- 1Department of Food Science and Technology, Biotechnical Faculty, University of Ljubljana, Ljubljana, Slovenia
- 2Department of Pediatrics, Faculty of Medicine, University of Maribor, Maribor, Slovenia
- 3Department of Biotechnology, Jožef Stefan Institute, Ljubljana, Slovenia
- 4Department of Biochemistry and Molecular and Structural Biology, Jožef Stefan Institute, Ljubljana, Slovenia
- 5Centre of Excellence for Integrated Approaches in Chemistry and Biology of Proteins, Ljubljana, Slovenia
- 6Department of Microbiology, Biochemistry, Molecular Biology and Biotechnology, Faculty of Agriculture and Life Sciences, University of Maribor, Maribor, Slovenia
Campylobacter jejuni, a Gram-negative bacterium, is one of the most common causes of foodborne illness worldwide. Its adhesion mechanism is mediated by several bacterial factors, including flagellum, protein adhesins, lipooligosaccharides, proteases, and host factors, such as surface glycans on epithelial cells and mucins. Fungal lectins, specialized carbohydrate-binding proteins, can bind to specific glycans on host and bacterial cells and thus influence pathogenesis. In this study, we investigated the effects of fungal lectins and protease inhibitors on the adhesion of C. jejuni to model biotic surfaces (mucin, fibronectin, and collagen) and Caco-2 cells as well as the invasion of Caco-2 cells. The lectins Marasmius oreades agglutinin (MOA) and Laccaria bicolor tectonin 2 (Tec2) showed remarkable efficacy in all experiments. In addition, different pre-incubations of lectins with C. jejuni or Caco-2 cells significantly inhibited the ability of C. jejuni to adhere to and invade Caco-2 cells, but to varying degrees. Pre-incubation of Caco-2 cells with selected lectins reduced the number of invasive C. jejuni cells the most, while simultaneous incubation showed the greatest reduction in adherent C. jejuni cells. These results suggest that fungal lectins are a promising tool for the prevention and treatment of C. jejuni infections. Furthermore, this study highlights the potential of fungi as a rich reservoir for novel anti-adhesive agents.
1 Introduction
Campylobacter jejuni is the most common bacterial cause of human gastroenteritis (called campylobacteriosis) worldwide. In the EU, campylobacteriosis is the most commonly reported foodborne disease, with an estimated annual cost of €2.4 billion (ECDC, 2023). Livestock and pets represent reservoirs of C. jejuni, which is well adapted and exposed to many antibiotics. Such exposure contributes to its increasing resistance to antibiotics, including those used to treat human diseases (ECDC, 2023), and thus compromises the effectiveness of antibiotic treatments. Thus, research is under way to find new, alternative, non-antibiotic interventions (Dai et al., 2020).
Protein and glycan interactions between hosts and bacteria play an important role in bacterial adhesion and invasion of the gastrointestinal tract. C. jejuni is ingested via contaminated food and enters intestinal crypts lined with mucus (Figure 1) containing the glycoprotein mucin. C. jejuni is attracted to mucin and metabolizes its components, thereby increasing its own motility and reproduction, which contributes to its successful colonization of intestinal mucosa (Alemka et al., 2012). Upon contact with enterocytes, C. jejuni secretes the trypsin-like high temperature requirement A serine protease (HtrA), which cleaves a temporary opening in cell junctions (Boehm et al., 2012). This enables C. jejuni to undergo paracellular transport to the basolateral side, which is rich in fibronectin and collagen. Fibronectin is part of the extracellular matrix and a known binding target for the adhesins CadF (Campylobacter adhesion to fibronectin) and FlpA (fibronectin-like protein A) of C. jejuni (Kuusela et al., 1989). This additional binding to different molecules in the extracellular matrix enables the internalization of C. jejuni into host cells (Kovács et al., 2020).
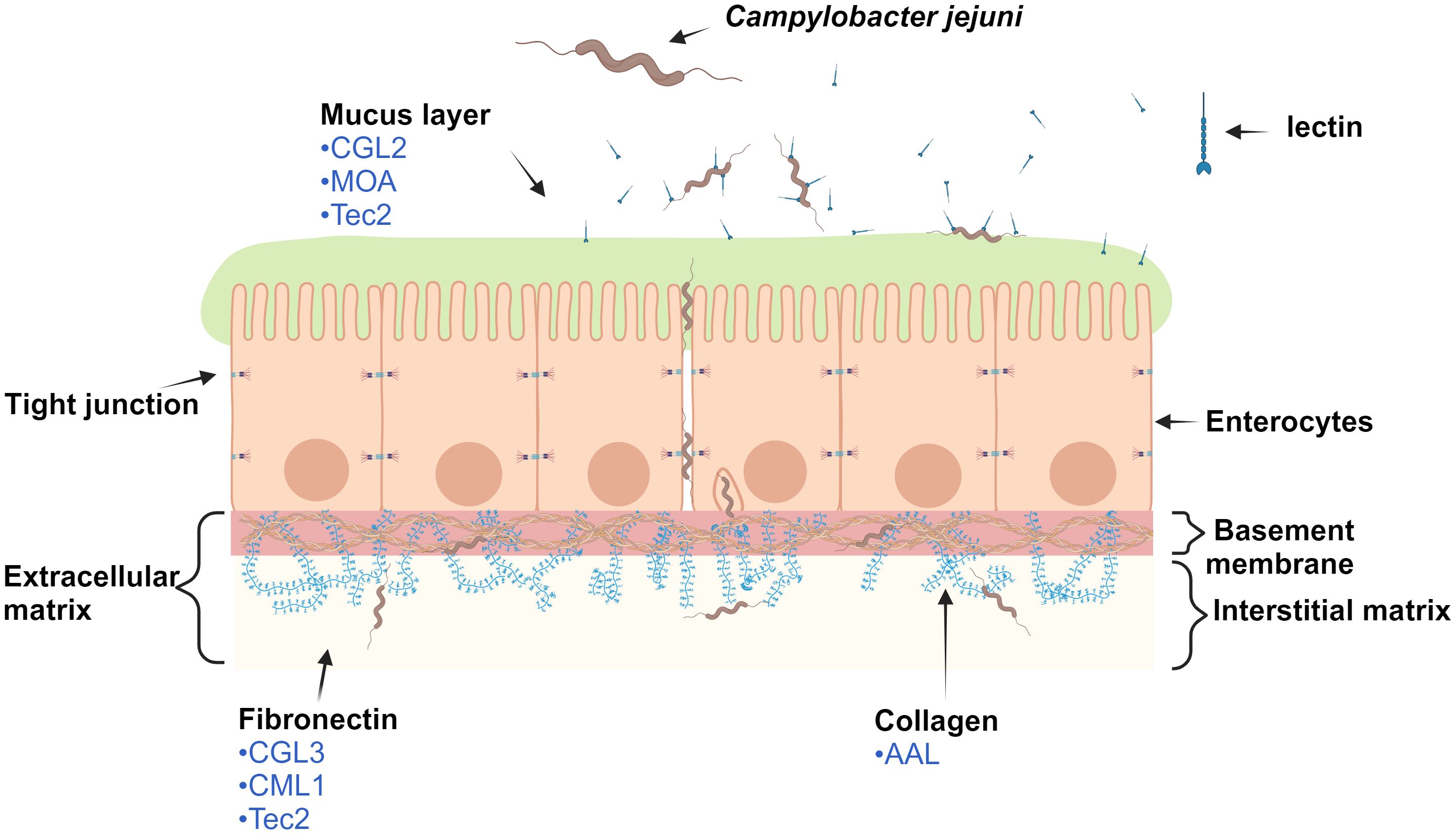
Figure 1 Schematic representation of the monolayer of epithelial cells (enterocytes) and selected macromolecules of the extracellular matrix of the human digestive tract. For clarity, only macromolecules of the extracellular matrix that are involved in paracellular transport and internalization of Campylobacter jejuni into the host cell are shown. The lectins (in blue) displayed have been identified by our experiments as the most effective in reducing C. jejuni adhesion. Note: elements are not to scale. The image was created using BioRender.com. Abbreviations are defined in Table 1.
Glycans present on the surface of C. jejuni greatly influence its interactions with surfaces (Sabotič et al., 2023). Various polysaccharides (e.g. capsular polysaccharides and lipopolysaccharides), N-linked glycoproteins, and flagellar O-linked glycoproteins of the C. jejuni define the collection of common glycans present on the surface of C. jejuni: galactose (Gal), N-acetylgalactosamine (GalNAc), and, to a lesser extent, N-acetylglucosamine (GlcNAc), mannose (Man), N-acetylneuraminic acid (Neu5Ac), and fucose (Fuc) (Corcoran and Moran, 2007; Turonova et al., 2016). Another structure present on the C. jejuni surface is the O-methyl phosphoramidate modification, which is important for cellular interactions and infection (Van Alphen et al., 2014). Interestingly, the presence of surface glycans seems to be strain-specific and affected by environmental factors, such as temperature (Day et al., 2009, 2013; Kilcoyne et al., 2014).
