcal carriage and disease stimulate production of antibodies against different neisserial glycoforms in humans. We found that most of the Ethiopian patients (83%) infected with serogroup A ST-7 N. meningitidis and a proportion of the control group (24%) without any history of meningococcal disease, had antibodies against neisserial protein glycan antigens (Naess et al., 2023). In addition, by using a bactericidal assay comparing a wild type meningococcal A strain and a glycosylation-null variant strain, it was shown that the protein glycan antigens may protect against bactericidal killing by antibodies in Ethiopian patient sera, possibly by masking protein epitopes important for bactericidal killing and thus protection against meningococcal disease (Naess et al., 2023). The pilin glycan may be a target for anti-Gal IgA antibodies in natural human serum that has been reported to bind to meningococcal pili and block complement-mediated lysis (Hamadeh et al., 1995).
There are several successful vaccines targeting capsular polysaccharides in bacteria, such as the highly immunogenic and efficient conjugated vaccines against Streptococcus pneumoniae, Haemophilus influenzae type b, and N. meningitidis capsular polysaccharides for serogroups A, C, W and Y. These carbohydrate-based vaccines are made by conjugation of extracted polysaccharides to different carrier proteins (Lindberg, 1999). Bacterial protein glycosylation pathways can also be exploited in glyco-engineering to create glycoconjugates by using oligosaccharyltransferases to generate bacterial vaccines (Feldman et al., 2005; Price et al., 2016). To our knowledge however, the only engineered vaccines targeting glycan antigens of glycoproteins involve the Campylobacter jejuni N-glycan developed for chickens. Two different glycoconjugate vaccines were constructed; the N-glycan attached to a protein carrier or fused to the Escherichia coli lipopolysaccharide-core. Vaccination of chickens with either showed reduction in C. jejuni colonization and induced glycan-specific IgY responses (Nothaft and Szymanski, 2010). Transplantation of microbiota in combination with vaccination further increased the vaccine-induced antigen-specific IgY responses (Nothaft et al., 2021). It is worth noting in this context that the majority of the N-glycan found in C. jejuni is found in its free oligosaccharide form (Nothaft et al., 2009).
5 Glycosylation pathway and pgl gene content
An overview of the currently known glycosylation pathways in pathogenic Neisseria is shown in Figure 1. The pgl core locus products function in the synthesis of Und-PP monosaccharides on the cytoplasmic face of the inner membrane (PglB/B2, PglC, PglD) and translocation into the periplasm (PglF). PglB is a bifunctional protein (acetyltransferase/phosphorglycosyl-transferase) responsible for synthesis of N,N′-diacetyl-bacillosamine (diNAcBac), while the variant PglB2 (ATP grasp/phosphorglycosyl-transferase) is responsible for synthesis of glyceramido acetamido trideoxyhexose (GATDH) (Chamot-Rooke et al., 2007). PglA and PglE are galactosyltransferases that elaborate the mono- and disaccharide, respectively, by adding galactose (Gal) (Aas et al., 2007). PglH/H2 is a glycosyltransferase that generate glucose (Glc) or N-acetylglucosamine (GlcNAc)-containing disaccharides, respectively. Alternatively, PglH2 can extend the PglA disaccharide to a GlcNAc terminating trisaccharide (Børud et al., 2011, 2014). It has also recently been shown that pglG alleles from N. meningitidis are associated with incorporation of an N-acetylhexosamine (HexNAc) at the third position (Wang et al., 2021). Diminished level of pilin-linked glycan in N. gonorrhoeae pglF mutants, together with PilF homology to ABC transporter-type flippases implies a role in the translocation of the Und-PP-linked glycan across the periplasmic membrane (Aas et al., 2007; Hartley et al., 2011). PglF continuously translocates the glycans into the periplasm during synthesis and cause some of the observed microheterogeneity. PglO (aka PglL) is the O-OTase that transfers the glycan to protein substrates (Power et al., 2006; Aas et al., 2007). A recent study by Hadjineophytou and colleagues demonstrate how different neisserial PglOs have distinct protein targeting activities (Hadjineophytou et al., 2022). In addition, neisserial glycoforms can be further modified via O-acetylation mediated by the acetyltransferase PglI (Aas et al., 2007; Børud et al., 2014). Altogether, 31 distinct glycoforms have been identified in pathogenic Neisseria; all of these are found within N. meningitidis while N. gonorrhoeae (lacking pglB2) is only capable of synthesizing 15 different glycoforms (Figure 2) (Anonsen et al., 2017). The high glycan diversity reveals that the enzymes acting downstream of the synthesis of the Und-PP-linked saccharides (PglB/PglB2-associated glycosyl-1-phosphate transferase, PglF flippase, and PglO O-OTase) retain relaxed donor specificity.
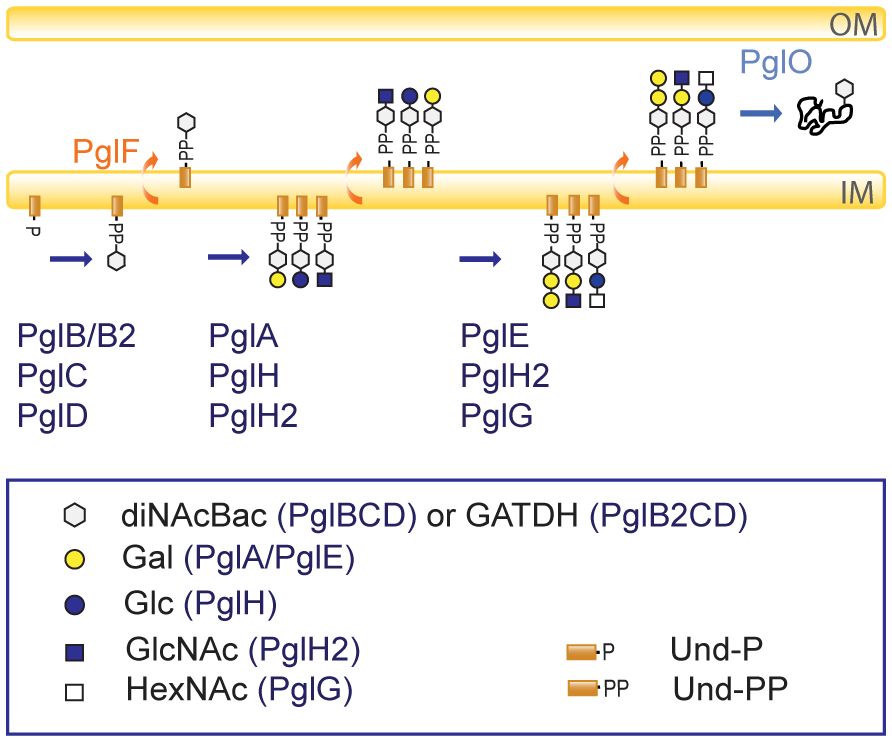
Figure 1 Simplified overview of the O-linked protein glycosylation pathway in N. gonorrhoeae and N. meningitidis. PglB/PglB2, PglC, and PglD synthesizes the undecaprenyl diphosphate (Und-PP) -linked monosaccharides. The PglB variant synthesizes the monosaccharide N, N′-diacetylbacillosamine (diNAcBac), while the PglB2 variant is responsible for synthesis of glyceramido-acetamido trideoxyhexose (GATDH). PglF translocates the synthesized glycans into the periplasm continuously. The glycosyltransferases PglA, PglH, PglH2 adds galactose (Gal), glucose (Glc) or N-acetylglucosamine (GlcNAc), respectively, to the monosaccharides. The glycosyltransferases PglE, PglH2 and PglG add galactose (Gal), glucose, N-acetylglucosamine (GlcNAc) or an N-acetylhexosamine (HexNAc), respectively, to the disaccharides. The O-OTase PglO transfers the glycans onto proteins in the periplasm. O-acetylation is mediated by the acetyltransferase PglI (not shown here, see Figure 2 for further details on acetylation). The figure legend shows the symbols used with the involved Pgl protein in parenthesis. OM, outer membrane; IM, inner membrane. All glycans in the figure can have either diNAcBac or GATDH as the first sugar and are therefore colored white (generic) according to the Symbol Nomenclature for Glycans (https://www.ncbi.nlm.nih.gov/glycans/snfg.html).
