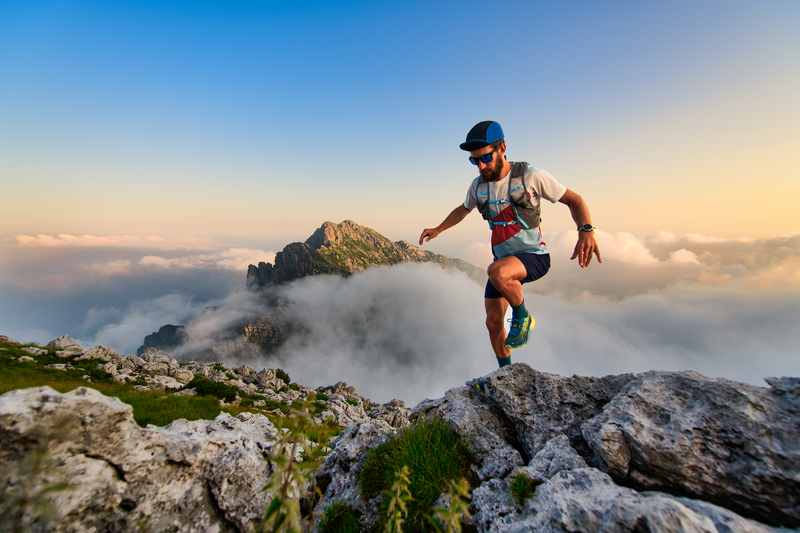
95% of researchers rate our articles as excellent or good
Learn more about the work of our research integrity team to safeguard the quality of each article we publish.
Find out more
ORIGINAL RESEARCH article
Front. Cell. Infect. Microbiol. , 15 May 2024
Sec. Antibiotic Resistance and New Antimicrobial drugs
Volume 14 - 2024 | https://doi.org/10.3389/fcimb.2024.1390934
Avian colibacillosis (AC), caused by infection with Escherichia coli (E. coli), is a major threat to poultry health, food safety and public health, and results in high mortality and significant economic losses. Currently, new drugs are urgently needed to replace antibiotics due to the continuous emergence and increasing resistance of multidrug-resistant (MDR) strains of E. coli caused by the irrational use of antibiotics in agriculture and animal husbandry. In recent years, antimicrobial peptides (AMPs), which uniquely evolved to protect the host, have emerged as a leading alternative to antibiotics in clinical settings. CATH-2, a member of the antimicrobial cathelicidin peptide family, has been reported to have antibacterial activity. To enhance the antimicrobial potency and reduce the adverse effects on animals, we designed five novel AMPs, named C2-1, C2-2, C2-3, C2-4 and C2-5, based on chicken CATH-2, the secondary structures of these AMPs were consistently α-helical and had an altered net charge and hydrophobicity compared to those of the CATH-2 (1-15) sequences. Subsequently, the antimicrobial activities of CATH-2 (1-15) and five designed peptides against MDR E. coli were evaluated in vitro. Specifically, C2-2 showed excellent antimicrobial activity against either the ATCC standard strain or veterinary clinical isolates of MDR E. coli, with concentrations ranging from 2-8 μg/mL. Furthermore, C2-2 maintained its strong antibacterial efficacy under high temperature and saline conditions, demonstrating significant stability. Similarly, C2-2 retained a high level of safety with no significant hemolytic activity on chicken mature red blood cells or cytotoxicity on chicken kidney cells over the concentration range of 0-64 μg/mL. Moreover, the administration of C2-2 improved the survival rate and reduced the bacterial load in the heart, liver and spleen during MDR E. coli infection in chickens. Additionally, pathological damage to the heart, liver and intestine was prevented when MDR E. coli infected chickens were treated with C2-2. Together, our study showed that C2-2 may be a promising novel therapeutic agent for the treatment of MDR E. coli infections and AC.
Avian colibacillosis (AC) is an infectious disease caused by the avian pathogen E. coli (APEC), that results in fibrinoid lesions around internal organs (Gao et al., 2018; Nicolas et al., 2023). This disease can lead to primary infection as well as secondary infection with other viruses or pathogens (Sargeant et al., 2019; Walker et al., 2020). Antibiotics play an important role in the prevention and treatment of AC (Roth et al., 2019), but their overuse has led to the development of multidrug-resistant (MDR) E. coli strains (Kumar et al., 2020). By 2030, more than half of incoming E. coli isolates could be resistant to third-generation cephalosporins (Alvarez-Uria et al., 2018). This complicates the treatment of AC and presents a significant obstacle to the agricultural industry. Additionally, excessive antibiotic use is also linked to drug residues in soil, groundwater and animal-derived foods, posing a significant threat to human health (Ghimpeţeanu et al., 2022; Huang et al., 2022; Shengnan et al., 2023). Many countries have banned the use of antibiotics in animal feed to eliminate their adverse effects (Treiber and Beranek-Knauer, 2021; Getachew et al., 2023). Moreover, second, third and fourth generation antibiotics have been developed, most of which are chemically modified from the original antibiotics (Voulgaris et al., 2019; Rusu and Buta, 2021). Unfortunately, these new antibiotics do not fully address the problems associated with E. coli resistance because the antibacterial mechanisms remain unchanged. Therefore, it is necessary to develop new effective drugs against E. coli infections, especially for MDR strains.