As previously shown by Klančnik et al (Klančnik et al., 2017a), fungal fruiting bodies represent a rich source of interesting antimicrobial and anti-adhesive substances. Of these, lectins and protease inhibitors (PIs) function as innate protein defense systems against amoebozoans, insects, and nematodes. After ingestion, lectins bind to specific glycans in the digestive tract and can cause cell disruption, whereas PIs inhibit the digestive proteases of fungivores (Sabotič et al., 2015). We hypothesize that fungal lectins may bind to specific glycans of C. jejuni, glycans on biotic surfaces, and intestinal epithelial cells, while PIs affect the enzymes secreted by C. jejuni, consequently affecting the attachment of C. jejuni to different surfaces. Therefore, the aim of this study was to evaluate fungal lectin- and PI-mediated modulation of C. jejuni adhesion to model surfaces and Caco-2 cells and C. jejuni invasion of Caco-2 cells.
2 Methods
2.1 Bacterial strain and growth conditions
Campylobacter jejuni NCTC 11168 (National Collection of Type Cultures) was used in this study. The strain was stored at −80°C in 20% glycerol (Kemika, Croatia) and 80% Mueller Hinton broth (Oxoid, UK). The strain was cultivated on Karmali agar (Oxoid, UK) with a selective supplement (SR0167E, Oxoid, UK) for 24 h at 42°C in a microaerobic atmosphere (85% N2, 10% CO2, 5% O2). Cultures were transferred to Mueller Hinton agar (Oxoid, UK) and incubated again for 24 h at 42°C in a microaerobic atmosphere. Bacterial concentration was determined spectrophotometrically (Perkin Elmer, USA) to 0.1 optical density (OD) at 600 nm. The suspension was further diluted by transferring 0.1 mL of the suspension to 9.9 mL of 2× Mueller Hinton broth to obtain an inoculum of approximately 1 × 105 cells/mL. For the Caco-2 assay, a concentration of 1 × 108 cells/mL (OD600 = 0.2) was prepared in Dulbecco’s modified Eagle’s medium (DMEM).
2.2 Fungal lectins and PIs
Recombinant fungal lectins and PIs were prepared in the Escherichia coli expression system using different expression vectors. The following recombinant proteins were prepared as previously described: Clitocybe nebularis lectin [CNL, UniProt ID: B2ZRS9 (Pohleven et al., 2012)]; Macrolepiota procera lectin [MpL, Uniprot ID: F6KMV5 (Žurga et al., 2014)]; the macrocypins (Sabotič et al., 2009) macrocypin 1 (Mcp1, Uniprot ID: B9V973), macrocypin 3 (Mcp3, Uniprot ID: B9V979), and macrocypin 4 (Mcp4, Uniprot ID: B9V982); cocaprin 1 [CCP1, Uniprot ID: A8PCJ3 (Renko et al., 2022)]; and cospin [PIC, Uniprot ID: D0EWJ0 (Sabotič et al., 2012)].
The following lectins were produced as His-tagged recombinant proteins in E. coli BL21(DE3) using ZYM-5052 autoinduction medium for 4 h at 37°C and 20 h at 18°C (Studier, 2005): Coprinopsis galectin 2 (CGL2, UniProt ID: Q9P4R8 [Walser et al., 2004)], Coprinopsis galectin 3 (CGL3, UniProt ID: Q206Z5 [Wälti, 2008)], Marasmius oreades agglutinin [MOA, UniProt ID: Q8X123 (Kruger et al., 2002; Grahn et al., 2007)], Agaricus bisporus lectin [ABL, UniProt ID: Q00022 (Carrizo et al., 2005)], the Sordaria macrospora transcript associated with perithecial development [TAP1, UniProt ID: F7VWP8 (Nowrousian and Cebula, 2005)], Aleuria aurantia lectin [AAL, UniProt ID: P18891 (Wimmerova et al., 2003; Olausson et al., 2011)], Coprinopsis cinerea lectin 2 [CCL2, UniProt ID: B3GA02 (Schubert et al., 2012)], C. cinerea mucin-binding lectin 1 [CML1, UniProt ID: B3VS76 (Bleuler-Martinez et al., 2022)], and Laccaria bicolor tectonin 2 [Tec2, UniProt ID: B0CZL6 (Wohlschlager et al., 2014)].
Bacteria were harvested with centrifugation and sonicated in buffer A (30 mM Tris, pH 7.5, 400 mM NaCl) with 1 mg/mL lysozyme and cOmplete™ EDTA-free Protease Inhibitor Cocktail (Roche, Switzerland). Lectins were purified (Supplementary Figure 1) using a two-step purification protocol with His-tag affinity chromatography [HisTrap FF 5 mL column (Cytiva, USA) in buffer A with 10 mM imidazole for binding and 300 mM imidazole for elution] and size-exclusion chromatography [HiPrep 26/60 Sephacryl S-200 HR or HiPrep 26/60 Sephacryl S-100 HR column (Cytiva, USA) in buffer A]. They were then stored in phosphate-buffered saline (PBS; Oxoid, UK) in aliquots at −20°C until use. The plant lectin concanavalin A (ConA; L-1000, Vector Labs) was used as a control.
2.3 Preparation of model biotic surfaces on microtiter plates
Biotic surfaces were prepared with collagen type I solution from rat tail, fibronectin from human plasma, and mucin type II from porcine stomach (all from Sigma Aldrich, Germany). Stock solutions of these macromolecules were prepared according to the manufacturer’s instructions and were diluted with sterile PBS to obtain working solutions. For mucin type II, we chose a concentration of 1 mg/mL (Shi et al., 2000; Celebioglu et al., 2017). For collagen and fibronectin, we optimized the concentrations based on the largest number of adherent C. jejuni cells and chose 50 µg/mL and 10 µg/mL, respectively. To prepare the biotic surfaces, 200 µL of each solution at the working concentration was added to each well and incubated for 24 h at 4°C. Excess solution was carefully removed, and the wells were washed with sterile PBS immediately before experiments.
2.4 Testing the effects of lectins and PIs on C. jejuni adhesion to abiotic and biotic model surfaces
The effects of lectins on C. jejuni adhesion were tested on one abiotic and three biotic model surfaces. Nunc 96-well polystyrene microtiter plates (Thermo Fisher, USA) were used to test C. jejuni adhesion to abiotic surfaces and to prepare biotic model surfaces.
Before testing lectin-mediated modulation of C. jejuni adhesion, we tested whether lectins (at different concentrations) affect C. jejuni growth during 24 h incubation at 42°C (Supplementary Figure 2) by measuring OD600 at inoculation and at the end of cultivation (Varioskan LUX, Thermo Fisher, USA). The effects of 17 lectins (Table 1) on the adhesion of C. jejuni to abiotic and biotic model surfaces were tested at 250 µg/mL, except for MOA, which was used at 125 µg/mL due to its inhibitory effect on growth at 250 µg/mL. The stock solutions of lectins were diluted to 500 µg/mL with sterile PBS buffer and mixed with the C. jejuni inoculum in each well of the microtiter plate at a ratio of 1:1 to a final volume of 200 µL. The microtiter plate was shaken (600 rpm, 2 min) (Thermo Fisher, USA) and incubated for 24 h at 42°C in a microaerobic atmosphere. Then each well was washed three times, and adherent cells were removed by sonication followed by Koch’s dilution and drop plating, as described in (Klančnik et al., 2017b). After incubation for 24 h at 42°C in a microaerobic atmosphere, colony-forming units (CFU) on plates were counted. Experiments were conducted in three independent biological and technical replicates.