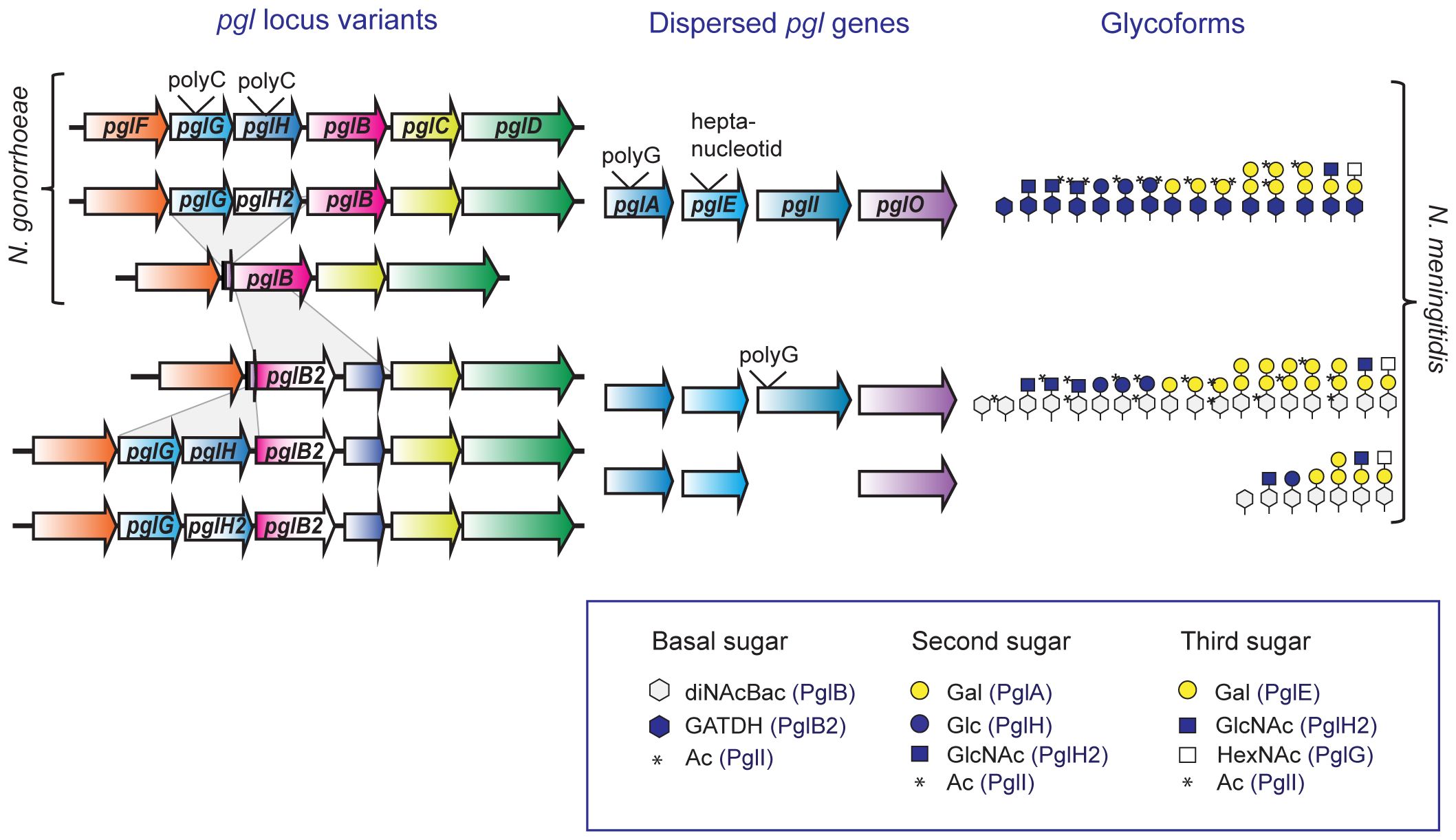
Figure 2 Protein glycosylation gene variants and glycoform outcome in pathogenic Neisseria. An overview of the pgl genes present in different pgl loci variants and the currently known glycoforms synthesized by N. gonorrhoeae and N. meningitidis carrying these gene combinations. The pgl genes pglF, pglG, pglH, pglB/B2, pglC, and pglD are linked together in the pgl locus, whereas pglA, pglE, pglI and pglO are dispersed elsewhere. The two major recombination events in the pgl loci are shown, the pglB2-ORF8 insertion and the pglG-pglH deletion, see main text for more details. The genes pglA, pglE, pglG and pglH/H2 contains phase variable tracts as shown, although there are also non-phase variable allele variants for these genes. The pglI can be missing or phase variable in N. meningitidis, but non-phase variable in N. gonorrhoeae. The figure legend shows the symbols used with the involved Pgl protein in parenthesis. diNAcBac, N, N′-diacetylbacillosamine; GATDH, glyceramido-acetamido trideoxyhexose; Gal, galactose; Glc, glucose; HexNAc, N-acetylhexosamine; GlcNAc, N-acetylglucosamine; Ac, acetyl. Contrary to the generic white color of the first sugar in Figure 1, we here used white for GATDH glycoform variants and blue for diNAcBac variants according to the Symbol Nomenclature for Glycans (https://www.ncbi.nlm.nih.gov/glycans/snfg.html).