Antimicrobial peptides (AMPs), which are components of the innate immune system, play a crucial role in defense against external pathogens (Torres et al., 2019; Ciulla and Gelain, 2023). AMPs are commonly present in various organisms and typically range from 12 to 50 amino acids, half of which contain arginine and lysine residues (Valdez-Miramontes et al., 2021). These peptides were primarily found on animal surfaces in close proximity to pathogens, including the skin, ears, eyes, and epithelial lining of the tongue, trachea, lungs, intestines, bone marrow, and testes (Hancock and Scott, 2000). AMPs have been reported to effectively suppress various microorganisms, such as gram-positive or gram-negative bacteria, fungi, parasites, viruses and tumor cells. The majority of AMPs directly target the bacterial cell membrane, producing antimicrobial effects (Mourtada et al., 2019), and some have multiple targets within the bacterial cell (Torres et al., 2019), a unique mechanism for killing MDR bacteria (Xuan et al., 2023). Subsequent studies have shown that AMPs have various additional functions, including inducing differentiation, and chemotaxis; activating various leukocyte types; inhibiting lipopolysaccharide-induced effects; promoting DNA uptake and phagocytosis; and promoting wound healing (Semple and Dorin, 2012; Mwangi et al., 2019). Hence, the development of AMPs as superior alternatives to antibiotics for the treatment of bacterial infections, especially those caused by drug-resistant bacteria, has great potential for expansion (Rima et al., 2021; Dong et al., 2022).
Chickens have three primary families of antimicrobial peptides: defensins, cathelicidins and LEAP-2. CATH-1 to CATH-3 and CATH-B1, members of the cathelicidin family, have been identified as four specific antimicrobial peptides (Goitsuka et al., 2007; Achanta et al., 2012). Among them, CATH-2, which is found mainly in heterophilic cells and consists of two helices (Cuperus et al., 2013), has been reported to have antibacterial and immunoregulatory properties (Coorens et al., 2017; van Harten et al., 2018; Scheenstra et al., 2019). Importantly, its antimicrobial activity is unaffected by salt concentration (Zhang and Sunkara, 2014), making it a valuable template for novel AMP design.However, the therapeutic use of CATH-2 is limited by its high cytotoxicity and relatively weak antibacterial activity (Askari et al., 2021; Botelho Sampaio de Oliveira et al., 2023). In this study, we further optimized CATH-2 (1-15), a peptide derived from CATH-2, by intercepting the core antibacterial region and reducing the cytotoxicity of the original sequence (van Dijk et al., 2009; Xiao et al., 2009). The antibacterial activity against E. coli and the stability and safety of five designed peptides, C2-1, C2-2, C2-3, C2-4 and C2-5, were subsequently evaluated in vitro. Surprisingly, compared with CATH-2 (1-15) and most other designed peptides, C2-2 showed superior antibacterial activity against standard strains and veterinary clinical MDR strains of E. coli. In addition, C2-2 maintained stable antibacterial activity at high temperatures and in saline solutions while maintaining a high level of safety within a specific concentration range. Furthermore, C2-2 administration significantly reduced the mortality, bacterial load and pathological damage induced by MDR E. coli infection in chickens. In conclusion, this study showed that C2-2 may be a potent candidate against standard and MDR E. coli infections.
The 1-day-old chickens used in this study were obtained from Shanxi Jiabo Agricultural and Animal Husbandry Development Co., Ltd. The chicks were group-housed and given ad libitum access to food and water until 21 days of age.
Five new AMPs were generated through the incorporation of amino acid substitutions into the CATH-2 (1-15) sequence. CATH-2 (1-15) (RFGRFLRKIRRFRPK), C2-1 (RFGRFLRKIRRFIPK), C2-2 (RFGRFLRKIRRMRLK), C2-3 (RFGRHLRKIIRFRIK), C2-4 (RFGRNLRKIRRFWPK), and C2-5 (RFGRFLRKIRFFRLK) were synthesized by PeptideValley. The purity of the products was determined to be greater than 95% by high-performance liquid chromatography (HPLC) and mass spectrometry.
The physicochemical properties of the AMPs were analyzed using ProtParam software (https://web.expasy.org/protparam/). The spatial structure of the AMPs was constructed using PRBS (https://mobyle2.rpbs.univ-paris-diderot.fr/cgi-bin/portal.py#welcome), while helical wheel models of the resulting peptides were created using HeliQuest (https://heliquest.ipmc.cnrs.fr/).
E. coli (ATCC 8739) and clinical isolates of E. coli (E1, E9, E12, E14, E16, and E18) were stored at the Animal Biochemical Laboratory of Shanxi Agricultural University and grown in Luria-Bertani (LB) broth as previously reported (Zhao et al., 2021). Circular 6 mm paper pieces were prepared with filter paper and autoclaved for sterilization. Solutions of penicillin (PG), florfenicol (FF), ciprofloxacin (CIP), enrofloxacin (ENR), and gentamicin (GM) were prepared according to the Clinical and Laboratory Standards Institute (CLSI). Then, 0.5 mL of antibiotic solution was added to 100 sterilized papers. Subsequently, the paper pieces were fully infiltrated and dried in a vacuum drying oven to produce antibiotic susceptibility papers. The E. coli strain was retrieved from the -20°C freezer and activated by incubating it on LB agar media at 37°C for 24h. Individual colonies were selected and transferred to LB liquid media. Thereafter, the E. coli suspension was obtained by shaking at 37°C and 160 r/min for 18h. The obtained suspension was centrifuged at 5000 r/min for 5min, after which the supernatant was discarded, and the bacteria were resuspended in fresh medium to a concentration of 1×106 CFU/mL. Two hundred microliters of the bacterial suspension mentioned above was spread evenly onto LB agar medium. Then, the antibiotic susceptibility papers were placed on the medium surface using sterile tweezers. After 24h of incubation at 37°C, the diameter of the inhibition zone was measured to determine the susceptibility of E. coli to antibiotics.
The minimum inhibitory concentrations (MICs) of the AMPs were determined using the microbroth dilution technique (van Harten et al., 2021). One hundred microliters of bacterial suspension at a concentration of 1×106 CFU/mL was pipetted into a 96-well plate. Then, 100 μL of AMP or gentamicin at various concentrations was added, while the bacterial suspension without AMPs and blank medium were used as controls. The plate was incubated at a constant temperature of 37°C for 18h, after which the turbidity of the wells was observed to determine the MIC. Fifty microlitres of liquid was extracted and plated on LB agar from wells exhibiting complete bacterial growth inhibition. The minimum bactericidal concentration (MBC) of the AMPs was assessed by counting colonies following a 24h incubation at 37°C.