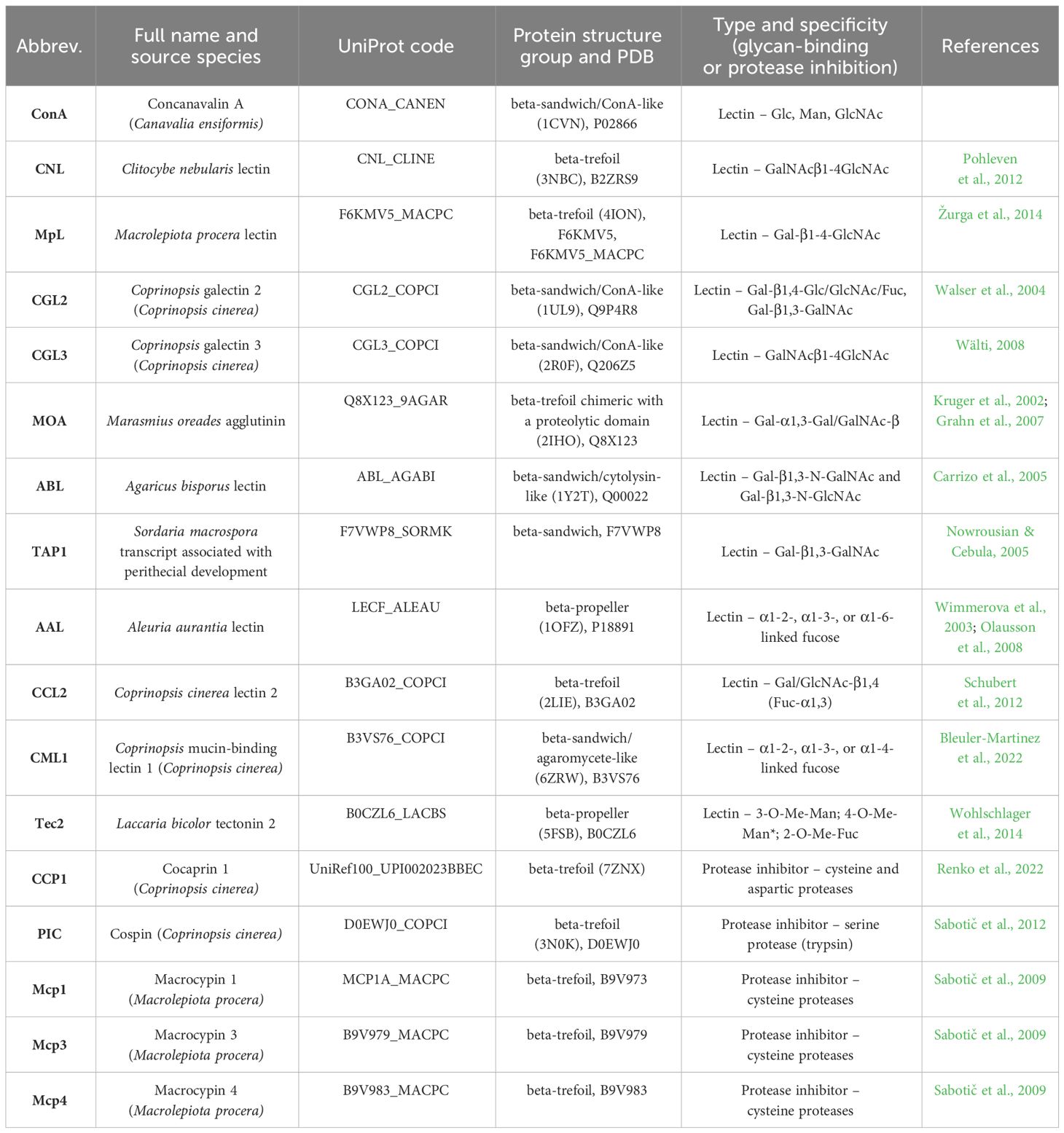
Table 1 Fungal proteins (lectins and protease inhibitors) used in this study with additional information regarding their origin, UniProt code, structure, specificity, and study reference.
2.5 Caco-2 cell cultivation
Caco-2 cells derived from human colon adenocarcinomas are a reliable model for the study of adhesion and invasion, thanks to their differentiation into cells that mimic intestinal epithelial cells with tight junctions, microvilli and specific enzymes and transporters (Arndt et al., 2011; Wong et al., 2018). These cells are known to facilitate studies on gut function and microbial interactions and represent a stable, reproducible and manageable alternative to primary or non-cancerous cell lines, where viability and complexity of culture often pose problems. Therefore, Caco-2 cells are invaluable for studying the pathogenesis of C. jejuni and shed light on the function of the intestinal barrier and the mechanisms of bacterial adhesion and invasion.
Caco-2 cell line (DSMZ no. ACC 169) was acquired from Leibniz Institute – DSMZ (Braunschweig, Germany) and was cultured in DMEM (Sigma-Aldrich, USA), supplemented with 5% (v/v) foetal bovine serum (Gibco, USA), 2 mM L-glutamine, 100 units/mL penicillin, and 100 µg/mL streptomycin (all from Merck, Germany) in tissue culture flasks at 37°C in a humidified 5% CO2 atmosphere. Caco-2 cells were seeded into 96-well tissue culture plates (Corning, USA) at 100 µL/well for the cytotoxicity assay (at 1 × 105 cells/mL) and adhesion and invasion assay (at 7 × 105 cells/mL) and grown as described above until confluence.
2.6 Cytotoxicity assay on Caco-2 cells
The cytotoxic effects of selected lectins (ConA, CGL2, CGL3, MOA, AAL, Tec2, and CCL2) were determined using the 3-(4,5-dimethylthiazol-2-yl)-2,5-diphenyltetrazolium bromide (MTT) colorimetric assay (Mosmann, 1983). After 48 h of incubation, Caco-2 cells were treated with lectins diluted in DMEM supplemented with 5% foetal bovine serum. For each lectin concentration (250–0.24 μg/mL), 100 µL/well was added to the plates in six replicates, with three repetitions. Afterwards, MTT solution (5 mg/mL) was prepared in PBS and added to each well. Microtiter plates were shaken for 5 min and then incubated for another 4 h at 37°C under 5% CO2. Then the MTT solution was carefully removed, and plates were left to dry overnight. After the addition of acid-isopropanol (0.04% HCl), the absorbance of the samples was measured using a microplate reader at 570 and 630 nm. Wells containing cells without lectins served as negative controls. In this way, we obtained non-toxic concentrations of lectins for Caco-2 cells, which were used for further studies: 250 µg/mL ConA, 60 µg/mL CGL2, 60 µg/mL CGL3, 0.24 µg/mL MOA, 250 µg/mL AAL, 10 µg/mL Tec2, and 250 µg/mL CCL2 (Supplementary Table 1).
2.7 Testing the effects of lectins on C. jejuni adhesion to and invasion of Caco-2 cells
To evaluate the preventive effectiveness of selected fungal lectins in reducing C. jejuni adhesion to and invasion of Caco-2 cells, we implemented three distinct incubation treatments. These treatments allowed sufficient time for the lectin to adhere to either C. jejuni cells (Treatment I) or Caco-2 cells (Treatment II), or simultaneous inoculation with C. jejuni cells into the Caco-2 cell culture (Treatment III).
2.7.1 Treatment I: pre-incubation of lectins with C. jejuni before application to Caco-2 cells
Lectins were prepared in 1 mL of DMEM to each non-toxic concentration (defined above) to obtain working solutions. Bacterial inoculums in DMEM (1 mL) containing no antibiotics and with approximately 108 CFU/mL of C. jejuni were suspended in the lectin solutions and incubated for 1 h at 42°C in a microaerobic atmosphere. This mixture was then added to confluent monolayers of Caco-2 cells and incubated for 2 h at 37°C in a humified atmosphere containing 5% CO2.
2.7.2 Treatment II: pre-incubation of lectins with Caco-2 cells before adding C. jejuni
Working solutions of lectins were added to Caco-2 cell monolayers and incubated for 1 h at 37°C under 5% CO2. Then, bacterial inoculums were added to cell monolayers and incubated for 2 h at 37°C in a humified atmosphere containing 5% CO2 to allow bacterial adhesion.
2.7.3 Treatment III: simultaneous inoculation of lectins and C. jejuni
Working solutions of lectins and bacterial inoculums were inoculated simultaneously to cell monolayers, and the plates were incubated for 2 h at 37°C in a humified atmosphere containing 5% CO2 to allow adhesion and invasion.
Controls without lectins, prepared by adding only bacterial inoculum, were kept under the same conditions. The effects of lectins on the adhesive and invasive ability of C. jejuni were observed in biological and technical triplicates. After washing twice with 200 μL of DMEM without antibiotics, DMEM containing 100 μg/mL gentamicin (Sigma Aldrich, Germany) was added to determine the number of invaded C. jejuni. After 1 h, the monolayers were lysed with 200 μL of 1 mL/L (v/v) Triton-X100 (Sigma Aldrich, Germany). The number of intracellular bacteria were determined for all three treatments with cultivability assays 24 h post-infection. A similar procedure but without gentamicin treatment (Šikić Pogačar et al., 2009) was performed to determine the total number of adherent and internalized bacteria. The difference between the numbers of total and intracellular bacteria was calculated to obtain the number of adherent C. jejuni cells.
2.8 Statistical analysis
The results were expressed as mean ± standard deviation of LOG10 (CFU/mL) (Supplementary Tables 2, 4). To calculate all relative differences, the mean logarithmic value of a treated sample was subtracted from the mean logarithmic value of an untreated sample. The absolute antilog value of the difference was expressed as a percentage with mean standard deviation (±%). Statistical analysis was performed with IBM SPSS Statistics 23 (Statsoft Inc., USA). A Shapiro-Wilk test of normality was performed to determine the distribution of the data. One-way ANOVA with Brown-Forsythe test was used, and a p-value of < 0.05 was considered statistically significant. Correlation analysis using Pearson’s test and graphical representation of all statistical analyses were performed using Graphpad prism 10.1 (GraphPad Software, USA).