Genomic analyses of Neisseria species have shown variation due to horizontal gene transfer, both at the level of sequence diversity and gene content variation (Kong et al., 2013). Within neisserial pgl genes, there are both intra-species and inter-species genetic variation (Figure 2). There are two major polymorphisms at the core pgl locus; the variable presence of pglG and pglH, and the mutually exclusive presence of pglB and pglB2. While pglB, pglG and pglH are found in both pathogenic species, pglB2 are only found within N. meningitidis and commensal Neisseria. The pglB2-ORF8 fragment have been inserted into the pglB gene, and both PglB and PglB2 have the same N-terminal domain with phosphoglycosyl-transferase activity (Kahler et al., 2001; Power et al., 2003). Neisserial strains carrying deletions of pglG and pglH/H2 still bear conserved traces of the 5′ end of pglG and 3′ end of pglH; suggesting that the intact state is ancestral and that a deletion event likely occurred once and then spread through the populations (Børud et al., 2011). The presence of pglG-pglH was reported in 67% of strains in a predominantly meningococcal strain collection (Power et al., 2003) and in our previous study to 81% of gonococcal strains, 65% of meningococcal strains, and 94% of commensal strains (Børud et al., 2011). In addition, we have reported that polymorphism also exist at gene level as described for pglH/pglH2 where a single non-synonymous mutation accounts for the glycoform switch from Glc to GlcNAc (Børud et al., 2014).
The glycosyltransferase genes pglA and pglE are not linked to the pgl loci or one another, and they are present in all pathogenic strains while absent in most commensal species. Hadjineophytou and colleagues suggest that the terminating galactose residues may have favorable function for the pathogenic Neisseria, for example in regard to recognition of the immune system, glycoproteins abundance or properties, or with variable metabolic costs (Hadjineophytou et al., 2022). The authors also proposed that the elaboration of monosaccharides followed two different pathways; the pglA-pglE pathway whose products add galactose to generate di- and trisaccharides and the pathway involving the pglG-pglH/H2 insertion resulting in glucose- and/or GlcNAc -containing glycans (Hadjineophytou et al., 2022). Furthermore, as both pathways are active in several neisserial strains, competition and redundancy may be responsible for amorphic and hypomorphic pglA and pglH alleles, as well as the pglG-pglH deletion (Børud et al., 2011; Johannessen et al., 2012; Hadjineophytou et al., 2022).
The glycan diversity appears to be even more complex in commensals than the two pathogenic species (Hadjineophytou et al., 2019). Since variant commensal pgl genes could be introduced by homologous recombination, this could significantly contribute to further glycan diversity in pathogenic Neisseria. In support of this, Jen et al. identified disaccharides and trisaccharides with an uncharacterized basal sugar in N. meningitidis serogroup A ST-2859 (Jen et al., 2023).
6 Glycan diversity and immune escape
Bacterial genetic variation is important to avoid the adaptive immune response, and extensive allelic diversity in the genus Neisseria has been described, particularly in genes under antigenic selection pressure. The mechanism of phase variation involves reversible, spontaneous changes in the expression of specific genes, and it is facilitated by a phenomenon known as slippage during DNA replication within simple DNA repeats, either in the promoters or within the open reading frames. Slippage and variations in the number of repetitive DNA sequences leads to altered gene expression or frameshift mutations. This dynamic process allows bacteria to rapidly switch on or off the expression of certain genes; promoting adaptability and facilitating survival in response to changing environmental pressures. In pathogenic Neisseria more than 100 phase variable genes was postulated (Snyder et al., 2001), including several pgl genes that have mononucleotide or polynucleotide repeats tracts (Power et al., 2003). In a more recent analysis using a substantially higher number of genomes, the number of phase variable genes was reduced to a maximum of 47 and 54 per genome of N. meningitidis and N. gonorrhoeae, respectively (Wanford et al., 2018).
Slipped-strand mispairing-induced phase variation occurs repeatedly, creating heterogeneity within the population, and manifesting in a diverse range of phenotypes. It has been shown that a single isolate has the capacity to express up to 15 different glycans by combination of glycosyltransferases and the O-acetylase (Anonsen et al., 2017; Wang et al., 2021). The phase variable expression of pglA, pglE, pglG pglH/H2 and pglI genes thus results in intrastrain glycan length variation (Power et al., 2003; Aas et al., 2007; Power et al., 2007; Børud et al., 2011; Anonsen et al., 2017; Wang et al., 2021). Consequently, the population consistently harbors variants pre-adapted to various environmental conditions and allowing them to withstand genetic bottlenecks by adjusting gene expression levels accordingly.
Protein glycan variation mediated by phase variation is unique to Neisseria, and the frequency of phase variable pgl genes appear to be higher in N. meningitidis than N. gonorrhoeae and is only found in the closely related commensals Neisseria lactamica and Neisseria polysaccharea (Børud et al., 2011; Wanford et al., 2018; Wang et al., 2021). For instance, the O-acetylation on oligosaccharides is subject to the phase-variable expression of pglI in N. meningitidis, while in N. gonorrhoeae pglI is non-phase variable and always expressed. As such, on–off modulation of PglI expression in N. meningitidis results in alterations between oligosaccharides with or without acetylation modification and thus increases the basic repertoires of oligosaccharides (Anonsen et al., 2017). Moreover, while pglI is present in all N. gonorrhoeae, it is absent in most commensals and some sequence types of N. meningitidis. Also, the pglA and pglE glycosyltransferase genes are absent in nearly all commensals, except for N. lactamica and N. polysaccharea (Børud et al., 2011; Hadjineophytou et al., 2019).
Within-host evolution involves the adaptation of a bacterial pathogen to colonization within a specific host. In N. meningitidis, within-host evolution is proposed to occur during the initial colonization phases by favoring variants that evade host immunity and colonize the epithelium and without necessarily selecting for increased fitness in invasive contexts. In one study, during an accidental human passage it was shown that pglA and pglI were turned off in the isolate retrieved from blood culture and not in the laboratory parental strain. Such simple sequence repeats tract variation led to altered glycoform expression, from diNAcBac-Gal-AcGal to diNAcBac-Glc, after human passage. The authors proposed that such changes could confer an advantage to the bacteria to escape the immune system (Omer et al., 2011).
Klughammer and colleagues examining paired isolates from throat swabs and blood culture in four patients with invasive meningococcal disease and detected phase variation of pilC1 and pglI, along with gene conversion events in pilE (Klughammer et al., 2017). In a recent study, sequence analysis revealed within-host genetic changes in paired meningococcal carriage isolates from Ethiopia when analyzing 50 carriers with samples taken six to nine weeks apart (Bårnes et al., 2017). Among the most frequently altered genes were genes belonging to the restriction/modification systems, opacity proteins and genes involved in pilin antigenic variation and protein glycosylation (pglG, pglH and pglI) (Bårnes et al., 2017). These pgl genes showed phase variable differences resulting in on-off expression within the paired isolates; ranging from 60% of the pairs for pglE and 20% for pglA (Børud et al., 2018). Similarly, in another study by Mustapha and colleagues comparing paired carriage isolates from 188 individuals, the most frequently altered genes were pilE, the opa loci, and the modA12 methyltransferase genes (Mustapha et al., 2021). Additionally, the phase variable pgl genes pglA, pglE, pglH and pglI exhibited high variability, and also a subset of genes underwent frequent microevolution during transmission but reached fixation during persistent carriage, including pilus biogenesis (pilH, pilT, pilU and pilQ) and glycosylation genes (pglD) (Mustapha et al., 2021). A comparable genetic pattern was noted in a controlled human infection study involving N. lactamica (Pandey et al., 2018). The study revealed that among hpuA, fetA, and hsdS, pilE and pilin glycosyltransferase genes (pglA and pglH) exhibited the highest variability during a one-month carriage period (Pandey et al., 2018).