The sterilization kinetics assay was performed following previous methods (Peng et al., 2022; Yong Fang et al., 2023). Gentamicin, C2-1 and C2-2 at a concentration of 8 μg/mL each were added to a bacterial suspension of 1×105 CFU/mL, and incubated at 37°C for 0, 15, 30, 60, 120, or 240min. At each time point, 10 μL of sample was diluted in 1 mL of PBS, and 100 μL of the dilution was plated on LB agar plates. After incubating at 37°C for 24h, the number of colonies was counted.
The MICs of the AMPs against E. coli (ATCC 8739) at different temperatures and physiological salt concentrations were evaluated. The thermal stability of the AMPs was assessed by incubating them at a concentration of 500 μg/mL for 1h at 0, 37, and 100°C, using untreated AMPs as a control. The salt ion stability of the AMPs was determined by incubating them at a concentration of 500 μg/mL in solutions of NaCl (150 mM), KCl (4.5 mM), MgCl2 (1 mM), and CaCl2 (2.5 mM) for 1h, using untreated samples as controls. The changes in the MICs of the AMPs were assessed following these experimental procedures.
Fresh blood was harvested from chickens, anticoagulated with 0.2% sodium heparin, and centrifuged at 1000 r/min for 10min to remove the supernatant. The lower erythrocytes were collected, gently washed twice with sterile PBS, and subsequently prepared as a 2% erythrocyte suspension. The erythrocyte suspension was mixed with AMPs at different concentrations in equal volumes. Erythrocytes treated with PBS served as a negative control, while erythrocytes treated with 2% Triton X-100 served as a positive control. The mixture was incubated at 37°C for 1h, after which the absorbance at 570 nm was measured using a multifunctional enzyme marker.
Chicken renal cells were trypsinized, seeded at 5000 cells per well in 96-well plates, and incubated for 24h. The cell culture medium was subsequently discarded, and AMPs were added at different concentrations to the wells, which were subsequently filtered through a 0.22 μm filter to eliminate bacteria. The mixture was incubated for another 24h, after which 50 μL of 3-(4,5-dimethyl-2-thiazolyl)-2, 5-diphenyl tetrazolium bromide (MTT) reagent (5 mg/mL) was directly added to each well. The mixture was incubated for 4h. Then 50 μL of MTT reagent (5 mg/mL) was added directly to each well and incubated for another 4h. To dissolve the crystals, 150 μL of dimethyl sulfoxide (DMSO) was added, and the mixture was vortexed for 10min. The absorbance was measured at 490 nm using a multifunctional enzyme marker.
Fifty 21-day-old chickens were randomly divided into five groups: blank control (500 μL of normal saline), E. coli infection (500 μL of normal saline), C2-2 treatment (200 μg/mL, 500 μL), gentamicin treatment (200 μg/mL, 500 μL) and enrofloxacin treatment (200 μg/mL, 500 μL). Except for the blank control group injected with 500 μL of normal saline, the other four groups were injected with 500 μL of 3×109 CFU/mL E. coli (E16) into the pectoral muscles. Then, normal saline, C2-2, gentamicin and enrofloxacin were injected through the pectoral muscles 4h after E. coli infection according to the above methods. Clinical symptoms and were observed and documented for the chickens in each group after infection. After 72h, the chickens in each group were euthanized and examined for internal organ damage. Paraffin sections were prepared for microscopic examination of pathological changes. Under sterile conditions, 1 g samples of heart, liver, and spleen were collected, and each sample was supplemented with 20 mL of sterile PBS. After thorough grinding, the sample was diluted to the appropriate multiple, and 100 μL of the diluent was spread onto LB agar medium. The plates were incubated for 24h, after which the colonies were counted.
Statistical analysis was performed using one-way analysis of variance (ANOVA). The data represent three independent experiments and are presented as mean ± SEM. A p-value of< 0.05 was considered to indicate statistical significance.
The sequences and physicochemical properties of the peptides used are listed in Table 1. CATH-2 (1-15) is an N-terminal truncated peptide of chicken CATH-2 that consists of 15 amino acids and has an α-helical secondary structure and 8 positive charges. Based on the CATH-2 (1-15) sequence, five peptides, named C2-1 to C2-5, were designed by substituting amino acids to modify their charge and hydrophobicity. C2-2 was positively charged with 8 net charges, and the others had 7 net charges. Among them, CATH-2 (1-15) had the lowest hydrophobicity (0.103), and C2-5 had the highest hydrophobicity (0.355). According to the average hydrophilicity, C2-4 had the strongest hydrophilicity, followed by CATH-2 (1-15), and C2-2 ranked third. The predicted secondary structures showed that all five peptides were α-helical (Figure 1A).The spiral-wheel model highlighted changes in the hydrophobic moment, as well as the hydrophobic and hydrophilic characteristics of the AMPs (Figure 1B). All six peptides had an amphipathic structure that formed a hydrophilic and hydrophobic side.
Figure 1 Structure prediction of AMPs. (A) Prediction of the spatial structure of AMPs. (B) Helical wheel projections of AMPs. Residues are color-coded: polar basic residues in blue, non-polar hydrophobic residues in yellow, glycine in grey, and proline represented by green circles. The hydrophobic moment is marked by a black arrow on the helical wheel.
To comprehensively and accurately evaluate the effects of AMPs on different strains of E. coli, we isolated 6 strains of E. coli (E1, E9, E12, E14, E16, and E18) from poultry farms in Shanxi Province and analyzed their resistance to penicillin, florfenicol, ciprofloxacin, enrofloxacin and gentamicin, which are commonly used in the aquaculture industry for the treatment of E. coli infections. The results indicated that the 6 E. coli strains were all resistant to penicillin, florfenicol and enrofloxacin. Moreover, only the E14 strain of E. coli was sensitive to ciprofloxacin, while three strains (E12, E14, and E16) of E. coli were susceptible to gentamicin. In summary, E. coli strains E1 and E18 were found to have relatively high levels of resistance (Table 2).