3 Results
First, we tested whether the lectins have any effect on C. jejuni growth (Supplementary Figure 1). The lectins and PIs (at 250 µg/mL) did not affect growth, except MOA, which showed an inhibitory effect at 250 µg/mL and was thus used at 125 µg/mL (Supplementary Figure 2). Therefore, we assumed that lectins at the selected concentrations do not reduce C. jejuni growth but affect other bacterial characteristics, of which we tested adhesion to different surfaces and Caco-2 cell invasion.
3.1 Lectin and PI-mediated modulation of C. jejuni adhesion to abiotic and biotic model surfaces
The effects of 12 lectins (ConA as a control lectin and 11 lectins with known glycan-binding specificity) and 5 PIs on C. jejuni adhesion to abiotic and biotic (mucin, fibronectin, and collagen) model surfaces were tested (Figure 2, Supplementary Tables 2, 3). The effects were observed as significant decreases or increases in the numbers of adhered C. jejuni cells on the selected surfaces. CCL2 was the only lectin that did not significantly affect C. jejuni adhesion to abiotic or biotic model surfaces. Of the 17 lectins and PIs, 12 significantly decreased C. jejuni adhesion to mucin type II, 7 significantly decreased C. jejuni adhesion to polystyrene and fibronectin, and 6 significantly decreased C. jejuni adhesion to collagen type II. MOA was the only lectin that effectively decreased C. jejuni adhesion to all selected surfaces. CML1 and Tec2 significantly decreased C. jejuni adhesion to all biotic model surfaces but not polystyrene. CCP1 significantly decreased C. jejuni adhesion only to polystyrene.
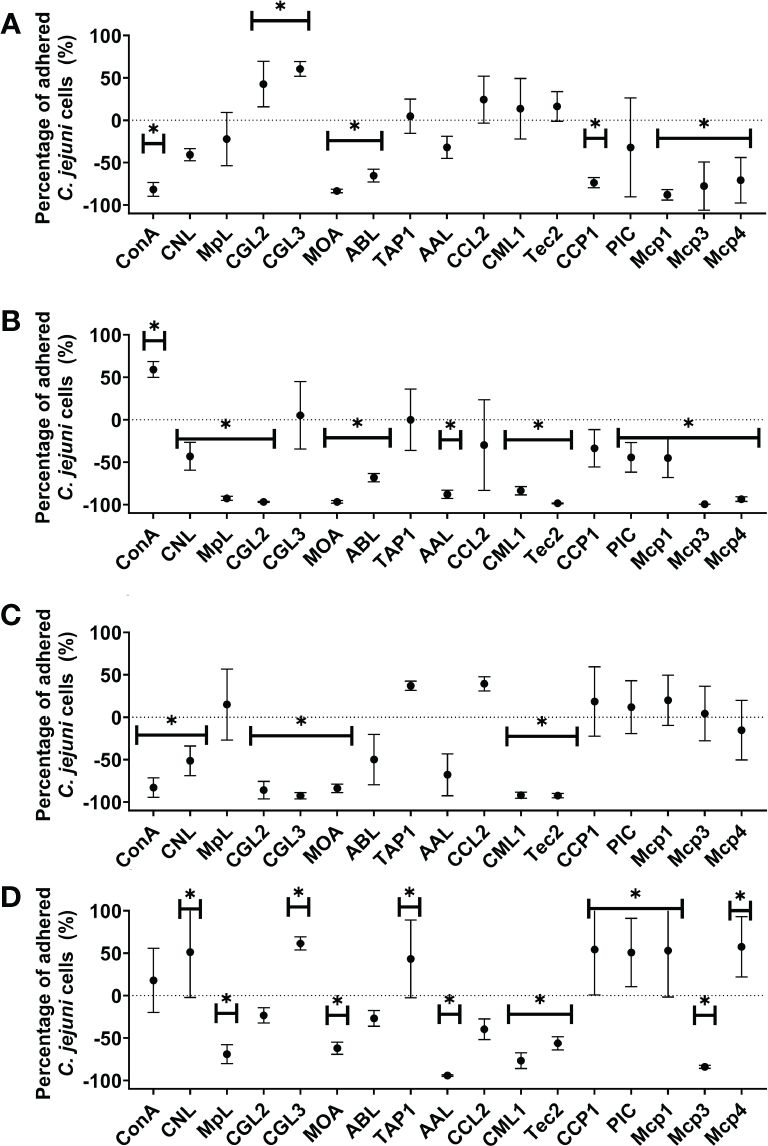
Figure 2 The effects of fungal lectins and protease inhibitors (PIs) on Campylobacter jejuni adhesion (A–D). Relative differences between C jejuni adhered cells to (A) abiotic (polystyrene) and (B–D) biotic surfaces [mucin type II (B), fibronectin (C), and collagen type I (D)] after 24 h of co-incubation with or without lectins or PIs (% ± SD). Statistically significant changes are marked with *(p-value< 0.05). Experiments were conducted in three independent biological and technical replicates. Abbreviations are defined in Table 1.
Conversely, certain lectins and PIs also increased C. jejuni adhesion to collagen type II (7), polystyrene, (2), and mucin type (1). None of the lectins increased C. jejuni adhesion to fibronectin. Interestingly, TAP1 significantly increased C. jejuni adhesion to collagen but did not affect adhesion to any other surface (Supplementary Table 3).
Correlation analysis between biotic surfaces using Pearson’s method was also performed (Supplementary Figure 3). The results show a strong correlation between the effects of selected lectins and PIs on the modulation of C. jejuni adhesion to collagen type I and mucin type II (Pearson correlation coefficient value of 0.59 and P-value of 0.01).
3.2 C. jejuni adhesion to and invasion of Caco-2 cells
To further evaluate the anti-adhesive potential of fungal lectins, an adhesion and invasion assay with C. jejuni and human intestinal epithelial Caco-2 cells was employed (Figure 3, Supplementary Tables 4, 5). For this purpose, the lectins that decreased C. jejuni adhesion to different model surfaces by more than 90% (Supplementary Table 3) i.e. CGL2, CGL3, MOA, AAL, and Tec2, as well as control lectin ConA were selected. For the negative control, CCL2 was chosen as it did not affect C. jejuni adhesion to the model surfaces (Supplementary Table 3). Non-toxic lectin concentrations, which were determined by evaluating the effects of lectins on cell proliferation, were used (Supplementary Table 1). In addition, different pre-incubation combinations of lectins, C. jejuni, and Caco-2 cells were used (Figure 4). The purpose of these pre-incubations was to determine whether the binding of lectins to either bacterial or Caco-2 cells plays a more important role in the observed decrease in the number of adherent or invasive cells during the 2 h co-incubation (Figure 3).
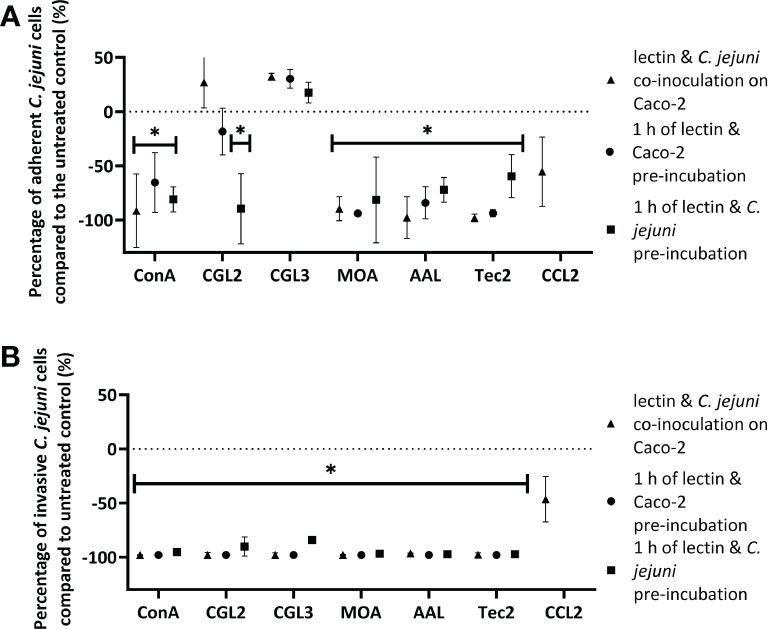
Figure 3 The effects off fungal lectins on Campylobacter jejuni adhesion to and invasion of Caco-2 cells. Values are given as relative changes in the numbers of adherent (A) or invasive (B) C jejuni cells relative to the untreated control (% ± SD). Statistically significant changes are marked with *(p-value< 0.05). Three conditions were tested: 2 h of co-incubation of lectins, C jejuni, and Caco-2 cells (triangles), 1 h of pre-incubation of lectins with Caco-2 cells (circles), and 1 h of pre-incubation of lectins with C jejuni (squares) before the final 2 h co-incubation of C jejuni with Caco-2 cells. Due to the lack of effect in adherent or invaded C jejuni cells, the lectin CCL2 was only tested using the method of simultaneous co-inoculation of lectin and C jejuni on Caco-2 cells. Experiments were conducted in three independent biological and technical replicates. Abbreviations are defined in Table 1.