There are also cases where homologous recombination within pgl genes results in permanent change of expressed glycans. One study compared genomes of serogroup Y, ST-23 clonal complex, and hypothesized that emergence of a late strain type was primarily due to antigenic changes that allowed escape from population immunity. Among the differences was a recombination event in the pgl loci exchanging pglB in the early strain type with pglB2 in the late strain type (Krauland et al., 2012). The phenotypic consequences for replacement of the pglB allele with pglB2, is synthesis of GATDH - based glycoforms instead of diNAcBac glycoforms (Chamot-Rooke et al., 2007; Børud et al., 2010). The same recombination event exchanging pglB with pglB2 was observed in two of 37 N. meningitidis serogroup A ST-7 isolates (Naess et al., 2023).
Another study detected sequence type specific homologous recombination within the pgl loci through genomic analysis of 100 isolates representing the clonal replacement of the hyper virulent serogroup A N. meningitidis clone ST-7 with the ST-2859 descendant clone. The authors suggested that this emphasized the role of protein glycosylation diversity in immune evasion (Lamelas et al., 2014). Our recent genome analysis of N. meningitidis serogroup A ST-7 isolates identified an IS element within pglH, and the pgl loci homolog recombination described by Lamelas and colleagues thus replaced the IS - disrupted pglH and likely led to increased glycan variability in ST-2859 compared to ST-7 isolates (Naess et al., 2023).
7 Concluding Remarks
In conclusion, glycosylation undoubtedly impacts on the antigenicity and immunogenicity of proteins, especially for the abundant and surface exposed pilin that has been extensively studied. As summarized above, several studies suggest that N. meningitidis evade the immune system by changing their protein glycan structures (Krauland et al., 2012; Lamelas et al., 2014; Gault et al., 2015). However, further studies are essential to confirm this hypothesis and to explore the mechanisms and potential differences in pathogenic and commensal Neisseria.
Understanding the diversity and biological role(s) of glycosylation employed by Neisseria can be crucial for developing effective vaccines and treatments, especially for N. gonorrhoeae where antimicrobial resistance is high and vaccine development has been difficult. Absence of natural protection after repeated gonorrhea infections or in previous vaccine attempts must, to some degree, be attributable to the high antigenically variable surface antigens in N. gonorrhoeae. A number of potential antigens are being considered and the general consensus is that a successful vaccine will incorporate multiple antigens. Attempts to identify conserved antigens through comparative genomics are ongoing but this approach has limitations because they overlook those whose structural features not linearly templated within genome sequences such as PTMs. Researchers are actively studying potential targets for intervention and to design strategies that can counteract the immune evasion tactics of these bacteria.
Author contributions
BB: Writing – review & editing, Writing – original draft. MK: Writing – review & editing, Writing – original draft.
Funding
The author(s) declare financial support was received for the research, authorship, and/or publication of this article. BB is supported by funds from the Renée og Bredo Grimsgaard’s Foundation, and by the Bacterial Vaccines (BactiVac) Network. BactiVac is funded by the GCRF Networks in Vaccines Research and Development which was co-funded by the MRC and BBSRC. Additional support was provided by The Department of Health and Social Care as part of the Global AMR Innovation Fund (GAMRIF), a UK aid programme that supports early-stage innovative research in underfunded areas of antimicrobial resistance (AMR) research and development for the benefit of those in low- and middle-income countries (LMICs), who bear the greatest burden of AMR. The views expressed in this publication are those of the author(s) and not necessarily those of the UK Department of Health and Social Care.
Conflict of interest
The authors declare that the research was conducted in the absence of any commercial or financial relationships that could be construed as a potential conflict of interest.
Publisher’s note
All claims expressed in this article are solely those of the authors and do not necessarily represent those of their affiliated organizations, or those of the publisher, the editors and the reviewers. Any product that may be evaluated in this article, or claim that may be made by its manufacturer, is not guaranteed or endorsed by the publisher.
References
Aas, F. E., Egge-Jacobsen, W., Winther-Larsen, H. C., Lovold, C., Hitchen, P. G., Dell, A., et al. (2006). Neisseria gonorrhoeae type IV pili undergo multisite, hierarchical modifications with phosphoethanolamine and phosphocholine requiring an enzyme structurally related to lipopolysaccharide phosphoethanolamine transferases. J. Biol. Chem. 281, 27712–27723. doi: 10.1074/jbc.M604324200
Aas, F. E., Vik, A., Vedde, J., Koomey, M., Egge-Jacobsen, W. (2007). Neisseria gonorrhoeae O-linked pilin glycosylation: functional analyses define both the biosynthetic pathway and glycan structure. Mol. Microbiol. 65, 607–624. doi: 10.1111/j.1365-2958.2007.05806.x
Anonsen, J. H., Borud, B., Vik, A., Viburiene, R., Koomey, M. (2017). Structural and genetic analyses of glycan O-acetylation in a bacterial protein glycosylation system: evidence for differential effects on glycan chain length. Glycobiology 27, 888–899. doi: 10.1093/glycob/cwx032
Anonsen, J. H., Egge-Jacobsen, W., Aas, F. E., Borud, B., Koomey, M., Vik, A. (2012a). Novel protein substrates of the phospho-form modification system in Neisseria gonorrhoeae and their connection to O-linked protein glycosylation. Infect. Immun. 80, 22–30. doi: 10.1128/IAI.05920-11