The antimicrobial activities of CATH-2 (1-15) and the designed peptides were tested against 7 strains of E. coli. C2-1 and C2-2 exhibited potent antibacterial activity against 6 MDR E. coli strains. The MICs of C2-1 ranged from 0.5 to 16 μg/mL, and 0.5 μg/mL could inhibit the growth of E9. The MICs of C2-2 ranged from 2 to 8 μg/mL. Compared with the CATH-2 (1-15), it increased the inhibitory effect on six MDR E. coli strains by 2 to 32 times. The inhibitory effects of C2-3 and C2-5 were slightly weaker than those of C2-1 and C2-2. The inhibitory effect of C2-4 was lower than that of the CATH-2 (1-15), which had the poorest inhibitory effect. The MICs of gentamicin were between 4- and 64 μg/mL (Table 3). The MBC values of the AMPs further confirmed the above results (Table 4). In brief, the MBC values of CATH-2 (1-15) and the designed peptides were found to be greater than the MIC values. It is worth noting that the MBC values of C2-2 remained within the range of 2-8 μg/mL. The results suggested that C2-1 and C2-2 exhibited potent in vitro inhibitory effects on several MDR E. coli strains.
In this study, the bactericidal kinetics of C2-1, C2-2, and gentamicin were evaluated against E. coli (ATCC8739), indicating their efficacy against bacteria. Gentamicin at a concentration of 8 μg/mL exhibited the most potent bactericidal effect, as it completely eradicated bacteria within 60min. C2-2 showed the second most potent bactericidal activity, while C2-1 had the least potent effect, requiring 240min for complete bacterial eradication (Figure 2).
Figure 2 Bacterial survival after treatment with peptides. The bacterial suspension of E. coli (ATCC 8739) was incubated with C2-1 and C2-2, each at a concentration of 8 μg/mL of gentamicin, for 4h at 37°C to determine the number of viable bacteria. Data represent three independent experiments and are presented as mean ± SEM.
The thermal stability and salt ion stability of AMP are two important factors limiting its clinical use and were also tested in this study. As shown in Table 5, after 1h of incubation at 100°C, CATH-2 (1-15) exhibited a 2-fold increase in antimicrobial efficacy, whereas C2-5 experienced a 4-fold reduction under the same conditions, while the MICs of the other four designed peptides remained constant. In addition, antimicrobial activity of CATH-2 (1-15) increased 2-fold in the presence of sodium ions and fourfold in the presence of potassium and magnesium ions. C2-1 demonstrated a 4-fold increase in antimicrobial activity with sodium ions but a fourfold decrease with calcium ions. C2-2 displayed a 16-fold increase in antimicrobial activity with sodium ions, potassium ions, or magnesium ions and a 2-fold increase with calcium ions. C2-3 experienced a 4-fold increase in antimicrobial activity with potassium or magnesium ions. C2-4 exhibited a 2-fold decrease in antimicrobial activity with sodium, potassium, or calcium ions, while C2-5 exhibited a fourfold decrease with sodium, potassium, or magnesium ions and an eightfold decrease with calcium ions. In general, with the exception of C2-4 and C2-5, the other analogs consistently retained potent antimicrobial activity at diverse temperatures or under salt ion conditions.
Table 5 The MIC (μg/mL) values of AMPs against E. coli (ATCC 8739) at different temperatures and salt solutions.
In this study, we investigated the hemolytic activity of peptides derived from CATH-2 on mature chicken erythrocytes. Except for C2-1, the remaining four peptides exhibited no significant hemolytic activity compared to that of CATH-2 (1-15) (Figure 3A). In addition, cytotoxicity is an important indicator of the safety of AMPs. Except for C2-1, no significant cytotoxicity against chicken kidney cells was observed after treatment with the designed peptides in the concentration range of 0-64 μg/mL (Figure 3B).
Figure 3 Safety of the designed peptides. (A) Hemolytic activities of the peptides at various concentrations upon incubation with chicken erythrocytes for 24h. (B) Cytotoxicity of the peptides at various concentrations upon incubation with chicken kidney cells for 24h. Data represent three independent experiments and are presented as mean ± SEM. *P < 0.05; **P < 0.01; ***P < 0.001; ns, not significant.
The E16 strain of E. coli was selected for chicken infection based on its resistance and because a previous study reported that E16 exhibited greater toxicity. To determine the role of C2-2 in preventing E. coli infection in vivo, chickens were infected with 1.5× 109 CFU of E16 with or without C2-2 administration, and survival was monitored over time. The blank control group showed no abnormal changes after 3 days of infection and maintained 100% survival. In contrast, the E. coli infection group exhibited the most severe clinical symptoms, such as depression, loss of appetite, drooping wings, sensitivity to cold, and discharge of yellowish-green feces, which were present 12h post infection. Mortality of normal infected chickens occurred at 1 day post infection, whereas infected chickens treated with C2-2 began to die at 2 days post infection. In addition, the survival rate of E. coli infected chickens in the presence of C2-2 was 70% by day 3, which was markedly greater than that of chickens not receiving C2-2 administration (30%) (Figure 4A). The 3-day mortality rate of the C2-2 treatment group was intermediate between that of the gentamicin and enrofloxacin treatment groups at 10% and 50%, respectively. There was no difference in survival rate between the enrofloxacin-treated and untreated groups, and the survival rate in both groups was significantly lower than that in the gentamicin-treated group, which was due to the presence of gentamicin-sensitive and enrofloxacin-resistant E16 strains (Figure 4A). To investigate whether the attenuation of pathogenesis noted above was due to a decreased burden of E. coli in the chickens administered C2-2, we analyzed the bacterial loads in the heart, liver and spleen after 3 days of infection. The results showed that C2-2 and gentamicin significantly reduced the bacterial loads in the heart, liver and spleen of infected chickens, whereas enrofloxacin affected only the heart bacterial load. The liver bacterial burden in the E. coli infection group was 1.61×107 CFU/g, and the liver bacterial load in the C2-2 treatment group was 2.37×104 CFU/g, which was reduced by approximately 680 times, indicating that C2-2 treatment had the greatest effect on the bacterial load in the liver (Figure 4B).