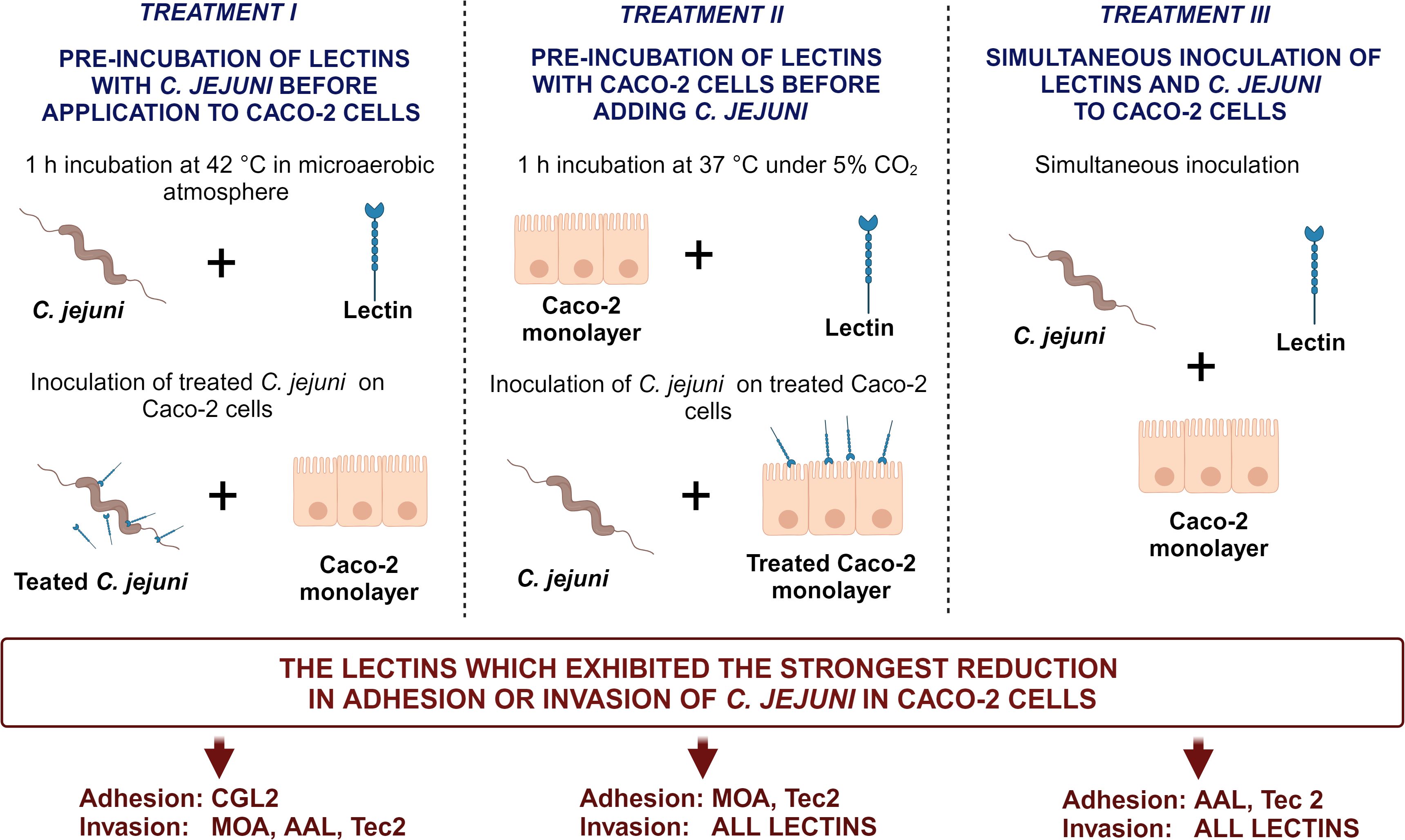
Figure 4 Schematic overview of three distinct (pre-)incubation procedures in the Caco-2 adhesion and invasion assay. The lectins yielding the most pronounced reduction for each treatment are listed at the bottom of the figure. The image was created using BioRender.com. Abbreviations are defined in Table 1.
Lectin-mediated modulation of C. jejuni adhesion to biotic surfaces and Caco-2 cells differed between different lectins (Supplementary Tables 3, 5). Furthermore, the type of pre-incubation also affected adhesion to Caco-2 cells (Figures 3, 4). ConA, MOA, AAL, and Tec2 decreased adhesion when incubated simultaneously with C. jejuni and when pre-incubated with Caco-2 cells for 1 h. Conversely, CGL2 and CGL3 did not affect C. jejuni adhesion when co-inoculated with C. jejuni, whereas pre-incubation of CGL2 with C. jejuni decreased adhesion. Pre-incubation of CGL2 with both C. jejuni and Caco-2 cells decreased bacterial adhesion to Caco-2 cells and even more notably decreased C. jejuni invasion of Caco-2 cells (Figure 3, Supplementary Table 5). Simultaneous incubation decreased invasion below the detection limit of our method (< 2 log CFU/mL) for ConA, CGL2, CGL3, and MOA and by almost a factor of 2 log values for AAL and Tec2. After lectins and Caco-2 cells were pre-incubated for 1 h, no invasion was detected for any of the lectins (< 2 log CFU/mL). Pre-incubation of lectins and C. jejuni for 1 h was least effective compared to other pre-incubation combinations, as invasion was observed for all treated Caco-2 cells (Figure 3, Supplementary Tables 4, 5). Nevertheless, the number of invaded cells was still decreased by a factor of 1 log.
4 Discussion
Understanding surface adhesion, which is an early stage of biofilm formation, is crucial for developing reliable and efficient prevention strategies to control foodborne pathogens. Therefore, we tested whether fungal (glycan-binding) lectins and PIs can reduce the presence of C. jejuni cells on different surfaces. Our results show that different lectins can increase or decrease adhesion with varying efficacy depending on the surface. We can thus assume that C. jejuni adapts and utilizes different adhesion processes depending on the surface it comes into contact with.
In this study, C. jejuni adhesion to an abiotic surface (polystyrene) was decreased by the lectins MOA, ConA, and ABL and the PIs CCP1, Mcp1, Mcp3, and Mcp4. Polystyrene is a commonly used material in the food industry and is particularly well colonized by microorganisms. Hydrophobic interactions are the main driving force for the initial attachment of C. jejuni cells to such surfaces (Katsikogianni et al., 2004). As suspected, lectins and PIs had limited effects (less than 90% decrease in adhesion) on interactions between C. jejuni and the abiotic surface, as polystyrene has no specific glycan targets.
Many of the lectins that were most potent in decreasing adhesion to mucin, e.g. MOA, AAL, ABL, CML1, and Tec2, have a glycan-binding target that is present on mucins and the surface of C. jejuni (Kruger et al., 2002; Wimmerova et al., 2003; Carrizo et al., 2005; Corcoran and Moran, 2007; Grahn et al., 2007; Olausson et al., 2008; Wohlschlager et al., 2014; Turonova et al., 2016; Bleuler-Martinez et al., 2022). The main glycans of mucin type II are GalNAc, GlcNAc, Fuc, Gal, and Neu5Ac (Schömig et al., 2016). Conversely, the core oligosaccharides of C. jejuni lipopolysaccharides consist of 3-deoxy-α-D-manno-oct-2-ulopyranosonic acid, L-glycero-D-manno-heptose, glucose (Glc), Gal, Neu5Ac, and GalNAc (Karlyshev et al., 2005). As the first line of gastrointestinal tract defense, mucins provide a convenient target for gastrointestinal pathogens to adhere to and subsequently invade the cells of the intestinal epithelium (McGuckin et al., 2011). As an essential chemoattractant for C. jejuni, mucins are also critically involved in C. jejuni colonization of the underlying epithelium, which requires increased flagellar gene expression and motility (Reid et al., 2008) Our results show that many lectins decrease adhesion, suggesting that they may prevent C. jejuni invasion of host cells by preventing the initial attachment and transition of C. jejuni into its pathogenic phenotype (Hong et al., 2014).
Campylobacter jejuni had the strongest affinity for fibronectin compared with the other surfaces tested. Three lectins (CGL3, CML1, and Tec2) decreased adhesion by more than 90%. Fibronectin is known to be a target for numerous bacterial proteins (from both Gram-positive and Gram-negative bacteria), which generally function as bacterial adhesins, i.e. fibronectin-binding proteins (Henderson et al., 2011). These proteins are critical for the first step of host cell invasion (Talukdar et al., 2020; Konkel et al., 2020). Fibronectin has fewer glycan-binding sites than the other biotic surfaces used in this study and is mainly composed of Man-, Neu5Ac-, Fuc-, GlcNAc-, and Gal-binding sites (Hsiao et al., 2017). However, the C. jejuni adhesins CadF and FlpA target the protein part of fibronectin (Konkel et al., 2020). This may explain the lower number of lectins that can effectively decrease adhesion to fibronectin compared to other biotic surfaces (Supplementary Table 4), highlighting the importance of finding an effective approach for inhibiting C. jejuni adhesion to fibronectin.