Anonsen, J. H., Vik, A., Egge-Jacobsen, W., Koomey, M. (2012b). An extended spectrum of target proteins and modification sites in the general O-linked protein glycosylation system in Neisseria gonorrhoeae. J. Proteome Res. 11, 5781–5793. doi: 10.1021/pr300584x
Baarda, B. I., Zielke, R. A., Holm, A. K., Sikora, A. E. (2021). Comprehensive bioinformatic assessments of the variability of neisseria gonorrhoeae vaccine candidates. mSphere 6, 1–18. doi: 10.1128/mSphere.00977-20
Bårnes, G. K., Brynildsrud, O. B., Børud, B., Workalemahu, B., Kristiansen, P. A., Beyene, D., et al. (2017). Whole genome sequencing reveals within-host genetic changes in paired meningococcal carriage isolates from Ethiopia. BMC Genomics 18, 407. doi: 10.1186/s12864-017-3806-3
Bennett, J. S., Jolley, K. A., Earle, S. G., Corton, C., Bentley, S. D., Parkhill, J., et al. (2012). A genomic approach to bacterial taxonomy: an examination and proposed reclassification of species within the genus Neisseria. Microbiol. (Reading) 158, 1570–1580. doi: 10.1099/mic.0.056077-0
Børud, B., Aas, F. E., Vik, A., Winther-Larsen, H. C., Egge-Jacobsen, W., Koomey, M. (2010). Genetic, structural, and antigenic analyses of glycan diversity in the O-linked protein glycosylation systems of human Neisseria species. J. Bacteriol 192, 2816–2829. doi: 10.1128/JB.00101-10
Børud, B., Anonsen, J. H., Viburiene, R., Cohen, E. H., Samuelsen, A. B. C., Koomey, M. (2014). Extended glycan diversity in a bacterial protein glycosylation system linked to allelic polymorphisms and minimal genetic alterations in a glycosyltransferase gene. Mol. Microbiol. 94, 688–699. doi: 10.1111/mmi.12789
Børud, B., Bårnes, G. K., Brynildsrud, O. B., Fritzsønn, E., Caugant, D. A. (2018). Genotypic and phenotypic characterization of the O-linked protein glycosylation system reveals high glycan diversity in paired meningococcal carriage isolates. J. Bacteriology 200, JB.00794–17. doi: 10.1128/JB.00794-17
Børud, B., Viburiene, R., Hartley, M. D., Paulsen, B. S., Egge-Jacobsen, W., Imperiali, B., et al. (2011). Genetic and molecular analyses reveal an evolutionary trajectory for glycan synthesis in a bacterial protein glycosylation system. Proc. Natl. Acad. Sci. 108, 9643–9648. doi: 10.1073/pnas.1103321108
Boslego, J. W., Tramont, E. C., Chung, R. C., Mcchesney, D. G., Ciak, J., Sadoff, J. C., et al. (1991). Efficacy trial of a parenteral gonococcal pilus vaccine in men. Vaccine 9, 154–162. doi: 10.1016/0264-410X(91)90147-X
Chamot-Rooke, J., Mikaty, G., Malosse, C., Soyer, M., Dumont, A., Gault, J., et al. (2011). Posttranslational modification of pili upon cell contact triggers N. meningitidis dissemination. Science 331, 778–782. doi: 10.1126/science.1200729
Chamot-Rooke, J., Rousseau, B., Lanternier, F., Mikaty, G., Mairey, E., Malosse, C., et al. (2007). Alternative Neisseria spp. type IV pilin glycosylation with a glyceramido acetamido trideoxyhexose residue. Proc. Natl. Acad. Sci. U.S.A. 104, 14783–14788. doi: 10.1073/pnas.0705335104
Chu, C. L., Yu, Y. L., Kung, Y. C., Liao, P. Y., Liu, K. J., Tseng, Y. T., et al. (2012). The immunomodulatory activity of meningococcal lipoprotein Ag473 depends on the conformation made up of the lipid and protein moieties. PloS One 7, e40873. doi: 10.1371/journal.pone.0040873
Edwards, J. L., Brown, E. J., Uk-Nham, S., Cannon, J. G., Blake, M. S., Apicella, M. A. (2002). A co-operative interaction between Neisseria gonorrhoeae and complement receptor 3 mediates infection of primary cervical epithelial cells. Cell Microbiol. 4, 571–584. doi: 10.1046/j.1462-5822.2002.t01-1-00215.x
Egge-Jacobsen, W., Salomonsson, E. N., Aas, F. E., Forslund, A. L., Winther-Larsen, H. C., Maier, J., et al. (2011). O-linked glycosylation of the PilA pilin protein of Francisella tularensis: identification of the endogenous protein-targeting oligosaccharyltransferase and characterization of the native oligosaccharide. J. Bacteriol 193, 5487–5497. doi: 10.1128/JB.00383-11
Elhenawy, W., Scott, N. E., Tondo, M. L., Orellano, E. G., Foster, L. J., Feldman, M. F. (2016). Protein O-linked glycosylation in the plant pathogen Ralstonia solanacearum. Glycobiology 26, 301–311. doi: 10.1093/glycob/cwv098
Feldman, M. F., Wacker, M., Hernandez, M., Hitchen, P. G., Marolda, C. L., Kowarik, M., et al. (2005). Engineering N-linked protein glycosylation with diverse O antigen lipopolysaccharide structures in Escherichia coli. Proc. Natl. Acad. Sci. U.S.A. 102, 3016–3021. doi: 10.1073/pnas.0500044102
Fussenegger, M., Rudel, T., Barten, R., Ryll, R., Meyer, T. F. (1997). Transformation competence and type-4 pilus biogenesis in Neisseria gonorrhoeae–a review. Gene 192, 125–134. doi: 10.1016/S0378-1119(97)00038-3
Gault, J., Ferber, M., Machata, S., Imhaus, A. F., Malosse, C., Charles-Orszag, A., et al. (2015). Neisseria meningitidis type IV pili composed of sequence invariable pilins are masked by multisite glycosylation. PloS Pathog. 11, e1005162. doi: 10.1371/journal.ppat.1005162
Hadjineophytou, C., Anonsen, J. H., Svingerud, T., Mortimer, T. D., Grad, Y. H., Scott, N. E., et al. (2022). Sculpting the bacterial O-glycoproteome: functional analyses of orthologous oligosaccharyltransferases with diverse targeting specificities. mBio 13, e0379721. doi: 10.1128/mbio.03797-21
Hadjineophytou, C., Anonsen, J. H., Wang, N., Ma, K. C., Viburiene, R., Vik, Å., et al. (2019). Genetic determinants of genus-level glycan diversity in a bacterial protein glycosylation system. PloS Genet. 15, e1008532. doi: 10.1371/journal.pgen.1008532
Hadjineophytou, C., Loh, E., Koomey, M., Scott, N. E. (2024). Combining FAIMS based glycoproteomics and DIA proteomics reveals widespread proteome alterations in response to glycosylation occupancy changes in Neisseria gonorrhoeae. Proteomics, e2300496. doi: 10.1002/pmic.202300496
Hagblom, P., Segal, E., Billyard, E., So, M. (1985). Intragenic recombination leads to pilus antigenic variation in Neisseria gonorrhoeae. Nature 315, 156–158. doi: 10.1038/315156a0
Haghi, F., Peerayeh, S. N., Siadat, S. D., Zeighami, H. (2012). Recombinant outer membrane secretin PilQ(406–770) as a vaccine candidate for serogroup B Neisseria meningitidis. Vaccine 30, 1710–1714. doi: 10.1016/j.vaccine.2011.12.076