Figure 4 C2-2 inhibits MDR E. coli infection in vivo. (A) Protective efficacy in chickens in each group after infection. (B) Organ bacterial load in the E. coli infection model. (C) Pathological changes of heart, liver and intestine in different treatment groups. (D) Pathological changes in heart, liver and jejunum of chickens in each experimental group. NS: normal saline; GM: gentamicin; ENR: enrofloxacin. Data represent three independent experiments and are presented as mean ± SEM. *P < 0.05; **P < 0.01; ***P < 0.001; ns, not significant.
After the chickens died, an autopsy was conducted to examine the pathological changes in the heart, liver, and intestine. Chickens infected with MDR E. coli had a heart surface covered with a yellow fibrinous exudate. The liver was enlarged, tender in texture, yellow in color, and occasionally covered with yellow-white cellulose exudate. In the intestines of the chickens, congestion, hemorrhage and enlargement of the mesenteric lymph nodes were observed to varying degrees. Some of the affected chickens exhibited symptoms of splenomegaly and severe thymic congestion. Overall, in contrast to those in the E. coli infection group, there was a significant reduction in organ lesions in both the C2-2 and gentamicin treatment groups (Figure 4C). The histologic sections of the heart, liver and jejunum from the different treatment groups are presented in Figure 4D. Compared with tose in the negative control group, the E. coli infection group and the enrofloxacin treatment group exhibited significant thickening of the external cardiac membrane, extensive fibrous coverage on the surface, swelling, and rupture of myocardial fibers, as well as fibrous exudates in the interstitial spaces. Hepatocytes showed swelling and granular degeneration, accompanied by disordered arrangement of hepatocyte cords and marked infiltration of inflammatory cells. The jejunal villi were observed to be disrupted, and the intestinal mucosa was congested and swollen with infiltration of inflammatory cells. In the C2-2 treatment group and the gentamicin treatment group, the myocardial fibers were arranged neatly without any granular degeneration of cells, with only a small amount of fibrinous exudate on the surface. The liver lobules appeared normal in structure with visible hepatocytes and no granular degeneration. The jejunal villi were relatively structurally intact with a small number of inflammatory cells in the submucosa.
AC caused by E. coli infection is associated with respiratory and systemic diseases (Alber et al., 2021). The primary treatment for AC involves antibiotics, which contribute to the increasing occurrence of MDR E. coli (Christensen et al., 2021). In this study, drug susceptibility testing was performed on clinical isolates of E. coli, revealing their MDR nature. This finding confirms the widespread presence of MDR E. coli, which poses a significant threat to the global agricultural industry and emphasizes the need to develop drugs to combat drug-resistant bacterial infections.
The antimicrobial activity of AMPs primarily depends on the physical and structural characteristics of the peptide, including its net charge, hydrophobicity, amphiphilicity, and sequence length (Torres et al., 2019). Minor alterations in the amino acid sequence can entirely alter the physical and chemical properties of an AMP. In particular, the net charge and hydrophobicity are thought to influence bacterial binding. Bacterial cell membranes, which are mainly lipid-based, carry a substantial negative charge and can interact with positively charged AMPs (Dwivedi et al., 2023). Nevertheless, excessive hydrophobicity can result in increased hemolytic activity. Peptide amphiphilicity closely correlates with the hydrophobic moment, which, in turn, is directly influenced by the secondary structure of the peptide (Eisenberg et al., 1982). Thus, the structure of the peptide is critical for antimicrobial activity. CATH-2 (1-15) has shown antimicrobial activity against a wide range of bacteria (Sharma et al., 2022). However, its antibacterial activity is relatively weak, and its effectiveness against MDR E. coli has not been thoroughly investigated. This study aimed to modify the amino acid composition of CATH-2 (1-15) to regulate hydrophobicity and charge, leading to the design of five AMPs. The feasibility of using these AMPs as antibiotic replacements for the treatment of MDR E. coli infections was investigated.
Among the six AMPs evaluated in this study, C2-4 had the lowest net charge and hydrophobicity, resulting in the weakest antimicrobial activity. The MICs of CATH-2 (1-15) and C2-2 against MDR E. coli were 4-128 μg/mL and 2-8 μg/mL, respectively. This difference may be attributed to the similar net charges of CATH-2 (1-15) and C2-2, but C2-2 possessed greater hydrophobicity than CATH-2 (1-15). Compared to C2-2, C2-1 had a slightly lower net charge but greater hydrophobicity and amphiphilicity, resulting in enhanced antimicrobial activity. However, the elevated hydrophobicity of C2-1 was also associated with a significant increase in hemolytic activity, which limited its clinical application. These findings suggest that the antimicrobial activity of peptides is not determined by a specific amino acid sequence or physicochemical property. Instead, it is related to the overall amino acid composition of the peptide (Findlay et al., 2010).
In vitro bacterial inhibition experiments revealed that the MICs of C2-2 against MDR E. coli ranged from 2 to 8 μg/mL. The difference in the inhibitory concentration exhibited by the AMPs against different strains of bacteria may be attributed to the differences in the membrane lipid composition of different MDR strains, drug-resistant genes, the intracellular environment, and other factors, which may affect the combination of the AMPs with the bacteria to exert antimicrobial effects. Additionally, at a concentration of 8 μg/mL, C2-2 entirely eradicated E. coli within 2h, demonstrating a faster sterilization rate. Given the complex that AMPs encounter upon entering the body, this study examined changes in the MICs of AMPs under adverse conditions such as low temperature, high temperature, and elevated salt ion concentrations. Normally, the positive charge carried by salt ions affects the binding of AMPs to bacteria, thereby influencing antimicrobial activity (Shenrui et al., 2023). Notably, C2-2 exhibited strong antimicrobial activity even in these challenging environments, consistent with previous findings (Xiao et al., 2006). This is because C2-2 is unaffected by salt ions, which can impair its antistructural properties, and metal ions are themselves bacteriostatic, disrupting cell membranes and facilitating the bacteriostatic effects of the AMPs.