Surprisingly, many lectins and PIs (e.g. CGL3 and Mcp1) increased the number of C. jejuni cells that adhered to collagen but decreased the number of C. jejuni cells that adhered to other biotic surfaces. Collagen is the target of surface-anchored adhesins and other virulence factors (produced by both Gram-positive and Gram-negative bacteria) that mediate adhesion to host cells and tissues and enable colonization, invasion, and biofilm formation (Singh et al., 2012; Arora et al., 2021). Glycosylation of collagen consists of Gal and Glc-Gal units forming the disaccharide Glc(α1-2)-Gal(β1-O) (Hennet, 2019). This bottleneck in glycan diversity may explain why certain lectins did not affect adhesion. Interestingly, the lectins that most increased C. jejuni adhesion (CGL3, TAP1, and CNL) have similar targets (GalNAcβ1-4GlcNAc and Gal-β1,3-GalNAc). Conversely, the lectin MpL has the specific target Gal-β1-4-GlcNAc and decreased adhesion to collagen, highlighting the complex mechanism underlying C. jejuni adhesion.
Although we do not know whether the lectins used in this study bind to glycans on C. jejuni or the tested biotic surfaces, their effects on adhesion were surface-dependent. MOA was the most effective and only lectin to decrease adhesion to all surfaces tested (abiotic and biotic). The specific glycan target of MOA is Gal-α1,3-Gal/GalNAc, and Gal subunits are found on all the biotic surfaces used in this study and the surface of C. jejuni. Thus, the possibility of a synergistic effect should be considered. Day et al (Day et al., 2009). showed that C. jejuni 11168 can bind to Gal, Fuc, Neu5Ac, Man, glucosamine, and glycosaminoglycans. Therefore, we can argue that MOA might outcompete C. jejuni for binding sites on surfaces. Furthermore, CML1 and Tec2 decreased C. jejuni adhesion to all biotic surfaces. CML1 decreased adhesion to fibronectin by more than 90%, whereas Tec2 decreased adhesion to fibronectin and mucin type II by more than 90%.
In addition, we performed a correlation analysis using Pearson’s method (Supplementary Figure 3), which shows a strong correlation between the effects of lectins on C. jejuni adhesion to collagen type I and mucin type II. This substantiates our results because both these biotic surfaces contain the same glycans consisting of Gal and Glc, common targets for the tested lectins.
For the Caco-2 assay, lectins (ConA, CGL2, CGL3, MOA, AAL, Tec2 and CCL2) were used in three different (pre-)incubation procedures, aiming to evaluate their effectiveness in inhibiting bacterial adhesion and invasion. These processes represent critical initial stages in C. jejuni infection and subsequent disease progression (Figure 4). CCL2 was selected as a control because it did not affect C. jejuni adhesion to the biotic surfaces studied. As expected, it did not significantly decrease C. jejuni adhesion or Caco-2 cell invasion, underscoring the validity of our previous tests on biotic surfaces. Furthermore, AAL, ConA, and MOA have previously been shown to bind to C. jejuni (Turonova et al., 2016), and Day et al (Day et al., 2009). showed that ConA can decrease adhesion of C. jejuni NCTC 11168 at 37°C, which is consistent with our results. ConA binds to Man and GlcNAc, which are found on the surface of Caco-2 cells, and to Glc, which is found on the surface of C. jejuni. Thus, the results differed depending on the pre-incubation assay, with the greatest decrease in adhesion and invasion in Caco-2 cells obtained when the selected lectin and C. jejuni were added simultaneously to Caco-2 cells (Figure 3).
CGL2 and CGL3 are similar lectins that bind to beta-galactosides. Interestingly, they did not decrease C. jejuni adhesion except after 1 h of pre-incubation of CGL2 and C. jejuni. Nevertheless, both lectins lowered C. jejuni invasion after simultaneous inoculation and 1 h pre-incubation of each lectin and Caco-2 cells compared with our method’s limit of detection. By contrast, MOA, which was used at a lower concentration than the other lectins, targets Gal or GalNAc disaccharides in α-configuration, i.e. glycans found on biotic surfaces such as Caco-2 and C. jejuni cells. It decreased adhesion and invasion in all combinations, confirming the results of our previous tests on biotic surfaces. In addition, AAL and Tec2 bind to Fuc and methylated Man/Fuc, respectively, which are found on the surface of Caco-2 cells (Wong et al., 2018), and had a similar effect as MOA.
As shown by Louwen et al (Louwen et al., 2008), C. jejuni adhesion and invasion are differentially regulated. Adhesion depends more on proteins such as fibronectin-binding proteins (Henderson et al., 2011), whereas invasion is more influenced by the glycan composition of the outer membrane of C. jejuni; in particular, sialylation plays an important role in epithelial cell invasion. The glycan composition of Caco-2 cells varies and changes during differentiation and maturation (Wong et al., 2018). This may explain why different studies, e.g. Arndt et al (Arndt et al., 2011). and Tao et al (Tao et al., 2008), showed different binding affinities of lectins to Caco-2 cells, indicating the importance of experimental conditions when comparing results. The application of lectins holds promising potential across various domains, including food safety protocols, therapeutic interventions, probiotic therapy development, and biomaterial functionalization. These endeavors collectively aim to address the growing challenge of antibiotic resistance (Konozy et al., 2022).
5 Conclusion
An anti-adhesion approach proves to be a viable strategy in mitigating the persistence of foodborne pathogens in food-processing environments and their ability to invade hosts and cause disease. The inhibitory effects of lectins and PIs on C. jejuni adhesion differs between polystyrene, biotic surfaces, and Caco-2 cells. Our results emphasize that when conducting anti-adhesion tests for screening purposes, it is essential to use specific biotic surfaces rather than polystyrene. This is due to the involvement of different adhesion mechanisms, leading to potentially significant variations in results across different surfaces. Additionally, our results were influenced by different pre-incubation approaches, emphasizing the crucial role of methodological approaches when assessing lectins and PIs. Notably, the fungal lectins MOA and Tec2 significantly decreased C. jejuni adhesion to all surfaces and invasion of Caco-2 cells, even at lower concentrations (0.24 µg/mL for MOA and 10 µg/mL for Tec2). This highlights their promising potential as an alternative preventive method to combat the global spread of C. jejuni or a promising adjuvant to already existing curative treatments.
Data availability statement
The original contributions presented in the study are included in the article/Supplementary Material. Further inquiries can be directed to the corresponding author.
Ethics statement
Ethical approval was not required for the studies on humans in accordance with the local legislation and institutional requirements because only commercially available established cell lines were used.
Author contributions
BJ: Data curation, Formal analysis, Investigation, Methodology, Writing – original draft. MŠP: Formal analysis, Investigation, Methodology, Writing – review & editing. MS: Conceptualization, Data curation, Formal analysis, Investigation, Writing – review & editing. TT: Formal analysis, Investigation, Methodology, Writing – review & editing. KK: Formal analysis, Investigation, Methodology, Writing – review & editing. DT: Funding acquisition, Project administration, Writing – review & editing. TL: Funding acquisition, Investigation, Project administration, Writing – review & editing. JS: Conceptualization, Formal analysis, Funding acquisition, Investigation, Methodology, Project administration, Validation, Visualization, Writing – review & editing. AK: Conceptualization, Data curation, Funding acquisition, Investigation, Project administration, Resources, Supervision, Visualization, Writing – review & editing.
Funding
The author(s) declare that financial support was received for the research, authorship, and/or publication of this article. This work was supported by the Slovenian Research Agency (grant numbers J4-3088, J4-4548, P4-0116, P4-0432, J4-4555 and J4-2543).
Acknowledgments
We thank Dr. Eva Lasic for editing and reviewing the manuscript. We thank Maria Pesterean for technical support. We thank Prof. Dr. Markus Künzler (Institute of Microbiology, Department of Biology, Eidgenössische Technische Hochschule Zürich, Switzerland) for the gift of plasmids encoding lectins AAL, CCL2, CGL2, CGL3, PIC, MOA, and TAP1, and acknowledge Prof. Påhlsson (Linköping University, Sweden) for AAL and Dr. Minou Nowrousian (Ruhr University Bochum, Germany) for TAP1. The MOA-encoding plasmid was kindly provided to Prof. Markus Künzler by Prof. Ute Krengel (University of Oslo, Norway) with permission of Prof. Irwin Goldstein (University of Michigan).
Conflict of interest
The authors declare that the research was conducted in the absence of any commercial or financial relationships that could be construed as a potential conflict of interest.
Publisher’s note
All claims expressed in this article are solely those of the authors and do not necessarily represent those of their affiliated organizations, or those of the publisher, the editors and the reviewers. Any product that may be evaluated in this article, or claim that may be made by its manufacturer, is not guaranteed or endorsed by the publisher.