Hamadeh, R. M., Estabrook, M. M., Zhou, P., Jarvis, G. A., Griffiss, J. M. (1995). Anti-Gal binds to pili of Neisseria meningitidis: the immunoglobulin A isotype blocks complement-mediated killing. Infect. Immun. 63, 4900–4906. doi: 10.1128/iai.63.12.4900-4906.1995
Harding, C. M., Nasr, M. A., Kinsella, R. L., Scott, N. E., Foster, L. J., Weber, B. S., et al. (2015). Acinetobacter strains carry two functional oligosaccharyltransferases, one devoted exclusively to type IV pilin, and the other one dedicated to O-glycosylation of multiple proteins. Mol. Microbiol. 96, 1023–1041. doi: 10.1111/mmi.12986
Hartley, M. D., Morrison, M. J., Aas, F. E., Børud, B., Koomey, M., Imperiali, B. (2011). Biochemical characterization of the O-linked glycosylation pathway in Neisseria gonorrhoeae responsible for biosynthesis of protein glycans containing N,N’-diacetylbacillosamine. Biochemistry 50, 4936–4948. doi: 10.1021/bi2003372
Hegge, F. T., Hitchen, P. G., Aas, F. E., Kristiansen, H., Lovold, C., Egge-Jacobsen, W., et al. (2004). Unique modifications with phosphocholine and phosphoethanolamine define alternate antigenic forms of Neisseria gonorrhoeae type IV pili. Proc. Natl. Acad. Sci. U.S.A. 101, 10798–10803. doi: 10.1073/pnas.0402397101
Holst, J., Martin, D., Arnold, R., Huergo, C. C., Oster, P., O’hallahan, J., et al. (2009). Properties and clinical performance of vaccines containing outer membrane vesicles from Neisseria meningitidis. Vaccine 27 Suppl 2, B3–12. doi: 10.1016/j.vaccine.2009.04.071
Hoogeboom, R., Tolar, P. (2016). Molecular mechanisms of B cell antigen gathering and endocytosis. Curr. Top. Microbiol. Immunol. 393, 45–63. doi: 10.1007/82_2015_476
Humbert, M. V., Christodoulides, M. (2018). Immunization with recombinant truncated Neisseria meningitidis-Macrophage Infectivity Potentiator (rT-Nm-MIP) protein induces murine antibodies that are cross-reactive and bactericidal for Neisseria gonorrhoeae. Vaccine 36, 3926–3936. doi: 10.1016/j.vaccine.2018.05.069
Iwashkiw, J. A., Seper, A., Weber, B. S., Scott, N. E., Vinogradov, E., Stratilo, C., et al. (2012). Identification of a general O-linked protein glycosylation system in Acinetobacter baumannii and its role in virulence and biofilm formation. PloS Pathog. 8, e1002758. doi: 10.1371/journal.ppat.1002758
Jen, F. E., Abrahams, J. L., Schulz, B. L., Lamelas, A., Pluschke, G., Jennings, M. P. (2023). High-Frequency Changes in Pilin Glycosylation Patterns during Neisseria meningitidis Serogroup a Meningitis Outbreaks in the African Meningitis Belt. ACS Infect. Dis. 9, 1451–1457. doi: 10.1021/acsinfecdis.3c00149
Jen, F. E., Warren, M. J., Schulz, B. L., Power, P. M., Swords, W. E., Weiser, J. N., et al. (2013). Dual pili post-translational modifications synergize to mediate meningococcal adherence to platelet activating factor receptor on human airway cells. PloS Pathog. 9, e1003377. doi: 10.1371/journal.ppat.1003377
Jennings, M. P., Jen, F. E., Roddam, L. F., Apicella, M. A., Edwards, J. L. (2011). Neisseria gonorrhoeae pilin glycan contributes to CR3 activation during challenge of primary cervical epithelial cells. Cell Microbiol. 13, 885–896. doi: 10.1111/cmi.2011.13.issue-6
Johannessen, C., Koomey, M., Børud, B. (2012). Hypomorphic glycosyltransferase alleles and recoding at contingency Loci influence glycan microheterogeneity in the protein glycosylation system of Neisseria species. J. Bacteriol 194, 5034–5043. doi: 10.1128/JB.00950-12
Kahler, C. M., Martin, L. E., Tzeng, Y. L., Miller, Y. K., Sharkey, K., Stephens, D. S., et al. (2001). Polymorphisms in pilin glycosylation Locus of Neisseria meningitidis expressing class II pili. Infect. Immun. 69, 3597–3604. doi: 10.1128/IAI.69.6.3597-3604.2001
Klughammer, J., Dittrich, M., Blom, J., Mitesser, V., Vogel, U., Frosch, M., et al. (2017). Comparative Genome Sequencing Reveals Within-Host Genetic Changes in Neisseria meningitidis during Invasive Disease. PloS One 12, e0169892. doi: 10.1371/journal.pone.0169892
Kong, Y., Ma, J. H., Warren, K., Tsang, R. S., Low, D. E., Jamieson, F. B., et al. (2013). Homologous recombination drives both sequence diversity and gene content variation in Neisseria meningitidis. Genome Biol. Evol. 5, 1611–1627. doi: 10.1093/gbe/evt116
Krauland, M. G., Dunning Hotopp, J. C., Riley, D. R., Daugherty, S. C., Marsh, J. W., Messonnier, N. E., et al. (2012). Whole genome sequencing to investigate the emergence of clonal complex 23 Neisseria meningitidis serogroup Y disease in the United States. PloS One 7, e35699. doi: 10.1371/journal.pone.0035699
Ku, S. C., Schulz, B. L., Power, P. M., Jennings, M. P. (2009). The pilin O-glycosylation pathway of pathogenic Neisseria is a general system that glycosylates AniA, an outer membrane nitrite reductase. Biochem. Biophys. Res. Commun. 378, 84–89. doi: 10.1016/j.bbrc.2008.11.025
Lamelas, A., Harris, S. R., Roltgen, K., Dangy, J. P., Hauser, J., Kingsley, R. A., et al. (2014). Emergence of a new epidemic Neisseria meningitidis serogroup A Clone in the African meningitis belt: high-resolution picture of genomic changes that mediate immune evasion. MBio 5, e01974–e01914. doi: 10.1128/mBio.01974-14
Leduc, I., Connolly, K. L., Begum, A., Underwood, K., Darnell, S., Shafer, W. M., et al. (2020). The serogroup B meningococcal outer membrane vesicle-based vaccine 4CMenB induces cross-species protection against Neisseria gonorrhoeae. PloS Pathog. 16, e1008602. doi: 10.1371/journal.ppat.1008602
Leuzzi, R., Serino, L., Scarselli, M., Savino, S., Fontana, M. R., Monaci, E., et al. (2005). Ng-MIP, a surface-exposed lipoprotein of Neisseria gonorrhoeae, has a peptidyl-prolyl cis/trans isomerase (PPIase) activity and is involved in persistence in macrophages. Mol. Microbiol. 58, 669–681. doi: 10.1111/j.1365-2958.2005.04859.x
Lindberg, A. A. (1999). Glycoprotein conjugate vaccines. Vaccine 17 Suppl 2, S28–S36. doi: 10.1016/S0264-410X(99)00232-7