Previous studies have shown that treatment of chicken embryos with D-CATH-2, an analog of CATH-2, results in a significant reduction in the number of pathogenic E. coli and in the respiratory bacterial load (Cuperus et al., 2016). Our present study produced similar results, as treatment of MDR E. coli-infected chicks with C2-2 significantly improved the survival rate of infected chicks and markedly reduced bacterial counts in the hearts, livers, and spleens of the affected chicks. This study developed a low-toxicity, efficient, and stable AMP, providing a foundation for the development of drugs targeting MDR E. coli. However, the inhibitory mechanism of C2-2 against MDR E. coli and its immunomodulatory effects on this organism remain unclear and warrant further investigation.
The original contributions presented in the study are included in the article/supplementary material, Further inquiries can be directed to the corresponding authors.
The animal study was approved by the Animal Care Committee of Shanxi Agricultural University. The study was conducted in accordance with the local legislation and institutional requirements.
XG: Methodology, Writing – original draft. SH: Methodology, Writing – original draft. WS: Resources, Writing – review & editing. LC: Investigation, Writing – review & editing. TK: Investigation, Writing – review & editing. BW: Resources, Writing – review & editing. SC: Conceptualization, Funding acquisition, Writing – review & editing.
The author(s) declare financial support was received for the research, authorship, and/or publication of this article. This research was funded by the National Natural Science Foundation of China (grant number: 82102409), the Earmarked Fund for Modern Agro-industry Technology Research System of Shanxi Province, the Key Research and Development Program of Shanxi Province (grant number: 201903D221013), the Distinguished and Excellent Young Scholar Cultivation Project of Shanxi Agricultural University (grant number: 2023YQPYGC01), and the Horizontal Scientific Research Project of Shanxi Agricultural University (grant number: 2022HX124), the Shanxi Province Doctoral and Post-doctoral Award Funds (grant number: SXBYKY2023002).
The authors declare that the research was conducted in the absence of any commercial or financial relationships that could be construed as a potential conflict of interest.
All claims expressed in this article are solely those of the authors and do not necessarily represent those of their affiliated organizations, or those of the publisher, the editors and the reviewers. Any product that may be evaluated in this article, or claim that may be made by its manufacturer, is not guaranteed or endorsed by the publisher.
Achanta, M., Sunkara, L. T., Dai, G., Bommineni, Y. R., Jiang, W., Zhang, G. (2012). Tissue expression and developmental regulation of chicken cathelicidin antimicrobial peptides. J. Anim. Sci. Biotechnol 3, 15. doi: 10.1186/2049-1891-3-15
Alber, A., Stevens, M. P., Vervelde, L. (2021). The bird’s immune response to avian pathogenic Escherichia coli. Avian Pathol 50, 382–391. doi: 10.1080/03079457.2021.1873246
Alvarez-Uria, G., Gandra, S., Mandal, S., Laxminarayan, R. (2018). Global forecast of antimicrobial resistance of Escherichia coli and Klebsiella pneumoniae in invasive isolates. Int. J. Infect. Dis 68, 50-53. doi: 10.1016/j.ijid.2018.01.011
Askari, P., Namaei, M. H., Ghazvini, K., Hosseini, M. (2021). In vitro and in vivo toxicity and antibacterial efficacy of melittin against clinical extensively drug-resistant bacteria. BMC Pharmacol. Toxicol 22, 42. doi: 10.1186/s40360-021-00503-z
Botelho Sampaio de Oliveira, K., Lopes Leite, M., Albuquerque Cunha, V., Brito da Cunha, N., Luiz Franco, O. (2023). Challenges and advances in antimicrobial peptide development. Drug Discovery Today 28, 103629. doi: 10.1016/j.drudis.2023.103629
Christensen, H., Bachmeier, J., Bisgaard, M. (2021). New strategies to prevent and control avian pathogenic Escherichia coli (APEC). Avian Pathol 50, 370-381. doi: 10.1080/03079457.2020.1845300
Ciulla, M. G., Gelain, F. (2023). Structure–activity relationships of antibacterial peptides. Microbial Biotechnol 16, 757-777. doi: 10.1111/1751-7915.14213
Coorens, M., Schneider, V. A. F., de Groot, A. M., van Dijk, A., Meijerink, M., Wells, J. M., et al. (2017). Cathelicidins inhibit Escherichia coli-induced TLR2 and TLR4 activation in a viability-dependent manner. J. Immunol 199, 1418-1428. doi: 10.4049/jimmunol.1602164
Cuperus, T., Coorens, M., van Dijk, A., Haagsman, H. P. (2013). Avian host defense peptides. Dev. Comp. Immunol. 41, 352–369. doi: 10.1016/j.dci.2013.04.019
Cuperus, T., van Dijk, A., Matthijs, M. G. R., Veldhuizen, E. J. A., Haagsman, H. P. (2016). Protective effect of in ovo treatment with the chicken cathelicidin analog D-CATH-2 against avian pathogenic E. coli. Sci. Rep 6, 26622. doi: 10.1038/srep26622
Dong, C., Zhang, R., Xu, L. (2022). Antimicrobial peptides: an overview of their structure, function and mechanism of action. Protein Pept. Lett 29, 641-650. doi: 10.2174/0929866529666220613102145
Dwivedi, M., Parmar, M. D., Mukherjee, D., Yadava, A., Yadav, H., Saini, N. P. (2023). Biochemistry, mechanistic intricacies, and therapeutic potential of Antimicrobial Peptides: an alternative to traditional Antibiotics. Curr. Medicinal Chem. doi: 10.2174/0109298673268458230926105224
Eisenberg, D., Weiss, M. R., Terwilliger, C. T. (1982). The helical hydrophobic moment: a measure of the amphiphilicity of a helix. Nature 299, 371-374. doi: 10.1038/299371a0
Findlay, B., Zhanel, G. G., Schweizer, F. (2010). Cationic amphiphiles, a new generation of antimicrobials inspired by the natural antimicrobial peptide scaffold. Antimicrobial Agents Chemother. 54, 4049-4058. doi: 10.1128/AAC.00530-10