Supplementary material
The Supplementary Material for this article can be found online at: https://www.frontiersin.org/articles/10.3389/fcimb.2024.1391758/full#supplementary-material
References
Alemka, A., Corcionivoschi, N., Bourke, B. (2012). Defense and adaptation: the complex inter-relationship between Campylobacter jejuni and mucus. Front. Cell Infect. Microbiol. 2. doi: 10.3389/fcimb.2012.00015
Arndt, N. X., Tiralongo, J., Madge, P. D., Von Itzstein, M., Day, C. J. (2011). Differential carbohydrate binding and cell surface glycosylation of human cancer cell lines. J. Cell Biochem. 112, 2230–2240. doi: 10.1002/jcb.23139
Arora, S., Gordon, J., Hook, M. (2021). Collagen binding proteins of gram-positive pathogens. Front. Microbiol. 12. doi: 10.3389/fmicb.2021.628798
Bleuler-Martinez, S., Varrot, A., Olieric, V., Schubert, M., Vogt, E., Fetz, C., et al. (2022). Structure-function relationship of a novel fucoside-binding fruiting body lectin from Coprinopsis cinerea exhibiting nematotoxic activity. Glycobiology 32, 600–615. doi: 10.1093/glycob/cwac020
Boehm, M., Hoy, B., Rohde, M., Tegtmeyer, N., Bæk, K. T., Oyarzabal, O. A., et al. (2012). Rapid paracellular transmigration of Campylobacter jejuni across polarized epithelial cells without affecting TER: Role of proteolytic-active HtrA cleaving E-cadherin but not fibronectin. Gut Pathog. 4, 1–12. doi: 10.1186/1757-4749-4-3
Carrizo, M. E., Capaldi, S., Perduca, M., Irazoqui, F. J., Nores, G. A., Monaco, H. L. (2005). The antineoplastic lectin of the common edible mushroom (Agaricus bisporus) has two binding sites, each specific for a different configuration at a single epimeric hydroxyl. J. Biol. Chem. 280, 10614–10623. doi: 10.1074/jbc.M411989200
Celebioglu, H. U., Olesen, S. V., Prehn, K., Lahtinen, S. J., Brix, S., Abou Hachem, M., et al. (2017). Mucin- and carbohydrate-stimulated adhesion and subproteome changes of the probiotic bacterium Lactobacillus acidophilus NCFM. J. Proteomics 163, 102–110. doi: 10.1016/j.jprot.2017.05.015
Corcoran, A. T., Moran, A. P. (2007). Influence of growth conditions on diverse polysaccharide production by Campylobacter jejuni. FEMS Immunol. Med. Microbiol. 49, 124–132. doi: 10.1111/j.1574-695X.2006.00178.x
Dai, L., Sahin, O., Grover, M., Zhang, Q. (2020). New and alternative strategies for the prevention, control, and treatment of antibiotic-resistant Campylobacter. Trans. Res. 223, 76–88. doi: 10.1016/j.trsl.2020.04.009
Day, C. J., Tiralongo, J., Hartnell, R. D., Logue, C. A., Wilson, J. C., von Itzstein, M., et al. (2009). Differential carbohydrate recognition by Campylobacter jejuni strain 11168: influences of temperature and growth conditions. PloS One 4, e4927. doi: 10.1371/journal.pone.0004927
Day, C. J., Tram, G., Hartley-Tassell, L. E., Tiralongo, J., Korolik, V. (2013). Assessment of glycan interactions of clinical and avian isolates of Campylobacter jejuni. BMC Microbiol. 13, 228. doi: 10.1186/1471-2180-13-228
ECDC (2023). The european union one health 2022 zoonoses report. EFSA J. 12, e8442. doi: 10.2903/J.EFSA.2023.8442
Grahn, E., Askarieh, G., Holmner, Å., Tateno, H., Winter, H. C., Goldstein, I. J., et al. (2007). Crystal structure of the Marasmius oreades mushroom lectin in complex with a xenotransplantation epitope. J. Mol. Biol. 369, 710–721. doi: 10.1016/j.jmb.2007.03.016
Henderson, B., Nair, S., Pallas, J., Williams, M. A. (2011). Fibronectin: a multidomain host adhesin targeted by bacterial fibronectin-binding proteins. FEMS Microbiol. Rev. 35, 147–200. doi: 10.1111/j.1574-6976.2010.00243.x
Hennet, T. (2019). Collagen glycosylation. Curr. Opin. Struct. Biol. 56, 131–138. doi: 10.1016/j.sbi.2019.01.015
Hong, S., Cha, I., Kim, N. O., Seo, J. B., Kim, S. Y., Kim, J. H., et al. (2014). Comparative proteomic label-free analysis of Campylobacter jejuni NCTC 11168 cultured with porcine mucin. Foodborne Pathog. Dis. 11, 240–247. doi: 10.1089/FPD.2013.1596
Hsiao, C.-T., Cheng, H. W., Huang, C. M., Li, H. R., Ou, M. H., Huang, J. R., et al. (2017). Fibronectin in cell adhesion and migration via N-glycosylation. Oncotarget 8, 70653. doi: 10.18632/oncotarget.v8i41
Karlyshev, A. V., Ketley, J. M., Wren, B. W. (2005). The Campylobacter jejuni glycome. FEMS Microbiol. Rev. 29, 377–390. doi: 10.1016/J.FMRRE.2005.01.003
Katsikogianni, M., Missirlis, Y. F., Harris, L., Douglas, J. (2004). Concise review of mechanisms of bacterial adhesion to biomaterials and of techniques used in estimating bacteria-material interactions. Eur. Cell Mater 8, 37–57. doi: 10.22203/eCM
Kilcoyne, M., Twomey, M. E., Gerlach, J. Q., Kane, M., Moran, A. P., Joshi, L. (2014). Campylobacter jejuni strain discrimination and temperature-dependent glycome expression profiling by lectin microarray. Carbohydr Res. 389, 123–133. doi: 10.1016/j.carres.2014.02.005
Klančnik, A., Megušar, P., Sterniša, M., Jeršek, B., Bucar, F., Smole Možina, S., et al. (2017a). Aqueous extracts of wild mushrooms show antimicrobial and antiadhesion activities against bacteria and fungi. Phytotherapy Res. 31, 1971–1976. doi: 10.1002/ptr.5934
Klančnik, A., Šikić Pogačar, M., Trošt, K., Tušek Žnidarič, M., Mozetič Vodopivec, B., Smole Možina, S. (2017b). Anti-Campylobacter activity of resveratrol and an extract from waste Pinot noir grape skins and seeds, and resistance of Camp. jejuni planktonic and biofilm cells, mediated via the CmeABC efflux pump. J. Appl. Microbiol. 122, 65–77. doi: 10.1111/JAM.13315
Konkel, M. E., Talukdar, P. K., Negretti, N. M., Klappenbach, C. M. (2020). Taking control: Campylobacter jejuni binding to fibronectin sets the stage for cellular adherence and invasion. Front. Microbiol. 11. doi: 10.3389/fmicb.2020.00564
Konozy, E. H. E., Osman, M. E. M., Dirar, A. I., Ghartey-Kwansah, G. (2022). Plant lectins: A new antimicrobial frontier. BioMed. Pharmacother. 155, 113735. doi: 10.1016/j.biopha.2022.113735
Kovács, J. K., Cox, A., Schweitzer, B., Maróti, G., Kovács, T., Fenyvesi, H., et al. (2020). Virulence traits of inpatient Campylobacter jejuni isolates, and a transcriptomic approach to identify potential genes maintaining intracellular survival. Microorganisms 8, 531. doi: 10.3390/microorganisms8040531
Kruger, R. P., Winter, H. C., Simonson-Leff, N., Stuckey, J. A., Goldstein, I. J., Dixon, J. E. (2002). Cloning, expression, and characterization of the Galα1,3Gal high affinity lectin from the mushroom Marasmius oreades. J. Biol. Chem. 277, 15002–15005. doi: 10.1074/jbc.M200165200
Kuusela, P., Moran, A. P., Vartio, T., Kosunen, T. U. (1989). Interaction of Campylobacter jejuni with extracellular matrix components. Biochim. Biophys. Acta (BBA) - Gen. Subj. 993, 297–300. doi: 10.1016/0304-4165(89)90180-3
Louwen, R., Heikema, A., Van Belkum, A., Ott, A., Gilbert, M., Ang, W., et al. (2008). The sialylated lipooligosaccharide outer core in Campylobacter jejuni is an important determinant for epithelial cell invasion. Infect. Immun. 76, 4431–4438. doi: 10.1128/IAI.00321-08
McGuckin, M. A., Lindén, S. K., Sutton, P., Florin, T. H. (2011). Mucin dynamics and enteric pathogens. Nat. Rev. Microbiol. 9, 265–278. doi: 10.1038/nrmicro2538
Mosmann, T. (1983). Rapid colorimetric assay for cellular growth and survival: application to proliferation and cytotoxicity assays. J. Immunol. Methods 65, 55–63. doi: 10.1016/0022-1759(83)90303-4