Lithgow, K. V., Scott, N. E., Iwashkiw, J. A., Thomson, E. L., Foster, L. J., Feldman, M. F., et al. (2014). A general protein O-glycosylation system within the Burkholderia cepacia complex is involved in motility and virulence. Mol. Microbiol. 92, 116–137. doi: 10.1111/mmi.12540
Liu, Y., Hammer, L. A., Liu, W., Hobbs, M. M., Zielke, R. A., Sikora, A. E., et al. (2017). Experimental vaccine induces Th1-driven immune responses and resistance to Neisseria gonorrhoeae infection in a murine model. Mucosal Immunol. 10, 1594–1608. doi: 10.1038/mi.2017.11
Marceau, M., Forest, K., Beretti, J. L., Tainer, J., Nassif, X. (1998). Consequences of the loss of O-linked glycosylation of meningococcal type IV pilin on piliation and pilus-mediated adhesion. Mol. Microbiol. 27, 705–715. doi: 10.1046/j.1365-2958.1998.00706.x
Mcchesney, D., Tramont, E. C., Boslego, J. W., Ciak, J., Sadoff, J., Brinton, C. C. (1982). Genital antibody response to a parenteral gonococcal pilus vaccine. Infect. Immun. 36, 1006–1012. doi: 10.1128/iai.36.3.1006-1012.1982
Micoli, F., Maclennan, C. A. (2020). Outer membrane vesicle vaccines. Semin. Immunol. 50, 101433. doi: 10.1016/j.smim.2020.101433
Mustapha, M. M., Marsh, J. W., Shutt, K. A., Schlackman, J., Ezeonwuka, C., Farley, M. M., et al. (2021). Transmission dynamics and microevolution of neisseria meningitidis during carriage and invasive disease in high school students in Georgia and maryland 2006–2007. J. Infect. Dis. 223, 2038–2047. doi: 10.1093/infdis/jiaa674
Naess, L. M., Maugesten, I. S., Caugant, D. A., Kassu, A., Aseffa, A., Borud, B. (2023). Genetic, functional, and immunogenic analyses of the O-linked protein glycosylation system in neisseria meningitidis serogroup A ST-7 isolates. J. Bacteriol 205, e0045822. doi: 10.1128/jb.00458-22
Nassif, X., Marceau, M., Pujol, C., Pron, B., Beretti, J. L., Taha, M. K. (1997). Type-4 pili and meningococcal adhesiveness. Gene 192, 149–153. doi: 10.1016/S0378-1119(96)00802-5
Nothaft, H., Liu, X., Mcnally, D. J., Li, J. J., Szymanski, C. M. (2009). Study of free oligosaccharides derived from the bacterial-glycosylation pathway. Proc. Natl. Acad. Sci. United States America 106, 15019–15024. doi: 10.1073/pnas.0903078106
Nothaft, H., Perez-Munoz, M. E., Yang, T., Murugan, A. V. M., Miller, M., Kolarich, D., et al. (2021). Improving chicken responses to glycoconjugate vaccination against campylobacter jejuni. Front. Microbiol. 12, 734526. doi: 10.3389/fmicb.2021.734526
Nothaft, H., Szymanski, C. M. (2010). Protein glycosylation in bacteria: sweeter than ever. Nat. Rev. Microbiol. 8, 765–778. doi: 10.1038/nrmicro2383
Omer, H., Rose, G., Jolley, K. A., Frapy, E., Zahar, J. R., Maiden, M. C., et al. (2011). Genotypic and phenotypic modifications of Neisseria meningitidis after an accidental human passage. PloS One 6, e17145. doi: 10.1371/journal.pone.0017145
Pandey, A., Cleary, D. W., Laver, J. R., Gorringe, A., Deasy, A. M., Dale, A. P., et al. (2018). Microevolution of Neisseria lactamica during nasopharyngeal colonisation induced by controlled human infection. Nat. Commun. 9, 4753. doi: 10.1038/s41467-018-07235-5
Parge, H. E., Forest, K. T., Hickey, M. J., Christensen, D. A., Getzoff, E. D., Tainer, J. A. (1995). Structure of the fibre-forming protein pilin at 2.6 A resolution. Nature 378, 32–38. doi: 10.1038/378032a0
Power, P. M., Ku, S. C., Rutter, K., Warren, M. J., Limnios, E. A., Tapsall, J. W., et al. (2007). The phase-variable allele of the pilus glycosylation gene pglA is not strongly associated with strains of Neisseria gonorrhoeae isolated from patients with disseminated gonococcal infection. Infect. Immun. 75, 3202–3204. doi: 10.1128/IAI.01501-06
Power, P. M., Roddam, L. F., Rutter, K., Fitzpatrick, S. Z., Srikhanta, Y. N., Jennings, M. P. (2003). Genetic characterization of pilin glycosylation and phase variation in Neisseria meningitidis. Mol. Microbiol. 49, 833–847. doi: 10.1046/j.1365-2958.2003.03602.x
Power, P. M., Seib, K. L., Jennings, M. P. (2006). Pilin glycosylation in Neisseria meningitidis occurs by a similar pathway to wzy-dependent O-antigen biosynthesis in Escherichia coli. Biochem. Biophys. Res. Commun. 347, 904–908. doi: 10.1016/j.bbrc.2006.06.182
Price, N. L., Goyette-Desjardins, G., Nothaft, H., Valguarnera, E., Szymanski, C. M., Segura, M., et al. (2016). Glycoengineered outer membrane vesicles: A novel platform for bacterial vaccines. Sci. Rep. 6, 24931. doi: 10.1038/srep24931
Robertson, J. N., Vincent, P., Ward, M. E. (1977). The preparation and properties of gonococcal pili. J. Gen. Microbiol. 102, 169–177. doi: 10.1099/00221287-102-1-169
Rosenqvist, E., Musacchio, A., Aase, A., Hoiby, E. A., Namork, E., Kolberg, J., et al. (1999). Functional activities and epitope specificity of human and murine antibodies against the class 4 outer membrane protein (Rmp) of Neisseria meningitidis. Infect. Immun. 67, 1267–1276. doi: 10.1128/IAI.67.3.1267-1276.1999
Semchenko, E. A., Day, C. J., Seib, K. L. (2017). MetQ of neisseria gonorrhoeae is a surface-expressed antigen that elicits bactericidal and functional blocking antibodies. Infect. Immun. 85, 1101–1111. doi: 10.1128/IAI.00898-16
Shewell, L. K., Jen, F. E., Jennings, M. P. (2017). Refinement of immunizing antigens to produce functional blocking antibodies against the AniA nitrite reductase of Neisseria gonorrhoeae. PloS One 12, e0182555. doi: 10.1371/journal.pone.0182555
Sikora, A. E., Gomez, C., Le Van, A., Baarda, B. I., Darnell, S., Martinez, F. G., et al. (2020). A novel gonorrhea vaccine composed of MetQ lipoprotein formulated with CpG shortens experimental murine infection. Vaccine 38, 8175–8184. doi: 10.1016/j.vaccine.2020.10.077
Snyder, L., Butcher, S. A., Saunders, N. J. (2001). Comparative whole-genome analyses reveal over 100 putative phase-variable genes in the pathogenic Neisseria spp. Microbiol. (Reading) 147, 2321–2332. doi: 10.1099/00221287-147-8-2321
Stimson, E., Virji, M., Barker, S., Panico, M., Blench, I., Saunders, J., et al. (1996). Discovery of a novel protein modification: alpha-glycerophosphate is a substituent of meningococcal pilin. Biochem. J. 316, 29–33. doi: 10.1042/bj3160029