Gao, J., Duan, X., Li, X., Cao, H., Wang, Y., Zheng, S. J. (2018). Emerging of a highly pathogenic and multi-drug resistant strain of Escherichia coli causing an outbreak of colibacillosis in chickens. Infection Genet. Evol. 65, 392-398. doi: 10.1016/j.meegid.2018.08.026
Getachew, K., Debebe, A., Birhanu, A. (2023). Isolation, identification, and molecular characterization of probiotic bacteria from locally selected Ethiopian free range chickens gastrointestinal tract. Poultry Sci. 103, 103311. doi: 10.1016/j.psj.2023.103311
Ghimpeţeanu, O. M., Pogurschi, E. N., Popa, D. C., Dragomir, N., Drăgotoiu, T., Mihai, O. D., et al. (2022). Antibiotic use in livestock and residues in food-A public health threat: A review. Foods 11, 1430. doi: 10.3390/foods11101430
Goitsuka, R., Chen, C.-L. H., Benyon, L., Asano, Y., Kitamura, D., Cooper, M. D. (2007). Chicken cathelicidin-B1, an antimicrobial guardian at the mucosal M cell gateway. Proc. Natl. Acad. Sci. U. S. A. 104, 15063-15068. doi: 10.1073/pnas.0707037104
Hancock, E. R., Scott, G. M. (2000). The role of antimicrobial peptides in animal defenses. Proc. Natl. Acad. Sci. U. S. A. 97, 8856-8861. doi: 10.1073/pnas.97.16.8856
Huang, F., Hong, Y., Mo, C., Huang, P., Liao, X., Yang, Y. (2022). Removal of antibiotic resistance genes during livestock wastewater treatment processes: Review and prospects. Front. Vet. Sci. 9, 1054316. doi: 10.3389/fvets.2022.1054316
Kumar, S. B., Arnipalli, S. R., Ziouzenkova, O. (2020). Antibiotics in food chain: the consequences for antibiotic resistance. Antibiotics (Basel) 9, 688. doi: 10.3390/antibiotics9100688
Mourtada, R., Herce, H. D., Yin, D. J., Moroco, J. A., Wales, T. E., Engen, J. R., et al. (2019). Design of stapled antimicrobial peptides that are stable, nontoxic and kill antibiotic-resistant bacteria in mice. Nat. Biotechnol. 37, 1186-1197. doi: 10.1038/s41587-019-0222-z
Mwangi, J., Hao, X., Lai, R., Zhang, Z.-Y. (2019). Antimicrobial peptides: new hope in the war against multidrug resistance. Zoological Res 40, 488-505. doi: 10.24272/j.issn.2095-8137.2019.062
Nicolas, M., Faurie, A., Girault, M., Lavillatte, S., Menanteau, P., Chaumeil, T., et al. (2023). In ovo administration of a phage cocktail partially prevents colibacillosis in chicks. Poultry Sci 102, 102967. doi: 10.1016/j.psj.2023.102967
Peng, C., Liu, Y., Shui, L., Zhao, Z., Mao, X., Liu, Z. (2022). Mechanisms of action of the antimicrobial peptide cecropin in the killing of Candida albicans. Life 12, 1581. doi: 10.3390/life12101581
Rima, M., Rima, M., Fajloun, Z., Sabatier, J.-M., Bechinger, B., Naas, T. (2021). Antimicrobial peptides: A potent alternative to antibiotics. Antibiotics 10, 1095. doi: 10.3390/antibiotics10091095
Roth, N., Käsbohrer, A., Mayrhofer, S., Zitz, U., Hofacre, C., Domig, K. J. (2019). The application of antibiotics in broiler production and the resulting antibiotic resistance in Escherichia coli: A global overview. Poult Sci. 98, 1791–1804. doi: 10.3382/ps/pey539
Rusu, A., Buta, E. L. (2021). The development of third-generation tetracycline antibiotics and new perspectives. Pharmaceutics 13, 2085. doi: 10.3390/pharmaceutics13122085
Sargeant, J. M., Bergevin, M. D., Churchill, K., Dawkins, K., Deb, B., Dunn, J., et al. (2019). The efficacy of antibiotics to control colibacillosis in broiler poultry: a systematic review. Anim. Health Res. Rev 20, 263-273. doi: 10.1017/S1466252319000264
Scheenstra, M. R., van den Belt, M., Tjeerdsma-van Bokhoven, J. L. M., Schneider, V. A. F., Ordonez, S. R., van Dijk, A., et al. (2019). Cathelicidins PMAP-36, LL-37 and CATH-2 are similar peptides with different modes of action. Sci. Rep. 9, 4780. doi: 10.1038/s41598-019-41246-6
Semple, F., Dorin, J. R. (2012). β-Defensins: multifunctional modulators of infection, inflammation and more? J. Innate Immun. 4, 337-348. doi: 10.1159/000336619
Sharma, P., Sharma, S., Joshi, S., Barman, P., Bhatt, A., Maan, M., et al. (2022). Design, characterization and structure–function analysis of novel antimicrobial peptides based on the N-terminal CATH-2 fragment. Sci. Rep. 12, 12058. doi: 10.1038/s41598-022-16303-2
Shengnan, L., Brim Stevy, O., Shih-Hsin, H., Qixing, Z., Fengxiang, L. (2023). Drinking water sources as hotspots of antibiotic-resistant bacteria (ARB) and antibiotic resistance genes (ARGs): Occurrence, spread, and mitigation strategies. J. Water Process Eng. 53, 103907. doi: 10.1016/j.jwpe.2023.103907
Shenrui, X., Peng, T., Qi, T., Tao, W., Yakun, D., Huiyang, F., et al. (2023). Enhancing the stability of antimicrobial peptides: From design strategies to applications. Chem. Eng. J. 475, 145923. doi: 10.1016/j.cej.2023.145923
Torres, M. D. T., Sothiselvam, S., Lu, T. K., de la Fuente-Nunez, C. (2019). Peptide design principles for antimicrobial applications. J. Mol. Biol. 431, 3547-3567. doi: 10.1016/j.jmb.2018.12.015
Treiber, F. M., Beranek-Knauer, H. (2021). Antimicrobial residues in food from animal origin-A review of the literature focusing on products collected in stores and markets worldwide. Antibiotics (Basel) 10, 534. doi: 10.3390/antibiotics10050534
Valdez-Miramontes, C. E., De Haro-Acosta, J., Aréchiga-Flores, C. F., Verdiguel-Fernández, L., Rivas-Santiago, B. (2021). Antimicrobial peptides in domestic animals and their applications in veterinary medicine. Peptides 142, 170576. doi: 10.1016/j.peptides.2021.170576
van Dijk, A., Molhoek, E. M., Veldhuizen, E. J. A., Bokhoven, J.L.M.T.-v., Wagendorp, E., Bikker, F., et al. (2009). Identification of chicken cathelicidin-2 core elements involved in antibacterial and immunomodulatory activities. Mol. Immunol. 46, 2465-2473. doi: 10.1016/j.molimm.2009.05.019
van Harten, R. M., Tjeerdsma-van Bokhoven, J. L. M., de Greeff, A., Balhuizen, M. D., van Dijk, A., Veldhuizen, E. J. A., et al. (2021). d-enantiomers of CATH-2 enhance the response of macrophages against Streptococcus suis serotype 2. J. Advanced Res. 6, 63.