Nowrousian, M., Cebula, P. (2005). The gene for a lectin-like protein is transcriptionally activated during sexual development, but is not essential for fruiting body formation in the filamentous fungus Sordaria macrospora. BMC Microbiol. 5, 64. doi: 10.1186/1471-2180-5-64
Olausson, J., Åström, E., Jonsson, B. H., Tibell, L. A. E., Påhlsson, P. (2011). Production and characterization of a monomeric form and a single-site form of Aleuria aurantia lectin. Glycobiology 21, 34–44. doi: 10.1093/glycob/cwq129
Olausson, J., Tibell, L., Jonsson, B. H., Påhlsson, P. (2008). Detection of a high affinity binding site in recombinant Aleuria aurantia lectin. Glycoconj J. 25, 753–762. doi: 10.1007/s10719-008-9135-7
Pohleven, J., Renko, M., Magister, Š., Smith, D. F., Künzler, M., Štrukelj, B., et al. (2012). Bivalent carbohydrate binding is required for biological activity of Clitocybe nebularis lectin (CNL), the N,N′-diacetyllactosediamine (GalNAcβ1-4GlcNAc,LacdiNAc)-specific lectin from basidiomycete C. nebularis. J. Biol. Chem. 287, 10602–10612. doi: 10.1074/jbc.M111.317263
Reid, A. N., Pandey, R., Palyada, K., Naikare, H., Stintzi, A. (2008). Identification of Campylobacter jejuni genes involved in the response to acidic pH and stomach transit. Appl. Environ. Microbiol. 74, 1583–1597. doi: 10.1128/AEM.01507-07
Renko, M., Zupan, T., Plaza, D. F., Schmieder, S. S., Nanut, M. P., Kos, J., et al. (2022). Cocaprins, β-trefoil fold inhibitors of cysteine and aspartic proteases from Coprinopsis cinerea. Int. J. Mol. Sci. 23, 4916. doi: 10.3390/ijms23094916
Sabotič, J., Bleuler-Martinez, S., Renko, M., Caglič, P. A., Kallert, S., Štrukelj, B., et al. (2012). Structural basis of trypsin inhibition and entomotoxicity of cospin, serine protease inhibitor involved in defense of Coprinopsis cinerea fruiting bodies. J. Biol. Chem. 287, 3898–3907. doi: 10.1074/jbc.M111.285304
Sabotič, J., Janež, N., Volk, M., Klančnik, A. (2023). Molecular structures mediating adhesion of Campylobacter jejuni to abiotic and biotic surfaces. Vet. Microbiol. 287, 109918. doi: 10.1016/j.vetmic.2023.109918
Sabotič, J., Ohm, R. A., Künzler, M. (2015). Entomotoxic and nematotoxic lectins and protease inhibitors from fungal fruiting bodies. Appl. Microbiol. Biotechnol. 100, 91–111. doi: 10.1007/S00253-015-7075-2
Sabotič, J., Popovič, T., Puizdar, V., Brzin, J. (2009). Macrocypins, a family of cysteine protease inhibitors from the basidiomycete Macrolepiota procera. FEBS J. 276, 4334–4345. doi: 10.1111/J.1742-4658.2009.07138.X
Schömig, V. J., Käsdorf, B. T., Scholz, C., Bidmon, K., Lieleg, O., Berensmeier, S. (2016). An optimized purification process for porcine gastric mucin with preservation of its native functional properties. RSC Adv. 6, 44932–44943. doi: 10.1039/C6RA07424C
Schubert, M., Bleuler-Martinez, S., Butschi, A., Wälti, M. A., Egloff, P., Stutz, K., et al. (2012). Plasticity of the β-trefoil protein fold in the recognition and control of invertebrate predators and parasites by a fungal defence system. PloS Pathog. 8, e1002706. doi: 10.1371/annotation/088ea07b-d578-4586-9707-160143d4f1be
Shi, L., Ardehali, R., Caldwell, K. D., Valint, P. (2000). Mucin coating on polymeric material surfaces to suppress bacterial adhesion. Colloids Surf B Biointerfaces 17, 229–239. doi: 10.1016/S0927-7765(99)00121-6
Šikić Pogačar, M., Rubeša Mihaljević, R., Klančnik, A., Brumini, G., Abram, M., Smole Možina, S. (2009). Survival of stress exposed Campylobacter jejuni in the murine macrophage J774 cell line. Int. J. Food Microbiol. 129, 68–73. doi: 10.1016/j.ijfoodmicro.2008.11.010
Singh, B., Fleury, C., Jalalvand, F., Riesbeck, K. (2012). Human pathogens utilize host extracellular matrix proteins laminin and collagen for adhesion and invasion of the host. FEMS Microbiol. Rev. 36, 1122–1180. doi: 10.1111/j.1574-6976.2012.00340.x
Studier, F. W. (2005). Protein production by auto-induction in high density shaking cultures. Protein Expr Purif 41, 207–234. doi: 10.1016/j.pep.2005.01.016
Talukdar, P. K., Negretti, N. M., Turner, K. L., Konkel, M. E. (2020). Molecular dissection of the Campylobacter jejuni cadF and flpA virulence proteins in binding to host cell fibronectin. Microorganisms 8, 389. doi: 10.3390/microorganisms8030389
Tao, S. C., Li, Y., Zhou, J., Qian, J., Schnaar, R. L., Zhang, Y., et al. (2008). Lectin microarrays identify cell-specific and functionally significant cell surface glycan markers. Glycobiology 18, 761–769. doi: 10.1093/glycob/cwn063
Turonova, H., Neu, T. R., Ulbrich, P., Pazlarova, J., Tresse, O. (2016). The biofilm matrix of Campylobacter jejuni determined by fluorescence lectin-binding analysis. Biofouling 32, 597–608. doi: 10.1080/08927014.2016.1169402
Van Alphen, L. B., Wenzel, C. Q., Richards, M. R., Fodor, C., Ashmus, R. A., Stahl, M., et al. (2014). Biological roles of the O-methyl phosphoramidate capsule modification in Campylobacter jejuni. PloS One 9 1, e87051. doi: 10.1371/journal.pone.0087051
Walser, P. J., Haebel, P. W., Künzler, M., Sargent, D., Kües, U., Aebi, M., et al. (2004). Structure and functional analysis of the fungal galectin CGL2. Structure 12, 689–702. doi: 10.1016/j.str.2004.03.002
Wälti, M. A. (2008). Structural basis for chitotetraose coordination by cgl3, a novel galectin-related protein from Coprinopsis cinerea. J. Mol. Biol. 379, 146–159. doi: 10.1016/j.jmb.2008.03.062
Wimmerova, M., Mitchell, E., Sanchez, J. F., Gautier, C., Imberty, A. (2003). Crystal structure of fungal lectin: six-bladed beta-propeller fold and novel fucose recognition mode for Aleuria aurantia lectin. J. Biol. Chem. 278, 27059–27067. doi: 10.1074/jbc.M302642200
Wohlschlager, T., Butschi, A., Grassi, P., Sutov, G., Gauss, R., Hauck, D., et al. (2014). Methylated glycans as conserved targets of animal and fungal innate defense. Proc. Natl. Acad. Sci. U.S.A. 111, E2787–E2796. doi: 10.1073/pnas.1401176111
Wong, M., Xu, G., Park, D., Barboza, M., Lebrilla, C. B. (2018). Intact glycosphingolipidomic analysis of the cell membrane during differentiation yields extensive glycan and lipid changes. Sci. Rep. 8, 1–10. doi: 10.1038/s41598-018-29324-7
Keywords: Campylobacter jejuni, fungal lectins, fungal protease inhibitors, Caco-2 assay, adhesion, invasion
Citation: Jug B, Šikić Pogačar M, Sterniša M, Tumpej T, Karničar K, Turk D, Langerholc T, Sabotič J and Klančnik A (2024) Modulation of Campylobacter jejuni adhesion to biotic model surfaces by fungal lectins and protease inhibitors. Front. Cell. Infect. Microbiol. 14:1391758. doi: 10.3389/fcimb.2024.1391758
Received: 26 February 2024; Accepted: 03 April 2024;
Published: 22 April 2024.
Edited by:
Nasib Singh, Eternal University, IndiaReviewed by:
Alexis Rodríguez, Autonomous University of the State of Morelos, MexicoLiangqian Huang, Nanjing University, China
Copyright © 2024 Jug, Šikić Pogačar, Sterniša, Tumpej, Karničar, Turk, Langerholc, Sabotič and Klančnik. This is an open-access article distributed under the terms of the Creative Commons Attribution License (CC BY). The use, distribution or reproduction in other forums is permitted, provided the original author(s) and the copyright owner(s) are credited and that the original publication in this journal is cited, in accordance with accepted academic practice. No use, distribution or reproduction is permitted which does not comply with these terms.
*Correspondence: Anja Klančnik, YW5qYS5rbGFuY25pa0BiZi51bmktbGouc2k=