Stimson, E., Virji, M., Makepeace, K., Dell, A., Morris, H. R., Payne, G., et al. (1995). Meningococcal pilin: a glycoprotein substituted with digalactosyl 2,4-diacetamido-2,4,6-trideoxyhexose. Mol. Microbiol. 17, 1201–1214. doi: 10.1111/j.1365-2958.1995.mmi_17061201.x
Stork, M., Bos, M. P., Jongerius, I., De Kok, N., Schilders, I., Weynants, V. E., et al. (2010). An outer membrane receptor of Neisseria meningitidis involved in zinc acquisition with vaccine potential. PloS Pathog. 6, e1000969. doi: 10.1371/journal.ppat.1000969
Trotter, C. L., Greenwood, B. M. (2007). Meningococcal carriage in the African meningitis belt. Lancet Infect. Dis. 7, 797–803. doi: 10.1016/S1473-3099(07)70288-8
Unemo, M., Shafer, W. M. (2014). Antimicrobial resistance in Neisseria gonorrhoeae in the 21st century: past, evolution, and future. Clin. Microbiol. Rev. 27, 587–613. doi: 10.1128/CMR.00010-14
Varki, A. (2011). Evolutionary forces shaping the Golgi glycosylation machinery: why cell surface glycans are universal to living cells. Cold Spring Harb. Perspect. Biol. 3, 1–14. doi: 10.1101/cshperspect.a005462
Vik, A., Aas, F. E., Anonsen, J. H., Bilsborough, S., Schneider, A., Egge-Jacobsen, W., et al. (2009). Broad spectrum O-linked protein glycosylation in the human pathogen Neisseria gonorrhoeae. Proc. Natl. Acad. Sci. 106, 4447–4452. doi: 10.1073/pnas.0809504106
Vik, A., Aspholm, M., Anonsen, J. H., Borud, B., Roos, N., Koomey, M. (2012). Insights into type IV pilus biogenesis and dynamics from genetic analysis of a C-terminally tagged pilin: a role for O-linked glycosylation. Mol. Microbiol. 85, 1166–1178. doi: 10.1111/j.1365-2958.2012.08166.x
Virji, M., Saunders, J. R., Sims, G., Makepeace, K., Maskell, D., Ferguson, D. J. (1993). Pilus-facilitated adherence of Neisseria meningitidis to human epithelial and endothelial cells: modulation of adherence phenotype occurs concurrently with changes in primary amino acid sequence and the glycosylation status of pilin. Mol. Microbiol. 10, 1013–1028. doi: 10.1111/j.1365-2958.1993.tb00972.x
Viviani, V., Biolchi, A., Pizza, M. (2022). Synergistic activity of antibodies in the multicomponent 4CMenB vaccine. Expert Rev. Vaccines 21, 645–658. doi: 10.1080/14760584.2022.2050697
Viviani, V., Fantoni, A., Tomei, S., Marchi, S., Luzzi, E., Bodini, M., et al. (2023). OpcA and PorB are novel bactericidal antigens of the 4CMenB vaccine in mice and humans. NPJ Vaccines 8, 54. doi: 10.1038/s41541-023-00651-9
Wanford, J. J., Green, L. R., Aidley, J., Bayliss, C. D. (2018). Phasome analysis of pathogenic and commensal Neisseria species expands the known repertoire of phase variable genes, and highlights common adaptive strategies. PloS One 13, e0196675. doi: 10.1371/journal.pone.0196675
Wang, N., Anonsen, J. H., Hadjineophytou, C., Reinar, W. B., Borud, B., Vik, A., et al. (2021). Allelic polymorphisms in a glycosyltransferase gene shape glycan repertoire in the O-linked protein glycosylation system of Neisseria. Glycobiology 31, 477–491. doi: 10.1093/glycob/cwaa073
Weiser, J. N., Goldberg, J. B., Pan, N., Wilson, L., Virji, M. (1998). The phosphorylcholine epitope undergoes phase variation on a 43-kilodalton protein in Pseudomonas aeruginosa and on pili of Neisseria meningitidis and Neisseria gonorrhoeae. Infect. Immun. 66, 4263–4267. doi: 10.1128/IAI.66.9.4263-4267.1998
Williams, J. N., Weynants, V., Poolman, J. T., Heckels, J. E., Christodoulides, M. (2014). Immuno-proteomic analysis of human immune responses to experimental Neisseria meningitidis outer membrane vesicle vaccines identifies potential cross-reactive antigens. Vaccine 32, 1280–1286. doi: 10.1016/j.vaccine.2013.12.070
Wolfgang, M., Lauer, P., Park, H. S., Brossay, L., Hebert, J., Koomey, M. (1998). PilT mutations lead to simultaneous defects in competence for natural transformation and twitching motility in piliated Neisseria gonorrhoeae. Mol. Microbiol. 29, 321–330. doi: 10.1046/j.1365-2958.1998.00935.x
Zhang, Q. Y., Deryckere, D., Lauer, P., Koomey, M. (1992). Gene conversion in Neisseria gonorrhoeae: evidence for its role in pilus antigenic variation. Proc. Natl. Acad. Sci. U.S.A. 89, 5366–5370. doi: 10.1073/pnas.89.12.5366
Zhou, J. Y., Cobb, B. A. (2021). Glycans in immunologic health and disease. Annu. Rev. Immunol. 39, 511–536. doi: 10.1146/annurev-immunol-101819-074237
Zhu, T., Mcclure, R., Harrison, O. B., Genco, C., Massari, P. (2019). Integrated Bioinformatic Analyses and Immune Characterization of New Neisseria gonorrhoeae Vaccine Antigens Expressed during Natural Mucosal Infection. Vaccines (Basel) 7, 1–23. doi: 10.3390/vaccines7040153
Zhu, W., Thomas, C. E., Chen, C. J., Van Dam, C. N., Johnston, R. E., Davis, N. L., et al. (2005). Comparison of immune responses to gonococcal PorB delivered as outer membrane vesicles, recombinant protein, or Venezuelan equine encephalitis virus replicon particles. Infect. Immun. 73, 7558–7568. doi: 10.1128/IAI.73.11.7558-7568.2005
Keywords: immunogenicity, glycan diversity, immune escape, Neisseria gonorrhoeae, Neisseria meningitidis
Citation: Børud B and Koomey M (2024) Sweet complexity: O-linked protein glycosylation in pathogenic Neisseria. Front. Cell. Infect. Microbiol. 14:1407863. doi: 10.3389/fcimb.2024.1407863
Received: 27 March 2024; Accepted: 29 April 2024;
Published: 14 May 2024.
Edited by:
Christopher W Reid, Bryant University, United StatesReviewed by:
Gary Jarvis, San Francisco, United StatesJoseph P Dillard, University of Wisconsin-Madison, United States
Copyright © 2024 Børud and Koomey. This is an open-access article distributed under the terms of the Creative Commons Attribution License (CC BY). The use, distribution or reproduction in other forums is permitted, provided the original author(s) and the copyright owner(s) are credited and that the original publication in this journal is cited, in accordance with accepted academic practice. No use, distribution or reproduction is permitted which does not comply with these terms.
*Correspondence: Bente Børud, YmVudGUuYm9ydWRAZmhpLm5v