van Harten, R., van Woudenbergh, E., van Dijk, A., Haagsman, H. (2018). Cathelicidins: immunomodulatory antimicrobials. Vaccines. doi: 10.3390/vaccines6030063
Voulgaris, G. L., Voulgari, M. L., Falagas, M. E. (2019). Developments on antibiotics for multidrug resistant bacterial Gram-negative infections. Expert Rev. Anti Infect. Ther. 17, 387–401. doi: 10.1080/14787210.2019.1610392
Walker, G. K., Suyemoto, M. M., Gall, S., Chen, L., Thakur, S., Borst, L. B. (2020). The role of Enterococcus faecalis during co-infection with avian pathogenic Escherichia coli in avian colibacillosis. Avian Pathol. 49, 589-599. doi: 10.1080/03079457.2020.1796926
Xiao, Y., Cai, Y., Bommineni, Y. R., Fernando, S. C., Prakash, O., Gilliland, S. E., et al. (2006). Identification and functional characterization of three chicken cathelicidins with potent antimicrobial activity. J. Biol. Chem. 281, 2858-2867. doi: 10.1074/jbc.M507180200
Xiao, Y., Herrera, A. I., Bommineni, Y. R., Soulages, J. L., Prakash, O., Zhang, G. (2009). The central kink region of fowlicidin-2, an alpha-helical host defense peptide, is critically involved in bacterial killing and endotoxin neutralization. J. Innate Immun. 1, 268-280. doi: 10.1159/000174822
Xuan, J., Feng, W., Wang, J., Wang, R., Zhang, B., Bo, L., et al. (2023). Antimicrobial peptides for combating drug-resistant bacterial infections. Drug Resistance Updates 68, 100954. doi: 10.1016/j.drup.2023.100954
Yong Fang, Z., Shi Xian, C., Ke Jing, M., Xin Yu, Z., Ming Yi, J., Chang Jer, W., et al. (2023). de Novo-designed antimicrobial peptides with broad-spectrum antimicrobial potency and rapid wound disinfection. Colloids Surfaces A: Physicochem. Eng. Aspects 667, 131355.
Zhang, G., Sunkara, L. (2014). Avian antimicrobial host defense peptides: from biology to therapeutic applications. Pharmaceuticals 7, 220-247. doi: 10.3390/ph7030220
Zhao, X., Wang, L., Zhu, C., Xia, X., Zhang, S., Wang, Y., et al. (2021). The antimicrobial peptide MPX protects against Enterohemorrhagic Escherichia coli O157:H7 infection, inhibits inflammation, and enhances the intestinal epithelial barrier. Front. Microbiol 12, 644887. doi: 10.3389/fmicb.2021.644887
Keywords: Avian colibacillosis, MDR, E. coli, CATH-2, C2-2
Citation: Hao S, Shi W, Chen L, Kong T, Wang B, Chen S and Guo X (2024) CATH-2-derived antimicrobial peptide inhibits multidrug-resistant Escherichia coli infection in chickens. Front. Cell. Infect. Microbiol. 14:1390934. doi: 10.3389/fcimb.2024.1390934
Received: 24 February 2024; Accepted: 18 April 2024;
Published: 15 May 2024.
Edited by:
Rima El-Herte, Creighton University, United StatesReviewed by:
Joel E. Lopez-Meza, Michoacana University of San Nicolás de Hidalgo, MexicoCopyright © 2024 Hao, Shi, Chen, Kong, Wang, Chen and Guo. This is an open-access article distributed under the terms of the Creative Commons Attribution License (CC BY). The use, distribution or reproduction in other forums is permitted, provided the original author(s) and the copyright owner(s) are credited and that the original publication in this journal is cited, in accordance with accepted academic practice. No use, distribution or reproduction is permitted which does not comply with these terms.
*Correspondence: Shuming Chen, MTM4MzQ4MzQxODNAMTYzLmNvbQ==; Xiaomin Guo, Z3VveGlhb21pbkBzeGF1LmVkdS5jbg==
†These authors have contributed equally to this work
Disclaimer: All claims expressed in this article are solely those of the authors and do not necessarily represent those of their affiliated organizations, or those of the publisher, the editors and the reviewers. Any product that may be evaluated in this article or claim that may be made by its manufacturer is not guaranteed or endorsed by the publisher.
Research integrity at Frontiers
Learn more about the work of our research integrity team to safeguard the quality of each article we publish.